- 1Laboratory of Molecular Biology, Department of Synthetic Chemistry and Biological Chemistry, Graduate School of Engineering, Kyoto University, Kyoto, Japan
- 2The Hakubi Center for Advanced Research, Kyoto University, Kyoto, Japan
Psychiatric disorders are caused by complex and diverse factors, and numerous mechanisms have been proposed for the pathogenesis of these disorders. Accumulating evidence suggests that oxidative stress is one of the general factors involved in the pathogenesis/pathophysiology of major psychiatric disorders, including bipolar disorder, depression, anxiety disorder, and schizophrenia. Indeed, some clinical trials have shown improvement of the symptoms of these disorders by antioxidant supplementation. However, the molecular basis for the relationship between oxidative stress and the pathogenesis of psychiatric disorders remains largely unknown. In general, Ca2+ channels play central roles in neuronal functions, including neuronal excitability, neurotransmitter release, synaptic plasticity, and gene regulation, and genes that encode Ca2+ channels have been found to be associated with psychiatric disorders. Notably, a class of Ca2+-permeable transient receptor potential (TRP) cation channels is activated by changes in cellular redox status, whereby these TRP channels can link oxidative stress to Ca2+ signals. Given the unique characteristic of redox-sensitive TRP channels, these channels could be a target for delineating the pathogenesis or pathophysiology of psychiatric disorders. In this review, we summarize the outcomes of clinical trials for antioxidant treatment in patients with psychiatric disorders and the current insights into the physiological/pathological significance of redox-sensitive TRP channels in the light of neural functions, including behavioral phenotypes, and discuss the potential role of TRP channels in the pathogenesis of psychiatric disorders. Investigation of redox-sensitive TRP channels may lead to the development of novel therapeutic strategies for the treatment of psychiatric disorders.
Introduction
Psychiatric disorders, which are chronic, recurrent, and devastating disorders, are one of the main causes of disability worldwide, with the current understanding of psychiatric disorders remaining limited due to the complex and diverse nature of these disorders. Based on a 2017 survey, the number of patients with psychiatric disorders is extremely high worldwide (284 million cases of anxiety disorders; 264 million cases of depression; 46 million cases of bipolar disorder; 20 million cases of schizophrenia) (Ritchie and Roser, 2018); therefore, therapeutic strategies for the treatment of psychiatric disorders are urgently required.
Accumulating evidence suggests that oxidative stress is one of the general factors involved in the pathogenesis/pathophysiology of psychiatric disorders (Ng et al., 2008), with antioxidant levels seeming to correlate with the degree of severity of psychiatric disorders (Zhang et al., 2003; Machado-Vieira et al., 2007; Sarandol et al., 2007), and antioxidant treatment has been shown to improve psychiatric symptoms in some clinical trials (Dakhale et al., 2005; Sivrioglu et al., 2007; Ellegaard et al., 2019). Moreover, markers of oxidative stress, including lipid peroxidation products and oxidized DNA, have been shown to be elevated in the blood of patients with psychiatric disorders. While the molecular mechanisms by which oxidative stress induces psychiatric disorders are still largely unknown, the lines of evidence strongly suggest that oxidative stress is associated with the physiology/pathology of psychiatric disorders.
Ca2+ channels mediate a number of neuronal functions, including neuronal excitability, neurotransmitter release, synapticplasticity, and gene regulation. Many lines of evidence from human studies have indicated a critical contribution of voltage-dependent Ca2+ channels (VDCC) to the pathogenesis/pathophysiology of psychiatric disorders (Sklar et al., 2002, 2011; Ferreira et al., 2008; Ripke et al., 2011, 2014). However, since VDCCs control multiple critical physiological functions in the central nervous system (CNS), targeting VDCCs could cause complicated and uncontrollable results. Thus, targeting the “cart” that modulates Ca2+ signaling upon oxidative stress rather than the “horse” that governs the CNS system may be a promising approach and could reduce unwanted side effects.
Among the Ca2+-permeable cation channels, transient receptor potential (TRP) channels have attracted attention as unique sensors of a wide variety of stresses, including oxidative stress (Mori et al., 2016). Given that redox-sensitive TRP channels induce Ca2+ influx upon oxidative stress, these channels could be mediators that link oxidative stress to dysregulated Ca2+ signaling in the pathogenesis/pathophysiology of psychiatric disorders.
In the first part of this review, we introduce the literature that examines oxidative stress in psychiatric disorders. In the second part, we review the significance of Ca2+ signaling in psychiatric disorders and provide current insights into the roles of redox-sensitive TRP channels in the functioning of neurons and glia as well as the development of neuronal connectivity and the function of the higher brain.
Oxidative Stress in Psychiatric Disorders
Cellular redox status is determined by the balance between the levels of intracellular antioxidants and redox reactive species, including reactive oxygen species (ROS) and other electrophilic molecules, which can cause oxidative damage to membrane lipids, proteins, and DNA (Figure 1A). While a decrease in antioxidant enzymes shifts the cellular redox status to oxidative conditions, intriguingly, the expression of antioxidant enzymes is induced upon oxidative stress via oxidant defense transcription factors, such as NRF2 and NF-κB; therefore, both enhanced and suppressed antioxidant expression can be associated with oxidative stress (Figure 1B).
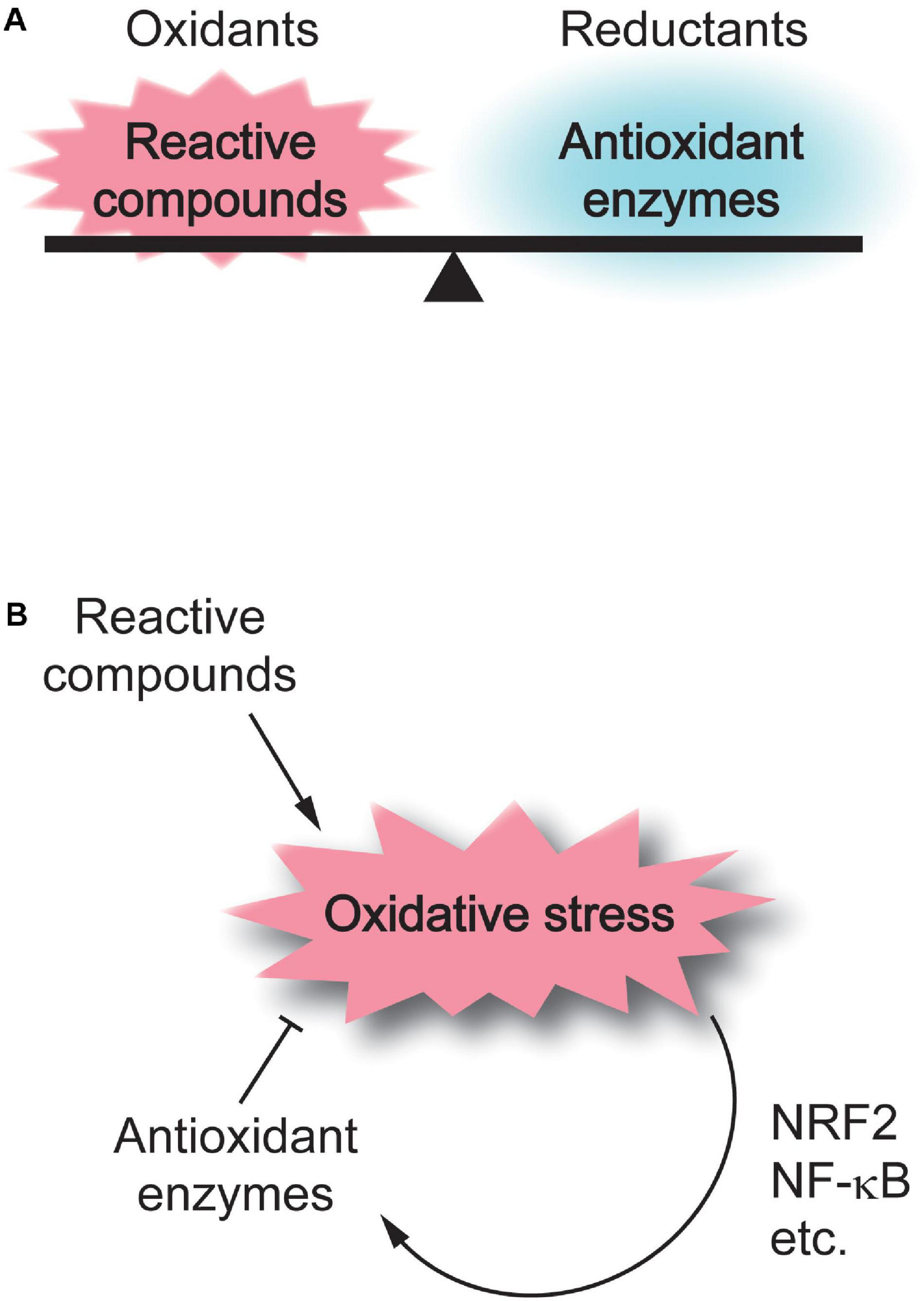
Figure 1. Regulation of cellular redox homeostasis. (A) Cellular redox status is determined by the balance between the levels of intracellular reactive compounds and antioxidant enzymes. (B) Reactive compounds shift the cellular redox status to oxidative conditions, while antioxidant enzymes neutralize oxidative stress. The expression of antioxidant enzymes is induced upon oxidative stress via oxidant defense transcription factors, such as NRF2 and NF-κB.
It is highly controversial whether the antioxidant system is upregulated or downregulated in psychiatric disorders. In bipolar disorder, the activities of superoxide dismutases (SODs) and catalase (CAT) have been found to be decreased in the blood (Ranjekar et al., 2003), while the activity of glutathione peroxidases (GPXs) has been shown to be comparable between bipolar patients and healthy individuals (Kuloglu et al., 2002c; Ranjekar et al., 2003).
In contrast, several studies have demonstrated increased SOD activities in bipolar disorder (Abdalla et al., 1986; Kuloglu et al., 2002c). In major depression and anxiety disorders, such as obsessive-compulsive disorder, panic disorder, and social phobia, the activity of SODs alone or SODs and GPXs has been shown to be increased (Kuloglu et al., 2002a, b; Khanzode et al., 2003; Atmaca et al., 2004; Sarandol et al., 2007). In schizophrenia, higher activities of SODs (Abdalla et al., 1986; Kuloglu et al., 2002c) and GPXs have been reported in the blood of patients (Kuloglu et al., 2002c), while several other studies have reported that the activities of SODs, CAT, and GPXs are lower in schizophrenic patients than in healthy controls (Ranjekar et al., 2003; Ben Othmen et al., 2008).
Some studies have reported a correlation between antioxidant activities in the blood and the degree of severity of psychiatric disorders. In a finding which suggests that antioxidant level is an indicator of disease severity and that lithium, a primary treatment for bipolar disorder, directly or indirectly normalizes redox status, one study that compared healthy controls with either unmedicated or lithium-treated patients in manic episodes of bipolar disorder indicates that, while SOD activity was significantly higher in manic patients compared with controls, it was indistinguishable between the lithium-treated group and the control group (Machado-Vieira et al., 2007). In patients with major depressive disorder, a significant positive correlation was found between the severity of the disease and SOD activity (Sarandol et al., 2007). Interestingly, serotonin reuptake inhibitors (SSRIs), which are antidepressant drugs, have been shown to decrease SOD activity (Bilici et al., 2001; Khanzode et al., 2003); however, SSRI treatment has also been reported to increase SOD activity in patients with major depressive disorder (Herken et al., 2007). In schizophrenic patients, SOD activity is significantly increased in the serum compared to control subjects and is decreased after treatment with atypical antipsychotics, such as clozapine, risperidone, olanzapine, quetiapine, and ziprasidone, in schizophrenic patients (Dakhale et al., 2004). It has also been reported that treatment with risperidone, an atypical antipsychotic, significantly decreased the blood SOD levels in schizophrenic patients and that decreased SOD levels may correlate with an improvement in symptoms (Zhang et al., 2003).
In contrast to the controversy on the effects of antioxidant activityon psychiatric disorders, overall, markers of oxidative stress, such as lipid peroxidation and oxidized DNA, have been shown to be elevated in patients with psychiatric disorders. An increase in one lipid peroxidation product, malondialdehyde (MDA), was reported in both bipolar disorder and schizophrenia (Kuloglu et al., 2002c). The level of thiobarbituric acid-reactive substances, another marker of lipid peroxidation, has also been reported to be increased in schizophrenia (Herken et al., 2001; Akyol et al., 2002). In major depression, a number of factors that maintain or disturb redox homeostasis are altered: depletion of ω-3 fatty acids, which suppress lipid peroxidation (Peet et al., 1998), elevation of MDA (Bilici et al., 2001; Khanzode et al., 2003; Sarandol et al., 2007), and increases in 8-hydroxy-2′-deoxyguanosine, a marker of oxidized DNA damage (Forlenza and Miller, 2006). In anxiety disorders, elevated MDA levels have been reported in obsessive-compulsive disorder (Kuloglu et al., 2002a; Ersan et al., 2006), panic disorder (Kuloglu et al., 2002b), and social phobia (Atmaca et al., 2004). These results provide evidence that oxidative stress is induced in psychiatric disorders and suggest both that the antioxidant system is upregulated through oxidant defense mechanisms and that decreased antioxidant activities lead to enhanced oxidative stress in some types of psychiatric disorders (Figure 1).
The potential significance of oxidative stress in psychiatricdisorders has been demonstrated by some clinical trials that reportedan improvement in symptoms through antioxidant treatment(Table 1). In patients with bipolar disorder, a randomized, double-blind, multicenter, placebo-controlled study of individuals (n = 75) showed that 24-week treatment with N-acetyl cysteine (NAC) significantly improved depressive symptoms (Berk et al., 2008), while a three-arm, 16-week, double-blind, randomized, placebo-controlled NAC treatment trial (n = 181) with depressive symptoms in bipolar disorder provided overall negative results, with no significant differences between groups detected at the primary outcome but some positive secondary signals were detected (Berk et al., 2019), suggesting that longer-term supplementation of NAC is required for the amelioration. In patients with major depressive disorder, a double-blind, randomized, placebo-controlled, clinical trial was performed with NAC as an adjunctive treatment in 269 participants, and the results exhibited limited but significant effects of adjunctive NAC treatment in reducing depressive symptoms in patients with major depressive disorder (Berk et al., 2014). Notably, meta-analysis was carried out to aggregate the data on double-blind, randomized, placebo-controlled trials evaluating the effect of NAC treatment on depressive symptoms in a total of 574 participants, of whom 291 were randomized to receive NAC and 283 received a placebo, regardless of the main psychiatric conditions. The results demonstrated that treatment with NAC significantly improved depressive symptoms in patients with bipolar and major depressive disorders and in individuals with trichotillomania or heavy smoking (Fernandes et al., 2016). In patients with schizophrenia, a 12-week, double-blind, randomized, placebo-controlled, clinical trial has recently been performed to assess the effectiveness of NAC as an adjunctive treatment with conventional antipsychotic medications in 84 patients (Sepehrmanesh et al., 2018). This clinical trial demonstrated that NAC-treated patients showed significantly improved cognitive functions (Sepehrmanesh et al., 2018). In addition to NAC supplementation, co-treatment of antipsychotic drugs with both ω-3 fatty acids and vitamins E and C for 4 months improved psychotic symptoms in schizophrenic patients (Arvindakshan et al., 2003). There results suggest that oxidative stress is involved in the pathophysiology of psychiatric disorders and that antioxidant supplementation may have a suppressive effect on symptoms.
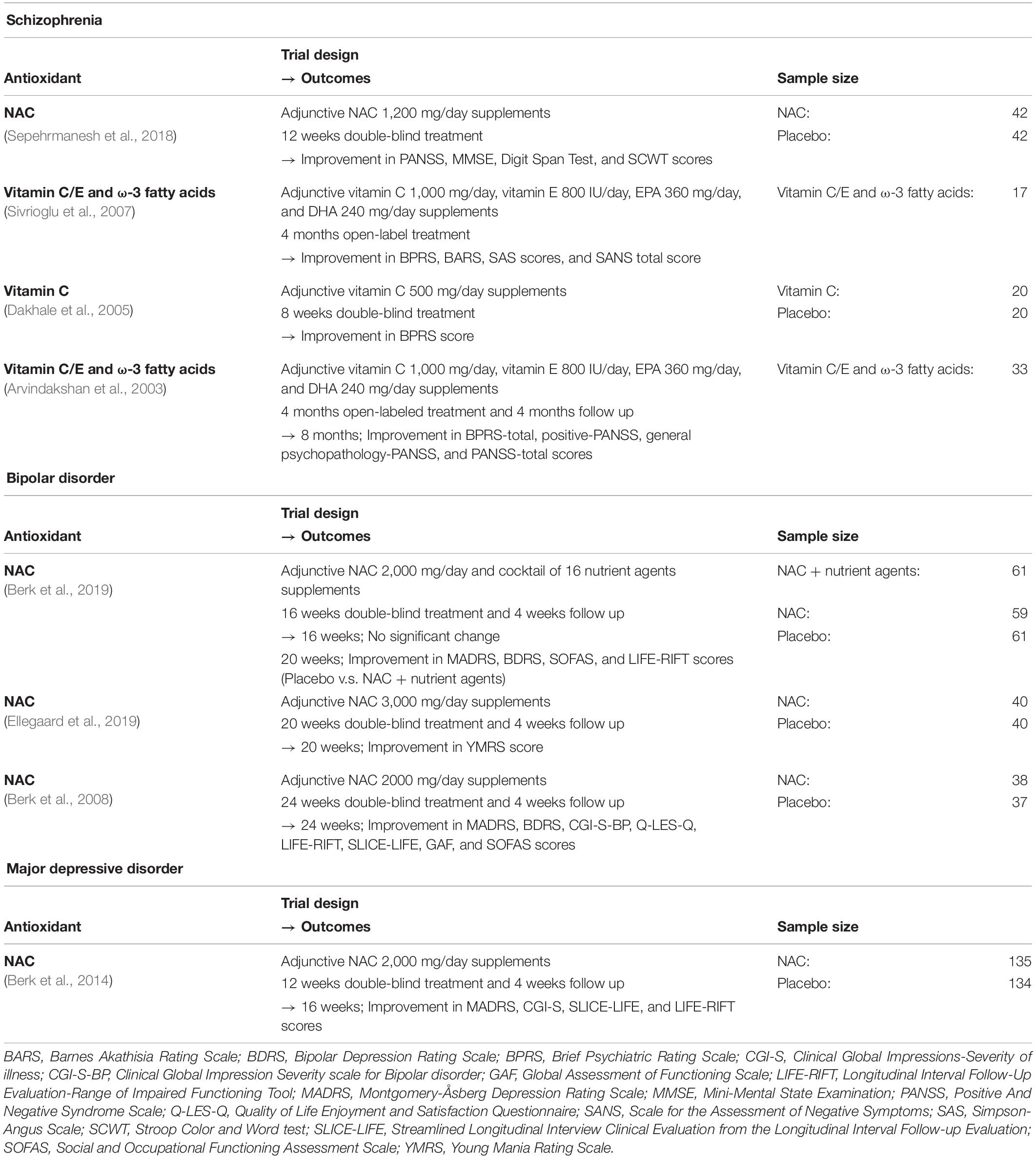
Table 1. Clinical trials for antioxidant treatment in patients with schizophrenia, bipolar disorder, and major depressive disorder.
Ca2+ Signaling in Psychiatric Disorders
In the CNS, Ca2+ signaling is pivotal for numerous cellular events, including neuronal excitability, neurotransmitter release, synaptic plasticity, and Ca2+-induced gene regulation (Catterall, 2011; Sawamura et al., 2017). Due to the fundamental role of Ca2+ signaling in the CNS, Ca2+ channels are thought to be a prime target for the pathogenesis of psychiatric disorders. Indeed, genes encoding Ca2+ channels have been shown to be associated with psychiatric disorders (McQuillin et al., 2006; Ripke et al., 2014; Griesi-Oliveira et al., 2015).
Among the Ca2+ channels, CACNA1C-encoded CaV1.2, an L-type VDCC, is strongly associated with psychiatric disorders (Sklar et al., 2002, 2011; Ferreira et al., 2008; Ripke et al., 2011, 2014). In humans, a risk-associated single nucleotide polymorphism (rs1006737) in CACNA1C predicts both increased hippocampal activity during emotional processing and higher prefrontal activity during executive cognition (Bigos et al., 2010). In rodents, conditional deletion of Cacna1c in the hippocampus and cortex results in severe impairment of hippocampus-dependent spatial memory based on the Morris water maze test (Moosmang et al., 2005; White et al., 2008). Acute pharmacological blockade of CaV1.2, but not chronic genetic inactivation, impairs the acquisition of fear learning (Langwieser et al., 2010), while anterior cingulate cortex-limited deletion of Cacna1c in mice impairs observational fear learning (Jeon et al., 2010). Constitutive Cacna1c heterozygous knockout (KO) mice, forebrain-specific conditional Cacna1c KO mice, and prefrontal cortex-specific Cacna1c knockdown mice show increased anxiety-like behavior in the elevated-plus maze test (Lee et al., 2012). The behavioral phenotypes in mice with genetic or pharmacological inhibition of CaV1.2 are summarized in the previous literature (Nakao et al., 2015).
Clinical studies with CaV1.2 blockers for psychiatric disorders have been summarized in a previous review paper (Kabir et al., 2017). While some clinical trials using CaV1.2 blockers have demonstrated improved symptoms of psychiatric disorders, the effect remains controversial (Kabir et al., 2017). Notably, since CaV1.2 controls multiple critical physiological functions in various organs and tissues, including the vascular system, the sinoatrial node, cardiomyocytes, pancreatic islets, adrenal medulla, and intestinal smooth muscle (Zamponi et al., 2015), the use of CaV1.2 blockers could cause highly complicated and uncontrollable results.
Redox-Sensitive TRP Channels
Among the Ca2+-permeable channels, a class of TRP channels is activated by oxidative stress (so-called redox-sensitive TRP channels). Given the critical role of oxidative stress and aberrant Ca2+ signaling in psychiatry disorders, it is possible that redox-sensitive TRP channels serve as a cue for understanding the molecular pathogenesis of these disorders.
The trp was originally identified through the genetic studies of the Drosophila phototransduction mutant (Montell and Rubin, 1989). The term “TRP” is derived from “transient receptor potential” as the trp mutant photoreceptors fail to generate the Ca2+-dependent sustained phase of receptor potential and therefore fail to induce subsequent Ca2+-dependent adaptation to light in Drosophila. In mammals, 28 TRP homologs have been identified since the cloning of the Drosophila trp gene (Montell and Rubin, 1989). They are divided into six subfamilies: TRPC (canonical), TRPV (vanilloid), TRPM (melastatin), TRPP (polycystin), TRPML (mucolipin), and TRPA (ankyrin) groups, according to the amino acid homology (Clapham, 2003; Nilius et al., 2007). TRP channels are putative six-transmembrane polypeptide subunits and assemble as tetramers to form a variety of Ca2+-permeable cation channels. Because of their distinct activation mechanisms and biophysical properties, TRP channels are highly suited to function in sensory receptor cells, either as molecular sensors for environmental or endogenous stimuli or as modulators of signal transduction cascades downstream of metabotropic receptors. Indeed, TRP channels play crucial roles in many types of senses, including touch, taste, and smell in mammals (Clapham, 2003; Voets et al., 2005; Moran et al., 2011). Thus, in marked contrast to VDCCs and fast ligand-gated Ca2+-permeable channels that participate in specialized cellular functions, TRP channels play divergent roles in cell physiology and pathophysiology.
Among TRP channels, one particular group of TRP channels, including TRPM2, TRPC5, TRPV1–V4, and TRPA1 channels, function as exquisite sensors of redox status. The redox-sensitive TRP channels induce Ca2+ entry into the cells in response to cellular redox perturbation and can convert oxidative stress information into Ca2+ signals. Details of redox-sensitive TRP channels have been summarized in several review papers from our group (Takahashi and Mori, 2011; Takahashi et al., 2011a; Kozai et al., 2013; Sakaguchi and Mori, 2020).
TRPM2 channel, the first redox-sensitive TRP channel to be identified, is activated by H2O2 through the production of nicotinamide adenine dinucleotide and its metabolites, ADP-ribose and cyclic ADP-ribose (Hara et al., 2002; Perraud et al., 2005). A later study proposed that oxidation of the methionine located in the ADP-ribose binding region of TRPM2 sensitizes the channel activities (Kashio et al., 2012). H2O2-activated Ca2+ or cation influx through TRPM2 induces cell death (Hara et al., 2002) and insulin secretion in pancreatic β-cells (Togashi et al., 2006; Uchida et al., 2011). Furthermore, studies using Trpm2 KO mice have shown that TRPM2 widely contributes to the innate immune system in monocytes, macrophages, neutrophils, and natural killer cells (Yamamoto et al., 2008; Knowles et al., 2011; Di et al., 2012; Hiroi et al., 2013; Rah et al., 2015).
In addition to the indirect redox-sensing mechanism via TRPM2, direct redox-sensing mechanisms involving cysteine (Cys) modification have emerged as mechanisms that underly the activation of various TRP channels (Yoshida et al., 2006; Takahashi and Mori, 2011; Takahashi et al., 2011b). TRPC5 channel, for example, is activated by nitric oxide (NO), reactive disulfides, and H2O2 via the oxidative modification of Cys residues (Cys553 and Cys558) located on the N-terminal side of the pore-forming region between S5 and S6 transmembrane helices in mouse TRPC5 (Yoshida et al., 2006). Interestingly, TRPC5 is also activated by the reducing agent dithiothreitol and extracellular-reduced thioredoxin (Xu et al., 2008). Both thermosensor channels (TRPV1, TRPV3, and TRPV4) and the closest relatives of TRPC5 (TRPC1 and TRPC4) have Cys residues corresponding to Cys553 and Cys558 on the TRPC5 protein and are activated by NO, reactive disulfides, and H2O2 (Yoshida et al., 2006). The mustard oil or wasabi sensor TRPA1 channel, which is predominantly expressed in sensory and vagal neurons, is also activated via modification of Cys residues located in the N-terminal ankyrin repeat domain (Hinman et al., 2006; Macpherson et al., 2007; Takahashi et al., 2008) and has the highest oxidation sensitivity among all TRP channel family members (Takahashi et al., 2011b).
Notably, all the redox-sensitive TRP channels are expressed to some extent in the CNS and affect neuronal and glial functions. Given this fact, together with the evidence that both Ca2+ signals and oxidative stress are critical mediators in psychiatric disorders, it is possible that redox-sensitive TRP channels could be a target for delineating the pathogenesis or pathophysiology of psychiatric disorders.
Neuronal and Glial Functions of Redox-Sensitive TRP Channels
In neuronal circuits, excitation-inhibition balance is considered a framework for understanding the mechanisms of psychiatric disorders (Sohal and Rubenstein, 2019). TRPM2 channel is abundantly expressed in the brain (Hara et al., 2002; Kraft et al., 2004; Fonfria et al., 2006) and H2O2-induced Ca2+ influx via TRPM2 in neurons was initially identified to induce cell death (Kaneko et al., 2006). Later studies have suggested several important roles for TRPM2 in the physiology of the CNS. TRPM2 activation by ADPR has been suggested as a regulator of VDCC and NMDAR via a rise in intracellular Ca2+ concentration ([Ca2+]i) in cultured hippocampal pyramidal neurons (Olah et al., 2009). In CA3-CA1 synapses, the loss of TRPM2 selectively impairs NMDAR-dependent long-term depression (LTD), while it does not affect long-term potentiation (LTP) (Xie et al., 2011). The impaired LTD in Trpm2 KO mice is rescued by dopamine D2 receptor stimulation through the reduction of phosphorylated GSK-3β, suggesting that TRPM2 plays a key role in hippocampal synaptic plasticity via the GSK-3β signaling pathway. In guinea pig midbrain slices, TRPM2 has been shown to be required for NMDA-induced burst firing and to contribute to H2O2-dependent modulation of substantia nigra pars reticulata (SNr) GABAergic neurons (Lee et al., 2013). Given that an increase in burst firing in SNr GABAergic neurons is observed in Parkinson’s disease and that oxidative stress is linked to Parkinson’s disease, TRPM2 could be associated with the pathophysiology of Parkinson’s disease.
Quantitative post-mortem investigations have convincingly demonstrated abnormalities in the glial cells of patients with psychiatric disorders (Cotter et al., 2001). In addition to neurons and immune cells, TRPM2 has been shown to be functionally expressed in glial cells, such as the microglia and astrocytes (Kraft et al., 2004; Kaneko et al., 2006; Bond and Greenfield, 2007). Oxidative stress induced by the inhibition of intracellular GSH biosynthesis with D,L-buthionine-S,R-sulfoximine (BSO) elicits Ca2+ influx potentially via TRPM2 in astrocytes isolated from humans (Lee et al., 2010). In rat cultured astrocytes, tert-butyl hydroperoxide-induced oxidative stress upregulates TRPM2 mRNA within 1 h – the transcripts were peaked at 4 h and were still apparent at 24 h post-stress (Bond and Greenfield, 2007), suggesting that glial TRPM2 expression is enhanced upon oxidative stress in disease conditions, including psychiatric disorders, and mediates the pathophysiology of disease.
TRPC5 is predominantly expressed in the brain, where it can formheterotetrameric complexes with TRPC1 and TRPC4 channel subunits. Recently, TRPC1, TRPC4, and TRPC5 have been demonstrated to assemble into heteromultimers in mouse brains, but not with other TRP family members, based on quantitative high-resolution mass spectrometry (Bröker-Lai et al., 2017). Brain slices prepared from Trpc5 KO and Trpc4 KO mice exhibit significant reductions in the synaptic responses that are mediated by the activation of Gq/11 protein-coupled receptors (e.g., group I metabotropic glutamate receptors and cholecystokinin [CCK] 2 receptors) in the amygdala (Riccio et al., 2009, 2014). As a consequence, deficiency of Trpc5 or Trpc4 induces anxiolytic behavior in mice (Riccio et al., 2009, 2014) (see “Redox-Sensitive TRP Channels in Higher Brain Functions” section). In hippocampal neurons from Trpc1/Trpc4/Trpc5 triple KO mice, action potential-triggered excitatory postsynaptic currents (EPSCs) are significantly reduced, whereas the frequency, amplitude, and kinetics of quantal miniature EPSC signaling remains unchanged (Bröker-Lai et al., 2017). Consistent with this observation, evoked postsynaptic responses in hippocampal slice recordings and transient potentiation after tetanic stimulation have been reported to be decreased in triple KO mice (Bröker-Lai et al., 2017). In layer III pyramidal neurons in the entorhinal cortex, enhancement of neuronal excitability induced by CCK, one of the most abundant neuropeptides in the brain, is significantly inhibited by intracellular application of the antibody to TRPC5, suggesting the involvement of TRPC5 channels in neuropeptide-mediated neuronal excitability (Wang et al., 2011). TRPC5 has been also demonstrated to mediate muscarinic receptor-induced slowing after depolarization, which is observed in the pyramidal cells of the cerebral cortex (Yan et al., 2009). Interestingly, TRPC1, TRPC4, and TRPC5 channels are activated by leptin, a hormone that plays a key role in appetite, overeating, and obesity, via the Jak2-PI3 kinase-PLCγ1 pathway in hypothalamic proopiomelanocortin neurons (Qiu et al., 2010). Taken together, these lines of evidence suggest that TRPC1, TRPC4, and TRPC5 channels are involved in various physiological processes, including memory and appetite, by regulating neuronal functions.
TRPC1, TRPC4, and TRPC5 channels have also been shown to be expressed in astrocytes (Song et al., 2005; Malarkey et al., 2008). In the results suggesting that the TRPC1, TRPC4, and TRPC5 channels are functionally expressed in astrocytes and also modulate neuronal circuits, high-resolution imaging revealed that store-operated Ca2+ entry (SOCE) mediated by TRPC1 is linked not only functionally but also structurally to the ER Ca2+ stores in mouse astrocytes (Golovina, 2005). TRPC1-mediated Ca2+ entry in response to mechanical and pharmacological stimulation has been shown to contribute to glutamate release from rat astrocytes (Malarkey et al., 2008).
TRPV1 is broadly expressed in the brain regions, including theolfactory nuclei, cerebral cortex, dentate gyrus, thalamus, hypothalamus, periaqueductal gray, superior colliculus, locus coeruleus, and cerebellar cortex (Roberts et al., 2004). TRPV1 is involved in various forms of synaptic plasticity, which have been well-summarized in previous review papers (Kauer and Gibson, 2009; Ramírez-Barrantes et al., 2016; Sawamura et al., 2017). In the hippocampus, TRPV1 mediates LTD at excitatory synapses onto CA1 interneurons (Gibson et al., 2008). The excitatory synapses of the hippocampal CA1 interneurons are depressed by TRPV1 agonist capsaicin and the potent endogenous TRPV1 activator 12-(S)-HPETE (Gibson et al., 2008). TRPV1 activation by capsaicin or by endocannabinoid anandamide depressed somatic but not dendritic inhibitory inputs onto dentate granule cells, suggesting that TRPV1 modulates GABAergic synaptic transmission in a compartment-specific manner (Chávez et al., 2014). Interestingly, acute inescapable stress on an elevated platform suppressed LTP and facilitated LTD at the hippocampal CA3-CA1 synapse, but the TRPV1 agonist capsaicin effectively prevented these effects, suggesting that TRPV1 contributes to stress-induced impairment of spatial memory (Li et al., 2008) (see “Redox-Sensitive TRP Channels in Higher Brain Functions” section).
TRPA1, the channel that has the highest oxidation sensitivity among the TRP channels, is expressed in the brain (Stokes et al., 2006), including in the hippocampus (Lee et al., 2016), the dentate gyrus (Koch et al., 2011), the supraoptic nucleus (Yokoyama et al., 2011), the nucleus tractus solitarii (Sun et al., 2009), and the somatosensory cortex (Kheradpezhouh et al., 2017). TRPA1 is also expressed in astrocytes and regulates basal [Ca2+]i (Shigetomi et al., 2012, 2013; Takizawa et al., 2018). TRPA1 inhibition, which decreases resting [Ca2+]i in astrocytes, impairs interneuron inhibitory synapse efficacy by reducing GAT-3-mediated GABA transport, resulting in the elevation of extracellular GABA (Shigetomi et al., 2012). Furthermore, astrocyte TRPA1 channels are required for constitutive D-serine release into the extracellular space, which contributes to NMDA receptor-dependent LTP in CA3-CA1 synapses (Shigetomi et al., 2013). In rat astrocyte cell line C6 cells and primary cultured astrocytes, a TRPA1-mediated spontaneous rise of [Ca2+]i modulated the spontaneous release of peptide hormones from astrocytes (Takizawa et al., 2018). Recently, our group reported that TRPA1 acts as an acute hypoxia sensor in brainstem astrocytes through O2-dependent protein translocation and triggers ATP release from astrocytes, which potentiates respiratory center activity (Uchiyama et al., 2020). Interestingly, vascular endothelial cell-specific Trpa1 KO exacerbates cerebral infarcts following permanent middle cerebral artery occlusion in mice (Pires and Earley, 2018), suggesting that TRPA1 in cerebral artery endothelial cells is activated by oxidative stress under ischemia and protects from cerebral ischemia through the secretion of vasodilators, including NO. Taken together, TRPA1 is expressed in diverse cell types within the brain, including glial and endothelial cells, and promotes the secretion of factors that modulate neuronal circuits and vascular tonus upon oxidative stress in disease conditions.
Redox-Sensitive TRP Channels in the Establishment of Neuronal Connectivity
Abnormalities in neurites are considered to be involved in the pathogenesis of psychiatric disorders because they can cause impaired neuronal connectivity (Penzes et al., 2011). TRPC1 positively regulates netrin-1-induced growth-cone turning in cultured Xenopus spinal neurons (Wang and Poo, 2005; Shim et al., 2009), while it is not involved in brain-derived neurotrophic factor (BDNF)-induced growth-cone turning in cultured rat cerebellar granule cells (Li et al., 2005). Both TRPC1 and TRPC3 channels are required for leptin-sensitive current and leptin-induced spine formation in cultured hippocampal neurons (Dhar et al., 2014). Interestingly, SOCE mediated by TRPC1 is required for the proliferation of adult hippocampal neural progenitor cells (Li et al., 2012). Activation of TRPC4 induces inhibition and arborization of neurite growth in cultured hippocampal neurons (Jeon et al., 2013). TRPC5 that interacts with the growth-cone-enriched protein stathmin 2 has been shown to be a negative regulator of neurite extension and growth-cone morphology (Greka et al., 2003). In semaphorin 3A-induced neuronal growth-cone collapse, calpain has been demonstrated to cleave and activate TRPC5 channels, suggesting that TRPC5 acts downstream of the semaphorin signaling that causes changes in neuronal growth-cone morphology (Kaczmarek et al., 2012). Neurotrophin-3-induced Ca2+ influx via TRPC5 inhibits neuronal dendritic growth through activation of Ca2+/calmodulin kinase (CaMK) IIα (He et al., 2012). TRPC5 channels have also been shown to induce the activation of Ca2+/calmodulin kinase kinase (CaMKK) and the γ-isoform of CaMKI (CaMKIγ), followed by the promotion of axon formation in cultured hippocampal neurons (Davare et al., 2009). In the cerebellar cortex, Trpc5 deficiency causes the formation of long and highly branched granule neuron dendrites with impaired dendritic claw differentiation, resulting in gait and motor coordination deficits (Puram et al., 2011). Mechanistically, TRPC5 forms a complex specifically with CaMKIIβ and induces the CaMKIIβ-dependent phosphorylation of ubiquitin ligase Cdc20-APC at the centrosome, suggesting the significance of the TRPC5/CaMKIIβ/Cdc20-APC signaling axis in the regulation of dendritic morphology (Puram et al., 2011). Thus, the TRPC1, TRPC4, and TRPC5 channels are key regulators for proper establishment of neuronal connectivity.
In cultured cortical neurons, pharmacological perturbation of TRPM2 markedly increases the axonal growth, and the neurons isolated from Trpm2 KO mice have significantly longer neurites and a greater number of spines than those isolated from control mice (Jang et al., 2014). In addition, the blockage of TRPM2 reverses lysophosphatidic acid induced suppression of neurite growth (Jang et al., 2014), suggesting that TRPM2 is a negative regulator of neurite outgrowth.
Redox-Sensitive TRP Channels in Higher Brain Functions
Genome scanning for 47 bipolar disorder families has shown that thereis a susceptibility locus near the telomere on chromosome 21q (Straub et al., 1994). Fine mapping linkage analysis identified that TRPM2 on chromosome 21q is associated with bipolar disorder (McQuillin et al., 2006). Two SNPs of TRPM2 were found in the analysis: a base pair change in exon 11 of TRPM2, which causes an amino acid substitution (aspartic acid to glutamic acid: D543E), and a non-conservative change from arginine to cysteine (R755C). Notably, the D543E mutation induces loss of function of TRPM2 activities, and Trpm2 KO mice exhibit bipolar disorder-related behaviors, such as mood disturbances and impairments in social cognition (Jang et al., 2015) (Table 2). Interestingly, disruption of Trpm2 increases phosphorylation of GSK-3, which is implicated as one of the main targets of lithium (Xie et al., 2011; Jang et al., 2015). Although the significance of GSK-3 in the pathology of bipolar disorder is still debated (Alda, 2015), these observations suggest that dysregulation of GSK-3 could, at least in part, account for the bipolar disorder-related behaviors induced by hypoactive TRPM2 mutations. Furthermore, a SNP of TRPM2 has recently been identified as a risk factor for bipolar disorder in a Japanese population (Mahmuda et al., 2020). These lines of evidence suggest a link between defects in TRPM2 activities and the pathology of bipolar disorder.
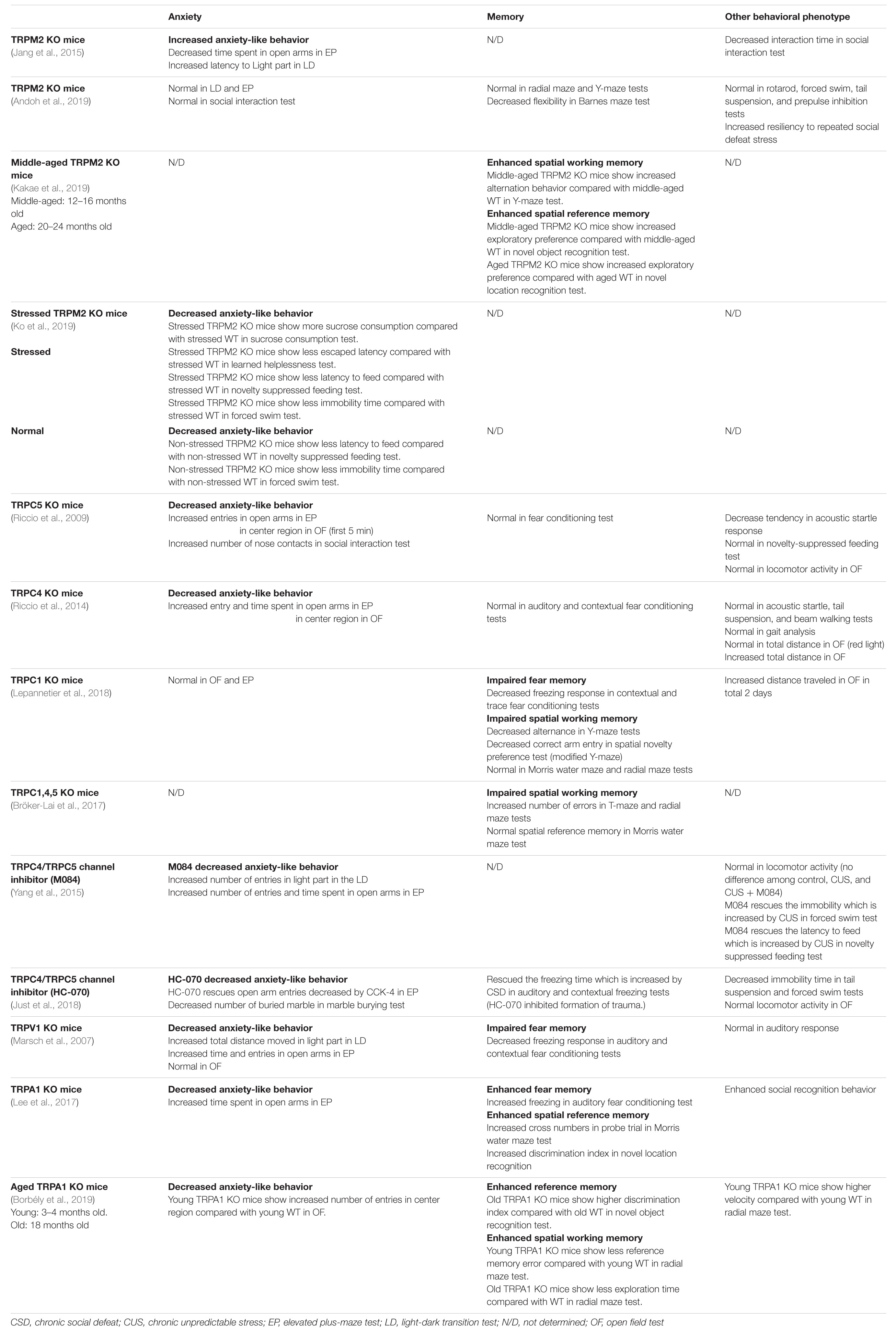
Table 2. Behavioral phenotypes in mice with genetic or pharmacological inactivation of redox-sensitive TRP channels.
In addition to the connection between TRPM2 and bipolar disorder, accumulating evidence has indicated the impact of TRPM2 on major depressive disorder. In a mouse model of depression via chronic unpredictable stress, disruption of Trpm2 produced antidepressant-like behaviors accompanied by reduced ROS, suppressed ROS-induced calpain activation, and enhanced phosphorylation of two Cdk5 targets, synapsin 1 and histone deacetylase 5, which are linked to synaptic function and gene expression, respectively (Ko et al., 2019). Notably, while middle-aged (12–16 months) and aged (20–24 months) wild-type (WT) mice exhibited memory dysfunction compared with young (2–3 months) WT mice, these characteristics were undetectable in Trpm2 KO mice (Kakae et al., 2019) (Table 2), potentially owing to defects in the sensing of oxidative stress, whose levels are increased by aging (Tan et al., 2018). Trpm2 deficiency also attenuates social avoidance induced by repeated social defeat stress in mice (Andoh et al., 2019). Under physiological conditions, a comprehensive behavioral test battery has shown that Trpm2 KO mice exhibit no behavioral phenotypes in light/dark transition, rotarod, elevated plus maze, social interaction, prepulse inhibition, Y-maze, forced swim, cued and contextual fear conditioning, and tail suspension tests (Andoh et al., 2019), whereas Trpm2 KO mice were also reported to exhibit bipolar disorder-related behaviors (Jang et al., 2015) (Table 2). Differences in the genetic backgrounds of mice or the animal facilities and equipment used in the behavioral analyses could explain the inconsistency of the results. Collectively, these findings suggest that disruption of Trpm2 potentially induces behavioral phenotypes in pathological conditions that could be related to oxidative stress.
TRPC4 and TRPC5 are highly expressed in the cortex and amygdala, the regions thought to be crucial in regulating anxiety (Riccio et al., 2009, 2014). Importantly, previous works with transgenic mice suggest that TRPC4 and TRPC5 play important roles in amygdala function, and thus inhibition of TRPC4 and TRPC5 have anxiolytic effects (Riccio et al., 2009, 2014). This implicates the significance of both TRPC4 and TRPC5 in innate fear function, which represents a key response to environmental stress. Trpc5 KO mice exhibited diminished innate fear levels in response to innately aversive stimuli (Riccio et al., 2009) (Table 2). Trpc4 KO mice also showed suppressed Gα q/11-dependent responses in the amygdala, which regulate fear-related behavioral processes, and decreased anxiety-like behaviors (Riccio et al., 2014) (see “Neuronal and Glial Functions of Redox-Sensitive TRP Channels” section) (Table 2). Trpc4 knockdown in the lateral amygdala via lentivirus-mediated gene delivery of RNAi mirrored the behavioral phenotype of constitutive Trpc4 KO mice. Given that the animal model for the analysis of innate fear responses is limited, Trpc4 and Trpc5 KO mice may be useful animal models for investigating the mechanisms underlying the regulation of innate fear responses. It is worth noting that there is a caveat regarding interpretation of the behavioral data because Trpc5 KO mice have deficits in gait and motor coordination (Puram et al., 2011).
In contrast to Trpc4 and Trpc5 KO mice, deletion of the Trpc1 gene impairs fear memory formation but not innate fear behavior (Lepannetier et al., 2018), suggesting that TRPC1 regulates fear-related behavioral processes through distinct mechanisms from the TRPC5- and TRPC4-mediated pathways. In hippocampal functions, deletion of Trpc1 impaired functioning in the Y-maze task, and the triple deficiency of Trpc1/Trpc4/Trpc5 induced reduced performance in the T-maze and radial maze tests, while both Trpc1 single KO and Trpc1/Trpc4/Trpc5 triple KO mice showed normal acquisition in the Morris water maze task (Bröker-Lai et al., 2017; Lepannetier et al., 2018), suggesting that TRPC1 and possibly both TRPC4 and TRPC5 regulate spatial working memory but not spatial reference memory.
Pharmacological analysis in rats revealed that the TRPV1 antagonist, capsazepine, attenuates anxiety-like behaviors in the elevated-plus maze test (Kasckow et al., 2004). Consistent with this observation, Trpv1 KO mice exhibited less anxiety-related behaviors in the elevated-plus maze test and in the light/dark test compared to their WT littermates (Marsch et al., 2007) (Table 2). Furthermore, Trpv1 KO mice showed less freezing in auditory and contextual fear conditioning tests, suggesting that TRPV1 regulates fear memory formation (Marsch et al., 2007). Under stressful conditions, in vivo activation of TRPV1 by intrahippocampal or intragastrical infusion of capsaicin prevented the impairing spatial memory retrieval caused by acute inescapable stress by placing mice on an elevated platform (Li et al., 2008), suggesting that activation of TRPV1 induces anti-stress effects.
Trpa1 KO mice displayed decreased anxiety-like behaviors in elevated-plus maze, better performance in the fear conditioning and novel location recognition tests, and enhanced social recognition behavior (Lee et al., 2017) (Table 2), possibly due, at least in part, to the evidence that TRPA1 negatively regulates hippocampal functions potentially through the suppression of neurite outgrowth (Lee et al., 2017). However, there is a caveat regarding the interpretation of the behavioral data because Trpa1 KO mice are suggested to exhibit impaired motor function through axonal bundle fragmentation, downregulation of myelin basic protein, and decreased mature oligodendrocyte population in the brain (Lee et al., 2017); therefore, the observed behavioral phenotypes could be merely due to impaired motor functions rather than hippocampal functions. Interestingly, TRPA1 in astrocytes is implicated to partially contribute to cuprizone-induced demyelination, which is frequently used for the animal model of multiple sclerosis (Kriszta et al., 2020), raising the possibility that there is a connection between TRPA1 expression or function and psychiatric disorders. Notably, another behavioral analysis using aged (18-month-old) Trpa1 KO mice supports the notion that TRPA1 negatively regulates hippocampal functions in an age-dependent manner (Borbély et al., 2019) (Table 2). Thus, these lines of evidence suggest that TRPA1 is a negative regulator of brain functions, raising the possibility that there is a connection between TRPA1 expression or function and psychiatric disorders; however, further studies are required to validate this hypothesis.
Perspective
As described above, accumulating evidence has indicated that most redox-sensitive TRP channels have significant impacts on higher brain functions even though the role of oxidative stress as the activation trigger of the channels in this context is not fully understood. Comprehensive analysis of gene expression in normal brains and the brains of patients with psychiatric disorders would delineate the significance of redox-sensitive TRP channels in these disorders, where notably, TRPM2 expression is enhanced in hippocampal tissue samples from patients with major depressive disorder (Ko et al., 2019), and, furthermore, TRPA1 is upregulated in astrocytes of the hippocampus in the mouse model of Alzheimer’s disease, in which oxidative stress is deeply involved (Lee et al., 2016). Given that oxidative stress is associated with psychiatric disorders and that the expression of redox-sensitive TRP channels is induced by oxidative stress partly via the activation of NRF2, an oxidant defense transcription factor (Takahashi et al., 2018), it is possible that upregulated redox-sensitive TRP channels are involved in the pathogenesis or pathophysiology of psychiatric disorders (Figures 1, 2).
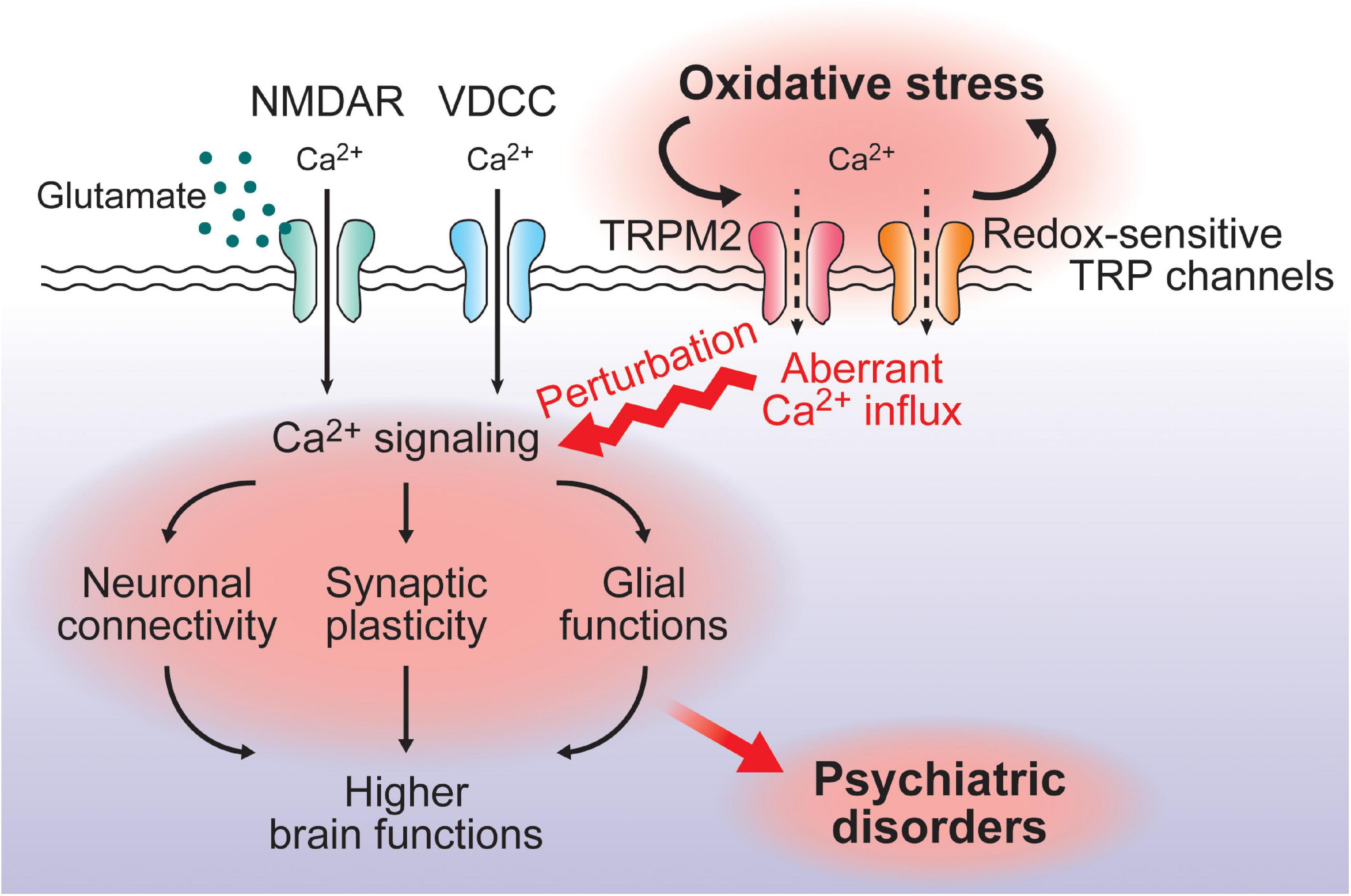
Figure 2. A putative model for the impact of redox-sensitive TRPchannels in the pathogenesis/pathophysiology of psychiatricdisorders. VDCC- or NMDAR-mediated Ca2+ signaling playsfundamental roles in neural functions such as neuronal connectivity, synaptic plasticity, and glial functions, contributing to higher brain functions. Oxidative stress alters expression and activities of redox-sensitive TRP channels, which further enhances oxidative stress and induces a perturbation of the Ca2+ signaling mediated by VDCC or NMDAR. This could be associated with the pathogenesis/pathophysiology of psychiatric disorders. Notably, SNPs of TRPM2 have been identified as a risk factor for bipolar disorder.
The concept of excitation-inhibition balance in neuropsychiatricdisorders has been considered as a framework for investigating theirmechanisms (Sohal and Rubenstein, 2019). Given that TRPA1 has the highestoxidation sensitivity among the TRP channels (Takahashi et al., 2011b; Mori et al., 2016), it is likely that TRPA1 senses oxidative stressacutely and moderates the excitation-inhibition balance by regulating astrocyte resting [Ca2+]i, which affects inhibitory synapse efficacy through the reduction of GABA transport (Shigetomi et al., 2012). Recently, several studies have shown that treatment with novel TRPC4/TRPC5 channel inhibitors produce antidepressant and anxiolytic-like effects in mice, suggesting that TRPC4 and TRPC5 channels are novel molecular candidates for the treatment of psychiatric symptoms (Yang et al., 2015; Just et al., 2018) (Table 2). Thus, the investigation of redox-sensitive TRP channels in the pathogenesis/pathophysiology of psychiatric disorders may lead to the development of novel therapeutic strategies for the treatment of these disorders.
Author Contributions
AN and NT generated the first draft of the manuscript. All authors contributed to the revisions.
Conflict of Interest
The authors declare that the research was conducted in the absence of any commercial or financial relationships that could be construed as a potential conflict of interest.
References
Abdalla, D. S., Monteiro, H. P., Oliveira, J. A., and Bechara, E. J. (1986). Activities of superoxide dismutase and glutathione peroxidase in schizophrenic and manic-depressive patients. Clin. Chem. 32, 805–807. doi: 10.1093/clinchem/32.5.805
Akyol, Ö, Herken, H., Uz, E., Fadıllıoğlu, E., Ünal, S., Sögüt, S., et al. (2002). The indices of endogenous oxidative and antioxidative processes in plasma from schizophrenic patients: the possible role of oxidant/antioxidant imbalance. Prog. Neuropsychopharmacol. Biol. Psychiatry 26, 995–1005. doi: 10.1016/S0278-5846(02)00220-8
Alda, M. (2015). Lithium in the treatment of bipolar disorder: pharmacology and pharmacogenetics. Mol. Psychiatry 20, 661–670. doi: 10.1038/mp.2015.4
Andoh, C., Nishitani, N., Hashimoto, E., Nagai, Y., Takao, K., Miyakawa, T., et al. (2019). TRPM2 confers susceptibility to social stress but is essential for behavioral flexibility. Brain Res. 1704, 68–77. doi: 10.1016/j.brainres.2018.09.031
Arvindakshan, M., Ghate, M., Ranjekar, P. K., Evans, D. R., and Mahadik, S. P. (2003). Supplementation with a combination of ω-3 fatty acids and antioxidants (vitamins E and C) improves the outcome of schizophrenia. Schizophr. Res. 62, 195–204. doi: 10.1016/S0920-9964(02)00284-0
Atmaca, M., Tezcan, E., Kuloglu, M., Ustundag, B., and Tunckol, H. (2004). Antioxidant enzyme and malondialdehyde valuesin social phobia before and after citalopram treatment. Eur. Arch. Psychiatry Clin. Neurosci. 254, 231–235. doi: 10.1007/s00406-004-0484-3
Ben Othmen, L., Mechri, A., Fendri, C., Bost, M., Chazot, G., Gaha, L., et al. (2008). Altered antioxidant defense system in clinically stable patients with schizophrenia and their unaffected siblings. Prog. Neuropsychopharmacol. Biol. Psychiatry 32, 155–159. doi: 10.1016/j.pnpbp.2007.08.003
Berk, M., Copolov, D. L., Dean, O., Lu, K., Jeavons, S., Schapkaitz, I., et al. (2008). N-acetyl cysteine for depressive symptoms in bipolar disorder—a double-blind randomized placebo-controlled trial. Biol. Psychiatry 64, 468–475. doi: 10.1016/j.biopsych.2008.04.022
Berk, M., Dean, O. M., Cotton, S. M., Jeavons, S., Tanious, M., Kohlmann, K., et al. (2014). The efficacy of adjunctive N-acetylcysteine in major depressive disorder: a double-blind, randomized, placebo-controlled trial. J. Clin. Psychiatry 75, 628–636. doi: 10.4088/JCP.13m08454
Berk, M., Turner, A., Malhi, G. S., Ng, C. H., Cotton, S. M., Dodd, S., et al. (2019). A randomised controlled trial of a mitochondrial therapeutic target for bipolar depression: mitochondrial agents, N-acetylcysteine, and placebo. BMC Med. 17:18. doi: 10.1186/s12916-019-1257-1
Bigos, K. L., Mattay, V. S., Callicott, J. H., Straub, R. E., Vakkalanka, R., Kolachana, B., et al. (2010). Genetic variation in CACNA1C affects brain circuitries related to mental illness. Arch. Gen. Psychiatry 67, 939–945. doi: 10.1001/archgenpsychiatry.2010.96
Bilici, M., Efe, H., Köroğlu, M. A., Uydu, H. A., Bekaroğlu, M., and Değer, O. (2001). Antioxidative enzyme activities and lipid peroxidation in major depression: alterations by antidepressant treatments. J. Affect. Disord. 64, 43–51. doi: 10.1016/S0165-0327(00)00199-3
Bond, C. E., and Greenfield, S. A. (2007). Multiple cascade effects of oxidative stress on astroglia. Glia 55, 1348–1361. doi: 10.1002/glia.20547
Borbély, É, Payrits, M., Hunyady, Á, Mezö, G., and Pintér, E. (2019). Important regulatory function of transient receptor potential ankyrin 1 receptors in age-related learning and memory alterations of mice. GeroScience 41, 643–654. doi: 10.1007/s11357-019-00083-1
Bröker-Lai, J., Kollewe, A., Schindeldecker, B., Pohle, J., Nguyen Chi, V., Mathar, I., et al. (2017). Heteromeric channels formed by TRPC1, TRPC4 and TRPC5 define hippocampal synaptic transmission and working memory. EMBO J. 36, 2770–2789. doi: 10.15252/embj.201696369
Catterall, W. A. (2011). Voltage-gated calcium channels. Cold Spring Harb. Perspect. Biol. 3:a003947. doi: 10.1101/cshperspect.a003947
Chávez, A. E., Hernández, V. M., Rodenas-Ruano, A., Chan, C. S., and Castillo, P. E. (2014). Compartment-specific modulation of GABAergic synaptic transmission by TRPV1 channels in the dentate gyrus. J. Neurosci. 34, 16621–16629. doi: 10.1523/JNEUROSCI.3635-14.2014
Clapham, D. E. (2003). TRP channels as cellular sensors. Nature 426, 517–524. doi: 10.1038/nature02196
Cotter, D. R., Pariante, C. M., and Everall, I. P. (2001). Glial cell abnormalities in major psychiatric disorders: the evidence and implications. Brain Res. Bull. 55, 585–595. doi: 10.1016/S0361-9230(01)00527-5
Dakhale, G., Khanzode, S., Khanzode, S., Saoji, A., Khobragade, L., and Turankar, A. (2004). Oxidative damage and schizophrenia: the potential benefit by atypical antipsychotics. Neuropsychobiology 49, 205–209. doi: 10.1159/000077368
Dakhale, G. N., Khanzode, S. D., Khanzode, S. S., and Saoji, A. (2005). Supplementation of vitamin C with atypical antipsychotics reduces oxidative stress and improves the outcome of schizophrenia. Psychopharmacology 182, 494–498. doi: 10.1007/s00213-005-0117-1
Davare, M. A., Fortin, D. A., Saneyoshi, T., Nygaard, S., Kaech, S., Banker, G., et al. (2009). Transient receptor potential canonical 5 channels activate Ca2 + /calmodulin kinase Iγ to promote axon formation in hippocampal neurons. J. Neurosci. 29, 9794–9808. doi: 10.1523/JNEUROSCI.1544-09.2009
Dhar, M., Wayman, G. A., Zhu, M., Lambert, T. J., Davare, M. A., and Appleyard, S. M. (2014). Leptin-induced spine formation requires TrpC channels and the CaM kinase cascade in the hippocampus. J. Neurosci. 34, 10022–10033. doi: 10.1523/JNEUROSCI.2868-13.2014
Di, A., Gao, X.-P., Qian, F., Kawamura, T., Han, J., Hecquet, C., et al. (2012). The redox-sensitive cation channel TRPM2 modulates phagocyte ROS production and inflammation. Nat. Immunol. 13, 29–34. doi: 10.1038/ni.2171
Ellegaard, P. K., Licht, R. W., Nielsen, R. E., Dean, O. M., Berk, M., Poulsen, H. E., et al. (2019). The efficacy of adjunctive N-acetylcysteine in acute bipolar depression: a randomized placebo-controlled study. J. Affect. Disord. 245, 1043–1051. doi: 10.1016/j.jad.2018.10.083
Ersan, S., Bakir, S., Erdal Ersan, E., and Dogan, O. (2006). Examination of free radical metabolism and antioxidant defence system elements in patients with obsessive–compulsive disorder. Prog. Neuropsychopharmacol. Biol. Psychiatry 30, 1039–1042. doi: 10.1016/j.pnpbp.2006.03.034
Fernandes, B. S., Dean, O. M., Dodd, S., Malhi, G. S., and Berk, M. (2016). N-acetylcysteine in depressive symptoms and functionality: a systematic review and meta-analysis. J. Clin. Psychiatry 77, 457–466. doi: 10.4088/JCP.15r09984
Ferreira, M. A. R., O’Donovan, M. C., Meng, Y. A., Jones, I. R., Ruderfer, D. M., Jones, L., et al. (2008). Collaborative genome-wide association analysis supports a role for ANK3 and CACNA1C in bipolar disorder. Nat. Genet. 40, 1056–1058. doi: 10.1038/ng.209
Fonfria, E., Murdock, P. R., Cusdin, F. S., Benham, C. D., Kelsell, R. E., and McNulty, S. (2006). Tissue distribution profiles of the human TRPM cation channel family. J. Recept. Signal Transduct. Res. 26, 159–178. doi: 10.1080/10799890600637506
Forlenza, M. J., and Miller, G. E. (2006). Increased serum levels of 8-Hydroxy-2′-deoxyguanosine in clinical depression. Psychosom. Med. 68, 1–7. doi: 10.1097/01.psy.0000195780.37277.2a
Gibson, H. E., Edwards, J. G., Page, R. S., Van Hook, M. J., and Kauer, J. A. (2008). TRPV1 channels mediate long-term depression at synapses on hippocampal interneurons. Neuron 57, 746–759. doi: 10.1016/j.neuron.2007.12.027
Golovina, V. A. (2005). Visualization of localized store-operated calcium entry in mouse astrocytes. Close proximity to the endoplasmic reticulum. J. Physiol. 564, 737–749. doi: 10.1113/jphysiol.2005.085035
Greka, A., Navarro, B., Oancea, E., Duggan, A., and Clapham, D. E. (2003). TRPC5 is a regulator of hippocampal neurite length and growth cone morphology. Nat. Neurosci. 6, 837–845. doi: 10.1038/nn1092
Griesi-Oliveira, K., Acab, A., Gupta, A. R., Sunaga, D. Y., Chailangkarn, T., Nicol, X., et al. (2015). Modeling non-syndromic autism and the impact of TRPC6 disruption in human neurons. Mol. Psychiatry 20, 1350–1365. doi: 10.1038/mp.2014.141
Hara, Y., Wakamori, M., Ishii, M., Maeno, E., Nishida, M., Yoshida, T., et al. (2002). LTRPC2 Ca2 + -permeable channel activated by changes in redox status confers susceptibility to cell death. Mol. Cell 9, 163–173. doi: 10.1016/S1097-2765(01)00438-5
He, Z., Jia, C., Feng, S., Zhou, K., Tai, Y., Bai, X., et al. (2012). TRPC5 channel is the mediator of neurotrophin-3 in regulating dendritic growth via CaMKIIα in rat hippocampal neurons. J. Neurosci. 32, 9383–9395. doi: 10.1523/JNEUROSCI.6363-11.2012
Herken, H., Gurel, A., Selek, S., Armutcu, F., Ozen, M. E., Bulut, M., et al. (2007). Adenosine deaminase, nitric oxide, superoxide dismutase, and xanthine oxidase in patients with major depression: impact of antidepressant treatment. Arch. Med. Res. 38, 247–252. doi: 10.1016/j.arcmed.2006.10.005
Herken, H., Uz, E., Özyurt, H., Söğüt, S., Virit, O., and Akyol, Ö (2001). Evidence that the activities of erythrocyte free radical scavenging enzymes and the products of lipid peroxidation are increased in different forms of schizophrenia. Mol. Psychiatry 6, 66–73. doi: 10.1038/sj.mp.4000789
Hinman, A., Chuang, H., Bautista, D. M., and Julius, D. (2006). TRP channel activation by reversible covalent modification. Proc. Natl. Acad. Sci. U.S.A. 103, 19564–19568. doi: 10.1073/pnas.0609598103
Hiroi, T., Wajima, T., Negoro, T., Ishii, M., Nakano, Y., Kiuchi, Y., et al. (2013). Neutrophil TRPM2 channels are implicated in the exacerbation of myocardial ischaemia/reperfusion injury. Cardiovasc. Res. 97, 271–281. doi: 10.1093/cvr/cvs332
Jang, Y., Lee, M. H., Lee, J., Jung, J., Lee, S. H., Yang, D.-J., et al. (2014). TRPM2 mediates the lysophosphatidic acid-induced neurite retraction in the developing brain. Pflüg. Arch. Eur. J. Physiol. 466, 1987–1998. doi: 10.1007/s00424-013-1436-4
Jang, Y., Lee, S. H., Lee, B., Jung, S., Khalid, A., Uchida, K., et al. (2015). TRPM2, a susceptibility gene for bipolar disorder, regulates glycogen synthase kinase-3 activity in the brain. J. Neurosci. 35, 11811–11823. doi: 10.1523/JNEUROSCI.5251-14.2015
Jeon, D., Kim, S., Chetana, M., Jo, D., Ruley, H. E., and Lin, S.-Y. et al. (2010). Observational fear learning involves affective pain system and Cav1.2 Ca2 + channels in ACC. Nat. Neurosci. 13, 482–488. doi: 10.1038/nn.2504
Jeon, J.-P., Roh, S.-E., Wie, J., Kim, J., Kim, H., Lee, K.-P., et al. (2013). Activation of TRPC4β by Gαi subunit increases Ca2 + selectivity and controls neurite morphogenesis in cultured hippocampal neuron. Cell Calcium 54, 307–319. doi: 10.1016/j.ceca.2013.07.006
Just, S., Chenard, B. L., Ceci, A., Strassmaier, T., Chong, J. A., Blair, N. T., et al. (2018). Treatment with HC-070, a potent inhibitor of TRPC4 and TRPC5, leads to anxiolytic and antidepressant effects in mice. PLoS One 13:e0191225. doi: 10.1371/journal.pone.0191225
Kabir, Z. D., Martínez-Rivera, A., and Rajadhyaksha, A. M. (2017). From gene to behavior: L-type calcium channel mechanisms underlying neuropsychiatric symptoms. Neurotherapeutics 14, 588–613. doi: 10.1007/s13311-017-0532-0
Kaczmarek, J. S., Riccio, A., and Clapham, D. E. (2012). Calpain cleaves and activates the TRPC5 channel to participate in semaphorin 3A-induced neuronal growth cone collapse. Proc. Natl. Acad. Sci. U.S.A. 109, 7888–7892. doi: 10.1073/pnas.1205869109
Kakae, M., Miyanohara, J., Morishima, M., Nagayasu, K., Mori, Y., Shirakawa, H., et al. (2019). Pathophysiological role of TRPM2 in age-related cognitive impairment in mice. Neuroscience 408, 204–213. doi: 10.1016/j.neuroscience.2019.04.012
Kaneko, S., Kawakami, S., Hara, Y., Wakamori, M., Itoh, E., Minami, T., et al. (2006). A Critical Role of TRPM2 in neuronal cell death by hydrogen peroxide. J. Pharmacol. Sci. 101, 66–76. doi: 10.1254/jphs.FP0060128
Kasckow, J. W., Mulchahey, J. J., and Geracioti, T. D. Jr. (2004). Effects of the vanilloid agonist olvanil and antagonist capsazepine on rat behaviors. Prog. Neuropsychopharmacol. Biol. Psychiatry 28, 291–295. doi: 10.1016/j.pnpbp.2003.10.007
Kashio, M., Sokabe, T., Shintaku, K., Uematsu, T., Fukuta, N., Kobayashi, N., et al. (2012). Redox signal-mediated sensitization of transient receptor potential melastatin 2 (TRPM2) to temperature affects macrophage functions. Proc. Natl. Acad. Sci. U.S.A. 109, 6745–6750. doi: 10.1073/pnas.1114193109
Kauer, J. A., and Gibson, H. E. (2009). Hot flash: TRPV channels in the brain. Trends Neurosci. 32, 215–224. doi: 10.1016/j.tins.2008.12.006
Khanzode, S. D., Dakhale, G. N., Khanzode, S. S., Saoji, A., and Palasodkar, R. (2003). Oxidative damage and major depression: the potential antioxidant action of selective serotonin re-uptake inhibitors. Redox Rep. 8, 365–370. doi: 10.1179/135100003225003393
Kheradpezhouh, E., Choy, J. M. C., Daria, V. R., and Arabzadeh, E. (2017). TRPA1 expression and its functional activation in rodent cortex. Open Biol. 7:160314. doi: 10.1098/rsob.160314
Knowles, H., Heizer, J. W., Li, Y., Chapman, K., Ogden, C. A., Andreasen, K., et al. (2011). Transient Receptor Potential Melastatin 2 (TRPM2) ion channel is required for innate immunity against Listeria monocytogenes. Proc. Natl. Acad. Sci. U.S.A. 108, 11578–11583. doi: 10.1073/pnas.1010678108
Ko, S. Y., Wang, S. E., Lee, H. K., Jo, S., Han, J., Lee, S. H., et al. (2019). Transient receptor potential melastatin 2 governs stress-induced depressive-like behaviors. Proc. Natl. Acad. Sci. U.S.A. 116, 1770–1775. doi: 10.1073/pnas.1814335116
Koch, M., Kreutz, S., Böttger, C., Grabiec, U., Ghadban, C., Korf, H.-W., et al. (2011). The cannabinoid WIN 55,212-2-mediated protection of dentate gyrus granule cells is driven by CB1 receptors and modulated by TRPA1 and Cav2.2 channels. Hippocampus 21, 554–564. doi: 10.1002/hipo.20772
Kozai, D., Ogawa, N., and Mori, Y. (2013). Redox regulation of transient receptor potential channels. Antioxid. Redox Signal. 21, 971–986. doi: 10.1089/ars.2013.5616
Kraft, R., Grimm, C., Grosse, K., Hoffmann, A., Sauerbruch, S., Kettenmann, H., et al. (2004). Hydrogen peroxide and ADP-ribose induce TRPM2-mediated calcium influx and cation currents in microglia. Am. J. Physiol. Cell Physiol. 286, C129–C137. doi: 10.1152/ajpcell.00331.2003
Kriszta, G., Nemes, B., Sándor, Z., Ács, P., Komoly, S., Berente, Z., et al. (2020). Investigation of cuprizone-induced demyelination in mGFAP-driven conditional transient receptor potential ankyrin 1 (TRPA1) receptor knockout mice. Cells 9:81. doi: 10.3390/cells9010081
Kuloglu, M., Atmaca, M., Tezcan, E., Gecici, Ö, Tunckol, H., and Ustundag, B. (2002a). Antioxidant enzyme activities and malondialdehyde levels in patients with obsessive-compulsive disorder. Neuropsychobiology 46, 27–32. doi: 10.1159/000063573
Kuloglu, M., Atmaca, M., Tezcan, E., Ustundag, B., and Bulut, S. (2002b). Antioxidant enzyme and malondialdehyde levels in patients with panic disorder. Neuropsychobiology 46, 186–189. doi: 10.1159/000067810
Kuloglu, M., Ustundag, B., Atmaca, M., Canatan, H., Tezcan, A. E., and Cinkilinc, N. (2002c). Lipid peroxidation and antioxidant enzyme levels in patients with schizophrenia and bipolar disorder. Cell Biochem. Funct. 20, 171–175. doi: 10.1002/cbf.940
Langwieser, N., Christel, C. J., Kleppisch, T., Hofmann, F., Wotjak, C. T., and Moosmang, S. (2010). Homeostatic switch in hebbian plasticity and fear learning after sustained loss of Cav1.2 calcium channels. J. Neurosci. 30, 8367–8375. doi: 10.1523/JNEUROSCI.4164-08.2010
Lee, A. S., Ra, S., Rajadhyaksha, A. M., Britt, J. K., De Jesus-Cortes, H., Gonzales, K. L., et al. (2012). Forebrain elimination of cacna1c mediates anxiety-like behavior in mice. Mol. Psychiatry 17, 1054–1055. doi: 10.1038/mp.2012.71
Lee, C. R., Machold, R. P., Witkovsky, P., and Rice, M. E. (2013). TRPM2 channels are required for NMDA-induced burst firing and contribute to H2O2-dependent modulation in substantia nigra pars reticulata GABAergic neurons. J. Neurosci. 33, 1157–1168. doi: 10.1523/JNEUROSCI.2832-12.2013
Lee, K.-I., Lee, H.-T., Lin, H.-C., Tsay, H.-J., Tsai, F.-C., Shyue, S.-K., et al. (2016). Role of transient receptor potential ankyrin 1 channels in Alzheimer’s disease. J. Neuroinflammation 13:92. doi: 10.1186/s12974-016-0557-z
Lee, K.-I., Lin, H.-C., Lee, H.-T., Tsai, F.-C., and Lee, T.-S. (2017). Loss of transient receptor potential ankyrin 1 channel deregulates emotion, learning and memory, cognition, and social behavior in mice. Mol. Neurobiol. 54, 3606–3617. doi: 10.1007/s12035-016-9908-0
Lee, M., Cho, T., Jantaratnotai, N., Wang, Y. T., McGeer, E., and McGeer, P. L. (2010). Depletion of GSH in glial cells induces neurotoxicity: relevance to aging and degenerative neurological diseases. FASEB J. 24, 2533–2545. doi: 10.1096/fj.09-149997
Lepannetier, S., Gualdani, R., Tempesta, S., Schakman, O., Seghers, F., Kreis, A., et al. (2018). Activation of TRPC1 channel by metabotropic glutamate receptor mGluR5 modulates synaptic plasticity and spatial working memory. Front. Cell. Neurosci. 12:318. doi: 10.3389/fncel.2018.00318
Li, H.-B., Mao, R.-R., Zhang, J.-C., Yang, Y., Cao, J., and Xu, L. (2008). Antistress effect of TRPV1 channel on synaptic plasticity and spatial memory. Biol. Psychiatry 64, 286–292. doi: 10.1016/j.biopsych.2008.02.020
Li, M., Chen, C., Zhou, Z., Xu, S., and Yu, Z. (2012). A TRPC1-mediated increase in store-operated Ca2 + entry is required for the proliferation of adult hippocampal neural progenitor cells. Cell Calcium 51, 486–496. doi: 10.1016/j.ceca.2012.04.014
Li, Y., Jia, Y.-C., Cui, K., Li, N., Zheng, Z.-Y., Wang, Y., et al. (2005). Essential role of TRPC channels in the guidance of nerve growth cones by brain-derived neurotrophic factor. Nature 434, 894–898. doi: 10.1038/nature03477
Machado-Vieira, R., Andreazza, A. C., Viale, C. I., Zanatto, V., Cereser, V., Vargas, R., et al. (2007). Oxidative stress parameters in unmedicated and treated bipolar subjects during initial manic episode: a possible role for lithium antioxidant effects. Neurosci. Lett. 421, 33–36. doi: 10.1016/j.neulet.2007.05.016
Macpherson, L. J., Dubin, A. E., Evans, M. J., Marr, F., Schultz, P. G., Cravatt, B. F., et al. (2007). Noxious compounds activate TRPA1 ion channels through covalent modification of cysteines. Nature 445, 541–545. doi: 10.1038/nature05544
Mahmuda, N. A., Yokoyama, S., Munesue, T., Hayashi, K., Yagi, K., Tsuji, C., et al. (2020). One single nucleotide polymorphism of the TRPM2 channel gene identified as a risk factor in bipolar disorder associates with autism spectrum disorder in a japanese population. Diseases 8:4. doi: 10.3390/diseases8010004
Malarkey, E. B., Ni, Y., and Parpura, V. (2008). Ca2 + entry through TRPC1 channels contributes to intracellular Ca2 + dynamics and consequent glutamate release from rat astrocytes. Glia 56, 821–835. doi: 10.1002/glia.20656
Marsch, R., Foeller, E., Rammes, G., Bunck, M., Kössl, M., Holsboer, F., et al. (2007). Reduced anxiety, conditioned fear, and hippocampal long-term potentiation in transient receptor potential vanilloid type 1 receptor-deficient mice. J. Neurosci. 27, 832–839. doi: 10.1523/JNEUROSCI.3303-06.2007
McQuillin, A., Bass, N. J., Kalsi, G., Lawrence, J., Puri, V., Choudhury, K., et al. (2006). Fine mapping of a susceptibility locus for bipolar and genetically related unipolar affective disorders, to a region containing the C21ORF29 and TRPM2 genes on chromosome 21q22.3. Mol. Psychiatry 11, 134–142. doi: 10.1038/sj.mp.4001759
Montell, C., and Rubin, G. M. (1989). Molecular characterization of the drosophila trp locus: a putative integral membrane protein required for phototransduction. Neuron 2, 1313–1323. doi: 10.1016/0896-6273(89)90069-X
Moosmang, S., Haider, N., Klugbauer, N., Adelsberger, H., Langwieser, N., Müller, J., et al. (2005). Role of hippocampal Cav1.2 Ca2 + channels in NMDA receptor-independent synaptic plasticity and spatial memory. J. Neurosci. 25, 9883–9892. doi: 10.1523/JNEUROSCI.1531-05.2005
Moran, M. M., McAlexander, M. A., Bíró, T., and Szallasi, A. (2011). Transient receptor potential channels as therapeutic targets. Nat. Rev. Drug Discov. 10, 601–620. doi: 10.1038/nrd3456
Mori, Y., Takahashi, N., Polat, O. K., Kurokawa, T., Takeda, N., and Inoue, M. (2016). Redox-sensitive transient receptor potential channels in oxygen sensing and adaptation. Pflüg. Arch. Eur. J. Physiol. 468, 85–97. doi: 10.1007/s00424-015-1716-2
Nakao, A., Miki, T., Shoji, H., Nishi, M., Takeshima, H., Miyakawa, T., et al. (2015). Comprehensive behavioral analysis of voltage-gated calcium channel beta-anchoring and -regulatory protein knockout mice. Front. Behav. Neurosci. 9:141. doi: 10.3389/fnbeh.2015.00141
Ng, F., Berk, M., Dean, O., and Bush, A. I. (2008). Oxidative stress in psychiatric disorders: evidence base and therapeutic implications. Int. J. Neuropsychopharmacol. 11, 851–876. doi: 10.1017/S1461145707008401
Nilius, B., Owsianik, G., Voets, T., and Peters, J. A. (2007). Transient receptor potential cation channels in disease. Physiol. Rev. 87, 165–217. doi: 10.1152/physrev.00021.2006
Olah, M. E., Jackson, M. F., Li, H., Perez, Y., Sun, H.-S., Kiyonaka, S., et al. (2009). Ca2 + -dependent induction of TRPM2 currents in hippocampal neurons. J. Physiol. 587, 965–979. doi: 10.1113/jphysiol.2008.162289
Peet, M., Murphy, B., Shay, J., and Horrobin, D. (1998). Depletion of Omega-3 fatty acid levels in red blood cell membranes of depressive patients. Biol. Psychiatry 43, 315–319. doi: 10.1016/S0006-3223(97)00206-0
Penzes, P., Cahill, M. E., Jones, K. A., VanLeeuwen, J.-E., and Woolfrey, K. M. (2011). Dendritic spine pathology in neuropsychiatric disorders. Nat. Neurosci. 14, 285–293. doi: 10.1038/nn.2741
Perraud, A.-L., Takanishi, C. L., Shen, B., Kang, S., Smith, M. K., Schmitz, C., et al. (2005). Accumulation of free ADP-ribose from mitochondria mediates oxidative stress-induced gating of TRPM2 cation channels. J. Biol. Chem. 280, 6138–6148. doi: 10.1074/jbc.M411446200
Pires, P. W., and Earley, S. (2018). Neuroprotective effects of TRPA1 channels in the cerebral endothelium following ischemic stroke. eLife 7:e35316. doi: 10.7554/eLife.35316
Puram, S. V., Riccio, A., Koirala, S., Ikeuchi, Y., Kim, A. H., Corfas, G., et al. (2011). A TRPC5-regulated calcium signaling pathway controls dendrite patterning in the mammalian brain. Genes Dev. 25, 2659–2673. doi: 10.1101/gad.174060.111
Qiu, J., Fang, Y., Rønnekleiv, O. K., and Kelly, M. J. (2010). Leptin excites proopiomelanocortin neurons via activation of TRPC channels. J. Neurosci. 30, 1560–1565. doi: 10.1523/JNEUROSCI.4816-09.2010
Rah, S.-Y., Kwak, J.-Y., Chung, Y.-J., and Kim, U.-H. (2015). ADP-ribose/TRPM2-mediated Ca2 + signaling is essential for cytolytic degranulation and antitumor activity of natural killer cells. Sci. Rep. 5:9482. doi: 10.1038/srep09482
Ramírez-Barrantes, R., Cordova, C., Poblete, H., Muñoz, P., Marchant, I., Wianny, F., et al. (2016). Perspectives of TRPV1 function on the neurogenesis and neural plasticity. Neural Plast. 2016:e1568145. doi: 10.1155/2016/1568145
Ranjekar, P. K., Hinge, A., Hegde, M. V., Ghate, M., Kale, A., Sitasawad, S., et al. (2003). Decreased antioxidant enzymes and membrane essential polyunsaturated fatty acids in schizophrenic and bipolar mood disorder patients. Psychiatry Res. 121, 109–122. doi: 10.1016/S0165-1781(03)00220-8
Riccio, A., Li, Y., Moon, J., Kim, K.-S., Smith, K. S., Rudolph, U., et al. (2009). Essential role for TRPC5 in amygdala function and fear-related behavior. Cell 137, 761–772. doi: 10.1016/j.cell.2009.03.039
Riccio, A., Li, Y., Tsvetkov, E., Gapon, S., Yao, G. L., Smith, K. S., et al. (2014). Decreased anxiety-like behavior and Gαq/11-dependent responses in the amygdala of mice lacking TRPC4 channels. J. Neurosci. 34, 3653–3667. doi: 10.1523/JNEUROSCI.2274-13.2014
Ripke, S., Neale, B. M., Corvin, A., Walters, J. T. R., Farh, K.-H., Holmans, P. A., et al. (2014). Biological insights from 108 schizophrenia-associated genetic loci. Nature 511, 421–427. doi: 10.1038/nature13595
Ripke, S., Sanders, A. R., Kendler, K. S., Levinson, D. F., Sklar, P., Holmans, P. A., et al. (2011). Genome-wide association study identifies five new schizophrenia loci. Nat. Genet. 43, 969–976. doi: 10.1038/ng.940
Ritchie, H. and Roser, M. (2018). Mental Health. Our WorldData. Available online at: https://ourworldindata.org/mental-health (accessed April 24, 2020).
Roberts, J. C., and Davis, J. B. and Benham, C. D. (2004). [3H]Resiniferatoxin autoradiography in the CNS of wild-type and TRPV1 null mice defines TRPV1 (VR-1) protein distribution. Brain Res. 995, 176–183. doi: 10.1016/j.brainres.2003.10.001
Sakaguchi, R., and Mori, Y. (2020). Transient receptor potential (TRP) channels: biosensors for redox environmental stimuli and cellular status. Free Radic. Biol. Med. 146, 36–44. doi: 10.1016/j.freeradbiomed.2019.10.415
Sarandol, A., Sarandol, E., Eker, S. S., Erdinc, S., Vatansever, E., and Kirli, S. (2007). Major depressive disorder is accompanied with oxidative stress: short-term antidepressant treatment does not alter oxidative–antioxidative systems. Hum. Psychopharmacol. Clin. Exp. 22, 67–73. doi: 10.1002/hup.829
Sawamura, S., Shirakawa, H., Nakagawa, T., Mori, Y., and Kaneko, S. (2017). “TRP channels in the brain: what are they there for?,” in Neurobiology of TRP Channels Frontiers in Neuroscience, ed. T. L. R. Emir (Boca Raton, FL: CRC Press/Taylor & Francis).
Sepehrmanesh, Z., Heidary, M., Akasheh, N., Akbari, H., and Heidary, M. (2018). Therapeutic effect of adjunctive N-acetyl cysteine (NAC) on symptoms of chronic schizophrenia: a double-blind, randomized clinical trial. Prog. Neuropsychopharmacol. Biol. Psychiatry 82, 289–296. doi: 10.1016/j.pnpbp.2017.11.001
Shigetomi, E., Jackson-Weaver, O., Huckstepp, R. T., O’Dell, T. J., and Khakh, B. S. (2013). TRPA1 channels are regulators of astrocyte basal calcium levels and long-term potentiation via constitutive d-serine release. J. Neurosci. 33, 10143–10153. doi: 10.1523/JNEUROSCI.5779-12.2013
Shigetomi, E., Tong, X., Kwan, K. Y., Corey, D. P., and Khakh, B. S. (2012). TRPA1 channels regulate astrocyte resting calcium and inhibitory synapse efficacy through GAT-3. Nat. Neurosci. 15, 70–80. doi: 10.1038/nn.3000
Shim, S., Yuan, J. P., Kim, J. Y., Zeng, W., Huang, G., Milshteyn, A., et al. (2009). Peptidyl-Prolyl isomerase FKBP52 controls chemotropic guidance of neuronal growth cones via regulation of TRPC1 channel opening. Neuron 64, 471–483. doi: 10.1016/j.neuron.2009.09.025
Sivrioglu, E. Y., Kirli, S., Sipahioglu, D., Gursoy, B., and Sarandöl, E. (2007). The impact of ω-3 fatty acids, vitamins E and C supplementation on treatment outcome and side effects in schizophrenia patients treated with haloperidol: an open-label pilot study. Prog. Neuropsychopharmacol. Biol. Psychiatry 31, 1493–1499. doi: 10.1016/j.pnpbp.2007.07.004
Sklar, P., Gabriel, S. B., McInnis, M. G., Bennett, P., Lim, Y.-M., Tsan, G., et al. (2002). Family-based association study of 76 candidate genes in bipolar disorder: BDNF is a potential risk locus. Mol. Psychiatry 7, 579–593. doi: 10.1038/sj.mp.4001058
Sklar, P., Ripke, S., Scott, L. J., Andreassen, O. A., Cichon, S., Craddock, N., et al. (2011). Large-scale genome-wide association analysis of bipolar disorder identifies a new susceptibility locus near ODZ4. Nat. Genet. 43, 977–983. doi: 10.1038/ng.943
Sohal, V. S., and Rubenstein, J. L. R. (2019). Excitation-inhibition balance as a framework for investigating mechanisms in neuropsychiatric disorders. Mol. Psychiatry 24, 1248–1257. doi: 10.1038/s41380-019-0426-0
Song, X., Zhao, Y., Narcisse, L., Duffy, H., Kress, Y., Lee, S., et al. (2005). Canonical transient receptor potential channel 4 (TRPC4) co-localizes with the scaffolding protein ZO-1 in human fetal astrocytes in culture. Glia 49, 418–429. doi: 10.1002/glia.20128
Stokes, A., Wakano, C., Koblan-Huberson, M., Adra, C. N., Fleig, A., and Turner, H. (2006). TRPA1 is a substrate for de-ubiquitination by the tumor suppressor CYLD. Cell. Signal. 18, 1584–1594. doi: 10.1016/j.cellsig.2005.12.009
Straub, R. E., Lehner, T., Luo, Y., Loth, J. E., Shao, W., Sharpe, L., et al. (1994). A possible vulnerability locus for bipolar affective disorder on chromosome 21q22.3. Nat. Genet. 8, 291–296. doi: 10.1038/ng1194-291
Sun, B., Bang, S.-I., and Jin, Y.-H. (2009). Transient receptor potential A1 increase glutamate release on brain stem neurons. NeuroReport 20, 1002–1006. doi: 10.1097/WNR.0b013e32832d2219
Takahashi, N., Chen, H.-Y., Harris, I. S., Stover, D. G., Selfors, L. M., Bronson, R. T., et al. (2018). Cancer cells co-opt the neuronal redox-sensing channel TRPA1 to promote oxidative-stress tolerance. Cancer Cell 33, 985.e7–1003.e7. doi: 10.1016/j.ccell.2018.05.001
Takahashi, N., Kozai, D., Kobayashi, R., Ebert, M., and Mori, Y. (2011a). Roles of TRPM2 in oxidative stress. Cell Calcium 50, 279–287. doi: 10.1016/j.ceca.2011.04.006
Takahashi, N., Kuwaki, T., Kiyonaka, S., Numata, T., Kozai, D., Mizuno, Y., et al. (2011b). TRPA1 underlies a sensing mechanism for O2. Nat. Chem. Biol. 7, 701–711. doi: 10.1038/nchembio.640
Takahashi, N., Mizuno, Y., Kozai, D., Yamamoto, S., Kiyonaka, S., Shibata, T., et al. (2008). Molecular characterization of TRPA1 channel activation by cysteine-reactive inflammatory mediators. Channels 2, 287–298. doi: 10.4161/chan.2.4.6745
Takahashi, N., and Mori, Y. (2011). TRP channels as sensors and signal integrators of redox status changes. Front. Pharmacol. 2:58. doi: 10.3389/fphar.2011.00058
Takizawa, M., Harada, K., Nakamura, K., and Tsuboi, T. (2018). Transient receptor potential ankyrin 1 channels are involved in spontaneous peptide hormone release from astrocytes. Biochem. Biophys. Res. Commun. 501, 988–995. doi: 10.1016/j.bbrc.2018.05.097
Tan, B. L., Norhaizan, M. E., Liew, W.-P.-P., and Sulaiman Rahman, H. (2018). Antioxidant and oxidative stress: a mutual interplay in age-related diseases. Front. Pharmacol. 9:1162. doi: 10.3389/fphar.2018.01162
Togashi, K., Hara, Y., Tominaga, T., Higashi, T., Konishi, Y., Mori, Y., et al. (2006). TRPM2 activation by cyclic ADP-ribose at body temperature is involved in insulin secretion. EMBO J. 25, 1804–1815. doi: 10.1038/sj.emboj.7601083
Uchida, K., Dezaki, K., Damdindorj, B., Inada, H., Shiuchi, T., Mori, Y., et al. (2011). Lack of TRPM2 impaired insulin secretion and glucose metabolisms in mice. Diabetes Metab. Res. Rev. 60, 119–126. doi: 10.2337/db10-0276
Uchiyama, M., Nakao, A., Kurita, Y., Fukushi, I., Takeda, K., Numata, T., et al. (2020). O2-dependent protein internalization underlies astrocytic sensing of acute hypoxia by restricting multimodal TRPA1 channel responses. Curr. Biol. 30, 3378.e7–3396.e7. doi: 10.1016/j.cub.2020.06.047
Voets, T., Talavera, K., Owsianik, G., and Nilius, B. (2005). Sensing with TRP channels. Nat. Chem. Biol. 1, 85–92. doi: 10.1038/nchembio0705-85
Wang, G. X., and Poo, M. (2005). Requirement of TRPC channels in netrin-1-induced chemotropic turning of nerve growth cones. Nature 434, 898–904. doi: 10.1038/nature03478
Wang, S., Zhang, A.-P., Kurada, L., Matsui, T., and Lei, S. (2011). Cholecystokinin facilitates neuronal excitability in the entorhinal cortex via activation of TRPC-like channels. J. Neurophysiol. 106, 1515–1524. doi: 10.1152/jn.00025.2011
White, J. A., McKinney, B. C., John, M. C., Powers, P. A., Kamp, T. J., and Murphy, G. G. (2008). Conditional forebrain deletion of the L-type calcium channel CaV1.2 disrupts remote spatial memories in mice. Learn. Mem. 15, 1–5. doi: 10.1101/lm.773208
Xie, Y.-F., Belrose, J. C., Lei, G., Tymianski, M., Mori, Y., MacDonald, J. F., et al. (2011). Dependence of NMDA/GSK-3β Mediated Metaplasticity on TRPM2 Channels at Hippocampal CA3-CA1 Synapses. Mol. Brain 4:44. doi: 10.1186/1756-6606-4-44
Xu, S.-Z., Sukumar, P., Zeng, F., Li, J., Jairaman, A., English, A., et al. (2008). TRPC channel activation by extracellular thioredoxin. Nature 451, 69–72. doi: 10.1038/nature06414
Yamamoto, S., Shimizu, S., Kiyonaka, S., Takahashi, N., Wajima, T., Hara, Y., et al. (2008). TRPM2-mediated Ca2 + influx induces chemokine production in monocytes that aggravates inflammatory neutrophil infiltration. Nat. Med. 14, 738–747. doi: 10.1038/nm1758
Yan, H.-D., Villalobos, C., and Andrade, R. (2009). TRPC channels mediate a muscarinic receptor-induced afterdepolarization in cerebral cortex. J. Neurosci. 29, 10038–10046. doi: 10.1523/JNEUROSCI.1042-09.2009
Yang, L.-P., Jiang, F.-J., Wu, G.-S., Deng, K., Wen, M., Zhou, X., et al. (2015). Acute Treatment with a Novel TRPC4/C5 channel inhibitor produces antidepressant and anxiolytic-like effects in mice. PLoS One 10:e0136255. doi: 10.1371/journal.pone.0136255
Yokoyama, T., Ohbuchi, T., Saito, T., Sudo, Y., Fujihara, H., Minami, K., et al. (2011). Allyl isothiocyanates and cinnamaldehyde potentiate miniature excitatory postsynaptic inputs in the supraoptic nucleus in rats. Eur. J. Pharmacol. 655, 31–37. doi: 10.1016/j.ejphar.2011.01.011
Yoshida, T., Inoue, R., Morii, T., Takahashi, N., Yamamoto, S., Hara, Y., et al. (2006). Nitric oxide activates TRP channels by cysteine S-nitrosylation. Nat. Chem. Biol. 2, 596–607. doi: 10.1038/nchembio821
Zamponi, G. W., Striessnig, J., Koschak, A., and Dolphin, A. C. (2015). The physiology, pathology, and pharmacology of voltage-gated calcium channels and their future therapeutic potential. Pharmacol. Rev. 67, 821–870. doi: 10.1124/pr.114.009654
Keywords: oxidative stress, Ca2+ signaling, TRP channels, behavior, psychiatric disorders
Citation: Nakao A, Matsunaga Y, Hayashida K and Takahashi N (2021) Role of Oxidative Stress and Ca2+ Signaling in Psychiatric Disorders. Front. Cell Dev. Biol. 9:615569. doi: 10.3389/fcell.2021.615569
Received: 09 October 2020; Accepted: 25 January 2021;
Published: 11 February 2021.
Edited by:
Sandra Derouiche, National Institute for Physiological Sciences (NIPS), JapanCopyright © 2021 Nakao, Matsunaga, Hayashida and Takahashi. This is an open-access article distributed under the terms of the Creative Commons Attribution License (CC BY). The use, distribution or reproduction in other forums is permitted, provided the original author(s) and the copyright owner(s) are credited and that the original publication in this journal is cited, in accordance with accepted academic practice. No use, distribution or reproduction is permitted which does not comply with these terms.
*Correspondence: Akito Nakao, bmFrYW9Ac2JjaGVtLmt5b3RvLXUuYWMuanA=; Nobuaki Takahashi, dGFrYWhhc2hpQHNiY2hlbS5reW90by11LmFjLmpw