- 1Laboratory of Pulmonary Investigation, Carlos Chagas Filho Biophysics Institute, Federal University of Rio de Janeiro, Rio de Janeiro, Brazil
- 2National Institute of Science and Technology for Regenerative Medicine, Rio de Janeiro, Brazil
- 3Biosystems & Integrative Sciences Institute, Faculty of Sciences, University of Lisbon, Lisbon, Portugal
- 4Department of Medicine, College of Medicine, University of Vermont Larner, Burlington, VT, United States
Extracellular vesicles (EVs) have emerged as a potential therapy for several diseases. These plasma membrane-derived fragments are released constitutively by virtually all cell types—including mesenchymal stromal cells (MSCs)—under stimulation or following cell-to-cell interaction, which leads to activation or inhibition of distinct signaling pathways. Based on their size, intracellular origin, and secretion pathway, EVs have been grouped into three main populations: exosomes, microvesicles (or microparticles), and apoptotic bodies. Several molecules can be found inside MSC-derived EVs, including proteins, lipids, mRNA, microRNAs, DNAs, as well as organelles that can be transferred to damaged recipient cells, thus contributing to the reparative process and promoting relevant anti-inflammatory/resolutive actions. Indeed, the paracrine/endocrine actions induced by MSC-derived EVs have demonstrated therapeutic potential to mitigate or even reverse tissue damage, thus raising interest in the regenerative medicine field, particularly for lung diseases. In this review, we summarize the main features of EVs and the current understanding of the mechanisms of action of MSC-derived EVs in several lung diseases, such as chronic obstructive pulmonary disease (COPD), pulmonary infections [including coronavirus disease 2019 (COVID-19)], asthma, acute respiratory distress syndrome (ARDS), idiopathic pulmonary fibrosis (IPF), and cystic fibrosis (CF), among others. Finally, we list a number of limitations associated with this therapeutic strategy that must be overcome in order to translate effective EV-based therapies into clinical practice.
Introduction
Mesenchymal stromal cells (MSCs) have been widely studied for their potential regenerative and immunomodulatory actions in several preclinical experimental models and in early stage clinical trials of lung diseases (Lalu et al., 2012; Cruz and Rocco, 2020; Lopes-Pacheco et al., 2020). Although their mechanisms of action have yet to be fully elucidated, MSCs administered either locally or systemically have the ability to release a mix of bioactive molecules (Phinney et al., 2015; Cruz and Rocco, 2020; Lopes-Pacheco et al., 2020), which promote activation of endogenous repair pathways (Lai et al., 2015) and reprogramming of immune cells, leading to modulation of both inflammatory and remodeling processes (Bell et al., 2012; Cruz and Rocco, 2020; Lopes-Pacheco et al., 2020). The MSC secretome consists of an extensive mix of bioactive molecules that coordinate processes within the inflammatory microenvironment, influencing tissue regeneration (Zhao et al., 2010). These molecules include growth factors, cytokines/chemokines, and extracellular vesicles (EVs) that promote inhibition of cellular apoptosis and disorganized deposition of extracellular matrix and/or modulation of immune responses (Waszak et al., 2012; Phinney et al., 2015; Mohammadipoor et al., 2018; de Castro et al., 2019; Popowski et al., 2020).
The conditioned medium (CM) of MSCs yields bioactive products from which EVs can be isolated. Notably, administration of MSC-derived CM can promote protective actions similar to those of the parent cells, which suggests that secretion of trophic factors might be the key mechanism underlying the therapeutic effects of MSCs (Lai et al., 2015; Mohammadipoor et al., 2018). Furthermore, administration of MSC secretome-derived bioactive products, including EVs, is associated with additional safety advantages compared to living cells, since EVs do not carry the potential risks of whole-cell transplants. MSC secretome products are non-oncogenic and less immunogenic than living cells, can be administered intravascularly without the risk of causing clots, and can be sterilized, handled, and stored more easily, with no need for cryoprotectants (e.g., dimethyl sulfoxide) (Goolaerts et al., 2014; Giebel et al., 2017; Gimona et al., 2017). Therefore, MSC secretome-derived bioactive products have become an emerging therapeutic option to replace the administration of MSCs as potent therapies for different lung disorders.
Several studies have demonstrated that EVs act on cell-to-cell communication and, consequently, exert a relevant role in the paracrine/endocrine actions induced by MSC-based therapy. According to the minimal criteria established by the International Society for Extracellular Vesicles (ISEV), EVs are small spherical membrane fragments that can be classified into three different types: exosomes, microvesicles (MVs) or microparticles, and apoptotic bodies (Theìry et al., 2018). Essentially every cell type can secrete EVs, although their cargo differs significantly. In fact, EVs can modify the fate and phenotype of recipient cells (Camussi et al., 2010). Additionally, EVs can also be harvested in vivo from several body fluids such as blood, breast milk, bronchoalveolar lavage fluid (BALF), serum, and even urine (Almqvist et al., 2008; Baglio et al., 2012). Therefore, persistent circulating EVs in biological fluids can serve as an indicator for the diagnosis of several diseases, providing significant information regarding individual pathological and physiological status (Eissa, 2013; Asef et al., 2018).
Many parameters have been used for EV characterization. These include biogenesis pathway, flotation density on a sucrose gradient, ionic composition, lipid composition, protein cargo, and sedimentation rate and size, among others. It is important to note that none of these parameters is definitive or exclusive to any specific type of EV (Theìry et al., 2018); therefore, they should be well-documented to ensure reproducibility. Ideally, EVs should be characterized by quantifiable physical characteristics, biochemical composition, and functional assays (Witwer et al., 2019). Our knowledge regarding EVs is continuously expanding, but many questions remain unanswered. In this review, we describe the main characteristics of different EVs with a particular focus on MSC-derived EVs. We also outline the state of the science regarding the potential of these bioactive products as therapy for various lung diseases. Finally, we summarize the key advantages and limitations that should be considered in order to translate effective EV-based therapies into the clinical scenario.
Extracellular Vesicles
Biogenesis and Classification of Extracellular Vesicles
Extracellular vesicles are plasma membrane-derived structures, ranging from 30 nm to 5 μm in diameter, that are limited by a phospholipid bilayer. These structures comprise a heterogeneous population of vesicles that contain various bioactive molecules (proteins, lipids, DNA, mRNA, and microRNAs), which confer differences in their biological activities (Theìry et al., 2018; Witwer et al., 2019) (Figure 1). Eirin et al. (2014) found almost 400 miRNAs analyzed in MSC-derived EVs, and the levels of four were significantly higher in EVs compared to parent MSCs: miR-148a, miR-378, miR-532-5p, and let-7f. Additionally, a significant difference was found between the protein levels expressed in MSCs and those measured in their EVs. These include proteins associated with angiogenesis, apoptosis, blood coagulation, extracellular matrix remodeling, and inflammation, which demonstrated a higher expression in EVs compared to their parent MSCs (Eirin et al., 2014). Nevertheless, further studies are necessary to determine whether these differences between the biological activity and content of EVs and their parent MSCs are indeed responsible for the distinct therapeutic action of EVs.
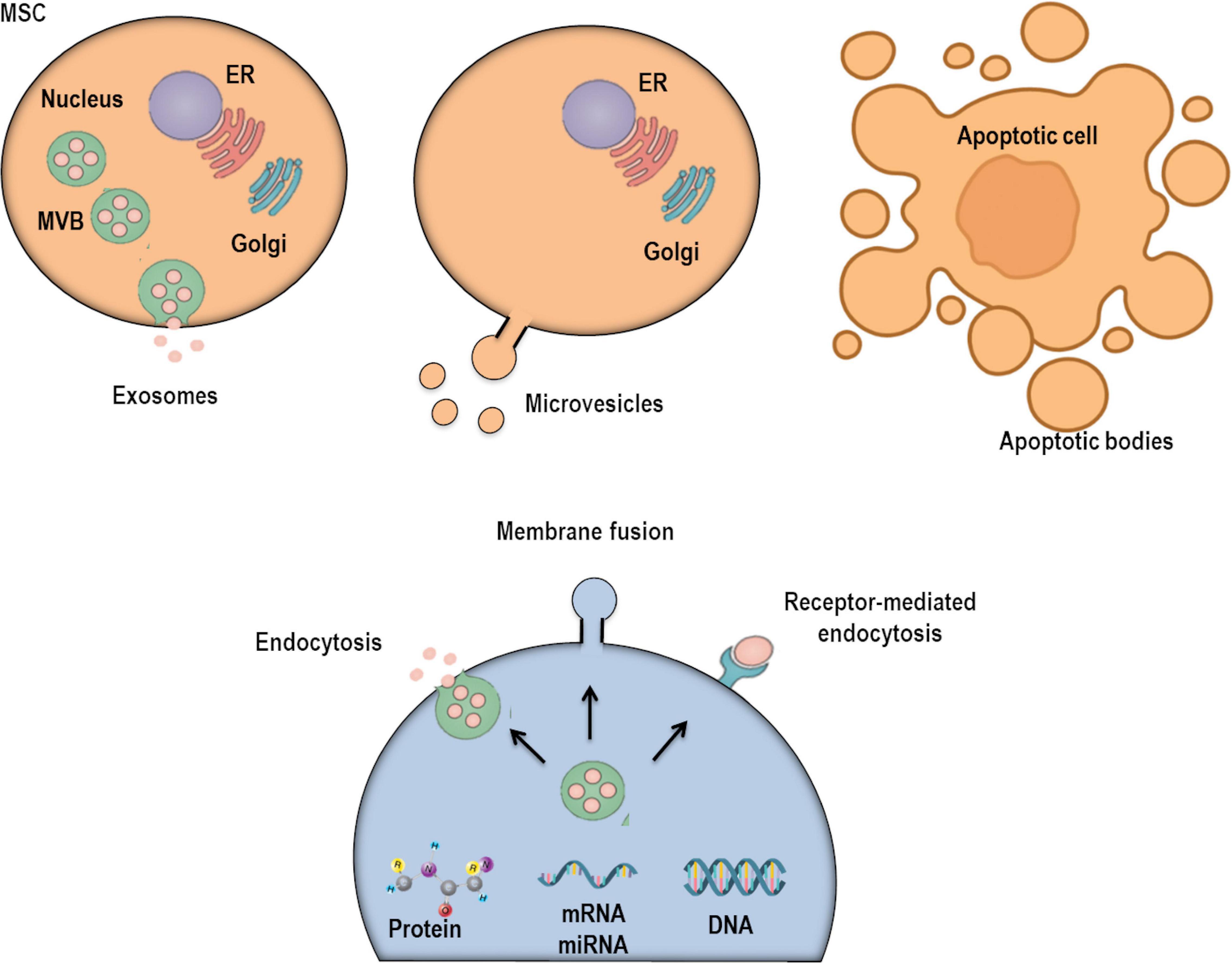
Figure 1. Extracellular vesicles (EVs) are currently classified into three subpopulations depending on their subcellular origin, secretion mechanism, and size: exosomes, microvesicles, and apoptotic bodies. There are several mechanisms through which EVs may interact with recipient/target cells: interactions with plasma membrane (PM) receptors, internalization into endocytic compartments, and fusion with PM.
Based on the minimal criteria for definition, characterization, and studies of EVs (Theìry et al., 2018; Witwer et al., 2019), cells are able to produce and secrete three main types of EVs: exosomes, MVs, and apoptotic bodies. These are grouped based on their cellular origin, secretion pathway, and size (Figure 1). Exosomes were observed for the first time in reticulocytes of rodents (Harding et al., 1983) and sheep (Pan et al., 1985) in the 1980s. These structures are nano-sized vesicles (30–150 nm) with a homogeneous shape (Cocucci et al., 2009), derived from specialized compartments—the late endosomes or multivesicular bodies (MVBs)—and released into the extracellular compartment by exocytosis (Trajkovic et al., 2008; Henne et al., 2013; Kowal et al., 2014; Guiot et al., 2019). They are released constitutively from cells by chemical and physical stimuli: soluble factors and shear stress, respectively (Kinoshita et al., 2017). During exosome biogenesis, the limiting membrane of the MVB buds inward, forming intraluminal vesicles (ILVs). These structures are then released into the extracellular environment as exosomes after fusion with the plasma membrane. This process is intermediated by p53-regulated exocytosis, which is dependent on cytoskeletal activation, although independent of calcium influx (Hessvik and Llorente, 2018). The endosomal sorting complex required for transport (ESCRT) is also necessary for ILV delivery into MVBs (Hessvik and Llorente, 2018). MVBs can then take two pathways: (1) fuse with the plasma membrane by using RAB GTPases in an ESCRT-independent mechanism or (2) fuse with lysosomes for cargo degradation (Frydrychowicz et al., 2015; Fujita et al., 2015). Importantly, during the exocytosis of this vesicle, it can integrate multiple proteins from the plasma membrane: tetraspanins, membrane transporters, ion channels, fusion proteins (CD9, CD63, CD81, and CD82), Tsg10, raft proteins, major histocompatibility complex (MHC) proteins, and targeting/adhesion molecules (Gon et al., 2020). This suggests that the composition of the exosomal limiting membrane can vary depending on cellular origin or activation of intracellular signaling pathways. MVs are structures ranging from 0.1 to 1 μm in diameter, which originate from outward budding and scission of the plasma membrane (Simoncini et al., 2009). Their biogenesis begins with translocation of phosphatidylserine into the outer membrane leaflet (Cocucci et al., 2009). Thereafter, a contraction of cytoskeletal structures occurs, releasing the nascent MVs into the extracellular compartment (Cocucci et al., 2009). Notably, a wide range of plasma membrane proteins has been associated with the process of MV scission, including members of the ADP-ribosylation factor small G-protein subfamily, ARF1 and ARF6, and TSG101 (a component of the ESCRT) (Kinoshita et al., 2017). MVs are also enriched in ceramide and sphingomyelin and have high levels of proteins associated with lipid rafts, cholesterol, and phosphatidylserine. Furthermore, the biological activities of MVs are largely variable, as their bioactive cargo is dependent on cellular origin and stimuli that can alter intracellular signaling (Kinoshita et al., 2017). Once these particles are released in the extracellular compartment, they can affect the microenvironment by altering the phenotype of recipient cells (Cocucci et al., 2009). Finally, apoptotic bodies are larger EVs (1–5 μm in size) derived from dying cells destined to be cleared by phagocytic cells (Kerr et al., 1972; Atkin-Smith et al., 2015; Theìry et al., 2018). These vesicles are characterized by exposing phosphatidylserine to the extracellular compartment and containing histones and fragmented DNA (Kerr et al., 1972; Atkin-Smith et al., 2015; O’Farrell and Yang, 2019). They can also contain cell organelles and non-coding RNAs. Indeed, recent studies have suggested that recipient cells might obtain certain genetic information by engulfing apoptotic bodies (Galleu et al., 2017; Abreu et al., 2019; O’Farrell and Yang, 2019).
Isolating pure EV populations is difficult because different EV types can overlap in size and density (Theìry et al., 2018; Witwer et al., 2019). In fact, a preparation containing EVs < 200 nm can be called as “small EVs,” whereas EVs > 200 nm are called “medium or large EVs” (Theìry et al., 2018). However, this definition is based on EV size; exosomes, MVs, and apoptotic bodies differ according to their biogenesis pathway (Witwer et al., 2019). Therefore, establishment of standard methods that can efficiently enable the separation of a subpopulation of EVs remains a major challenge.
As mentioned above, EV contents are composed of different types of nucleic acids, proteins, and lipids; however, certain organellar contents (e.g., mitochondria) can also be transported within MVs and apoptotic bodies. Notably, EV cargo and certain biological activities may reflect, at least partially, features of their cellular origin (Chen J. et al., 2014), as well as their mode of biogenesis and exogenous stimuli. Two major databases store information regarding EVs: (1) the VESICLEPEDIA1 collects information on different EV contents, while (2) ExoCarta2 collects information on the exosomes derived from different cell types and organisms.
Extracellular vesicles exert a critical role in the intercellular compartment through distinct mechanisms. These include direct binding to surface receptors, inhibition/activation of intracellular pathways, horizontal transfer of genetic information, and protein and lipid delivery (Tetta et al., 2011). Due to the remarkable ability of EVs to modify the fate and phenotype of recipient cells (Akyurekli et al., 2015), they have become a promising therapeutic option. In particular, MSC-derived EVs have demonstrated, in multiple in vitro and in vivo models, the potential to circumvent some of the safety risks related to whole-cell transplantation. Nevertheless, further insights on the cargo of EVs from different origins and how these can be modulated to enhance tissue regeneration are still required (Baglio et al., 2012; Akyurekli et al., 2015).
Methods for Extracellular Vesicle Isolation
Several methods have been employed to isolate EVs, each of which has its own benefits and disadvantages. Conventional methods include the measurement of EV size and flotation density through gel filtration, microfiltration, and ultracentrifugation (the most commonly used technique) (Thery et al., 2006; Tauro et al., 2012; Taylor and Shah, 2015; Theìry et al., 2018; Witwer et al., 2019). Sequential centrifugation steps (Mendt et al., 2018) and ultrafiltration are methods for large-scale EV production (Bari et al., 2019). Additional methods based on EV solubility have emerged, including precipitation by using polyethylene glycol, protamine, or sodium acetate (Deregibus et al., 2016; Gámez-Valero et al., 2016). Some methods based on interactions with molecules on the EV surface have also been investigated (Taylor and Shah, 2015; Konoshenko et al., 2018). In short, the best EV isolation technique depends on time and cost, and different techniques may result in different products with different therapeutic effects.
Characterization of Extracellular Vesicles
The ISEV recommends that EV characterization should be carried out using biomarkers from different categories, which include cytosolic and transmembrane proteins (Camussi et al., 2010; Lötvall et al., 2014). To further investigate population heterogeneity, the ISEV recommends the use of two additional methods, such as single-particle tracking methods and electron microscopy (Camussi et al., 2010; Lötvall et al., 2014; Witwer et al., 2017; Theìry et al., 2018; Witwer et al., 2019).
Measurement of Extracellular Vesicle Size
It is important to note that samples will always be composed of different types of EVs, regardless of which method is employed to isolate and separate them. Therefore, measurement of EV count and size is useful to ensure similarity across studies. Some commercially available particle analyzers have been frequently used to carry out these measurements. NanoSight (NanoSight, Amesbury, United Kingdom) can visualize and measure particle size, number, and concentration in real time. Zetaview (Particle Metrix, Germany) is an alternative that can measure hydrodynamic particle size, zeta potential, and concentration. The qNano system (Izon Sciences Ltd., Palmerston North, New Zealand) can measure EV amount and size by using a tunable resistive pulse-sensing technology.
Biological Activities and Potential Mechanisms of Action of Extracellular Vesicles
There are several mechanisms by which EVs may interact with recipient cells. These include plasma membrane receptors, fusion of EVs with the plasma membrane itself, and internalization into endocytic compartments (Phinney et al., 2015). After interaction with recipient cells, EVs may release their cargo in the cytosol, leading to inhibition or activation of certain signaling pathways. A subset of mRNAs and miRNAs present in EV cargo may also alter gene expression of recipient cells, leading to modulation of biological activities (Table 1). Furthermore, EVs may induce reprogramming and alter the phenotype and/or behavior of recipient cells (Camussi et al., 2013). Nevertheless, the exchange of vesicle cargo can occur in a two-way fashion, i.e., from MSCs to damaged cells or from a harmful microenvironment to MSCs. In this context, Dooner et al. (2008) reported that bone marrow-derived MSCs started to express genes for lung-specific proteins when cocultured with injured lung cells; these proteins include club cell-specific components as well as surfactant proteins. This phenomenon may be attributed to the EV-mediated transfer of lung-specific mRNAs from the damaged lung cells to bone marrow cells (Dooner et al., 2008).
Human MSC-derived EVs have been more widely studied in the last few years (Gomzikova et al., 2019; Gowen et al., 2020; Zhang B. et al., 2020). These vesicles have a cargo composed of ribonucleoproteins that are involved in the synthesis and intracellular trafficking of RNAs, suggesting a distribution and dynamic rearrangement of RNA related to cell differentiation, development, and repair—all of which can contribute to a more efficient recovery process after damage (Figure 2) (Collino et al., 2010). On the other hand, mounting evidence indicates that MSC-derived EVs are able to promote immunomodulatory actions. These include inhibition of immune cell differentiation, polarization of macrophages, expansion of regulatory T cells, as well as production and secretion of anti-inflammatory and resolutive mediators (Mokarizadeh et al., 2012; de Castro et al., 2019) (Figure 2). In this context, MSCs or their EVs have been shown to promote macrophage polarization toward an M2 anti-inflammatory profile rather than the conventional M1 pro-inflammatory phenotype, which can also contribute to inflammation resolution and tissue repair (Zhang et al., 2014; Abreu et al., 2018; Mansouri et al., 2019). A specific inflammatory environment can stimulate MSCs to produce and secrete subsets of EVs that are able to act on endogenous repair pathways and modulate immune responses (Hofmann et al., 2012; Tomasoni et al., 2013). Eirin et al. (2014) found that MSC-derived EVs overexpress certain transcription factor mRNAs, such as MDFIC, NRIP1, and POU3F1. Genes associated with adipogenesis (CEBPA and KLF7) and angiogenesis (HES1, HGF, and TCF4) were also found to be upregulated (Eirin et al., 2014).
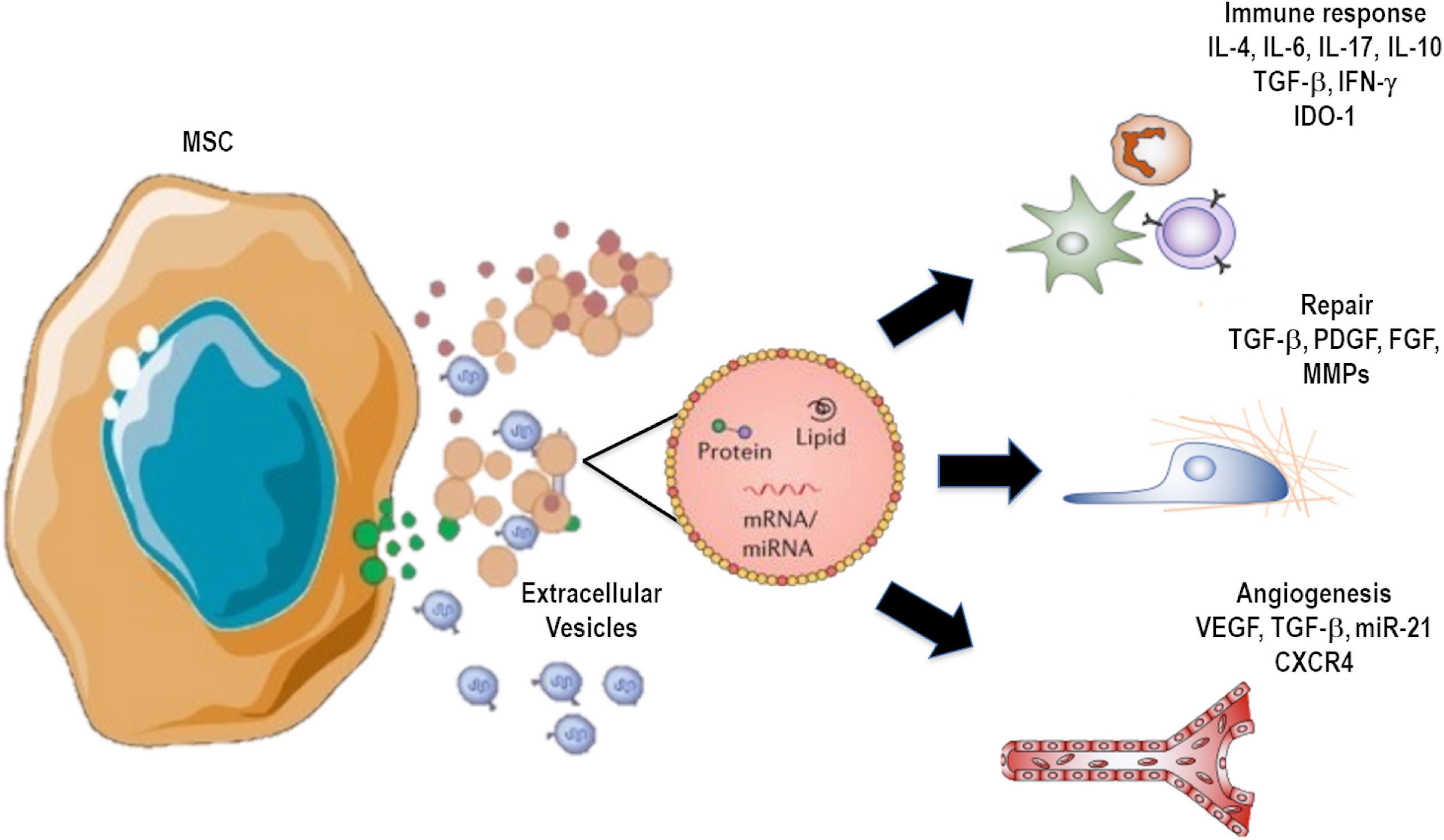
Figure 2. Extracellular vesicle (EV) cargo can contain several molecules, including proteins, mRNA, microRNAs, DNAs, and lipids, as well as organelles that can be transferred to recipient cells, inducing modulation of immune responses and remodeling processes.
Extracellular vesicles derived from immune or damaged cells may stimulate differentiation of resident stem cells or recruit stem-like cells present in other organs in order to promote a more efficient tissue repair (Bruno and Camussi, 2013). EVs can also exert immunomodulatory actions depending on their cellular origin or external stimulation (Bourdonnay et al., 2015; de Castro et al., 2019). For example, mycobacteria-infected alveolar macrophages are known to release vesicles containing pathogen-derived pro-inflammatory molecules that upregulate heat-shock protein 70, which then stimulates the nuclear factor kappa B (NF-κB) pathway by promoting activation of toll-like receptors (TLRs) and downstream signaling (Katsuda et al., 2013; Bruno et al., 2015; Fujita et al., 2015). Furthermore, EVs derived from lipopolysaccharide (LPS)-activated monocytes have been shown to induce apoptosis of recipient cells by transfer and activation of the caspase-1 cascade (Sarkar et al., 2009). On the other hand, EVs secreted by dendritic cells are able to promote humoral responses against antigens processed by these cells before EV purification, which yields potent protection against infections (Qazi et al., 2010; Bruno et al., 2015).
Extracellular Vesicle-Secreting Cells in the Respiratory Tract
Extracellular vesicles can be produced and secreted by virtually all cell types in the respiratory system. An increasing number of studies have characterized EVs from alveolar macrophages, bronchial epithelial cells (BECs), fibroblasts, type II pneumocytes, and vascular endothelial cells under normal physiological or pathological conditions (Kulshreshtha et al., 2013). In this context, BECs have been considered a main source of pulmonary EVs (Kulshreshtha et al., 2013); release can be stimulated by environmental components, such as cigarette smoke, which induce injury of these cells and promote their release of pro-inflammatory cytokines and chemokines (Kadota et al., 2016). BECs are able to secrete EVs of various sizes, which can contain a wide range of cargoes such as membrane-tethered mucins (MUC)-1, -4, and -6 (Kesimer et al., 2009; Kesimer and Gupta, 2015). These components are present on the surface of BEC-derived EVs and can neutralize human influenza A viruses (Kesimer et al., 2009). One study in a mouse model of ovalbumin-induced allergic asthma found a twofold increase in counts of immune cell-derived EVs and increased expression of immune cell-derived miRNAs (including miR-142a and miR-223) after allergy induction (Pua et al., 2019). By using a cell type-specific membrane marker, the authors found that the majority of EVs released into BALF were derived from airway-lining epithelium (Pua et al., 2019).
Alveolar macrophages are also major sources of EVs (Kulshreshtha et al., 2013). Macrophage derived-EVs can induce differentiation of other macrophages through miRNA-223 transfer (Ismail et al., 2013). Procoagulant EVs can also be released by macrophages in response to cigarette smoke (Li et al., 2010), upregulating the production of pro-inflammatory mediators by lung epithelial cells. Intercellular adhesion molecule-1, interleukin-8, and monocyte chemotactic protein-1 are some important mediators that were observed to be upregulated by cigarette smoke exposure (Cordazzo et al., 2014).
Vascular endothelial cells can produce and secrete EVs that participate in several biological processes, depending on their cargo. These processes include angiogenesis, coagulation, endothelial function, and inflammation (Kadota et al., 2016). EVs may activate fibroblasts and stimulate their differentiation into myofibroblasts, contributing to excessive deposition of extracellular matrix components, or prevent the extensive activation of these cells and thus maintain homeostasis (Lacy et al., 2019). B cells are able to secrete EVs that activate or inhibit several immune cell populations (Raposo et al., 1996). Furthermore, EVs secreted by dendritic cells appear to modulate immune reactions by activating T and B cells, suggesting intercellular communications to modulate airway inflammation and allergic reactions.
Extracellular Vesicles in Lung Disease Pathogenesis and Therapy
Although the effects of MSC therapy in experimental models of lung diseases have been extensively investigated (Lopes-Pacheco et al., 2016, 2020; Cruz and Rocco, 2020), the study of EVs as a possible alternative strategy to MSC administration is less well explored, and many questions still need to be further elucidated (Table 2). Nevertheless, a growing number of experimental studies have investigated the potential of MSC-derived EVs as a therapy for lung diseases. In this section, we summarize some important findings from experimental research of EV-based therapies for chronic obstructive pulmonary disease (COPD), pulmonary infections [including coronavirus disease 2019 (COVID-19)], asthma, acute respiratory distress syndrome (ARDS), idiopathic pulmonary fibrosis (IPF), and cystic fibrosis (CF), among others.
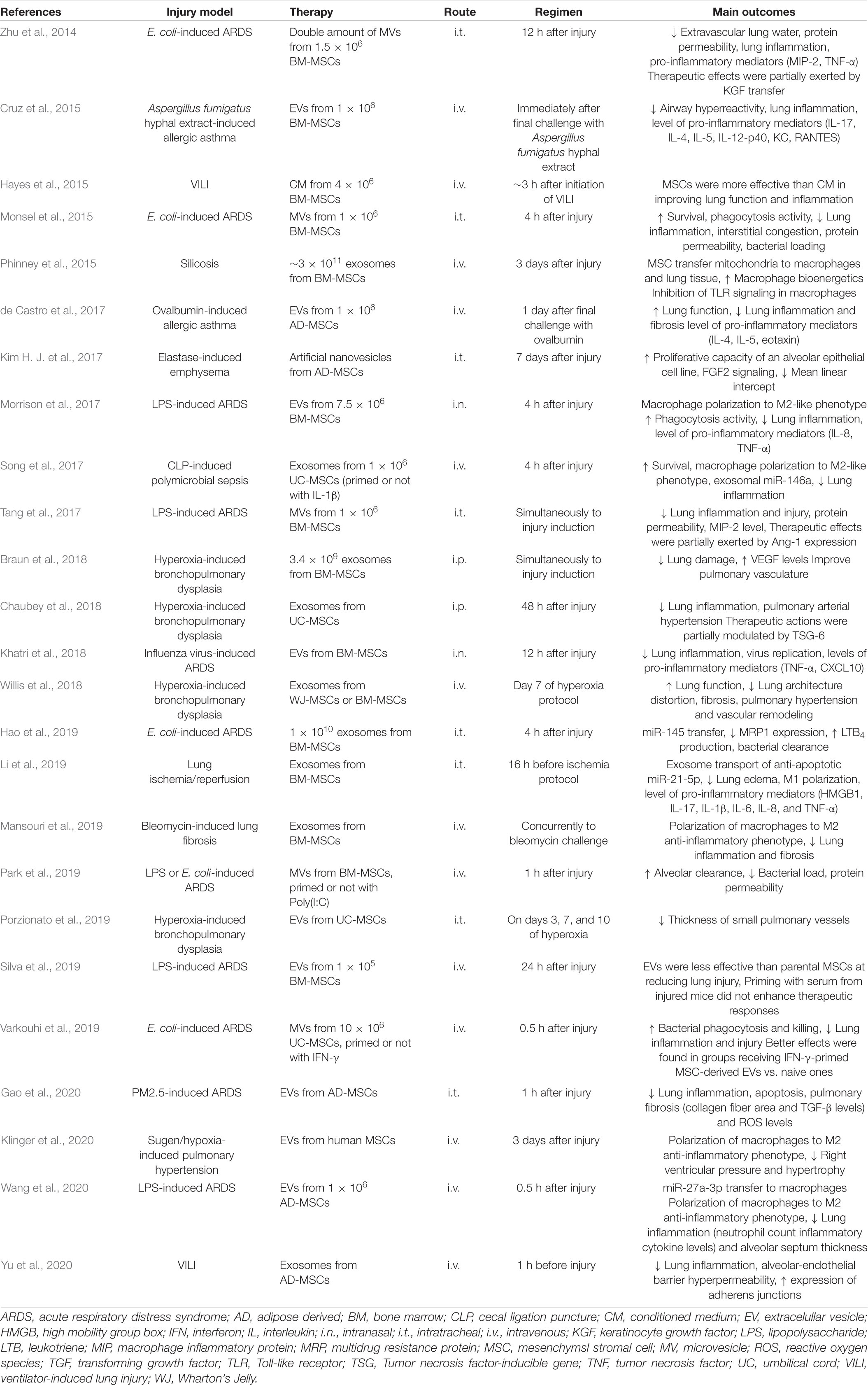
Table 2. Studies evaluating effects of administration of mesenchymal stromal cell-derived extracellular vesicles in models of lung diseases.
Chronic Obstructive Pulmonary Disease
Chronic obstructive pulmonary disease is characterized by a restriction of airflow due to airway obstruction that is progressive and partially irreversible (Singh et al., 2019), among several other respiratory symptoms. During exacerbations, COPD patients often require pharmacological intervention and hospitalization to alleviate symptoms (Padilha et al., 2015; Global Initiative for Chronic Obstructive Lung Disease [GOLD], 2020).
Several studies have demonstrated that EVs can be used not only as a potential therapeutic strategy but also as an important biomarker of COPD diagnosis and prognosis. Some reports suggest that stress-induced lung-derived EVs participate in the pathogenetic cascade of COPD (Frydrychowicz et al., 2015; Fujita et al., 2018). For example, activated neutrophils are able to produce and secrete exosomes that carry surface-bound neutrophil elastase. This molecule can lead to extensive alveolar deterioration due to its ability to withstand degradation by α1-antitrypsin (Genschmer et al., 2019).
Infection is a major cause of complications in COPD patients, as it may result in exacerbations, which worsen the excessive production of mucus, prevent effective mucociliary clearance in the airways, and aggravate disease progression and severity (Sethi and Murphy, 2008). As virtually all cells are able to secrete EVs, analysis of these can help in the identification of specific features of the individual and pathogen, including the initial focus and etiologic agent of infection (Rodrigues et al., 2018). If pathogen-induced EVs are released during an infectious process, these can carry relevant molecular information regarding pathogen replication and interaction with host immune responses, as well as possible diagnostic bioindicators (Rodrigues et al., 2018). In this context, Takahashi et al. (2012) found that endothelial cell-derived EVs were overexpressed in COPD patients, especially during exacerbations, highlighting the potential of EVs to predict these exacerbations. Furthermore, studies observed that some miRNAs found within EVs may correlate directly with COPD severity by participating in disease development and progression (Savarimuthu Francis et al., 2014). Cigarette smoke exposure can modify the cargo of EVs released by BECs, with upregulation of several miRNAs (miR-500a-5p, miR-574-5p, miR-656-5p, miR-3180-5p, and miR-3913-5p) and downregulation of three miRNAs (miR-222-5p, miR-618, and miR-130b-5p) (Corsello et al., 2019). In another study reporting the effects of EV transfer on COPD pathogenesis, the authors indicated that microparticles can promote the release of a subset of miRNAs (miR-125a, miR-126, and miR-191) to resident macrophages, which are responsible for the removal of apoptotic cells (Serban et al., 2016). Exosomal miR-21 derived from BECs has been associated with myofibroblast differentiation in response to cigarette smoke, since smokers present a high level of exosomal miR-21 compared to healthy controls (Serban et al., 2016; Xu et al., 2018). Therefore, more studies investigating exosomal miRNA cargo should help further our understanding of the role of circulating miRNAs as potential biomarkers. Tan et al. (2017) evaluated the levels of serum-derived exosomes in COPD patients during exacerbations and in stable disease. These patients presented higher levels of serum-derived exosomes, which correlated with the levels of C-reactive protein (CRP), interleukin-6 (IL-6), and soluble tumor necrosis factor receptor 1 (sTNFR1) expression, suggesting a role of these vesicles during COPD exacerbations (Tan et al., 2017).
Some recent reports have indicated that most of the immunomodulatory actions and reparative ability of MSCs are recapitulated by their EVs and, therefore, MSC-derived EVs appear to be a viable substitute for whole cell-based therapy (Ahn et al., 2018; Khatri et al., 2018). In a model of elastase-induced emphysema, the effects of EVs harvested from adipose tissue-derived MSCs were compared with those of artificially developed nanovesicles obtained from this same cell type (Kim Y.S. et al., 2017). The authors found a higher proliferation rate of lung epithelial cells after exposure to lower doses of artificial nanovesicles than after exposure to the natural exosomes and even higher doses of adipose tissue-derived MSCs; this suggests that artificial nanovesicles might have both economic and clinical advantages in the treatment of individuals with emphysema (Kim Y.S. et al., 2017). Nevertheless, identification of the best route of administration for EV therapy in the clinics remains an important factor that must be investigated (Aliotta et al., 2016).
Some molecular engineering techniques have been investigated to modify and enhance the therapeutic potential of EVs. Both exogenous and endogenous modifications have been widely employed. The first consists of the incorporation of a desired cargo (RNAs, proteins, or even small-molecule compounds) into or onto isolated EVs, while the latter involves stimulating cells in a way that ensures the incorporation of the desired cargo into EVs during their synthesis (Wiklander et al., 2019). Co-incubation, electroporation, and sonication are some exogenous methods to package desired cargo into EVs that have demonstrated success in several studies. The incorporation of curcumin by EVs is a successful example that has been shown to improve the bioavailability of this compound and anti-inflammatory actions in distinct models of inflammation (Sun et al., 2010; Alvarez-Erviti et al., 2011; Didiot et al., 2016; Lamichhane et al., 2016). On the other hand, the most common endogenous methods include genetic modification of parent cells to induce overexpression of a certain RNA or protein, which in turn increases its level in EVs (Wiklander et al., 2019). EVs can serve as a “shield” and protect miRNAs from digestion and degradation, while also enabling an efficient delivery of the cargo to target cells (Etheridge et al., 2011; Minciacchi et al., 2015; Zhang et al., 2017). Furthermore, the use of patient-derived EVs can provide additional benefits, as these possess high cell specificity and may evade immune-system surveillance, thus lowering the risk of reaction or rejection in the recipient (Zhang et al., 2017).
Pulmonary Infections
Lee et al. (2009) were able to demonstrate that certain bacterial species, such as Bacillus subtilis and Staphylococcus aureus, produce EVs. Kim et al. (2015) demonstrated that EVs derived from Escherichia coli cultures can increase IL-17A and TNF-α levels, thus intensifying lung inflammation in experimental emphysema. Furthermore, COPD patients possess lung-derived EV microbiomes that are significantly distinct from their whole lung tissue microbiomes (Kim H. J. et al., 2017).
In addition to bacterial infection, viruses are common triggers of COPD exacerbations (Hewitt et al., 2016). In fact, some reports suggest that respiratory viruses might have adapted to usurp resident cell-derived EVs for the transmission and dissemination of viral particles and virus-expressed molecules, such as miRNAs, mRNAs, and viral proteins (Altan-Bonnet, 2016). These can be then transported to uninfected neighbor cells or reach the bloodstream by distinct mechanisms (Rodrigues et al., 2018).
Conversely, EVs derived from swine bone marrow MSCs were able to reduce viral replication and shedding and secretion of pro-inflammatory mediators in a porcine model of influenza (Khatri et al., 2018). Based on the current knowledge of the therapeutic potential of MSC-derived EVs on lung diseases, a growing number of research groups has hypothesized that these bioactive products might be an effective strategy against severe acute respiratory syndrome coronavirus 2 (SARS-CoV-2), the novel coronavirus responsible for the ongoing active COVID-19 pandemic (Lopes-Pacheco et al., 2021). Due to the urgent need for an effective therapy against this devastating disease, administration of exosomes from allogeneic bone marrow MSCs (ExoFloTM) was evaluated in a small, non-randomized, open-label cohort study of individuals with severe COVID-19. ExoFloTM demonstrated a good safety profile and was able to improve oxygenation and biomarkers associated with inflammation (Sengupta et al., 2020). This study had several limitations, such as the dearth of information regarding ExoFloTM (EV characterization, biological properties, proposed biological or therapeutic actions); efficacy remains to be demonstrated in large-scale clinical studies. A clinical trial evaluating exosome inhalation for SARS-CoV-2-induced pneumonia is in progress (NCT04491240).
The use of pathogen-derived EVs and/or their bioactive cargo as EV-based vaccines has also been investigated with some positive results (Roy et al., 2011). Clinical trials assessing EVs of viral origin demonstrated advantages compared to conventional vaccines against infectious diseases, since EVs cannot multiply or divide, which suggests that they are safer, less tumorigenic, and less infectious. Preclinical and clinical trials of EV-based vaccines used as antimicrobial treatments have observed good tolerability and feasibility. Nevertheless, further investigation is necessary to validate the use of EV-based vaccines in humans and demonstrate persistent immunostimulatory actions (Wiklander et al., 2019).
Asthma
Asthma is a chronic inflammatory disease characterized by airway narrowing and hyperresponsiveness (GINA, 2020). The use of long-acting β2-adrenoceptor agonists combined with inhaled corticosteroids is able to achieve disease control in most asthmatic individuals; however, those with severe asthma might be resistant to treatment, and these drugs are unable to reverse established remodeling (Papierniak et al., 2013). Asthma presents distinct phenotypes, which have specific pathological and clinical features and respond differently to pharmacotherapies (Gauthier et al., 2015; Ray et al., 2015). These aspects are likely associated with individual genetic background, type of allergen, and which cell types participate in asthma pathogenesis (Stokes and Casale, 2016). In this context, different types of EVs, especially exosomes, have been recognized as important players in the inflammatory and remodeling processes of asthma.
Extracellular vesicles obtained from different sources may be used as disease markers or even have therapeutic potential in asthma. BALF-derived EVs from asthmatic patients demonstrated increased levels of IL-4 and leukotriene C4 (Torregrosa Paredes et al., 2012). In experimental allergic asthma, administration of BALF-derived EVs from asthmatic mice reduced airway inflammation and hyperresponsiveness (Prado et al., 2008). In another study, sensitization and challenge with house dust mite (HDM) extract promoted an 8.9-fold increase in EV content in BALF (Gon et al., 2017), which corroborates the observation that EV content can differ significantly among asthmatic patients (Francisco-Garcia et al., 2019). HDM exposure led to significant alterations in the expression of over 130 miRNAs in EVs, with an upregulation of 31 genes (including IL-5Ra and IL-13, which are potential targets of miR-574-5p and miR-346, respectively). The amount of lung cell-derived EVs was reduced when GW4869, an exosome generation inhibitor, was administered, ameliorating allergic asthma symptoms in a murine model (Gon et al., 2017).
In recent years, evidence has emerged regarding the therapeutic potential of MSC-derived EVs for the treatment of allergic asthma. MSC-derived EV therapy is able to upregulate IL-10 and transforming growth factor-β1 (TGF-β1), which in turn are responsible for the recruitment and expansion of regulatory T cells (Du et al., 2018), decrease the number of eosinophils in both lung tissue and BALF while also modulating airway remodeling (de Castro et al., 2017), and mitigate TH2/TH1-induced lung inflammation and airway hyperresponsiveness (Cruz et al., 2015). A recent clinical study demonstrated that administration of bone marrow-derived mononuclear cells was well-tolerated and safe and improved some quality of life parameters in three patients with severe asthma (Aguiar et al., 2020). Two clinical studies (NCT02192736 and NCT03137199) designed to evaluate the potential therapeutic actions of MSCs and their trophic factors in individuals with severe asthma are ongoing. However, the safety and efficacy of EVs in asthmatic patients remain to be investigated.
Acute Respiratory Distress Syndrome
The acute respiratory distress syndrome is characterized by disruption of the alveolar-capillary membrane, with intense neutrophil inflow into the alveolar space, increased levels of pro-inflammatory cytokines/chemokines, surfactant dysfunction, and presence of protein-rich pulmonary edema fluid, resulting in severe hypoxemia (Lopes-Pacheco et al., 2020). Several therapeutic and supportive approaches have been developed; however, mortality rates remain high (35–45%) (Bellani et al., 2016).
Several cell therapies (MSCs, embryonic stem cells, induced pluripotent stem cells, endothelial progenitor cells) have been investigated as potential therapeutic strategies in experimental models of ARDS (Monsel et al., 2014; Lopes-Pacheco et al., 2020). The few early stage clinical trials that were completed showed a good safety profile of MSC administration (Zheng et al., 2014; McIntyre et al., 2018; Matthay et al., 2019), but efficacy still needs to be demonstrated in large-scale clinical studies.
In animal models of LPS-induced ARDS, intratracheal instillation of human MSC-derived MVs reduced lung edema (Zhu et al., 2014; Wang et al., 2020); however, knockdown of angiopoietin-1 or keratinocyte growth factor (KGF) mRNA in MSC-derived MVs abrogated these protective effects (Tang et al., 2017). Furthermore, administration of human MSC-derived MVs improved survival and decreased bacterial growth, protein permeability, and lung inflammation in experimental E. coli-induced pneumonia (Monsel et al., 2015). Recently, in an ex vivo model of severe bacterial pneumonia using perfused human lung tissue, intravenous instillation of MSC-derived EVs decreased bacterial load, lung edema, and protein permeability, restoring alveolar fluid clearance, corroborating previous findings in animal models (Park et al., 2019).
The reparative potential of MSC-derived EVs in lung endothelial and epithelial cells has been extensively investigated, as these are the main cell types injured in ARDS. Hu et al. (2018) observed that MSC-derived EVs were able to recover human lung vascular endothelial cells by transferring angiopoietin-1 mRNA to cells, preventing formation of actin “stress fibers.” However, exploring the actions of MSCs or their EVs on repair of pulmonary vascular dysfunction in ARDS will require future studies. Similarly, the effects of MSC-derived EVs on the bioenergetics of the alveolar epithelium have been explored in only a few investigations. Monsel et al. (2015) observed that MSC-derived EVs are able to restore intracellular ATP levels and survival of alveolar epithelial type II cells damaged by exposure to certain inflammatory factors [IL-1β, interferon-γ (IFN-γ), and TNF-α].
Among the mechanisms associated with the beneficial actions of MSC-derived EVs in ARDS is their ability to promote immunomodulatory responses, e.g., regulating T- and B-cells (Budoni et al., 2013; Chen et al., 2016; Di Trapani et al., 2016), and macrophage polarization, leading to a shift from the classical M1 to an anti-inflammatory M2-like profile (Ti et al., 2015; Abreu et al., 2018, 2019; Rodrigues et al., 2018; Wang et al., 2020) likely via transfer of miR-27a-3p (Wang et al., 2020). MSC-induced EVs can also increase the phagocytosis capacity of macrophages in part by mitochondrial transfer from EVs to damaged alveolar monocytes/macrophages, which results in enhanced oxidative phosphorylation (Morrison et al., 2017). The antimicrobial actions of EVs were even greater when MSCs were primed with a TLR-3 agonist or IFN-γ before vesicle production and release (Monsel et al., 2015; Park et al., 2019; Varkouhi et al., 2019). Conversely, priming MSCs with serum obtained from injured mice was not associated with enhancement of EV actions in LPS-induced ARDS (Silva et al., 2019). Antiviral effects of MSC-derived EVs have also been reported (Chan et al., 2016; Qian et al., 2016).
Acute respiratory distress syndrome patients who survive the initial inflammatory insult can progress to a dysregulated proliferative and fibrotic process in lung tissue (Burnham et al., 2014), thus impairing lung function and potentially compromising quality of life (Orme et al., 2003). In experimental ARDS, MSC-derived EVs reduced not only lung inflammation but also fibrosis (Gao et al., 2020). Although prevention of lung fibrosis following ARDS remains the best approach to improve long-term prognosis, a better understanding of the potential ability of MSC-derived EVs to prevent or reverse post-ARDS lung fibrosis is warranted.
Idiopathic Pulmonary Fibrosis
Idiopathic pulmonary fibrosis represents ∼30% of all interstitial lung disorders and leads to respiratory failure within 5 years after disease onset (Sauleda et al., 2018). Tissue fibrosis is induced by elevated production of profibrotic mediators, mainly TGF-β. This growth factor has the ability to stimulate deposition of extracellular matrix components by promoting fibroblast activation/proliferation in damaged tissue, promoting persistence of apoptosis-resistant myofibroblasts, and activating the epithelial–mesenchymal transition (Lopes-Pacheco et al., 2016; de Oliveira et al., 2017). The potential of EVs in lung fibrosis remains to be further investigated.
Evidence of the role of exosomes in the pathophysiology of pulmonary fibrosis is still unclear. Some studies have shown that the profibrotic effects of TGF-β are regulated via programmed death ligand-1 (PD-L1). TGF-β induces PD-L1 via both Smad-dependent and -independent pathways; PD-L1 has also been shown to exert a previously unexpected role in the fibroproliferative actions of TGF-β and, when secreted into EVs, can act in a paracrine manner, providing greater opportunities for effective therapeutic intervention. A recent study demonstrated that TGF-β priming can considerably increase the amount of PD-L1 in fibroblast-derived EVs, which, in turn, induced a decrease in T-cell proliferation while increasing fibroblast migration in a PD-L1-dependent manner (Kang et al., 2019). Further investigations on the inhibition or promotion of profibrotic TGF-β signaling may reveal potential targets for the treatment of IPF and other lung fibrosis disorders.
Significant upregulation of miR-21-5p within serum-derived exosomes has been observed in experimental bleomycin-induced lung fibrosis (Makiguchi et al., 2016; Li et al., 2019). Baseline levels of miR-21-5p were found to correlate significantly with disease progression and were able to predict mortality during a 30-month follow-up in patients (Makiguchi et al., 2016; Li et al., 2019). MSC-derived EVs promoted immunomodulatory actions in experimental bleomycin-induced lung fibrosis, reducing both inflammation and remodeling (Tan et al., 2018; Mansouri et al., 2019). Another study also demonstrated the ability of MSC-derived EVs to suppress TGF-β-induced myofibroblast differentiation (Shentu et al., 2017).
It is important to note that quantification of the total amount of serum-derived exosomes can be largely variable. Therefore, implementation of standard methods to quantify certain microRNAs in clinical samples is still a challenge. The identification of more disease-specific biomarkers can be of great assistance to address this issue. As exosomes and microRNAs within cells might reflect pulmonary fibrosis status and severity, these may be combined with conventional biomarkers such as KL6, metalloprotease activity, and surfactant proteins A and D to obtain insight into the pathophysiology of IPF and patients’ clinical condition.
Cystic Fibrosis
Cystic fibrosis is a genetic disorder caused by mutations in the gene that encodes the CF transmembrane conductance regulator (CFTR) protein, which plays an important role as a bicarbonate and chloride channel at the surface of epithelial cells. Although the disease leads to a multisystem dysfunction, the major causes of morbidity and mortality are related to the respiratory disorder (mucus accumulation in the airways, chronic inflammation, and persistent infections, which result in a progressive decline of lung function) (Lopes-Pacheco, 2016).
Although almost 2,100 CFTR gene variants have been identified, the most common CF-causing mutation is the deletion of a phenylalanine residue at position 508 (F508del), which is found in ∼80% of CF patients (Lopes-Pacheco, 2020). This mutation leads to misfolding of the CFTR protein, which is then prematurely degraded by proteasomes (Lopes-Pacheco, 2020). As a consequence, CF patients are more susceptible to infection by certain pathogens, including S. aureus and Pseudomonas aeruginosa. Additionally, high concentrations of IL-8 and other neutrophil chemokines are usually observed in CF airways, as is intense neutrophil recruitment (Downey et al., 2009). This chronic inflammatory process leads to increased levels of oxidative burst, elastase, and pro-inflammatory mediators in the airways (Tabary et al., 2006; Mitri et al., 2020).
Microvesicles derived from CF granulocytes have been associated with an extensive presence of neutrophils in the airways and aggregation of these structures on the epithelial surface of the respiratory tract in CF patients (Tabary et al., 2006; Asef et al., 2018). These vesicles are associated with chronic inflammation and with the pro-inflammatory response characteristic of CF. Furthermore, evidence suggests that CF epithelial cells release vesicles of a particular size into the airways and BALF, which can be useful as biomarkers (Baixauli et al., 2014). The amount and type of mucin on the surface of the exosome are also indicative of mucus obstruction in CF patients (Batson et al., 2016). Therefore, as CFTR function is impaired in CF epithelia, exosomes may be a useful tool to reestablish normal CFTR function (Vituret et al., 2016). Indeed, EVs have been investigated as vectors for the transfer of wild-type CFTR into CF cells (Vituret et al., 2016).
Based on the foregoing, EVs may be a promising therapeutic approach for CF, given their anti-inflammatory and antioxidant actions in experimental models. Although the precise EV cargo related to these actions remains to be further exploited, some authors reported a correlation between upregulation of peroxisome proliferator-activated receptor-γ (PPARγ) and EV administration, thus inducing a modulation of downstream effectors [NF-κB and heme oxygenase-1 (HO-1)] of these pathways (Zulueta et al., 2018). Nevertheless, most investigations have focused on the potential replacement of functional CFTR using exosomes as possible vectors. In this context, some reports demonstrated successful transduction of exosomes derived from A549 cells (a human adenocarcinoma alveolar basal epithelial cell line) and Calu-3 (an epithelial cell line) with an adenoviral vector overexpressing green fluorescent protein (GFP)-tagged CFTR. This approach was able to deliver the functional protein into CFTR-deficient epithelial cells (Vituret et al., 2016; Zulueta et al., 2018). Nevertheless, further studies are necessary to investigate the potential use of EVs as vectors in CF therapies.
Extracellular Vesicle Therapy in Other Lung Diseases
Mesenchymal stromal cell-derived exosomes demonstrated cytoprotective actions similar to those of their parent cells in experimental pulmonary artery hypertension induced by hypoxia (Lee et al., 2012). MSC-derived EVs were also able to promote protective actions against the abnormal increase in right ventricular systolic pressure and right ventricular hypertrophy, which follow hypoxia exposure (Klinger et al., 2020). Specifically, MSC-derived exosome therapy upregulated hypoxia-induced mitogenic factors and suppressed inflammation by modulating early hypoxic signaling pathways. Furthermore, these vesicles were able to induce an alternative activation of alveolar macrophages (Klinger et al., 2020). Administration of either MSCs or their EVs was able to reduce mean pulmonary arterial pressure and mean right ventricular pressure in experimental monocrotaline-induced pulmonary arterial hypertension (Chen J. Y. et al., 2014; Aliotta et al., 2016). In another study, Willis et al. (2018) used a unique subset of MSC exosomes, namely, flotillin 1+ exosomes. The authors reported that administration of these vesicles led to improvements in lung development and morphology, as well as a reduction of tissue fibrosis and vascular endothelial remodeling in experimental hyperoxia in newborn mice (Willis et al., 2018). In another study using this model, umbilical cord MSC-derived EVs reduced lung inflammation and right ventricular hypertension in part due to exosomal TNF-stimulated gene 6 (TSG-6) (Chaubey et al., 2018).
Mesenchymal stromal cells-shed exosomes have demonstrated the ability to transfer certain regulatory microRNAs that resulted in modulation of TLR signaling and macrophage-secreted cytokine levels in an animal model of silica-induced lung inflammation and fibrosis (Phinney et al., 2015); miR-451, a factor that was highly abundant in these vesicles, is known to act as a macrophage migration inhibitory factor and inhibit TNF-induced signaling in response to silica (Phinney et al., 2015). In this context, Bandeira et al. (2019) demonstrated that MSC-derived EVs can reduce lung inflammation, fractional area of granuloma, and collagen deposition in experimental silicosis. Additionally, MSCs can transfer depolarized mitochondria via EVs that are captured by phagocytes, mainly macrophages. This process results in an enhancement of cellular bioenergetics (Morrison et al., 2017).
Limitations and Outlook
Further research is required to better elucidate several questions and overcome barriers that currently preclude the use of EVs as a therapeutic approach in the clinic. First, the precise molecular cargo of EVs remains to be determined and may differ depending on factors such as cell type, culture conditions, and extraction and purification method, with potential impact on EV functional actions (Antounians et al., 2019). Some recent studies have identified certain microRNAs and proteins in EV cargo, which appear to be involved in EV therapeutic effects. These include miR-27a-3p, miR-30b-3p, miR-126, and miR-145 (Wu et al., 2018; Hao et al., 2019; Yi et al., 2019; Zhou et al., 2019; Wang et al., 2020) as well as angiopoietin-1, hepatocyte growth factor (HGF), and KGF (Zhu et al., 2014; Tang et al., 2017; Wang et al., 2017). Precise determination of EV cargo may help elucidate the mechanisms underlying EV-based therapies, as well as potentially enrich specific molecules to further enhance therapeutic actions.
Second, although some recommendations for EV isolation and characterization have been proposed, these procedures need to be further standardized. Most experimental studies have not included information regarding good manufacturing practices, which hinders investigation of the therapeutic actions of EVs in clinical studies. Several other factors need to be discussed and optimized to allow translation of EV research to clinical practice, including scalability, manufacturing, distribution, and regulation (for a review, see Maumus et al., 2020); establishment of quality control measures along each step of the manufacturing process may minimize variations, even among different batches. All of these technical hurdles have yet to be addressed, although some good manufacturing practices have been proposed elsewhere (Gimona et al., 2017; Witwer et al., 2019). Furthermore, EV isolation and loading also remain costly and inefficient processes.
Third, heterogeneity between studies and experimental designs should be considered; differences in models used, as well as genomics, gene regulation, expression and development, and epigenetic factors, must be taken into account before extrapolating findings to humans.
Fourth, EVs have been isolated from MSCs derived from different sources. The most commonly used MSC sources have been bone marrow, adipose tissue, and umbilical cord, which are known to exert distinct degrees of efficacy when living cells are administered therapeutically (Antunes et al., 2014; Silva et al., 2018; Kern et al., 2019). The best dose and route of administration of EVs to achieve the greatest therapeutic response in each lung disease remain to be determined (Cruz and Rocco, 2020; Lopes-Pacheco et al., 2020). In fact, the dose and route of administration of EVs have been largely variable across studies, which poses a substantial challenge to comparative analysis among these investigations. The therapeutic index and biodistribution of EVs remain unknown. In this context, a single dose of EVs has been demonstrated to induce therapeutic effects in several studies, but multiple doses should be considered, as this approach has consistently been associated with superior outcomes in cell-based therapy (Lopes-Pacheco et al., 2013; Poggio et al., 2018; Castro et al., 2020). Lastly, most in vivo studies have been performed in small animals (mainly rodents), with only a few reports in large animal models or ex vivo human lungs.
In conclusion, EV-based therapy has demonstrated the ability to reduce lung inflammation and remodeling in several animal models and holds therapeutic promise for clinical use in lung diseases. However, despite the effects observed in experimental models, additional studies are required to develop an effective EV-based lung therapy to be used in the clinical scenario.
Author Contributions
SA and ML-P contributed to the design and conceptualization, original draft, editing, and review for intellectual content. DW contributed to the review for intellectual content. PR contributed to the design and conceptualization, editing, and review for intellectual content. All the authors read and approved the final version of the manuscript.
Funding
This work was supported by the Brazilian Council for Scientific and Technological Development (CNPq), the Rio de Janeiro State Research Foundation (FAPERJ), and the Brazilian Ministry of Science, Technology, Information, and Communications COVID-19 Network (RedeVírus MCTIC).
Conflict of Interest
The authors declare that the research was conducted in the absence of any commercial or financial relationships that could be construed as a potential conflict of interest.
Acknowledgments
The authors would like to express their gratitude to Mr. Filippe Vasconcellos (São Paulo), Brazil, for his assistance in editing the manuscript.
Footnotes
References
Abreu, S. C., Lopes-Pacheco, M., da Silva, A. L., Xisto, D. G., de Oliveira, T. B., Kitoko, J. Z., et al. (2018). Eicosapentaenoic acid enhances the effects of mesenchymal stromal cell therapy in experimental allergic asthma. Front. Immunol. 9:1147. doi: 10.3389/fimmu.2018.01147
Abreu, S. C., Xisto, D. G., de Oliveira, T. B., Blanco, N. G., de Castro, L. L., Kitoko, J. Z., et al. (2019). Serum from asthmatic mice potentiates the therapeutic effects of mesenchymal stromal cells in experimental allergic asthma. Stem Cells Transl. Med. 8, 301–312. doi: 10.1002/sctm.18-0056
Aguiar, F. S., Melo, A. S., Araújo, M. A. S., Cardoso, A. P., de Souza, S. A. L., Lopes-Pacheco, M., et al. (2020). Autologous bone marrow-derived mononuclear cell therapy in three patients with severe asthma. Stem Cell Res. Ther. 11:167.
Ahn, S. Y., Park, W. S., Kim, Y. E., Sung, D. K., Sung, S. I., Ahn, J. Y., et al. (2018). Vascular endothelial growth factor mediates the therapeutic efficacy of mesenchymal stem cell-derived extracellular vesicles against neonatal hyperoxic lung injury. Exp. Mol. Med. 50:26.
Akyurekli, C., Le, Y., Richardson, R. B., Fergusson, D., Tay, J., and Allan, D. S. (2015). A systematic review of preclinical studies on the therapeutic potential of mesenchymal stromal cell-derived microvesicles. Stem Cell Rev. Rep. 11, 150–160. doi: 10.1007/s12015-014-9545-9
Aliotta, J. M., Pereira, M., Wen, S., Dooner, M. S., Del Tatto, M., Papa, E., et al. (2016). Exosomes induce and reverse monocrotaline-induced pulmonary hypertension in mice. Cardiovasc. Res. 110, 319–330. doi: 10.1093/cvr/cvw054
Almqvist, N., Lonnqvist, A., Hultkrantz, S., and Telemo, E. (2008). Serum-derived exosomes from antigen-fed mice prevent allergic sensitization in a model of allergic asthma. Immunology 125, 21–27. doi: 10.1111/j.1365-2567.2008.02812.x
Altan-Bonnet, N. (2016). Extracellular vesicles are the Trojan horses of viral infection. Curr. Opin. Microbiol. 32, 77–81. doi: 10.1016/j.mib.2016.05.004
Alvarez-Erviti, L., Seow, Y., Yin, H., Betts, C., Lakhal, S., and Wood, M. J. A. (2011). Delivery of siRNA to the mouse brain by systemic injection of targeted exosomes. Nat. Biotechnol. 29, 341–345. doi: 10.1038/nbt.1807
Antounians, L., Tzanetakis, A., Pellerito, O., Catania, V. D., Sulistyo, A., Montalva, L., et al. (2019). The regenerative potential of amniotic fluid stem cells extracellular vesicles: lessons learned by comparing different isolation techniques. Sci. Rep. 9:1837.
Antunes, M. A., Abreu, S. C., Cruz, F. F., Teixeira, A. C., Lopes-Pacheco, M., Bandeira, E., et al. (2014). Effects of different mesenchymal stromal cell sources and delivery routes in experimental emphysema. Respir. Res. 15:118.
Asef, A., Mortaz, E., Jamaati, H., and Velayati, A. (2018). Immunologic role of extracellular vesicles and exosomes in the pathogenesis of cystic fibrosis. Tanaffos 17, 66–72.
Atkin-Smith, G. K., Tixeira, R., Paone, S., Mathivanan, S., Colling, C., Liem, M., et al. (2015). A novel mechanism of generating extracellular vesicles during apoptosis via a beads-on-a-string membrane structure. Nat. Commun. 6:7439.
Baglio, S. R., Pegtel, D. M., and Baldini, N. (2012). Mesenchymal stem cell secreted vesicles provide novel opportunities in (stem) cell-free therapy. Front. Physiol. 3:359. doi: 10.3389/fphys.2012.00359
Baixauli, F., López-Otín, C., and Mittelbrunn, M. (2014). Exosomes and autophagy: coordinated mechanisms for the maintenance of cellular fitness. Front. Immunol. 5:403. doi: 10.3389/fimmu.2014.00403
Bandeira, E., Oliveira, H., Silva, J. D., Menna-Barreto, R. F. S., Takyia, C. M., Suk, J. S., et al. (2019). Therapeutic effects of adipose-tissue-derived mesenchymal stromal cells and their extracellular vesicles in experimental silicosis. Respir. Res. 19:104.
Bari, E., Ferrarotti, I., Di Silvestre, D., Grisoli, P., Barzon, V., Balderacchi, A., et al. (2019). Adipose mesenchymal extracellular vesicles as alpha-1-antitrypsin physiological delivery systems for lung regeneration. Cells 8:E965. doi: 10.3390/cells8090965
Batson, B., Arifuzzaman, M., Haridass, P., Dand, H., Mieczkowski, P., Ribeiro, C., et al. (2016). Qualitative and quantitative changes of gel forming mucins and exosomes in response to infection and inflammation in the airways. Am. J. Respir. Crit. Care Med. 2016, A5565–A5565.
Bell, G. I., Meschino, M. T., Hughes-Large, J. M., Broughton, H. C., Xenocostas, A., and Hess, D. A. (2012). Combinatorial human progenitor cell transplantation optimizes islet regeneration through secretion of paracrine factors. Stem Cells Dev. 21, 1863–1876. doi: 10.1089/scd.2011.0634
Bellani, G., Laffey, J. G., Pham, T., Fan, E., Brochard, L., Esteban, A., et al. (2016). Epidemiology, patterns of care, and mortality for patients with acute respiratory distress syndrome in intensive care units in 50 countries. JAMA 315, 788–800. doi: 10.1001/jama.2016.0291
Bourdonnay, E., Zaslona, Z., Penke, L. R. K., Speth, J. M., Schneider, D. J., Przybranowski, S., et al. (2015). Transcellular delivery of vesicular SOCS proteins from macrophages to epithelial cells blunts inflammatory signaling. J. Exp. Med. 212, 729–742. doi: 10.1084/jem.20141675
Braun, R. K., Chetty, C., Balasubramaniam, V., Centanni, R., Haraldsdottir, K., Hematti, P., et al. (2018). Intraperitoneal injection of MSC-derived exosomes prevent experimental bronchopulmonary dysplasia. Biochem. Biophys. Res. Commun. 503, 2653–2658. doi: 10.1016/j.bbrc.2018.08.019
Bruno, S., and Camussi, G. (2013). Role of mesenchymal stem cell-derived microvesicles in tissue repair. Pediatr. Nephrol. 28, 2249–2254. doi: 10.1007/s00467-013-2413-z
Bruno, S., Deregibus, M. C., and Camussi, G. (2015). The secretome of mesenchymal stromal cells: role of extracellular vesicles in immunomodulation. Immunol. Lett. 168, 154–158. doi: 10.1016/j.imlet.2015.06.007
Budoni, M., Fierabracci, A., Luciano, R., Petrini, S., Di Ciommo, V., and Muraca, M. (2013). The immunosuppressive effect of mesenchymal stromal cells on B lymphocytes is mediated by membrane vesicles. Cell Transpl. 22, 369–379. doi: 10.3727/096368911x582769b
Burnham, E. L., Janssen, W. J., Riches, D. W., Moss, M., and Downey, G. P. (2014). The fibroproliferative response in acute respiratory distress syndrome: mechanisms and clinical significance. Eur. Respir. J. 43, 276–285. doi: 10.1183/09031936.00196412
Camussi, G., Deregibus, M. C., Bruno, S., and Biancone, L. (2010). Exosomes/microvesicles as a mechanism of cell-to-cell communication. Kidney Int. 78, 838–848. doi: 10.1038/ki.2010.278
Camussi, G., Deregibus, M. C., and Cantaluppi, V. (2013). Role of stem-cell-derived microvesicles in the paracrine action of stem cells. Biochem. Soc. Trans. 41, 283–287. doi: 10.1042/bst20120192
Castro, L. L., Kitoko, J. Z., Xisto, D. G., Olsen, P. C., and Guedes, H. L. M. (2020). Multiple doses of adipose tissue-derived mesenchymal stromal cells induce immunosuppression in experimental asthma. Stem Cells Transl. Med. 9, 250–260. doi: 10.1002/sctm.19-0120
Chan, M. C., Kuok, D. I., Leung, C. Y., Hui, K. P. Y., Valkenburg, S. A., Lau, E. H. Y., et al. (2016). Human mesenchymal stromal cells reduce influenza A H5N1-associated acute lung injury in vitro and in vivo. Proc. Natl. Acad. Sci. U.S.A. 113, 3621–3626. doi: 10.1073/pnas.1601911113
Chaubey, S., Thueson, S., Ponnalagu, D., Alam, M. A., Gheorghe, C. P., Aghai, Z., et al. (2018). Early gestational mesenchymal stem cell secretome attenuates experimental bronchopulmonary dysplasia in part via exosome-associated factor TSG-6. Stem Cell Res. Ther. 9:173.
Chen, J., Liu, Z., Hong, M. M., Zhang, H., Chan, C., Xiao, M., et al. (2014). Proangiogenic compositions of microvesicles derived from human umbilical cord mesenchymal stem cells. PLoS One 9:e115316. doi: 10.1371/journal.pone.0115316
Chen, J. Y., An, R., Liu, Z. J., Wang, J.-J., Chen, S.-Z., Hong, M.-M., et al. (2014). Therapeutic effects of mesenchymal stem cell-derived microvesicles on pulmonary arterial hypertension in rats. Acta Pharmacol. Sin. 35, 1121–1128. doi: 10.1038/aps.2014.61
Chen, W., Huang, Y., Han, J., Yu, L., Li, Y., Lu, Z., et al. (2016). Immunomodulatory effects of mesenchymal stromal cells-derived exosome. Immunol. Res. 64, 831–840. doi: 10.1007/s12026-016-8798-6
Chen, W.-X., Zhou, J., Zhou, S.-S., Zhang, Y.-D., Ji, T.-Y., Zhang, X.-L., et al. (2020). Microvesicles derived from human Wharton’s jelly mesenchymal stem cells enhance autophagy and ameliorate acute lung injury via delivery of miR-100. Stem Cell Res. Ther. 11:113.
Cocucci, E., Racchetti, G., and Meldolesi, J. (2009). Shedding microvesicles: artefacts no more. Trends Cell Biol. 19, 43–51. doi: 10.1016/j.tcb.2008.11.003
Collino, F., Deregibus, M. C., Bruno, S., Sterpone, L., Aghmo, G., Viltono, L., et al. (2010). Microvesicles derived from adult human bone marrow and tissue specific mesenchymal stem cells shuttle selected pattern of miRNAs. PLoS One 5:e11803. doi: 10.1371/journal.pone.0011803
Cordazzo, C., Petrini, S., Neri, T., Lombardi, S., Carmazzi, Y., Pedrinelli, R., et al. (2014). Rapid shedding of proinflammatory microparticles by human mononuclear cells exposed to cigarette smoke is dependent on Ca2+ mobilization. Inflamm. Res. 63, 539–547. doi: 10.1007/s00011-014-0723-7
Corsello, T., Kudlicki, A. S., Garofalo, R. P., and Casola, A. (2019). Cigarette smoke condensate exposure changes RNA content of extracellular vesicles released from small airway epithelial cells. Cells 8:1652. doi: 10.3390/cells8121652
Cruz, F. F., Borg, Z. D., Goodwin, M., Sokocevic, D., Wagner, D. E., Coffey, A., et al. (2015). Systemic administration of human bone marrow-derived mesenchymal stromal cell extracellular vesicles ameliorates Aspergillus Hyphal extract-induced allergic airway inflammation in immunocompetent mice. Stem Cells Transl. Med. 4, 1302–1316. doi: 10.5966/sctm.2014-0280
Cruz, F. F., and Rocco, P. R. M. (2020). The potential of mesenchymal stem cell therapy for chronic lung disease. Expert Rev. Respir. Med. 14, 31–39. doi: 10.1080/17476348.2020.1679628
de Castro, L. L., Lopes-Pacheco, M., Weiss, D. J., Cruz, F. F., and Rocco, P. R. M. (2019). Current understanding of the immunosuppressive properties of mesenchymal stromal cells. J. Mol. Med. 97, 605–618. doi: 10.1007/s00109-019-01776-y
de Castro, L. L., Xisto, D. G., Kitoko, J. Z., Cruz, F. F., Olsen, P. C., Redondo, P. A. G., et al. (2017). Human adipose tissue mesenchymal stromal cells and their extracellular vesicles act differentially on lung mechanics and inflammation in experimental allergic asthma. Stem Cell Res. Ther. 8:151.
de Oliveira, H. D., de Melo, E. B. B., Silva, J. D., Kitoko, J. Z., Gutfilen, B., Barboza, T., et al. (2017). Therapeutic effects of boné marrow-derived mononuclear cells from healthy or silicotic donors on recipient silicosis mice. Stem Cell Res. Ther. 8:259.
Deregibus, M. C., Figliolini, F., D’Antico, S., Manzini, P. M., Pasquino, C., De Lena, M., et al. (2016). Charge-based precipitation of extracellular vesicles. Int. J. Mol. Med. 38, 1359–1366.
Di Trapani, M., Bassi, G., Midolo, M., Gatti, A., Kamga, P. T., Cassaro, A., et al. (2016). Differential and transferable modulatory effects of mesenchymal stromal cell-derived extracellular vesicles on T. B and NK cell functions. Sci. Rep. 6:24120.
Didiot, M. C., Hall, L. M., Coles, A. H., Haraszti, R. A., Godinho, B. M., Chase, K., et al. (2016). Exosome-mediated delivery of hydrophobically modified siRNA for huntingtin mRNA silencing. Mol. Ther. 24, 1836–1847. doi: 10.1038/mt.2016.126
Dooner, M. S., Aliotta, J. M., Pimentel, J., Dooner, G. J., Abedi, M., Colvin, G., et al. (2008). Conversion potential of marrow cells into lung cells fluctuates with cytokine-induced cell cycle. Stem Cells Dev. 17, 207–219. doi: 10.1089/scd.2007.0195
Downey, D. G., Bell, S. C., and Elborn, J. S. (2009). Neutrophils in cystic fibrosis. Thorax 64, 81–88.
Du, Y. M., Zhuansun, Y. X., Chen, R., Lin, L., Lin, Y., and Li, J.-G. (2018). Mesenchymal stem cell exosomes promote immunosuppression of regulatory T cells in asthma. Exp. Cell. Res. 363, 114–120. doi: 10.1016/j.yexcr.2017.12.021
Eirin, A., Riester, S. M., Zhu, X. Y., Tang, H., Evans, J. M., O’Brien, D., et al. (2014). MicroRNA and mRNA cargo of extracellular vesicles from porcine adipose tissue-derived mesenchymal stem cells. Gene 551, 55–64. doi: 10.1016/j.gene.2014.08.041
Eissa, N. T. (2013). The exosome in lung diseases: message in a bottle. J. Allergy Clin. Immunol. 131, 904–905. doi: 10.1016/j.jaci.2013.01.021
Etheridge, A., Lee, I., Hood, L., Galas, D., and Wang, K. (2011). Extracellular microRNA: a new source of bioindicators. Mutat. Res. 717, 85–90.
Fang, S.-B., Zhang, H.-Y., Wang, C., He, B.-X., Liu, X.-Q., Meng, X.-C., et al. (2020). Small extracellular vesicles derived from human mesenchymal stromal cells prevent group 2 innate lymphoid cell-dominant allergic airway inflammation through delivery of miR-146a-5p. J. Extracell. Ves. 9:1723260. doi: 10.1080/20013078.2020.1723260
Francisco-Garcia, A. S., Garrido-Martín, E. M., Rupani, H., Lau, L. C. K., Martinez-Nunez, R. T., Howarth, P. H., et al. (2019). Small RNA species and microRNA Profiles are altered in severe asthma nanovesicles from broncho alveolar lavage and associate with impaired lung function and inflammation. Noncod. RNA 5:51. doi: 10.3390/ncrna5040051
Frydrychowicz, M., Kolecka-Bednarczyk, A., Madejczyk, M., Yasar, S., and Dworachi, G. (2015). Exosomes - structure, biogenesis and biological role in non-small-cell lung cancer. Scand. J. Immunol. 81, 2–10. doi: 10.1111/sji.12247
Fujita, Y., Kadota, T., Araya, J., Ochiya, T., and Kuwano, K. (2018). Extracellular vesicles: new players in lung immunity. Am. J. Respir. Cell Mol. Biol. 58, 560–565.
Fujita, Y., Kosaka, N., Araya, J., Kuwano, K., and Ochiya, T. (2015). Extracellular vesicles in lung microenvironment and pathogenesis. Trends Mol. Med. 21, 533–542. doi: 10.1016/j.molmed.2015.07.004
Galleu, A., Riffo-Vasquez, Y., Trento, C., Lomas, C., Dolvetti, L., Cheung, T. S., et al. (2017). Apoptosis in mesenchymal stromal cells induces in vivo recipiente-mediated immunomodulation. Sci. Transl. Med. 9:eaam7828. doi: 10.1126/scitranslmed.aam7828
Gámez-Valero, A., Monguió-Tortajada, M., Carreras-Planella, L., La Franquesa, M., Beyer, K., and Borràs, F. E. (2016). Size-exclusion chromatography-based isolation minimally alters extracellular vesicles’ characteristics compared to precipitating agents. Sci. Rep. 6:33641.
Gao, Y., Sun, J., Dong, C., Zhao, M., Hu, Y., and Jin, F. (2020). Extracellular vesicles derived from adipose mesenchymal stem cells alleviate PM2.5-induced lung injury and pulmonary fibrosis. Med. Sci. Monit. 26:e922782.
Gauthier, M., Ray, A., and Wenzel, S. E. (2015). Evolving concepts of asthma. Am. J. Respir. Crit. Care Med. 192, 660–668. doi: 10.1164/rccm.201504-0763pp
Genschmer, K. R., Russell, D. W., Lal, C., Szul, T., Bratcher, P. E., Noerager, B. D., et al. (2019). Activated PMN exosomes: pathogenic entities causing matrix destruction and disease in the lung. Cell 176, 113–126. doi: 10.1016/j.cell.2018.12.002
Giebel, B., Lambros, K., and Verena, B. (2017). Clinical potential of mesenchymal stem/stromal cell-derived extracellular vesicles. Stem Cell Invest. 4:84. doi: 10.21037/sci.2017.09.06
Gimona, M., Pachler, K., Laner-Plamberger, S., Schallmoser, K., and Rohde, E. (2017). Manufacturing of human extracellular vesicle-based therapeutics for clinical use. Int. J. Mol. Sci. 18:1190. doi: 10.3390/ijms18061190
Global Initiative for Chronic Obstructive Lung Disease [GOLD] (2020). Global Strategy for the Diagnosis, Management, and Prevention of Chronic Obstructive Pulmonary Disease. Available online at: https://goldcopd.org/gold-reports/ (accessed August 30, 2020).
GINA (2020). Global Strategy for Asthma Management and Prevention. Global Initiative for Asthma 2020. Available online at: https://ginasthma.org/2020-gina-report-global-strategy-for-asthma- management-and-prevention/ (accessed August 30, 2020).
Gomzikova, M. O., James, V., and Rizvanov, A. A. (2019). Therapeutic application of mesenchymal stem cells derived extracellular vesicles for immunomodulation. Front. Immunol. 10:2663. doi: 10.3389/fimmu.2019.02663
Gon, Y., Maruoka, S., Inoue, T., Kuroda, K., Yamagishi, K., Kozu, Y., et al. (2017). Selective release of miRNAs via extracellular vesicles is associated with house-dust mite allergen-induced airway inflammation. Clin. Exp. Allergy 47, 1586–1598. doi: 10.1111/cea.13016
Gon, Y., Shimizu, T., Mizumura, K., Maruoka, S., and Hikichi, M. (2020). Molecular techniques for respiratory diseases: MicroRNA and extracellular vesicles. Respirology 25, 149–160. doi: 10.1111/resp.13756
Goolaerts, A., Pellan-Randrianarison, N., Larghero, J., Vanneaus, V., Uzunhan, Y., Gille, T., et al. (2014). Conditioned media from mesenchymal stromal cells restore sodium transport and preserve epithelial permeability in an in vitro model of acute alveolar injury. Am. J. Physiol. Lung Cell Mol. Physiol. 306, L975–L985.
Gowen, A., Shahjin, F., Chand, S., Odegaard, K. E., and Yelamanchili, S. V. (2020). Mesenchymal stem cell-derived extracellular vesicles: challenges in clinical applications. Front. Cell Dev. Biol. 8:149. doi: 10.3389/fcell.2020.00149
Guiot, J., Struman, I., Louis, E., Louis, R., Malaise, M., and Njock, M.-S. (2019). Exosomal miRNAs in lung diseases: from biologic function to therapeutic targets. J. Clin. Med. 8:1345. doi: 10.3390/jcm8091345
Hao, Q., Gudapati, V., Monsel, A., Park, J. H., and Ho, S. (2019). Mesenchymal stem cell-derived extracellular vesciles decrese lung injury in Mice. J. Immunol. 203, 1961–1972.
Harding, C., Heuser, J., and Stahl, P. (1983). Receptor-mediated endocytosis of transferrin and recycling of the transferrin receptor in rat reticulocytes. J. Cell. Biol. 97, 329–339. doi: 10.1083/jcb.97.2.329
Hayes, M., Curley, G. F., Masterson, C., Devaney, J., O’Toole, D., and Laffey, J. G. (2015). Mesenchymal stromal cells are more effective than the MSC secretome in diminishing injury and enhancing recovery following ventilator-induced lung injury. Intens. Care Med. Exp. 3:29.
Henne, W. M., Stenmark, H., and Emr, S. D. (2013). Molecular mechanisms of the membrane sculpting ESCRT pathway. Cold Spring Harb. Perspect. Biol. 5:a016766. doi: 10.1101/cshperspect.a016766
Hessvik, N. P., and Llorente, A. (2018). Current knowledge on exosome biogenesis and release. Cell. Mol. Life Sci. 75, 193–208. doi: 10.1007/s00018-017-2595-9
Hewitt, R., Farne, H., Ritchie, A., Luke, E., Johnston, S. L., and Mallia, P. (2016). The role of viral infections in exacerbations of chronic obstructive pulmonary disease and asthma. Ther. Adv. Respir. Dis. 10, 158–174.
Hofmann, N. A., Ortner, A., Jacamo, R. O., Reinisch, A., Schallmoser, K., Rohban, R., et al. (2012). Oxygen sensing mesenchymal progenitors promote neo-vasculogenesis in a humanized mouse model in vivo. PLoS One 7:e44468. doi: 10.1371/journal.pone.0044468
Hu, S., Park, J., Liu, A., Lee, J., Zhang, X., Hao, Q., et al. (2018). Mesenchymal stem cell microvesicles restore protein permeability across primary cultures of injured human lung microvascular endothelial cells. Stem Cells Transl. Med. 7, 615–624. doi: 10.1002/sctm.17-0278
Ismail, N., Wang, Y., Dakhlallah, D., Moldovan, L., Agarwal, K., Batter, K., et al. (2013). Macrophage microvesicles induce macrophage differentiation and miR-223 transfer. Blood 121, 984–995. doi: 10.1182/blood-2011-08-374793
Kadota, T., Fujita, Y., Yoshioka, Y., Araya, J., Kuwano, K., and Ochiya, T. (2016). Extracellular vesicles in chronic obstructive pulmonary disease. Int. J. Mol. Sci. 17:1801.
Kang, J.-H., Jung, M.-Y., Choudhury, M., and Leof, E. B. (2019). Transforming growth factor beta induces fibroblasts to express and release the immunomodulatory protein PD-L1 into extracellular vesicles. FASEB J. 34, 2213–2226. doi: 10.1096/fj.201902354r
Katsuda, T., Kosaka, N., Takeshita, F., and Ochiya, T. (2013). The therapeutic potential of mesenchymal stem cell-derived extracellular vesicles. Proteomics 13, 1637–1653.
Kern, S., Eichler, H., Stoeve, J., Klüter, H., and Bieback, K. (2019). Comparative analysis of mesenchymal stem cells from bone marrow, umbilical cord blood, or adipose tissue. Stem Cells 24, 1294–1301. doi: 10.1634/stemcells.2005-0342
Kerr, J. F., Wyllie, A. H., and Currie, A. R. (1972). Apoptosis: a basic biological phenomenon with wide-ranging implications in tissue kinetics. Br. J. Cancer 26, 239–257. doi: 10.1038/bjc.1972.33
Kesimer, M., and Gupta, R. (2015). Physical characterization and profiling of airway epithelial derived exosomes using light scattering. Methods 87, 59–63. doi: 10.1016/j.ymeth.2015.03.013
Kesimer, M., Scull, M., Brighton, B., DeMaria, G., Burns, K., O’Neal, W., et al. (2009). Characterization of exosome-like vesicles released from human tracheobronchial ciliated epithelium: a possible role in innate defense. FASEB J. 23, 1858–1868. doi: 10.1096/fj.08-119131
Khatri, M., Richardson, L. A., and Meulia, T. (2018). Mesenchymal stem cell-derived extracellular vesicles attenuate influenza virus-induced acute lung injury in a pig model. Stem Cell Res. Ther. 9:17.
Kim, H. J., Kim, Y. S., Kim, K. H., Choi, J.-P., Kim, Y.-K., Yun, S., et al. (2017). The microbiome of the lung and its extracellular vesicles in nonsmokers, healthy smokers and COPD patients. Exp. Mol. Med. 49:e316. doi: 10.1038/emm.2017.7
Kim, Y. S., Kim, J. Y., Cho, R., Shin, D.-M., Lee, S. W., and Oh, Y.-M. (2017). Adipose stem cell-derived nanovesicles inhibit emphysema primarily via an FGF2-dependent pathway. Exp. Mol. Med. 49:e284. doi: 10.1038/emm.2016.127
Kim, Y. S., Lee, W.-H., Choi, E.-J., Choi, J.-P., Heo, Y. J., Gho, Y. S., et al. (2015). Extracellular vesicles derived from Gram-negative bacteria, such as Escherichia coli, induce emphysema mainly via IL-17A-mediated neutrophilic inflammation. J. Immunol. 194, 3361–3368. doi: 10.4049/jimmunol.1402268
Kinoshita, T., Yip, K. W., Spence, T., and Liu, F.-F. (2017). MicroRNAs in extracellular vesicles: potential cancer bioindicators. J. Hum. Genet. 62, 67–74. doi: 10.1038/jhg.2016.87
Klinger, J. R., Pereira, M., Del Tatto, M., Brodsky, A. S., Wu, Q. K., Dooner, M. S., et al. (2020). Mesenchymal stem cells extracellular vesicles reverse Sugen/hypoxia pulmonary hypertension in rats. Am. J. Respir. Cell. Mol. Biol. 62, 577–587. doi: 10.1165/rcmb.2019-0154oc
Konoshenko, M. Y., Lekchnov, E. A., Vlassov, A. V., and Laktionov, P. P. (2018). Isolation of extracellular vesicles: general methodologies and latest trends. Biomed. Res. Int. 2018:8545347.
Kowal, J., Tkach, M., and Theìry, C. (2014). Biogenesis and secretion of exosomes. Curr. Opin. Cell Biol. 29, 116–125. doi: 10.1016/j.ceb.2014.05.004
Kulshreshtha, A., Ahmad, T., Agrawal, A., and Ghosh, B. (2013). Proinflammatory role of epithelial cell-derived exosomes in allergic airway inflammation. J. Allergy Clin. Immunol. 131, 1194–1203. doi: 10.1016/j.jaci.2012.12.1565
Lacy, S. H., Woeller, C. F., Thatcher, T. H., Pollack, S. J., Small, E. M., Sime, P. J., et al. (2019). Activated human lung fibroblasts produce extracellular vesicles with antifibrotic prostaglandins. Am. J. Respir. Cell Mol. Biol. 60, 269–278. doi: 10.1165/rcmb.2017-0248oc
Lai, R. C., Yeo, R. W., and Lim, S. K. (2015). Mesenchymal stem cell exosomes. Semin. Cell Dev. Biol. 40, 82–88.
Lalu, M. M., McIntyre, L., Pugliese, C., Fergusson, D., Winston, B. W., Marshall, J. C., et al. (2012). Safety of cell therapy with mesenchymal stromal cells (SafeCell), a systematic review and meta-analysis of clinical trials. PLoS One 7:e47559. doi: 10.1371/journal.pone.0047559
Lamichhane, T. N., Jeyaram, A., Patel, D. B., Parajuli, B., Livingston, N. K., Arumudasaamy, N., et al. (2016). Oncogene knockdown via active loading of small rnas into extracellular vesicles by sonication. Cell. Mol. Bioeng. 9, 315–324. doi: 10.1007/s12195-016-0457-4
Lee, C., Mitsialis, S. A., Aslam, M., Vitali, S. H., Vergadi, E., Konstantinou, G., et al. (2012). Exosomes mediate the cytoprotective action of mesenchymal stromal cells on hypoxia-induced pulmonary hypertension. Circulation 126, 2601–2611. doi: 10.1161/circulationaha.112.114173
Lee, E.-Y., Choi, D.-Y., Kim, D.-K., Kim, J.-W., Park, J. O., Kim, S., et al. (2009). Gram-positive bacteria produce membrane vesicles: proteomics-based characterization of Staphylococcus aureus-derived membrane vesicles. Proteomics 9, 5425–5436. doi: 10.1002/pmic.200900338
Li, J. W., Wie, L., Han, Z., and Chen, Z. (2019). Mesenchymal stromal cells-derived exosomes alleviate ischemia/reperfusion injury in mouse lung by transporting anti-apoptotic miR-21-5p. Eur. J. Pharmacol. 852, 68–76. doi: 10.1016/j.ejphar.2019.01.022
Li, M., Yu, D., Williams, K. J., and Liu, M.-L. (2010). Tobacco smoke induces the generation of procoagulant microvesicles from human monocytes/macrophages. Arterioscler. Thromb. Vasc. Biol. 30, 1818–1824. doi: 10.1161/atvbaha.110.209577
Lopes-Pacheco, M. (2016). CFTR modulators: shedding light on precision medicine for cystic fibrosis. Front. Pharmacol. 7:275. doi: 10.3389/fphar.2016.00275
Lopes-Pacheco, M. (2020). CFTR modulators: the changing face of cystic fibrosis in the era of precision medicine. Front. Pharmacol. 10:1662. doi: 10.3389/fphar.2019.01662
Lopes-Pacheco, M., Bandeira, E., and Morales, M. M. (2016). Cell-based therapy for silicosis. Stem Cells Int. 2016:5091838.
Lopes-Pacheco, M., Robba, C., Rocco, P. R. M., and Pelosi, P. (2020). Current understanding of the therapeutic benefits of mesenchymal stem cells in acute respiratory distress syndrome. Cell Biol. Toxicol. 36, 83–102. doi: 10.1007/s10565-019-09493-5
Lopes-Pacheco, M., Silva, P. L., Cruz, F. F., Battaglini, D., Robba, C., Pelosi, P., et al. (2021). Pathogenesis and multiple organ injury in COVID-19 and potential therapeutic strategies. Front. Physiol. 12:593223. doi: 10.3389/fphys.2021.593223
Lopes-Pacheco, M., Xisto, D. G., Ornellas, F. M., Antunes, M. A., Abreu, S. C., Rocco, P. R. M., et al. (2013). Repeated administration of bone marrow-derived cells prevents disease progression in experimental silicosis. Cell. Physiol. Biochem. 32, 1681–1694. doi: 10.1159/000356603
Lötvall, J., Hill, A. F., Hochberg, F., Buzás, E. I., De Vizio, D., Fardiner, C., et al. (2014). Minimal experimental requirements for definition of extracellular vesicles and their functions: a position statement from the International society for extracellular vesicles. J. Extracell. Ves. 3:26913. doi: 10.3402/jev.v3.26913
Makiguchi, T., Yamada, M., Yoshioka, Y., Sugiura, H., and Kaorai, A. (2016). Serum extracellular vesicular miR-21- 5p is a predictor of the prognosis in idiopathic pulmonary fibrosis. Respir. Res. 17:110.
Mansouri, N., Willis, G. R., Fernandez-Gonzalez, A., Reis, M., Nassiri, S., Mitsialis, S. A., et al. (2019). Mesenchymal stromal cell exosomes prevent and revert experimental pulmonar fibrosis through modulation of monocyte phenotypes. JCI Insight 4:e128060.
Matthay, M. A., Calfee, C. S., Zhuo, H., Thompson, B. T., Wilson, J. F., Levitt, J. E., et al. (2019). Treatment with allogeneic mesenchymal stromal cells for moderate to severe acute respiratory distress syndrome (START study), a randomised phase 2a safety trial. Lancet Respir. Med. 7, 154–162. doi: 10.1016/s2213-2600(18)30418-1
Maumus, M., Rozier, P., Boulestreau, J., Jorgensen, C., and Noël, D. (2020). Mesenchymal stem cell-derived extracellular vesicles: opportunities and challenges for clinical translation. Front. Bioeng. Biotechnol. 8:997. doi: 10.3389/fbioe.2020.00997
McIntyre, L. A., Stewart, D. J., Mei, S. H. J., Courtman, D., Watpool, I., Granton, J., et al. (2018). Cellular immunotherapy for septic shock. A phase I clinical trial. Am. J. Respir. Crit. Care Med. 197, 337–347. doi: 10.1164/rccm.201705-1006oc
Mendt, M., Kamerkar, S., Sugimoto, H., McAndrews, K. M., Wu, C. C., Gagea, M., et al. (2018). Generation and testing of clinical-grade exosomes for pancreatic cancer. JCI Insight 3:e99263.
Minciacchi, V. R., Freeman, M. R., and Di Vizio, D. (2015). Extracellular vesicles in cancer: exosomes, microvesicles and the emerging role of large oncosomes. Semin. Cell Dev. Biol. 40, 41–51. doi: 10.1016/j.semcdb.2015.02.010
Mitri, C., Xu, Z., Bardin, P., Corvol, H., Touqui, L., and Tabary, O. (2020). Novel anti-inflammatory approaches for cystic fibrosis lung disease. Identification of molecular targets and design of innovative therapies. Front. Pharmacol. 11:1096. doi: 10.3389/fphar.2020.01096
Mohammadipoor, A., Antebi, B., Batchinsky, A. I., and Cancion, L. C. (2018). Therapeutic potential of products derived from mesenchymal stem/stromal cells in pulmonary disease. Respir. Res. 19:218.
Mokarizadeh, A., Delirezh, N., Morshedi, A., Mosayebi, G., Darshid, A.-A., and Mardani, K. (2012). Microvesicles derived from mesenchymal stem cells: potent organelles for induction of tolerogenic signaling. Immunol. Lett. 147, 47–54. doi: 10.1016/j.imlet.2012.06.001
Monsel, A., Zhu, Y. G., Gennai, S., Hao, Q., Hu, S., Rouby, J.-J., et al. (2015). Therapeutic effects of human mesenchymal stem cell-derived microvesicles in severe pneumonia in mice. Am. J. Respir. Crit. Care Med. 192, 324–336. doi: 10.1164/rccm.201410-1765oc
Monsel, A., Zhu, Y. G., Gennai, S., Hao, Q., Liu, J., and Lee, J. W. (2014). Cell-based therapy for acute organ injury: preclinical evidence and ongoing clinical trials using mesenchymal stem cells. Anesthesiology 121, 1099–1121.
Morrison, T. J., Jackson, M. V., Cunningham, E. K., Kissenpfenning, A., McAuley, D. F., O’Kane, C. M., et al. (2017). Mesenchymal stromal cells modulate macrophages in clinically relevant lung injury models by extracellular vesicle mitochondrial transfer. Am. J. Respir. Crit. Care Med. 196, 1275–1286. doi: 10.1164/rccm.201701-0170oc
O’Farrell, H. E., and Yang, I. A. (2019). Extracellular vesicles in chronic obstructive pulmonary disease (COPD). J. Thorac. Dis. 11, (Suppl. 17), S2141–S2154.
Orme, J. Jr., Romney, J. S., Hopkins, R. O., Pope, D., Chan, K. J., Thomsen, G., et al. (2003). Pulmonary function and health-related quality of life in survivors of acute respiratory distress syndrome. Am. J. Respir. Crit. Care Med. 167, 690–694. doi: 10.1164/rccm.200206-542oc
Padilha, G. A., Henriques, I., Lopes-Pacheco, M., Abreu, S. C., Oliveira, M. V., Morales, M. M., et al. (2015). Therapeutic effects of LASSBio-596 innan elastase-induced mouse model of emphysema. Front. Physiol. 6:267. doi: 10.3389/fphys.2015.00267
Pan, B. T., Teng, K., Wu, C., Adam, M., and Johnstone, R. M. (1985). Electron microscopic evidence for externalization of the transferrin receptor in vesicular form in sheep reticulocytes. J. Cell Biol. 101, 942–948. doi: 10.1083/jcb.101.3.942
Papierniak, E. S., Lowenthal, D. T., and Harman, E. (2013). Novel therapies in asthma: leukotriene antagonists, biologic agents, and beyond. Am. J. Ther. 20, 79–103. doi: 10.1097/mjt.0b013e31826915c2
Park, J., Kim, S., Lim, H., Liu, A., Hu, S., Lee, J., et al. (2019). Therapeutic effects of human mesenchymal stem cell microvesicles in an ex vivo perfused human lung injured with severe E. coli pneumonia. Thorax 74, 43–50. doi: 10.1136/thoraxjnl-2018-211576
Phinney, D. G., Di Giuseppe, M., Njah, J., Sala, E., Shiva, S., St Croix, C. M., et al. (2015). Mesenchymal stem cells use extracellular vesicles to outsource mitophagy and shuttle microRNAs. Nat. Commun. 6:8472.
Poggio, H. A., Antunes, M. A., Rocha, N. N., Kitoko, J. Z., Morales, M. M., Olsen, P. C., et al. (2018). Impact of one versus two doses of mesenchymal stromal cells on lung and cardiovascular repair in experimental emphysema. Stem Cell Res. Ther. 9:293.
Popowski, K., Lutz, H., Hu, S., George, A., Phuong-Uyen, D., and Cheng, K. (2020). Exosome therapeutics for lung regenerative medicine. J. Extracell. Ves. 9:1785161. doi: 10.1080/20013078.2020.1785161
Porzionato, A., Zaramella, P., Dedja, A., Guidolin, D., Van Wemmel, K., Macchi, V., et al. (2019). Intratracheal administration of clinical-grade mesenchymal stem cell-derived extracellular vesicles reduces lung injury in a rat model of bronchopulmonary dysplasia. Am. J. Physiol. Lung Cell Mol. Physiol. 316, L6–L19.
Prado, N., Marazuela, E. G., Segura, E., Fernández-García, H., Villalba, M., Théry, C., et al. (2008). Exosomes from bronchoalveolar fluid of tolerized mice prevent allergic reaction. J. Immunol. 181, 1519–1525. doi: 10.4049/jimmunol.181.2.1519
Pua, H. H., Happ, H. C., Gray, C. J., Mar, D. J., Chiou, N.-T., Hesse, L. E., et al. (2019). Increased hematopoietic extracellular RNAs and vesicles in the lung during allergic airway responses. Cell Rep. 26, 933–944. doi: 10.1016/j.celrep.2019.01.002
Qazi, K. R., Torregrosa Paredes, P., Dahlberg, B., Grunewald, J., Eklund, A., and Gabrielsson, S. (2010). Proinflammatory exosomes in bronchoalveolar lavage fluid of patients with sarcoidosis. Thorax 65, 1016–1024. doi: 10.1136/thx.2009.132027
Qian, X., Xu, C., Fang, S., Zhao, P., Wang, Y., Liu, H., et al. (2016). Exosomal MicroRNAs derived from umbilical mesenchymal stem cells inhibit Hepatitis C virus infection. Stem Cells Transl. Med. 5, 1190–1203. doi: 10.5966/sctm.2015-0348
Raposo, G., Nijman, H. W., Stoorvogel, W., Liejendekker, R., Harding, C. V., Melief, C. J., et al. (1996). B lymphocytes secrete antigen-presenting vesicles. J. Exp. Med. 183, 1161–1172. doi: 10.1084/jem.183.3.1161
Ray, A., Oriss, T. B., and Wenzel, S. E. (2015). Emerging molecular phenotypes of asthma. Am. J. Physiol. Cell. Mol. Physiol. 308, L130–L140.
Rodrigues, M., Fan, J., Lyon, C., Wan, M., and Hu, Y. (2018). Role of extracellular vesicles in viral and bacterial infections: pathogenesis, diagnostics, and therapeutics. Theranostics 8, 2709–2727. doi: 10.7150/thno.20576
Roy, K., Hamilton, D. J., Munson, G. P., and Fleckenstein, J. M. (2011). Outer membrane vesicles induce immune responses to virulence proteins and protect against colonization by enterotoxigenic Escherichia coli. Clin. Vaccine Immunol. 18, 1803–1808. doi: 10.1128/cvi.05217-11
Sarkar, A., Mitra, S., Mehta, S., Raices, R., and Wewers, M. D. (2009). Monocyte derived microvesicles deliver a cell death message via encapsulated caspase-1. PLoS One 4:e7140. doi: 10.1371/journal.pone.0007140
Sauleda, J., Núnez, B., Saula, E., and Soriano, J. B. (2018). Idiopathic pulmonary fibrosis: epidemiology, natural history, phenotypes. Med. Sci. (Basel). 6:110. doi: 10.3390/medsci6040110
Savarimuthu Francis, S. M., Davidson, M. R., Tan, M. E., Wright, C. M., Clarke, B. E., Duhig, E. E., et al. (2014). MicroRNA-34c is associated with emphysema severity and modulates SERPINE1 expression. BMC Genom. 15:88. doi: 10.1186/1471-2164-15-88
Sengupta, V., Sengupta, S., Lazo, A., Woods, P., Nolan, A., and Bremer, N. (2020). Exosomes derived from bone marrow mesenchymal stem cells as treatment for severe COVID-19. Stem Cells Dev. 29, 747–754. doi: 10.1089/scd.2020.0080
Serban, K. A., Rezania, S., Petrusca, D. N., Poirier, C., Cao, D., Justice, M. J., et al. (2016). Structural and functional characterization of endothelial microparticles released by cigarette smoke. Sci. Rep. 6:31596.
Sethi, S., and Murphy, T. F. (2008). Infection in the pathogenesis and course of chronic obstructive pulmonary disease. N. Engl. J. Med. 359, 2355–2365.
Shentu, T.-P., Huang, T.-S., Cernelc-Kohan, M., Chan, J., Wong, S. S., Espinoza, C. R., et al. (2017). Thy-1 dependent uptake of mesenchymal stem cell-derived extracellular vesicles blocks myofibroblastic differentiation. Sci. Rep. 7:18052.
Silva, J. D., de Castro, L. L., Braga, C. L., Oliveira, G. P., Trivelin, A. S., Barbosa-Junior, C. M., et al. (2019). Mesenchymal stromal cells are more effective than their extracellular vesicles at reducing lung injury regardless of acute respiratory distress syndrome etiology. Stem Cells Int. 2019, 8262849.
Silva, J. D., Lopes-Pacheco, M., Paz, A. H. R., Cruz, F. F., Melo, E. B., de Oliveira, M. V., et al. (2018). Mesenchymal stem cells from bone marrow, adipose tissue, and lung tissue differentially mitigate lung and distal organ damage in experimental acute respiratory distress syndrome. Crit. Care Med. 46, e132–e140.
Simoncini, S., Njock, M.-S., Robert, S., Camoin-Jau, L., Sampol, J., Harlé, J.-R., et al. (2009). TRAIL/Apo2L mediates the release of procoagulant endothelial microparticles induced by thrombin in vitro: a potential mechanism linking inflammation and coagulation. Circ. Res. 104, 943–951. doi: 10.1161/circresaha.108.183285
Singh, D., Agusti, A., Anzueto, A., Barners, P. J., Bourbeau, J., Celli, B. R., et al. (2019). Global strategy for the diagnosis, management, and prevention of chronic obstructive lung disease: the GOLD science committee report 2019. Eur. Respir. J. 53, 1900164.
Song, Y., Dou, H., Li, X., Zhao, X., Li, Y., Liu, D., et al. (2017). Exosomal miR-146a contributes to the enhanced therapeutic efficacy of interleukin-1β-primed mesenchymal stem cells against sepsis. Stem Cells 35, 1208–1221. doi: 10.1002/stem.2564
Stokes, J. R., and Casale, T. B. (2016). Characterization of asthma endotypes: implications for therapy. Ann. Allergy Asthma Immunol. 117, 121–125. doi: 10.1016/j.anai.2016.05.016
Sun, D., Zhuang, X., Xiang, X., Liu, Y., Zhang, S., Liu, C., et al. (2010). A novel nanoparticle drug delivery system: the anti-inflammatory activity of curcumin is enhanced when encapsulated in exosomes. Mol. Ther. 18, 1606–1614. doi: 10.1038/mt.2010.105
Tabary, O., Corvol, H., Boncoeur, E., Chadelat, K., Fitting, C., Cavaillon, J. M., et al. (2006). Adherence of airway neutrophils and inflammatory response are increased in CF airway epithelial cell-neutrophil interactions. Am. J. Physiol. Lung Cell Mol. Physiol. 290, L588–L596.
Takahashi, T., Kobayashi, S., Fujino, N., Suzuki, T., Ota, C., He, M., et al. (2012). Increased circulating endothelial microparticles in COPD patients: a potential bioindicator for COPD exacerbation susceptibility. Thorax 67:1067. doi: 10.1136/thoraxjnl-2011-201395
Tan, D. B. A., Armitage, J., Teo, T. H., Ong, N. E., Shin, H., and Moodley, Y. P. (2017). Elevated levels of circulating exosome in COPD patients are associated with systemic inflammation. Respir. Med. 132, 261–264. doi: 10.1016/j.rmed.2017.04.014
Tan, J. L., Lau, S. N., Leaw, B., Nguyen, H. P. T., Salamonsen, L. A., Saad, M. I., et al. (2018). Annion epithelial cell-derived exosomes restric lung injury and enhance endogenous lung repair. Stem Cells Transl. Med. 7, 180–196. doi: 10.1002/sctm.17-0185
Tang, X. D., Shi, L., Monsel, A., Li, X.-Y., Zhu, H.-L., Zhu, Y.-G., et al. (2017). Mesenchymal stem cell microvesicles attenuate acute lung injury in mice partly mediated by Ang-1 mRNA. Stem Cells 35, 1849–1859. doi: 10.1002/stem.2619
Tauro, B. J., Greening, D. W., Mathias, R. A., Ji, H., Mathivanan, S., Scott, A. M., et al. (2012). Comparison of ultracentrifugation, density gradient separation, and immunoaffinity capture methods for isolating human colon cancer cell line LIM1863-derived exosomes. Methods 56, 293–304. doi: 10.1016/j.ymeth.2012.01.002
Taylor, D. D., and Shah, S. (2015). Methods of isolating extracellular vesicles impact down-stream analyses of their cargoes. Methods 87, 3–10. doi: 10.1016/j.ymeth.2015.02.019
Tetta, C., Bruno, S., Fonsato, V., Deregibus, M. C., and Camussi, G. (2011). The role of microvesicles in tissue repair. Organogenesis 7, 105–115. doi: 10.4161/org.7.2.15782
Thery, C., Amigorena, S., Raposo, G., and Clayton, A. (2006). Isolation and characterization of exosomes from cell culture supernatants and biological fluids. Curr. Protoc. Cell Biol. 3:Unit3.22.
Theìry, C., Witwer, K. W., Aikawa, E., Alcaraz, M. J., Anderson, J. D., Andriantsitohaina, R., et al. (2018). Minimal information for studies of extracellular vesicles 2018 (MISEV2018), a position statement of the international society for extracellular vesicles and update of the MISEV2014 guidelines. J. Extracell. Ves. 7:1535750.
Ti, D., Hao, H., Tong, C., Liu, J., Dong, L., Zheng, J., et al. (2015). LPS-preconditioned mesenchymal stromal cells modify macrophage polarization for resolution of chronic inflammation via exosome-suttled let-7b. J. Transl. Med. 13:308.
Tomasoni, S., Longaretti, L., Rota, C., Mirigi, M., Conti, S., Gotti, E., et al. (2013). Transfer of growth factor receptor mRNA via exosomes unravels the regenerative effect of mesenchymal stem cells. Stem Cells Dev. 22, 772–780. doi: 10.1089/scd.2012.0266
Torregrosa Paredes, P., Esser, J., Admyre, C., Nord, M., Rahman, Q. K., Lukic, A., et al. (2012). Bronchoalveolar lavage fluid exosomes contribute to cytokine and leukotriene production in allergic asthma. Allergy 67, 911–919. doi: 10.1111/j.1398-9995.2012.02835.x
Trajkovic, K., Hsu, C., Chiantia, S., Rajendran, L., Wenzel, D., Wieland, F., et al. (2008). Ceramide triggers budding of exosome vesicles into multivesicular Endosomes. Science 319, 1244–1247. doi: 10.1126/science.1153124
Varkouhi, A. K., Jerkic, M., Ormesher, L., Gagnon, S., Goyal, S., Rabani, R., et al. (2019). Extracellular vesicles from interferon-gamma-primed human umbilical cord mesenchymal stromal cells reduce Escherichia coli-induced acute lung injury in rats. Anesthesiology 130, 778–790. doi: 10.1097/aln.0000000000002655
Vituret, C., Gallay, K., Confort, M.-P., Ftaich, N., Matei, C. I., Archer, F., et al. (2016). Transfer of the cystic fibrosis transmembrane conductance regulator to human cystic fibrosis cells mediated by extracellular vesicles. Hum. Gene Ther. 27, 166–183. doi: 10.1089/hum.2015.144
Wang, H., Zheng, R., Chen, Q., Shao, J., Yu, J., and Hu, S. (2017). Mesenchymal stem cells microvesicles stabilizes endotelial barrier function partly mediated by hepatocyte growth factor (HGF). Stem Cell Res. Ther. 8:211.
Wang, J., Huang, R., Xu, Q., Zheng, G., Qiu, G., Ge, M., et al. (2020). Mesenchymal stem cell-derived extracellular vesicles alleviate acute lung injury via transfer of miR-27a-3p. Crit. Care Med. 48, e599–e610.
Waszak, P., Alphonse, R., Vadivel, A., Ionescu, L., Eaton, F., and Thébaud, B. (2012). Preconditioning enhances the paracrine effect of mesenchymal stem cells in preventing oxygen-induced neonatal lung injury in rats. Stem Cells Dev. 21, 2789–2797. doi: 10.1089/scd.2010.0566
Wiklander, O. P. B., Brennan, M. Á, Lötvall, J., Breakefield, X. O., and El Andaloussi, S. (2019). Advances in therapeutic applications of extracellular vesicles. Sci. Transl. Med. 11:eaav8521. doi: 10.1126/scitranslmed.aav8521
Willis, G. R., Fernandez-Gonzalez, A., Anastas, J., Vitali, S. H., Liu, X., Ericsson, M., et al. (2018). Mesenchymal Stromal cell Exosomes ameliorate experimental bronchopulmonary Dysplasia and restore lung function through macrophage immunomodulation. Am. J. Respir. Crit. Care Med. 197, 104–116. doi: 10.1164/rccm.201705-0925oc
Witwer, K. W., Soekmadji, C., Hill, A. F., Wauben, M. H., Buzás, E. I., De Vizio, D., et al. (2017). Updating the MISEV minimal requirements for extracellular vesicle studies: building bridges to reproducibility. J. Extracell. Ves. 6:1396823. doi: 10.1080/20013078.2017.1396823
Witwer, K. W., Van Balkom, B. W. M., Bruno, S., Choo, A., Dominici, M., Gimona, M., et al. (2019). Defining mesenchymal stromal cell (MSC)-derived small extracellular vesicles for therapeutic applications. J. Extracell. Ves. 29:1609206. doi: 10.1080/20013078.2019.1609206
Wu, X., Liu, Z., Hu, L., Gu, W., and Zhu, L. (2018). Exosomes derived from endothelial progenitor cells ameliorate acute lung injury by transferring miR-126. Exp. Cell Res. 370, 13–23. doi: 10.1016/j.yexcr.2018.06.003
Xu, H., Ling, M., Xue, J., Dai, X., Sun, Q., Chen, C., et al. (2018). Exosomal microRNA-21 derived from bronchial epithelial cells is involved in aberrant epithelium-fibroblast cross-talk in COPD induced by cigarette smoking. Theranostics 8, 5419–5433. doi: 10.7150/thno.27876
Yi, X., Wei, X., Lv, H., Na, Y., Li, L., Lu, P., et al. (2019). Exosomes derived from microRNA-30b-3p-overexpressing mesenchymal stem cells protect against lipopolysaccharide-induced acute lung injury by inhibiting SAA3. Exp. Cell Res. 383:111454. doi: 10.1016/j.yexcr.2019.05.035
Yu, Q., Wang, D., Wen, X., Tang, X., Qi, D., He, J., et al. (2020). Adipose-derived exosomes protect the pulmonary endothelial barrier in ventilator-induced lung injury by inhibiting the TRPV4/Ca2+ signaling pathway. Am. J. Physiol. Lung Cell Mol Physiol. 318, L723–L741.
Zhang, B., Tian, X., Hao, J., Xu, G., and Zhang, W. (2020). Mesenchymal stem cell-derived extracellular vesicles in tissue regeneration. Cell Transpl. 29:0963689720908500. doi: 10.1177/0963689720908500
Zhang, B., Yin, Y., Lai, R. C., Tan, S. S., Choo, A. B. H., and Lim, S. K. (2014). Mesenchymal stem cells secrete immunologically active exosomes. Stem Cells Dev. 23, 1233–1244. doi: 10.1089/scd.2013.0479
Zhang, C., Wang, P., Mohammed, A., Zhou, Z., Zhang, S., Ni, S., et al. (2020). Function of Adiopse-derived mesenchymal stem cells in monocrotaline-induced pulmonary arterial hypertension through miR-191 via regulation of BMPR2. Biomed. Res. Int. 2019:2858750.
Zhang, D., Lee, H., Zhu, Z., Minhas, J. K., and Jin, Y. (2017). Enrichment of selective miRNAs in exosomes and delivery of exosomal miRNAs in vitro and in vivo. Am. J. Physiol. Lung Cell Mol. Physiol. 312, L110–L121.
Zhao, S., Wehner, R., Bornhauser, M., Wassmuth, R., Bachmann, M., and Schmitz, M. (2010). Immunomodulatory properties of mesenchymal stromal cells and their therapeutic consequences for immune-mediated disorders. Stem Cells Dev. 19, 607–614. doi: 10.1089/scd.2009.0345
Zheng, G., Huang, L., Tong, H., Shu, Q., Hu, Y., Ge, M., et al. (2014). Treatment of acute respiratory distress syndrome with allogeneic adipose-derived mesenchymal stem cells: a randomized, placebo-controlled pilot study. Respir. Res. 15:39. doi: 10.1186/1465-9921-15-39
Zhou, Y., Li, P., Goodwin, A. J., Cook, J. A., Halushka, P. V., Chang, E., et al. (2019). Exosomes from endothelial progenitor cells improve outcomes of the lipopolysaccharide-induced acute lung injury. Crit. Care 23:44.
Zhu, Y. G., Feng, X. M., Abbott, J., Fang, X.-H., Hao, Q., Monsel, A., et al. (2014). Human mesenchymal stem cell microvesicles for treatment of Escherichia coli endotoxin-induced acute lung injury in mice. Stem Cells 32, 116–125. doi: 10.1002/stem.1504
Keywords: biomarkers, cell therapy, extracellular vesicles, inflammation, remodeling, respiratory disease, animal models
Citation: Abreu SC, Lopes-Pacheco M, Weiss DJ and Rocco PRM (2021) Mesenchymal Stromal Cell-Derived Extracellular Vesicles in Lung Diseases: Current Status and Perspectives. Front. Cell Dev. Biol. 9:600711. doi: 10.3389/fcell.2021.600711
Received: 30 August 2020; Accepted: 11 January 2021;
Published: 15 February 2021.
Edited by:
Khalid Ahmed Al-Anazi, Oncology Center, King Fahad Specialist Hospital, Saudi ArabiaReviewed by:
Heedoo Lee, Changwon National University, South KoreaAsma M. Al-Jasser, Riyadh Regional Laboratory, Ministry of Health, Saudi Arabia
Copyright © 2021 Abreu, Lopes-Pacheco, Weiss and Rocco. This is an open-access article distributed under the terms of the Creative Commons Attribution License (CC BY). The use, distribution or reproduction in other forums is permitted, provided the original author(s) and the copyright owner(s) are credited and that the original publication in this journal is cited, in accordance with accepted academic practice. No use, distribution or reproduction is permitted which does not comply with these terms.
*Correspondence: Patricia R. M. Rocco, cHJtcm9jY29AZ21haWwuY29t
†These authors share first authorship