- Department of Biological Sciences, Dartmouth College, Hanover, NH, United States
PLK1 is a conserved mitotic kinase that is essential for the entry into and progression through mitosis. In addition to its canonical mitotic functions, recent studies have characterized a critical role for PLK-1 in regulating the polarization and asymmetric division of the one-cell C. elegans embryo. Prior to cell division, PLK-1 regulates both the polarization of the PAR proteins at the cell cortex and the segregation of cell fate determinants in the cytoplasm. Following cell division, PLK-1 is preferentially inherited to one daughter cell where it acts to regulate the timing of centrosome separation and cell division. PLK1 also regulates cell polarity in asymmetrically dividing Drosophila neuroblasts and during mammalian planar cell polarity, suggesting it may act broadly to connect cell polarity and cell cycle mechanisms.
Introduction
Asymmetric cell division is a process in which a dividing cell gives rise to daughter cells with differing fate, size and/or function. In bacteria and yeast, asymmetric divisions are widespread and give rise to cells that differ in morphology, function or replicative capacity (Macara and Mili, 2008; Kysela et al., 2013). In metazoans, asymmetric divisions contribute to the diversification of cell types during embryonic development and are also required to maintain tissue homeostasis in adults, for example through the continued asymmetric division of stem cells (Knoblich, 2010). While diverse molecular mechanisms control asymmetric divisions in unicellular and multicellular organisms (Macara and Mili, 2008), coordination between cell polarization and cell cycle progression lies at the heart of each asymmetric division: factors are polarized prior to cell division such that they are partitioned unequally to the daughter cells upon cytokinesis (Li, 2013; Venkei and Yamashita, 2018). Therefore, understanding the interplay between cell polarization, cell cycle and cell division mechanisms is central to understanding how cells divide asymmetrically (Knoblich, 2010; Noatynska et al., 2010).
Polo-like kinase 1 (PLK1) is a conserved and essential mitotic kinase that regulates centrosome duplication and maturation, mitotic entry, bipolar spindle formation, chromosome segregation, and cytokinesis (Barr et al., 2004; Petronczki et al., 2008; Archambault and Glover, 2009; Pintard and Archambault, 2018). PLK1 activity is relatively low during interphase and increases at the G2/M transition before falling again after anaphase. Abnormally high PLK1 activity during G2 and M-phase is associated with tumorigenesis, and has therefore been extensively studied as a target for cancer therapeutics (Strebhardt and Ullrich, 2006). In addition to its canonical mitotic functions, important roles for PLK1 in asymmetric cell division have been characterized. In this review, we focus on the role of PLK-1 during the asymmetric division of the one-cell C. elegans embryo (zygote). We describe the mechanisms by which PLK-1 both regulates the polarization of the worm zygote and as well as the ways in which the asymmetric inheritance of PLK-1 contributes to differences between daughter cells at the two-cell stage.
Overview of PLK1 Structure and Regulation
polo was first identified in Drosophila (Sunkel and Glover, 1988) and its homologs include S. cerevisiae Cdc5, S. pombe Plo1, C. elegans PLK-1 and human PLK1 (Archambault and Glover, 2009). PLK1 contains an N-terminal Serine/Threonine kinase domain that is highly conserved among polo-like kinases and is similar to those in Aurora kinases and calcium/calmodulin-dependent kinases (Zitouni et al., 2014). PLK1 kinase activity is stimulated by phosphorylation of the activation loop in the PLK1 kinase domain by Aurora A kinase, which depends on the Aurora A cofactor Bora (Jang et al., 2002; Macurek et al., 2008; Seki et al., 2008; Tavernier et al., 2015b) (Figure 1). The C-terminus features a non-catalytic Polo box domain (PBD) that binds phosphopeptides generated by either PLK1 itself (self-primed) or by other kinases (non-self primed) (Zitouni et al., 2014) (Figure 1). The interaction between the PBD and various binding partners guides PLK1 localization to different cellular structures such as the centrosome, kinetochores, nuclear envelope and midbody (Lee et al., 1998; Elia et al., 2003). Additionally, the interaction between the PBD and its binding partners relieves the autoinhibition of PLK1 kinase activity by the intramolecular interaction between the PBD and the kinase domain, thereby coupling PLK1 localization with its activation toward specific substrates (Elia et al., 2003; Lowery et al., 2005; Zitouni et al., 2014) (Figure 1).
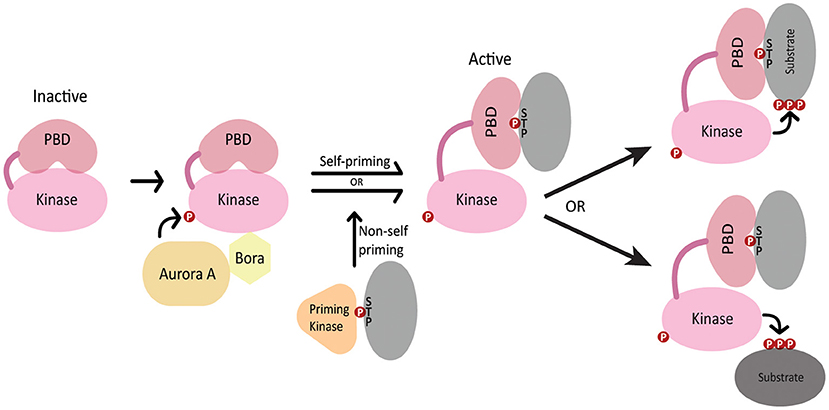
Figure 1. Regulation of PLK1 activation and localization. In the inactive state, the kinase domain activation loop is not phosphorylated and the PBD domain autoinhibits the kinase domain. Aurora A and SPAT/Bora stimulate kinase activity through phosphorylation of the PLK1 activation loop. The PBD domain binds phosphopeptides generated by PLK1 (self-primed) or by a priming kinase (non-self-primed), which relieves autoinhibition of the kinase domain. The PBD interacts either with PLK1 substrates or localizes PLK-1 in proximity to its substrates.
Introduction to the Asymmetric Division of the C. elegans Embryo
The one-cell C. elegans embryo undergoes an asymmetric division to give rise to an anterior daughter cell named AB and a posterior daughter cell named P1. AB and P1 differ in several respects: AB gives rise exclusively to somatic lineages whereas P1 gives rise to both somatic and germline lineages, AB is larger than P1, and AB divides roughly 2 min before P1 with a mitotic spindle oriented orthogonally to the P1 spindle (Rose and Gonczy, 2014; Griffin, 2015). All aspects of this asymmetric division depend on the polarization of the embryo by the conserved PAR polarity regulators.
The PAR proteins include the Anterior PAR proteins (aPARs) PAR-3, PAR-6 and PKC-3/aPKC and the Posterior PAR proteins (pPARs) PAR-1 and PAR-2. The aPARs and pPARs antagonize each other's cortical association such that any region of the cortex is typically occupied by either the aPARs or the pPARs, but not both (Lang and Munro, 2017). The PAR proteins are deposited in the oocyte and remain symmetrically distributed as the oocyte matures and as the newly fertilized embryo completes meiosis II. Upon the completion of meiosis, several symmetry breaking cues at the posterior pole combine to trigger the precisely timed, robust, and rapid polarization of the embryo (Goldstein and Hird, 1996). These cues include microtubules and Aurora A kinase activity that emanates from the sperm-donated centrosome at the posterior end (Tsai and Ahringer, 2007; Motegi et al., 2011; Klinkert et al., 2019; Zhao et al., 2019) and redox signaling from mitochondria near the posterior cortex (De Henau et al., 2020). These cues trigger the establishment of polarity, which takes roughly 10 min and results in the concentration of the aPARs at the anterior cortex and the pPARs at the posterior cortex. During polarity establishment, anteriorly-directed cortical actomyosin flows help to sweep the cortical aPARs out of the posterior domain, thereby allowing pPARs to load from the cytoplasm onto the posterior cortex (Cheeks et al., 2004; Munro et al., 2004; Goehring et al., 2011; Gubieda et al., 2020). These asymmetries are maintained for ~10 min until the embryo divides. From their polarized domains, the PAR proteins regulate the polarization of both cortical and cytoplasmic factors along the anterior/posterior (A/P) axis that result in the differences in size, fate, spindle orientation and cell division timing between AB and P1.
Consistent with its canonical role in the cell cycle, PLK-1 regulates meiotic and mitotic progression in the C. elegans zygote (Chase et al., 2000), including by promoting nuclear envelope breakdown (Rahman et al., 2015, 2020; Martino et al., 2017; Velez-Aguilera et al., 2020) and centrosome maturation (Woodruff et al., 2015). Here, we focus on PLK-1's contributions to the asymmetric division of the zygote as both a regulator at multiple stages of the zygote polarization and as a factor whose asymmetric inheritance contributes directly to differences between AB and P1 (Figures 2A,B). It is important to keep in mind that because complete depletion of PLK-1 activity results in sterility, the early embryonic functions of PLK-1 described below reflect the phenotypes of embryos partially depleted of PLK-1 activity.
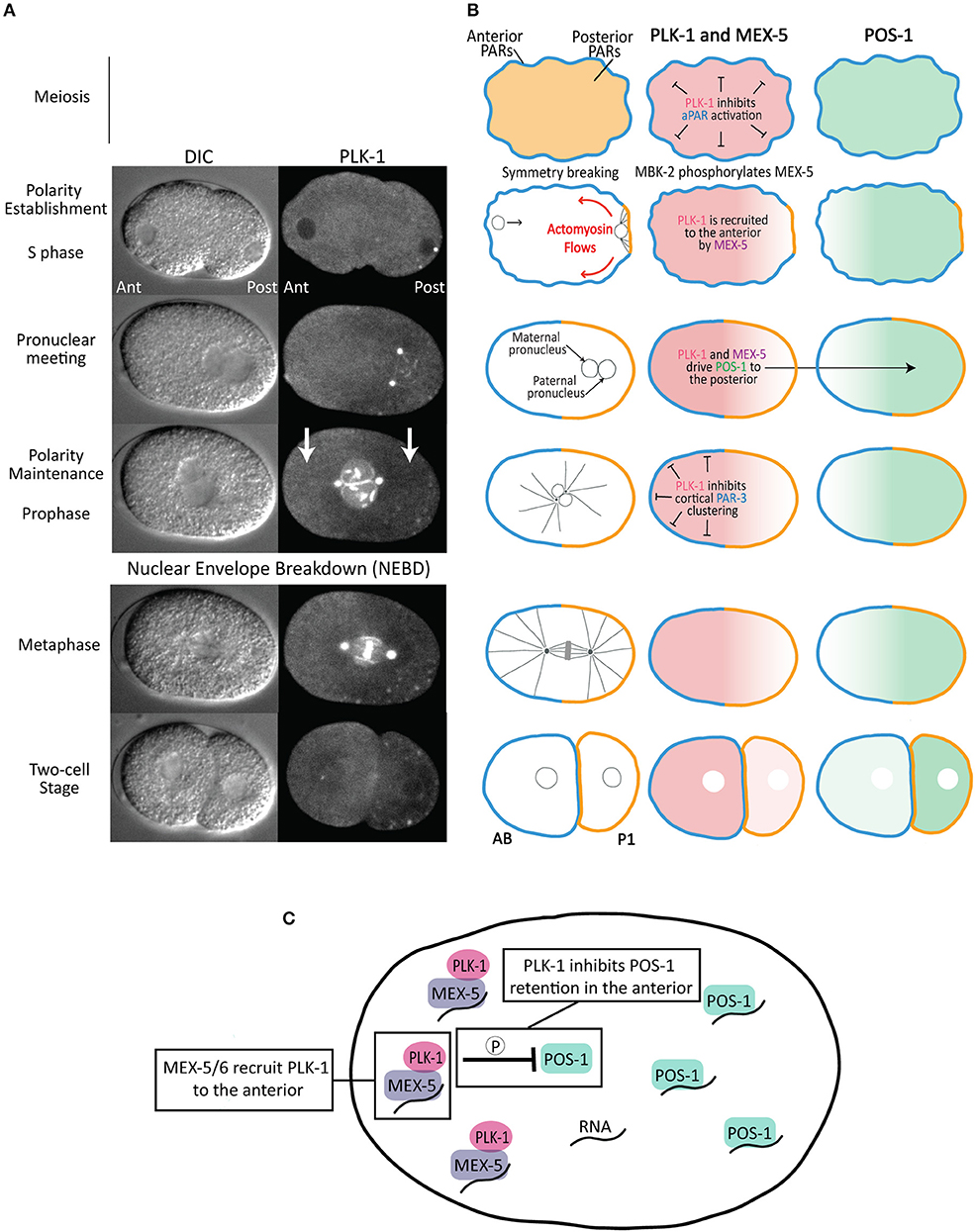
Figure 2. Asymmetric division of the C. elegans zygote. (A) DIC and fluorescence images of a one-cell embryo expressing PLK-1::sGFP (Martino et al., 2017) from polarity establishment through its asymmetric division. Note that, in addition to its localization to the nuclear envelope, centrosomes and chromosomes, there is a cytoplasmic pool that is enriched in the anterior cytoplasm relative to the posterior cytoplasm (indicated by white arrows). (B) Schematics of aPAR (blue), pPAR (yellow), PLK-1, MEX-5 and POS-1 localization during the asymmetric division of the zygote. The stages are as indicated in (A). The left panels indicate the position of the maternal and paternal pro-nuclei (black circles), microtubules (black lines) and chromosomes (gray) in the mitotic spindle. (C) Model for the mechanism by which MEX-5 and PLK-1 regulate POS-1 segregation. MEX-5 and PLK-1 are retained in the anterior cytoplasm, likely through the association of MEX-5 with RNA. PLK-1 phosphorylates POS-1 to inhibit its retention in the anterior. As a consequence, POS-1 enriches in the posterior cytoplasm, presumably on RNA. This model predicts POS-1 dephosphorylation is required for its retention in the posterior.
PLK-1 Regulates Polarization of the C. elegans Zygote
PLK-1 Suppresses Premature Polarization During Meiosis
The C. elegans oocyte remains apolar as it matures, is fertilized and completes two meiotic divisions. In the maturing oocyte, the pPARs occupy the cell cortex and the aPARs are restricted to the cytoplasm. By anaphase II of meiosis, the PAR proteins have switched locations with the aPARs at the cell cortex and the pPARs restricted to the cytoplasm (Reich et al., 2019), positioning them to respond to the posterior symmetry breaking cues upon the completion of meiosis (Figure 2B). PLK-1 and Aurora A (AIR-1) suppress the premature cortical loading of the anterior PARs, thereby ensuring polarization is only established following the completion of meiosis. Depletion of either AIR-1 or PLK-1 results in the premature cortical localization of aPARs and the restriction of the pPARs to the cytoplasm in oocytes and meiotic embryos. This recruitment of the aPARs to the cortex “activates” the PAR polarity system prematurely (Figure 2B), such that normally cryptic symmetry breaking cues present in the meiotic embryo, including regions of high membrane curvature and microtubules associated with the meiotic spindle, can trigger polarization before the completion of meiosis (Wallenfang and Seydoux, 2000; Kapoor and Kotak, 2019; Klinkert et al., 2019; Reich et al., 2019; Zhao et al., 2019). As a result, air-1 and plk-1 depleted embryos display a range of polarity defects, including bipolarity, reversed polarity and mispositioned PAR domains (Noatynska et al., 2010; Klinkert et al., 2019; Reich et al., 2019; Zhao et al., 2019). Suppressing activation of the PAR system until after the completion of meiosis ensures the embryo only polarizes in response to the multiple coordinate symmetry breaking cues from the posterior end, resulting in the rapid and highly stereotyped establishment of the anterior/posterior polarity axis.
In the future, it will be important to determine whether PLK-1 and/or AIR-1 is the principal inhibitor of PAR network activation. Depletion of SPAT/Bora, which facilitates the activation of PLK-1 by AIR-1, results in similar polarity defects as depletion of either AIR-1 or PLK-1 (Noatynska et al., 2010). This observation is consistent with PLK-1 acting downstream of AIR-1 to suppress activation of the PAR network. Additionally, it will be important to identify the relevant substrates and mechanisms that suppress activation. As discussed in the next section, PLK-1 phosphorylates PAR-3 to suppress its cortical localization in mitotic one-cell embryos (Dickinson et al., 2017), suggesting this could be one possible mechanism by which PLK-1 might suppress aPAR cortical loading in oocytes and meiotic embryos.
PLK-1 Disassembles PAR-3 Clusters During Polarity Maintenance
Polarity establishment coincides with the dramatic flow of the contractile actomyosin cortex from the posterior toward the anterior (Figure 2B). These actomyosin flows help move the aPARs out of the posterior, clearing the way for pPARs to load from the cytoplasm onto the posterior cortex (Munro et al., 2004; Goehring et al., 2011). During actomyosin flows, the aPARs colocalize in prominent cortical clusters that stabilize their cortical association and help entrain them within actomyosin flows, thereby promoting segregation to the anterior (Tabuse et al., 1998; Hung and Kemphues, 1999; Beers and Kemphues, 2006; Sailer et al., 2015; Dickinson et al., 2017; Rodriguez et al., 2017; Wang et al., 2017). PAR-3 is critical for aPAR clustering: PAR-3 forms clusters in the absence of either PKC-3 or PAR-6, is required for PKC-3 clustering and contains an oligomerization domain that mediates its clustering (Dickinson et al., 2017; Rodriguez et al., 2017; Wang et al., 2017). Actomyosin flows cease when they reach roughly the midpoint of the A/P axis and shortly thereafter PAR-3 clusters disperse as the embryo enters mitosis and the maintenance phase of polarization.
The assembly of PAR-3 into cortical clusters is inhibited by PLK-1. In plk-1(RNAi) embryos, PAR-3 clusters form prematurely (before symmetry breaking), potentially reflecting the premature activation of the PAR network in meiotic embryos discussed above (Dickinson et al., 2017; Reich et al., 2019) (Figure 2B). Additionally, PAR-3 clusters fail to disassemble during maintenance phase in plk-1 mutant embryos. The PAR-3 N-terminus contains two putative PBD binding motifs, both of which are required for the PLK-1 PBD domain to bind PAR-3 in a yeast two-hybrid assay. This interaction also depends on the phosphopeptide-binding motif in the PLK-1 PBD domain, suggesting that priming phosphorylation of PAR-3 stimulates an interaction between PLK-1 and PAR-3 (Dickinson et al., 2017). PAR-3 contains two putative PLK-1 phosphorylation sites (Thr32 and Thr89), one of which was shown to be phosphorylated by PLK-1 in vitro. Importantly, phosphomimetic substitutions at these residues prevent both PAR-3 oligomerization and PAR-3 localization to the cell cortex (Dickinson et al., 2017). Taken together, these data suggest that relatively low levels of PLK-1 activity during interphase allows PAR-3 cluster formation and entrainment in actomyosin flows during polarity establishment. PLK-1 activity increases at the transition from establishment to maintenance phase, at which point PLK-1 phosphorylates PAR-3 to disperse aPAR clusters (Figure 2B). This dispersal makes PAR-3 insensitive to subsequent actomyosin flows during cytokinesis (Dickinson et al., 2017). In the future, it will be interesting to learn how PLK-1 activity toward PAR-3 is coordinated with the transition from polarity establishment to polarity maintenance. For example, the global increase in PLK-1 activity as the embryo enters mitosis could trigger PAR-3 cluster disassembly. Alternately, there may be unknown mechanisms that temporally control PLK-1 activity specifically toward PAR-3. Consistent with the latter possibility, PLK-1 acts prior to maintenance phase to drive the segregation of the cytoplasmic fate determinant POS-1, as discussed below (Han et al., 2018).
PLK-1 Controls Segregation of POS-1
A key output of the PAR polarity system is to control the segregation of cytoplasmic cell fate determinants along the A/P axis of the one-cell embryo, leading to the preferential partitioning of somatic factors to AB and germline factors to P1 (Figure 2B). The polarization of cytoplasmic proteins is not caused by local protein degradation or local protein synthesis, but rather by their preferential retention in either the anterior or posterior cytoplasm, which leads to their accumulation in that cytoplasmic domain (Tenlen et al., 2008; Daniels et al., 2009, 2010; Griffin et al., 2011; Wu et al., 2015, 2018). The posterior kinase PAR-1 inhibits the retention of its substrate the RNA-binding protein MEX-5 in the posterior cytoplasm, leading to MEX-5 segregation to the anterior cytoplasm (Pagano et al., 2007; Tenlen et al., 2008; Griffin et al., 2011). In turn, MEX-5, along with the highly similar protein MEX-6 (MEX-5/6 hereafter), controls the segregation of germline factors to the posterior cytoplasm by inhibiting their retention in the anterior (Schubert et al., 2000) (Figure 2B). For example, MEX-5/6 inhibit the retention of the RNA-binding protein POS-1 in the anterior, leading to the progressive accumulation of POS-1 in the posterior (Farley et al., 2008; Wu et al., 2015; Han et al., 2018). Both the retention of MEX-5 (and presumably MEX-6) in the anterior and the retention of POS-1 in the posterior depends on their ability to bind RNA (Griffin et al., 2011; Han et al., 2018), suggesting they may accumulate on RNA in the anterior and posterior cytoplasm, respectively (Figure 2C).
The ability of MEX-5/6 to drive POS-1 segregation to the posterior depends on PLK-1. The priming kinase MBK-2 phosphorylates MEX-5 and MEX-6 (on MEX-5 residue Thr186), generating a binding site for the PBD domain of PLK-1 (Nishi et al., 2008). MBK-2 becomes active upon the completion of meiosis, suggesting the interaction between MEX-5 and PLK-1 is likely coupled to this transition and to the onset of polarization (Stitzel et al., 2006, 2007; Maruyama et al., 2007; Cheng et al., 2009). As a consequence of its interaction with MEX-5/6, the cytoplasmic pool of PLK-1 becomes enriched in the anterior cytoplasm (Nishi et al., 2008) (Figures 2A,B). In addition, binding to MEX-5 increases PLK-1 kinase activity in vitro, likely by relieving PLK-1 autoinhibition by the PBD domain (Nishi et al., 2008). Similar to mex-5/6 mutant embryos, POS-1 segregation fails in embryos in which PLK-1 has been depleted, in which PLK-1 kinase activity has been inhibited or in embryos in which the interaction between PLK-1 and MEX-5 has been disrupted through mutation of the priming phosphorylation site on MEX-5 (Thr186) (Han et al., 2018). In vitro, PLK-1 phosphorylates a cluster of residues in the POS-1 C-terminal region. In vivo, a non-phosphorylatable allele of POS-1 fails to segregate because it is inappropriately retained in the anterior cytoplasm. In contrast, a phosphomimetic allele of POS-1 fails to segregate because it is not retained in the posterior cytoplasm (Han et al., 2018). These data suggest MEX-5/PLK-1 complexes in the anterior cytoplasm phosphorylate POS-1 to inhibit its retention in the anterior (Figure 2C). Because RNA-binding is required for MEX-5 retention in the anterior and for POS-1 retention in the posterior, one possibility is that MEX-5 recruits PLK-1 to RNA in the anterior where it is positioned to inhibit POS-1 retention on RNA. In contrast to PLK-1 regulation of PAR-3 disassembly, PLK-1 regulates POS-1 segregation throughout both polarity establishment and maintenance phases, suggesting that at least the cytoplasmic pool of PLK-1 is active during interphase.
POS-1 is one of a collection of germline RNA-binding proteins that segregate to the posterior cytoplasm in response to MEX-5/6 (Schubert et al., 2000). Many of these germline proteins concentrate in germ granules (called P granules in C. elegans) which are non-membranous, phase-separated condensates composed primarily of RNA and RNA-binding proteins (Updike and Strome, 2010; Seydoux, 2018). The relatively high concentration of MEX-5/6 in the anterior cytoplasm causes P granules to disassemble, whereas P granules assemble and grow in the posterior cytoplasm (Brangwynne et al., 2009). One mechanism that disassembles P granules in the anterior is the sequestration of RNA by MEX-5/6, which starves P granule proteins of the RNA they need to assemble in granules (Saha et al., 2016; Smith et al., 2016). Interestingly, there is genetic evidence that PLK-1 may also act with MEX-5/6 to stimulate P granule disassembly: both depletion of PLK-1 by RNAi and mutation of the PLK-1 binding site on MEX-5 results in the inappropriate stabilization of P granules in the anterior cytoplasm (Nishi et al., 2008; Wu et al., 2019). Whether PLK-1 plays a direct role in P granule disassembly, for example by inhibiting P granule assembly through direct phosphorylation of P granule protein(s), awaits further study.
PLK-1 Asymmetry Contributes to Differences Between AB and P1
In addition to the role of PLK-1 in regulating asymmetries before the division of the one cell embryo, the asymmetric inheritance of PLK-1 following cell division also contributes to differences in the timing of centrosome separation and cell division between AB and P1. The preferential inheritance of PLK-1 to AB derives from the interaction between PLK-1 and MEX-5 and the resultant enrichment of PLK-1 in the anterior cytoplasm before cell division (Nishi et al., 2008).
PLK-1 Regulation of Cell Division Asymmetry
As in many animal embryos, C. elegans development begins with a series of rapid, reductive cleavages that alternate between interphase and mitosis and lack G1 and G2 phases. Beginning at the two-cell stage, cells in the germline P lineage divide more slowly than their somatic sister cells. For example, P1 divides roughly 2 min after AB (Noatynska et al., 2013; Tavernier et al., 2015a) as a result of the combined effect of differences in the activities of several cell cycle regulators. Part of the cell division asynchrony is due to delayed DNA replication in P1 and the preferential activation of the ATL-1/CHK-1 DNA replication checkpoint in P1, which delays P1's entry into mitosis (Brauchle et al., 2003; Benkemoun et al., 2014). In addition, the preferential inheritance of both PLK-1 and cyclin B3 promotes the advanced timing of AB division (Budirahardja and Gönczy, 2008; Rivers et al., 2008; Michael, 2016). PLK-1 promotes mitotic entry in part through phosphorylation and activation of CDC25. Indeed, the enrichment of PLK-1 in AB correlates with higher levels of nuclear CDC25 in AB than in P1 (Rivers et al., 2008) (Figure 3). Partial depletion of either PLK-1 or CDC25 causes a more substantial delay in the division of P1 than the division of AB, consistent with the idea that the low levels of PLK-1 and CDC25 in P1 are limiting (Budirahardja and Gönczy, 2008; Rivers et al., 2008).
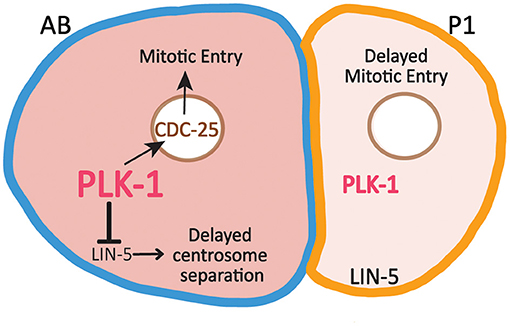
Figure 3. Asymmetric inheritance of PLK-1 contributes to differences in the timing of cell division and centrosome separation between AB and P1. Relatively high levels of PLK-1 in AB promotes mitotic entry by increasing nuclear levels of CDC25, which contributes to the faster cell division timing in AB relative to P1. PLK-1 also acts to reduce cortical LIN-5 levels in AB, which delays centrosome separation in AB.
PLK-1 Regulation of Centrosome Separation in AB
In addition to promoting mitotic entry, the enrichment of PLK-1 in AB also delays centrosome separation in AB relative to P1 (Figure 3). LIN-5 (the C. elegans NUMA ortholog) is a dynein binding protein present in the cytoplasm and at the cell cortex. PLK-1 acts to reduce the cortical levels of LIN-5 in AB relative to P1 (Bondaz et al., 2019). LIN-5 promotes the separation of centrosomes by exerting force on centrosomes through astral microtubules that reach the cortex. As a result of the differences in cortical LIN-5 levels, centrosome separation is delayed in AB relative to P1. Embryos depleted of KLP-7 (the C. elegans MCAK ortholog) are particularly sensitive to the low levels of cortical LIN-5 in AB and therefore exhibit enhanced delay in the timing of centrosome in AB. This delay can be suppressed through depletion in PLK-1 which increases cortical LIN-5 levels in AB (Bondaz et al., 2019). In mammalian cells, PLK1 regulates cortical NUMA levels directly, suggesting PLK-1 may directly target LIN-5 in AB to reduce its cortical association (Kiyomitsu and Cheeseman, 2012; Sana et al., 2018).
Conclusion
In addition to its role in the asymmetric division of the C. elegans zygote, PLK1 also contributes to asymmetric division and cell polarization in other cell types. During the asymmetric division of Drosophila neuroblasts, the asymmetric localization of cortical cell fate determinants is regulated by Polo (the C. elegans ortholog of PLK-1). The membrane-associated proteins Pros, Numb, Miranda and Partner of Numb (Pon) are uniformly distributed during interphase. During late prophase, Polo directly phosphorylates Pon, leading to the asymmetric localization of PON and its binding partner Numb to the basal cortex (Wang et al., 2007). In polo mutants, Pon and Numb are not asymmetrically localized to basal cortex, causing a failure of asymmetric division that leads to neuroblast over-proliferation. Additionally, increased Polo activity at the daughter centrosomes relative to mother centrosomes is essential for the retention of the daughter centrosome in the neuroblast cell following cell division (Januschke et al., 2013; Conduit et al., 2014; Gallaud et al., 2020). The extent to which the resulting asymmetric inheritance of mother and daughter centrosomes regulates the fate of apical and basal daughter cells remains an open area of investigation. Similarly, during the asymmetric division of S. cerevisiae cells, increased Cdc5 activity at the mother centrosome (spindle pole body) is essential for its asymmetric inheritance to the newly born daughter cell (Maekawa et al., 2017). Non-random inheritance of mother and daughter centrosomes is widespread and may contribute to the partitioning of aging/rejuvenation programs between daughter cells or in the asymmetric inheritance of centrosome-associated fate determinants (Macara and Mili, 2008; Pelletier and Yamashita, 2012; Manzano-Lopez et al., 2019; Sunchu and Cabernard, 2020). PLK1 has also been shown to regulate planar cell polarity in mammalian epithelial cells through phosphorylation of CELSR-1 (Flamingo in Drosophila), which triggers CELSR-1 clearance from the cell surface as cells prepare to undergo cytokinesis (Shrestha et al., 2015). These studies, along with the numerous ways in which PLK-1 regulates the asymmetric division of the C. elegans zygote, raise the possibility that PLK1 may act broadly and in diverse ways to control both cell polarization and cell cycle progression. In the future, it will be interesting to learn in which contexts PLK1's role in cell polarization is coordinated with its role in cell cycle progression and in which contexts PLK1 acts independently in these two processes.
Author Contributions
All authors listed have made a substantial, direct and intellectual contribution to the work, and approved it for publication.
Funding
This work was supported by NIH Grants Nos. R01GM110194 and R35GM136302 to EG.
Conflict of Interest
The authors declare that the research was conducted in the absence of any commercial or financial relationships that could be construed as a potential conflict of interest.
References
Archambault, V., and Glover, D. M. (2009). Polo-like kinases: conservation and divergence in their functions and regulation. Nat. Rev. Mol. Cell Biol. 10, 265–275. doi: 10.1038/nrm2653
Barr, F. A., Sillje, H. H., and Nigg, E. A. (2004). Polo-like kinases and the orchestration of cell division. Nat. Rev. Mol. Cell Biol. 5, 429–440. doi: 10.1038/nrm1401
Beers, M., and Kemphues, K. (2006). Depletion of the co-chaperone CDC-37 reveals two modes of PAR-6 cortical association in C. elegans embryos. Development 133, 3745–3754. doi: 10.1242/dev.02544
Benkemoun, L., Descoteaux, C., Chartier, N. T., Pintard, L., and Labbe, J.-C. (2014). PAR-4/LKB1 regulates DNA replication during asynchronous division of the early C. elegans embryo. J. Cell Biol. 205, 447–455. doi: 10.1083/jcb.201312029
Bondaz, A., Cirillo, L., Meraldi, P., and Gotta, M. (2019). Cell polarity-dependent centrosome separation in the C. elegans embryo. J. Cell Biol. 218, 4112–4126. doi: 10.1083/jcb.201902109
Brangwynne, C. P., Eckmann, C. R., Courson, D. S., Rybarska, A., Hoege, C., Gharakhani, J., et al. (2009). Germline P granules are liquid droplets that localize by controlled dissolution/condensation. Science 324, 1729–1732. doi: 10.1126/science.1172046
Brauchle, M., Baumer, K., and Gönczy, P. (2003). Differential activation of the DNA replication checkpoint contributes to asynchrony of cell division in C. elegans embryos. Curr. Biol. 13, 819–827. doi: 10.1016/S0960-9822(03)00295-1
Budirahardja, Y., and Gönczy, P. (2008). PLK-1 asymmetry contributes to asynchronous cell division of C. elegans embryos. Development 135, 1303–1313. doi: 10.1242/dev.019075
Chase, D., Serafinas, C., Ashcroft, N., Kosinski, M., Longo, D., Ferris, D. K., et al. (2000). The polo-like kinase PLK-1 is required for nuclear envelope breakdown and the completion of meiosis in Caenorhabditis elegans. Genesis 26, 26–41. doi: 10.1002/(SICI)1526-968X(200001)26:1<26::AID-GENE6>3.0.CO;2-O
Cheeks, R. J., Canman, J. C., Gabriel, W. N., Meyer, N., Strome, S., and Goldstein, B. (2004). C. elegans PAR proteins function by mobilizing and stabilizing asymmetrically localized protein complexes. Curr. Biol. 14, 851–862. doi: 10.1016/j.cub.2004.05.022
Cheng, K. C.-C., Klancer, R., Singson, A., and Seydoux, G. (2009). Regulation of MBK-2/DYRK by CDK-1 and the pseudophosphatases EGG-4 and EGG-5 during the oocyte-to-embryo transition. Cell 139, 560–572. doi: 10.1016/j.cell.2009.08.047
Conduit, P. T., Feng, Z., Richens, J. H., Baumbach, J., Wainman, A., Bakshi, S. D., et al. (2014). The centrosome-specific phosphorylation of Cnn by Polo/Plk1 drives Cnn scaffold assembly and centrosome maturation. Dev. Cell 28, 659–669. doi: 10.1016/j.devcel.2014.02.013
Daniels, B. R., Dobrowsky, T. M., Perkins, E. M., Sun, S. X., and Wirtz, D. (2010). MEX-5 enrichment in the C. elegans early embryo mediated by differential diffusion. Development 137, 2579–2585. doi: 10.1242/dev.051326
Daniels, B. R., Perkins, E. M., Dobrowsky, T. M., Sun, S. X., and Wirtz, D. (2009). Asymmetric enrichment of PIE-1 in the Caenorhabditis elegans zygote mediated by binary counterdiffusion. J. Cell Biol. 184, 473–479. doi: 10.1083/jcb.200809077
De Henau, S., Pages-Gallego, M., Pannekoek, W. J., and Dansen, T. B. (2020). Mitochondria-derived H2O2 promotes symmetry breaking of the C. elegans zygote. Dev. Cell 53, 263–271. doi: 10.1016/j.devcel.2020.03.008
Dickinson, D. J., Schwager, F., Pintard, L., Gotta, M., and Goldstein, B. (2017). A single-cell biochemistry approach reveals PAR complex dynamics during cell polarization. Dev. Cell. 42, 416–434. doi: 10.1016/j.devcel.2017.07.024
Elia, A. E., Rellos, P., Haire, L. F., Chao, J. W., Ivins, F. J., Hoepker, K., et al. (2003). The molecular basis for phosphodependent substrate targeting and regulation of Plks by the Polo-box domain. Cell 115, 83–95. doi: 10.1016/S0092-8674(03)00725-6
Farley, B. M., Pagano, J. M., and Ryder, S. P. (2008). RNA target specificity of the embryonic cell fate determinant POS-1. RNA 14, 2685–2697. doi: 10.1261/rna.1256708
Gallaud, E., Ramdas Nair, A., Horsley, N., Monnard, A., Singh, P., Pham, T. T., et al. (2020). Dynamic centriolar localization of Polo and Centrobin in early mitosis primes centrosome asymmetry. PLoS Biol. 18:e3000762. doi: 10.1371/journal.pbio.3000762
Goehring, N. W., Trong, P. K., Bois, J. S., Chowdhury, D., Nicola, E. M., Hyman, A. A., et al. (2011). Polarization of PAR proteins by advective triggering of a pattern-forming system. Science 334, 1137–1141. doi: 10.1126/science.1208619
Goldstein, B., and Hird, S. N. (1996). Specification of the anteroposterior axis in Caenorhabditis elegans. Development 122, 1467–1474.
Griffin, E. E. (2015). Cytoplasmic localization and asymmetric division in the early embryo of Caenorhabditis elegans. Wiley Interdiscip. Rev. Dev. Biol. 4, 267–282. doi: 10.1002/wdev.177
Griffin, E. E., Odde, D. J., and Seydoux, G. (2011). Regulation of the MEX-5 gradient by a spatially segregated kinase/phosphatase cycle. Cell 146, 955–968. doi: 10.1016/j.cell.2011.08.012
Gubieda, A. G., Packer, J. R., Squires, I., Martin, J., and Rodriguez, J. (2020). Going with the flow: insights from Caenorhabditis elegans zygote polarization. Philos. Trans. R. Soc. Lond. B. Biol. Sci. 375:20190555. doi: 10.1098/rstb.2019.0555
Han, B., Antkowiak, K. R., Fan, X., Rutigliano, M., Ryder, S. P., and Griffin, E. E. (2018). Polo-like kinase couples cytoplasmic protein gradients in the C. elegans zygote. Curr Biol. 28, 60–69. doi: 10.1016/j.cub.2017.11.048
Hung, T. J., and Kemphues, K. J. (1999). PAR-6 is a conserved PDZ domain-containing protein that colocalizes with PAR-3 in Caenorhabditis elegans embryos. Development 126, 127–135.
Jang, Y. J., Ma, S., Terada, Y., and Erikson, R. L. (2002). Phosphorylation of threonine 210 and the role of serine 137 in the regulation of mammalian polo-like kinase. J. Biol. Chem. 277, 44115–44120. doi: 10.1074/jbc.M202172200
Januschke, J., Reina, J., Llamazares, S., Bertran, T., Rossi, F., Roig, J., et al. (2013). Centrobin controls mother-daughter centriole asymmetry in Drosophila neuroblasts. Nat. Cell Biol. 15, 241–248. doi: 10.1038/ncb2671
Kapoor, S., and Kotak, S. (2019). Centrosome Aurora A regulates RhoGEF ECT-2 localisation and ensures a single PAR-2 polarity axis in C. elegans embryos. Development 146:dev174565. doi: 10.1242/dev.174565
Kiyomitsu, T., and Cheeseman, I. M. (2012). Chromosome- and spindle-pole-derived signals generate an intrinsic code for spindle position and orientation. Nat. Cell Biol. 14, 311–317. doi: 10.1038/ncb2440
Klinkert, K., Levernier, N., Gross, P., Gentili, C., von Tobel, L., Pierron, M., et al. (2019). Aurora A depletion reveals centrosome-independent polarization mechanism in Caenorhabditis elegans. Elife 8:e44552. doi: 10.7554/eLife.44552
Knoblich, J. A. (2010). Asymmetric cell division: recent developments and their implications for tumour biology. Nat. Rev. Mol. Cell Biol. 11, 849–860. doi: 10.1038/nrm3010
Kysela, D. T. P., Brown, J. B., Huang, K. C., and Brun, Y. V. (2013). Biological consequences and advantages of asymmetric bacterial Growth 67, 417–435. doi: 10.1146/annurev-micro-092412-155622
Lang, C. F., and Munro, E. (2017). The PAR proteins: from molecular circuits to dynamic self-stabilizing cell polarity. Development 144, 3405–3416. doi: 10.1242/dev.139063
Lee, K. S., Grenfell, T. Z., Yarm, F. R., and Erikson, R. L. (1998). Mutation of the polo-box disrupts localization and mitotic functions of the mammalian polo kinase Plk. Proc. Natl. Acad. Sci. U.S.A. 95, 9301–9306. doi: 10.1073/pnas.95.16.9301
Li, R. (2013). The art of choreographing asymmetric cell division. Dev. Cell. 25, 439–450. doi: 10.1016/j.devcel.2013.05.003
Lowery, D. M., Lim, D., and Yaffe, M. B. (2005). Structure and function of Polo-like kinases. Oncogene 24, 248–259. doi: 10.1038/sj.onc.1208280
Macara, I., and Mili, S. (2008). Polarity and differential inheritance—universal attributes of life? Cell 135, 801–812. doi: 10.1016/j.cell.2008.11.006
Macurek, L., Lindqvist, A., Lim, D., Lampson, M. A., Klompmaker, R., Freire, R., et al. (2008). Polo-like kinase-1 is activated by aurora A to promote checkpoint recovery. Nature 455, 119–123. doi: 10.1038/nature07185
Maekawa, H., Neuner, A., Ruthnick, D., Schiebel, E., Pereira, G., and Kaneko, Y. (2017). Polo-like kinase Cdc5 regulates Spc72 recruitment to spindle pole body in the methylotrophic yeast Ogataea polymorpha. Elife 6:e24340. doi: 10.7554/eLife.24340
Manzano-Lopez, J., Matellan, L., Alvarez-Llamas, A., Blanco-Mira, J. C., and Monje-Casas, F. (2019). Asymmetric inheritance of spindle microtubue-organizing centers preserves replicative lifespan 21, 952–965. doi: 10.1038/s41556-019-0364-8
Martino, L., Morchoisne-Bolhy, S., Cheerambathur, D. K., Van Hove, L., Dumont, J., Joly, N., et al. (2017). Channel nucleoporins recruit PLK-1 to nuclear pore complexes to direct nuclear envelope breakdown in C. elegans. Dev. Cell. 43, 157–171. doi: 10.1016/j.devcel.2017.09.019
Maruyama, R., Velarde, N. V., Klancer, R., Gordon, S., Kadandale, P., Parry, J. M., et al. (2007). EGG-3 regulates cell-surface and cortex rearrangements during egg activation in Caenorhabditis elegans. Curr. Biol. 17, 1555–1560. doi: 10.1016/j.cub.2007.08.011
Michael, W. M. (2016). Cyclin CYB-3 controls both S-phase and mitosis and is asymmetrically distributed in the early C. elegans embryo. Development. 143, 3119–3127. doi: 10.1242/dev.141226
Motegi, F., Zonies, S., Hao, Y., Cuenca, A. A., Griffin, E., and Seydoux, G. (2011). Microtubules induce self-organization of polarized PAR domains in Caenorhabditis elegans zygotes. Nat. Cell Biol. 13, 1361–1367. doi: 10.1038/ncb2354
Munro, E., Nance, J., and Priess, J. R. (2004). Cortical flows powered by asymmetrical contraction transport PAR proteins to establish and maintain anterior-posterior polarity in the early C. elegans embryo. Dev. Cell. 7, 413–424. doi: 10.1016/j.devcel.2004.08.001
Nishi, Y., Rogers, E., Robertson, S. M., and Lin, R. (2008). Polo kinases regulate C. elegans embryonic polarity via binding to DYRK2-primed MEX-5 and MEX-6. Development 135, 687–697. doi: 10.1242/dev.013425
Noatynska, A., Panbianco, C., and Gotta, M. (2010). SPAT-1/Bora acts with Polo-like kinase 1 to regulate PAR polarity and cell cycle progression. Development 137, 3315–3325. doi: 10.1242/dev.055293
Noatynska, A., Tavernier, N., Gotta, M., and Pintard, L. (2013). Coordinating cell polarity and cell cycle progression: what can we learn from flies and worms? Open Biol. 3:130083. doi: 10.1098/rsob.130083
Pagano, J. M., Farley, B. M., Mccoig, L. M., and Ryder, S. P. (2007). Molecular basis of RNA recognition by the embryonic polarity determinant MEX-5. J. Biol. Chem. 282, 8883–8894. doi: 10.1074/jbc.M700079200
Pelletier, L., and Yamashita, Y. M. (2012). Centrosome asymmetry and inheritance during animal development. Curr Opin Cell BioI. 24, 541–546. doi: 10.1016/j.ceb.2012.05.005
Petronczki, M., Lenart, P., and Peters, J. M. (2008). Polo on the rise-from mitotic entry to cytokinesis with Plk1. Dev. Cell. 14, 646–659. doi: 10.1016/j.devcel.2008.04.014
Pintard, L., and Archambault, V. (2018). A unified view of spatio-temporal control of mitotic entry: polo kinase as the key. Open Biol. 8:180114. doi: 10.1098/rsob.180114
Rahman, M., Chang, I. Y., Harned, A., Maheshwari, R., Amoateng, K., Narayan, K., and Cohen-Fix, O. (2020). C. elegans pronuclei fuse after fertilization through a novel membrane structure. J. Cell Biol. 219:e201909137. doi: 10.1083/jcb.201909137
Rahman, M. M., Munzig, M., Kaneshiro, K., Lee, B., Strome, S., Muller-Reichert, T., and Cohen-Fix, O. (2015). Caenorhabditis elegans polo-like kinase PLK-1 is required for merging parental genomes into a single nucleus. Mol. Biol. Cell. 26, 4718–4735. doi: 10.1091/mbc.E15-04-0244
Reich, J. D., Hubatsch, L., Illukkumbura, R., Peglion, F., Bland, T., Hirani, N., et al. (2019). Regulated activation of the PAR polarity network ensures a timely and specific response to spatial cues. Curr. Biol. 29, 1911–1923. doi: 10.1016/j.cub.2019.04.058
Rivers, D. M., Moreno, S., Abraham, M., and Ahringer, J. (2008). PAR proteins direct asymmetry of the cell cycle regulators Polo-like kinase and Cdc25. J. Cell Biol. 180, 877–885. doi: 10.1083/jcb.200710018
Rodriguez, J., Peglion, F., Martin, J., Hubatsch, L., Reich, J., Hirani, N., et al. (2017). aPKC cycles between functionally distinct PAR protein assemblies to drive cell polarity. Dev. Cell. 42, 400–415. doi: 10.1016/j.devcel.2017.07.007
Rose, L., and Gonczy, P. (2014). “Polarity establishment, asymmetric division and segregation of fate determinants in early C. elegans embryos (December 30, 2014),” in WormBook, ed The C. elegans Research Community (WormBook). Avalable online at: http://www.wormbook.org
Saha, S., Weber, C. A., Nousch, M., Adame-Arana, O., Hoege, C., Hein, M. Y., et al. (2016). Polar positioning of phase-separated liquid compartments in cells regulated by an mRNA competition mechanism. Cell 166, 1572–1584. doi: 10.1016/j.cell.2016.08.006
Sailer, A., Anneken, A., Li, Y., Lee, S., and Munro, E. (2015). Dynamic opposition of clustered proteins stabilizes cortical polarity in the C. elegans zygote. Dev. Cell. 35, 131–142. doi: 10.1016/j.devcel.2015.09.006
Sana, S., Keshri, R., Rajeevan, A., Kappor, S., and Kotak, S. (2018). PLK1 regulates spindle orientation by phosphorylating NuMA in human cells. Life Sci. Alliance. 1:e201800223. doi: 10.26508/lsa.201800223
Schubert, C. M., Lin, R., de Vries, C. J., Plasterk, R. H., and Priess, J. R. (2000). MEX-5 and MEX-6 function to establish soma/germline asymmetry in early C. elegans embryos. Mol Cell. 5, 671–682. doi: 10.1016/S1097-2765(00)80246-4
Seki, A., Coppinger, J. A., Jang, C. Y., Yates, J. R., and Fang, G. (2008). Bora and the kinase Aurora a cooperatively activate the kinase Plk1 and control mitotic entry. Science 320, 1655–1658. doi: 10.1126/science.1157425
Seydoux, G. (2018). The P Granules of C. elegans: a genetic model for the study of RNA-protein condensates. J. Mol. Biol. 430, 4702–4710. doi: 10.1016/j.jmb.2018.08.007
Shrestha, R., Little, K. A., Tamayo, J. V., Li, W., Perlman, D. H., and Devenport, D. (2015). Mitotic control of planar cell polarity by Polo-like kinase 1. Dev. Cell. 33, 522–534. doi: 10.1016/j.devcel.2015.03.024
Smith, J., Calidas, D., Schmidt, H., Lu, T., Rasoloson, D., and Seydoux, G. (2016). Spatial patterning of P granules by RNA-induced phase separation of the intrinsically-disordered protein MEG-3. Elife 5:e21337. doi: 10.7554/eLife.21337
Stitzel, M. L., K. C.-,Cheng, C., and Seydoux, G. (2007). Regulation of MBK-2/Dyrk kinase by dynamic cortical anchoring during the oocyte-to-zygote transition. Curr. Biol. 17, 1545–1554. doi: 10.1016/j.cub.2007.08.049
Stitzel, M. L., Pellettieri, J., and Seydoux, G. (2006). The C. elegans DYRK kinase MBK-2 marks oocyte proteins for degradation in response to meiotic maturation. Curr. Biol. 16, 56–62. doi: 10.1016/j.cub.2005.11.063
Strebhardt, K., and Ullrich, A. (2006). Targeting polo-like kinase 1 for cancer therapy. Nat. Rev. Cancer. 6:321–330. doi: 10.1038/nrc1841
Sunchu, B., and Cabernard, C. (2020). Principles and mechanisms of asymmetric cell division. Development 147:dev167650. doi: 10.1242/dev.167650
Sunkel, C. E., and Glover, D. M. (1988). Polo, a mitotic mutant of Drosophila displaying abnormal spindle poles. J. Cell Sci. 89, 25–38.
Tabuse, Y., Izumi, Y., Piano, F., Kemphues, K. J., Miwa, J., and Ohno, S. (1998). Atypical protein kinase C cooperates with PAR-3 to establish embryonic polarity in Caenorhabditis elegans. Development 125, 3607–3614.
Tavernier, N., Labbe, J. C., and Pintard, L. (2015a). Cell cycle timing regulation during asynchronous divisions of the early C. elegans embryo. Exp. Cell Res. 337, 243–248. doi: 10.1016/j.yexcr.2015.07.022
Tavernier, N., Noatynska, A., Panbianco, C., Martino, L., Van Hove, L., Schwager, F., et al. (2015b). Cdk1 phosphorylates SPAT-1/Bora to trigger PLK-1 activation and drive mitotic entry in C. elegans embryos. J. Cell Biol. 208, 661–669. doi: 10.1083/jcb.201408064
Tenlen, J. R., Molk, J. N., London, N., Page, B. D., and Priess, J. R. (2008). MEX-5 asymmetry in one-cell C. elegans embryos requires PAR-4- and PAR-1-dependent phosphorylation. Development 135, 3665–3675. doi: 10.1242/dev.027060
Tsai, M. C., and Ahringer, J. (2007). Microtubules are involved in anterior-posterior axis formation in C. elegans embryos. J. Cell Biol. 179, 397–402. doi: 10.1083/jcb.200708101
Updike, D., and Strome, S. (2010). P granule assembly and function in Caenorhabditis elegans germ cells. J. Androl. 31, 53–60. doi: 10.2164/jandrol.109.008292
Velez-Aguilera, G., Nkombo Nkoula, S., Ossareh-Nazari, B., Link, J., Paouneskou, D., Van Hove, L., et al. (2020). PLK-1 promotes the merger of the parental genome into a single nucleus by triggering lamina disassembly. Elife 9:e59510. doi: 10.7554/eLife.59510
Venkei, Z. G., and Yamashita, Y. M. (2018). Emerging mechanisms of asymmetric stem cell division. J. Cell Biol. 217, 3785–3795. doi: 10.1083/jcb.201807037
Wallenfang, M. R., and Seydoux, G. (2000). Polarization of the anterior-posterior axis of C. elegans is a microtubule-directed process. Nature 408, 89–92. doi: 10.1038/35040562
Wang, H., Ouyang, Y., Somers, W. G., Chia, W., and Lu, B. (2007). Polo inhibits progenitor self-renewal and regulates Numb asymmetry by phosphorylating Pon. Nature 449, 96–100. doi: 10.1038/nature06056
Wang, S. C. T., Low, Y. F., Nishimura, Y., Gole, L., Yu, W., et al. (2017). Cortical forces and CDC-42 control clustering of PAR proteins for Caenorhabditis elegans embryonic polarization. Nat. Cell Biol. 19, 988–995. doi: 10.1038/ncb3577
Woodruff, J. B., Wueseke, O., Viscardi, V., Mahamid, J., Ochoa, S. D., Bunkenborg, J., et al. (2015). Centrosomes. Regulated assembly of a supramolecular centrosome scaffold in vitro. Science 348, 808–812. doi: 10.1126/science.aaa3923
Wu, Y., Han, B., Gauvin, T. J., Smith, J., Singh, A., and Griffin, E. E. (2019). Single-molecule dynamics of the P granule scaffold MEG-3 in the Caenorhabditis elegans zygote. Mol. Biol. Cell. 30, 333–345. doi: 10.1091/mbc.E18-06-0402
Wu, Y., Han, B., Li, Y., Munro, E., Odde, D. J., and Griffin, E. E. (2018). Rapid diffusion-state switching underlies stable cytoplasmic gradients in the Caenorhabditis elegans zygote. Proc. Natl. Acad. Sci. U.S.A. 115, E8440–E8449. doi: 10.1073/pnas.1722162115
Wu, Y., Zhang, H., and Griffin, E. E. (2015). Coupling between cytoplasmic concentration gradients through local control of protein mobility in the Caenorhabditis elegans zygote. Mol. Biol. Cell. 26, 2963–2970. doi: 10.1091/mbc.E15-05-0302
Zhao, P., Teng, X., Tantirimudalige, S. N., Nishikawa, M., Wohland, T., Toyama, Y., et al. (2019). Aurora-A breaks symmetry in contractile actomyosin networks independently of its role in centrosome maturation. Dev. Cell. 48, 631–645 e636. doi: 10.1016/j.devcel.2019.02.012
Keywords: PLK1 (Polo-like Kinase 1), C. elegans, asymmetric cell division (ACD), cell polarity, PAR proteins
Citation: Kim AJ and Griffin EE (2021) PLK-1 Regulation of Asymmetric Cell Division in the Early C. elegans Embryo. Front. Cell Dev. Biol. 8:632253. doi: 10.3389/fcell.2020.632253
Received: 22 November 2020; Accepted: 21 December 2020;
Published: 21 January 2021.
Edited by:
Yi Wu, UCONN Health, United StatesReviewed by:
Monica Gotta, Université de Genève, SwitzerlandAna Carmena, Instituto de Neurociencias de Alicante (IN), Spain
Copyright © 2021 Kim and Griffin. This is an open-access article distributed under the terms of the Creative Commons Attribution License (CC BY). The use, distribution or reproduction in other forums is permitted, provided the original author(s) and the copyright owner(s) are credited and that the original publication in this journal is cited, in accordance with accepted academic practice. No use, distribution or reproduction is permitted which does not comply with these terms.
*Correspondence: Erik E. Griffin, ZXJpay5lLmdyaWZmaW5AZGFydG1vdXRoLmVkdQ==
†ORCID: Amelia J. Kim orcid.org/0000-0002-4178-699X
Erik E. Griffin orcid.org/0000-0001-9958-2466