- 1Department of Orthodontics and Dentofacial Orthopedics, Graduate School of Dentistry, Osaka University, Suita, Japan
- 2The Affiliated Stomatology Hospital, Zhejiang University School of Medicine, Hangzhou, China
- 3Key Laboratory of Oral Biomedical Research of Zhejiang Province, Zhejiang University School of Stomatology, Hangzhou, China
- 4Division for Interdisciplinary Dentistry, Osaka University Graduate School of Dentistry, Suita, Japan
- 5Department of Oral Immunology and Infectious Diseases, University of Louisville School of Dentistry, Louisville, KY, United States
- 6Stowers Institute for Medical Research, Kansas City, MO, United States
- 7Department of Anatomy and Cell Biology, University of Kansas Medical Center, Kansas City, KS, United States
The first and second branchiomeric (branchial arch) muscles are craniofacial muscles that derive from branchial arch mesoderm. In mammals, this set of muscles is indispensable for jaw movement and facial expression. Defects during embryonic development that result in congenital partial absence of these muscles can have significant impact on patients’ quality of life. However, the detailed molecular and cellular mechanisms that regulate branchiomeric muscle development remains poorly understood. Herein we investigated the role of retinoic acid (RA) signaling in developing branchiomeric muscles using mice as a model. We administered all-trans RA (25 mg/kg body weight) to Institute of Cancer Research (ICR) pregnant mice by gastric intubation from E8.5 to E10.5. In their embryos at E13.5, we found that muscles derived from the first branchial arch (temporalis, masseter) and second branchial arch (frontalis, orbicularis oculi) were severely affected or undetectable, while other craniofacial muscles were hypoplastic. We detected elevated cell death in the branchial arch mesoderm cells in RA-treated embryos, suggesting that excessive RA signaling reduces the survival of precursor cells of branchiomeric muscles, resulting in the development of hypoplastic craniofacial muscles. In order to uncover the signaling pathway(s) underlying this etiology, we focused on Pitx2, Tbx1, and MyoD1, which are critical for cranial muscle development. Noticeably reduced expression of all these genes was detected in the first and second branchial arch of RA-treated embryos. Moreover, elevated RA signaling resulted in a reduction in Dlx5 and Dlx6 expression in cranial neural crest cells (CNCCs), which disturbed their interactions with branchiomeric mesoderm cells. Altogether, we discovered that embryonic craniofacial muscle defects caused by excessive RA signaling were associated with the downregulation of Pitx2, Tbx1, MyoD1, and Dlx5/6, and reduced survival of cranial myogenic precursor cells.
Introduction
Craniofacial muscles comprise two groups: (1) extraocular muscles, which control eye movement which derive from cranial mesoderm, and (2) branchiomeric muscles, which derive from branchial arch mesoderm. The first branchial arch (BA1, dorsal/rostral maxillary process and a ventral/caudal mandibular process) mesoderm gives rise to progenitors of jaw muscles, including the temporalis, pterygoid, masseter, mylohyoid and anterior digastric; the second branchial arch (BA2, hyoid arch) mesoderm gives rise to progenitors of facial expression muscles, including the auricularis, buccinator, posterior digastric, frontalis, orbicularis oculi, quadratus labii, and zygomaticus; and other caudal BA-derived muscles are associated with laryngeal and pharyngeal muscles (Noden, 1983, 1986; Trainor et al., 1994; Lescroart et al., 2010). Other muscles within the head such as the intrinsic and extrinsic muscles of the tongue derive from occipital somites, whose myogenic precursors migrate as the hypoglossal cord (Noden, 1983; Huang et al., 1999; Noden and Francis-West, 2006; Parada et al., 2012).
Branchiomeric muscles have a gene regulatory program distinct from that of the trunk muscles. For example, Pitx2 and Tbx1 are important upstream regulators of skeletal myogenesis in the branchial arches but not myogenesis in the trunk (Grifone and Kelly, 2007). Pitx2 is a paired-related homeobox gene that regulates transcription of the myogenic regulatory factor genes (MRFs) as well as genes encoding essential factors for proliferation and survival of muscle progenitors in the branchial arches (Dong et al., 2006). Although Pitx2 is expressed in the mesodermal cores of all BAs when myoblasts can be detected, its expression is required to establish premyoblast specification only in BA1 (Shih et al., 2007). Tbx1, which is expressed in the premyoblast mesoderm in BA1 and BA2, collaborates with Pitx2 as part of the core myogenic program to generate head muscles. Additionally, these genes are known to collaborate with other core myogenic program factors to generate head muscles (Dong et al., 2006; Dastjerdi et al., 2007). Similarly, Tbx1 is required for activating transcription of MRFs, such as Myf5 and MyoD, at the onset of myogenic commitment in branchial mesoderm (Kelly et al., 2004). Moreover, Tbx1 mutant mice present with sporadic failure of development of muscles that normally originate from BAs (Jerome and Papaioannou, 2001; Dastjerdi et al., 2007; Grifone et al., 2008).
Cranial neural crest cells (CNCCs) are another important cell population which populate the BAs together with mesodermal cells (Trainor et al., 1994; Trainor and Tam, 1995; Chai et al., 2000). Via their interactions with mesodermal cells, CNCCs direct branchiomeric muscle development (Noden, 1983, 1986; Heude et al., 2010; Ziermann et al., 2018). Distal-less homeobox (DLX) proteins provide CNCCs with patterning information and intra-arch polarity along the dorsoventral/proximodistal axis (Heude et al., 2010; Minoux and Rijli, 2010). Dlx5 and Dlx6 are expressed in CNCCs of the mandibular process, and are necessary for CNCCs-mesoderm interactions during craniofacial myogenesis, since inactivation of Dlx5 and Dlx6 results in the loss of jaw muscles and compromised tongue development (Heude et al., 2010).
Previous studies have shown that RA signaling is essential for ocular, jaw and branchial muscle development (Bothe et al., 2011; Bohnsack et al., 2012; Rhinn and Dolle, 2012; Kurosaka et al., 2017; Wang et al., 2019). Retinoic acid (RA) is derived from liposoluble vitamin A (retinol) and in vitro, low concentrations of RA enhance skeletal myogenesis in stem cells and myoblast cell lines by regulating muscle progenitor factors and/or MRF expression (Edwards and McBurney, 1983; Albagli-Curiel et al., 1993; Halevy and Lerman, 1993; Kennedy et al., 2009). During heart development, RA signaling is required for ventricular myocyte proliferation (Lavine and Ornitz, 2008; Nakajima, 2019). However, the function of RA signaling in vivo during craniofacial muscle development is poorly understood.
In this study, we showed that maternal RA-exposure resulted in malformation of branchiomeric muscles derived from BA1 and BA2. This phenotype was associated with elevated cell death of branchial mesodermal cells, from which branchiomeric muscles arise. Additionally, excessive RA signaling resulted in reduced expression of Pitx2 and Tbx1, which underpinned the myogenic specification and determination defects in BA1 and BA2. Moreover, excessive RA signaling repressed Dlx5 and Dlx6 expression in CNCCs in the proximal region of the BAs, which disturbed CNCCs-mesoderm interactions during branchiomeric muscle development. Taken together, our results have revealed novel molecular and cellular mechanisms linking elevated RA signaling and branchiomeric muscle malformation.
Results
Excessive RA Signaling Result in BA1- and BA2-Derived Muscle Malformations
The first and second branchial arch mesoderm cells contribute to the jaw muscles – the temporalis, pterygoid, masseter, mylohyoid and anterior digastric, and to the facial expression muscles – the auricularis, buccinator, posterior digastric, frontalis, orbicularis oculi, quadratus labii, zygomaticus and others, respectively (Figure 1M) (Lescroart et al., 2010). To examine the effect of exogenous RA signaling on branchiomeric muscle development, we performed in situ hybridization using Myogenin as a myogenic determination marker (Hasty et al., 1993) in heads of E13.5 RA-treated embryos (Figures 1A–L). Muscles derived from BA1 displayed specific and consistent abnormalities in association with elevated RA signaling. The temporalis and masseter muscles failed to form (Figures 1B,H,J,L), and the pterygoid, mylohyoid and anterior digastric muscles were present, but were reduced in size and thickness, and the buccinator muscles were fragmented, as evidenced by the expression of Myogenin (Figures 1D,H,J,L). In the case of muscles derived from BA2, the frontalis and orbicularis oculi muscles were missing, whereas the others were hypoplastic and disorganized compared to controls (Figures 1D,F). The phenotypes of individual muscles derived from BA1 and BA2 are summarized in Figure 1N. Both intrinsic and extrinsic tongue muscles which originate from the occipital somite (Noden and Francis-West, 2006), formed normally and did not show any noticeable structural abnormality in RA-treated heads at E13.5 (Figures 1G–L and Supplementary Figure 1). Together, these data showed that excessive RA signaling results in defects in the development of BA1- and BA2-derived cranial muscles. In order to understand the cellular mechanism underlying this phenotype, we further analyzed the myogenic developmental process in RA-treated embryos.
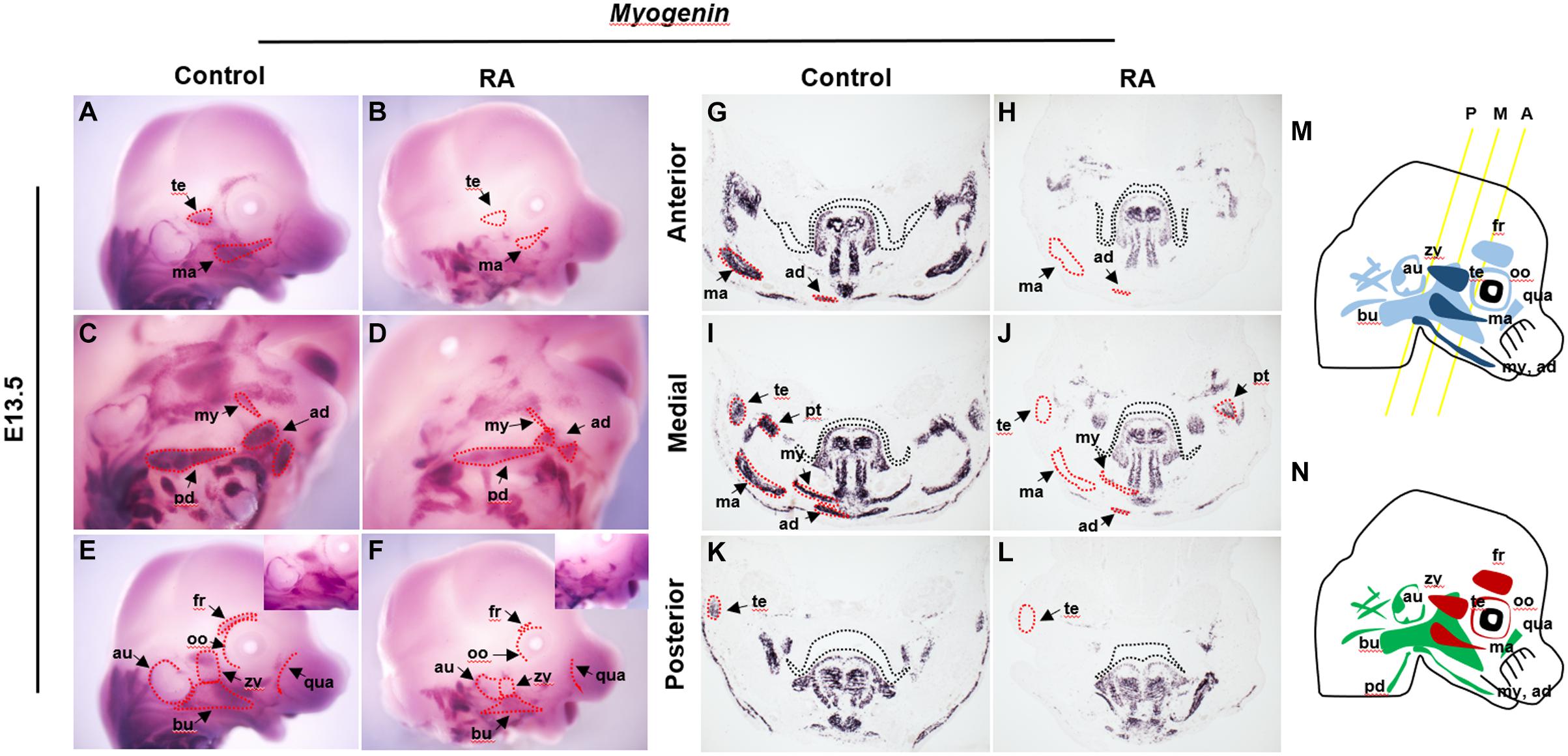
Figure 1. Abnormalities of BA1 and BA2 derived muscles in RA-treated embryos. Myogenin in situ hybridization for whole-mount (A–F) and frontal sections from anterior (G,H), medial (I,J), and posterior (K,L) regions (see M for approximate levels of sections along the anterior-posterior axis) of E13.5 control (A,C,E,G,I,K) and RA-treated (B,D,F,H,J,L) embryos. In (G–L) black dashed lines indicate the margin of palatal shelf and tongue primordium. In RA-treated embryos, the BA1-derived temporalis (te) and masseter (ma) muscles are absent (marked by red dashed lines in B,H,J,L); pterygoid (pt), mylohyoid (my) and anterior digastric (ad) muscles are reduced to small components (compare in D,H,J,L to C,G,I,K). (C–F) The RA-treated BA2-derived muscles are either absent (red dashed line) or replaced by a few muscle fibers. Higher magnifications are shown in upper right boxes of (E,F). (M,N) Schematics of BA1- and BA2-derived muscles. (M) Dark blue indicates muscles of BA1 origin; light blue indicates muscles of BA2 origin. Yellow lines illustrate the levels of frontal sections shown in (G–L). A, anterior; M, medial; P, posterior. (N) Red indicates absent in RA-treated embryos; green indicates reduced in RA-treated embryos. ad, anterior digastric muscle; au, auricularis muscle; bu, buccinators muscle; ma, masseter muscle; my, mylohyoid muscle; oo, orbicularis oculi muscle; pd, posterior digastric muscle; pt, pterygoid muscle; qua, quadratus labii muscle; te, temporalis muscle; zy, zygomaticus muscle.
Conversely, we also assessed the effect of reduced RA signaling on developing craniofacial muscle using conditional Rdh10 knock out embryos (CreErt2;Rdh10flox/flox), which exhibit a severe reduction of RA signaling in the craniofacial region (Kurosaka et al., 2017). Interestingly, most of the cranial muscles showed subtle differences between control and CreErt2;Rdh10flox/flox embryos (Supplementary Figure 2).
Elevated RA Signaling Affects Myogenic Gene Expression During Myogenesis in BA1 and BA2
We examined the expression patterns of Pitx2 and Tbx1, which mark muscle precursor cells in BA1 and BA2. In the control embryos, Pitx2 was expressed at E10.5 in the mesodermal core of BA1 and BA2, while in the RA-treated embryos Pitx2 expression was substantially reduced (Figures 2A,B). In contrast, increased Pitx2 expression could be observed in the epithelium of BA1 (Figures 2A,B). Tbx1 expression was also reduced in BA1 and BA2 of E10.5 RA-treated embryos compared to controls (Figures 2C,D). These results indicated that excessive RA signaling interferes with the expression of Pitx2 and Tbx1, which are essential for differentiation of BA1 and BA2 myogenic mesoderm.
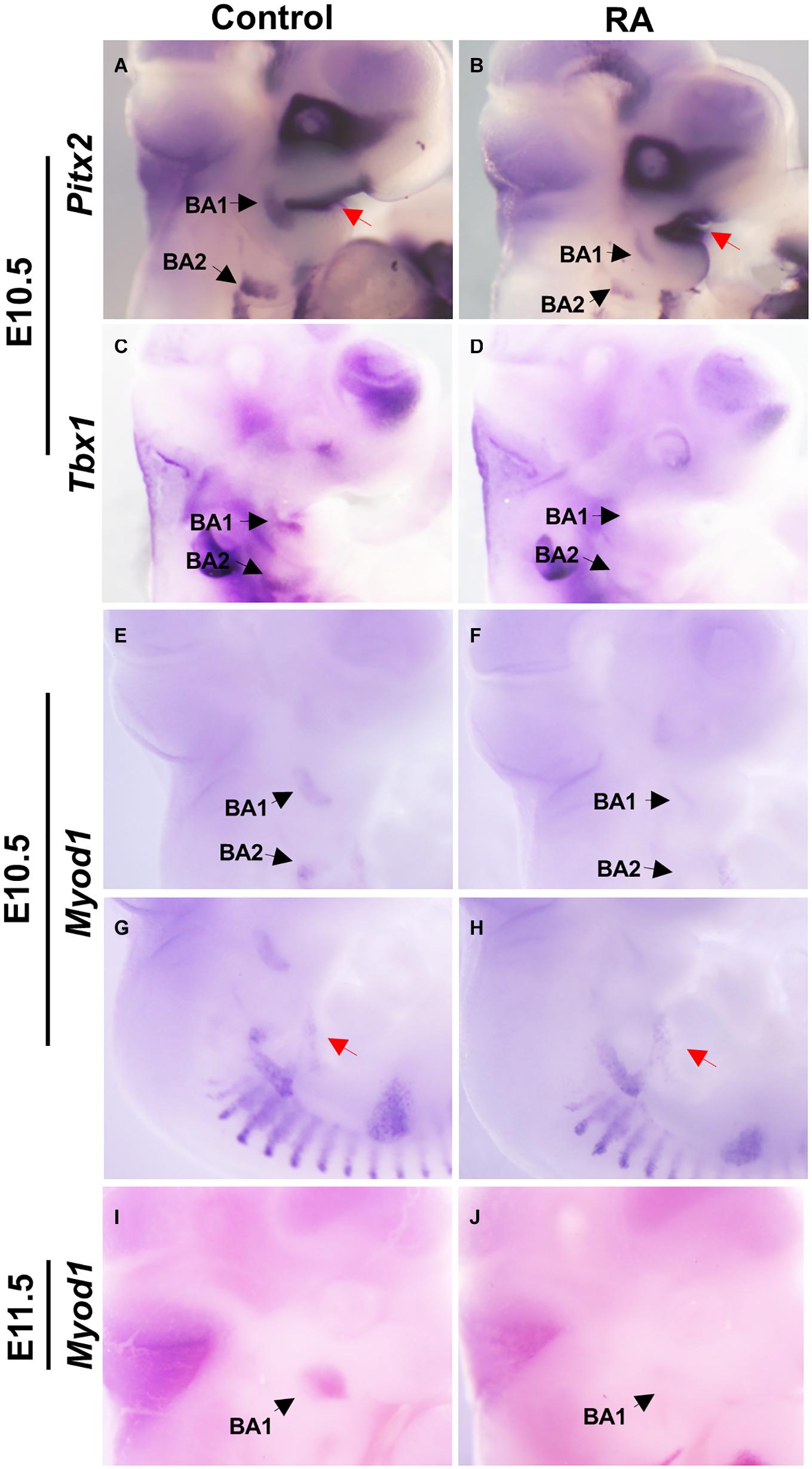
Figure 2. Disruption of early myogenic markers in BA1 and BA2 of RA-treated embryos. Lateral views of whole-mount in situ hybridization for Pitx2 (A,B), Tbx1 (C,D), and MyoD1 (E–J) in E10.5-E11.5 BA1 and BA2 of control (A,C,E,G,I) and RA-treated (B,D,F,H,J) embryos. In BA1 and BA2 at E10.5, the expression of the early markers of myogenic specification, Pitx2 (A,B) and Tbx1 (C,D), is severely affected by the RA treatment. However, the expression of Pitx2 is higher in the oral ectoderm in RA-treated embryos (A,B, red arrows). (E–J) MyoD1, a marker of myogenic determination, fails to be expressed in the BA1 and BA2 in embryos treated with RA at E10.5 and E11.5, but no significant change is observed in the hypoglossal cord (E,F, red arrows). Black arrows denote BA1 and BA2 hybridization signal or absence of signal.
The myogenic determination factor MyoD1 is under the control of both Pitx2 and Tbx1 (Braun and Gautel, 2011). In E10.5 control embryos, MyoD1 was expressed in BA1 and BA2 myoblasts, which later develop into the masticatory and facial premuscle masses at E11.5. In RA-treated embryos, MyoD1 expression was a substantially reduced in BA1 and BA2, which is indicative of a defect in myogenic determination (and later differentiation). Furthermore, this is likely an effect of perturbed specification due to reduced Pitx2 and Tbx1 expression in BA1 and BA2 (Figures 2E,F,I,J). In contrast, MyoD1 expression in the hypoglossal cord, from which the tongue myoblasts migrate (Huang et al., 1999), remained unchanged in the RA-treated group (Figures 2G,H).
Collectively, these findings indicate that excessive RA perturbs BA1 and BA2 muscle precursor specification and determination. We also analyzed the expression of Aldh1a2 and Aldh1a3, which encode indispensable enzymes for synthesizing RA, in RA-treated and RA loss-of-function, CreErt2;Rdh10flox/flox embryos. We detected subtle differences in the expression of these genes in the developing craniofacial region, between control and RA-treated embryos. This was also true for the expression of Aldh1a2 in Rdh10 mutant embryos, whereas in contrast Aldh1a3 expression was substantially reduced in the head (Supplementary Figure 3).
Increased Apoptosis of the Muscle Progenitor Cells in BA1 and BA2 Contributes to RA-Induced Branchiomeric Muscle Defects
Pitx2 and Tbx1 have previously been implicated in proliferation and survival of muscle progenitor cells in the branchiomeric muscles (Kelly et al., 2004; Dong et al., 2006; Shih et al., 2007) and we observed altered Pitx2 and Tbx1 expression in E10.5 RA-treated embryos. We further investigated the behavior of BA1 and BA2 muscle progenitor cells via staining for Islet1 (Nathan et al., 2008) in conjunction with phosphorylated Histone H3 (pHH3) and TUNEL to assess cell proliferation and cell death, respectively. Cell proliferation was unchanged in the muscle progenitor cells of both BA1 and BA2 in E10.5 RA-treated embryos compared to controls (Figures 3A,B and Table 1). In contrast, increased cell death was detected in the muscle progenitor cells of both BA1 and BA2 in E10.5 RA-treated embryos compared to controls (Figures 3C,D and Table 1). These results demonstrate that excessive RA signaling results in cell death in muscle progenitor cells in both BA1 and BA2.
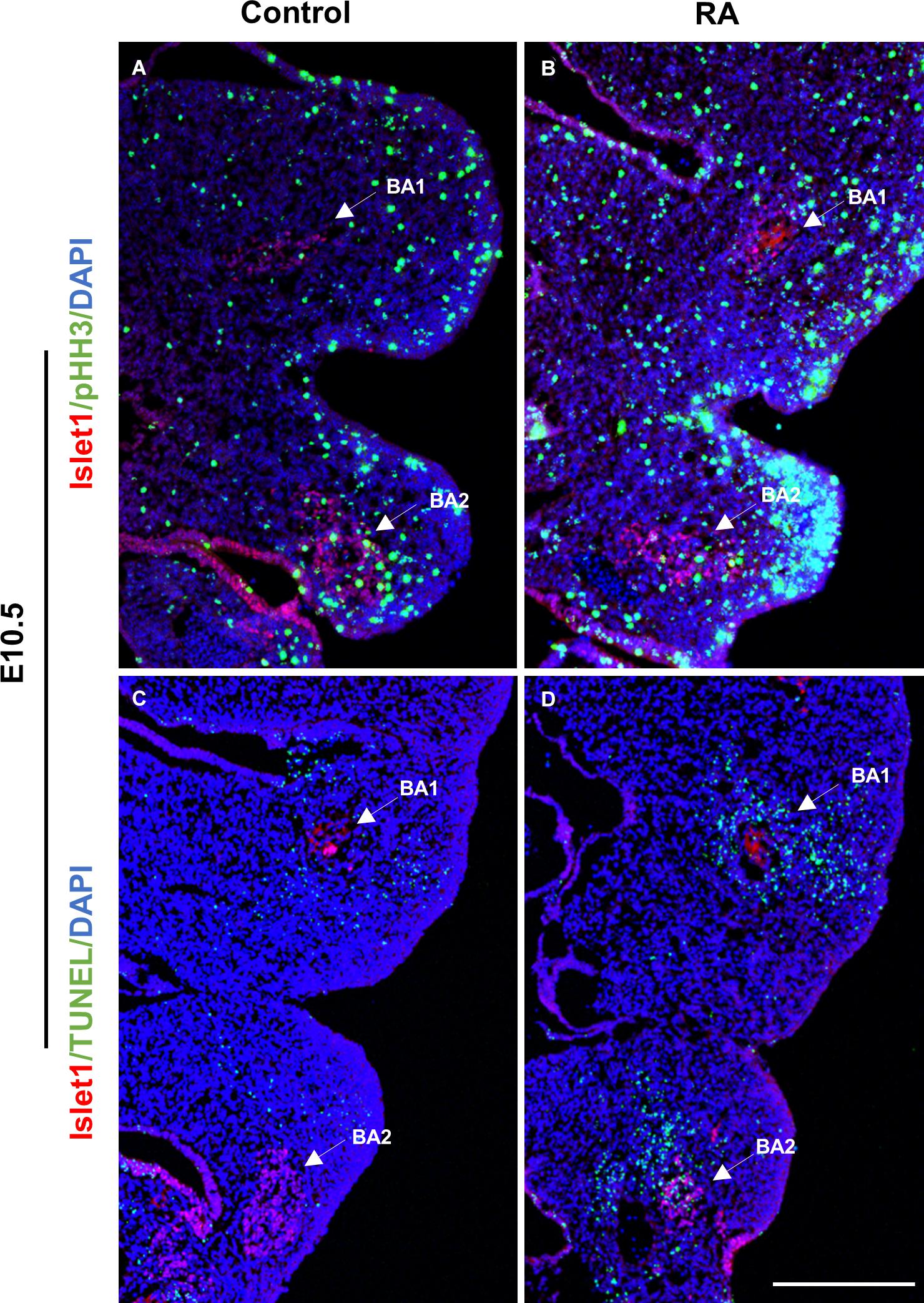
Figure 3. Unaltered proliferation but increased apoptosis in the muscle progenitor cells of the BA1 and BA2 at E10.5 after RA treatment. Immunofluorescent detection of pHH3 (green; A,B) and TUNEL (green; C,D) in transverse sections of control (A,C) and RA-treated (B,D) E10.5 embryos. Arrows indicate Islet1-positive (red) muscle progenitor cells in the BA1 and BA2 regions. Scale bar: 250 μm.

Table 1. Cell proliferation and cell death in ISLET1 positive cells in the developing branchial arches (BA).
Abnormalities of BA1-Derived Jaws Are Associated With Dlx5 and Dlx6 Expression Defects in RA-Treated Embryos
CNCC-mesoderm interactions are crucial for proper branchiomeric myogenesis (Grenier et al., 2009) and we have previously shown that excessive RA signaling at E8.5 affects CNCC development (Wang et al., 2019). Therefore, we hypothesized that a defect in CNCC development could underly the etiology of RA-induced BA1- and BA2- derived muscle malformation. To test this idea, we assessed the activity of Dlx5 and Dlx6, which are expressed by CNCCs and are required for the myogenic differentiation and patterning of craniofacial muscles (Heude et al., 2010). Dlx5 and Dlx6 expression was down-regulated in the proximal but not regions of distal branchial arches in E10.5 RA-treated embryos. The reduction was more pronounced in BA1 than BA2 (Figures 4A–D). Therefore, the BA1-derived muscle anomalies observed in the RA-treated embryos likely manifest as a result of perturbed Pitx2 and Tbx1 expression in the progenitor branchial arch mesoderm, together with loss of Dlx5 and Dlx6 expression by CNCCs in the proximal regions BA1, which impacts proximal-distal patterning. We also detected bilateral fusion of the upper and lower jaw in RA-treated embryos (Figures 4E–H). To evaluate the phenotype in detail, we dissected the mandibular structures from skeletal preparations of E18.5 embryos. In the control embryos, the main features – the coronoid process, the condylar process, the angular process and the molar alveolus – could be recognized (Figure 4G, box). In contrast to the controls, the position around the coronoid process and dentary bone in the mandible of RA-treated embryos was fused to the posterior/lateral position of maxilla (Figure 4H, box). Additionally, the condylar process was not detectable and the angular process was reduced in size (Figure 4H, box). The altered expression of Dlx5 and Dlx6 in the BAs could also have contributed to causing these defects (Vieux-Rochas et al., 2007; Vieux-Rochas et al., 2010).
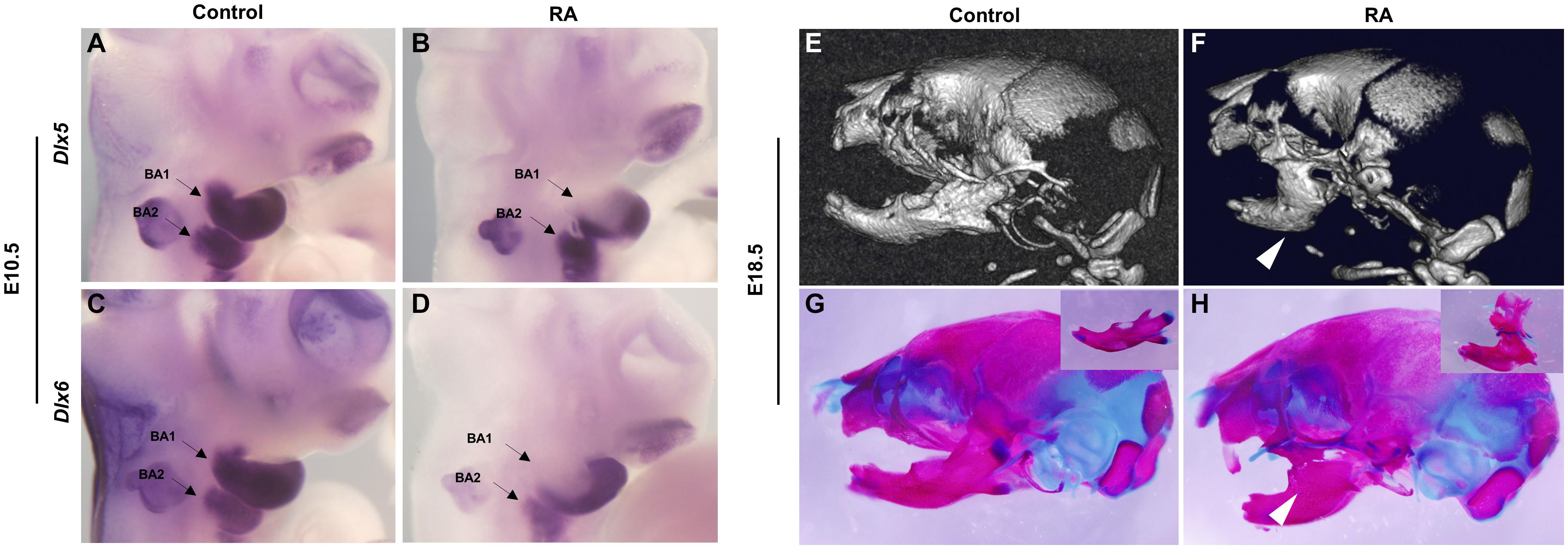
Figure 4. Abnormalities of BA1-derived jaws and associated Dlx5 and Dlx6 expression defects in RA-treated embryos. Lateral views of whole-mount in situ hybridization for Dlx5 (A,B) and Dlx6 (C,D) in E10.5 BA1 and BA2 of control (A,C) and RA-treated (B,D) embryos. (B,D) The expression of Dlx5 and Dlx6 in BA1 is lost in proximal but not in distal regions of RA-treated embryos at E10.5. In BA2, the expression of Dlx5 and Dlx6 is not affected in E10.5 RA-treated embryos. Arrows denote BA1 and BA2 hybridization signal or absence of signal. Micro-CT scans (E,F) and Alizarin red (bone) and Alcian blue (cartilage) stained skeletal preparations (G,H) of E18.5 control (E,G) and RA- treated (F,H) embryos. The white arrowheads in (F,H) mark the syngnathia in RA- treated embryos. Higher magnifications of dissected jaws are shown in the boxes of (G,H).
The Expression Pattern of Retinoic Acid Receptors in Developing Craniofacial Region With Elevated or Reduced RA Signaling
RA signaling is mediated by a nuclear receptor superfamily consisting of multiple RARs (RARα, RARβ, and RARγ) and their heterodimeric binding partner RXRs (RXRα, RXRβ, and RXRγ). In order to further analyze the effects of enhanced and reduced RA signaling during craniofacial muscle development, the expression of RARs and RXRs was analyzed in both RA-treated and CreErt2;Rdh10flox/flox embryos whose dams were administered tamoxifen at E7.0. Overall, we detected subtle differences in the expression levels and patterns of both RARs and RXRs between the control group and RA-treated embryos at E11.0 except that Rxra showed reduced expression in the developing frontonasal process (Supplementary Figure 4). In contrast, the expression of RARa, RARb, RXRa, RXRb, and RXRg was substantially reduced in the developing craniofacial region of E11.5 CreErt2;Rdh10flox/flox embryos compared to controls(Supplementary Figure 4).
Discussion
The first and second branchiomeric (branchial arch) muscles form part of the craniofacial musculature. Abnormal development of these muscles during embryogenesis results in congenital defects in the muscles of mastication and facial expression. Surgical reconstruction of malformed branchiomeric muscles is complex, often requires multiple procedures, and is not routinely available in many healthcare systems. Therefore, understanding the detailed molecular and cellular mechanisms regulating branchiomeric muscle development is critical to our understanding of the etiology of congenital muscle defects and to develop possible therapies. Previous reports have shown that RA signaling is essential for ocular and BA development in the craniofacial region (Sandell et al., 2007; Bohnsack et al., 2012; Rhinn and Dolle, 2012). In this study, we demonstrated that excessive embryonic RA signaling causes branchiomeric muscle malformations due to defects in myogenic specification in BA1 and BA2. This phenotype was associated with elevated apoptosis of muscle progenitor cells in BA1 and BA2. Furthermore, RA-induced proximal loss of Dlx5 and Dlx6 expression disturbs critical cellular interactions between CNCCs and muscle precursor cells during branchiomeric muscle development (Heude et al., 2010).
Diverse Reactions of Muscle Precursor Cells to RA Signaling
Numerous studies have attempted to elucidate the effects of stage- and/or dose- dependent effects of RA signaling on the development of various different muscles. It has been reported that RA signaling exhibits different effects on the differentiation of embryonic stem cells into cardiomyocytes depending on the timing of RA supplementation (Edwards and McBurney, 1983; Wobus et al., 1997; Nakajima, 2019). Compared with the levels of RA that enhance cardiomyogenesis, lower levels of RA signaling are known to enhance skeletal myogenesis (Edwards and McBurney, 1983; Halevy and Lerman, 1993). Previous studies have shown that both facial and tongue muscles are severely affected after RA-treatment (200 mg/kg) at E8.0 in the mouse fetus (Padmanabhan and Ahmed, 1997). We examined the effects of dose and timing of RA administration in our previous work on the effect of exaggerated RA signaling midgestational development. As a result, we found that daily administration of 25 mg/kg RA from E8.5 to E10.5 had the effect of disrupting multiple craniofacial structures (Wang et al., 2019). Also, in the present study, we detected BA1- and BA2-derived muscle malformations but minimal effects of RA-treatment on tongue muscle development. Our results indicate that proper RA signaling is essential for survival of muscle precursor cells in the branchial arch mesoderm. Branchiomeric muscle development is mediated by signaling and genetic pathways that are distinct from those of extraocular, tongue and laryngeal muscles in the head (Braun and Gautel, 2011). Interestingly, our study showed that in contrast to the induction of branchiomeric muscle defects, excess RA signaling did not cause defects in MyoD1 expression in the hypoglossal cord before E13.5. Altogether, these results indicate that muscle precursor cells react to RA signaling in different ways depending on the tissue type, developmental timing and dosage of RA. Interestingly, reduced RA signaling did not show as strong a disruption of BA1- and BA2-derived muscle development as elevated RA signaling. These results indicate that craniofacial muscle progenitor cells respond differently to elevated and reduced RA signaling.
Pitx2 mouse mutants exhibit a failure of BA1-derived muscle development in association with altered MyoD expression and elevated apoptosis of undifferentiated muscle progenitor cells (Dong et al., 2006). RA signaling has also been implicated in directly regulating Pitx2 in embryonic eye development (Kumar and Duester, 2010) and it has been also shown that RA signaling and Pitx2 regulate CNCCs during zebrafish development (Chawla et al., 2016). Exogenous RA (bead implantation) in chick embryos impacts the expression of the myogenic markers Pitx2 and Tbx1 (Bothe et al., 2011). Furthermore, Retinoid X receptor (RXR) co-localizes with PITX2 in extraocular muscle cells (Hebert et al., 2017). Together with our results, this clearly shows that perturbed RA signaling results in downregulation of Pitx2 in BA1 and BA2, which in turn results in branchiomeric muscle defects. However, although muscles derived from BA1 are absent in Pitx2 mutants, the muscles derived from BA2 are merely distorted (Shih et al., 2007). To explain the severe defects in muscles derived from BA2 in our study in association with elevated RA signaling, we examined the premyoblast specification marker, Tbx1. Tbx1 mutants exhibit severe perturbation or absence of both BA1 and BA2 derived muscles (Kelly et al., 2004). Furthermore, Pitx2 and Tbx1 are known to be molecular partners that regulate parallel pathways of common target genes in craniofacial muscle development (Dong et al., 2006; Bothe et al., 2011; Braun and Gautel, 2011). Additionally, interactions between Tbx1 and RA signaling have been demonstrated in a mouse model of DiGeorge syndrome (Ryckebusch et al., 2010). Consistent with these findings, we observed a substantial reduction of Tbx1 expression in the mesodermal core of BA1 and BA2 in RA-treated embryos. Taken together, our results further support the conclusion that elevated RA signaling results in defects in the specification of undifferentiated muscle progenitor cells in BA1 and BA2, and thus contributes to the etiology of branchiomeric muscle malformation (Figure 5).
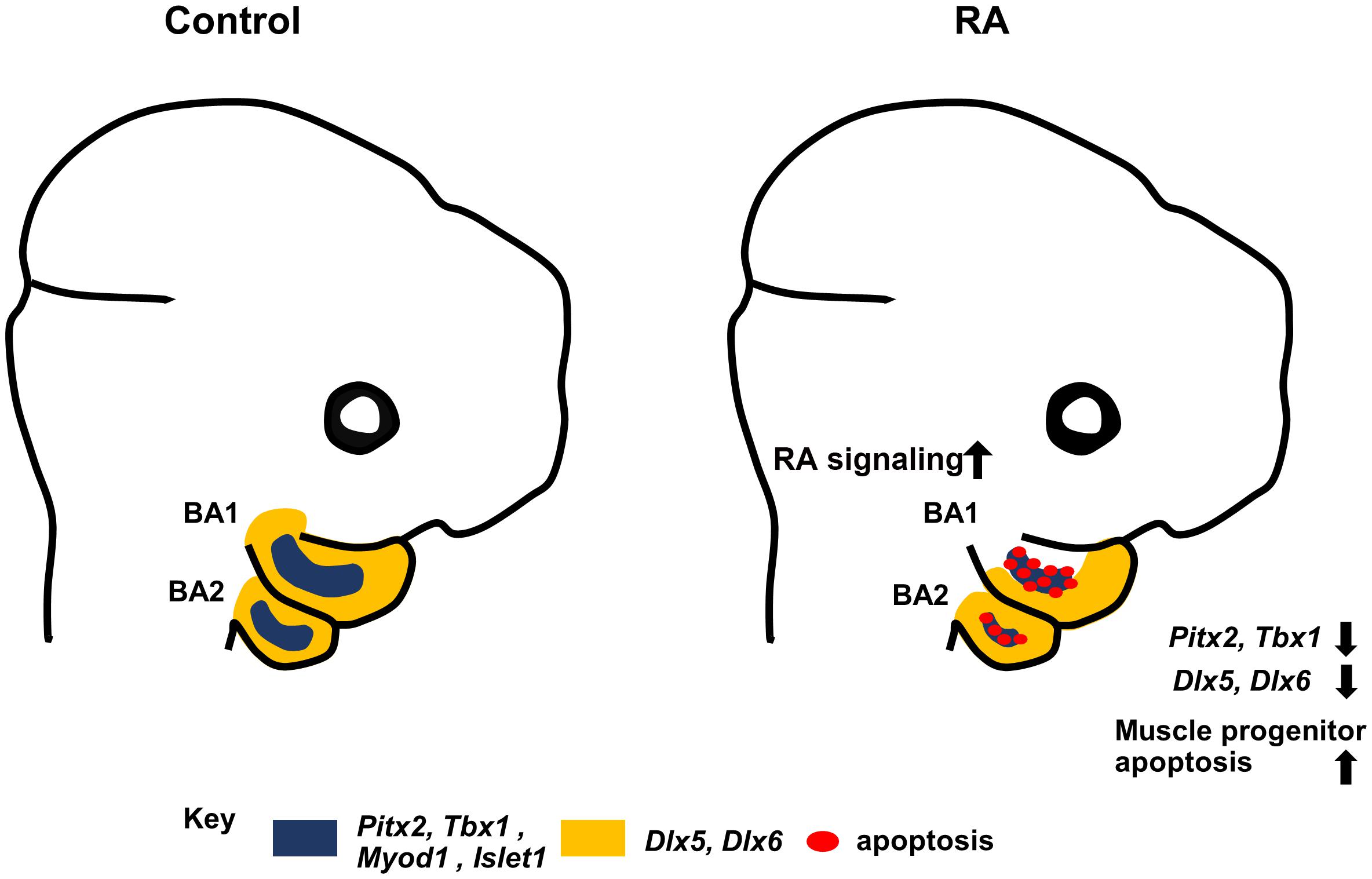
Figure 5. Summary diagram. Excess RA signaling results in disrupted expression of Pitx2, Tbx1, MyoD1, and Islet1 (blue) in myogenic progenitors, and Dlx5/6 (yellow) in CNCCs, together with elevated muscle progenitor apoptosis, which contributes to branchiomeric muscle defects in BA1 and BA2.
RA-Induced Proximal Loss of Dlx5/6 Impacts Branchiomeric Muscle Development
Previous in vitro and in vivo studies showed that proper RA signaling is essential for normal NCC development (Lee et al., 1995; Niederreither et al., 2003; Wang et al., 2019). Furthermore, CNCCs surround mesoderm cells in BAs that give rise to myogenic progenitors (Trainor et al., 1994; Trainor and Tam, 1995), and these cellular interactions are crucial for branchiomeric muscle formation (Noden, 1983, 1986; Chai et al., 2000; Sambasivan et al., 2011). For instance, Dlx5-positive CNCCs have been reported to guide the migration of muscle progenitors derived from BAs (Sugii et al., 2017). Additionally, CNCCs induce cranial myogenic formation by secreting inhibitory factors of the BMP and WNT signaling pathways in the BAs of chick embryos (Tzahor et al., 2003). In the present study, Dlx5/6 expression was decreased in the proximal region of BA1 and BA2. The loss of Dlx5/6 is known to impact muscle patterning and differentiation during the later stages of branchiomeric muscle development, however, Dlx5/6 do not affect the expression of the myogenic specification markers (Heude et al., 2010). Taken together, these facts suggest that several mechanisms contribute to the branchiomeric muscle defects observed in the present study. Firstly, early branchiomeric muscle formation is inhibited by defects in CNCC development (Heude et al., 2010) and subsequent differentiation and patterning are affected by altered Dlx5/6 expression in BAs as a result of disturbed RA signaling. Failure of Dlx5/6 expression leads to intrinsic tongue and sublingual muscle defects and masticatory muscle defects (Heude et al., 2010). Interestingly, in our study, reduced proximal Dlx5/6 expression led to masticatory muscle progenitor cell defects, but intrinsic tongue and mylohyoid muscles, which derive from a different mesodermal source, were present. We speculate that elevated RA has a small effect on mesodermal precursors of those muscles at the stages and under the conditions we have investigated. Additionally, since muscle attachments to bone are critical for shaping bone during development, it is possible that absence of the muscles of mastication is one reason for retarded condylar and angular processes development in the mandibles RA-treated embryos.
Expression of Retinoic Acid Receptors in Developing Head With Disturbed RA Signaling
Retinoic acid receptors mediate RA signaling and thus play important roles in transducing RA signaling during embryonic development (Rhinn and Dolle, 2012). In the present study, we detected subtle differences in the expression of most RARs and RXRs between E11.0 control and RA-treated embryos, with the exception of RXRa, which showed reduced expression in the RA-treated group. These results indicated that daily administration of 25 mg/kg RA from E8.5 to E10.5 does not critically influence the expression of either RARs or RXRs during craniofacial development. In contrast, we detected a substantial diminishment in retinoic acid receptor expression in embryos in which RA signaling was reduced. There is a large degree of functional redundancy between retinoic acid receptors (Mark et al., 2009), hence, further investigations including genetic complementation experiments will be required to reveal the effects of altered expression of retinoic acid receptors on craniofacial muscle development.
Materials and Methods
Animals and RA Administration
Pregnant female Institute of Cancer Research (ICR) mice (CLEA, Japan) were administered all-trans RA (25 mg/kg body weight) (Sigma-Aldrich) by oral gavage. All of the mice were housed with a 12 h dark-light cycle in which the light phase started from 8 a.m. RA (25 mg/ml in dimethylsulfoxide) was diluted 1/10 in corn oil just before use. Control animals were given the equivalent volume of the carrier. Oral gavage was performed once per day at gestation stages (from E8.5 to E10.5). Embryonic stage E0.5 was defined on the morning of vaginal plug confirmation. The approximate somite number in ICR mice at E8.5 was seven pairs.
Rdh10flox/flox and Cre-ERT2 mice were maintained and used as previously described (Sandell et al., 2007, 2012; Kurosaka et al., 2017). In order to eliminate RDH10 from developing embryos, Rdh10flox/flox female mice were crossed with Cre-ERT2:Rdh10flox/flox male mice followed by administration of tamoxifen at E7.0 as previously reported (Kurosaka et al., 2017). Cre-ERT2 mice carry the gene for Cre recombinase fused to the estrogen receptor T2 cassette inserted into the Rosa 26 locus (Ventura et al., 2007). Consequently, recombination takes place in a ubiquitous manner after administration of tamoxifen (Hayashi and McMahon, 2002). Rdh10flox/flox embryos were used as control samples throughout the study.
All animal experiments were performed in accordance with the guidelines of the Animal Care and Use Committee of the Osaka University Graduate School of Dentistry, Osaka, Japan. The committee on the ethics of animal experiments of the same university approved the study protocol (permit number: 26-028-0, 26-020-0).
Micro-CT
We used the R_ mCT2 system (Rigaku) with scanning parameters 50 kV, 200 μA to perform micro-CT. Scans were reconstructed and analyzed using 3D viewer and Volume Rendering Control software (Rigaku), according to standardized protocols.
Bone and Cartilage Staining
E18.5 embryos were skinned and eviscerated. The embryos were fixed in 100% ethanol overnight and then stained for 24 h with Alcian Blue (150 μg/ml in 20 ml of glacial acetic acid and 80 ml of 95% ethanol). After washing in 100% ethanol, soft tissues were dissolved in 2% KOH overnight and stained with Alizarin Red (50 μg/ml in 1% KOH) overnight. Stained embryos were kept in 20% glycerol/1% KOH until skeletons became clearly visible. Embryos were stored in 50% glycerol/50% water.
In situ Hybridization
Whole-mount and sectional in situ hybridization was performed as described with minor modifications using digoxigenin (DIG)-UTP (Roche)-labeled antisense RNA probes corresponding to the sequences of Myogenin, Pitx2, Tbx1, Myod1, Dlx5/6, Aldh1a2, Aldh1a3, Rara, Rarb, Rarg, Rxra, Rxrb, and Rxrg. Sequences used were previously reported in the Allen Brain Atlas1. For all in situ hybridization analyses, a minimum of three embryos of each sample were examined per probe.
Analysis of Apoptosis and Cell Proliferation
Analyses of apoptotic cells were performed using an in situ cell death detection kit (Roche) following the manufacturer’s instructions. For analyses of proliferation, samples were incubated with a mouse anti-pHH3 antibody (1:200, Millipore) at 4°C overnight followed by secondary Alexa-Fluor-488 donkey anti-mouse IgG (1:200, Invitrogen) for 6 h at room temperature for sections and overnight at 4°C for whole embryos. To label muscle progenitor cells in BA1 and BA2, sections were counterstained with a goat anti-Islet1 antibody (5 μg/ml, Abcam) at 4°C overnight, followed by secondary antibody (Alexa-Fluor-546 donkey anti-goat IgG, 1:200, Molecular Probes). Cells in at least five adjacent sections were counted in each assay. Statistical significance was assessed using Fisher’s exact test.
Data Availability Statement
The raw data supporting the conclusions of this article will be made available by the authors, without undue reservation.
Ethics Statement
The animal study was reviewed and approved by Animal Care and Use Committee of the Osaka University Graduate School of Dentistry.
Author Contributions
QW and HK: conceptualization. QW, LX, MS, YU, LS, PT, HK, JM, and TY: methodology, resources, and writing-review and editing. QW, HK, and TY: validation and writing original draft. QW, HK and JM: formal analysis and investigation. HK, YU, and TY: funding acquisition. All authors contributed to the article and approved the submitted version.
Funding
This research was funded by JSPS KAKENHI Grants 15H05687, 16K15836, 19H03858 to HK, 20K18754 to YU, 17K19754, 15H02577 to TY, the Nakatomi Foundation research grant and a Takeda Science Foundation research grant, project 82001028 supported by NSFC. This project was technically supported by the Research Center for Ultra-High-Voltage Electron Microscopy of Osaka University.
Conflict of Interest
The authors declare that the research was conducted in the absence of any commercial or financial relationships that could be construed as a potential conflict of interest.
Acknowledgments
We thank the members of the Department of Orthodontics and Dentofacial Orthopedics, Graduate School of Dentistry, Osaka University, for their insights and constructive comments throughout the course of this project and completion of this manuscript.
Supplementary Material
The Supplementary Material for this article can be found online at: https://www.frontiersin.org/articles/10.3389/fcell.2021.596838/full#supplementary-material
Supplementary Figure 1 | Transmission electron microscope (TEM) image of intrinsic tongue muscles. TEM images of control (A) and RA treated (B) intrinsic tongue muscle at E13.5. Scale bars; 500 nm. MF, muscle fiber.
Supplementary Figure 2 | In situ hybridization of Myogenin in E13.0 head. Myogenin in situ hybridization for whole-mount in E13.0 Control (left) and Cre- ERT2:Rdh10flox/flox (Right) head. au, auricularis muscle; bu, buccinators muscle; ma, masseter muscle; te, temporalis muscle; zy, zygomaticus muscle.
Supplementary Figure 3 | In situ hybridization of Aldh1a2 and Aldh1a3 in E10.5 branchial arches. Whole mount in situ hybridization of Aldh1a2 and Aldh1a3 in E10.5 RA-treated and Cre Ert2;Rdh10flox/flox embryos. The labels on the left indicate the genes whose expression was examined and the labels on the top indicate the condition of the sample treatments.
Supplementary Figure 4 | In situ hybridization of Retinoic acid receptors (Rars and Rxrs) in E10.5 branchial arches. Whole mount in situ hybridization of Rara, Rarb, Rarg, Rxra, Rxrb, and Rxrg in RA-treated and CreErt2;Rdh10flox/flox embryos. Labels on the left indicate the genes whose expression was examined and labels on the top indicate the condition of the sample treatments. Asterisks indicate the position where different expression profiles could be detected among comparable samples.
Footnotes
References
Albagli-Curiel, O., Carnac, G., Vandromme, M., Vincent, S., Crepieux, P., and Bonnieu, A. (1993). Serum-induced inhibition of myogenesis is differentially relieved by retinoic acid and triiodothyronine in C2 murine muscle cells. Differentiation 52, 201–210. doi: 10.1111/j.1432-0436.1993.tb00632.x
Bohnsack, B. L., Kasprick, D. S., Kish, P. E., Goldman, D., and Kahana, A. (2012). A zebrafish model of axenfeld-rieger syndrome reveals that pitx2 regulation by retinoic acid is essential for ocular and craniofacial development. Invest. Ophthalmol. Vis. Sci. 53, 7–22. doi: 10.1167/iovs.11-8494
Bothe, I., Tenin, G., Oseni, A., and Dietrich, S. (2011). Dynamic control of head mesoderm patterning. Development 138, 2807–2821.
Braun, T., and Gautel, M. (2011). Transcriptional mechanisms regulating skeletal muscle differentiation, growth and homeostasis. Nat. Rev. Mol. Cell Biol. 12, 349–361.
Chai, Y., Jiang, X., Ito, Y., Bringas, P. Jr., Han, J., Rowitch, D. H., et al. (2000). Fate of the mammalian cranial neural crest during tooth and mandibular morphogenesis. Development 127, 1671–1679.
Chawla, B., Schley, E., Williams, A. L., and Bohnsack, B. L. (2016). Retinoic acid and Pitx2 regulate early neural crest survival and migration in craniofacial and ocular development. Birth Defects Res. B Dev. Reprod. Toxicol. 107, 126–135. doi: 10.1002/bdrb.21177
Dastjerdi, A., Robson, L., Walker, R., Hadley, J., Zhang, Z., Rodriguez-Niedenfuhr, M., et al. (2007). Tbx1 regulation of myogenic differentiation in the limb and cranial mesoderm. Dev. Dyn. 236, 353–363. doi: 10.1002/dvdy.21010
Dong, F., Sun, X., Liu, W., Ai, D., Klysik, E., Lu, M. F., et al. (2006). Pitx2 promotes development of splanchnic mesoderm-derived branchiomeric muscle. Development 133, 4891–4899. doi: 10.1242/dev.02693
Edwards, M. K., and McBurney, M. W. (1983). The concentration of retinoic acid determines the differentiated cell types formed by a teratocarcinoma cell line. Dev. Biol. 98, 187–191.
Grenier, J., Teillet, M. A., Grifone, R., Kelly, R. G., and Duprez, D. (2009). Relationship between neural crest cells and cranial mesoderm during head muscle development. PLoS One 4:e4381. doi: 10.1371/journal.pone.0004381
Grifone, R., Jarry, T., Dandonneau, M., Grenier, J., Duprez, D., and Kelly, R. G. (2008). Properties of branchiomeric and somite-derived muscle development in Tbx1 mutant embryos. Dev. Dyn. 237, 3071–3078. doi: 10.1002/dvdy.21718
Grifone, R., and Kelly, R. G. (2007). Heartening news for head muscle development. Trends Genet. 23, 365–369. doi: 10.1016/j.tig.2007.05.002
Halevy, O., and Lerman, O. (1993). Retinoic acid induces adult muscle cell differentiation mediated by the retinoic acid receptor-alpha. J. Cell Physiol. 154, 566–572.
Hasty, P., Bradley, A., Morris, J. H., Edmondson, D. G., Venuti, J. M., Olson, E. N., et al. (1993). Muscle deficiency and neonatal death in mice with a targeted mutation in the myogenin gene. Nature 364, 501–506. doi: 10.1038/364501a0
Hayashi, S., and McMahon, A. P. (2002). Efficient recombination in diverse tissues by a tamoxifen-inducible form of Cre: a tool for temporally regulated gene activation/inactivation in the mouse. Dev. Biol. 244, 305–318. doi: 10.1006/dbio.2002.0597
Hebert, S. L., Fitzpatrick, K. R., McConnell, S. A., Cucak, A., Yuan, C., and McLoon, L. K. (2017). Effects of retinoic acid signaling on extraocular muscle myogenic precursor cells in vitro. Exp. Cell Res. 361, 101–111. doi: 10.1016/j.yexcr.2017.10.007
Heude, E., Bouhali, K., Kurihara, Y., Kurihara, H., Couly, G., Janvier, P., et al. (2010). Jaw muscularization requires Dlx expression by cranial neural crest cells. Proc. Natl. Acad. Sci. U.S.A. 107, 11441–11446. doi: 10.1073/pnas.1001582107
Huang, R., Zhi, Q., Izpisua-Belmonte, J. C., Christ, B., and Patel, K. (1999). Origin and development of the avian tongue muscles. Anat. Embryol. 200, 137–152.
Jerome, L. A., and Papaioannou, V. E. (2001). DiGeorge syndrome phenotype in mice mutant for the T-box gene, Tbx1. Nat. Genet. 27, 286–291. doi: 10.1038/85845
Kelly, R. G., Jerome-Majewska, L. A., and Papaioannou, V. E. (2004). The del22q11.2 candidate gene Tbx1 regulates branchiomeric myogenesis. Hum. Mol. Genet. 13, 2829–2840.
Kennedy, K. A., Porter, T., Mehta, V., Ryan, S. D., Price, F., Peshdary, V., et al. (2009). Retinoic acid enhances skeletal muscle progenitor formation and bypasses inhibition by bone morphogenetic protein 4 but not dominant negative beta-catenin. BMC Biol. 7:67. doi: 10.1186/1741-7007-7-67
Kumar, S., and Duester, G. (2010). Retinoic acid signaling in perioptic mesenchyme represses Wnt signaling via induction of Pitx2 and Dkk2. Dev. Biol. 340, 67–74. doi: 10.1016/j.ydbio.2010.01.027
Kurosaka, H., Wang, Q., Sandell, L., Yamashiro, T., and Trainor, P. A. (2017). Rdh10 loss-of-function and perturbed retinoid signaling underlies the etiology of choanal atresia. Hum. Mol. Genet. 26, 1268–1279. doi: 10.1093/hmg/ddx031
Lavine, K. J., and Ornitz, D. M. (2008). Fibroblast growth factors and Hedgehogs: at the heart of the epicardial signaling center. Trends Genet. 24, 33–40. doi: 10.1016/j.tig.2007.10.007
Lee, Y. M., Osumi-Yamashita, N., Ninomiya, Y., Moon, C. K., Eriksson, U., and Eto, K. (1995). Retinoic acid stage-dependently alters the migration pattern and identity of hindbrain neural crest cells. Development 121, 825–837.
Lescroart, F., Kelly, R. G., Le Garrec, J. F., Nicolas, J. F., Meilhac, S. M., and Buckingham, M. (2010). Clonal analysis reveals common lineage relationships between head muscles and second heart field derivatives in the mouse embryo. Development 137, 3269–3279. doi: 10.1242/dev.050674
Mark, M., Ghyselinck, N. B., and Chambon, P. (2009). Function of retinoic acid receptors during embryonic development. Nucl. Recept. Signal. 7:e002.
Minoux, M., and Rijli, F. M. (2010). Molecular mechanisms of cranial neural crest cell migration and patterning in craniofacial development. Development 137, 2605–2621.
Nathan, E., Monovich, A., Tirosh-Finkel, L., Harrelson, Z., Rousso, T., Rinon, A., et al. (2008). The contribution of Islet1-expressing splanchnic mesoderm cells to distinct branchiomeric muscles reveals significant heterogeneity in head muscle development. Development 135, 647–657. doi: 10.1242/dev.007989
Niederreither, K., Vermot, J., Le Roux, I., Schuhbaur, B., Chambon, P., and Dolle, P. (2003). The regional pattern of retinoic acid synthesis by RALDH2 is essential for the development of posterior pharyngeal arches and the enteric nervous system. Development 130, 2525–2534. doi: 10.1242/dev.00463
Noden, D. M. (1983). The embryonic origins of avian cephalic and cervical muscles and associated connective tissues. Am. J. Anat. 168, 257–276.
Noden, D. M., and Francis-West, P. (2006). The differentiation and morphogenesis of craniofacial muscles. Dev. Dyn. 235, 1194–1218.
Padmanabhan, R., and Ahmed, I. (1997). Retinoic acid-induced asymmetric craniofacial growth and cleft palate in the TO mouse fetus. Reprod. Toxicol. 11, 843–860. doi: 10.1016/s0890-6238(97)00068-3
Parada, C., Han, D., and Chai, Y. (2012). Molecular and cellular regulatory mechanisms of tongue myogenesis. J. Dent. Res. 91, 528–535.
Rhinn, M., and Dolle, P. (2012). Retinoic acid signalling during development. Development 139, 843–858.
Ryckebusch, L., Bertrand, N., Mesbah, K., Bajolle, F., Niederreither, K., Kelly, R. G., et al. (2010). Decreased levels of embryonic retinoic acid synthesis accelerate recovery from arterial growth delay in a mouse model of DiGeorge syndrome. Circ. Res. 106, 686–694. doi: 10.1161/CIRCRESAHA.109.205732
Sambasivan, R., Kuratani, S., and Tajbakhsh, S. (2011). An eye on the head: the development and evolution of craniofacial muscles. Development 138, 2401–2415.
Sandell, L. L., Lynn, M. L., Inman, K. E., McDowell, W., and Trainor, P. A. (2012). RDH10 oxidation of Vitamin A is a critical control step in synthesis of retinoic acid during mouse embryogenesis. PLoS One 7:e30698. doi: 10.1371/journal.pone.0030698
Sandell, L. L., Sanderson, B. W., Moiseyev, G., Johnson, T., Mushegian, A., Young, K., et al. (2007). RDH10 is essential for synthesis of embryonic retinoic acid and is required for limb, craniofacial, and organ development. Genes Dev. 21, 1113–1124. doi: 10.1101/gad.1533407
Shih, H. P., Gross, M. K., and Kioussi, C. (2007). Cranial muscle defects of Pitx2 mutants result from specification defects in the first branchial arch. Proc. Natl. Acad. Sci. U.S.A. 104, 5907–5912. doi: 10.1073/pnas.0701122104
Sugii, H., Grimaldi, A., Li, J., Parada, C., Vu-Ho, T., Feng, J., et al. (2017). The Dlx5-FGF10 signaling cascade controls cranial neural crest and myoblast interaction during oropharyngeal patterning and development. Development 144, 4037–4045. doi: 10.1242/dev.155176
Trainor, P. A., and Tam, P. P. (1995). Cranial paraxial mesoderm and neural crest cells of the mouse embryo: co-distribution in the craniofacial mesenchyme but distinct segregation in branchial arches. Development 121, 2569–2582.
Trainor, P. A., Tan, S. S., and Tam, P. P. (1994). Cranial paraxial mesoderm: regionalisation of cell fate and impact on craniofacial development in mouse embryos. Development 120, 2397–2408.
Tzahor, E., Kempf, H., Mootoosamy, R. C., Poon, A. C., Abzhanov, A., Tabin, C. J., et al. (2003). Antagonists of Wnt and BMP signaling promote the formation of vertebrate head muscle. Genes Dev. 17, 3087–3099. doi: 10.1101/gad.1154103
Ventura, A., Kirsch, D. G., McLaughlin, M. E., Tuveson, D. A., Grimm, J., Lintault, L., et al. (2007). Restoration of p53 function leads to tumour regression in vivo. Nature 445, 661–665.
Vieux-Rochas, M., Bouhali, K., Baudry, S., Fontaine, A., Coen, L., and Levi, G. (2010). Irreversible effects of retinoic acid pulse on Xenopus jaw morphogenesis: new insight into cranial neural crest specification. Birth. Defects Res. B Dev. Reprod. Toxicol. 89, 493–503. doi: 10.1002/bdrb.20269
Vieux-Rochas, M., Coen, L., Sato, T., Kurihara, Y., Gitton, Y., Barbieri, O., et al. (2007). Molecular dynamics of retinoic acid-induced craniofacial malformations: implications for the origin of gnathostome jaws. PLoS One 2:e510. doi: 10.1371/journal.pone.0000510
Wang, Q., Kurosaka, H., Kikuchi, M., Nakaya, A., Trainor, P. A., and Yamashiro, T. (2019). Perturbed development of cranial neural crest cells in association with reduced sonic hedgehog signaling underlies the pathogenesis of retinoic-acid-induced cleft palate. Dis. Model. Mech. 12:dmm040279. doi: 10.1242/dmm.040279
Wobus, A. M., Kaomei, G., Shan, J., Wellner, M. C., Rohwedel, J., Ji, G., et al. (1997). Retinoic acid accelerates embryonic stem cell-derived cardiac differentiation and enhances development of ventricular cardiomyocytes. J. Mol. Cell Cardiol. 29, 1525–1539. doi: 10.1006/jmcc.1997.0433
Keywords: cranial muscle development, retinoic acid signaling, craniofacial abnormalities, muscle progenitor cell, muscle differentiation
Citation: Wang Q, Xu L, Miura J, Saha MK, Uemura Y, Sandell LL, Trainor PA, Yamashiro T and Kurosaka H (2021) Branchiomeric Muscle Development Requires Proper Retinoic Acid Signaling. Front. Cell Dev. Biol. 9:596838. doi: 10.3389/fcell.2021.596838
Received: 20 August 2020; Accepted: 12 May 2021;
Published: 09 July 2021.
Edited by:
Marcela Buchtova, Czech Academy of Sciences, CzechiaReviewed by:
Linda McLoon, University of Minnesota, United StatesAntionette Williams, Ann & Robert H. Lurie Children’s Hospital of Chicago, United States
Copyright © 2021 Wang, Xu, Miura, Saha, Uemura, Sandell, Trainor, Yamashiro and Kurosaka. This is an open-access article distributed under the terms of the Creative Commons Attribution License (CC BY). The use, distribution or reproduction in other forums is permitted, provided the original author(s) and the copyright owner(s) are credited and that the original publication in this journal is cited, in accordance with accepted academic practice. No use, distribution or reproduction is permitted which does not comply with these terms.
*Correspondence: Hiroshi Kurosaka, a3Vyb3Nha2FAZGVudC5vc2FrYS11LmFjLmpw