- 1Developmental Genetics Laboratory, Department of Biological Sciences, Indian Institute of Science Education and Research (IISER) Mohali, Mohali, India
- 2Molecular Cell and Developmental Biology Laboratory, Department of Biological Sciences, Indian Institute of Science Education and Research (IISER) Mohali, Mohali, India
Over the years, Drosophila has served as a wonderful genetically tractable model system to unravel various facets of tissue-resident stem cells in their microenvironment. Studies in different stem and progenitor cell types of Drosophila have led to the discovery of cell-intrinsic and extrinsic factors crucial for stem cell state and fate. Though initially touted as the ATP generating machines for carrying various cellular processes, it is now increasingly becoming clear that mitochondrial processes alone can override the cellular program of stem cells. The last few years have witnessed a surge in our understanding of mitochondria’s contribution to governing different stem cell properties in their subtissular niches in Drosophila. Through this review, we intend to sum up and highlight the outcome of these in vivo studies that implicate mitochondria as a central regulator of stem cell fate decisions; to find the commonalities and uniqueness associated with these regulatory mechanisms.
Introduction
Adult stem cells are rare populations of undifferentiated cells that reside among the differentiated cells in fully developed tissues. During the postnatal life, these multipotent cells possess two defining characteristics: (a) the capacity to self-renew themselves and (b) differentiate into a limited number of mature cell types (Fuchs and Chen, 2012; Sánchez Alvarado and Yamanaka, 2014). Usually, the adult stem cells are maintained in a quiescent state (Cheung and Rando, 2013), and they proliferate and differentiate only upon activation (Post and Clevers, 2019). In doing so, they are dynamically involved in remodeling the tissue in response to turnover (Barker et al., 2010), damage (Goldman and Poss, 2020), and disease (Clevers and Watt, 2018; Naik et al., 2018). Therefore, the precise balance between adult stem cell self-renewal and differentiation is crucial for tissue growth and homeostasis.
Within a tissue, adult stem cells are located in a specialized microenvironment termed as the niche (Schofield, 1978; Kimble and White, 1981). Though the actual architecture of the niche and its components vary for different tissues, the niche typically has a spatial organization that provides anatomical and functional interactions critical for stem cell maintenance (Xie and Spradling, 1998), their proliferation (Kiger, 2001), and fate specification (Ferraro et al., 2010). Mutual and dynamic cell-cell interaction between niche and stem cells that involve secreted factors and signaling molecules elaborated or induced by niche cells (Huelsken et al., 2001; Chacon-Martinez et al., 2018), niche ECM proteins (Fuchs et al., 2000), and the physical forces attributed by niche’s mechanical scaffold (Kahn et al., 2009) and contribute significantly in maintaining the stem cell identity (Morrison and Spradling, 2008; Rezza et al., 2014). Apart from these local signals, increasing evidence highlights a pivotal role for distinct metabolic, systemic, and environmental signals in stem cell physiology and lineage specification (Drummond-Barbosa, 2008, 2019; Murphy, 2008; Shyh-Chang et al., 2013; Speder and Brand, 2018). Importantly, it is gradually becoming apparent from the recent findings that metabolites (Harvey et al., 2019) and nutrients (Ochocki and Simon, 2013) modulate stem cell fate by actively participating in controlling their intracellular signaling and enzymatic activities (Shyh-Chang and Ng, 2017). The role of metabolic intermediates in changing the epigenetic landscape of the stem cells and their progenies in terms of histone modifications (Yucel et al., 2019) and DNA methylation (DiTroia et al., 2019) is also much appreciated.
Mitochondria, classically known as “powerhouses” of a cell, are responsible for ATP production through oxidative phosphorylation (Oxphos) and sustained electron transport chain (ETC) activity. Besides their fundamental role in energy harvesting, mitochondria compartmentalize several metabolic pathways, such as the TCA cycle, fatty acid β-oxidation (FAO), and steroid metabolism (Newmeyer and Ferguson-Miller, 2003; McBride et al., 2006; Nunnari and Suomalainen, 2012). Functioning as a metabolic hub, mitochondria also play a critical role in integrating cell-intrinsic and extrinsic signals to regulate diverse processes that include calcium homeostasis (Vasington and Murphy, 1962), inflammation (Pearce and Pearce, 2013) and apoptosis (Green and Reed, 1998). Moreover, reactive oxygen species (ROS) generated as a byproduct of Oxphos, and the terminal and intermediate metabolites produced within the mitochondria can also act as retrograde signals to dictate gene expression, post-translational protein modifications, and bring about epigenetic modifications (Liu and Butow, 2006; Murphy, 2008; Tait and Green, 2012). Historically, relatively less attention was given to understand the role of mitochondria in stem cell biology, presumably because stem cells generally possess non-fused, spherical (immature form) mitochondria with poorly developed cristae (Lisowski et al., 2018). Therefore, it is generally believed that the stem cells rely more on glycolysis to generate energy for their proliferation. However, several studies in the last decade have unveiled the importance of mitochondria in controlling stem cell behavior, including their decisions to self-renew or differentiate (Mandal et al., 2011; Wanet et al., 2015; Bahat and Gross, 2019; Hinge et al., 2020).
The fruit fly, Drosophila melanogaster, is a versatile model organism used extensively in biomedical research to garner valuable information about regulatory pathways that facilitate our understanding of parallel pathways in humans. With the availability of sophisticated genetic tools, an extensive collection of mutants, and relatively easy accessibility to tissues, flies have provided a fantastic opportunity for in vivo analyses of adult stem cells (Yamashita et al., 2005; Jennings, 2011; Losick et al., 2011). To date, several stem cell populations have been identified in adult flies. While the Germline Stem Cells (GSCs), the Follicle Stem Cells (FSCs), the Escort Stem Cells (ESCs), and the Cyst Stem Cells (CyScs) are associated with the adult gonads (Margolis and Spradling, 1995), the adult gut harbors the Intestinal Stem Cells (ISCs; Micchelli and Perrimon, 2005; Ohlstein and Spradling, 2005; Takashima et al., 2008). Moreover, the presence of Muscle Stem Cells (MSCs; Chaturvedi et al., 2017) and Renal Stem Cells (RSCs; Singh et al., 2007) has been reported in the adult muscles and Malpighian tubules, respectively. Besides these, several stem cell populations that include Hematopoietic Stem Cells (HSCs; Dey et al., 2016) and Neural Stem Cells (NSCs; Truman and Bate, 1988; Bahat and Gross, 2019) are present as transient populations of stem cells during post-embryonic development. Studies of these different populations of stem cells have provided a high-resolution picture of the molecular mechanisms and cellular properties of stem cells that are conserved in mammals.
This review sets out to provide a comprehensive account of the recent advances in our understanding of mitochondria’s role in regulating GSCs, ISCs, NSCs, and hemocyte progenitors in Drosophila. In this context, it is essential to note that the role of mitochondria in HSCs, MSCs, and RSCs is yet to be implicated. Given that most of the analyses have been done in vivo, the findings are much more physiologically relevant. They provide relatively more accurate information about the importance of mitochondrial function in stem cell biology. Finally, we would highlight the mitochondrial regulatory mechanisms that are remarkably conserved across the different stem cell types, in contrast to those unique for a specific kind of stem cell.
Germline Stem Cells in Drosophila
Production of gametes (sperms and egg) in adult individuals relies upon a robust stem cell system capable of balancing self-renewal with differentiation. Irrespective of its nature, gametes produced from the GSCs hold the key to the perpetuation of a species. Drosophila GSCs are established during development and function throughout the reproductive life to produce gametes (McKearin and Spradling, 1990). Like all other organisms, the Drosophila GSCs reside in a defined anatomical niche that provides necessary signals to maintain the precise balance between GSC self-renewal and differentiation (Fuller and Spradling, 2007). In addition, the GSCs and their developing progenies also sense and respond to a plethora of systemic factors governed by diverse physiological inputs that include diet intake, the organism’s metabolic status, and other environmental factors (Drummond-Barbosa and Spradling, 2001; Ting, 2013; Drummond-Barbosa, 2019; Kahney et al., 2019). Given the intricate nature of integrating local and systemic signaling pathways to modulate the state and fate of GSCs, the germline has emerged as one of the best models for studying adult stem cell biology in vivo (Greenspan et al., 2015). Our understanding of the Drosophila GSCs has identified a wide range of mechanisms by which metabolic status in general and mitochondrial function, in particular, dictates GSC activities.
Mitochondrial Regulation of Male GSCs in Drosophila
The Drosophila testis (Figure 1A) harbors around 9–11 GSCs at its apical tip adjacent to a cluster of non-diving somatic cells known as the hub (HC) that serves as a primary cellular component of the male GSC niche (de Cuevas and Matunis, 2011). GSCs divide asymmetrically to produce two daughter cells. One daughter cell that remains close to the hub retains the stem cell identity. Simultaneously, the other that is displaced away from the hub (referred to as a goniablast, GB) initiates the differentiation (Figure 1A). The goniablast undergoes four rounds of mitotic divisions with incomplete cytokinesis to give rise to a cyst of 16 interconnected spermatogonia. In addition, each GSC remains surrounded by a pair of CySCs (Figure 1A), which divides and differentiates into cyst cells (CC), that form a protective layer around the developing spermatogonia. Spermatogonia differentiate into spermatocytes, which by meiotic division give rise to spermatids that eventually mature to form sperms (Papagiannouli, 2014).
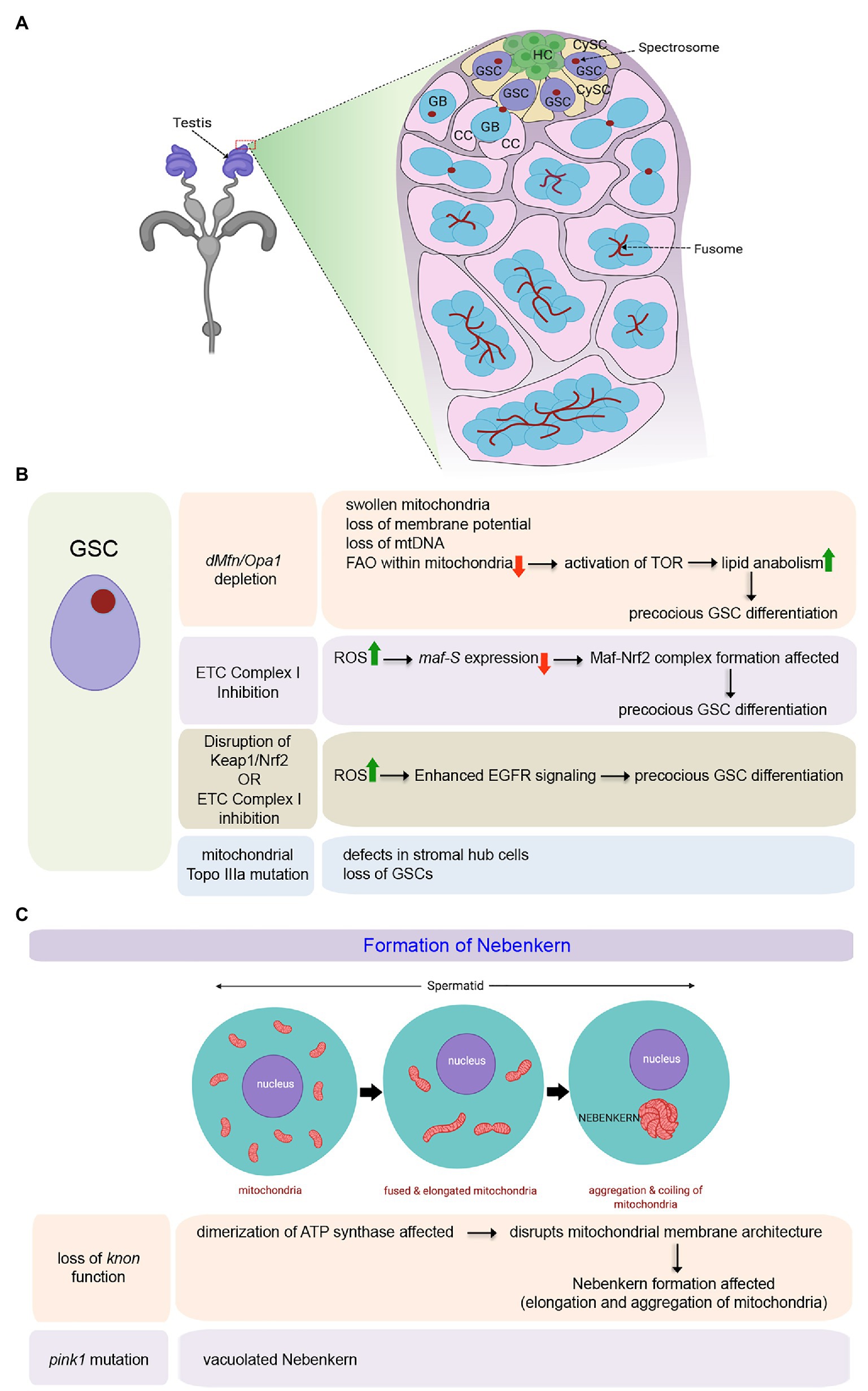
Figure 1. Role of mitochondria in maintenance and differentiation of male Germline Stem Cells (GSCs) in Drosophila. (A) Schematic representation of the tip of the adult testis showing the anatomical position of male GSCs and other associated cell types. The expanded forms of the acronyms used are provided in the text. (B) Table showing the diverse outcomes of manipulating mitochondrial structure and function in the male GSCs. (C) Schematic representation for mitochondrial fusion and aggregation to form the Nebenkern in the spermatids. Loss of knon and pink1 function affects Nebenkern formation.
Mitochondria are highly dynamic organelles capable of changing their shape continuously undergoing fission or fusion. Significantly, change in their morphology is closely related to their functionality (Westermann, 2010). The two genes, Drosophila homolog of Mitofusin (known as dMfn or Marf) and Optic Atrophy 1 (Opa1), responsible for the fusion of the outer and inner mitochondrial membranes, respectively, have been shown to play a pivotal role in maintaining the male GSCs (Sênos Demarco et al., 2019). It has been demonstrated that a block in mitochondrial fusion, either by dMfn or Opa1 depletion, leads to a gradual loss of GSCs over time as they undergo precocious differentiation (Figure 1B). In the absence of fusion, mitochondria within the GSCs become swollen with aberrant ultrastructures, including collapsed cristae. Other hallmarks of these aberrant mitochondria include loss of membrane potential, loss of mitochondrial DNA (mtDNA), and decreased distribution of mitochondrial contents without significant upregulation in the ROS levels. Mechanistically, the inability of GSCs to self-renew and its loss due to differentiation in the absence of mitochondrial fusion is instigated by lipid anabolism and increased lipid accumulation in these cells. In the absence of an active and fused mitochondrial network, FAO within the mitochondria gets significantly affected, resulting in an increase in the level of fatty acids within the GSCs. Increased fatty acid levels, in turn, activates TOR that stimulates SREBP-mediated lipid anabolism, leading to further accumulation of lipids through a positive feedback mechanism. Under these conditions, male GSCs fail to maintain and eventually differentiate (Figure 1B; Sênos Demarco et al., 2019). In this context, it is essential to note that the independent knockdown of FAO enzymes, either by genetic or by pharmacological means, also leads to a similar phenotype, as seen for blocking mitochondrial fusion in the GSCs (Sênos Demarco et al., 2019). The involvement of mitochondrial FAO in the maturation of sperm has also been evidenced in flies mutant for the gene scully (Barbas et al., 1998), which codes for the mitochondrial 3-hydroxyacyl-CoA dehydrogenase responsible for the conversion of hydroxyl-acyl-CoA to ketoacyl-CoA during FAO. scully mutant testes are reduced in size and lack maturing sperm. The mutant spermatocytes are characterized by the accumulation of cytoplasmic lipid inclusions and scarce and aberrant mitochondria. Thus mitochondrial fusion, which in turn supports increased FAO, is essential for the maintenance of the male GSCs.
The role of mitochondrial fission, on the other hand, has not been implicated in the maintenance of GSCs in adult flies (Senos Demarco and Jones, 2019). Mutant clones of the pro-fission gene, Dynamin-related protein (Drp1), have otherwise normal GSC numbers and function in adults. However, Drp1 does play a role in the maintenance of early germ cells in the larval testis. A block in mitochondrial fission by Drp1 depletion results in elevated ROS levels in the germ cells that led to activation of the EGFR pathway in the somatic cyst cells (Senos Demarco and Jones, 2019). This, in turn, induces a loss in GSC number and spermatogonia due to premature differentiation.
Inhibition of ETC complex I component ND75 (Drosophila homolog of human mitochondrial Complex I subunit NDUFS) also leads to precocious GSC differentiation by increased ROS levels in the Drosophila testis (Figure 1B; Tan et al., 2018). Gene expression profiling of the testes expressing ND75RNAi under the control of nos-Gal4, which is expressed in early-stage germ cells, identified Maf-S [a family member of basic region leucine zipper (bZIP)-type transcription factor Maf] as one of the effector molecules responsible for causing high ROS mediated early differentiation of GSCs. maf-S is transcriptionally downregulated by oxidative stress in the ND75 knocked down testis (Figure 1B). Independently, it has been demonstrated that in wild-type flies while knockdown of maf-S leads to GSC differentiation, maf-S over-expression leads to a tumorous overgrowth of GSC-like cells. The precocious differentiation of GSCs as observed upon oxidative stress can be suppressed by ectopic expression of Maf-S, thereby demonstrating that Maf-S acts as a downstream effector of ROS signaling. Mechanistically, Maf-S interacts genetically with the Keap1-Nrf2 redox management system to regulate ROS-associated GSC behavior in the Drosophila testis (Tan et al., 2018). The Kelch-like ECH-associated protein 1 (Keap1)-NF-E2-related factor 2 (Nrf2) redox management system is a crucial regulator of cellular redox homeostasis (Suzuki et al., 2019). Oxidative stress leads to the disruption of Keap1-mediated proteasomal degradation of Nrf2. As a result, the stable Nrf2 translocates into the nucleus to hetero-dimerize with the Maf proteins. Together, they transcriptionally activate genes associated with detoxification that include thioredoxin reductase and glutathione reductase. In the case of GSCs with attenuated Complex I activity, due to a reduction in maf-s expression, the formation of the Maf-Nrf2 complex gets affected. In turn, this might disrupt the expression of some detoxification genes, allowing the GSCs to differentiate. Though, the target genes that lead to precocious differentiation are yet to be known (Tan et al., 2018), these results further endorse the importance of fused mitochondria and Oxphos in GSC maintenance. A separate study has also demonstrated that reducing ROS levels either by inhibiting the activity of Keap1 or upon antioxidant treatment promotes the overgrowth of GSC-like cells (Tan et al., 2017). Whereas, reduction in GSC number due to precocious differentiation is associated with elevated levels of ROS induced by alteration in Keap1/Nrf2 activity. Notably, this ROS mediated GSC differentiation, observed either upon disrupting the Keap1/Nrf2 activity or upon attenuating ETC Complex I activity (Figure 1B), is an outcome of enhanced EGFR signaling as the expressions of the EGFR ligand spitz gets increased. In consistence, significantly enhanced p-Erk1/2 expression is detected in CySCs and cyst cells that provide the necessary cues for differentiation (Figure 1B; Tan et al., 2017).
Significant changes in the mitochondrial network characterize the development of spermatids from GSC (Tokuyasu, 1975; Sênos Demarco et al., 2019). The network of mitochondria gets reticular with each developmental stage. Following meiosis, they dramatically fuse to form a giant layered spherical structure in the spermatids termed as the Nebenkern (Figure 1C; Hales and Fuller, 1997). Apart from its distinct physical appearance, the Nebenkern has been reported to have an unconventionally large paralog of ATP synthase subunit d. The gene knotted onions (knon) encodes a testis-specific paralog of ATP synthase subunit d, essential for Nebenkern’s formation and subsequent dynamics (Sawyer et al., 2017). Knon has been proposed to prevent the mitochondrial inner membrane’s sharp positive curvature within the Nebenkern by altering ATP synthase’s dimerization. As a result, during Nebenkern formation, the inner mitochondrial membrane shows very shallow curvature and stays closely apposed to the outer membrane. This unusual mitochondrial membrane configuration is critical for mitochondrial elongation. Loss of Knon function disrupts the typical mitochondrial membrane architecture within the Nebenkern that, in turn, causes aberrant mitochondrial elongation leading to male sterility (Figure 1C; Sawyer et al., 2017). Another study has also evidenced the association of other ATP synthase subunits with defects in germ cell maturation (Yu et al., 2019). For instance, knockdown of the ATP synthase β subunit in early germ cells hinders germ cell maturation without any apparent effect on GSCs and spermatogonia. The hub and cyst cells also remain unaffected. Other major ATP synthase subunits, upon their loss, also impede germ cell maturation (Yu et al., 2019).
Control of GSC activity in the adult testis by mitochondrial function has also been evidenced in a few more independent studies. For instance, 33% of males with defects in mitochondrial Topoisomerase IIIα (a member of the conserved Type IA subfamily of topoisomerases) are completely sterile (Wu et al., 2010). In contrast, the rest have lingering fertility, which gets lost in 6 days. Topo IIIa mutant males progressively lose GSCs with concomitant defects in stromal hub cells outlining the stem cell niche (Figure 1B). Furthermore, inhibition of Drosophila pink1 leads to male sterility with defects in mitochondrial morphology and increased sensitivity to oxidative stress (Clark et al., 2006). In pink1 mutant males, though the nuclei appear to be normal, spermatids have vacuolated Nebenkerns (Figure 1C). Interestingly, the expression of human PINK1 in the Drosophila testes restores male fertility and normal Nebenkern morphology in pink1 mutants. Furthermore, Pink1 works in conjunction with Parkin, as loss of their individual function phenocopies each other and the defects of pink1 mutants can be rescued by overexpression of parkin (Clark et al., 2006).
Mitochondrial Regulation of Female GSCs in Drosophila
Drosophila females have two ovaries, typically comprised of 16–21 ovarioles (Figure 2A). GSCs are located in the germarium that constitutes the apical end of each ovariole (Kirilly and Xie, 2007). The germarium is followed by a series of developing egg chambers arranged linearly with the most mature egg chamber at the distal end. Within the germarium, 2–3 GSCs reside at the anterior tip in close proximity to the niche. The niche consists of a cluster of 5–7 cap cells (CC) that remain connected to 8–10 tightly packed disc-like terminal filament (TF) cells (Figure 2A). GSCs typically undergo asymmetric self-renewing divisions, producing one daughter stem cell that remains attached to the cap cell and a second daughter cell displaced from the niche. The daughter cell leaving the niche, generally termed as the cystoblast (CB), undergoes four rounds of amplifying division without cytokinesis to generate a 16-cell interconnected cyst (Greenspan et al., 2015).
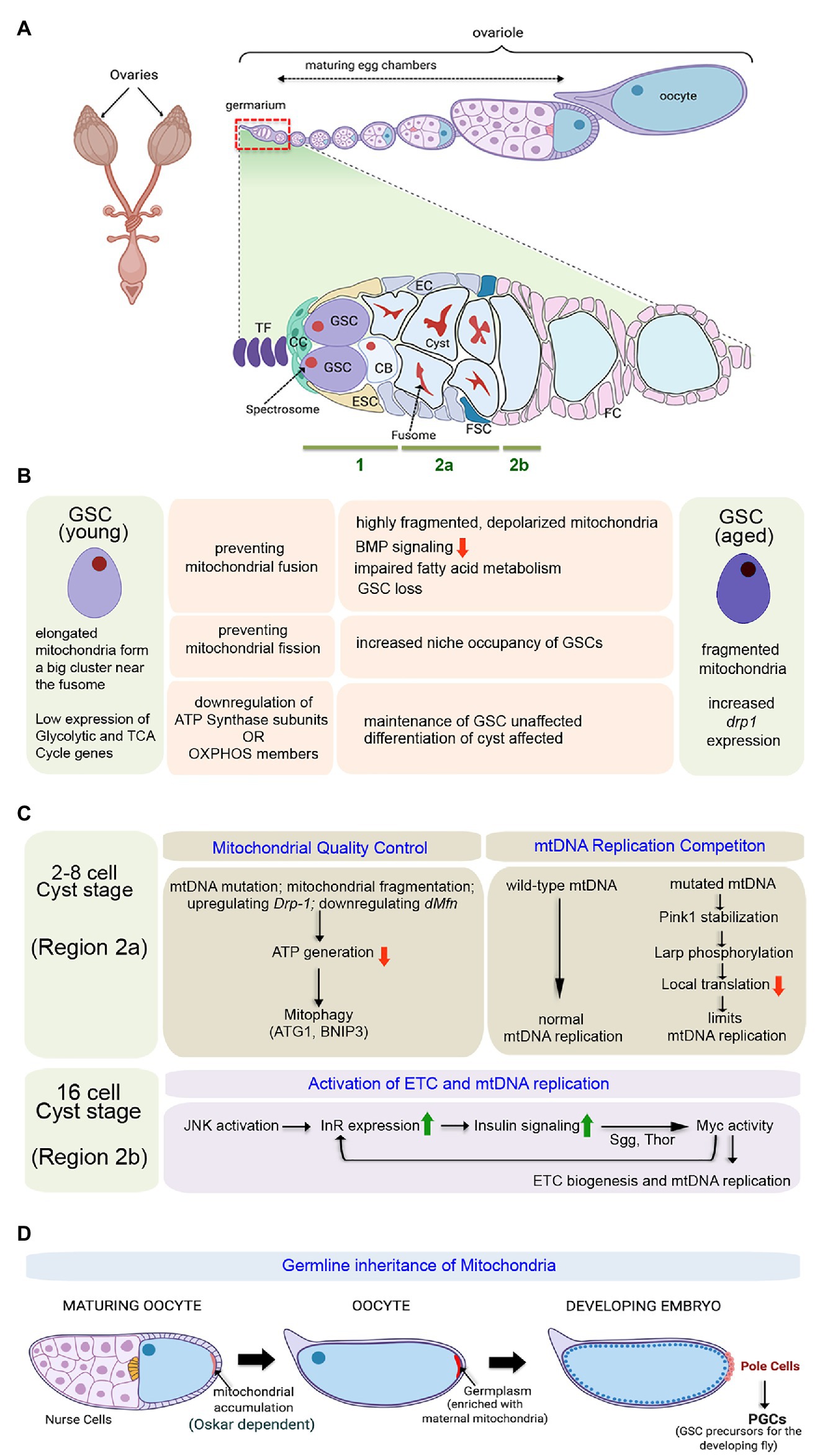
Figure 2. Importance of mitochondria in governing proliferation and differentiation of female GSCs in Drosophila. (A) Schematic representation of the adult ovary, a single ovariole showing the relative position of the germarium and the developing egg chambers, and a magnified view of the germarium displaying the anatomical position of the female GSCs, niche components, Escort Stem cells (ESCs), and Follicle Stem Cells (FSCs). The expanded forms of the acronyms used are provided in the text. (B) Table showing the diverse outcomes of manipulating mitochondrial structure and function in the young female GSCs. While young GSCs have elongated mitochondria, the aged ones are characterized by fragmented mitochondria. (C) Schematics of the pathways involved in mitochondrial quality control, mitochondrial DNA (mtDNA) replication competition, and activation of electron transport chain (ETC) and mtDNA replication in the developing cysts. (D) Diagrammatic representation of the process of germline inheritance of mitochondria through the pole cells.
Apart from the GSCs, the germarium harbors two types of somatic stem cells. The ESCs divide to generate escort cells (EC) that wrap around the cystoblast and encase the dividing cysts until they reach the stage of 16 cells (Figure 2A; Margolis and Spradling, 1995). After that, the cysts get surrounded by the somatic follicle epithelial cells (FC), and they bud off the germarium as individual egg chambers. About 2–3 FSCs located at the junction between regions 2a and 2b of the germarium divide asymmetrically to give rise to the follicle epithelial cells (Figure 2A; Margolis and Spradling, 1995). Eventually, the egg chamber develops into a mature egg chamber consisting of 15 nurse cells and an oocyte.
It has been observed that while the young GSCs are characterized by the presence of relatively elongated mitochondria forming a big cluster near the fusome, with aging, fragmented mitochondria are observed in the GSCs with a concomitant increase in the expression of the mitochondrial fission gene, drp1 (Amartuvshin et al., 2020). Inducing mitochondrial fission in young GSCs (in GSCs mutant for dmarf) mimics the condition observed in aged GSCs (Figure 2B). Interestingly, these GSCs with fragmented mitochondria, proliferate slowly, are low in BMP signaling, and demonstrate a tendency to move away from the niche and differentiate. Metabolically, these GSCs are characterized by low mitochondrial membrane potential and increased lipid accumulation due to defective fatty acid metabolism. In contrast, preventing mitochondrial fission (in GSCs mutant for drp1), as such, does not affect GSC division or maintenance. However, it seems that the drp1 mutant GSCs exhibit increased niche occupancy (Figure 2B), at least in part, due to increased E-cadherin expression. Preventing mitochondrial fission in aged GSCs enhances their maintenance by preventing their loss due to low mitochondrial membrane potential, decreased ROS levels, and reduced BMP signaling (Amartuvshin et al., 2020). Furthermore, it has been documented that proliferative aging of the GSCs causes dramatic decrease in cytochrome C oxidase activity, impaired mtDNA replication, and accumulation of mutations on mtDNA (Ren et al., 2017). Based on these strong correlations, mitochondrial fission can be considered as an important contributing factor for age-dependent decline in GSC activity, eventually responsible for the reduced hatching rate of embryos produced by old mothers.
Gene expression profiling studies revealed that the genes associated with glycolysis and Kreb’s cycle are expressed at very low levels in the young GSCs (Figure 2B; Teixeira et al., 2015). Notably, the transcript levels of the glycolytic enzyme Aldolase and that of the Kreb’s cycle enzyme Oxoglutarate dehydrogenase are hardly detectible, implying that the GSCs acquire ATP from sources other than internal oxidative metabolism. That the GSCs do not rely on Oxphos has been further evidenced by the observation that GSC specification and maintenance remain unaffected even after knocking down each of the 13 nuclear-encoded ATP synthase subunits. However, differentiation of the germ cells gets arrested upon knocking down ATP synthase subunits (Figure 2B). Impairing the processes of mitochondrial transcription, translation, and protein import machinery, associated with expression, assembly, and oligomerization of the ATP synthase, also exhibit similar differentiation defects as the cysts cannot move from four to eight-cell stage. Interestingly, the differentiation process of female GSCs is not dependent on the ATP synthesizing capacity of this Complex, as knockdown of other members of the Oxphos pathway does not disrupt differentiation. Instead, the ATP synthase dimerization-dependent mitochondrial crista maturation serves as the critical factor for cyst maturation and subsequent differentiation (Teixeira et al., 2015). Thus, ATP synthase is not essential for stem cell maintenance or the initiation of differentiation but instead plays a crucial role in cyst differentiation independent of Oxphos.
Given the fact that mitochondria are primarily maternally inherited and mtDNA replication does not initiate in early embryonic development, oocytes are furnished with large amounts of mitochondria to cater the energy demands of early embryogenesis (Tourmente et al., 1990). However, while furnishing the oocytes with a sufficient number of mitochondria, oogenesis also limits the transmission of defective mitochondria with mtDNA mutations. Therefore, quality control of the mitochondria (Figure 2C) is of pertinent importance as compared to the nuclear genome, mitochondria have high mutation rates, low recombination levels of mtDNA, and lack of an effective repair mechanism. In order to restrain the propagation of deleterious mitochondrial mutations in subsequent generations, female germline has a robust selection mechanism that is not observed in male germline or somatic tissues, including the ovary’s soma. At the 2–8 celled cyst stage, when mtDNA replication does not occur, mitochondrial fragmentation serves as an effective selection mechanism to drive out defective mitochondria (Lieber et al., 2019). Notably, the fragmented mitochondria that adopt a rounder morphology produce low ATP, which marks these mitochondria for removal by recruiting mitophagy proteins Atg1 and BNIP3. Interestingly, genetic means of mitochondrial fragmentation by upregulating pro-fission gene Drp-1 or downregulation of dMfn in female GSCs also leads to fragmentation of defective mitochondria at 2–8 celled cyst stage followed by their elimination (Lieber et al., 2019).
Intriguingly, mtDNA fitness and mitochondrial respiration are driven by an active ETC that is intertwined in the mitochondrial selection mechanism. Both mitochondrial respiration and mtDNA replication are quiescent in GSCs and dividing cysts but get markedly upregulated in the late germarium (Figure 2C). This transition is achieved by a feed-forward Insulin-Myc axis that promotes transcriptional activation of ETC genes and mtDNA replication (Wang et al., 2019). To begin with, transient activation of Jun N-terminal kinase (JNK) in the late germarium upregulates insulin receptor (InR) that boosts insulin signaling (IIS) to regulate post-translational Myc activity through Shaggy (Sgg) and 4-EBP (Thor). In turn, Myc controls ETC biogenesis and mtDNA replication. Importantly, though the expression of the InR and the initiation of insulin-Myc signaling are triggered by transient JNK signaling, Myc maintains IIS activity by boosting InR expression after JNK activity subsides. Inactivation of IIS and JNK signaling does not impact the number of eggs laid. However, it significantly reduces mtDNA’s deposition in the oocytes that negatively affects the hatching of eggs (Wang et al., 2019). Similarly, the abrogation of mtDNA replication by loss of topoisomerase IIIα (topo IIIα) leads to complete sterility. Here also, there is no defect in the egg-laying of topo IIIα mutant females, but their eggs are smaller in size with around 20-fold decrease in mtDNA content and do not hatch (Wu et al., 2010).
Apart from supplying the oocyte with adequate amounts of mtDNA, extensive mtDNA replication is essential to support another unique phenomenon termed as replication competition (Figure 2C). This refers to a process wherein wild-type mtDNA replicates and increases in mass to out-compete mtDNA carrying deleterious mutations (Zhang et al., 2019). This selective propagation of healthy mitochondria is aided by simultaneous phasing out of deleterious or damaged mitochondria. PINK1, the mitochondrial protein kinase, stabilizes on the outer membrane of the mitochondria harboring deleterious mtDNA mutations and stops the local protein synthesis by phosphorylating La-related protein (Larp), which serves as a translation stimulator. Inhibition of local protein translation on defective mitochondria limits their mtDNA replication and hence the transmission of deleterious mutations to the offspring. The absence of this mechanism in pink1B9 mutants leads to impaired mtDNA selective inheritance (Zhang et al., 2019).
Quite interestingly, the process of germline inheritance of mitochondria also begins during oogenesis (Figure 2D). As the oocyte matures, a striking accumulation of mitochondria and mtDNA occurs toward its posterior end (Cox, 2003). This process is facilitated by the microtubule driven streaming of the oocyte cytoplasm. The long isoform of the protein Oskar alters the actin cytoskeleton to trap the mitochondria at the posterior end (Hurd et al., 2016). Post-fertilization, the first cellularization event happens in the posterior end of the syncytial embryo with pole cell formation. Therefore, these pole cells inherit the posterior end’s oocyte cytoplasm, generally termed as the germplasm, a mixture of proteins, RNAs, and mitochondria that are deposited there during oogenesis. Later during development, the pole cells give rise to the Primordial Germ Cells (PGCs), the precursors for the GSCs of the progeny flies. Thus, this fascinating mechanism ensures the germline inheritance of mitochondria from the mother to the progeny’s GSCs (Hurd et al., 2016).
Besides the GSCs, proper functioning of the FSCs also depends on mitochondrial activity. An unbiased genetic screen revealed that mitochondrial dysfunction and subsequent rise in ROS levels lead to loss of FSCs (Wang et al., 2012). While the loss in FSC by excessive accumulation of ROS is mediated by the activation of JNK pathway in a subset of the mitochondrial mutants isolated in the screen, several other mutations affecting mitochondria also lead to loss of FSC by pathways unrelated to ROS production, that remain to be identified.
Intestinal Stem Cells of the Midgut and Their Regulation by Mitochondria
One of the rapidly turned over tissues in most animals is the intestinal epithelium, wherein cells are lost continuously from the surface. They are replenished by the proliferation of the resident ISCs (Casali and Batlle, 2009). Since their discovery, Drosophila’s ISCs have come up as an excellent model for adult stem cell biology (Micchelli and Perrimon, 2005; Ohlstein and Spradling, 2005; Takashima et al., 2008). They bear significant resemblance to their mammalian counterparts in terms of their modes of fate specification and their ability to respond to damage. Most of the signaling pathways involved in regulating mammalian epithelial stem cells have been evidenced to regulate the Drosophila ISCs (Casali and Batlle, 2009).
In adult flies, the ISCs are housed in the epithelial layer of the midgut, the functional equivalent of the mammalian intestine. Structurally, the Drosophila midgut is comprised of a single layer of epithelium consisting of two differentiated cell types – the absorptive enterocytes (ECs) characterized by a polyploid nucleus and the relatively smaller hormone-producing enteroendocrine (EE) cells (Figure 3A). The ISCs lying adjacent to the basement membrane remain interspersed in between the ECs and EE cells (Figure 3A) along the entire length of the midgut (Miguel-Aliaga et al., 2018). A complex niche that constitutes the neighboring differentiated midgut epithelial cells, the surrounding visceral muscles, and the tracheal cells govern self-renewal, proliferation, and differentiation of the ISCs (Jin et al., 2017). The ISC of the posterior midgut usually undergoes an asymmetric division to generate a new ISC and an intermediate progenitor, enteroblast (EB; Goulas et al., 2012). Interestingly, though at a lower frequency, the ISCs can undergo symmetric division to produce either two ISCs or two EBs. In its turn, the EB can differentiate into either an EC or into an EE cell. However, recent data support that the ISCs can also directly differentiate into EE cells (Biteau and Jasper, 2014).
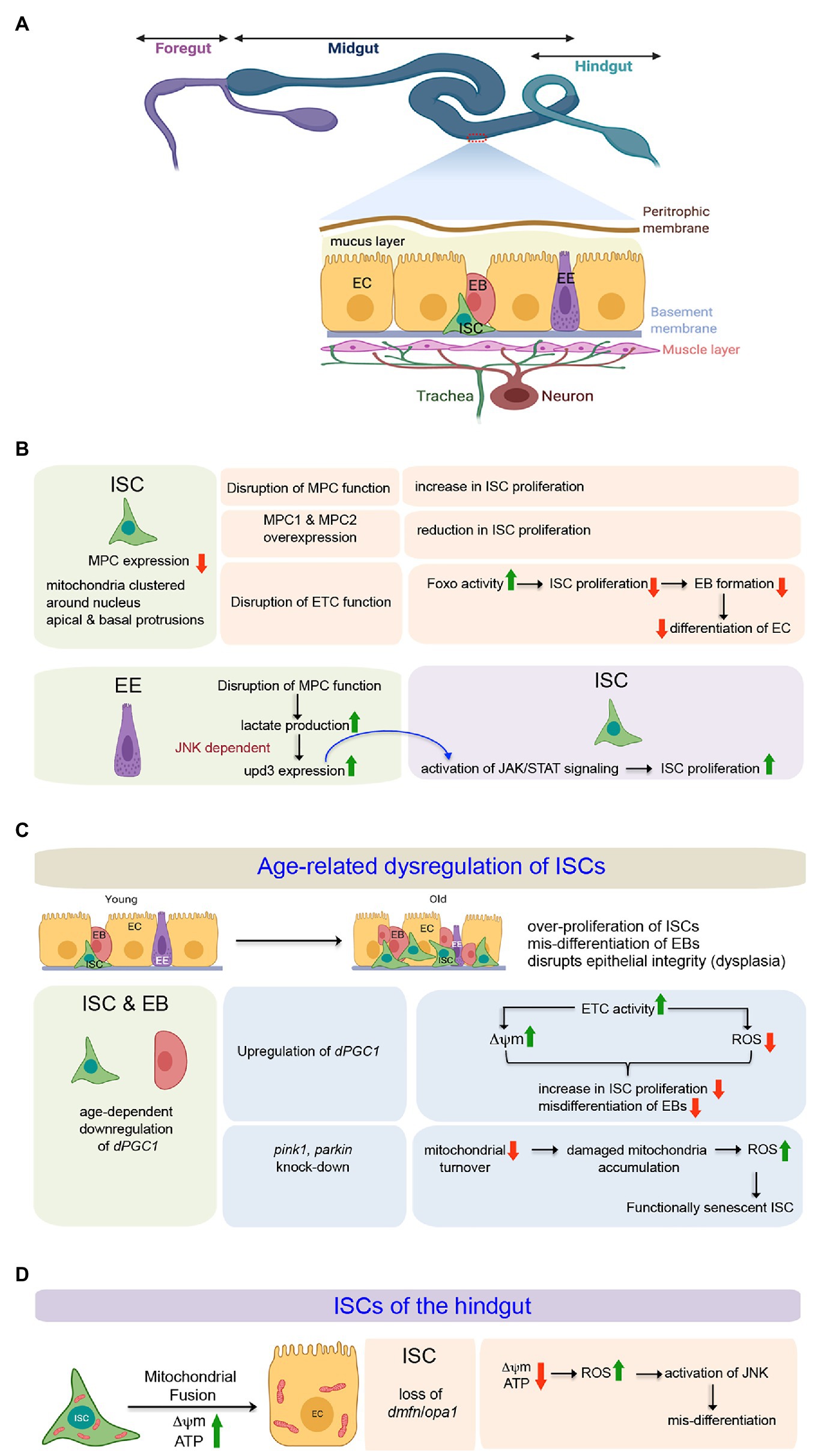
Figure 3. Involvement of mitochondria in defining the state and differentiation of Intestinal Stem Cells (ISCs) in the midgut and the hindgut of Drosophila. (A) Schematic representation of the adult gut of Drosophila highlighting the relative positions of the ISCs and the differentiating cell types in the midgut epithelium. The expanded forms of the acronyms used are provided in the text. (B) Table showing the importance of mitochondrial function to regulate proliferation and differentiation of midgut ISCs both in cell autonomous and non-autonomous manner. (C) Involvement of mitochondrial processes (biogenesis and turnover) during age-related dysregulation of ISCs in the midgut. (D) Mitochondrial fusion and its importance in the differentiation of hindgut ISCs.
The ISCs, EBs, and the ECs contain mitochondria clustered around their nuclei and packed, rather than randomly distributed, in their apical and basal protrusions (Figure 3B; Endow et al., 2019). The larger protrusions of the ISCs and ECs usually have larger numbers of clustered mitochondria. Despite having many mitochondria, the ISCs primarily rely on the glycolytic metabolic program to drive their robust proliferation (Schell et al., 2017). The possible reason behind the uncoupling of glycolysis from Oxphos is the low level of expression of the genes coding for Mitochondrial Pyruvate Carrier (MPC) in the ISCs. The MPC proteins, MPC1 and MPC2, form a complex on the mitochondrion’s inner membrane that is necessary and sufficient for efficient import of cytoplasmic pyruvate into the mitochondrial matrix (Bricker et al., 2012; Herzig et al., 2012). Within the matrix, the pyruvate dehydrogenase complex (PDH) converts pyruvate to acetyl-CoA that enters the TCA cycle. Thus, MPC serves as the bridge between cytoplasmic glycolysis and mitochondrial Oxphos. Disruption of MPC function, specifically in the ISCs, increases their rate of proliferation. Conversely, specific overexpression of MPC1 and MPC2 in ISCs or progenitors suppresses ISC proliferation (Figure 3B). Therefore, by limiting mitochondrial pyruvate flux, MPC plays a cell-autonomous role in maintaining the proliferation of ISCs (Schell et al., 2017). However, a very recent study demonstrates that mitochondrial Oxphos is essential for Drosophila ISC proliferation (Zhang et al., 2020). Genetic disruption of the mitochondrial ETC in the ISCs by manipulating the mitochondrial genome retards their proliferation rate, leading to the production of a limited number of EBs, which eventually fails to differentiate into ECs (Figure 3B). While RNAi mediated disruption of each ETC complex impairs ISC proliferation to some extent, a much stronger effect is observed upon knocking down members of complexes III and IV of the ETC. Notably, FOXO protein and its transcriptional activity are elevated in these ISCs and EBs without any detectable cellular ROS increase. Furthermore, knocking down foxo in these ISCs markedly suppresses the proliferation and lineage specification defects associated with the ETC disruption. Therefore, mitochondrial respiration is critical for Drosophila ISC proliferation and lineage specification and acts at least partially by repressing endogenous FOXO signaling (Zhang et al., 2020).
Quite interestingly, the mitochondrial function of the ECs also impacts the behavior of the ISCs (Figure 3B; Wisidagama and Thummel, 2019). Genetic loss of the MPC in ECs leads to increased lactate production by lactate dehydrogenase (LDH) that induces Upd3 expression in a JNK-dependent manner. The secreted cytokine Upd3 activates JAK/STAT signaling in the ISCs, promoting their proliferation. Interestingly, the increased proliferation of ISCs in response to the loss of MPC function in the EC can be suppressed by disrupting LDH function in the EC. However, under normal conditions, disruption of LDH in ECs does not impact ISC proliferation. These results imply the involvement of lactate in inducing ISC proliferation due to altered MPC function in the EC (Wisidagama and Thummel, 2019).
The Drosophila midgut exhibits dramatic changes as the animal ages (Figure 3C). One of the hallmark features is epithelial dysplasia, characterized by ISC overproliferation and aberrant differentiation of EBs (Rodriguez-Fernandez et al., 2020). Both cell-extrinsic and cell-intrinsic factors contribute to the continuous proliferative activation of ISCs. The cell-extrinsic factors include activation of JNK and PDGF/VEGF signaling by chronic production of ROS due to a shift in the composition of the gut microbiota (Buchon et al., 2009). A variety of intrinsic signaling factors that include a decline in mitochondrial function (Rera et al., 2011), drop in Nrf2 activity (Hochmuth et al., 2011), increased endoplasmic reticulum stress (Wang et al., 2014), as well as changes in autophagy (Rodriguez-Fernandez et al., 2019), contribute toward the disruption of ISC homeostasis. The overproliferating ISCs give rise to EBs that initiate but fail to differentiate into ECs and accumulate on the epithelium’s basal side, disrupting its structure and gut’s function. This age-related dysregulation is associated with a gradual decline in the expression of dPGC-1/spargel, which codes for the peroxisome proliferator-activated receptor γ coactivator 1, a central regulator of energy metabolism and mitochondrial biogenesis (Rera et al., 2011). Overexpression of dPGC-1, specifically in the ISCs and the EBs results in a delay of age-related dysregulation of the midgut (Figure 3C). The underlying mechanism involves an increase in the Complex I and Complex II activities of the mitochondrial ETC, which not only prevents the progressive loss of mitochondrial membrane potential but also lowers the ROS levels throughout the intestinal epithelium. Maintenance of mitochondrial activity and lowering ROS levels, in turn, abrogates the dramatic increase in ISC proliferation and accumulation of misdifferentiated daughter cells in the midgut that generally occurs during aging (Rera et al., 2011). Importantly, in the absence of transit-amplifying daughter cells due to direct differentiation of EBs into either of the two lineages, the effects of manipulating dPGC-1 in ISCs/EBs are not only restricted to the stem cells but are also reflected throughout the midgut. Nonetheless, it is evident from these studies that proper mitochondrial activity blocks age-dependint epithelial dysplasia of the gut by inhibiting the ISCs from undergoing overproliferation.
Interestingly, age-dependent downregulation of dPGC-1 in the midgut is associated with an age-dependent increase in the expression of the gene Indy (I’m not dead yet) that codes for a plasma membrane transporter of the Kreb’s cycle intermediate, citrate (Rogers and Rogina, 2014). The reduction of INDY activity, either by genetic means or by calorie restriction, leads to an increase in the midgut expression of dPGC-1 accompanied by enhanced mitochondrial biogenesis and reduction in ROS levels. These physiological changes prevent the ISCs from undergoing excessive proliferation and thereby preserve ISC homeostasis. Thus, by modulating dPGC-1, INDY functions as a physiological regulator of the mitochondrial function of the ISCs in response to changes in nutrient availability and organismal needs (Rogers and Rogina, 2014).
A remarkable improvement in age-dependent dysregulation of gut homeostasis has also been reported upon RNAi-mediated knockdown of the mitophagy related genes, pink1 and parkin in the ISCs/EBs (Figure 3C; Jones et al., 2017). ISC/EB–specific reduction of Pink1 and Parkin blocks mitochondrial turnover and eventually leads to the accumulation of damaged mitochondria with swollen or collapsed morphology and high ROS levels. Interestingly, despite having elevated ROS levels, the ISCs do not undergo uncontrolled proliferation, a hallmark feature for age-related changes in the midgut. Instead, the ISCs, with accumulated damaged mitochondria within them, become functionally senescent, thereby highlighting that: (a) ISCs use mitophagy as one strategy to maintain a healthy complement of mitochondria and (b) there exists a mechanism by which ISC/EB–specific mitochondrial dysfunction uncouples cellular and tissue aging to maintain the organization of the intestinal epithelium (Jones et al., 2017). Even though elevated levels of ROS upon downregulation of pink1 and parkin prevent excessive proliferation of the ISCs, increased ISC proliferation is generally observed upon treatment with the ROS-inducing compound Paraquat (Biteau et al., 2008) as well as in mutants for the ROS scavenging enzyme catalase (Choi et al., 2008). Conversely, treating flies with N-Acetyl-Cysteine and Glutathione limit ISC proliferation (Buchon et al., 2009). Analogous to female GSCs, the Keap1 and Nrf2 redox management system establishes a switch that controls the proliferation of ISCs (Hochmuth et al., 2011). In young flies, under homeostatic conditions, constitutively active Nrf2 induces antioxidant genes, such as gstD1, gclc, and jafrac1, to maintain low intracellular ROS levels and thereby prevents excessive proliferation. In contrast, in aged flies and during mitogenic and stress conditions, Keap1 mediated inhibition of Nrf2 prevents the antioxidant genes’ expression. As a result, the intracellular level of ROS increases, allowing the ISCs to proliferate (Hochmuth et al., 2011). Therefore, Keap1 and Nrf2 seem to control a shift from a resting, largely quiescent state of ISCs to a condition of active proliferation.
Mitochondrial Regulation of the ISCs of the Hindgut
Morphologically, the hindgut of adult Drosophila can be divided into four distinct regions. In the anterior-most part, adjacent to the midgut is the hindgut proliferation zone (HPZ). This is followed by the pylorus, the ileum, and the rectum. It has been documented that the HPZ contains a narrow band of ISCs that proliferates and differentiates into enterocytes to repair the pylorus in response to injury (Takashima et al., 2008; Fox and Spradling, 2009). While these ISCs have very few mitochondria that are round, small, and devoid of cristae, the differentiated adult hindgut enterocytes are characterized by densely packed mitochondria that are highly branched (Figure 3D; Deng et al., 2018). Impairing mitochondrial fusion by knocking-down of opa-1 or marf in the ISCs blocks EC differentiation without affecting their proliferation. However, blocking mitochondrial fission either by over-expression of marf or downregulating drp1 does not lead to differentiation defects. Importantly, the block in differentiation, as observed upon knocking down opa1, can be rescued by concomitant inhibition of drp-1. One possible reason for the differentiation defect of the hindgut ISCs knocked down for opa1 is ectopic induction of JNK signaling by elevated ROS levels as scavenging ROS leads to partial rescue of opa1RNAi-associated differentiation defects by downregulating JNK activity (Deng et al., 2018). Thus, the outcome of this study demonstrates that the self-renewal of the ISCs in the hindgut is mostly independent of mitochondrial function, and a progressive fusion process is essential for EC differentiation.
Role of Mitochondria in Neural Stem Cells
Adult Drosophila usually does not have NSCs. NSCs are present in the embryonic and larval stages, and at the onset of pupariation, they undergo growth restriction accompanied by terminal differentiation or apoptosis (Homem and Knoblich, 2012). Drosophila NSCs are also known as neuroblasts (NBs). During the embryonic stage, after their formation, the NBs delaminate from the neuro-epithelium and divide asymmetrically to give rise to another NB (self-renewal) and a ganglion mother cell (GMC; Hartenstein et al., 1987). Eventually, the GMC further divides and differentiates into a pair of neurons or glia. By late embryogenesis significant population of the NBs become quiescent, only to re-enter into active cell division in the larval stages (Sousa-Nunes et al., 2011). In the larvae, NBs are primarily found in the central brain and in the ventral nerve cord (Figure 4A). The central brain consists of three types of NBs, namely type I, type II, and mushroom body neuroblasts. Type I and Type II NBs differ from each other based on their division profile. While a Type I NB undergoes stereotypic division to give rise to one NB and one GMC that further divides to produce two neurons or glia, a Type II NB divides to generate one NB and another transit-amplifying cell, termed as the intermediate neural precursor (INP). The INP undergoes maturation, following which the matured INP divides asymmetrically to produce one GMC, and another matured INP. Therefore, repeated division of the matured INP gives rise to a pool of GMCs that differentiate into neurons or glia (Figure 4A; Reichert, 2011; Harding and White, 2018).
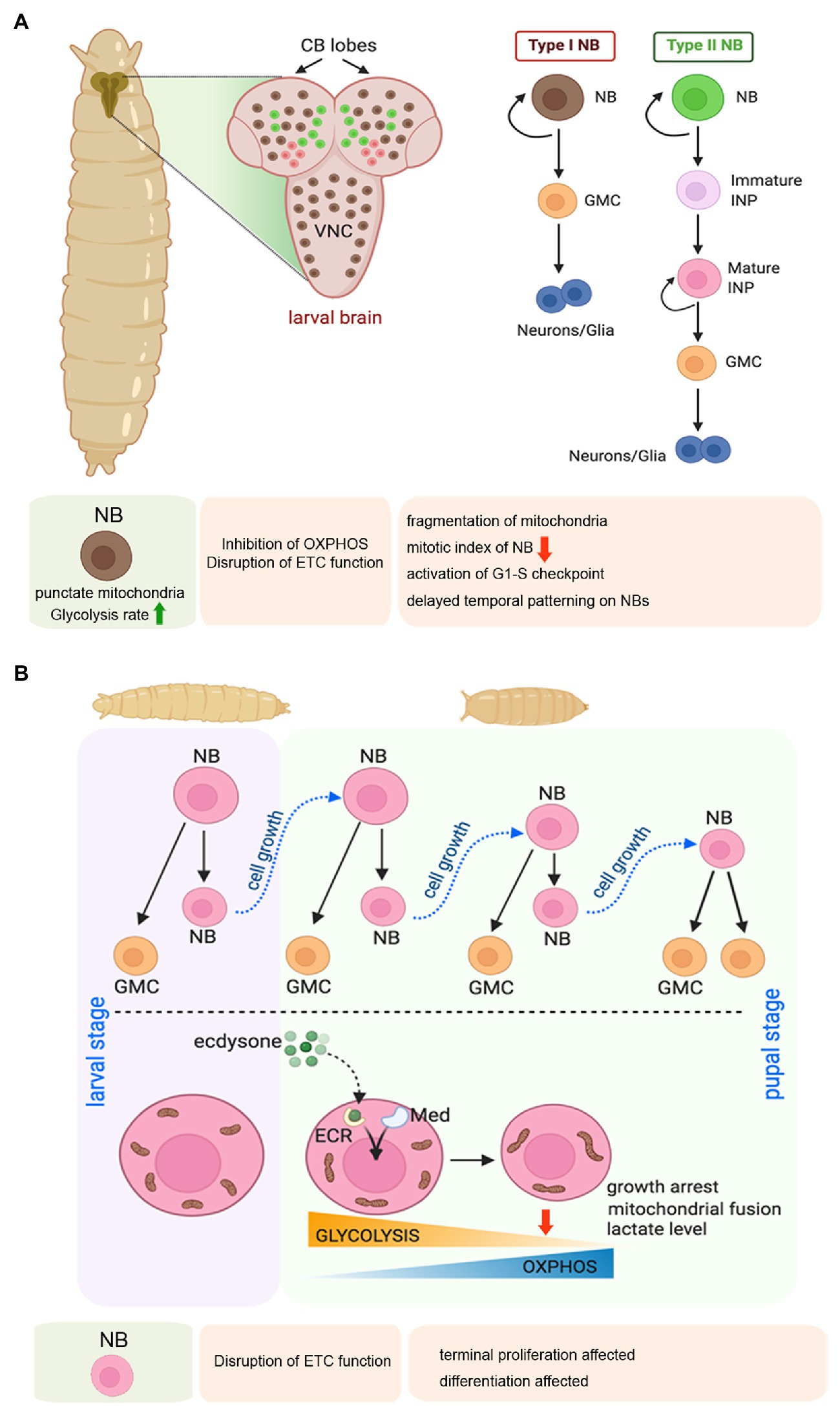
Figure 4. Mitochondrial regulation of larval neuroblast (NB) proliferation, and terminal differentiation of NBs during early pupal stage in Drosophila. (A) Schematic representation of the larval brain showing the relative position of Type 1 and Type 2 NBs, and their pattern of self-renewal and differentiation. The expanded forms of the acronyms used are provided in the text. Table showing the effects of disrupting mitochondrial function in the larval NBs. (B) Schematic representation of the changes in mitochondrial morphology and function associated with NB growth arrest and terminal differentiation at the larval to pupal transition.
One of the fascinating aspects of the Drosophila NSCs is their entry into a quiescent stage during late embryogenesis. These quiescent NSCs (qNSCs) again start dividing during early larval stages with the initiation of active feeding (Truman and Bate, 1988; Reichert, 2011). It has been observed that the qNSCs are characterized by a cytoplasmic protrusion (Endow et al., 2019), typically not observed in dividing NSCs. The cytoplasmic protrusions connect the qNSCs to the brain region known as neuropil. These cytoplasmic protrusions of qNSC are enriched with clustered mitochondria and have been proposed to be essential for maintaining the stemness in the quiescent stage. The protrusions have been proposed to connect qNSCs and its niche by forming the stem-cell-to-niche mitochondrial bridges that could sense niche signals (Endow et al., 2019). However, a detailed analysis in this intriguing finding is necessary to dissect the exact role of these cytoplasmic protrusions in qNSCs.
In larval stages, the proliferating NBs are characterized by punctate mitochondria and an increased rate of glycolysis (Figure 4A; Homem et al., 2014). Therefore, the NBs are thought to support their growth and proliferation by generating ATP through aerobic glycolysis rather than Oxphos. According to this school of thought, mitochondrial respiration is dispensable for the proliferating larval Drosophila NSCs. However, another study has evidenced that mitochondrial Oxphos is an essential contributor to the proliferation of the NBs and generates diversity through temporal patterning (van den Ameele and Brand, 2019). According to them, inhibition of Oxphos in the NBs throughout development results in the formation of smaller brains. In contrast, inhibition of glycolysis by NB-specific knockdown of phosphofructokinase (PFK), Aldolase, or phosphoglycerate kinase (PGK) does not affect brain size. Extending the thought further, they demonstrate that NB specific knockdown of Complex I and Complex V of the ETC causes mitochondrial fragmentation leading to a marked reduction of mitotic index in NBs of larval third instar VNC. Furthermore, attenuating Oxphos causes activation of the G1/S checkpoint. In turn, this delays temporal patterning of larval NSCs, implying that mitochondrial function is required for NSCs to progress from an early to a late temporal fate. Temporal patterning refers to a gradual process of differential gene expression that allows the NBs to generate progenies with diverse identities according to their developmental time (van den Ameele and Brand, 2019). Thus, temporal patterning helps in generating the diversity of neurons and glia within the CNS. Therefore, mitochondrial respiration is critical for NB proliferation and subsequent differentiation during the larval stage.
Survival and differentiation of the larval NBs also rely on their mitochondrial calcium homeostasis (Lee et al., 2016). It has been demonstrated that Miro, apart from its role in mitochondrial transport machinery, is required for NB maintenance mainly by regulating mitochondrial calcium homeostasis. Mechanistically, Polo Kinase-mediated phosphorylation promotes the localization and interaction of Miro with the calcium transporters at the ER-mitochondria contact site (ERMCS). Inactivation and overexpression of Miro, both impair the maintenance and lineage development of the NB; although through different mechanisms. While loss of Miro activity causes mitochondrial calcium depletion, and metabolic impairment leading to premature differentiation and loss of NB through a non-apoptotic mechanism, overexpression of Miro induces apoptotic response by causing mitochondrial calcium overload, oxidative stress, and activation of the apoptotic cascade. Beyond its role in NB survival, proliferation, and differentiation during larval development, a recent study has implicated the importance of mitochondria in immortalization of larval NBs during tumorigenesis (Bonnay et al., 2020). Employing single-cell transcriptomics, targeted metabolomics, and in vivo genetic screening, it has been demonstrated that extensive mitochondrial fusion mediated metabolic reprogramming is the rate-limiting step for immortalization of stem cells during tumorigenesis. The metabolic reprogramming includes a metabolic switch from glycolysis to Oxphos, and increased NAD+ biogenesis. Direct inhibition of Oxphos or that of mitochondrial fusion halts these transformed NBs in quiescence and prevents tumorigenesis.
The transition from larval to pupal phase is of prime importance for the Drosophila NBs (Figure 4B). In this period, the proliferating larval NBs enter a phase of growth restriction as they stop dividing within the first 20–30 h of pupation and differentiate or undergo apoptosis (Truman and Bate, 1988). This cell cycle exit of early pupal central brain NBs is mediated by a metabolic switch from glycolysis to Oxphos induced by the Ecdysone hormone and Mediator complex (Homem et al., 2014). This transition is associated with a dramatic shift in mitochondrial morphology. While the mitochondria of larval and early pupal brains show a punctate morphology, 8–10 h after pupa formation, the mitochondrial morphology changes to a more fused form, and making a reticular network. This change is accompanied by a marked drop in lactate levels, indicating a switch from glycolysis to Oxphos. Two independent studies (Homem et al., 2014; van den Ameele and Brand, 2019) have demonstrated that depletion of subunits of Complex I, III, IV, or V of the mitochondrial ETC in NSCs prevents their termination of proliferation and timely differentiation at the onset of pupal life. However, a difference exists in their interpretation of the underlying mechanism. Whereas one group suggests that Oxphos depletes metabolites for biosynthesis and reduces growth and induces NBs to stop proliferating in pupae (Homem et al., 2014), the other group argues that the termination of NB proliferation is a consequence of the temporal patterning defects caused by Oxphos dysfunction (van den Ameele and Brand, 2019). Nonetheless, both studies implicate the importance of Oxphos in cell cycle exit and terminal differentiation of the Drosophila NSCs.
Role of Mitochondria in Hemocyte Precursors
The process of hematopoiesis occurs in two waves in Drosophila. The first wave of hematopoiesis, referred to as the primitive hematopoiesis, occurs in the embryonic head mesoderm, giving rise to both circulating and sessile population of blood cells present throughout the life span of the flies (Tepass et al., 1994). The second wave of hematopoiesis or the definitive hematopoiesis occurs in a defined multi-lobed larval hematopoietic organ, the lymph gland (LG; Jung et al., 2005), which develops from the cardiogenic mesoderm during the embryonic period (Mandal et al., 2004). The founder cells of the lymph gland show several resemblances to the Aorta-Gonad-Mesonephros (AGM) HSCs (Figure 5A; Mandal et al., 2004; Dey et al., 2016). For their transient presence in the embryonic and first instar larval stages, these HSCs depend on the Decapentaplegic (Dpp) signal from the hematopoietic niche. Eventually, the HSCs divide and give rise to stem-like hemocyte progenitors (Dey et al., 2016). The role of mitochondria or metabolism in this transient population of HSCs is yet to be demonstrated.
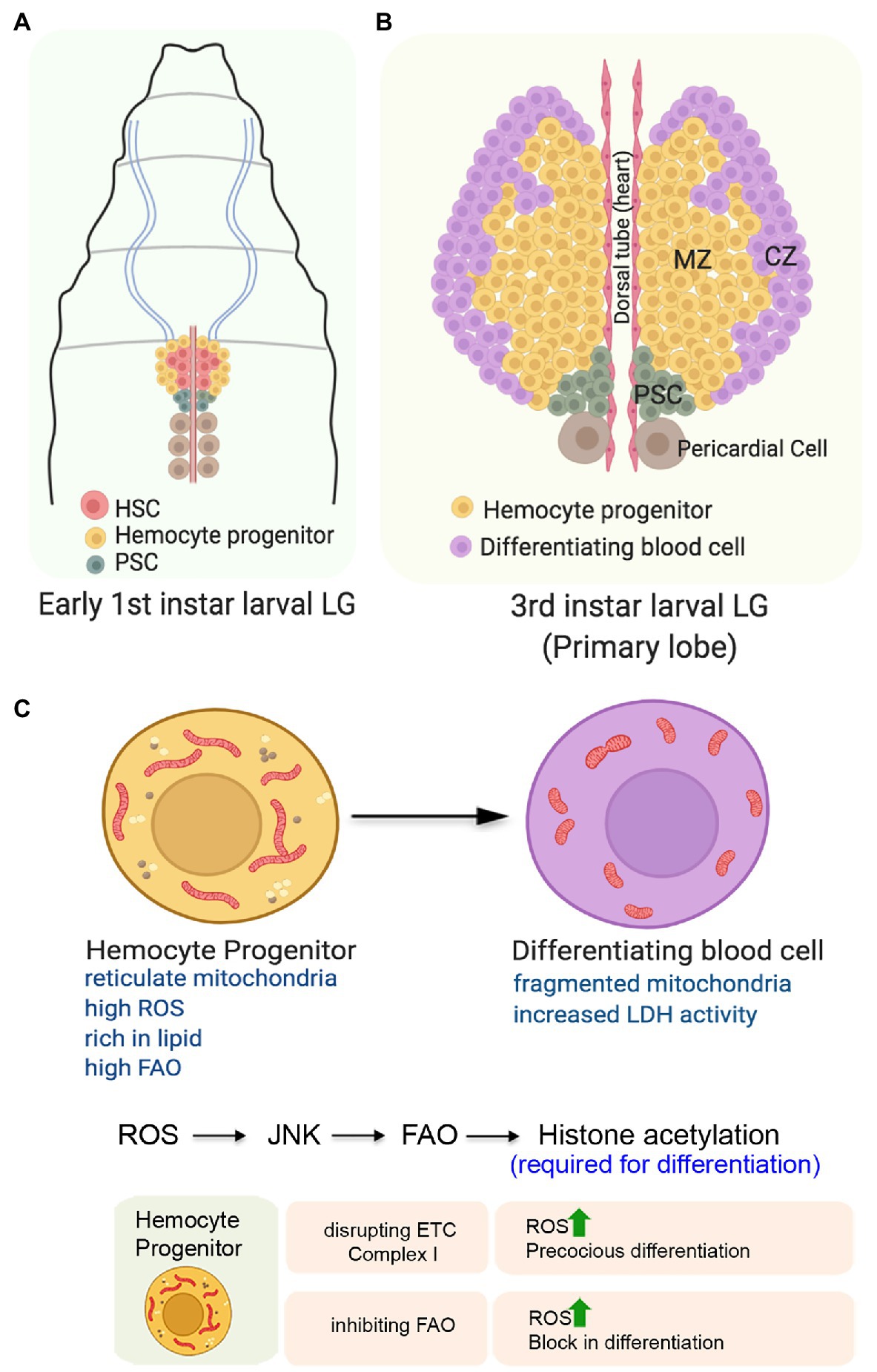
Figure 5. Role of mitochondria in determining the state and fate of hemocyte progenitors in the larval lymph gland of Drosophila. (A) Organization of the early first instar larval lymph gland showing the Hematopoietic Stem Cells (HSCs), the hemocyte progenitors, and the niche cells. (B) Schematic representation of the third instar larval lymph gland depicting the position of the different zones. The expanded forms of the acronyms used are provided in the text. (C) Changes in mitochondrial form and function and its importance for the differentiation of the hemocyte progenitors. Table showing the outcome of altering mitochondrial function in the hemocyte progenitors.
The first or the primary lobe of the third instar larval lymph gland consists of three zones (Figure 5B). The transit amplifying stem-like hemocyte progenitors present in the central region constitutes the medullary zone (MZ). These multipotent progenitor cells can give rise to all blood cell lineages that populate the gland’s outer periphery, referred to as the cortical zone (CZ). A group of 40–50 cells, located posterior to both of these zones forms the Posterior Signaling Center (PSC) that serves as the niche. Through an intricate regulatory network, the PSC maintains the homeostasis between the progenitors and the differentiating hemocytes (Evans et al., 2003; Banerjee et al., 2019).
The hemocyte progenitors in the primary lobes of the late third instar larval lymph gland have a fused and extensive reticular network of mitochondria (Figure 5C). Compared to the progenitors, the differentiating hemocytes with less reticular mitochondria, higher glucose uptake potential, and increased LDH enzymatic activity demonstrate their high glycolytic dependence (Tiwari et al., 2020). Though not established, this unexpected glycolytic dependence of the differentiating hemocytes might be because of their proliferative state. These differentiating hemocytes which demonstrate increased rate of cell division may rely more on glycolysis, rather than Oxphos. Together, these results suggest a metabolic dichotomy wherein differentition of the hemocyte precursors is associated with a shift from mitochondrial Oxphos to glycolysis.
Intriguingly, under normal physiological conditions, the hemocyte progenitors are characterized by elevated levels of ROS (Owusu-Ansah and Banerjee, 2009). It is believed that the increased levels of ROS actually primes these progenitors for differentiation as genetically scavenging ROS by overexpressing antioxidant protein Gtpx1 leads to a block in differentiation. Conversely, a further increase in ROS levels either in SOD2 hypomorphic mutants or by targeted knockdown of ETC Complex I subunits ND75 and ND42 leads to precocious differentiation of the hemocyte progenitors (Figure 5B). ROS mediated differentiation of the hemocytes is achieved by downregulation of the Polycomb group of genes in a JNK and FOXO dependent manner (Owusu-Ansah and Banerjee, 2009). In an independent study, it has been demonstrated that mitochondrial ROS promotes differentiation of hemocyte progenitors by reducing the levels of E-cadherin (Gao et al., 2014). Knocking down of the anti-oxidant genes, Sod2 and Catalase lead to a reduction in the levels of Shotgun protein (the fly homolog of E-cadherin) generally expressed at a relatively higher level in the MZ. Temporally, the drop in Shotgun levels precedes the loss of Odd-skipped-expressing hemocyte precursors. In contrary, over-expression of Shotgun prevents the hemocyte progenitor differentiation even under conditions of paraquat-induced oxidative stress.
A very recent study has provided an unexpected directionality in our understanding of the importance of mitochondrial function in the hemocyte progenitors (Tiwari et al., 2020). This study demonstrates that in the primary lobe of the third instar larval lymph glands, the hemocyte progenitors arrested in the G2/M phase of the cell cycle are rich in lipid content and express various FAO enzymes and Hnf4; the major transcription factor implicated in larval fat mobilization and FAO. Inhibiting mitochondrial FAO by genetic or pharmacological means leads to a block in the differentiation of hemocyte progenitors despite having high ROS levels in them (Figure 5B). Besides impeding differentiation, inhibiting FAO also disrupts the G2/M arrest. On the other hand, the upregulation of FAO in hemocyte progenitors leads to precocious G2/M arrest and differentiation. The mechanistic basis of FAO dependent hemocyte progenitor differentiation involves the requirement of Acetyl-CoA, the end product of FAO, for histone acetylation necessary for differentiation. Their genetic and molecular analyses reveal that FAO acts downstream to the ROS-JNK axis, as the expression of CPT1/whd (withered), the rate-limiting enzyme of FAO, is transcriptionally regulated by JNK (Tiwari et al., 2020). These findings provide an elegant connection between cellular signaling machinery and mitochondrial FAO to promote differentiation of the stem-like hemocyte progenitors in Drosophila.
Apart from the hemocyte progenitors, the niche cells present in the PSC that maintains blood progenitors can regulate the cellular immune response by sensing oxidative stress (Sinenko et al., 2011). While under normal developmental conditions, the PSC cells are characterized by low levels of ROS, attenuating mitochondrial function by knocking down ND75 causes a readily detectable increase in ROS levels. The phenotypic consequences of generating oxidative stress in the PSC cells is a significantly robust increase in the numbers of both circulating and lymph gland-resident lamellocytes, the specialized cells involved in innate immune responses. Most intriguingly, pathogen infection also induces ROS in the niche cells, resulting in the secretion of an epidermal growth factor-like cytokine signal that leads to the transdifferentiation of the circulating plasmatocytes into lamellocytes and the differentiation of hemocyte progenitors to lamellocytes.
Commonalities and Uniqueness
Lessons learned from Drosophila have provided valuable insights into our understanding of mitochondrial regulation of stem cell functions. Despite tissue-specific variations, identification of some processes portrays a typical unifying role of mitochondria in governing the stem cell state and fate. Most importantly, the unifying properties are not only restricted to different types of adult stem cells in flies, but are also apparent in a vast majority of mammalian adult stem cells. This remarkable nature of resemblance highlights the evolutionary conserved role of mitochondria in stem cell maintenance and renewal.
Akin to murine and human adult stem cells that include bone marrow derived mesenchymal stem cells (Chen et al., 2008), NSCs (Wang et al., 2010; Lange et al., 2016), and hematopoietic stem cells (Simsek et al., 2010; Miharada et al., 2011; Takubo et al., 2013; Yu et al., 2013), the ISCs and NSCs in flies are characterized by the presence of punctate mitochondria and are highly dependent on glycolysis for their maintenance and/or self-renewal. Interestingly, despite having reticulate mitochondrial network, most probably, the fly GSCs also does not rely on OXPHOS for their maintenance. Impairing ATP synthase activity bears no consequence on GSC state. Rather, the results indicate that the GSCs, in addition to glycolysis, rely on mitochondrial FAO for their maintenance. Genetic perturbation that inhibits mitochondrial fusion causes increased lipid accumulation due to defective mitochondrial FAO in both male and female GSCs. This leads to loss of stemness as they undergo precocious differentiation. In fact, the dependence of adult stem cells on mitochondrial FAO and lipid metabolism for their maintenance has also been evidenced in mammalian HSCs (Ito et al., 2012) and NSCs (Knobloch et al., 2013). Related to this, even different types of tumor-initiating stem cells or cancer stem cells (CSCs) utilize mitochondrial FAO for self-renewal and resistance to chemotherapy (Samudio et al., 2010; Chen et al., 2016). Alterations in lipid metabolism not only satisfy the energy demands and biomass production of CSCs but also play an important role in the activation of several important oncogenic signaling pathways and redox homeostasis (Carracedo et al., 2013; Park et al., 2016; Noto et al., 2017). In this context, it is important to note that, similar to the GSCs, the Drosophila hemocyte precursors of the developing larval lymph gland also harbor elongated and reticulate mitochondria and exhibit elevated levels of mitochondrial FAO. However, unlike that observed for GSCs, disrupting mitochondrial FAO impedes the differentiation of the precursors. In terms of changes in mitochondrial morphology associated with differentiation, the hemocyte precursors also stand out from other stem cells. While differentiation of the stem cells, in most instances, is associated with the gradual fusion of mitochondria forming a reticular network, the differentiating hemocytes in Drosophila have isolated and less reticulate mitochondria compared to their precursors.
Mitophagy refers to the process of selective degradation of deleterious/damaged mitochondria by autophagy and thus, holds a pivotal position for mitochondrial quality control in a cell. Accumulating evidence suggests that mitophagy related processes are vital for stem cell maintenance and homeostasis. Mitophagy mediated regulation of stem cell function has been very well documented in Drosophila female germline. Since the offsprings inherit most of their mitochondria from their mother and mitochondrial biogenesis does not initiate early during embryonic development, the quality and the number of mitochondria contributed by the mother in the egg is of prime importance. To achieve this, a robust system of mitochondrial quality control exists in the female germarium, wherein the fragmented mitochondria with low ATP signatures are earmarked for degradation and removed by the recruitment of mitophagy components Atg1 and BNIP3. Mitophagy in ISCs has also been implicated in maintaining the healthy complement of mitochondria and age-related downregulation of mitophagy components Pink1/Parkin causes accumulation of damaged mitochondria in ISCs-EBs, which alters their redox state and leads to their senescence.
Studies in the recent past have implicated mitochondrial ROS as an important factor to regulate stem cell activity. In adult stem cells, this role changes in a context dependent manner. For instance, osteogenic induction of human bone marrow-derived MSCs is associated with a reduction in the ROS level (Chen et al., 2008); whereas ROS generated by Complex III of the mitochondrial ETC is required to initiate adipocyte differentiation in primary human MSCs (Tormos et al., 2011). In flies also the effects of altered ROS levels vary from one adult stem cell type to another. While elevated ROS levels in male GSCs as well as in the hemocyte progenitors of the larval lymph gland lead to their precocious differentiation, increased ROS levels block differentiation of hindgut ISCs. Age-dependent impact of ROS on stem cell fate is also evidenced in the ISCs. Overproliferation and misdifferentiation of ISCs with a concomitant increase in the number of EBs, leading to epithelial dysplasia are an outcome of increased ROS.
Conclusion
In this review, we have put together the in vivo evidence that establishes how mitochondria influence stem cell function across a range of different tissue contexts in Drosophila. Besides highlighting the importance of mitochondrial morphology and dynamics in ascertaining the stemness and differentiation potential of a diverse array of stem cells, the outcome of these studies shed light on the changes in cellular redox state triggered by mitochondrial ROS responsible for impacting stem cell proliferation, differentiation, and senescence. Furthermore, these studies provide compelling evidence for a robust mechanism that ensures mitochondrial quality control, specifically during the onset of GSC differentiation in female flies. Despite the progress made, this research field is still in its infancy, as several issues need to be addressed. While, in some instances, the critical retrograde signaling pathways that control the process are still in the dark, in some other cases, the mechanisms that underpin the heterogeneity of mitochondrial control in different stem cell types remain undefined. Likewise, it is not evident what induces the age-dependent changes in mitochondrial structure and function that lead to stem cell aging and senescence. It is also essential to unveil the critical signaling pathways that mediate the senescence in response to mitochondrial dysfunction. Indeed, it would be intriguing to figure out whether the mechanistic basis for this process is identical in all stem cell populations or unique for each stem cell type.
With a trove of advanced genetic tools, easy accessibility of different types of stem cells, and advanced imaging techniques, Drosophila holds the promise to address most of these issues in the future. Application of the genetically encoded fluorescent probes, such as roGFPmito (Soberanes et al., 2009) and mitoTimer (Laker et al., 2014), would also help explore the mitochondrial oxidation state and mitochondrial protein turnover/segregation in defining the stem cell state. The advent of single-cell technologies, such as single-cell RNA sequencing, combined OMICS approaches, and the use of emerging sophisticated gene-editing methods would offer an unprecedented opportunity for investigations into the endogenous heterogeneity of mitochondrial regulation of stem cell behavior. Unraveling the mechanisms in flies might help us modulate mitochondrial function as effective strategies in regenerative medicine to control stem cell proliferation, activation, and aging.
Author Contributions
ST and SM were involved in conceptualization, design, and writing of the manuscript. SM prepared the models in collaboration with Lolitika Mandal. Both the authors contributed to the article and approved the submitted version.
Conflict of Interest
The authors declare that the research was conducted in the absence of any commercial or financial relationships that could be construed as a potential conflict of interest.
Acknowledgments
We thank Lolitika Mandal and other members of the laboratory for their insightful comments and suggestions on the manuscript. Models for this article have been “created with BioRender.com” by SM and LM. We sincerely apologize to the authors whose work we could not cite due to space constraints.
References
Amartuvshin, O., Lin, C. H., Hsu, S. C., Kao, S. H., Chen, A., Tang, W. C., et al. (2020). Aging shifts mitochondrial dynamics toward fission to promote germline stem cell loss. Aging Cell 19:e13191. doi: 10.1111/acel.13191
Bahat, A., and Gross, A. (2019). Mitochondrial plasticity in cell fate regulation. J. Biol. Chem. 294, 13852–13863. doi: 10.1074/jbc.REV118.000828
Banerjee, U., Girard, J. R., Goins, L. M., and Spratford, C. M. (2019). Drosophila as a genetic model for hematopoiesis. Genetics 211, 367–417. doi: 10.1534/genetics.118.300223
Barbas, J. A., Hämmerle, B., Ferrús, A., Ortuño-Sahagún, D., and Torroja, L. (1998). scully, an essential gene of Drosophila, is homologous to mammalian mitochondrial type II l-3-hydroxyacyl-CoA dehydrogenase/amyloid-β peptide-binding protein. J. Cell Biol. 141, 1009–1017.
Barker, N., Bartfeld, S., and Clevers, H. (2010). Tissue-resident adult stem cell populations of rapidly self-renewing organs. Cell Stem Cell 7, 656–670. doi: 10.1016/j.stem.2010.11.016
Biteau, B., Hochmuth, C. E., and Jasper, H. (2008). JNK activity in somatic stem cells causes loss of tissue homeostasis in the aging Drosophila gut. Cell Stem Cell 3, 442–455. doi: 10.1016/j.stem.2008.07.024
Biteau, B., and Jasper, H. (2014). Slit/Robo signaling regulates cell fate decisions in the intestinal stem cell lineage of Drosophila. Cell Rep. 7, 1867–1875. doi: 10.1016/j.celrep.2014.05.024
Bonnay, F., Veloso, A., Steinmann, V., Kocher, T., Abdusselamoglu, M. D., Bajaj, S., et al. (2020). Oxidative metabolism drives immortalization of neural stem cells during tumorigenesis. Cell 182, 1490–1507.e1419. doi: 10.1016/j.cell.2020.07.039
Bricker, D. K., Taylor, E. B., Schell, J. C., Orsak, T., Boutron, A., Chen, Y. C., et al. (2012). A mitochondrial pyruvate carrier required for pyruvate uptake in yeast, Drosophila, and humans. Science 337, 96–100. doi: 10.1126/science.1218099
Buchon, N., Broderick, N. A., Chakrabarti, S., and Lemaitre, B. (2009). Invasive and indigenous microbiota impact intestinal stem cell activity through multiple pathways in Drosophila. Genes Dev. 23, 2333–2344. doi: 10.1101/gad.1827009
Carracedo, A., Cantley, L. C., and Pandolfi, P. P. (2013). Cancer metabolism: fatty acid oxidation in the limelight. Nat. Rev. Cancer 13, 227–232. doi: 10.1038/nrc3483
Casali, A., and Batlle, E. (2009). Intestinal stem cells in mammals and Drosophila. Cell Stem Cell 4, 124–127. doi: 10.1016/j.stem.2009.01.009
Chacon-Martinez, C. A., Koester, J., and Wickstrom, S. A. (2018). Signaling in the stem cell niche: regulating cell fate, function and plasticity. Development 145:dev165399. doi: 10.1242/dev.165399
Chaturvedi, D., Reichert, H., Gunage, R. D., and Vijay Raghavan, K. (2017). Identification and functional characterization of muscle satellite cells in Drosophila. elife 6:e30107. doi: 10.7554/eLife.30107
Chen, C. T., Shih, Y. R., Kuo, T. K., Lee, O. K., and Wei, Y. H. (2008). Coordinated changes of mitochondrial biogenesis and antioxidant enzymes during osteogenic differentiation of human mesenchymal stem cells. Stem Cells 26, 960–968. doi: 10.1634/stemcells.2007-0509
Chen, C. L., Uthaya Kumar, D. B., Punj, V., Xu, J., Sher, L., Tahara, S. M., et al. (2016). NANOG metabolically reprograms tumor-initiating stem-like cells through tumorigenic changes in oxidative phosphorylation and fatty acid metabolism. Cell Metab. 23, 206–219. doi: 10.1016/j.cmet.2015.12.004
Cheung, T. H., and Rando, T. A. (2013). Molecular regulation of stem cell quiescence. Nat. Rev. Mol. Cell Biol. 14, 329–340. doi: 10.1038/nrm3591
Choi, N.-H., Kim, J.-G., Yang, D.-J., Kim, Y.-S., and Yoo, M.-A. (2008). Age-related changes in Drosophila midgut are associated with PVF2, a PDGF/VEGF-like growth factor. Aging Cell 7, 318–334. doi: 10.1111/j.1474-9726.2008.00380.x
Clark, I. E., Dodson, M. W., Jiang, C., Cao, J. H., Huh, J. R., Seol, J. H., et al. (2006). Drosophila pink1 is required for mitochondrial function and interacts genetically with parkin. Nature 441, 1162–1166. doi: 10.1038/nature04779
Clevers, H., and Watt, F. M. (2018). Defining adult stem cells by function, not by phenotype. Annu. Rev. Biochem. 87, 1015–1027. doi: 10.1146/annurev-biochem-062917-012341
Cox, R. T. (2003). A Balbiani body and the fusome mediate mitochondrial inheritance during Drosophila oogenesis. Development 130, 1579–1590. doi: 10.1242/dev.00365
de Cuevas, M., and Matunis, E. L. (2011). The stem cell niche: lessons from the Drosophila testis. Development 138, 2861–2869. doi: 10.1242/dev.056242
Deng, H., Takashima, S., Paul, M., Guo, M., and Hartenstein, V. (2018). Mitochondrial dynamics regulates Drosophila intestinal stem cell differentiation. Cell Death Dis. 4:17. doi: 10.1038/s41420-018-0083-0
Dey, N. S., Ramesh, P., Chugh, M., Mandal, S., and Mandal, L. (2016). Dpp dependent hematopoietic stem cells give rise to Hh dependent blood progenitors in larval lymph gland of Drosophila. elife 5:e18295. doi: 10.7554/eLife.18295
DiTroia, S. P., Percharde, M., Guerquin, M.-J., Wall, E., Collignon, E., Ebata, K. T., et al. (2019). Maternal vitamin C regulates reprogramming of DNA methylation and germline development. Nature 573, 271–275. doi: 10.1038/s41586-019-1536-1
Drummond-Barbosa, D. (2008). Stem cells, their niches and the systemic environment: an aging network. Genetics 180, 1787–1797. doi: 10.1534/genetics.108.098244
Drummond-Barbosa, D. (2019). Local and physiological control of germline stem cell lineages in Drosophila melanogaster. Genetics 213, 9–26. doi: 10.1534/genetics.119.300234
Drummond-Barbosa, D., and Spradling, A. C. (2001). Stem cells and their progeny respond to nutritional changes during Drosophila oogenesis. Dev. Biol. 231, 265–278. doi: 10.1006/dbio.2000.0135
Endow, S. A., Miller, S. E., and Ly, P. T. (2019). Mitochondria-enriched protrusions are associated with brain and intestinal stem cells in Drosophila. Commun. Biol. 2:427. doi: 10.1038/s42003-019-0671-4
Evans, C. J., Hartenstein, V., and Banerjee, U. (2003). Thicker than blood. Dev. Cell 5, 673–690. doi: 10.1016/S1534-5807(03)00335-6
Ferraro, F., Celso, C. L., and Scadden, D. (2010). Adult stem cels and their niches. Adv. Exp. Med. Biol. 695, 155–168. doi: 10.1007/978-1-4419-7037-4_11
Fox, D. T., and Spradling, A. C. (2009). The Drosophila hindgut lacks constitutively active adult stem cells but proliferates in response to tissue damage. Cell Stem Cell 5, 290–297. doi: 10.1016/j.stem.2009.06.003
Fuchs, E., and Chen, T. (2012). A matter of life and death: self-renewal in stem cells. EMBO Rep. 14, 39–48. doi: 10.1038/embor.2012.197
Fuchs, E., Li, Q., Mundschau, G., Bauer, C., and Raghavan, S. (2000). Conditional ablation of β1 integrin in skin. J. Cell Biol. 150, 1149–1160. doi: 10.1083/jcb.150.5.1149
Fuller, M. T., and Spradling, A. C. (2007). Male and female Drosophila germline stem cells: two versions of immortality. Science 316, 402–404. doi: 10.1126/science.1140861
Gao, H., Wu, X., Simon, L., and Fossett, N. (2014). Antioxidants maintain E-cadherin levels to limit Drosophila prohemocyte differentiation. PLoS One 9:e107768. doi: 10.1371/journal.pone.0112508
Goldman, J. A., and Poss, K. D. (2020). Gene regulatory programmes of tissue regeneration. Nat. Rev. Genet. 21, 511–525. doi: 10.1038/s41576-020-0239-7
Goulas, S., Conder, R., and Knoblich, J. A. (2012). The par complex and integrins direct asymmetric cell division in adult intestinal stem cells. Cell Stem Cell 11, 529–540. doi: 10.1016/j.stem.2012.06.017
Green, D. R., and Reed, J. C. (1998). Mitochondria and apoptosis. Science 281, 1309–1312. doi: 10.1126/science.281.5381.1309
Greenspan, L. J., de Cuevas, M., and Matunis, E. (2015). Genetics of gonadal stem cell renewal. Annu. Rev. Cell Dev. Biol. 31, 291–315. doi: 10.1146/annurev-cellbio-100913-013344
Hales, K. G., and Fuller, M. T. (1997). Developmentally regulated mitochondrial fusion mediated by a conserved, novel, predicted GTPase. Cell 90, 121–129. doi: 10.1016/S0092-8674(00)80319-0
Harding, K., and White, K. (2018). Drosophila as a model for developmental biology: stem cell-fate decisions in the developing nervous system. J. Dev. Biol. 6:25. doi: 10.3390/jdb6040025
Hartenstein, V., Rudloff, E., and Campos-Ortega, J. A. (1987). The pattern of proliferation of the neuroblasts in the wild-type embryo of Drosophila melanogaster. Rouxs Arch. Dev. Biol. 196, 473–485.
Harvey, A., Caretti, G., Moresi, V., Renzini, A., and Adamo, S. (2019). Interplay between metabolites and the epigenome in regulating embryonic and adult stem cell potency and maintenance. Stem Cell Rep. 13, 573–589. doi: 10.1016/j.stemcr.2019.09.003
Herzig, S., Raemy, E., Montessuit, S., Veuthey, J. L., Zamboni, N., Westermann, B., et al. (2012). Identification and functional expression of the mitochondrial pyruvate carrier. Science 337, 93–96. doi: 10.1126/science.1218530
Hinge, A., He, J., Bartram, J., Javier, J., Xu, J., Fjellman, E., et al. (2020). Asymmetrically segregated mitochondria provide cellular memory of hematopoietic stem cell replicative history and drive HSC attrition. Cell Stem Cell 26, 420–430.e426. doi: 10.1016/j.stem.2020.01.016
Hochmuth, C. E., Biteau, B., Bohmann, D., and Jasper, H. (2011). Redox regulation by Keap1 and Nrf2 controls intestinal stem cell proliferation in Drosophila. Cell Stem Cell 8, 188–199. doi: 10.1016/j.stem.2010.12.006
Homem, C. C. F., and Knoblich, J. A. (2012). Drosophila neuroblasts: a model for stem cell biology. Development 139, 4297–4310. doi: 10.1242/dev.080515
Homem, C. C. F., Steinmann, V., Burkard, T. R., Jais, A., Esterbauer, H., and Knoblich, J. A. (2014). Ecdysone and mediator change energy metabolism to terminate proliferation in Drosophila neural stem cells. Cell 158, 874–888. doi: 10.1016/j.cell.2014.06.024
Huelsken, J., Vogel, R., Erdmann, B., Cotsarelis, G., and Birchmeier, W. (2001). β-Catenin controls hair follicle morphogenesis and stem cell differentiation in the skin. Cell 105, 533–545. doi: 10.1016/S0092-8674(01)00336-1
Hurd, T. R., Herrmann, B., Sauerwald, J., Sanny, J., Grosch, M., and Lehmann, R. (2016). Long Oskar controls mitochondrial inheritance in Drosophila melanogaster. Dev. Cell 39, 560–571. doi: 10.1016/j.devcel.2016.11.004
Ito, K., Carracedo, A., Weiss, D., Arai, F., Ala, U., Avigan, D. E., et al. (2012). A PML-PPAR-delta pathway for fatty acid oxidation regulates hematopoietic stem cell maintenance. Nat. Med. 18, 1350–1358. doi: 10.1038/nm.2882
Jennings, B. H. (2011). Drosophila – a versatile model in biology & medicine. Mater. Today 14, 190–195. doi: 10.1016/S1369-7021(11)70113-4
Jin, Y., Patel, P. H., Kohlmaier, A., Pavlovic, B., Zhang, C., and Edgar, B. A. (2017). Intestinal stem cell pool regulation in Drosophila. Stem Cell Rep. 8, 1479–1487. doi: 10.1016/j.stemcr.2017.04.002
Jones, D. L., Ellisman, M. H., Perkins, G. A., and Koehler, C. L. (2017). Pink1 and Parkin regulate Drosophila intestinal stem cell proliferation during stress and aging. J. Cell Biol. 216, 2315–2327. doi: 10.1083/jcb.201610036
Jung, S. H., Evans, C. J., Uemura, C., and Banerjee, U. (2005). The Drosophila lymph gland as a developmental model of hematopoiesis. Development 132, 2521–2533. doi: 10.1242/dev.01837
Kahn, J., Shwartz, Y., Blitz, E., Krief, S., Sharir, A., Breitel, D. A., et al. (2009). Muscle contraction is necessary to maintain joint progenitor cell fate. Dev. Cell 16, 734–743. doi: 10.1016/j.devcel.2009.04.013
Kahney, E. W., Snedeker, J. C., and Chen, X. (2019). Regulation of Drosophila germline stem cells. Curr. Opin. Cell Biol. 60, 27–35. doi: 10.1016/j.ceb.2019.03.008
Kiger, A. A. (2001). Stem cell self-renewal specified by JAK-STAT activation in response to a support cell cue. Science 294, 2542–2545. doi: 10.1126/science.1066707
Kimble, J. E., and White, J. G. (1981). On the control of germ cell development in Caenorhabditis elegans. Dev. Biol. 81, 208–219. doi: 10.1016/0012-1606(81)90284-0
Kirilly, D., and Xie, T. (2007). The Drosophila ovary: an active stem cell community. Cell Res. 17, 15–25. doi: 10.1038/sj.cr.7310123
Knobloch, M., Braun, S. M., Zurkirchen, L., von Schoultz, C., Zamboni, N., Arauzo-Bravo, M. J., et al. (2013). Metabolic control of adult neural stem cell activity by Fasn-dependent lipogenesis. Nature 493, 226–230. doi: 10.1038/nature11689
Laker, R. C., Xu, P., Ryall, K. A., Sujkowski, A., Kenwood, B. M., Chain, K. H., et al. (2014). A novel MitoTimer reporter gene for mitochondrial content, structure, stress, and damagein vivo. J. Biol. Chem. 289, 12005–12015. doi: 10.1074/jbc.M113.530527
Lange, C., Turrero Garcia, M., Decimo, I., Bifari, F., Eelen, G., Quaegebeur, A., et al. (2016). Relief of hypoxia by angiogenesis promotes neural stem cell differentiation by targeting glycolysis. EMBO J. 35, 924–941. doi: 10.15252/embj.201592372
Lee, S., Lee, K. S., Huh, S., Liu, S., Lee, D. Y., Hong, S. H., et al. (2016). Polo kinase phosphorylates Miro to control ER-mitochondria contact sites and mitochondrial Ca(2+) homeostasis in neural stem cell development. Dev. Cell 37, 174–189. doi: 10.1016/j.devcel.2016.03.023
Lieber, T., Jeedigunta, S. P., Palozzi, J. M., Lehmann, R., and Hurd, T. R. (2019). Mitochondrial fragmentation drives selective removal of deleterious mtDNA in the germline. Nature 570, 380–384. doi: 10.1038/s41586-019-1213-4
Lisowski, P., Kannan, P., Mlody, B., and Prigione, A. (2018). Mitochondria and the dynamic control of stem cell homeostasis. EMBO Rep. 19:e45432. doi: 10.15252/embr.201745432
Liu, Z., and Butow, R. A. (2006). Mitochondrial retrograde signaling. Annu. Rev. Genet. 40, 159–185. doi: 10.1146/annurev.genet.40.110405.090613
Losick, V. P., Morris, L. X., Fox, D. T., and Spradling, A. (2011). Drosophila stem cell niches: a decade of discovery suggests a unified view of stem cell regulation. Dev. Cell 21, 159–171. doi: 10.1016/j.devcel.2011.06.018
Mandal, L., Banerjee, U., and Hartenstein, V. (2004). Evidence for a fruit fly hemangioblast and similarities between lymph-gland hematopoiesis in fruit fly and mammal aorta-gonadal-mesonephros mesoderm. Nat. Genet. 36, 1019–1023. doi: 10.1038/ng1404
Mandal, S., Lindgren, A. G., Srivastava, A. S., Clark, A. T., and Banerjee, U. (2011). Mitochondrial function controls proliferation and early differentiation potential of embryonic stem cells. Stem Cells 29, 486–495. doi: 10.1002/stem.590
Margolis, J., and Spradling, A. (1995). Identification and behavior of epithelial stem cells in the Drosophila ovary. Development 121, 3797–3807.
McBride, H. M., Neuspiel, M., and Wasiak, S. (2006). Mitochondria: more than just a powerhouse. Curr. Biol. 16, R551–R560. doi: 10.1016/j.cub.2006.06.054
McKearin, D. M., and Spradling, A. C. (1990). Bag-of-marbles: a Drosophila gene required to initiate both male and female gametogenesis. Genes Dev. 4, 2242–2251. doi: 10.1101/gad.4.12b.2242
Micchelli, C. A., and Perrimon, N. (2005). Evidence that stem cells reside in the adult Drosophila midgut epithelium. Nature 439, 475–479. doi: 10.1038/nature04371
Miguel-Aliaga, I., Jasper, H., and Lemaitre, B. (2018). Anatomy and physiology of the digestive tract of Drosophila melanogaster. Genetics 210, 357–396. doi: 10.1534/genetics.118.300224
Miharada, K., Karlsson, G., Rehn, M., Rorby, E., Siva, K., Cammenga, J., et al. (2011). Cripto regulates hematopoietic stem cells as a hypoxic-niche-related factor through cell surface receptor GRP78. Cell Stem Cell 9, 330–344. doi: 10.1016/j.stem.2011.07.016
Morrison, S. J., and Spradling, A. C. (2008). Stem cells and niches: mechanisms that promote stem cell maintenance throughout life. Cell 132, 598–611. doi: 10.1016/j.cell.2008.01.038
Murphy, M. P. (2008). How mitochondria produce reactive oxygen species. Biochem. J. 417, 1–13. doi: 10.1042/BJ20081386
Naik, S., Larsen, S. B., Cowley, C. J., and Fuchs, E. (2018). Two to tango: dialog between immunity and stem cells in health and disease. Cell 175, 908–920. doi: 10.1016/j.cell.2018.08.071
Newmeyer, D. D., and Ferguson-Miller, S. (2003). Mitochondria: releasing power for life and unleashing the machineries of death. Cell 112, 481–490. doi: 10.1016/S0092-8674(03)00116-8
Noto, A., De Vitis, C., Pisanu, M. E., Roscilli, G., Ricci, G., Catizone, A., et al. (2017). Stearoyl-CoA-desaturase 1 regulates lung cancer stemness via stabilization and nuclear localization of YAP/TAZ. Oncogene 36, 4573–4584. doi: 10.1038/onc.2017.75
Nunnari, J., and Suomalainen, A. (2012). Mitochondria: in sickness and in health. Cell 148, 1145–1159. doi: 10.1016/j.cell.2012.02.035
Ochocki, J. D., and Simon, M. C. (2013). Nutrient-sensing pathways and metabolic regulation in stem cells. J. Cell Biol. 203, 23–33. doi: 10.1083/jcb.201303110
Ohlstein, B., and Spradling, A. (2005). The adult Drosophila posterior midgut is maintained by pluripotent stem cells. Nature 439, 470–474. doi: 10.1038/nature04333
Owusu-Ansah, E., and Banerjee, U. (2009). Reactive oxygen species prime Drosophila haematopoietic progenitors for differentiation. Nature 461, 537–541. doi: 10.1038/nature08313
Papagiannouli, F. (2014). “Male Stem Cell Niche and Spermatogenesis in the Drosophila Testis — A Tale of Germline-Soma Communication” in Adult Stem Cell Niches. ed. S. Wislet-Gendebien (IntechOpen). doi: 10.5772/58756
Park, J. H., Vithayathil, S., Kumar, S., Sung, P. L., Dobrolecki, L. E., Putluri, V., et al. (2016). Fatty acid oxidation-driven Src links mitochondrial energy reprogramming and oncogenic properties in triple-negative breast cancer. Cell Rep. 14, 2154–2165. doi: 10.1016/j.celrep.2016.02.004
Pearce, E. L., and Pearce, E. J. (2013). Metabolic pathways in immune cell activation and quiescence. Immunity 38, 633–643. doi: 10.1016/j.immuni.2013.04.005
Post, Y., and Clevers, H. (2019). Defining adult stem cell function at its simplest: the ability to replace lost cells through mitosis. Cell Stem Cell 25, 174–183. doi: 10.1016/j.stem.2019.07.002
Reichert, H. (2011). Drosophila neural stem cells: cell cycle control of self-renewal, differentiation, and termination in brain development. Results Probl. Cell Differ. 53, 529–546. doi: 10.1007/978-3-642-19065-0_21
Ren, Q., Zhang, F., and Xu, H. (2017). Proliferation cycle causes age dependent mitochondrial deficiencies and contributes to the aging of stem cells. Genes (Basel) 8:397. doi: 10.3390/genes8120397
Rera, M., Bahadorani, S., Cho, J., Koehler, C. L., Ulgherait, M., Hur, J. H., et al. (2011). Modulation of longevity and tissue homeostasis by the Drosophila PGC-1 homolog. Cell Metab. 14, 623–634. doi: 10.1016/j.cmet.2011.09.013
Rezza, A., Sennett, R., and Rendl, M. (2014). Adult stem cell niches: cellular and molecular components. Curr. Top. Dev. Biol. 107, 333–372. doi: 10.1016/B978-0-12-416022-4.00012-3
Rodriguez-Fernandez, I. A., Qi, Y., and Jasper, H. (2019). Loss of a proteostatic checkpoint in intestinal stem cells contributes to age-related epithelial dysfunction. Nat. Commun. 10:1050. doi: 10.1038/s41467-019-08982-9
Rodriguez-Fernandez, I. A., Tauc, H. M., and Jasper, H. (2020). Hallmarks of aging Drosophila intestinal stem cells. Mech. Ageing Dev. 190:111285. doi: 10.1016/j.mad.2020.111285
Rogers, R. P., and Rogina, B. (2014). Increased mitochondrial biogenesis preserves intestinal stem cell homeostasis and contributes to longevity in Indy mutant flies. Aging 6, 335–350. doi: 10.18632/aging.100658
Samudio, I., Harmancey, R., Fiegl, M., Kantarjian, H., Konopleva, M., Korchin, B., et al. (2010). Pharmacologic inhibition of fatty acid oxidation sensitizes human leukemia cells to apoptosis induction. J. Clin. Invest. 120, 142–156. doi: 10.1172/JCI38942
Sánchez Alvarado, A., and Yamanaka, S. (2014). Rethinking differentiation: stem cells, regeneration, and plasticity. Cell 157, 110–119. doi: 10.1016/j.cell.2014.02.041
Sawyer, E. M., Brunner, E. C., Hwang, Y., Ivey, L. E., Brown, O., Bannon, M., et al. (2017). Testis-specific ATP synthase peripheral stalk subunits required for tissue-specific mitochondrial morphogenesis in Drosophila. BMC Cell Biol. 18:16. doi: 10.1186/s12860-017-0132-1
Schell, J. C., Wisidagama, D. R., Bensard, C., Zhao, H., Wei, P., Tanner, J., et al. (2017). Control of intestinal stem cell function and proliferation by mitochondrial pyruvate metabolism. Nat. Cell Biol. 19, 1027–1036. doi: 10.1038/ncb3593
Schofield, R. (1978). The relationship between the spleen colony-forming cell and the haemopoietic stem cell. Blood Cells 4, 7–25.
Senos Demarco, R., and Jones, D. L. (2019). Mitochondrial fission regulates germ cell differentiation by suppressing ROS-mediated activation of epidermal growth factor signaling in the Drosophila larval testis. Sci. Rep. 9:19695. doi: 10.1038/s41598-019-55728-0
Sênos Demarco, R., Uyemura, B. S., D’Alterio, C., and Jones, D. L. (2019). Mitochondrial fusion regulates lipid homeostasis and stem cell maintenance in the Drosophila testis. Nat. Cell Biol. 21, 710–720. doi: 10.1038/s41556-019-0332-3
Shyh-Chang, N., Daley, G. Q., and Cantley, L. C. (2013). Stem cell metabolism in tissue development and aging. Development 140, 2535–2547. doi: 10.1242/dev.091777
Shyh-Chang, N., and Ng, H. H. (2017). The metabolic programming of stem cells. Genes Dev. 31, 336–346. doi: 10.1101/gad.293167.116
Simsek, T., Kocabas, F., Zheng, J., Deberardinis, R. J., Mahmoud, A. I., Olson, E. N., et al. (2010). The distinct metabolic profile of hematopoietic stem cells reflects their location in a hypoxic niche. Cell Stem Cell 7, 380–390. doi: 10.1016/j.stem.2010.07.011
Sinenko, S. A., Shim, J., and Banerjee, U. (2011). Oxidative stress in the haematopoietic niche regulates the cellular immune response in Drosophila. EMBO Rep. 13, 83–89. doi: 10.1038/embor.2011.223
Singh, S. R., Liu, W., and Hou, S. X. (2007). The adult Drosophila malpighian tubules are maintained by multipotent stem cells. Cell Stem Cell 1, 191–203. doi: 10.1016/j.stem.2007.07.003
Soberanes, S., Urich, D., Baker, C. M., Burgess, Z., Chiarella, S. E., Bell, E. L., et al. (2009). Mitochondrial complex III-generated oxidants activate ASK1 and JNK to induce alveolar epithelial cell death following exposure to particulate matter air pollution. J. Biol. Chem. 284, 2176–2186. doi: 10.1074/jbc.M808844200
Sousa-Nunes, R., Yee, L. L., and Gould, A. P. (2011). Fat cells reactivate quiescent neuroblasts via TOR and glial insulin relays in Drosophila. Nature 471, 508–512. doi: 10.1038/nature09867
Speder, P., and Brand, A. H. (2018). Systemic and local cues drive neural stem cell niche remodelling during neurogenesis in Drosophila. elife 7:e30413. doi: 10.7554/eLife.30413
Suzuki, T., Muramatsu, A., Saito, R., Iso, T., Shibata, T., Kuwata, K., et al. (2019). Molecular mechanism of cellular oxidative stress sensing by Keap1. Cell Rep. 28, 746–758.e744. doi: 10.1016/j.celrep.2019.06.047
Tait, S. W., and Green, D. R. (2012). Mitochondria and cell signalling. J. Cell Sci. 125, 807–815. doi: 10.1242/jcs.099234
Takashima, S., Mkrtchyan, M., Younossi-Hartenstein, A., Merriam, J. R., and Hartenstein, V. (2008). The behaviour of Drosophila adult hindgut stem cells is controlled by Wnt and Hh signalling. Nature 454, 651–655. doi: 10.1038/nature07156
Takubo, K., Nagamatsu, G., Kobayashi, C. I., Nakamura-Ishizu, A., Kobayashi, H., Ikeda, E., et al. (2013). Regulation of glycolysis by Pdk functions as a metabolic checkpoint for cell cycle quiescence in hematopoietic stem cells. Cell Stem Cell 12, 49–61. doi: 10.1016/j.stem.2012.10.011
Tan, S. W. S., Lee, Q. Y., Wong, B. S. E., Cai, Y., and Baeg, G. H. (2017). Redox homeostasis plays important roles in the maintenance of the Drosophila testis germline stem cells. Stem Cell Rep. 9, 342–354. doi: 10.1016/j.stemcr.2017.05.034
Tan, S. W. S., Yip, G. W., Suda, T., and Baeg, G. H. (2018). Small Maf functions in the maintenance of germline stem cells in the Drosophila testis. Redox Biol. 15, 125–134. doi: 10.1016/j.redox.2017.12.002
Teixeira, F. K., Sanchez, C. G., Hurd, T. R., Seifert, J. R. K., Czech, B., Preall, J. B., et al. (2015). ATP synthase promotes germ cell differentiation independent of oxidative phosphorylation. Nat. Cell Biol. 17, 689–696. doi: 10.1038/ncb3165
Tepass, U., Fessler, L. I., Aziz, A., and Hartenstein, V. (1994). Embryonic origin of hemocytes and their relationship to cell death in Drosophila. Development 120, 1829–1837.
Ting, X. (2013). Control of germline stem cell self-renewal and differentiation in the Drosophila ovary: concerted actions of niche signals and intrinsic factors. Wiley Interdiscip. Rev. Dev. Biol. 2, 261–273. doi: 10.1002/wdev.60
Tiwari, S. K., Toshniwal, A. G., Mandal, S., and Mandal, L. (2020). Fatty acid β-oxidation is required for the differentiation of larval hematopoietic progenitors in Drosophila. elife 9, e53247. doi: 10.7554/eLife.53247
Tokuyasu, K. T. (1975). Dynamics of spermiogenesis in Drosophila melanogaster. VI. Significance of “onion” nebenkern formation. J. Ultrastruct. Res. 53, 93–112. doi: 10.1016/S0022-5320(75)80089-X
Tormos, K. V., Anso, E., Hamanaka, R. B., Eisenbart, J., Joseph, J., Kalyanaraman, B., et al. (2011). Mitochondrial complex III ROS regulate adipocyte differentiation. Cell Metab. 14, 537–544. doi: 10.1016/j.cmet.2011.08.007
Tourmente, S., Lecher, P., Degroote, F., and Renaud, M. (1990). Mitochondrial development during Drosophila oogenesis: distribution, density and in situ RNA hybridizations. Biol. Cell. 68, 119–127. doi: 10.1016/0248-4900(90)90296-F
Truman, J. W., and Bate, M. (1988). Spatial and temporal patterns of neurogenesis in the central nervous system of Drosophila melanogaster. Dev. Biol. 125, 145–157. doi: 10.1016/0012-1606(88)90067-X
van den Ameele, J., and Brand, A. H. (2019). Neural stem cell temporal patterning and brain tumour growth rely on oxidative phosphorylation. elife 8, e47887. doi: 10.7554/eLife.47887
Vasington, F. D., and Murphy, J. V. (1962). Ca ion uptake by rat kidney mitochondria and its dependence on respiration and phosphorylation. J. Biol. Chem. 237, 2670–2677. doi: 10.1016/S0021-9258(19)73805-8
Wanet, A., Arnould, T., Najimi, M., and Renard, P. (2015). Connecting mitochondria, metabolism, and stem cell fate. Stem Cells Dev. 24, 1957–1971. doi: 10.1089/scd.2015.0117
Wang, Z. A., Huang, J., and Kalderon, D. (2012). Drosophila follicle stem cells are regulated by proliferation and niche adhesion as well as mitochondria and ROS. Nat. Commun. 3:769. doi: 10.1038/ncomms1765
Wang, Z.-H., Liu, Y., Chaitankar, V., Pirooznia, M., and Xu, H. (2019). Electron transport chain biogenesis activated by a JNK-insulin-Myc relay primes mitochondrial inheritance in Drosophila. elife 8:e49309. doi: 10.7554/eLife.49309
Wang, J., Wakeman, T. P., Lathia, J. D., Hjelmeland, A. B., Wang, X. F., White, R. R., et al. (2010). Notch promotes radioresistance of glioma stem cells. Stem Cells 28, 17–28. doi: 10.1002/stem.261
Wang, L., Zeng, X., Ryoo, H. D., and Jasper, H. (2014). Integration of UPRER and oxidative stress signaling in the control of intestinal stem cell proliferation. PLoS Genet. 10:e1004568. doi: 10.1371/journal.pgen.1004568
Westermann, B. (2010). Mitochondrial fusion and fission in cell life and death. Nat. Rev. Mol. Cell Biol. 11, 872–884. doi: 10.1038/nrm3013
Wisidagama, D. R., and Thummel, C. S. (2019). Regulation of Drosophila intestinal stem cell proliferation by enterocyte mitochondrial pyruvate metabolism. G3 9, 3623–3630. doi: 10.1534/g3.119.400633
Wu, J., Feng, L., and Hsieh, T. S. (2010). Drosophila topo IIIalpha is required for the maintenance of mitochondrial genome and male germ-line stem cells. Proc. Natl. Acad. Sci. U. S. A. 107, 6228–6233. doi: 10.1073/pnas.1001855107
Xie, T., and Spradling, A. C. (1998). Decapentaplegic is essential for the maintenance and division of germline stem cells in the Drosophila ovary. Cell 94, 251–260. doi: 10.1016/S0092-8674(00)81424-5
Yamashita, Y. M., Fuller, M. T., and Jones, D. L. (2005). Signaling in stem cell niches: lessons from the Drosophila germline. J. Cell Sci. 118, 665–672. doi: 10.1242/jcs.01680
Yu, J., Chen, B., Zheng, B., Qiao, C., Chen, X., Yan, Y., et al. (2019). ATP synthase is required for male fertility and germ cell maturation in Drosophila testes. Mol. Med. Rep. 19, 1561–1570. doi: 10.3892/mmr.2019.9834
Yu, W. M., Liu, X., Shen, J., Jovanovic, O., Pohl, E. E., Gerson, S. L., et al. (2013). Metabolic regulation by the mitochondrial phosphatase PTPMT1 is required for hematopoietic stem cell differentiation. Cell Stem Cell 12, 62–74. doi: 10.1016/j.stem.2012.11.022
Yucel, N., Wang, Y. X., Mai, T., Porpiglia, E., Lund, P. J., Markov, G., et al. (2019). Glucose metabolism drives histone acetylation landscape transitions that dictate muscle stem cell function. Cell Rep. 27, 3939–3955.e3936. doi: 10.1016/j.celrep.2019.05.092
Zhang, F., Pirooznia, M., and Xu, H. (2020). Mitochondria regulate intestinal stem cell proliferation and epithelial homeostasis through FOXO. Mol. Biol. Cell 31, 1538–1549. doi: 10.1091/mbc.E19-10-0560
Keywords:Drosophila, metabolism, mitochondria, stem cell, regulation, maintenance, differentiation
Citation: Tiwari SK and Mandal S (2021) Mitochondrial Control of Stem Cell State and Fate: Lessons From Drosophila. Front. Cell Dev. Biol. 9:606639. doi: 10.3389/fcell.2021.606639
Edited by:
Richa Rikhy, Indian Institute of Science Education and Research, Pune, IndiaReviewed by:
Tina Mukherjee, Institute for Stem Cell Science and Regenerative Medicine (inStem), IndiaKasturi Mitra, University of Alabama at Birmingham, United States
Copyright © 2021 Tiwari and Mandal. This is an open-access article distributed under the terms of the Creative Commons Attribution License (CC BY). The use, distribution or reproduction in other forums is permitted, provided the original author(s) and the copyright owner(s) are credited and that the original publication in this journal is cited, in accordance with accepted academic practice. No use, distribution or reproduction is permitted which does not comply with these terms.
*Correspondence: Sudip Mandal, c3VkaXBAaWlzZXJtb2hhbGkuYWMuaW4=
†Present address: Satish Kumar Tiwari, Singapore Immunology Network (SIgN), Agency for Science, Technology and Research (A*STAR), Biopolis, Singapore, Singapore