- Department of Biology, Philipps-University Marburg, Marburg, Germany
Planar cell polarity and anisotropic cell behavior play critical roles in large-scale epithelial morphogenesis, homeostasis, wound repair, and regeneration. Cell–Cell communication and mechano-transduction in the second to minute scale mediated by E-cadherin complexes play a central role in the coordination and self-organization of cellular activities, such as junction dynamics, cell shape changes, and cell rearrangement. Here we review the current understanding in the interplay of cell polarity and cell dynamics during body axis elongation and dorsal closure in Drosophila embryos with a focus on E-cadherin dynamics in linking cell and tissue polarization and tissue-scale shape changes.
Introduction
Epithelia constitute the surface of organs in multicellular organisms and the units of many morphogenetic processes. Epithelial cells adhere to one another to form two-dimensional sheets and constitute permeability barriers for compartmentalization of the body, which is essential for the physiology and protection of the organs and even the whole organisms. Despite their physical integrity and stability, epithelial sheets are intrinsically dynamic and able to restructure in a time scale as fast as minutes (Gumbiner, 1992; Leptin, 1994; Lye and Sanson, 2011; Lv et al., 2019). During morphogenesis, epithelia undergo tissue-scale morphology changes, such as extension, closure, invagination, tubulation, and wrapping. Underlying those morphogenetic processes are cellular activities such as junction remodeling, cell shape changes, and cell rearrangement.
Planar polarity is based on molecular asymmetries within the epithelial sheet and cells and impinges on the cellular activities leading to tissue-scale shape changes. Cell junctions are at the center of the transition from cells to tissue. The mechanical link between is constituted by adherens junctions with E-cadherin (E-cad)–catenin complexes as the central component. Together with numerous associated proteins varying between cell types and developmental stages, the E-cad complex provides a mechanical link between the actomyosin networks of adjacent cells and coordinates their activities via mechanotransduction (Maître and Heisenberg, 2013; Leckband and de Rooij, 2014; Charras and Yap, 2018).
In this review, we will focus on recent progress in two processes of Drosophila embryogenesis, i.e., germband extension and dorsal closure. With these two case studies, we will discuss how cell and tissue polarization are coordinated to give rise to tissue-scale changes in visible morphology.
Drosophila Embryonic Epithelium
The first epitheliogenesis, termed cellularization, in Drosophila development is initiated when the zygotic genome is activated at the transition from syncytial to cellular morphology (Schmidt and Grosshans, 2018). Cell polarization and epithelial sheet formation are intrinsically linked during cellularization. As the plasma membrane ingresses, the cell cortex becomes polarized as visible by segregation of cortical markers. Initially assembling into spot junctions distributed along the lateral furrow, the E-cad–catenin complex coalesces into unmatured adherens junctions at the typical subapical position only by the end of cellularization. During gastrulation, the epithelial epidermis undergoes stage and position-dependent morphogenetic movements, such as tissue invagination (Leptin, 2005; Martin, 2020), folding (Wang et al., 2012), convergent extension (Kong et al., 2017; Paré and Zallen, 2020), compartmental boundaries formation (Sharrock and Sanson, 2020), and dorsal closure (Hayes and Solon, 2017; Kiehart et al., 2017) to name the most prominent ones.
DE-Cadherin and Adherens Junctions
Drosophila E-cadherin (DE-cadherin, DE-cad), known as Shotgun (Shg) in Drosophila, was identified as Armadillo (β-catenin) associated glycoprotein (Oda et al., 1994) and by the zygotic lethal mutation shotgun (Tepass et al., 1996; Uemura et al., 1996). Similar to classical cadherins in vertebrates, DE-cad is a single-transmembrane protein with seven cadherin repeats at its extracellular N-terminal region, followed by a cysteine-rich region, an EGF-like region and a laminin G domain. The cytoplasmic part contains binding sites for p120-catenin (Myster et al., 2003) and β-catenin (Pai et al., 1996), which leads to the assembly of the stereotypic cadherin–catenin complex at the core of adherens junctions (Figure 1A). DE-cad is proteolytically cleaved at its cysteine-rich region into two fragments after translation. The two fragments remain associated, however, via non-covalent interactions to form the mature protein (Oda and Tsukita, 1999). E-cad molecules undergo stable Ca2+-dependent homotypic interactions in trans between adjacent cells (Oda et al., 1994). The mammalian E-cad contains five cadherin repeats at its N-terminal portion. Of these, the most N-terminal-most cadherin domain engages in homophilic binding. In Drosophila, the four N-terminal-most cadherin domains have been reported to mediate the trans interaction (Figure 1B) (Nishiguchi et al., 2016). Beside the polypeptide backbone, post-translational modifications, such as glycosylation and phosphorylation, are essential for the functions of DE-cad and epithelial morphogenesis (Zhang et al., 2014; Chen et al., 2017).
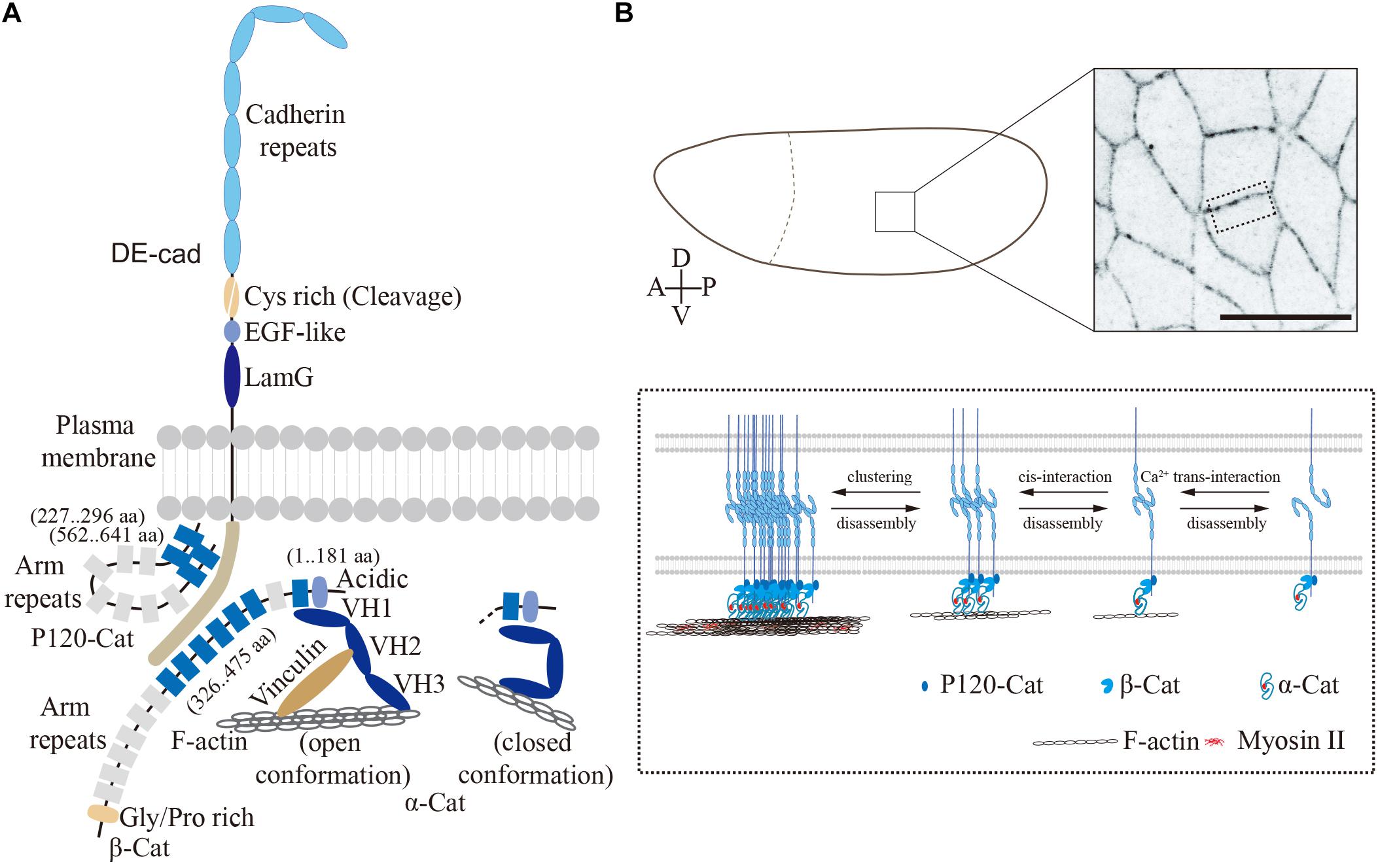
Figure 1. Drosophila embryonic epithelium. (A) The cadherin–catenin complex in Drosophila (modified from Harris, 2012). The single-transmembrane protein, DE-cadherin mediates cell–cell adhesion through homophilic interactions between its extracellular domain. In contrast, its cytoplasmic domain binding with p120-catenin, β-catenin, and α-catenin as cadherin–catenin complex forms the core of adherens junctions. Protein domains and interacting regions are indicated. (B) Confocal image of lateral epithelium from an embryo expressing E-Cad-GFP, scale bar is 10 μm. The close-up view on the bottom shows the adherens junctions and DE-cad clusters in Drosophila epithelium. DE-cad forms the adherens junctions via homophilic binding of N-terminal-most four extracellular cadherin domains, while the cytoplasmic domain binding with actomyosin network via Arm/ß-catenin and α-catenin.
In the fertilized egg and syncytial stage, DE-cad is more or less uniformly distributed within the plasma membrane and intracellular vesicles. The first junctions involving DE-cad are observed during cellularization (Cox et al., 1996; Müller and Wieschaus, 1996). Generic adherens junctions at a subapical position with an F-actin belt form and mature during late cellularization and gastrulation (stage 7–9), when the DE-cad density increases and coalesces into clusters and stable microdomains (Harris and Peifer, 2004; Cavey et al., 2008; Truong Quang et al., 2013). Beside the Ca2+-dependent interactions in trans, E-cadherin molecules bind to each other in cis within the same lipid bilayer to form super-molecular clusters (Figure 1B). Similar to mammalian cells (Engl et al., 2014; Wu et al., 2015), the DE-cad clusters require interactions with F-actin (Truong Quang et al., 2013). Non-muscle Myosin-II (Myosin-II) dependent tensile forces promote DE-cad clustering at cell contacts (Kale et al., 2018). However, the detailed mechanisms by which the cell cortex impinges on the DE-cad clusters remain elusive. In vitro studies revealed a function of the intracellular cadherin–catenin complex as a force sensor. Mechanical forces from actin cytoskeleton induce long-lived bonds in the cadherin-catenin complex (Buckley et al., 2014) and promote binding of the actin-binding protein Vinculin to α-catenin. In this way, a self-reinforcing system is established to strengthen the linkage between E-cad clusters and the actin cytoskeleton.
Armadillo is the Drosophila homolog of β-catenin, whose 13 copies of so-called Armadillo repeats are its characteristic feature (Peifer and Wieschaus, 1990). The N-terminal region and the first Armadillo repeat bind to α-catenin, while Armadillo repeats 3–8 are necessary and sufficient for DE-cad binding (Orsulic and Peifer, 1996; Pai et al., 1996), thus generating a bridge between the plasma membrane with E-cad and α-catenin with F-actin.
Within α-catenin, the VH1 domain mediates the interaction with ß-catenin (Oda et al., 1993; Pai et al., 1996) and the VH3 domain, binding to F-actin (Pokutta et al., 2008). Vertebrate α-catenin undergoes a reversible force-dependent change between two stable conformations (Choi et al., 2012; Rangarajan and Izard, 2012; Yao et al., 2014; Charras and Yap, 2018; Ishiyama et al., 2018). In the open conformation, when force is applied, α-catenin is bound on the one side to the Cadherin complex and the other side via the central mechanosensitive modulatory (M) domain to the D1 domain of Vinculin, thus bridging adherens junctions and F-actin. In contrast, when no force is applied, α-catenin changes into closed conformation with an inaccessible M-domain. In the closed conformation, α-catenin binds only to the Cadherin complex but not to Vinculin and its associated F-actin (Figure 1A). In Drosophila embryos, Vinculin colocalizes with E-cad (Kale et al., 2018), which is promoted by intracellular contracting forces and reduced following tissue relaxation (Kong et al., 2019).
p120-catenin is involved in endocytosis of the dynamic E-cadherin and Bazooka complexes in Drosophila embryos (Bulgakova and Brown, 2016). Binding of p120-catenin also appears to be mechanosensitive as recent research from Drosophila wing epithelium. In this system, p120-catenin is involved in E-cadherin turnover and epithelial viscoelasticity (Iyer et al., 2019). The numerous proteins associated with adherens junctions beyond the core complex have been discussed and reviewed by Harris (2012), for example.
In summary, adherens junctions with the E-cad–catenin complex at its core link the actin cytoskeletons of two neighboring cells in an epithelium (Figure 1). Spatial and temporal modulation of the complexes is a central feature of dynamic epithelia during embryogenesis.
Germband Extension: From Anterior–Posterior Pattern to Planar Polarity to Cell Intercalation
Drosophila germband extension serves as a paradigm for axis elongation by convergence and extension of an epithelial sheet (Figures 2A–C) (Kong et al., 2017). During germband extension, the lateral epidermis increases its length more than two-fold along the anterior–posterior (AP) axis, while correspondingly narrowing along the dorsal–ventral (DV) axis. The elongation of the tissue is largely due to polarized cell rearrangement by neighbor exchanges (Figures 2A–C) (Irvine and Wieschaus, 1994), whose key process is junction remodeling similar to a topological T1 transition (Figure 2C) (Weaire and Rivier, 1984). T1 transitions consist of two phases: (1) collapse of a junction (DV orientation, AP interfaces) leading to fusion of two 3x vertices into a single 4x vertex and (2) expansion of a new junction in perpendicular orientation (AP direction, DV interfaces) creating two new 3x vertices out of the transient 4x vertex (Figure 2C) (Bertet et al., 2004). A complex variant of T1 transitions, rosettes, are observed later in germband extension when multiple junctions collapse simultaneously to generate multiple fold vertices (rosette), which subsequently resolved by the formation of multiple new junctions (Figure 2C) (Blankenship et al., 2006).
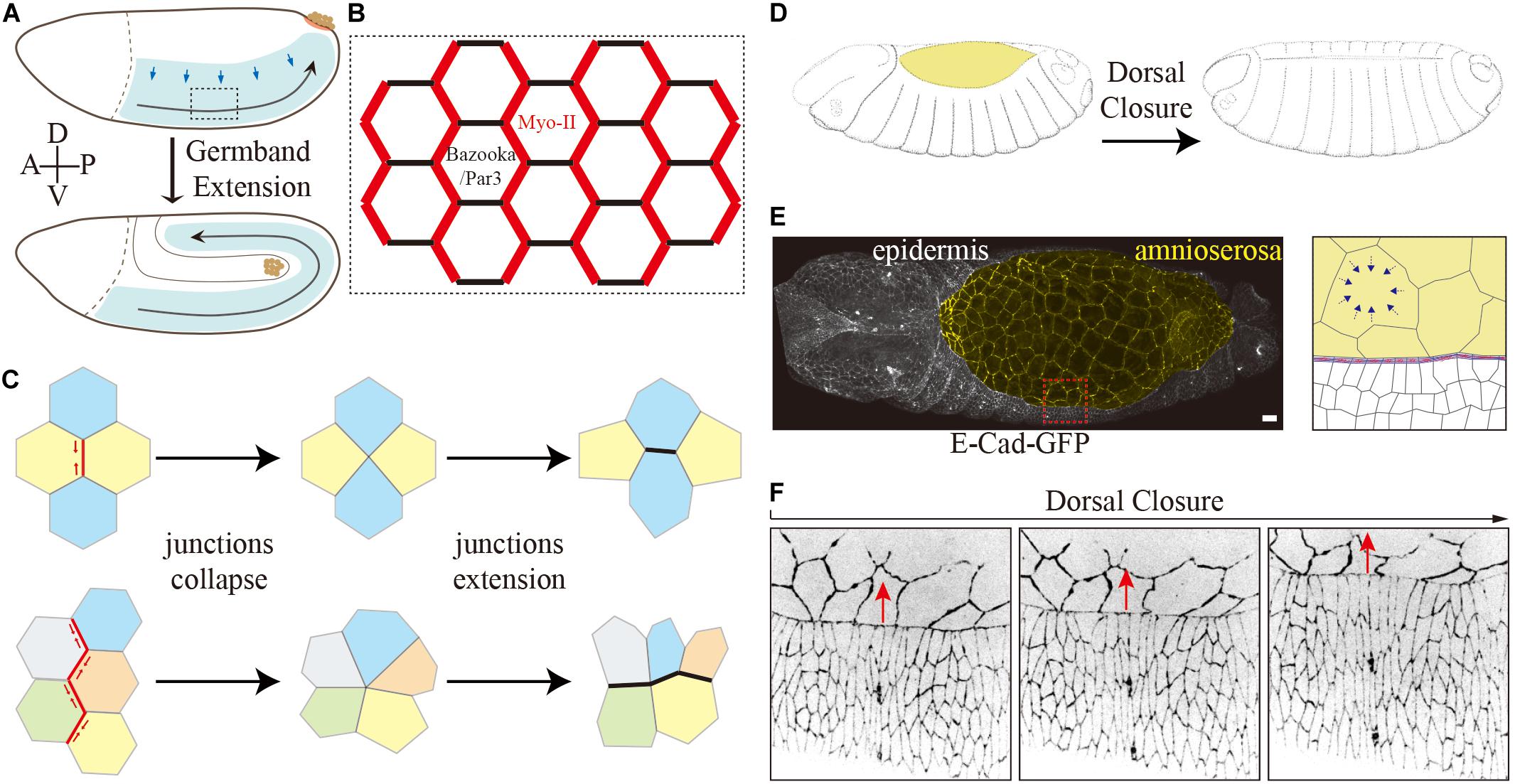
Figure 2. Two tissue-scale shapes change events during Drosophila embryogenesis. (A–C) Germband extension. (A) Schematic drawing of germband extension. (B) Polarized planer polarity of the lateral epidermis during germband extension. Myosin-II is enriched explicitly at AP interfaces, and Bazooka/PAR-3 is enriched at DV interfaces conversely in the intercalating cells. (C) Schematic representation of a simple and rosette-type T1 transition. (D–F) Dorsal closure. Schematic drawing of dorsal closure. Images modified from Hartenstein (1993) (D). It involves two different types of epithelial tissues and their coordination: amnioserosa (yellow) and epidermal cells (E). Confocal image of an embryo expressing E-Cad-GFP. The magnified view on the right shows the interface of amnioserosa and epidermal cells. The blue arrows indicate cell contraction. The leading edge cells polarize by the accumulation of filamentous actin and myosin II at the epidermis–amnioserosa interface in the form of an F-actin cable. (F) Time series of an embryo expressing E-Cad-GFP shows the shape of surrounding epidermal cells elongates along the dorsal–ventral axis during dorsal closure. The red arrows indicate the movements of epidermal cells during dorsal closure.
Myosin-II and the junction-associated actomyosin network on the one side and Baz/PAR-3 and adherens junction proteins on the other side show a complementary and polarized distribution at the junctions and thus reflect a planar polarity (Figure 2B) (Bertet et al., 2004; Zallen and Wieschaus, 2004; Blankenship et al., 2006). Myosin-II and F-actin, enriched at AP interfaces, generate contractile forces leading to junction collapse (Bertet et al., 2004; Zallen and Wieschaus, 2004; Blankenship et al., 2006; Rauzi et al., 2008; Fernandez-Gonzalez et al., 2009). The force is probably generated by a flow of contractile filaments away from the adherens junctions at the apical cortex (medial). In an isotropic case, this leads to apical contractions (Martin et al., 2009; Kong et al., 2019). In the planar polarized situation of the lateral epidermis, the force acts in an anisotropic fashion mainly on the junctions with a DV orientation to induce a junction collapse (Rauzi et al., 2010).
The cortical and junctional actomyosin network is the force-generating machinery in the cell. Myosin-II exists as an inactive hexametric complex, consisting of two heavy chains, two essential light chains (ELC) and two regulatory light chains (RLC) (Hartman and Spudich, 2012). The Rho signaling pathway is essential for this polarization in the lateral epidermis and Myosin-II activity. Myosin-II is activated by phosphorylation of the RLCs by Rho-kinase (Rok) among other protein kinases. During germband extension, Rok is enriched at AP interface (de Matos Simões et al., 2010), and activated by the G protein-coupled receptor (GPRC)-Rho1 signaling (Kerridge et al., 2016), involving Dp114RhoGEF and the subunits of trimeric G proteins, Gβ13F/Gγ1 (De Las Bayonas et al., 2019). The asymmetry in Rho1 and Rok activation leads to polarized Myosin-II activation at AP junctions (de Matos Simões et al., 2010; Simões et al., 2014). Ligands of the FGF family control the assembly of rosette-like mechanosensory organs in the migrating lateral line primordium of the zebrafish (Lecaudey et al., 2008; Nechiporuk and Raible, 2008). It was revealed that Fgfr-Ras-MAPK signaling is required for apical constriction via apical positioning of Rho-associated kinase (Harding and Nechiporuk, 2012), which could be a potential further mechanism for acto-myosin activation during Drosophila germband extension. In parallel Rho1 also activates the formin Diaphanous (Dia), which initiates DE-cad endocytosis leading to depletion of α-catenin (Levayer et al., 2011) and Baz/PAR-3 at AP interface (de Matos Simões et al., 2010; Simões et al., 2014).
The initial signal for polarization is provided by the striped expression of anteroposterior patterning genes (Irvine and Wieschaus, 1994). The striped and staggered expression of the primary pair-rule genes, runt, eve, and paired imposes a planar polarity on the tissue, which guides the orientation of T1 transitions and thus the directionality of cell intercalation. AP patterning of Drosophila embryo is controlled by a hierarchical genetic cascade starting with localized maternal determinants to the zygotic gap, pair-rule, and segment polarity genes (Nasiadka et al., 2002). The link between patterning genes and planar cell polarity is mediated by members of the Toll receptor (protein) family (Paré et al., 2014). The staggered expression of primary pair-rule genes induces a corresponding stripe-like expression of Toll-2, 6, 8 (Eldon et al., 1994; Kambris et al., 2002). Heterophilic interfaces at the AP interfaces between these Toll-2,6,8 proteins, lacking at DV interfaces, induce specific signaling different between AP and DV interfaces (Paré et al., 2014; Tetley et al., 2016). The molecular link between Toll receptors and Myo-II may be provided by the adhesion GPCR Cirl, which can bind to Toll-8 (Lavalou et al., 2020). The Toll-8-Cirl complex self-organizes to generate local asymmetric interfaces which are essential for planar polarizations of contractile interfaces. In addition to Toll-Rho signaling, the classical planar polarity system involving Frizzled and which mediates planar polarity in wings and eye imaginal discs may also be involved in germband extension (Yang and Mlodzik, 2015). Although Frizzled was reported to be enriched on vertical junctions during cell intercalation (Warrington et al., 2013), neither the Frizzled nor the major Wnt effector Disheveled appears to be required for germ-band extension (Zallen and Wieschaus, 2004; Warrington et al., 2013).
Dorsal Closure
Dorsal closure is another prominent morphogenetic process in Drosophila embryogenesis (Figures 2D–F) (Hayes and Solon, 2017; Kiehart et al., 2017). Dorsal closure involves two types of epithelial tissues and their coordination, i.e., the squamous amnioserosa and the columnar dorsal–lateral epidermis. After germband retraction, the extraembryonic amnioserosa bridges the left and right sheets of the dorsal epidermis (Figure 2E). Within about 4 h, the two lateral epidermal sheets on both sides of the embryo move toward the dorsal midline while the amnioserosa retreats and finally disappears (Figure 2D). The mechanical forces for the directed movement are provided from both tissues and their interface. The squamous amnioserosa cells display pulsatile isotropic contractions which lead to very regular oscillations of the cross-sectional area. On the tissue scale, the oscillations balance out each other due to their asynchrony during the stationary phase preceding dorsal closure. During dorsal closure, however, the contractions take over and lead to a gradual decrease of the total area of the amnioserosa. The decreasing area is compensated or promoted by the movement of the adjacent epidermis. Given several recent excellent reviews on the role of the amnioserosa cells (Hayes and Solon, 2017; Kiehart et al., 2017; Perez-Vale and Peifer, 2020), we will focus on the surrounding epidermis for the closure process in the following paragraphs.
The interface between the two tissues plays an important role. The dorsal-most epidermal cells, the leading edge cells, polarize by an accumulation of F-actin at the interface between their dorsal edge and the amnioserosa interface, which generates a prominent and contractile F-actin cable (Figure 2E) (Young et al., 1993; Kiehart et al., 2000). Meanwhile, the leading edge cells dramatically elongate along the DV direction as if they were pulled by the amnioserosa (Figure 2F) (Jacinto et al., 2002). This notion has remained untested. Both models are conceivable. In the passive model, the elongation of epidermal cells is due to pulling by the amnioserosa cell/actin cable contractions. In the active model, the epidermal cells elongate by an autonomous mechanism within the epidermis and thus generate a pushing force. A combination of both models would also be possible.
Tissue restricted Myo-II depletion in the amnioserosa or surrounding epidermis revealed that the Myo-II dependent contractions within the amnioserosa tissue but not actin cable are required for dorsal closure (Pasakarnis et al., 2016). However, the kinetics of the overall closure process appeared slower when Myo-II was depleted in the epidermis. Myo-II depletion in epidermis affects the contractility of all cells of the epidermis, not only the leading edge cells and the actin cable. Yet unidentified autonomous mechanisms could be affected within the epidermis. It is worth noting that Myo-II depletion specifically within the amnioserosa, also affected the actin cable structure (Pasakarnis et al., 2016). In these embryos, the actin cable initially formed but the cable structure disassembled partially during dorsal closure. These observations suggest a role of amnioserosa cell contractions for the cable structure. The elongation of epidermal cells might be due to pulling by the actin cable tension. The tension along the actin cable increases steadily over time, as revealed by the recoil velocity following UV laser-induced junction cutting (Saias et al., 2015). Opposing a role of the actin cable comes from the analysis of Zasp52 mutants embryos, which lack any actin cable but undergo an apparently normal dorsal closure (Ducuing and Vincent, 2016). Interestingly, the elongation of epidermal cells is still observed in Zasp52 mutants. These observations suggest that the elongation of epidermal cells is not only due to pulling by the actin cable.
Adherens Junctions at the Leading Edge Cells
Although the amnioserosa cells behave isotropically with respect to their oscillations, the cell junctions at the interface are polarized as seen not only by the actin cable but also by the junction and junction-associated proteins. The epidermis connects with amnioserosa cells via E-cad and integrin-mediated adhesions (Narasimha and Brown, 2004). Reduced E-cad levels impair cell contacts between leading edge cells and amnioserosa (Gorfinkiel and Arias, 2007). Correspondingly interface defects within the actin cable and edge cells of the amnioserosa were observed in α-catenin mutant embryos, in which the actin-binding domain was specifically deleted (Jurado et al., 2016). Further actin-binding proteins associated with adherens junctions were recently identified to localize at the interface. Although Canoe and Polychaetoid are not essential for the actin cable, the architecture and morphology of leading-edge cells were impaired in embryos depleted for those proteins (Manning et al., 2019). The Ajuba LIM protein (Jub), a force-sensitive protein, is enriched at the interface, and loss of Jub enhances dorsal closure defects in mutants defective for cell adhesion (Razzell et al., 2018). This protein accumulates at adherens junctions under tension and acts as a critical component of a negative-feedback loop, which stabilizes and distributes tension at adherens junctions at the interface (Rauskolb et al., 2019). These studies strongly suggest that adherens junctions have fundamental functions in adapting to mechanical forces and coordinate the tissue and cell interactions leading to morphogenesis.
Conclusion and Remarks
Within the lateral epidermis during gastrulation, the AP patterning system establishes a system of planar cell polarity, which polarizes junctional and cytoskeletal dynamics and subsequently directs cell rearrangement for the tissue-scale changes in morphology. The finding that members of the Toll-family of membrane receptors are involved in the polarization of the tissue has started to open the black box of molecular links between the transcriptional patterning machinery for axis formation and the cell biological machinery of contractile actomyosin clusters and cell adhesion complexes (Paré et al., 2014; Tetley et al., 2016; Lavalou et al., 2020). E-cad adhesion complexes are at the core of mechanical coordination between neighbors in epithelia. Its potential functions and the interactions with contractile actomyosin networks and other interaction partners provide ample options for fine-tuning sensory and signaling functions.
Yet missing is an integrative systems-type analysis involving mechanisms of coordination among the direct neighbors but also long-ranging influences to second and third neighbors. Analysis of the temporal and spatial coordination of the identified contractile and adhesive activities will be needed for the step from understanding the individual events such as a junction collapse to the tissue-scale shape changes during morphogenesis. Drosophila embryos provide a suitable and highly tractable system to study such questions in vivo.
Beyond the individual tissue, polarized and anisotropic tensions from the neighboring tissues have a potentially big impact on morphogenetic processes. The anisotropic tension by the posterior midgut during gastrulation pulls on the lateral epidermis, which is visible by a corresponding AP stretching of the cells during the onset of germband extension (Lye et al., 2015). This anisotropic tension with a gradual increase toward the posterior tip of the embryos transiently orientates newly formed junctions (Collinet et al., 2015). During germband extension cell stretching is diminished by cell rearrangement, even though the polarized tension remains on the tissue scale (Collinet et al., 2015; Lye et al., 2015). For a full understanding, it needs to be investigated whether and how E-Cad complexes and its interacting partners are involved in the coordination of local and tissue-scale forces during epithelium morphogenesis.
Similar tissue interactions are essential for the morphogenesis of the amnioserosa and dorsal closure. The two sheets of the dorsal epidermis are exposed to an anisotropic tension from the pulsating and contracting amnioserosa as well as the contractile actin cable. Cell elongation occurs not only in the leading edge cells but also in the further distant second and third and so forth neighbors in the epidermis (Figure 2F). It has remained unclear to which degree the elongation of the epidermal cells contributes to the closure process. How does the dorsal epidermis respond to and coordinate the polarized anisotropic tension with the cell shape changes? Adherens junctions and the binding proteins could be the potential candidates. For example, Arf-GEF Steppke is recruited to the myosin-rich adherens junction via coiled-coil heterodimerization with an adaptor protein, where the complex downregulates junctional tension and facilitates tissue stretching (West et al., 2017; Zheng et al., 2019). It is worth expanding the research focus from the amnioserosa and actin cable to the surrounding epidermis. As stated above the numerous proteins and processes associated with E-cad core complexes provide ample options for regulation and fine-tuning of morphogenetic processes.
Author Contributions
DK wrote the manuscript and drew the figures. JG revised the manuscript. JG and DK conceived the study and edited the manuscript. Both authors contributed to the article and approved the submitted version.
Funding
This work was supported by Deutsche Forschungsgemeinschaft (GR1945/10-1 and GR1945/10-2).
Conflict of Interest
The authors declare that the research was conducted in the absence of any commercial or financial relationships that could be construed as a potential conflict of interest.
References
Bertet, C., Sulak, L., and Lecuit, T. (2004). Myosin-dependent junction remodelling controls planar cell intercalation and axis elongation. Nature 429, 667–671. doi: 10.1038/nature02590
Blankenship, J. T., Backovic, S. T., Sanny, J. S., Weitz, O., and Zallen, J. A. (2006). Multicellular rosette formation links planar cell polarity to tissue morphogenesis. Dev. Cell 11, 459–470. doi: 10.1016/j.devcel.2006.09.007
Buckley, C. D., Tan, J., Anderson, K. L., Hanein, D., Volkmann, N., Weis, W. I., et al. (2014). Cell adhesion. The minimal cadherin-catenin complex binds to Actin filaments under force. Science 346:1254211. doi: 10.1126/science.1254211
Bulgakova, N. A., and Brown, N. H. (2016). Drosophila p120-catenin is crucial for endocytosis of the dynamic E-cadherin-Bazooka complex. J. Cell Sci. 129, 477–482. doi: 10.1242/jcs.177527
Cavey, M., Rauzi, M., Lenne, P. F., and Lecuit, T. (2008). A two-tiered mechanism for stabilization and immobilization of E-cadherin. Nature 453, 751–756. doi: 10.1038/nature06953
Charras, G., and Yap, A. S. (2018). Tensile forces and mechanotransduction at cell-cell junctions. Curr. Biol. 28, R445–R457.
Chen, Y. J., Huang, J., Huang, L., Austin, E., and Hong, Y. (2017). Phosphorylation potential of Drosophila E-Cadherin intracellular domain is essential for development and adherens junction biosynthetic dynamics regulation. Development 144, 1242–1248. doi: 10.1242/dev.141598
Choi, H. J., Pokutta, S., Cadwell, G. W., Bobkov, A. A., Bankston, L. A., Liddington, R. C., et al. (2012). αE-catenin is an autoinhibited molecule that Coactivates vinculin. Proc. Natl. Acad. Sci. U.S.A. 109, 8576–8581. doi: 10.1073/pnas.1203906109
Collinet, C., Rauzi, M., Lenne, P. F., and Lecuit, T. (2015). Local and tissue-scale forces drive oriented junction growth during tissue extension. Nat. Cell Biol. 17, 1247–1258. doi: 10.1038/ncb3226
Cox, R. T., Kirkpatrick, C., and Peifer, M. (1996). Armadillo is required for adherens junction assembly, cell polarity, and morphogenesis during Drosophila embryogenesis. J. Cell Biol. 134, 133–148. doi: 10.1083/jcb.134.1.133
De Las Bayonas, A. G., Philippe, J. M., Lellouch, A. C., and Lecuit, T. (2019). Distinct RhoGEFs activate apical and junctional contractility under control of G proteins during epithelial morphogenesis. Curr. Biol. 29, 3370–3385.e7.
de Matos Simões, M., Blankenship, J. T., Weitz, O., Farrell, D. L., Tamada, M., Fernandez-Gonzalez, R., et al. (2010). Rho-kinase directs Bazooka/Par-3 planar polarity during Drosophila axis elongation. Dev. Cell 19, 377–388. doi: 10.1016/j.devcel.2010.08.011
Ducuing, A., and Vincent, S. (2016). The actin cable is dispensable in directing dorsal closure dynamics but neutralizes mechanical stress to prevent scarring in the Drosophila embryo. Nat. Cell Biol. 18, 1149–1160. doi: 10.1038/ncb3421
Eldon, E., Kooyer, S., D’Evelyn, D., Duman, M., Lawinger, P., Botas, J., et al. (1994). The Drosophila 18 wheeler is required for morphogenesis and has striking similarities to Toll. Development 120, 885–899.
Engl, W., Arasi, B., Yap, L. L., Thiery, J. P., and Viasnoff, V. (2014). Actin dynamics modulate mechanosensitive immobilization of E-cadherin at adherens junctions. Nat. Cell Biol. 16, 587–594.
Fernandez-Gonzalez, R., Simoes Sde, M., Röper, J. C., Eaton, S., and Zallen, J. A. (2009). Myosin II dynamics are regulated by tension in intercalating cells. Dev. Cell 17, 736–743. doi: 10.1016/j.devcel.2009.09.003
Gorfinkiel, N., and Arias, A. M. (2007). Requirements for adherens junction components in the interaction between epithelial tissues during dorsal closure in Drosophila. J. Cell Sci. 120, 3289–3298. doi: 10.1242/jcs.010850
Harding, M. J., and Nechiporuk, A. V. (2012). Fgfr-Ras-MAPK signaling is required for apical constriction via apical positioning of Rho-associated kinase during mechanosensory organ formation. Development 139, 3130–3135. doi: 10.1242/dev.082271
Harris, T. J. (2012). Adherens junction assembly and function in the Drosophila embryo. Int. Rev. Cell Mol. Biol. 293, 45–83. doi: 10.1016/b978-0-12-394304-0.00007-5
Harris, T. J., and Peifer, M. (2004). Adherens junction-dependent and -independent steps in the establishment of epithelial cell polarity in Drosophila. J. Cell Biol. 167, 135–147. doi: 10.1083/jcb.200406024
Hartenstein, V. (1993). Atlas of Drosophila Development. Cold Spring Harbor, NJ: Cold Spring Harbor Laboratory Press.
Hartman, M. A., and Spudich, J. A. (2012). The myosin superfamily at a glance. J. Cell Sci. 125, 1627–1632. doi: 10.1242/jcs.094300
Hayes, P., and Solon, J. (2017). Drosophila dorsal closure: an orchestra of forces to zip shut the embryo. Mech. Dev. 144, 2–10. doi: 10.1016/j.mod.2016.12.005
Irvine, K. D., and Wieschaus, E. (1994). Cell intercalation during Drosophila germband extension and its regulation by pair-rule segmentation genes. Development 120, 827–841.
Ishiyama, N., Sarpal, R., Wood, M. N., Barrick, S. K., Nishikawa, T., Hayashi, H., et al. (2018). Force-dependent allostery of the α-catenin actin-binding domain controls adherens junction dynamics and functions. Nat. Commun. 9:5121.
Iyer, K. V., Piscitello-Gómez, R., Paijmans, J., Jülicher, F., and Eaton, S. (2019). Epithelial viscoelasticity is regulated by mechanosensitive E-cadherin turnover. Curr. Biol. 29, 578–591.e575.
Jacinto, A., Wood, W., Woolner, S., Hiley, C., Turner, L., Wilson, C., et al. (2002). Dynamic analysis of actin cable function during Drosophila dorsal closure. Curr. Biol. 12, 1245–1250. doi: 10.1016/s0960-9822(02)00955-7
Jurado, J., de Navascués, J., and Gorfinkiel, N. (2016). α-Catenin stabilizes cadherin-catenin complexes and modulates actomyosin dynamics to allow pulsatile apical contraction. J. Cell Sci. 129, 4496–4508. doi: 10.1242/jcs.193268
Kale, G. R., Yang, X., Philippe, J. M., Mani, M., Lenne, P. F., and Lecuit, T. (2018). Distinct contributions of tensile and shear stress on E-cadherin levels during morphogenesis. Nat. Commun. 9:5021.
Kambris, Z., Hoffmann, J. A., Imler, J.-L., and Capovilla, M. (2002). Tissue and stage-specific expression of the Tolls in Drosophila embryos. Gene Express. Patt. 2, 311–317. doi: 10.1016/s1567-133x(02)00020-0
Kerridge, S., Munjal, A., Philippe, J. M., Jha, A., de las Bayonas, A. G., Saurin, A. J., et al. (2016). Modular activation of Rho1 by GPCR signalling imparts polarized myosin II activation during morphogenesis. Nat. Cell Biol. 18, 261–270. doi: 10.1038/ncb3302
Kiehart, D. P., Crawford, J. M., Aristotelous, A., Venakides, S., and Edwards, G. S. (2017). Cell Sheet Morphogenesis: dorsal closure in Drosophila melanogaster as a model system. Annu. Rev. Cell Dev. Biol. 33, 169–202. doi: 10.1146/annurev-cellbio-111315-125357
Kiehart, D. P., Galbraith, C. G., Edwards, K. A., Rickoll, W. L., and Montague, R. A. (2000). Multiple forces contribute to cell sheet morphogenesis for dorsal closure in Drosophila. J. Cell Biol. 149, 471–490. doi: 10.1083/jcb.149.2.471
Kong, D., Lv, Z., Haring, M., Lin, B., Wolf, F., and Grosshans, J. (2019). In vivo optochemical control of cell contractility at single-cell resolution. EMBO Rep. 20:e47755.
Kong, D., Wolf, F., and Grosshans, J. (2017). Forces directing germ-band extension in Drosophila embryos. Mech. Dev. 144, 11–22. doi: 10.1016/j.mod.2016.12.001
Lavalou, J., Mao, Q., Harmansa, S., Kerridge, S., Lellouch, A. C., Philippe, J.-M., et al. (2020). Formation of polarized contractile interfaces by self-organized Toll-8/Cirl GPCR asymmetry. bioRxiv [Preprint], doi: 10.1101/2020.03.16.993758
Lecaudey, V., Cakan-Akdogan, G., Norton, W. H. J., and Gilmour, D. (2008). Dynamic Fgf signaling couples morphogenesis and migration in the Zebrafish lateral line primordium. Development 135, 2695–2705. doi: 10.1242/dev.025981
Leckband, D. E., and de Rooij, J. (2014). Cadherin adhesion and mechanotransduction. Annu. Rev. Cell Dev. Biol. 30, 291–315. doi: 10.1146/annurev-cellbio-100913-013212
Leptin, M. (1994). Morphogenesis. Control of epithelial cell shape changes. Curr. Biol. 4, 709–712. doi: 10.1016/s0960-9822(00)00156-1
Leptin, M. (2005). Gastrulation movements: the logic and the nuts and bolts. Dev. Cell 8, 305–320. doi: 10.1016/j.devcel.2005.02.007
Levayer, R., Pelissier-Monier, A., and Lecuit, T. (2011). Spatial regulation of Dia and Myosin-II by RhoGEF2 controls initiation of E-cadherin endocytosis during epithelial morphogenesis. Nat. Cell Biol. 13, 529–540. doi: 10.1038/ncb2224
Lv, Z., Lu, Q., and Dong, B. (2019). Morphogenesis: a focus on marine invertebrates. Mar. Life Sci. Technol. 1, 28–40. doi: 10.1007/s42995-019-00016-z
Lye, C. M., Blanchard, G. B., Naylor, H. W., Muresan, L., Huisken, J., Adams, R. J., et al. (2015). Mechanical coupling between endoderm invagination and axis extension in Drosophila. PLoS Biol. 13:e1002292. doi: 10.1371/journal.pbio.1002292
Lye, C. M., and Sanson, B. (2011). Tension and epithelial morphogenesis in Drosophila early embryos. Curr. Top. Dev. Biol. 95, 145–187. doi: 10.1016/b978-0-12-385065-2.00005-0
Maître, J. L., and Heisenberg, C. P. (2013). Three functions of cadherins in cell adhesion. Curr. Biol. 23, R626–R633.
Manning, L. A., Perez-Vale, K. Z., Schaefer, K. N., Sewell, M. T., and Peifer, M. (2019). The Drosophila Afadin and ZO-1 homologues canoe and polychaetoid act in parallel to maintain epithelial integrity when challenged by Adherens junction remodeling. Mol. Biol. Cell 30, 1938–1960. doi: 10.1091/mbc.e19-04-0209
Martin, A. C. (2020). The physical mechanisms of Drosophila gastrulation: mesoderm and endoderm invagination. Genetics 214, 543–560. doi: 10.1534/genetics.119.301292
Martin, A. C., Kaschube, M., and Wieschaus, E. F. (2009). Pulsed contractions of an actin-myosin network drive apical constriction. Nature 457, 495–499. doi: 10.1038/nature07522
Müller, H. A., and Wieschaus, E. (1996). armadillo, bazooka, and stardust are critical for early stages in formation of the zonula adherens and maintenance of the polarized blastoderm epithelium in Drosophila. J. Cell Biol. 134, 149–163. doi: 10.1083/jcb.134.1.149
Myster, S. H., Cavallo, R., Anderson, C. T., Fox, D. T., and Peifer, M. (2003). Drosophila p120catenin plays a supporting role in cell adhesion but is not an essential adherens junction component. J. Cell Biol. 160, 433–449. doi: 10.1083/jcb.200211083
Narasimha, M., and Brown, N. H. (2004). Novel Functions for Integrins in Epithelial Morphogenesis. Curr. Biol. 14, 381–385. doi: 10.1016/j.cub.2004.02.033
Nasiadka, A., Dietrich, B. H., and Krause, H. M. (2002). Anterior-posterior patterning in the Drosophila embryo. Adv. Dev. Biol. Biochem. 12, 155–204. doi: 10.1016/s1569-1799(02)12027-2
Nechiporuk, A., and Raible, D. W. (2008). FGF-dependent mechanosensory organ patterning in Zebrafish. Science 320, 1774–1777. doi: 10.1126/science.1156547
Nishiguchi, S., Yagi, A., Sakai, N., and Oda, H. (2016). Divergence of structural strategies for homophilic E-cadherin binding among bilaterians. J. Cell Sci. 129, 3309–3319. doi: 10.1242/jcs.189258
Oda, H., and Tsukita, S. (1999). Nonchordate classic cadherins have a structurally and functionally unique domain that is absent from chordate classic cadherins. Dev. Biol. 216, 406–422. doi: 10.1006/dbio.1999.9494
Oda, H., Uemura, T., Harada, Y., Iwai, Y., and Takeichi, M. (1994). A Drosophila homolog of cadherin associated with armadillo and essential for embryonic cell-cell adhesion. Dev. Biol. 165, 716–726. doi: 10.1006/dbio.1994.1287
Oda, H., Uemura, T., Shiomi, K., Nagafuchi, A., Tsukita, S., and Takeichi, M. (1993). Identification of a Drosophila homologue of alpha-catenin and its association with the armadillo protein. J. Cell Biol. 121, 1133–1140. doi: 10.1083/jcb.121.5.1133
Orsulic, S., and Peifer, M. (1996). An in vivo structure-function study of armadillo, the beta-catenin homologue, reveals both separate and overlapping regions of the protein required for cell adhesion and for wingless signaling. J. Cell Biol. 134, 1283–1300. doi: 10.1083/jcb.134.5.1283
Pai, L. M., Kirkpatrick, C., Blanton, J., Oda, H., Takeichi, M., and Peifer, M. (1996). Drosophila alpha-catenin and E-cadherin bind to distinct regions of Drosophila armadillo. J. Biol. Chem. 271, 32411–32420.
Paré, A. C., Vichas, A., Fincher, C. T., Mirman, Z., Farrell, D. L., Mainieri, A., et al. (2014). A positional Toll receptor code directs convergent extension in Drosophila. Nature 515, 523–527. doi: 10.1038/nature13953
Paré, A. C., and Zallen, J. A. (2020). Cellular, molecular, and biophysical control of epithelial cell intercalation. Curr. Top. Dev. Biol. 136, 167–193. doi: 10.1016/bs.ctdb.2019.11.014
Pasakarnis, L., Frei, E., Caussinus, E., Affolter, M., and Brunner, D. (2016). Amnioserosa cell constriction but not epidermal actin cable tension autonomously drives dorsal closure. Nat. Cell Biol. 18, 1161–1172. doi: 10.1038/ncb3420
Peifer, M., and Wieschaus, E. (1990). The segment polarity gene armadillo encodes a functionally modular protein that is the Drosophila homolog of human plakoglobin. Cell 63, 1167–1176. doi: 10.1016/0092-8674(90)90413-9
Perez-Vale, K. Z., and Peifer, M. (2020). Orchestrating morphogenesis: building the body plan by cell shape changes and movements. Development 147:dev191049. doi: 10.1242/dev.191049
Pokutta, S., Drees, F., Yamada, S., Nelson, W. J., and Weis, W. I. (2008). Biochemical and structural analysis of alpha-catenin in cell-cell contacts. Biochem. Soc. Trans. 36, 141–147. doi: 10.1042/bst0360141
Rangarajan, E. S., and Izard, T. (2012). The cytoskeletal protein α-catenin unfurls upon binding to vinculin. J. Biol. Chem. 287, 18492–18499. doi: 10.1074/jbc.m112.351023
Rauskolb, C., Cervantes, E., Madere, F., and Irvine, K. D. (2019). Organization and function of tension-dependent complexes at adherens junctions. J. Cell Sci. 132:jcs224063. doi: 10.1242/jcs.224063
Rauzi, M., Lenne, P. F., and Lecuit, T. (2010). Planar polarized actomyosin contractile flows control epithelial junction remodelling. Nature 468, 1110–1114. doi: 10.1038/nature09566
Rauzi, M., Verant, P., Lecuit, T., and Lenne, P. F. (2008). Nature and anisotropy of cortical forces orienting Drosophila tissue morphogenesis. Nat. Cell Biol. 10, 1401–1410. doi: 10.1038/ncb1798
Razzell, W., Bustillo, M. E., and Zallen, J. A. (2018). The force-sensitive protein Ajuba regulates cell adhesion during epithelial morphogenesis. J. Cell Biol. 217, 3715–3730. doi: 10.1083/jcb.201801171
Saias, L., Swoger, J., D’Angelo, A., Hayes, P., Colombelli, J., Sharpe, J., et al. (2015). Decrease in cell volume generates contractile forces driving dorsal closure. Dev. Cell 33, 611–621. doi: 10.1016/j.devcel.2015.03.016
Schmidt, A., and Grosshans, J. (2018). Dynamics of cortical domains in early Drosophila development. J. Cell Sci. 131:jcs212795.
Sharrock, T. E., and Sanson, B. (2020). Cell sorting and morphogenesis in early Drosophila embryos. Semin. Cell Dev. Biol. 107, 147–160. doi: 10.1016/j.semcdb.2020.07.010
Simões, S. M., Mainieri, A., and Zallen, J. A. (2014). Rho GTPase and Shroom direct planar polarized actomyosin contractility during convergent extension. J. Cell Biol. 204, 575–589. doi: 10.1083/jcb.201307070
Tepass, U., Gruszynski-DeFeo, E., Haag, T. A., Omatyar, L., Török, T., and Hartenstein, V. (1996). shotgun encodes Drosophila E-cadherin and is preferentially required during cell rearrangement in the neurectoderm and other morphogenetically active epithelia. Genes Dev. 10, 672–685. doi: 10.1101/gad.10.6.672
Tetley, R. J., Blanchard, G. B., Fletcher, A. G., Adams, R. J., and Sanson, B. (2016). Unipolar distributions of junctional myosin II identify cell stripe boundaries that drive cell intercalation throughout Drosophila axis extension. eLife 5:e12094.
Truong Quang, B. A., Mani, M., Markova, O., Lecuit, T., and Lenne, P. F. (2013). Principles of E-cadherin supramolecular organization in vivo. Curr. Biol. 23, 2197–2207. doi: 10.1016/j.cub.2013.09.015
Uemura, T., Oda, H., Kraut, R., Hayashi, S., Kotaoka, Y., and Takeichi, M. (1996). Zygotic Drosophila E-cadherin expression is required for processes of dynamic epithelial cell rearrangement in the Drosophila embryo. Genes Dev. 10, 659–671. doi: 10.1101/gad.10.6.659
Wang, Y. C., Khan, Z., Kaschube, M., and Wieschaus, E. F. (2012). Differential positioning of adherens junctions is associated with initiation of epithelial folding. Nature 484, 390–393. doi: 10.1038/nature10938
Warrington, S. J., Strutt, H., and Strutt, D. (2013). The Frizzled-dependent planar polarity pathway locally promotes E-cadherin turnover via recruitment of RhoGEF2. Development 140, 1045–1054. doi: 10.1242/dev.088724
Weaire, D., and Rivier, N. (1984). Soap, cells and statistics—random patterns in two dimensions. Contemp. Phys. 25, 59–99. doi: 10.1080/00107518408210979
West, J. J., Zulueta-Coarasa, T., Maier, J. A., Lee, D. M., Bruce, A. E. E., Fernandez-Gonzalez, R., et al. (2017). An actomyosin-Arf-GEF negative feedback loop for tissue elongation under stress. Curr. Biol. 27, 2260–2270.e2265.
Wu, Y., Kanchanawong, P., and Zaidel-Bar, R. (2015). Actin-delimited adhesion-independent clustering of E-cadherin forms the nanoscale building blocks of adherens junctions. Dev. Cell 32, 139–154. doi: 10.1016/j.devcel.2014.12.003
Yang, Y., and Mlodzik, M. (2015). Wnt-Frizzled/planar cell polarity signaling: cellular orientation by facing the wind (Wnt). Annu. Rev. Cell Dev. Biol. 31, 623–646. doi: 10.1146/annurev-cellbio-100814-125315
Yao, M., Qiu, W., Liu, R., Efremov, A. K., Cong, P., Seddiki, R., et al. (2014). Force-dependent conformational switch of α-catenin controls vinculin binding. Nat. Commun. 5:4525.
Young, P. E., Richman, A. M., Ketchum, A. S., and Kiehart, D. P. (1993). Morphogenesis in Drosophila requires nonmuscle myosin heavy chain function. Genes Dev. 7, 29–41. doi: 10.1101/gad.7.1.29
Zallen, J. A., and Wieschaus, E. (2004). Patterned gene expression directs bipolar planar polarity in Drosophila. Dev. Cell 6, 343–355. doi: 10.1016/s1534-5807(04)00060-7
Zhang, Y., Kong, D., Reichl, L., Vogt, N., Wolf, F., and Großhans, J. (2014). The glucosyltransferase Xiantuan of the endoplasmic reticulum specifically affects E-Cadherin expression and is required for gastrulation movements in Drosophila. Dev. Biol. 390, 208–220. doi: 10.1016/j.ydbio.2014.03.007
Keywords: Drosophila embryonic epithelium, DE-cadherin, planar polarity, non-muscle myosin-II, tissue-scale shape changes
Citation: Kong D and Großhans J (2020) Planar Cell Polarity and E-Cadherin in Tissue-Scale Shape Changes in Drosophila Embryos. Front. Cell Dev. Biol. 8:619958. doi: 10.3389/fcell.2020.619958
Received: 21 October 2020; Accepted: 07 December 2020;
Published: 23 December 2020.
Edited by:
Yi Wu, UCONN Health, United StatesReviewed by:
Jun Zhou, German Cancer Research Center (DKFZ), GermanyAna Carmena, Consejo Superior de Investigaciones Científicas (CSIC), Spain
Copyright © 2020 Kong and Großhans. This is an open-access article distributed under the terms of the Creative Commons Attribution License (CC BY). The use, distribution or reproduction in other forums is permitted, provided the original author(s) and the copyright owner(s) are credited and that the original publication in this journal is cited, in accordance with accepted academic practice. No use, distribution or reproduction is permitted which does not comply with these terms.
*Correspondence: Deqing Kong, ZGVxaW5nLmtvbmdAYmlvbG9naWUudW5pLW1hcmJ1cmcuZGU=