- 1INSERM UMRS-MD 1197, Institut de Recherche Biomédicale des Armées (IRBA), Clamart, France
- 2INSERM UMRS-MD 1197, Université Paris-Saclay, Hôpital Paul Brousse, Villejuif, France
- 3Mater Research Institute—The University of Queensland, Woolloongabba, QLD, Australia
- 4INSERM U1179, Université de Versailles Saint-Quentin-en-Yvelines (UVSQ), Versailles, France
Hematopoiesis and bone interact in various developmental and pathological processes. Neurogenic heterotopic ossifications (NHO) are the formation of ectopic hematopoietic bones in peri-articular muscles that develop following severe lesions of the central nervous system such as traumatic cerebral or spinal injuries or strokes. This review will focus on the hematopoietic facet of NHO. The characterization of NHO demonstrates the presence of hematopoietic marrow in which quiescent hematopoietic stem cells (HSC) are maintained by a functional stromal microenvironment, thus documenting that NHOs are neo-formed ectopic HSC niches. Similarly to adult bone marrow, the NHO permissive environment supports HSC maintenance, proliferation and differentiation through bidirectional signaling with mesenchymal stromal cells and endothelial cells, involving cell adhesion molecules, membrane-bound growth factors, hormones, and secreted matrix proteins. The participation of the nervous system, macrophages and inflammatory cytokines including oncostatin M and transforming growth factor (TGF)-β in this process, reveals how neural circuitry fine-tunes the inflammatory response to generate hematopoietic bones in injured muscles. The localization of NHOs in the peri-articular muscle environment also suggests a role of muscle mesenchymal cells and bone metabolism in development of hematopoiesis in adults. Little is known about the establishment of bone marrow niches and the regulation of HSC cycling during fetal development. Similarities between NHO and development of fetal bones make NHOs an interesting model to study the establishment of bone marrow hematopoiesis during development. Conversely, identification of stage-specific factors that specify HSC developmental state during fetal bone development will give more mechanistic insights into NHO.
Introduction
Heterotopic ossification (HO) is an abnormal development of bone tissue within soft tissue. HO can be hereditary such as Fibrodysplasia Ossificans Progressiva (FOP) or acquired following traumatic injuries and burns (Meyers et al., 2019). Among acquired HO, neurogenic heterotopic ossifications (NHO) are pathological formations of ectopic bones in peri-articular muscles following severe central nervous system (CNS) lesions such as traumatic brain injuries (TBI), stroke, cerebral anoxia or spinal cord injuries (SCI) (Genêt et al., 2011). NHOs develop near or around the hip, knee, elbow, and shoulder causing decreased range of motion which can extend to complete joint ankylosis, severe pain, nerve and vessel compression as it grows (Brady et al., 2018; Figure 1A). Large NHOs hamper functional recovery after CNS lesion and interfere with the rehabilitation program delaying potential neurological recovery (van Kampen et al., 2011). NHO incidence ranges from 10 to 23% in TBI patients, 10–53% in SCI patients (Garland, 1988; Brady et al., 2018) and up to 65% following blast injuries (Potter et al., 2007; Forsberg et al., 2009). The only curative option is surgical excision, but surgery remains challenging, especially when NHO entraps the affected joint, as well as proximal vessels and nerves.
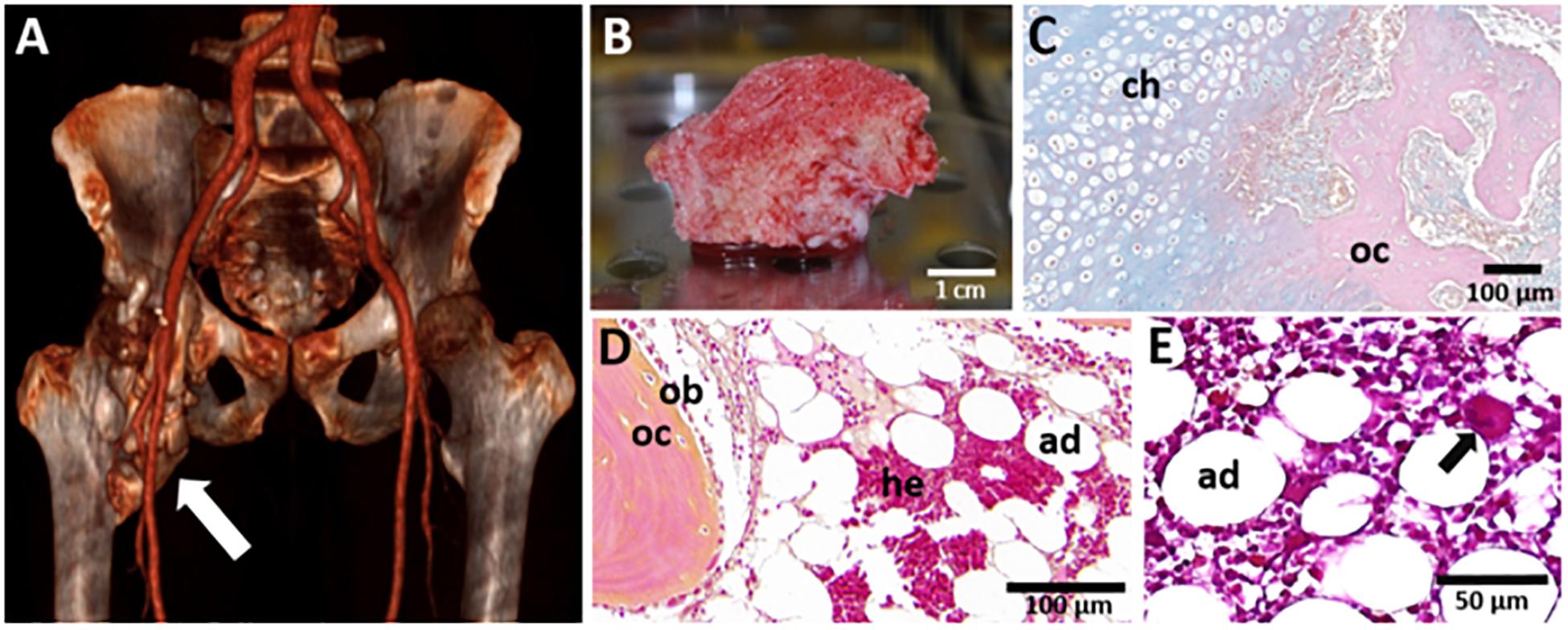
Figure 1. Macroscopic and microscopic views of NHO. (A) 3D scan of a NHO (white arrow) located in the right hip of a patient; (B) NHO biopsy resected during surgery displaying vascularized medullary cavities (scale bar 1 cm); (C) Alcian Blue-Nuclear Red staining of an NHO section showing the endochondral ossification process with the presence of chondrocytes (ch) and osteocytes (oc) (scale bar 100 μm). Hematoxylin-Phloxine-Safran staining of an NHO section showing the presence of (D) osteoblasts (ob), osteocytes (oc), and medullar cavities displaying adipocytes (ad), hematopoietic cells (he) (scale bar 100 μm) and (E) a megakaryocyte (black arrow) (scale bar 50 μm).
Despite identifying NHOs in World War I injured soldiers (Dejerine and Ceillier, 1918), their pathogenesis is still poorly understood. Since most of the previous studies in SCI/TBI patients were retrospective, only a clinically relevant animal model could provide insights into the early events of NHO pathogenesis. Until recently there was no animal model that included the CNS lesions (Brady et al., 2018). Most models of heterotopic ossification (HO) were based on the activation of bone morphogenetic proteins (BMPs) pathway or a constitutively active ACVR1 receptor mutations as found in FOP, an extremely rare genetic disease caused by activating point mutations of the ACVR1 gene encoding a type I BMP receptor (Kan et al., 2004; Shore et al., 2006; Chakkalakal et al., 2012). The relevance of these models to NHO is questionable because NHOs develop in a broad range of ethnicities in subjects otherwise genetically normal. To fill this knowledge gap, we established the first mouse model of NHO (Genêt et al., 2015). As NHO prevalence is high in multi-traumatic combat casualties (Forsberg et al., 2009; Citak et al., 2012), our model combines a SCI with muscle damage induced by an intramuscular injection of cardiotoxin. NHO only develops in the injured muscle when SCI is associated to the muscle injury, suggesting that it requires a dual insult (Alexander et al., 2020).
In NHO located near the hip, one of the most striking features is the presence of richly vascularized mature trabecular bones which contain medullary cavities filled with hematopoietic cells (HCs) (Davis et al., 2013; Torossian et al., 2017; Figure 1B). The pathological formation of an ectopic bone containing a hematopoietic bone marrow tissue in the adult is of foremost interest and the underlying mechanisms are yet to be fully elucidated. This review focuses on how animal models and studies performed with patient-derived cells can help further understand two key events of the establishment of ectopic hematopoiesis in NHO: the occurrence of a heterotopic ossification within skeletal muscle tissue, and the development of a functional hematopoiesis tissue within this heterotopic bone.
Fibro-Adipogenic Progenitors and Altered Muscle Environment, Two Major Players in the Pathogenesis of NHO
It is currently admitted that NHOs are the result of an endochondral ossification process although intramembranous ossification has also been suggested (Cipriano et al., 2009; Cholok et al., 2018). Foley et al. have highlighted the presence of chondrocytes and a cartilaginous matrix on NHO biopsies and pinpointed different stages of NHO development including lymphocytic infiltration, fibro-proliferation, neovascularization, cartilage formation, and endochondral bone formation (Foley et al., 2018). A similar thick cartilaginous matrix displaying chondrocytes adjacent to cancellous bone and marrow is described in NHO 3–4 months after initial injury and becomes thinner at a later stage of NHO development named the “maturation” stage (Wang et al., 2018). Our group and others have evidenced the presence of hematopoietic sites associated with chondrocytes, osteoblasts/osteocytes, and adipocytes in mature trabecular bone in human NHO biopsies (Davis et al., 2013; Torossian et al., 2017; Figures 1C,D). Thus, the progressive formation of a cartilage intermediate maturing into a mineralized bone matrix associated with a vascularization network offers a suitable environment for the recruitment and homing of circulating HCs (Figure 1E; Chan et al., 2009; Kollet et al., 2012).
Muscle Fibro-Adipogenic Progenitors: The Cells-of-Origin of NHO?
The development of heterotopic bones in muscles after severe CNS trauma raises interesting stem cell biology questions particularly regarding the cells-of-origin of NHO. Adult skeletal muscles contain two major types of progenitor cells participating in muscle regeneration. Myogenic satellite cells (SCs) are CD56 expressing stem cells located between the basal lamina and myofiber plasma membrane. To regenerate damaged myofibers, activated SCs proliferate, differentiate into myoblasts and fuse to form multinucleated myofibers with the support of macrophages, endothelial cells (ECs), fibroblasts and pericytes (see reviews: Collins et al., 2005; Bentzinger et al., 2013). On the other hand, fibro-adipogenic progenitors (FAPs) are interstitial mesenchymal stromal cells (MSCs) expressing platelet-derived growth factor receptor α (PDGFRα). FAPs provide growth factors and extracellular matrix to support SC proliferation and myogenic differentiation (see review: Joe et al., 2010; Wosczyna and Rando, 2018).
The cellular origin of NHO is still under investigation but numerous arguments incriminate FAPs rather than SCs. Both human SCs and FAPs show a capacity of osteoblastic differentiation in vitro, however, only PDGFRα+ FAPs maintained their osteogenic capacity in an in vivo ectopic bone model in immune-deficient mice (Oishi et al., 2013). In a burn injury/tenotomy mouse model, parabiosis experiments highlighted the involvement of circulating PDGFRα+ FAPs in the development of burn-induced HO in tendons (Loder et al., 2018). Another study combining parabiosis and a BMP-2-induced HO mouse model reported an abnormal accumulation PDGFRα+ FAPs associated with an in vivo osteogenic potential, although no circulating FAPs were detected (Eisner et al., 2020). In lineage-tracing experiments in which either SCs (via the endogenous Pax7 gene promotor) or FAPs (via a Prrx1 gene enhancer transgene) are specifically labeled, we find that following SCI, NHO are derived from Prrx1 expressing FAPs, not from Pax7 expressing SCs (Tseng et al., 2019).
The involvement of pericytes in HO remains debated (Matthews et al., 2016; Dey et al., 2017). Interestingly, a pericyte population expressing Glast was identified in a BMP-4-induced HO model with a subset co-expressing PDGFRα (Kan et al., 2013). Scleraxis (Scx)+ PDGFRα+ tenocytes are also involved in HO development in tendons using burn/tenotomy and BMP-induced mouse models and represent another interesting lead (Agarwal et al., 2017; Giordani et al., 2019). However, this model of burn-induced calcifying tendinopathy may be different from intramuscular NHO.
Hypoxia and Inflammation as Drivers of NHO Development in Skeletal Muscles?
The molecular microenvironment of the muscle can dramatically affect the behavior and fate of SCs and FAPs (Malecova et al., 2018). A hypoxic microenvironment, mainly linked to inflammation and vascular damage, is an initiator and driver of ossification in acquired HO and FOP mouse models through the activation of Hypoxia Inducible Factor-1α (Agarwal et al., 2016; Wang et al., 2016). The local or systemic production of inflammatory mediators that stimulate the recruitment of MSCs, endothelial progenitors or other stem cells from the bone marrow and alter tissue repair have been proposed to provide a microenvironment/matrix supporting mineralization (Wang et al., 2004; Davis et al., 2013). Signaling molecules including BMPs and TGF-β could contribute in this altered microenvironment (Dey et al., 2017; Wang et al., 2018). Mononucleated phagocytes recruited in the injured muscle play a key role, as treatment with clodronate loaded liposomes, which deplete phagocytes in vivo, abolished NHO onset (Genêt et al., 2015). In contrast, polymorphonuclear (neutrophils) and polynucleated (osteoclasts) phagocytes are not necessary for NHO development (Genêt et al., 2015; Tseng et al., 2020). SCI exacerbates macrophage infiltration into injured muscles with increased and persistent expression of oncostatin M (OSM) (Torossian et al., 2017), a cytokine participating in both inflammation and hematopoiesis (Tanaka and Miyajima, 2003; Stawski and Trojanowska, 2019). The persistent OSM expression in injured muscles was associated with a constant activation of JAK1/2-STAT3 signaling pathway in muscles developing NHO (Alexander et al., 2019). Conversely, NHO development was attenuated in OSM receptor deficient mice (Torossian et al., 2017) or after inhibition of JAK1/2-STAT3 signaling with the small JAK1/2 tyrosine kinase inhibitor ruxolitinib (Alexander et al., 2019). Likewise, over secretion of TGF-β1 by myeloid cells via CD47 in response to extended body burns has been shown to promote burn-induced HO development (Wang et al., 2018). The increased prevalence of NHO in SCI/TBI patients with infections or concomitant inflammation (Hendricks et al., 2007; Citak et al., 2012; Reznik et al., 2014) as well as the occurrence of peri-articular HO in mechanically ventilated and immobilized severe cases of COVID-19 further support the crucial role of inflammation in this process (Meyer et al., 2020; de l’Escalopier et al., 2021; Stoira et al., 2021).
Overall, these data provide a mechanistic link between persistent inflammation driving FAPs into an osteogenic fate and NHO development. Interestingly, histological sections of these ectopic bones reveal the presence of hematopoietic cells suggesting causality between abnormal FAP activation, inflammation and hematopoiesis within an osteogenic muscle microenvironment (Figure 2).
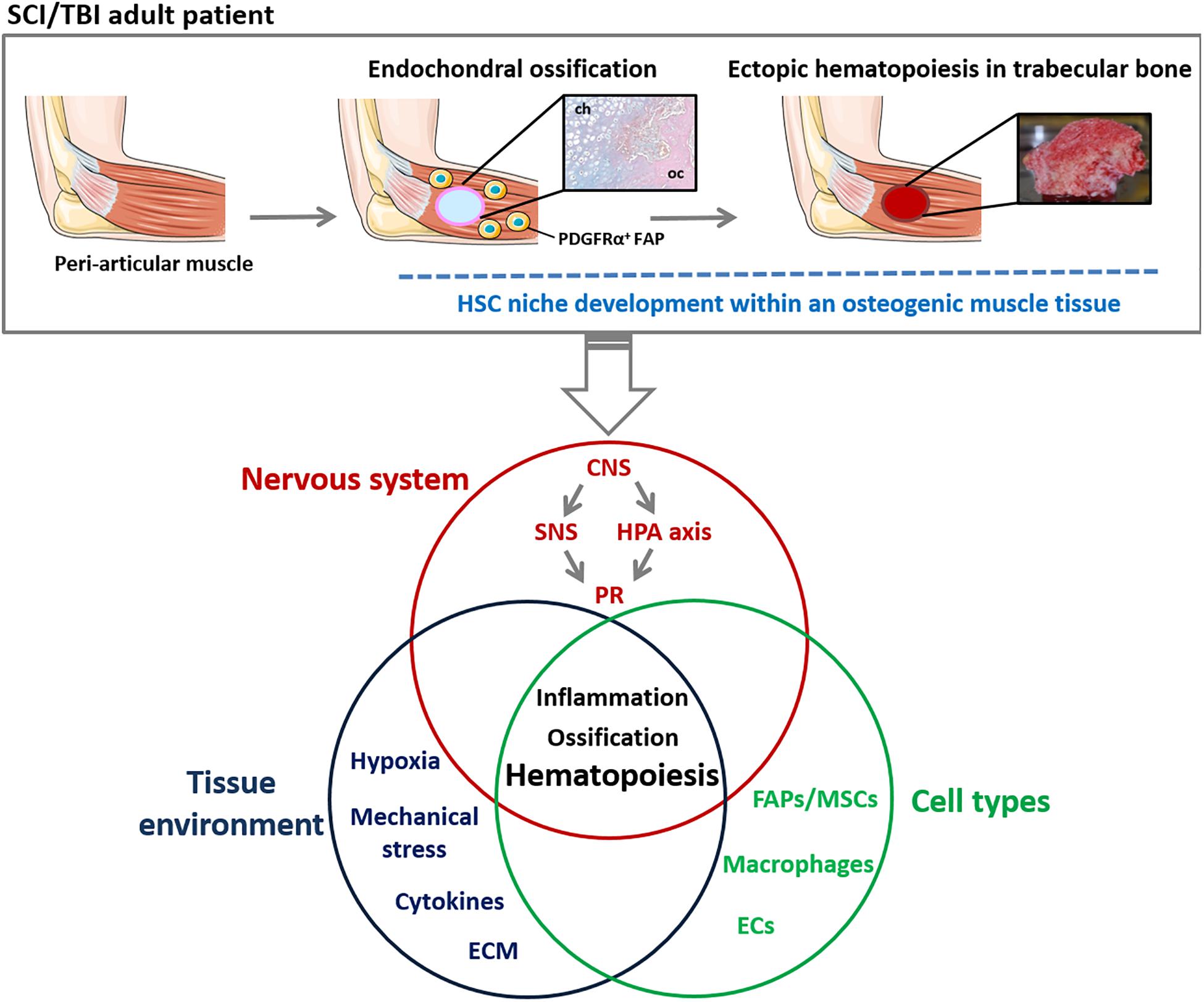
Figure 2. Schematic representation of mechanisms involved in ectopic hematopoietic bone development in NHO pathology. NHOs are the results of an endochondral ossification within peri-articular muscles of TBI/SCI adult patients. NHOs are characterized by the formation of a trabecular bone tissue housing HSC niches. A wide range of regulatory mechanisms are suggested to be involved in NHO pathogenesis including altered neuronal control, inflammation, macrophages and profound changes in muscle tissue environment. The singular localization of NHOs suggests a role of muscle-resident mesenchymal cells such as PDGFRα+ FAPs and bone metabolism in the development of ectopic hematopoiesis in adults. CNS, central nervous system; ECM, extra-cellular matrix; ECs, endothelial cells; FAP, fibro-adipogenic progenitor; HPA, hypothalamic-pituitary-adrenal; HSC, Hematopoietic stem cells; MSC, mesenchymal stromal cells; NHO, neurogenic heterotopic ossification; PR, peripheral response; SCI, spinal cord injury; SNS, sympathetic nervous system; TBI, traumatic brain injury.
CNS Lesion and NHO Development
Both peripheral (denervation) and central (SCI) neurologic lesions have an effect on FAPs, inducing STAT3 activation, high IL-6 secretory activity and abnormal proliferation (Madaro et al., 2018). Furthermore, CNS lesions deregulate the neuro-endocrine system causing the abnormal systemic release of a number of mediators that may trigger NHO development such as substance P (SP) or TGF-β (Genêt et al., 2015). High CNS lesions can also cause autonomic dysreflexia (AD), a life-threatening complication caused by the loss of the central control of the post-ganglionic sympathetic flow below the SCI. In retrospective studies, AD has been associated with higher prevalence of NHO in SCI and TBI patients (Hendricks et al., 2007; van Kampen et al., 2011; Putz et al., 2014). AD causes a major physiological challenge with high norepinephrine release, extreme hypertension combined with bradycardia. Whether any of the systemic drivers of AD lead to NHO development remain unexplored (Alexander et al., 2020). Severe CNS trauma also profoundly changes muscle spasticity and physical environment. The resulting mechanical stress and downstream mechano-transduction signals could facilitate NHO development because of their known effect on MSC osteogenic fate (Frith et al., 2018; Sun et al., 2018). Evidence of an abnormal activation of mechano-transductive effectors Rho/ROCK and YAP1 has recently been reported in a FOP model (Stanley et al., 2019). More interestingly, Daud et al. (1993) have shown that delayed start of passive movements to paralyzed limbs in SCI patients correlates with increased NHO occurrence.
NHO, a Favorable Environment for the Development of Hematopoietic Stem Cell Niches in Adults
The presence of ectopic hematopoietic bones developing in muscles following CNS injuries is puzzling for the hematologist since, in adults, hematopoiesis is physiologically restricted to the BM of skeletal bones.
During fetal development, blood formation occurs in discrete anatomical extraembryonic and intraembryonic niches, generating different hematopoietic cell (HC) types (Dzierzak and Speck, 2008; Waas and Maillard, 2017). First HCs emerge in the yolk sac (YS) and generate primitive erythroblasts, macrophages and megakaryocytes. A second wave of erythro-myeloid progenitors also derived from the YS gives rise to definitive erythroid, megakaryocyte, myeloid, and multipotent progenitors initiating fetal liver (FL) hematopoiesis (Luckett, 1978; Silver and Palis, 1997). Bona fide definitive HSCs emerge by budding from specialized ECs, known as hemogenic endothelium, in the dorsal aorta, vitelline and umbilical arteries (Oberlin et al., 2002, 2010; Boisset et al., 2010). These definitive HSCs then migrate to the FL where they undergo significant proliferation (Rybtsov et al., 2016; Zhang et al., 2019) and finally reach the fetal bones and especially the BM, their life-long residence, where they become predominantly quiescent in adults (Bowie et al., 2007; Copley and Eaves, 2013). In adults, BM HSCs act as a reserve for the blood system, remaining dormant for months or years, and yet can rapidly proliferate when needed following inflammatory or cytotoxic/radiotoxic challenges (Wilson et al., 2008; Batsivari et al., 2020). Apart from some pathological situations, the BM will remain the only hematopoietic tissue in healthy adults.
HSC Niches and Their Main Players Along Development and in Adult BM
Schofield first used the term “niche” to describe a putative HSC-specific environment in the BM that “preserved the reconstituting ability of stem cells” (Schofield, 1978). Successive specialized niches were identified during development, from the emergence of functional HSCs in the dorsal aorta, amplification in the FL and homeostasis in the adult BM (Gao et al., 2018; Daniel et al., 2020). The formation of the BM niche during fetal development has not been fully investigated, and the impact of specific niche components on fetal BM HSC phenotype, proliferation and function has still to be deciphered. In the adult, the precise nature of BM HSC niches has long been debated (Morrison and Scadden, 2014). The presence of two anatomically different niches was initially suggested: the central and the endosteal niches. Such a distinction is more and more challenged since HSCs tend to be more frequent in perivascular areas of the BM, in close proximity to ECs and perivascular stromal cells (Kokkaliaris et al., 2020) that are particularly numerous in the endosteal region (Nombela-Arrieta et al., 2013).
Within these niches, HSCs are maintained quiescent by a complex molecular interplay between cells from mesenchymal origin, ECs, neuronal cells and HSC progenies, such as megakaryocytes and macrophages. Diffusible factors including inflammatory cytokines and extra-cellular matrix components perfect this molecular network, subtly controlling the fate of HSCs (Méndez-Ferrer et al., 2020).
Apart from stromal cells, macrophages are essential to HSC regulation within niches. They are the most abundant HCs in the dorsal aorta when the number of intra-aortic hematopoietic cluster peaks, and are suggested to promote definitive HSC formation from the dorsal aorta hemogenic endothelium through pro-inflammatory signaling cascades (Yokomizo and Dzierzak, 2010). Among these pro-inflammatory signals (Luis et al., 2016; Hayashi et al., 2019), tumor necrosis factor (TNF) (Espín-Palazón et al., 2014), interferons (IFN) (Sawamiphak et al., 2014), IL-1 (Orelio et al., 2009), and OSM (Miyajima et al., 2000) play a major role in the regulation of embryonic and fetal hematopoiesis. Macrophages are also initiators of the endothelial-to-hematopoietic transition since hemogenic ECs that receive these cues, undergo endothelial-hematopoietic transition and form HSCs (Mariani et al., 2019). In adult hematopoiesis, macrophages exert several other functions in the BM niches. They participate in the retention of HSCs through their interactions with MSCs and possibly ECs and the modulation of the expression of proteins such as CXCL12 (Winkler et al., 2010), VCAM-1 and KIT ligand. Furthermore, supraphysiological expansion of the monocyte/macrophage compartment by prolonged administration of a stable recombinant form of macrophage colony-stimulating factor (CSF-1) expand the HSC compartment in the BM (Kaur et al., 2021). Reciprocally, BM-resident macrophages are necessary to reconstitute HSC niches after lethal irradiation and support HSC engraftment (Kaur et al., 2017, 2018). The stromal expression of CXCL12 follows a circadian regulation that is under the control of sympathetic nerve fibers, connecting macrophages and the nervous system in the regulation of HSC trafficking (Katayama et al., 2006; Méndez-Ferrer et al., 2020). In the context of infections or inflammatory stresses, macrophages and ECs produce G-CSF that participates in the mobilization of HSCs from the BM into the circulation while promoting myelopoiesis. G-CSF also contributes in suppressing osteoblast function by directly down-regulating CXCL12 expression in the endosteal niche or by indirect mechanisms including signals from the sympathetic nervous system (SNS) (Winkler et al., 2010; Christopher et al., 2011) (for review: Mitroulis et al., 2020).
Neuronal and Neuroendocrine Regulation of Bone and HSC Niche
In the context of NHO, it is noteworthy to integrate the role of the nervous system as an important regulator of bone remodeling and hematopoiesis homeostasis. Since the discovery of skeleton innervation by Calvo (1968), other groups including that of Paul Frenette have further explored from this pioneering observation and described the neuronal regulation of bone and BM (see for review: Maryanovich et al., 2018). In addition to a mineral constituent regulation, bone homeostasis is controlled by long-range signals such as leptin, glucocorticoids and parathyroid hormone produced by the adipose tissue, the adrenal glands, and the parathyroid glands, respectively, and by signals originating from the nervous system.
Besides its role in energy homeostasis, leptin plays a major role in neuroendocrine regulation and bone metabolism. The expression of leptin receptor on adult MSCs, osteoblasts and chondrocytes, suggests direct effects on bone growth and metabolism. Leptin can also indirectly modulate bone formation through effectors downstream of the hypothalamus such as estrogen, cortisol, IGF-1 and parathyroid hormone, and through activation of local adrenergic signaling at the osteoblast level via β2 adrenergic receptors (AR) (Upadhyay et al., 2015; Wang et al., 2020 for review). Leptin also inhibits the neuronal activity of serotonergic neurons and decrease brain-derived serotonin synthesis (see for review: Maryanovich et al., 2018; Karsenty, 2020). Sensory and sympathetic nerves also participate in bone homeostasis through neurotransmitters including nerve growth factor (NGF), calcitonin gene-related peptide (CGRP), SP, and semaphorin 3A and through norepinephrine/noradrenaline released by the SNS (Wang et al., 2020).
As reported above, HSCs are mainly located in perivascular areas of the adult BM, comprising both sinusoidal and arteriolar blood vessels. The arteriolar structures are highly innervated by SNS fibers. The neuroreticular complex formed by SNS nerves and perivascular MSCs has been reported to be a central regulator of HSC quiescence within BM niches (see for review: Maryanovich et al., 2018). Interestingly, a variable proportion of these perivascular stromal cells expressed neural-related markers such as LepR, NG2, and Nestin.
The close relationship between CNS, SNS, Parasympathetic Nervous System, bone metabolism and HSC migration, differentiation and self-renewal is illustrated by the important role of the circadian norepinephrine release by SNS nerves which triggers β3 AR and β2 AR expressed by BM mesenchymal cells and osteoblasts in both humans and mice (Golan et al., 2018, 2019). Cholinergic signaling in the BM via neurons from both the PNS and SNS in tandem with adrenergic signaling derived from the SNS is also reported to contribute to regulate the circadian regulation of CXCL12 expression in the BM (García-García et al., 2019; García-García and Méndez-Ferrer, 2020). Likewise, the CNS exerts long range regulation of HSCs. For instance, muscarinic type 1 acetylcholinergic receptors in the brain regulate HSC mobilizing response by stimulating the hypothalamic-pituitary-adrenal (HPA) axis and glucocorticoid secretion (Pierce et al., 2017). Similarly, afferent sensory nociceptive nerves in the BM have been found to regulate HSC expansion, differentiation and mobilization in concert with SNS nerves via CGRP release (Gao et al., 2020).
Beside its role in adult stem cell niche homeostasis, the neural system is also an early regulator of the embryonic niches when stem cells are specified. Apart from a direct innervation of the HSC niche, recent findings show different modes of neural control, including systemic delivery of CNS-derived hormones, locally by neural crest-derived MSCs, and intrinsically by HCs expressing neural receptors and neurotransmitters (Fitch et al., 2012; Damm and Clements, 2017). During development, hypoxia-induced neuronal synthesis of serotonin is a key process for embryonic HSC production in the aorta region. Neuronal serotonin activates the HPA axis and glucocorticoid receptor activity, which in turn, induces HSC production (Kwan et al., 2016). Although hypoxia was the only stress-inducing stimulus tested, it is conceivable that other common stresses that the embryo experiences such as temperature, metabolic, or oxidative stress would also promote blood cell formation through the HPA axis. Such mechanisms were also described in adults, where muscarinic acetylcholine receptors in the brain regulate HPA axis and glucocorticoid release by the adrenal glands that impact HSC trafficking (Pierce et al., 2017).
NHO: An Osteogenic Muscle Tissue That Houses HSC Niches!
Addressing whether HOs form bona fide HSC niches is challenging since these ectopic bone tissues develop in inflamed muscles following severe neurological lesions. Few groups have reported the presence of marrow-like tissue in HOs. Histological descriptions are reported after abdominal surgery (Wang et al., 2004; Christofi et al., 2008), aortic valve graft (Lis et al., 2009; Singh and Fleshman, 2011) or cervical spine meniscoid (Farrell et al., 2017). Furthermore, clonogenic hematopoietic progenitors associated with histologically-defined stromal cells have been described in HOs from severe combat-injured orthopedic patients (Davis et al., 2013). However, the evidence for functional HSC niches comes from studies in SCI and TBI patients (Torossian et al., 2017). These studies identified phenotypic CD34+ hematopoietic stem/progenitor cells in NHO marrows. Depending on patients, their level was equivalent or slightly lower than in the healthy BM. Some of those CD34+ cells were quiescent, expressed a side-population phenotype (Goodell, 2005) and were capable of long-term human hematopoietic reconstitution when transplanted into immunodeficient mice, thus meeting the functional definition of HSCs. NHO marrow also contained functional CD45–CD34–CD73+CD90+CD105+ MSCs able to differentiate in osteoblasts, adipocytes and chondrocytes and to support long-term human hematopoiesis in culture. More importantly, when seeded on hydroxyapatite scaffolds and implanted into nude mice, NHO-derived MSCs created a supportive osteogenic microenvironment for murine hematopoiesis (Torossian et al., 2017). In agreement with these results, their transcriptomic signature showed a molecular network required for HSC support. Intriguingly, this signature was associated with a neuronal imprinting, arguing in favor of the brain-bone-blood triad concept proposed by Lapidot (Lapidot and Kollet, 2010). ECs and their progenitors could also be isolated from NHO marrow according to their CD45–CD31+CD144+CD34+ phenotype. They were functional as demonstrated by colony formation on plastic, expansion in culture as a cobblestone monolayer, vascular network development in matrigel and overexpression of VCAM-1 and ICAM-1 after TNFα stimulation (Torossian et al., 2017).
By demonstrating that NHOs contain a marrow tissue in which HSCs can proliferate and differentiate within a suitable and functional osteogenic/mesenchymal and vascular microenvironment, these studies acknowledge that NHO ectopic bones house HSC niches. It is noteworthy that, in NHO patients, the altered neuronal control most likely contributes to the generation of hematopoietic bones comparable to the BM, in muscles. More importantly, the NHO paradigm emphasizes the role of muscle microenvironment and inflammation in their development (Figure 2).
Intriguingly, while the role of central, sympathetic and parasympathetic nervous systems in regulating hematopoiesis in the BM of skeletal bones is well described, it is not known whether the hematopoietic BM of NHOs is actually innervated, and if so, what roles these nerves would play in establishing, maintaining and regulating hematopoiesis in the NHO marrow.
Lessons From NHO for a Better Understanding of HSC Development
Independent studies of vertebrate hematopoietic development (Waas and Maillard, 2017; Dzierzak and Bigas, 2018) and NHO pathogenesis (Davis et al., 2013; Torossian et al., 2017) reveal that embryonic and adult NHO HSCs share similarities. Both develop in soft tissues within niches under the control of regulatory mechanisms including macrophages, inflammation, and the nervous system. However, significant changes in the composition of the HSC pool, as well as in their cell cycling properties and repopulating abilities are observed between the fetal hematopoietic tissues and the adult BM (Copley and Eaves, 2013; Mirshekar-Syahkal et al., 2014).
In Osterix-null (Osx–/–) mice that lack osteoblasts and osteolineage cells, the vasculature within the nascent bones and bone marrow can sustain multilineage proliferative progenitors but not long-term HSCs. As a result, wild-type HSC transplanted in Osx embryos engraft the liver but not the nascent BM. Therefore, interactions with osteoblasts within fetal bone regulate HSC quiescence and homing ability (Coşkun et al., 2014). In the adult BM, the role of osteolineage cells is more questionable since the deletion of Cxcl12 or Kitl gene from Osx+ osteoprogenitors has more effect on hematopoietic progenitors than on proper HSCs (Ding and Morrison, 2013). In contrast, both genes need to be expressed in ECs and in immature Lepr+ MSCs (that form osteoprogenitors) for HSCs to be maintained (Greenbaum et al., 2013). These results emphasize the importance of osteolineage cells, and most likely other cells from mesenchymal origin, in establishing and sustaining HSC phenotype, cell cycling balance and function during development and adult life.
Understanding the development of hematopoiesis in an adult osteogenic muscle environment as observed in NHO could help gain further insights on the role of bone forming cells in this process. Identification of stage-specific factors that orientate HSC developmental state during fetal bone development must be harnessed to gain more mechanistic insights into NHO development. Similarly, understanding the cellular origin of NHO, the role of inflammation and muscle environment might contribute to a better understanding of the impact of specific niche components on fetal BM HSC properties.
Conclusion
In the recent few years, knowledge about NHO pathogenesis has been considerably improved as accredited by the rapidly increasing number of publications in the field. These progresses were mainly due to the development of more suitable animal models and to the availability of patient samples thanks to well organized cohorts. The current review focusing on the hematopoietic features of NHO ossifications, attempts to recapitulate how a favorable environment for the development of bone with HSC niches can develop in adult muscles following central neurological lesions. It emphasizes the role of a persistent inflamed muscle environment driving FAPs to an osteogenic fate initiating the development of ossification followed by the establishment of a mature hematopoietic bone tissue.
However, there are still numerous questions in respect to the molecular mechanisms underlying this complex and multifactorial pathological process. Among those, the potential differences between normal endochondral ossification and neurogenic HO in terms of signaling events, cell type involvement and environment remains unanswered. Likewise, how an inflamed adult muscle environment becomes pro-osteogenic and thereafter hematopoietic, and what is the influence of altered nervous and neuroendocrine systems as well as hypoxia in this process? Does the neoformation of hematopoietic bones in muscles mimic what happens during development and can we learn from NHO for a better identification of stage-specific factors that specify HSC developmental state during fetal bone development? Is the impaired mobility of patients a trigger in the development of NHO and does an early and adequate mobilization of patients can avoid or at least reduce its evolution?
Gathering surgeons, clinicians, specialists in physical medicine/rehabilitation and researchers within a European/International consortium would be a provocative initiative for developing translational collaborative projects to better understand NHO pathogenesis and, armed with this knowledge, enable the identification of new targets to treat and if possible prevent NHO development. Moreover, such knowledge may also provide new insights for cell therapy needs and for improving treatment of blood and bone disorders.
Author Contributions
DG, FT, EO, KA, JG, H-WT, MS, J-PL, M-CL, and SB wrote the manuscript. DG, EO, M-CL, and SB prepared the figures. All authors revised the manuscript and approved the submitted version.
Conflict of Interest
The authors declare that the research was conducted in the absence of any commercial or financial relationships that could be construed as a potential conflict of interest.
Funding
This research was funded by project grant N° 2014 94 0902 and BIOMEDEF SAN-1-0225 from the French defense procurement agency (DGA). KA and H-WT are funded by Ideas Grant 1181053 and J-PL Research Fellowship 1136130 from the National Health and Medical Research Council of Australia.
Acknowledgments
We greatly thank Raphaël Amar, MD and Tristan Thiry, MD (AP-HP, Raymond-Poincaré Hospital, Garches) for providing the 3D scan photo. We also acknowledge the technical assistance of the “HistIM Facility” (Cochin Institute, Paris).
References
Agarwal, S., Loder, S. J., Cholok, D., Peterson, J., Li, J., Breuler, C., et al. (2017). Scleraxis-lineage cells contribute to ectopic bone formation in muscle and tendon. Stem Cells Dayt. Ohio 35, 705–710. doi: 10.1002/stem.2515
Agarwal, S., Loder, S., Brownley, C., Cholok, D., Mangiavini, L., Li, J., et al. (2016). Inhibition of Hif1α prevents both trauma-induced and genetic heterotopic ossification. Proc. Natl. Acad. Sci. U. S. A. 113, E338–E347. doi: 10.1073/pnas.1515397113
Alexander, K. A., Tseng, H.-W., Fleming, W., Jose, B., Salga, M., Kulina, I., et al. (2019). Inhibition of JAK1/2 tyrosine kinases reduces neurogenic heterotopic ossification after spinal cord injury. Front. Immunol. 10:377. doi: 10.3389/fimmu.2019.00377
Alexander, K. A., Tseng, H.-W., Salga, M., Genêt, F., and Levesque, J.-P. (2020). When the nervous system turns skeletal muscles into bones: how to solve the conundrum of neurogenic heterotopic ossification. Curr. Osteoporos. Rep. 18, 666–676. doi: 10.1007/s11914-020-00636-w
Batsivari, A., Haltalli, M. L. R., Passaro, D., Pospori, C., Lo Celso, C., and Bonnet, D. (2020). Dynamic responses of the haematopoietic stem cell niche to diverse stresses. Nat. Cell Biol. 22, 7–17. doi: 10.1038/s41556-019-0444-9
Bentzinger, C. F., Wang, Y. X., Dumont, N. A., and Rudnicki, M. A. (2013). Cellular dynamics in the muscle satellite cell niche. EMBO Rep. 14, 1062–1072. doi: 10.1038/embor.2013.182
Boisset, J.-C., van Cappellen, W., Andrieu-Soler, C., Galjart, N., Dzierzak, E., and Robin, C. (2010). In vivo imaging of haematopoietic cells emerging from the mouse aortic endothelium. Nature 464, 116–120. doi: 10.1038/nature08764
Bowie, M. B., Kent, D. G., Dykstra, B., McKnight, K. D., McCaffrey, L., Hoodless, P. A., et al. (2007). Identification of a new intrinsically timed developmental checkpoint that reprograms key hematopoietic stem cell properties. Proc. Natl. Acad. Sci. U. S. A. 104, 5878–5882. doi: 10.1073/pnas.0700460104
Brady, R. D., Shultz, S. R., McDonald, S. J., and O’Brien, T. J. (2018). Neurological heterotopic ossification: current understanding and future directions. Bone 109, 35–42. doi: 10.1016/j.bone.2017.05.015
Calvo, W. (1968). The innervation of the bone marrow in laboratory animals. Am. J. Anat. 123, 315–328. doi: 10.1002/aja.1001230206
Chakkalakal, S. A., Zhang, D., Culbert, A. L., Convente, M. R., Caron, R. J., Wright, A. C., et al. (2012). An Acvr1 R206H knock-in mouse has fibrodysplasia ossificans progressiva. J. Bone Miner. Res. 27, 1746–1756.
Chan, C. K. F., Chen, C.-C., Luppen, C. A., Kim, J.-B., DeBoer, A. T., Wei, K., et al. (2009). Endochondral ossification is required for haematopoietic stem-cell niche formation. Nature 457, 490–494. doi: 10.1038/nature07547
Cholok, D., Chung, M. T., Ranganathan, K., Ucer, S., Day, D., Davis, T. A., et al. (2018). Heterotopic ossification and the elucidation of pathologic differentiation. Bone 109, 12–21. doi: 10.1016/j.bone.2017.09.019
Christofi, T., Raptis, D. A., Kallis, A., and Ambasakoor, F. (2008). True trilineage haematopoiesis in excised heterotopic ossification from a laparotomy scar: report of a case and literature review. Ann. R. Coll. Surg. Engl. 90, W12–W14. doi: 10.1308/147870808X303056
Christopher, M. J., Rao, M., Liu, F., Woloszynek, J. R., and Link, D. C. (2011). Expression of the G-CSF receptor in monocytic cells is sufficient to mediate hematopoietic progenitor mobilization by G-CSF in mice. J. Exp. Med. 208, 251–260. doi: 10.1084/jem.20101700
Cipriano, C. A., Pill, S. G., and Keenan, M. A. (2009). Heterotopic ossification following traumatic brain injury and spinal cord injury. J. Am. Acad. Orthop. Surg. 17, 689–697. doi: 10.5435/00124635-200911000-00003
Citak, M., Suero, E. M., Backhaus, M., Aach, M., Godry, H., Meindl, R., et al. (2012). Risk factors for heterotopic ossification in patients with spinal cord injury: a case-control study of 264 patients. Spine 37, 1953–1957. doi: 10.1097/BRS.0b013e31825ee81b
Collins, C. A., Olsen, I., Zammit, P. S., Heslop, L., Petrie, A., Partridge, T. A., et al. (2005). Stem cell function, self-renewal, and behavioral heterogeneity of cells from the adult muscle satellite cell niche. Cell 122, 289–301. doi: 10.1016/j.cell.2005.05.010
Copley, M. R., and Eaves, C. J. (2013). Developmental changes in hematopoietic stem cell properties. Exp. Mol. Med. 45:e55. doi: 10.1038/emm.2013.98
Coşkun, S., Chao, H., Vasavada, H., Heydari, K., Gonzales, N., Zhou, X., et al. (2014). Development of the fetal bone marrow niche and regulation of hsc quiescence and homing ability by emerging osteolineage cells. Cell Rep. 9, 581–590. doi: 10.1016/j.celrep.2014.09.013
Damm, E. W., and Clements, W. K. (2017). Pdgf signalling guides neural crest contribution to the haematopoietic stem cell specification niche. Nat. Cell Biol. 19, 457–467. doi: 10.1038/ncb3508
Daniel, M. G., Rapp, K., Schaniel, C., and Moore, K. A. (2020). Induction of developmental hematopoiesis mediated by transcription factors and the hematopoietic microenvironment. Ann. N. Y. Acad. Sci. 1466, 59–72. doi: 10.1111/nyas.14246
Daud, O., Sett, P., Burr, R. G., and Silver, J. R. (1993). The relationship of heterotopic ossification to passive movements in paraplegic patients. Disabil. Rehabil. 15, 114–118. doi: 10.3109/09638289309166001
Davis, T. A., Lazdun, Y., Potter, B. K., and Forsberg, J. A. (2013). Ectopic bone formation in severely combat-injured orthopedic patients – a hematopoietic niche. Bone 56, 119–126. doi: 10.1016/j.bone.2013.05.016
de l’Escalopier, N., Mathieu, L., Duret, C., Banzet, S., Genêt, F., and Salga, M. (2021). Re: “high prevalence of heterotopic ossification in critically ill patients with severe COVID-19” by Stoira et al. Clin. Microbiol. Infect. doi: 10.1016/j.cmi.2020.12.038 Online ahead of print.
Dejerine, A., and Ceillier, A. (1918). Paraosteoarthropathies des paraplégiques par lésion médullaire; Etude clinique et radiographique. Ann. Méd. 5:497.
Dey, D., Wheatley, B. M., Cholok, D., Agarwal, S., Yu, P. B., Levi, B., et al. (2017). The traumatic bone: trauma-induced heterotopic ossification. Transl. Res. J. Lab. Clin. Med. 186, 95–111. doi: 10.1016/j.trsl.2017.06.004
Ding, L., and Morrison, S. J. (2013). Haematopoietic stem cells and early lymphoid progenitors occupy distinct bone marrow niches. Nature 495, 231–235. doi: 10.1038/nature11885
Dzierzak, E., and Bigas, A. (2018). Blood development: hematopoietic stem cell dependence and independence. Cell Stem Cell 22, 639–651. doi: 10.1016/j.stem.2018.04.015
Dzierzak, E., and Speck, N. A. (2008). Of lineage and legacy: the development of mammalian hematopoietic stem cells. Nat. Immunol. 9, 129–136. doi: 10.1038/ni1560
Eisner, C., Cummings, M., Johnston, G., Tung, L. W., Groppa, E., Chang, C., et al. (2020). Murine tissue-resident PDGFRα+ fibro-adipogenic progenitors spontaneously acquire osteogenic phenotype in an altered inflammatory environment. J. Bone Miner. Res. 35, 1525–1534. doi: 10.1002/jbmr.4020
Espín-Palazón, R., Stachura, D. L., Campbell, C. A., García-Moreno, D., Del Cid, N., Kim, A. D., et al. (2014). Proinflammatory signaling regulates hematopoietic stem cell emergence. Cell 159, 1070–1085. doi: 10.1016/j.cell.2014.10.031
Farrell, S. F., Petcu, E. B., Cornwall, J., Rivett, D. A., and Osmotherly, P. G. (2017). An ectopic intramedullary hematopoietic niche in a cervical spine meniscoid: a case report. Vasc. Cell 9:3. doi: 10.24238/13221-9-1-173
Fitch, S. R., Kimber, G. M., Wilson, N. K., Parker, A., Mirshekar-Syahkal, B., Göttgens, B., et al. (2012). Signaling from the sympathetic nervous system regulates hematopoietic stem cell emergence during embryogenesis. Cell Stem Cell 11, 554–566. doi: 10.1016/j.stem.2012.07.002
Foley, K. L., Hebela, N., Keenan, M. A., and Pignolo, R. J. (2018). Histopathology of periarticular non-hereditary heterotopic ossification. Bone 109, 65–70. doi: 10.1016/j.bone.2017.12.006
Forsberg, J. A., Pepek, J. M., Wagner, S., Wilson, K., Flint, J., Andersen, R. C., et al. (2009). Heterotopic ossification in high-energy wartime extremity injuries: prevalence and risk factors. J. Bone Joint Surg. Am. 91, 1084–1091. doi: 10.2106/JBJS.H.00792
Frith, J. E., Kusuma, G. D., Carthew, J., Li, F., Cloonan, N., Gomez, G. A., et al. (2018). Mechanically-sensitive miRNAs bias human mesenchymal stem cell fate via mTOR signalling. Nat. Commun. 9:257. doi: 10.1038/s41467-017-02486-0
Gao, X., Xu, C., Asada, N., and Frenette, P. S. (2018). The hematopoietic stem cell niche: from embryo to adult. Dev. Camb. Engl. 145:dev139691. doi: 10.1242/dev.139691
Gao, X., Zhang, D., Xu, C., Li, H., Caron, K. M., and Frenette, P. S. (2020). Nociceptive nerves regulate haematopoietic stem cell mobilization. Nature 589, 591–596. doi: 10.1038/s41586-020-03057-y
García-García, A., and Méndez-Ferrer, S. (2020). The autonomic nervous system pulls the strings to coordinate circadian HSC functions. Front. Immunol. 11:956. doi: 10.3389/fimmu.2020.00956
García-García, A., Korn, C., García-Fernández, M., Domingues, O., Villadiego, J., Martín-Pérez, D., et al. (2019). Dual cholinergic signals regulate daily migration of hematopoietic stem cells and leukocytes. Blood 133, 224–236. doi: 10.1182/blood-2018-08-867648
Garland, D. E. (1988). Clinical observations on fractures and heterotopic ossification in the spinal cord and traumatic brain injured populations. Clin. Orthop. 233, 86–101. doi: 10.1097/00003086-198808000-00011
Genêt, F., Jourdan, C., Schnitzler, A., Lautridou, C., Guillemot, D., Judet, T., et al. (2011). Troublesome heterotopic ossification after central nervous system damage: a survey of 570 surgeries. PLoS One 6:e16632. doi: 10.1371/journal.pone.0016632
Genêt, F., Kulina, I., Vaquette, C., Torossian, F., Millard, S., Pettit, A. R., et al. (2015). Neurological heterotopic ossification following spinal cord injury is triggered by macrophage-mediated inflammation in muscle. J. Pathol. 236, 229–240. doi: 10.1002/path.4519
Giordani, L., He, G. J., Negroni, E., Sakai, H., Law, J. Y. C., Siu, M. M., et al. (2019). High-dimensional single-cell cartography reveals novel skeletal muscle-resident cell populations. Mol. Cell 74, 609–621.e6. doi: 10.1016/j.molcel.2019.02.026
Golan, K., Kollet, O., Markus, R. P., and Lapidot, T. (2019). Daily light and darkness onset and circadian rhythms metabolically synchronize hematopoietic stem cell differentiation and maintenance: the role of bone marrow norepinephrine, tumor necrosis factor, and melatonin cycles. Exp. Hematol. 78, 1–10. doi: 10.1016/j.exphem.2019.08.008
Golan, K., Kumari, A., Kollet, O., Khatib-Massalha, E., Subramaniam, M. D., Ferreira, Z. S., et al. (2018). Daily onset of light and darkness differentially controls hematopoietic stem cell differentiation and maintenance. Cell Stem Cell 23, 572–585.e7. doi: 10.1016/j.stem.2018.08.002
Goodell, M. A. (2005). Stem cell identification and sorting using the Hoechst 33342 side population (SP). Curr. Protoc. Cytom. 9:Unit9.18. doi: 10.1002/0471142956.cy0918s33
Greenbaum, A., Hsu, Y.-M. S., Day, R. B., Schuettpelz, L. G., Christopher, M. J., Borgerding, J. N., et al. (2013). CXCL12 in early mesenchymal progenitors is required for haematopoietic stem-cell maintenance. Nature 495, 227–230. doi: 10.1038/nature11926
Hayashi, Y., Sezaki, M., and Takizawa, H. (2019). Development of the hematopoietic system: role of inflammatory factors. Wiley Interdiscip. Rev. Dev. Biol. 8:e341. doi: 10.1002/wdev.341
Hendricks, H. T., Geurts, A. C. H., van Ginneken, B. C., Heeren, A. J., and Vos, P. E. (2007). Brain injury severity and autonomic dysregulation accurately predict heterotopic ossification in patients with traumatic brain injury. Clin. Rehabil. 21, 545–553. doi: 10.1177/0269215507075260
Joe, A. W. B., Yi, L., Natarajan, A., Le Grand, F., So, L., Wang, J., et al. (2010). Muscle injury activates resident fibro/adipogenic progenitors that facilitate myogenesis. Nat. Cell Biol. 12, 153–163. doi: 10.1038/ncb2015
Kan, L., Hu, M., Gomes, W. A., and Kessler, J. A. (2004). Transgenic mice overexpressing BMP4 develop a fibrodysplasia ossificans progressiva (FOP)-like phenotype. Am. J. Pathol. 165, 1107–1115. doi: 10.1016/S0002-9440(10)63372-X
Kan, L., Peng, C.-Y., McGuire, T. L., and Kessler, J. A. (2013). Glast-expressing progenitor cells contribute to heterotopic ossification. Bone 53, 194–203. doi: 10.1016/j.bone.2012.12.008
Karsenty, G. (2020). The central regulation of bone mass: genetic evidence and molecular bases. Handb. Exp. Pharmacol. 262, 309–323. doi: 10.1007/164_2020_378
Katayama, Y., Battista, M., Kao, W.-M., Hidalgo, A., Peired, A. J., Thomas, S. A., et al. (2006). Signals from the sympathetic nervous system regulate hematopoietic stem cell egress from bone marrow. Cell 124, 407–421. doi: 10.1016/j.cell.2005.10.041
Kaur, S., Raggatt, L. J., Batoon, L., Hume, D. A., Levesque, J.-P., and Pettit, A. R. (2017). Role of bone marrow macrophages in controlling homeostasis and repair in bone and bone marrow niches. Semin. Cell Dev. Biol. 61, 12–21. doi: 10.1016/j.semcdb.2016.08.009
Kaur, S., Raggatt, L. J., Millard, S. M., Wu, A. C., Batoon, L., Jacobsen, R. N., et al. (2018). Self-repopulating recipient bone marrow resident macrophages promote long-term hematopoietic stem cell engraftment. Blood 132, 735–749. doi: 10.1182/blood-2018-01-829663
Kaur, S., Sehgal, A., Wu, A. C., Millard, S. M., Batoon, L., Sandrock, C. J., et al. (2021). Stable colony-stimulating factor 1 fusion protein treatment increases hematopoietic stem cell pool and enhances their mobilisation in mice. J. Hematol. Oncol. J. Hematol. Oncol. 14:3. doi: 10.1186/s13045-020-00997-w
Kokkaliaris, K., Kunz, L., Cabezas-Wallscheid, N., Christodoulou, C., Renders, S., Camargo, F., et al. (2020). Adult blood stem cell localization reflects the abundance of reported bone marrow niche cell types and their combinations. Blood 136, 2296–2307. doi: 10.1182/blood.2020006574
Kollet, O., Canaani, J., Kalinkovich, A., and Lapidot, T. (2012). Regulatory cross talks of bone cells, hematopoietic stem cells and the nervous system maintain hematopoiesis. Inflamm. Allergy Drug Targets 11, 170–180. doi: 10.2174/187152812800392760
Kwan, W., Cortes, M., Frost, I., Esain, V., Theodore, L. N., Liu, S. Y., et al. (2016). The central nervous system regulates embryonic hspc production via stress-responsive glucocorticoid receptor signaling. Cell Stem Cell 19, 370–382. doi: 10.1016/j.stem.2016.06.004
Lapidot, T., and Kollet, O. (2010). The brain-bone-blood triad: traffic lights for stem-cell homing and mobilization. Hematol. Am. Soc. Hematol. Educ. Program 2010, 1–6. doi: 10.1182/asheducation-2010.1.1
Lis, G. J., Litwin, J. A., Kapelak, B., Furgal-Borzych, A., Gajda, M., Cichocki, T., et al. (2009). Development of mature lamellar bone with a hematopoietic compartment in an aortic valve homograft. J. Heart Valve Dis. 18, 578–580.
Loder, S. J., Agarwal, S., Chung, M. T., Cholok, D., Hwang, C., Visser, N., et al. (2018). Characterizing the circulating cell populations in traumatic heterotopic ossification. Am. J. Pathol. 188, 2464–2473.
Luckett, W. P. (1978). Origin and differentiation of the yolk sac and extraembryonic mesoderm in presomite human and rhesus monkey embryos. Am. J. Anat. 152, 59–97.
Luis, T. C., Tremblay, C. S., Manz, M. G., North, T. E., King, K. Y., and Challen, G. A. (2016). Inflammatory signals in HSPC development and homeostasis: too much of a good thing? Exp. Hematol. 44, 908–912.
Madaro, L., Passafaro, M., Sala, D., Etxaniz, U., Lugarini, F., Proietti, D., et al. (2018). Denervation-activated STAT3-IL-6 signalling in fibro-adipogenic progenitors promotes myofibres atrophy and fibrosis. Nat. Cell Biol. 20, 917–927.
Malecova, B., Gatto, S., Etxaniz, U., Passafaro, M., Cortez, A., Nicoletti, C., et al. (2018). Dynamics of cellular states of fibro-adipogenic progenitors during myogenesis and muscular dystrophy. Nat. Commun. 9:3670.
Mariani, S. A., Li, Z., Rice, S., Krieg, C., Fragkogianni, S., Robinson, M., et al. (2019). Pro-inflammatory aorta-associated macrophages are involved in embryonic development of hematopoietic stem cells. Immunity 50, 1439–1452.e5.
Maryanovich, M., Takeishi, S., and Frenette, P. S. (2018). Neural regulation of bone and bone marrow. Cold Spring Harb. Perspect. Med. 8:a031344.
Matthews, B. G., Torreggiani, E., Roeder, E., Matic, I., Grcevic, D., and Kalajzic, I. (2016). Osteogenic potential of alpha smooth muscle actin expressing muscle resident progenitor cells. Bone 84, 69–77.
Méndez-Ferrer, S., Bonnet, D., Steensma, D. P., Hasserjian, R. P., Ghobrial, I. M., Gribben, J. G., et al. (2020). Bone marrow niches in haematological malignancies. Nat. Rev. Cancer 20, 285–298.
Meyer, C., Haustrate, M.-A., Nisolle, J. F., and Deltombe, T. (2020). Heterotopic ossification in COVID-19: a series of 4 cases. Ann. Phys. Rehabil. Med. 63, 565–567.
Meyers, C., Lisiecki, J., Miller, S., Levin, A., Fayad, L., Ding, C., et al. (2019). Heterotopic ossification: a comprehensive review. JBMR Plus 3:e10172.
Mirshekar-Syahkal, B., Fitch, S. R., and Ottersbach, K. (2014). Concise review: from greenhouse to garden: the changing soil of the hematopoietic stem cell microenvironment during development. Stem Cells Dayt. Ohio 32, 1691–1700.
Mitroulis, I., Kalafati, L., Bornhäuser, M., Hajishengallis, G., and Chavakis, T. (2020). Regulation of the bone marrow niche by inflammation. Front. Immunol. 11:1540.
Miyajima, A., Kinoshita, T., Tanaka, M., Kamiya, A., Mukouyama, Y., and Hara, T. (2000). Role of oncostatin M in hematopoiesis and liver development. Cytokine Growth. Factor. Rev. 11, 177–183.
Morrison, S. J., and Scadden, D. T. (2014). The bone marrow niche for haematopoietic stem cells. Nature 505, 327–334.
Nombela-Arrieta, C., Pivarnik, G., Winkel, B., Canty, K. J., Harley, B., Mahoney, J. E., et al. (2013). Quantitative imaging of haematopoietic stem and progenitor cell localization and hypoxic status in the bone marrow microenvironment. Nat. Cell Biol. 15, 533–543.
Oberlin, E., El Hafny, B., Petit-Cocault, L., and Souyri, M. (2010). Definitive human and mouse hematopoiesis originates from the embryonic endothelium: a new class of HSCs based on VE-cadherin expression. Int. J. Dev. Biol. 54, 1165–1173.
Oberlin, E., Tavian, M., Blazsek, I., and Péault, B. (2002). Blood-forming potential of vascular endothelium in the human embryo. Dev. Camb. Engl. 129, 4147–4157.
Oishi, T., Uezumi, A., Kanaji, A., Yamamoto, N., Yamaguchi, A., Yamada, H., et al. (2013). Osteogenic differentiation capacity of human skeletal muscle-derived progenitor cells. PLoS One 8:e56641.
Orelio, C., Peeters, M., Haak, E., van der Horn, K., and Dzierzak, E. (2009). Interleukin-1 regulates hematopoietic progenitor and stem cells in the midgestation mouse fetal liver. Haematologica 94, 462–469.
Pierce, H., Zhang, D., Magnon, C., Lucas, D., Christin, J. R., Huggins, M., et al. (2017). Cholinergic Signals from the CNS regulate G-CSF-Mediated HSC mobilization from bone marrow via a glucocorticoid signaling relay. Cell Stem Cell 20, 648–658.e4.
Potter, B. K., Burns, T. C., Lacap, A. P., Granville, R. R., and Gajewski, D. A. (2007). Heterotopic ossification following traumatic and combat-related amputations. Prevalence, risk factors, and preliminary results of excision. J. Bone Joint Surg. Am. 89, 476–486.
Putz, C., Helbig, L., Gerner, H. J., Zimmermann-Stenzel, M., and Akbar, M. (2014). Autonomic dysreflexia: a possible trigger for the development of heterotopic ossifications after traumatic spinal cord injury: a clinical longitudinal study. Eur. J. Trauma Emerg. Surg. 40, 721–726.
Reznik, J. E., Biros, E., Marshall, R., Jelbart, M., Milanese, S., Gordon, S., et al. (2014). Prevalence and risk-factors of neurogenic heterotopic ossification in traumatic spinal cord and traumatic brain injured patients admitted to specialised units in Australia. J. Musculoskelet. Neuronal. Interact. 14, 19–28.
Rybtsov, S., Ivanovs, A., Zhao, S., and Medvinsky, A. (2016). Concealed expansion of immature precursors underpins acute burst of adult HSC activity in foetal liver. Dev. Camb. Engl. 143, 1284–1289.
Sawamiphak, S., Kontarakis, Z., and Stainier, D. Y. R. (2014). Interferon gamma signaling positively regulates hematopoietic stem cell emergence. Dev. Cell 31, 640–653.
Schofield, R. (1978). The relationship between the spleen colony-forming cell and the haemopoietic stem cell. Blood Cells 4, 7–25.
Shore, E. M., Xu, M., Feldman, G. J., Fenstermacher, D. A., Cho, T.-J., Choi, I. H., et al. (2006). A recurrent mutation in the BMP type I receptor ACVR1 causes inherited and sporadic fibrodysplasia ossificans progressiva. Nat. Genet. 38, 525–527.
Silver, L., and Palis, J. (1997). Initiation of murine embryonic erythropoiesis: a spatial analysis. Blood 89, 1154–1164. doi: 10.1182/blood.V89.4.1154
Singh, N. K., and Fleshman, R. (2011). Extramedullary hematopoiesis on the aortic valve. Tex. Heart Inst. J. 38, 210–211.
Stanley, A., Heo, S.-J., Mauck, R. L., Mourkioti, F., and Shore, E. M. (2019). Elevated BMP and mechanical signaling through YAP1/RhoA poises FOP mesenchymal progenitors for osteogenesis. J. Bone Miner. Res. 34, 1894–1909. doi: 10.1002/jbmr.3760
Stawski, L., and Trojanowska, M. (2019). Oncostatin M and its role in fibrosis. Connect. Tissue Res. 60, 40–49. doi: 10.1080/03008207.2018.1500558
Stoira, E., Elzi, L., Puligheddu, C., Garibaldi, R., Voinea, C., and Chiesa, A. F. (2021). High prevalence of heterotopic ossification in critically ill patients with severe COVID-19. Clin. Microbiol. Infect. Online ahead of print.
Sun, M., Chi, G., Xu, J., Tan, Y., Xu, J., Lv, S., et al. (2018). Extracellular matrix stiffness controls osteogenic differentiation of mesenchymal stem cells mediated by integrin α5. Stem Cell Res. Ther. 9:52. doi: 10.1186/s13287-018-0798-0
Tanaka, M., and Miyajima, A. (2003). Oncostatin M, a multifunctional cytokine. Rev. Physiol. Biochem. Pharmacol. 149, 39–52. doi: 10.1007/s10254-003-0013-1
Torossian, F., Guerton, B., Anginot, A., Alexander, K. A., Desterke, C., Soave, S., et al. (2017). Macrophage-derived oncostatin M contributes to human and mouse neurogenic heterotopic ossifications. JCI Insight 2:e96034. doi: 10.1172/jci.insight.96034
Tseng, H.-W., Kulina, I., Salga, M., Fleming, W., Vaquette, C., Genêt, F., et al. (2020). Neurogenic heterotopic ossifications develop independently of granulocyte colony-stimulating factor and neutrophils. J. Bone Miner. Res. 35, 2242–2251. doi: 10.1002/jbmr.4118
Tseng, H.-W., Millard, S., Alexander, K., Fleming, W., Kulina, I., Nowlan, B., et al. (2019). Neurogenic heterotopic ossifications are derived from fibroadipogenic progenitors in the skeletal muscle not from satellite cells. Exp. Hematol. 76:S89. doi: 10.1016/j.exphem.2019.06.449
Upadhyay, J., Farr, O. M., and Mantzoros, C. S. (2015). The role of leptin in regulating bone metabolism. Metabolism 64, 105–113. doi: 10.1016/j.metabol.2014.10.021
van Kampen, P. J., Martina, J. D., Vos, P. E., Hoedemaekers, C. W. E., and Hendricks, H. T. (2011). Potential risk factors for developing heterotopic ossification in patients with severe traumatic brain injury. J. Head Trauma Rehabil. 26, 384–391. doi: 10.1097/HTR.0b013e3181f78a59
Waas, B., and Maillard, I. (2017). Fetal hematopoietic stem cells are making waves. Stem Cell Investig. 4:25. doi: 10.21037/sci.2017.03.06
Wang, D., Shurafa, M. S., Acharya, R., Strand, V. F., and Linden, M. D. (2004). Chronic abdominal pain caused by heterotopic ossification with functioning bone marrow: a case report and review of the literature. Arch. Pathol. Lab. Med. 128, 321–323. doi: 10.5858/2004-128-321-CAPCBH
Wang, H., Lindborg, C., Lounev, V., Kim, J.-H., McCarrick-Walmsley, R., Xu, M., et al. (2016). Cellular hypoxia promotes heterotopic ossification by amplifying BMP signaling. J. Bone Miner. Res. 31, 1652–1665. doi: 10.1002/jbmr.2848
Wang, X., Li, F., Xie, L., Crane, J., Zhen, G., Mishina, Y., et al. (2018). Inhibition of overactive TGF-β attenuates progression of heterotopic ossification in mice. Nat. Commun. 9:551. doi: 10.1038/s41467-018-02988-5
Wang, X.-D., Li, S.-Y., Zhang, S.-J., Gupta, A., Zhang, C.-P., and Wang, L. (2020). The neural system regulates bone homeostasis via mesenchymal stem cells: a translational approach. Theranostics 10, 4839–4850. doi: 10.7150/thno.43771
Wilson, A., Laurenti, E., Oser, G., van der Wath, R. C., Blanco-Bose, W., Jaworski, M., et al. (2008). Hematopoietic stem cells reversibly switch from dormancy to self-renewal during homeostasis and repair. Cell 135, 1118–1129. doi: 10.1016/j.cell.2008.10.048
Winkler, I. G., Sims, N. A., Pettit, A. R., Barbier, V., Nowlan, B., Helwani, F., et al. (2010). Bone marrow macrophages maintain hematopoietic stem cell (HSC) niches and their depletion mobilizes HSCs. Blood 116, 4815–4828. doi: 10.1182/blood-2009-11-253534
Wosczyna, M. N., and Rando, T. A. (2018). A muscle stem cell support group: coordinated cellular responses in muscle regeneration. Dev. Cell 46, 135–143. doi: 10.1016/j.devcel.2018.06.018
Yokomizo, T., and Dzierzak, E. (2010). Three-dimensional cartography of hematopoietic clusters in the vasculature of whole mouse embryos. Dev. Camb. Engl. 137, 3651–3661. doi: 10.1242/dev.051094
Keywords: neurogenic heterotopic ossifications, ectopic hematopoietic niche, muscle environment, inflammation, macrophages
Citation: Girard D, Torossian F, Oberlin E, Alexander KA, Gueguen J, Tseng H-W, Genêt F, Lataillade J-J, Salga M, Levesque J-P, Le Bousse-Kerdilès M-C and Banzet S (2021) Neurogenic Heterotopic Ossifications Recapitulate Hematopoietic Stem Cell Niche Development Within an Adult Osteogenic Muscle Environment. Front. Cell Dev. Biol. 9:611842. doi: 10.3389/fcell.2021.611842
Received: 29 September 2020; Accepted: 17 February 2021;
Published: 05 March 2021.
Edited by:
Monzur Murshed, McGill University, CanadaReviewed by:
Chan Gao, McGill University, CanadaZohreh Khavandgar, National Institute of Dental and Craniofacial Research (NIDCR), United States
Copyright © 2021 Girard, Torossian, Oberlin, Alexander, Gueguen, Tseng, Genêt, Lataillade, Salga, Levesque, Le Bousse-Kerdilès and Banzet. This is an open-access article distributed under the terms of the Creative Commons Attribution License (CC BY). The use, distribution or reproduction in other forums is permitted, provided the original author(s) and the copyright owner(s) are credited and that the original publication in this journal is cited, in accordance with accepted academic practice. No use, distribution or reproduction is permitted which does not comply with these terms.
*Correspondence: Dorothée Girard, ZG9yb3RoZWUuZ2lyYXJkQGluc2VybS5mcg==