- 1School of Life Sciences, Tsinghua University, Beijing, China
- 2Tsinghua-Peking Center for Life Sciences, Beijing, China
In the secretory pathway, the transfer of cargo from the ER to the Golgi involves dozens of proteins that localize at specific regions of the ER called ER exit sites (ERES), where cargos are concentrated preceding vesicular transport to the Golgi. Despite many years of research, we are missing crucial details of how this highly dynamic ER-Golgi interface is defined, maintained and functions. Mechanisms allowing secretion of large cargos such as the very abundant collagens are also poorly understood. In this context, Tango1, discovered in the fruit fly Drosophila and widely conserved in animal evolution, has received a lot of attention in recent years. Tango1, an ERES-localized transmembrane protein, is the single fly member of the MIA/cTAGE family, consisting in humans of TANGO1 and at least 14 different related proteins. After its discovery in flies, a specific role of human TANGO1 in mediating secretion of collagens was reported. However, multiple studies in Drosophila have demonstrated that Tango1 is required for secretion of all cargos. At all ERES, through self-interaction and interactions with other proteins, Tango1 aids ERES maintenance and tethering of post-ER membranes. In this review, we discuss discoveries on Drosophila Tango1 and put them in relation with research on human MIA/cTAGE proteins. In doing so, we aim to offer an integrated view of Tango1 function and the nature of ER-Golgi transport from an evolutionary perspective.
Introduction
The endoplasmic reticulum (ER) is the largest and most versatile organelle in eukaryotic cells, capable of generating, contacting and establishing coordinated relations with all other organelles and subcellular compartments. Among such relations, the one between the ER and the Golgi apparatus was the earliest to be recognized (Palade, 1975). ER-Golgi exchanges concentrate at ER exit sites (ERES): specialized ER regions where secretory cargos are collected prior to their trafficking to the Golgi (Bannykh et al., 1996). While dozens of proteins are required for exit of secretory cargo from the ER and entry into the Golgi, few are known to be involved in post-Golgi traffic (Lee et al., 2004; Barlowe and Miller, 2013), a clear indication that ER-to-Golgi cargo transfer at the ER-Golgi interface is the most critical step in secretion. In these same narrow ERES regions, in addition, traffic in the reverse Golgi-to-ER direction takes place as well (Roy Chowdhury et al., 2020). Despite their highly dynamic underlying nature, ERES are overall stable entities, raising the question of how they persist through time. Due to the high concentration of traffic regulators and proteins of the vesicle budding and fusion machineries, it has been postulated that ERES may behave as membrane-less liquid droplets (Hanna et al., 2018). Against this background, Tango1 (Transport and Golgi Organization 1), an ERES-localized protein discovered in the fruit fly Drosophila, has captured a great deal of interest for its role in ERES-Golgi coordination.
Tango1 is a transmembrane protein of the MIA/cTAGE (Melanoma Inhibitory Activity/Cutaneous T-cell lymphoma-associated antiGEn) family (Figure 1; Usener et al., 2003; Malhotra and Erlmann, 2011). Members of this family were found to be upregulated in melanoma samples before a role in secretion had been described (Bosserhoff et al., 1997; Stoll et al., 2001). The ER-lumenal portions of Tango1 and human TANGO1, encoded by MIA3, contain an SH3 domain, typically mediating protein-protein interactions in the cytoplasm, but exceptional in an ER-resident or secreted protein (Stoll and Bosserhoff, 2008). Their cytoplasmic portions, in turn, display conserved regions with no recognizable domains, but presumed to organize as coiled coils, and a C-terminal region that is poorly conserved but proline-rich, with multiple occurrences of consecutive prolines (Saito et al., 2009). Apart from MIA3, the human genome possesses 10 additional genes encoding MIA/cTAGE proteins. Among these, TANGO1 and TANGO1-like (TALI), encoded by MIA2, most resemble Drosophila Tango1. The rest contain exclusively the SH3 domain and ER-lumenal portion, or the cytoplasmic portion, presumably membrane-anchored in most cases.
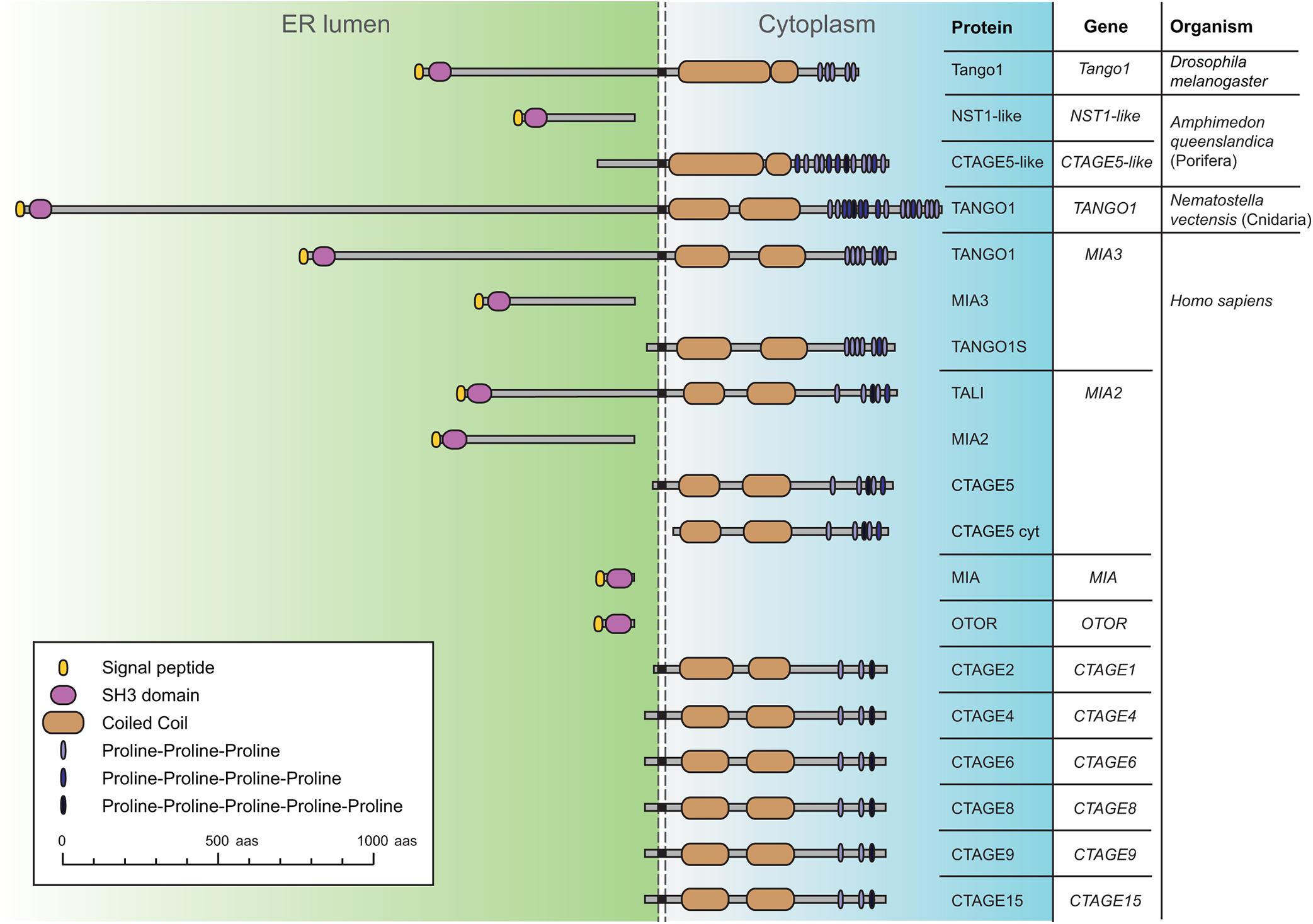
Figure 1. Schematic representation of Drosophila Tango1 and proteins of the MIA/cTAGE family, found exclusively in animals. A single representative is found in flies, consisting of an ER-lumenal part, displaying a highly conserved SH3 domain, and a cytoplasmic part, containing coiled coils and a C-terminal proline-rich region. In the sponge Amphimedon queenslandica, a representative of the phylum Porifera, the most basal metazoans, ER lumenal and cytoplasmic parts are found as separate proteins. In the sea anemone Nematostella vectensis, a cnidarian, a complete TANGO1 protein is found. Human MIA/cTAGE family members include TANGO1 and TANGO1-like (TALI) as complete proteins, but also ER-lumenal and transmembrane cytoplasmic forms, and also at least one predicted completely cytoplasmic CTAGE5 isoform (CTAGE5 cyt). Sequences represented and corresponding RefSeq accession numbers are: Dmel Tango1 (NP_609058.2), Aque NST1-like (XP_019849291.1), Aque CTAGE5-like (XP_019849290.1), Nvec TANGO1 (XP_032221684.1), Hsap TANGO1 (NP_940953.2), Hsap_MIA3 (XP_937620.1), Hsap_TANGO1S (NP_001287796.1), Hsap TALI (NP_001316143.1), Hsap MIA2 (NP_473365.3), Hsap CTAGE5 (NP_976231.1), Hsap CTAGE5cyt (XP_011535087.1), Hsap MIA (NP_001189482.1), Hsap OTOR (NP_064542.1), Hsap CTAGE2 (NP_758441.2), Hsap CTAGE4 (NP_940897.2), Hsap CTAGE6 (NP_848656.2), Hsap CTAGE8 (NP_001265436.1), Hsap CTAGE9 (NP_001139131.1), and Hsap CTAGE15 (NP_001008747.1).
Yeast has provided for many years a genetically amenable system for studying secretion. Genetic screenings in Saccharomyces cerevisiae have identified many secretory genes (Schekman, 2010; Barlowe and Miller, 2013). Drosophila, however, is increasingly becoming an excellent alternative to investigate secretion in a higher eukaryote. Most proteins known to play roles in secretion have fly homologues, including COPI and COPII components, Rab GTPases, SNAREs, TRAPP complex, p24 and Golgins (Kondylis and Rabouille, 2009; Muschalik and Munro, 2018). Another advantage of Drosophila is the availability of sophisticated genetic tools allowing tagging of endogenous proteins, forward genetic screening and complex loss- and gain-of function experiments that can evaluate physiological readouts and functional significance in real tissue and whole-animal contexts. An apparent difference in secretory pathway organization between Drosophila and humans is that in vertebrates ERES-derived vesicles fuse to form an ER-Golgi intermediate compartment (ERGIC) where cargo transits to a single juxtanuclear Golgi ribbon. In flies, like in non-vertebrate animals and plants (Glick and Nakano, 2009; Brandizzi and Barlowe, 2013), Golgi elements remain dispersed throughout the cytoplasm in close proximity to ERES, forming ERES-Golgi units (Ripoche et al., 1994; Kondylis et al., 2011). In the reduced space between Drosophila ERES and cis-Golgi, nonetheless, electron microscopy studies have shown the existence of pleiomorphic elements (Kondylis et al., 2005), revealing high complexity of this interface and a possible relation with the vertebrate ERGIC. Drosophila, finally, provides a very distinct benefit when compared to mammals in the form of limited gene redundancy. For instance, and in contrast with the enormous complexity of the human family, only one MIA/cTAGE protein exists in Drosophila, making flies an ideal system to address its function.
Discovery of Tango1 in Drosophila
A secretory function for Drosophila Tango1 (Figure 2A) was first uncovered in a genome-wide RNAi screening for genes required for secretion in Drosophila S2 cells (Bard et al., 2006), derived from embryonic macrophages. This screening selected genes for which depletion prevented secretion of ss-HRP (horseradish peroxidase with a signal sequence). A Tango1-V5 fusion, in addition, localized close to mid-Golgi marker ManII-GFP when expressed in S2 cells (Bard et al., 2006), consistent with ERES localization (Figure 2B). Gene hits in this screening that had not been characterized previously in Drosophila were named Tango1 to Tango14, for Transport and Golgi organization, representing a hypothetical class of animal-specific secretory genes that screenings in yeast could not have uncovered. Most of them retain to this date this Tango denomination in their official names, although the only one for which a direct involvement in secretion has been established is Tango1. In a second S2 cell RNAi screening, Tango1 was confirmed to be required for secretion, in this case of a secreted luciferase reporter, and ERES location of its product was observed again (Wendler et al., 2010). RNAi libraries used in the two screenings contained approximately 22,000 double stranded RNAs each and covered close to 100% of Drosophila protein-coding genes. Excluding hits nowadays mapping to non-coding or to multiple protein-coding genes, hit lists in the two screenings contained 240 (Bard et al., 2006) and 292 genes (Wendler et al., 2010). Of note, overlap of these two sets of genes, obtained using the same cell line and similar screening systems, was only 24 genes (Ke et al., 2018), which makes it the more remarkable that Tango1 was selected in both.
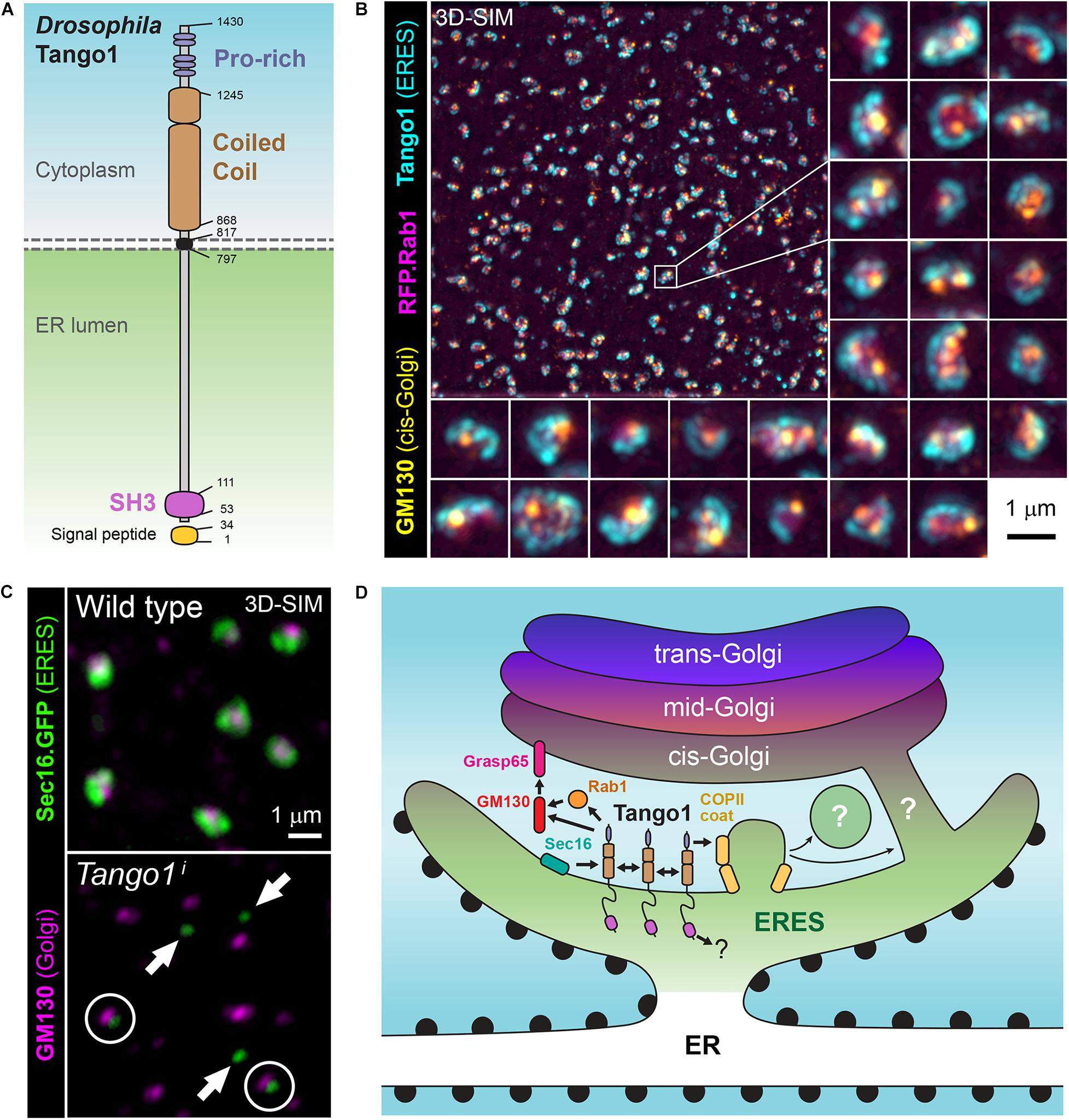
Figure 2. (A) Schematic representation of Drosophila Tango1. (B) Superresolution micrograph (3D-SIM) showing the localization of Tango1 (antibody staining) at ER exit sites (ERES) in a Drosophila fat body cell. RFP.Rab1 and cis-Golgi marker GM130 are shown as well. (C) Tango1 preserves ERES size and connection ERES-Golgi. Tango1 knock-down (Tango1i) causes decrease in the size of ERES, marked with Sec16.GFP, and their uncoupling from Golgi (cis-Golgi marker GM130). (D) Role of Drosophila Tango1 in defining ERES, tethering Golgi membranes and stabilizing the ER-Golgi interface at ERES-Golgi units. Interactions with itself, Grasp65, GM130, Rab1 and Sar1 have been documented (Liu et al., 2017). Additionally, human TANGO1 and other MIA/cTAGE proteins have been collectively found to interact with multiple ERES proteins and ER-Golgi traffic regulators.
A Collagen Specific Receptor?
After its discovery in flies, human TANGO1 was implicated in the transport of collagens from ERES to Golgi (Saito et al., 2009). At ERES, Golgi-bound, cargo-carrying vesicles are generated by the COPII complex, a set of proteins highly conserved in eukaryotes (Jensen and Schekman, 2011). Structural studies have shown that budding of COPII vesicles is mediated by the assembly of a vesicle-enclosing cage of 60–80 nm in diameter. In animals, many secreted proteins exceed these dimensions and, yet, they are efficiently secreted, raising questions on how this happens and whether specific mechanisms evolved in animals for secretion of large cargos (Fromme and Schekman, 2005). Examples of large secreted proteins include collagens, the main components of animal extracellular matrices, for which trimers assemble in the ER into 300–400 nm long semi-inflexible rods (Canty and Kadler, 2005), but also much larger proteins and protein complexes, such as lipoprotein particles and giant cuticular proteins of insects. Collagen-specific factors creating enlarged transporting vesicles have been postulated, TANGO1 among them (Malhotra and Erlmann, 2015).
TANGO1 silencing in human cells was reported to affect secretion of Collagen VII, but not of other types of collagen or other secreted proteins (Saito et al., 2009; Tanabe et al., 2016). The proline-rich region at the C-terminus of TANGO1 was shown to interact with the COPII coat, while its SH3 domain was described to bind specifically Collagen VII in the ER lumen (Saito et al., 2009). Soon after, however, MIA3 knock-out mice were revealed to exhibit defects in secretion of multiple collagens (Wilson et al., 2011), while Drosophila Tango1 was required for secretion of basement membrane Collagen IV (Pastor-Pareja and Xu, 2011). Activities of TANGO1 in both retarding COPII coat assembly and recruiting ERGIC membranes to nascent vesicles have been proposed as mechanisms by which TANGO1 could mediate formation of enlarged vesicles capable of transporting collagens (Malhotra and Erlmann, 2015; Santos et al., 2015). In this way, TANGO1 would collect collagen at ERES as a specific receptor while at the same time ensuring that a large enough vesicle grows to package it. Additional studies described specific requirements of MIA/cTAGE proteins CTAGE5 and TANGO1S in collagen secretion, and of TALI for secretion of ApoB-containing lipoparticles (Saito et al., 2011, 2014; Maeda et al., 2016; Santos et al., 2016; Tanabe et al., 2016). Other factors reported to affect secretion of collagen specifically were TRAPP complex component Sedlin (Venditti et al., 2012), ubiquitination of Sec31 by ubiquitin ligase KLHL12 (Jin et al., 2012), Syntaxin 18 and SNARE regulator Sly1 (Nogueira et al., 2014). Together with TANGO1 and other MIA/cTAGE proteins, they would be at the core of a collagen-specific, or large protein-specific, secretion pathway.
Not a Collagen Specific Receptor
Besides its advantages for secretion studies, Drosophila is a convenient model to investigate the biology of collagen and the extracellular matrix (Pastor-Pareja, 2020). Compared to the 28 types found in mammals, Drosophila possesses a reduced complement of collagens, consisting of basement membrane Collagen IV and Collagen XV/XVIII Multiplexin (Hynes and Zhao, 2000). Multiplexin expression, restricted to heart and nervous system, is dispensable for viability (Meyer and Moussian, 2009). Collagen IV, in contrast, is essential and abundantly present in most tissues. In flies, like in all animals, Collagen IV is the main component of basement membranes: polymers of extracellular matrix proteins that underlie epithelia and provide structural tissue support (Yurchenco, 2011). Drosophila Collagen IV is a 450 nm-long heterotrimer composed of α1 chain Collagen at 25C (Cg25C) and α2 chain Viking (Vkg) (Natzle et al., 1982; Lunstrum et al., 1988; Fessler and Fessler, 1989). In the larva, the main source of Collagen IV is fat body adipocytes (Pastor-Pareja and Xu, 2011), known for their role in lipid storage, but also as a very active secretory tissue that produces the serum proteins and clotting factors present in the hemolymph (insect blood). Besides Collagen IV, the other main basement membranes components are Laminin, Nidogen and Perlecan, all conserved from flies to humans as well.
Drosophila Tango1 has been repeatedly shown to be required for Collagen IV secretion (Pastor-Pareja and Xu, 2011; Lerner et al., 2013; Isabella and Horne-Badovinac, 2015; Zang et al., 2015; Ke et al., 2018; Sun et al., 2019). However, it was also found that Tango1 loss causes retention of other matrix proteins, such as Perlecan in ovarian follicle cells (Lerner et al., 2013), Papilin in the proventriculus (Zhang et al., 2014), BM-40-SPARC in blood cells (Tiwari et al., 2015) and Laminin in glia (Petley-Ragan et al., 2016). Our laboratory, therefore, decided to carry out a comprehensive analysis of the requirement of Tango1 in secretion (Liu et al., 2017). In this study, Tango1 loss in fat body led to ER retention of not just Collagen IV, but all monitored cargos, including general secretion markers VSVG and secreted GFP (GFP with a signal sequence). Furthermore, Tango1 is expressed in all cell types of the larva and localizes to all ERES, inconsistent with a collagen-specific or large-protein specific role (Liu et al., 2017). Highest expression of Tango1 was found in the salivary gland, a dedicated secretory organ where Tango1 is required for glue secretion (Liu et al., 2017) and secretory genes are highly expressed as a group (Abrams and Andrew, 2005). In all assayed tissues, Tango1 maintains the size and integrity of ERES-Golgi units, as ERES decrease in size and uncouple from Golgi upon Tango1 loss (Figure 2C), whereas Tango1 overexpression, conversely, created more and larger ERES (Liu et al., 2017). Furthermore, supporting an organizing function of Tango1 at the ERES-Golgi interface, the cytoplasmic part of Tango1 could rescue Tango1 loss in the fat body (Liu et al., 2017). Consistent with a general secretion role, Tango1 is needed for secretion of both large and small cargos in terminal cells of the tracheal system (Rios-Barrera et al., 2017), and for secretion of mucins by the male accessory gland and female spermatheca (Reynolds et al., 2019). In all, multiple phenotypic studies demonstrate that Tango1 is not specifically needed for transport of collagens, matrix or large proteins. It is, in contrast, required for general secretion at all ERES, where it maintains ERES size and proximity to Golgi.
The lack of a collagen-specific secretion pathway is further hinted by the results of an RNAi screening in Drosophila larval fat body (Ke et al., 2018). This screening, targeting 6,200 genes (about 60% of the protein-coding genome), found 88 genes for which silencing caused intracellular Collagen IV accumulation. Secondary screenings with additional secretory markers revealed that enzymes Prolyl-4-hydroxylase PH4αEFB and Lysyl-oxidase Plod, known to modify collagen post-translationally (Bunt et al., 2011; Pastor-Pareja and Xu, 2011), were the only hits affecting Collagen IV secretion specifically. All other hits affected general secretion, suggesting that collagen secretion does not use devoted mechanisms of vesicular transport. Of the 88 hits in this screening, only 15 were found in the previous S2 cell screenings, with just 7 hits at the intersection of all three: garz, Rab1, Use1, Slh, Syx18, Sec23, and Sec20 (Bard et al., 2006; Wendler et al., 2010; Ke et al., 2018). The little overlap between the two S2 cell screenings makes it difficult to draw conclusions. Nonetheless, a significant portion of hits in the fat body screening (32 out of 88) encode proteins known to be involved in traffic, including COPII and COPI proteins, SNAREs, TRAPP components, Grasp65, Rab1 and Rab-GTPase regulators (Ke et al., 2018). Remarkably, this screening was able to select known secretion components that neither of the two S2 cell screenings caught, such as Nsf2, Trs23, Trs33, Rep, Grasp65, and Loj (p24), as well as Zn transporter Catsup and SERCA, for which other studies have demonstrated secretory functions (Groth et al., 2013; Suisse and Treisman, 2019). By assaying secretion of an abundant endogenous protein in live animals, this screening may have led to the identification of novel secretory pathway components awaiting characterization. As for collagen secretion, the outcome of the screening points to the absence of a collagen-specific pathway. The requirement of Drosophila Tango1 in all secretory contexts, in turn, strongly suggests that human MIA/cTAGE5 proteins act redundantly to maintain ERES and facilitate general secretion. Nonetheless, the possibility remains that the general function of Tango1 in organizing ERES is separate from a specific function in mediating secretion of collagen or other cargos (Rios-Barrera et al., 2017). Indeed, Drosophila Tango1 loss seems to affect differentially secretion of Collagen IV when comparing its effects with those of Sec23 and Sar1 knock down on secretion of Collagen IV and secreted GFP (Liu et al., 2017). Whether these differential effects stem from true cargo binding specificity or from size-dependent quantitative differences in transport mode (vesicular vs. tubular carriers?) is currently one of the most pressing questions in the field.
Mechanisms of Tango1 Function
The function of Tango1 as a stabilizer of the ER-Golgi interface is thought to involve interactions with multiple other proteins (Figure 2D). Through its cytoplasmic part, Drosophila Tango1 has been shown to self-interact (Liu et al., 2017). This interaction maps to one of the coiled coil motifs (Reynolds et al., 2019). Importantly, a fully cytoplasmic version of Tango1 correctly localizes to ERES, showing that this portion of the protein is sufficient for localization (Liu et al., 2017). The cytoplasmic portions of human CTAGE5 and TANGO1 had been shown to interact before (Saito et al., 2011), and it is known as well that, similar to Drosophila Tango1, human TANGO1 can self-interact (Raote et al., 2018, 2020). Besides self-interaction and interaction with other MIA/cTAGE family members, TANGO1 and related proteins have been shown to bind multiple other proteins present at ERES, such as COPII coat protein SEC23 (Saito et al., 2009; Ma and Goldberg, 2016), SNARE regulator SLY (Nogueira et al., 2014), and ERES determinants SEC16 (Maeda et al., 2017) and SEC12 (Saito et al., 2014). Additionally, Drosophila Tango1 co-immunoprecipitates with Rab-GTPase Rab1 and cis-Golgi proteins Grasp65 and GM130 (Liu et al., 2017). Tango1, therefore, through its self-interaction and interaction with other proteins may act as a ER-Golgi interface stabilizer, contributing to concentrating and maintaining localization of all these proteins in a specific region of the ER and to tether post-ER membranes to ERES: Golgi directly in the case of Drosophila and ERGIC in vertebrates.
The role of the ER-lumenal part of Tango1, displaying a highly conserved SH3 domain, is still elusive. It has been shown that glycosylation of Drosophila Tango1 in the ER lumen prevents cleavage by Furin of this part of the protein (Zhang et al., 2014), suggestive of a proteolytic mechanism in the regulation of Tango1 function for which further details remain to be ascertained. Because Furin localizes to the trans-Golgi network and the endosomal compartments (Thomas, 2002), such cleavage is likely to occur there rather than at ERES, where most Tango1 is found due in part to recycling of the protein from the Golgi (Yuan et al., 2018). A role for the SH3 domain in binding cargos has been proposed. The SH3 domain in human TANGO1 was first reported to bind specifically Collagen VII, but not other collagens (Saito et al., 2009). Later, it was postulated that the SH3 domain could bind HSP47, a collagen chaperone, instead of collagens doing it directly (Ishikawa et al., 2016). Binding of TANGO1 to HSP47, containing an RDEL retrieval signal, would additionally ensure Golgi-to-ER recycling of TANGO1 via KDELR-mediated retrograde transport (Yuan et al., 2018). HSP47, also known as SERPINH1, is a Serpin rather than a typical chaperone. Serpin family members, of which 29 exist in Drosophila (Garrett et al., 2009), are highly specific protease inhibitors, some of them secreted proteins themselves, with diverse roles in the immune response. It has been claimed that Spn28F, lacking any RDEL or other putative ER retention motif, is the Drosophila homolog of HSP47/SERPINH1 (Sepulveda et al., 2018). However a phylogenetic analysis found no HSP47 orthologs outside of vertebrates (Kumar et al., 2017). A recent study, in addition, found HSP47 was absent from collagen carriers in human cells (Omari et al., 2020). Therefore, the relation between HSP47 and TANGO1, and, more generally, the role of the SH3 domain of Tango1, are still unclear.
What We Still Do Not Know About Tango1 and Collagen Secretion
Overwhelming evidence in Drosophila shows that Tango1 is required for general secretion. However, the question of whether collagen secretion requires any specific factors or uses a mode of ER-Golgi transport essentially different from the one other secreted proteins employ remains open. Besides TANGO1 and MIA/cTAGE proteins, loss of other factors of the general secretion machinery of eukaryotes have been linked to specific defects in collagen secretion in mammals (Boyadjiev et al., 2006; Jin et al., 2012; Venditti et al., 2012; Nogueira et al., 2014). An ER transmembrane protein, TMEM131, has been recently implicated in collagen secretion in C. elegans, Drosophila and human cells (Zhang et al., 2020), but a requirement for this protein in general secretion or ER homeostasis remains to be tested. It is possible that secretion of collagen and large cargo requires no specific factors, but imposes higher demands on the common secretory machinery. Because collagens are not just very large, but also very abundant (30% of the protein mass of the human body) mild general secretion defects may first become manifest in the form of intracellular collagen retention.
If not a collagen or large cargo receptor, what is the main role of Tango1? Tango1 could have evolved in animals to add stability to ERES, but it is unlikely to be the main upstream factor in an ERES determination cascade. A master role in ERES determination would correspond to Sec16 and Sec12, proteins that, unlike Tango1, are widely conserved from yeast to humans (Glick, 2014; Sprangers and Rabouille, 2015). Recently, it has been shown that phosphorylation of human TANGO1 at its C-terminus regulates ERES disassembly during mitosis (Maeda et al., 2020). However, the putative phosphorylation sites, conserved in vertebrates, do not seem conserved in Drosophila. TANGO1 and CTAGE5 have been shown to interact with both SEC16 and SEC12, and thus may cooperate with them in defining ERES through their multiple cytoplasmic interactions (Saito et al., 2014; Maeda et al., 2017). It remains to be seen if any of these interactions is a key high-affinity evolved interaction or rather Tango1 is a generally “sticky” protein ensuring tethering of post-ER membranes and concentration of many different factors at ERES. Interestingly, C. elegans has no Tango1 homolog (Erives, 2015), but contains TFG (Witte et al., 2011), absent in flies, which has been proposed to form oligomers that physically join ERES and ERGIC (Johnson et al., 2015; McCaughey et al., 2016). In this evolutionary context, therefore, alternative mechanisms may exist in animal cells to increase stability of ERES and capacity of ER-to-Golgi transport in terms of both cargo size and amount of cargo to be secreted. Unlike TFG, however, Tango1 is a transmembrane protein, and understanding the role of its ER-lumenal SH3 domain, its most conserved part, should be key for fully understanding its function.
Regardless of the specific role of Tango1 in maintaining ERES, the topology of the ER-Golgi interface and the nature of carrier membranes allowing efficient secretion of collagens and other large proteins are hotly debated (Mironov and Beznoussenko, 2019). Transport must involve COPII coated membranes, but these, logically, cannot be regular-sized COPII vesicles. Conflicting studies describe existence or absence of large vesicular carriers transporting collagen (Santos et al., 2015; Gorur et al., 2017; Raote et al., 2017; Yuan et al., 2018; McCaughey et al., 2019; Melville et al., 2019; Matsui et al., 2020; Omari et al., 2020). To be able to visualize collagen transport from ERES in cells, these studies prevent collagen trimerization through ascorbate depletion, followed by ascorbate readministration, which triggers resumption of transport. All these reports, consequently, should be taken with caution, as collagen monomers can still be secreted by cells (Pastor-Pareja and Xu, 2011). What is more, ER-phagy results in such situation (Omari et al., 2018), further complicating interpretation of structures formed under these conditions. The logical alternative to large vesicular carriers as mediators of collagen transport is direct ER-Golgi connection, suggested to occur in yeast (Kurokawa et al., 2014) and plants (daSilva et al., 2004), where ERES and Golgi are closely juxtaposed like in Drosophila and, probably, attached physically (Sparkes et al., 2009). ERES-ERGIC contact has been proposed as a transport mechanism in mammals as well (Malhotra and Erlmann, 2015; Raote and Malhotra, 2019). Given the narrow space in which Drosophila ERES-Golgi transport takes place, COPII-coated buds may frequently start fusion with cis-Golgi before separating from ERES, thus creating intermittent tubular connections through which all large cargos and most other cargos could reach the Golgi in flies and the ERGIC in vertebrates. Confirmation of this may require electron microscopy analysis of the ER-Golgi frontier, as light microscopy has not been able so far to provide sufficient resolution to settle arguments.
Author Contributions
All authors wrote the manuscript.
Funding
Work on secretion in our laboratory was supported by grants 91854207 and 31771600 from the Natural Science Foundation of China.
Conflict of Interest
The authors declare that the research was conducted in the absence of any commercial or financial relationships that could be construed as a potential conflict of interest.
References
Abrams, E. W., and Andrew, D. J. (2005). CrebA regulates secretory activity in the Drosophila salivary gland and epidermis. Development 132, 2743–2758. doi: 10.1242/dev.01863
Bannykh, S. I., Rowe, T., and Balch, W. E. (1996). The organization of endoplasmic reticulum export complexes. J. Cell Biol. 135, 19–35. doi: 10.1083/jcb.135.1.19
Bard, F., Casano, L., Mallabiabarrena, A., Wallace, E., Saito, K., Kitayama, H., et al. (2006). Functional genomics reveals genes involved in protein secretion and golgi organization. Nature 439, 604–607. doi: 10.1038/nature04377
Barlowe, C. K., and Miller, E. A. (2013). Secretory protein biogenesis and traffic in the early secretory pathway. Genetics 193, 383–410. doi: 10.1534/genetics.112.142810
Bosserhoff, A. K., Kondo, S., Moser, M., Dietz, U. H., Copeland, N. G., Gilbert, D. J., et al. (1997). Mouse CD-RAP/MIA gene: structure, chromosomal localization, and expression in cartilage and chondrosarcoma. Dev. Dyn. 208, 516–525. doi: 10.1002/(SICI)1097-0177(199704)208:4<516::AID-AJA7>3.0.CO;2-L
Boyadjiev, S. A., Fromme, J. C., Ben, J., Chong, S. S., Nauta, C., Hur, D. J., et al. (2006). Cranio-lenticulo-sutural dysplasia is caused by a SEC23A mutation leading to abnormal endoplasmic-reticulum-to-Golgi trafficking. Nat. Genet. 38, 1192–1197. doi: 10.1038/ng1876
Brandizzi, F., and Barlowe, C. (2013). Organization of the ER-Golgi interface for membrane traffic control. Nat. Rev. Mol. Cell Biol. 14, 382–392. doi: 10.1038/nrm3588
Bunt, S., Denholm, B., and Skaer, H. (2011). Characterisation of the drosophila procollagen lysyl hydroxylase, dPlod. Gene. Expr. Patterns 11, 72–78. doi: 10.1016/j.gep.2010.09.006
Canty, E. G., and Kadler, K. E. (2005). Procollagen trafficking, processing and fibrillogenesis. J. Cell Sci. 118(Pt 7), 1341–1353. doi: 10.1242/jcs.01731
daSilva, L. L., Snapp, E. L., Denecke, J., Lippincott-Schwartz, J., Hawes, C., and Brandizzi, F. (2004). Endoplasmic reticulum export sites and Golgi bodies behave as single mobile secretory units in plant cells. Plant Cell. 16, 1753–1771. doi: 10.1105/tpc.022673
Erives, A. J. (2015). Genes conserved in bilaterians but jointly lost with Myc during nematode evolution are enriched in cell proliferation and cell migration functions. Dev. Genes Evol. 225, 259–273. doi: 10.1007/s00427-015-0508-1
Fessler, J. H., and Fessler, L. I. (1989). Drosophila extracellular matrix. Annu. Rev. Cell Biol. 5, 309–339. doi: 10.1146/annurev.cb.05.110189.001521
Fromme, J. C., and Schekman, R. (2005). COPII-coated vesicles: flexible enough for large cargo? Curr. Opin. Cell Biol. 17, 345–352. doi: 10.1016/j.ceb.2005.06.004
Garrett, M., Fullaondo, A., Troxler, L., Micklem, G., and Gubb, D. (2009). Identification and analysis of serpin-family genes by homology and synteny across the 12 sequenced Drosophilid genomes. BMC Genomics 10:489. doi: 10.1186/1471-2164-10-489
Glick, B. S. (2014). Integrated self-organization of transitional ER and early Golgi compartments. BioEssays 36, 129–133. doi: 10.1002/bies.201300131
Glick, B. S., and Nakano, A. (2009). Membrane traffic within the Golgi apparatus. Annu. Rev. Cell Dev. Biol. 25, 113–132. doi: 10.1146/annurev.cellbio.24.110707.175421
Gorur, A., Yuan, L., Kenny, S. J., Baba, S., Xu, K., and Schekman, R. (2017). COPII-coated membranes function as transport carriers of intracellular procollagen I. J. Cell Biol. 216, 1745–1759. doi: 10.1083/jcb.201702135
Groth, C., Sasamura, T., Khanna, M. R., Whitley, M., and Fortini, M. E. (2013). Protein trafficking abnormalities in Drosophila tissues with impaired activity of the ZIP7 zinc transporter Catsup. Development 140, 3018–3027. doi: 10.1242/dev.088336
Hanna, M. G., Peotter, J. L., Frankel, E. B., and Audhya, A. (2018). Membrane transport at an organelle interface in the early secretory pathway: take your coat off and stay a while: evolution of the metazoan early secretory pathway. Bioessays 40:e1800004. doi: 10.1002/bies.201800004
Hynes, R. O., and Zhao, Q. (2000). The evolution of cell adhesion. J. Cell Biol. 150, F89–F96. doi: 10.1083/jcb.150.2.f89
Isabella, A. J., and Horne-Badovinac, S. (2015). Dynamic regulation of basement membrane protein levels promotes egg chamber elongation in Drosophila. Dev. Biol. 406, 212–221. doi: 10.1016/j.ydbio.2015.08.018
Ishikawa, Y., Ito, S., Nagata, K., Sakai, L. Y., and Bachinger, H. P. (2016). Intracellular mechanisms of molecular recognition and sorting for transport of large extracellular matrix molecules. Proc. Natl. Acad. Sci. U S A. 113, E6036–E6044. doi: 10.1073/pnas.1609571113
Jensen, D., and Schekman, R. (2011). COPII-mediated vesicle formation at a glance. J. Cell Sci. 124(Pt 1), 1–4. doi: 10.1242/jcs.069773
Jin, L., Pahuja, K. B., Wickliffe, K. E., Gorur, A., Baumgartel, C., Schekman, R., et al. (2012). Ubiquitin-dependent regulation of COPII coat size and function. Nature 482, 495–500. doi: 10.1038/nature10822
Johnson, A., Bhattacharya, N., Hanna, M., Pennington, J. G., Schuh, A. L., Wang, L., et al. (2015). TFG clusters COPII-coated transport carriers and promotes early secretory pathway organization. EMBO J. 34, 811–827. doi: 10.15252/embj.201489032
Ke, H., Feng, Z., Liu, M., Sun, T., Dai, J., Ma, M., et al. (2018). Collagen secretion screening in Drosophila supports a common secretory machinery and multiple Rab requirements. J. Genet. Genomics 45, 299–313. doi: 10.1016/j.jgg.2018.05.002
Kondylis, V., and Rabouille, C. (2009). The Golgi apparatus: lessons from drosophila. FEBS Lett. 583, 3827–3838. doi: 10.1016/j.febslet.2009.09.048
Kondylis, V., Spoorendonk, K. M., and Rabouille, C. (2005). dGRASP localization and function in the early exocytic pathway in Drosophila S2 cells. Mol. Biol. Cell 16, 4061–4072. doi: 10.1091/mbc.e04-10-0938
Kondylis, V., Tang, Y., Fuchs, F., Boutros, M., and Rabouille, C. (2011). Identification of ER Proteins Involved in the functional organisation of the early secretory pathway in drosophila cells by a targeted RNAi screen. PLoS One 6:e17173. doi: 10.1371/journal.pone.0017173
Kumar, A., Bhandari, A., Sarde, S. J., and Goswami, C. (2017). Ancestry & molecular evolutionary analyses of heat shock protein 47?kDa (HSP47/SERPINH1). Sci. Rep. 7:10394. doi: 10.1038/s41598-017-10740-0
Kurokawa, K., Okamoto, M., and Nakano, A. (2014). Contact of cis-Golgi with ER exit sites executes cargo capture and delivery from the ER. Nat. Commun. 5:3653. doi: 10.1038/ncomms4653
Lee, M. C. S., Miller, E. A., Goldberg, J., Orci, L., and Schekman, R. (2004). BI-directional protein transport between the er and golgi. Ann. Rev. Cell Dev. Biol. 20, 87–123. doi: 10.1146/annurev.cellbio.20.010403.105307
Lerner, D. W., McCoy, D., Isabella, A. J., Mahowald, A. P., Gerlach, G. F., Chaudhry, T. A., et al. (2013). A Rab10-dependent mechanism for polarized basement membrane secretion during organ morphogenesis. Dev. Cell 24, 159–168. doi: 10.1016/j.devcel.2012.12.005
Liu, M., Feng, Z., Ke, H., Liu, Y., Sun, T., Dai, J., et al. (2017). Tango1 spatially organizes ER exit sites to control ER export. J. Cell Biol. 216, 1035–1049. doi: 10.1083/jcb.201611088
Lunstrum, G. P., Bächinger, H. P., Fessler, L. I., Duncan, K. G., Nelson, R. E., and Fessler, J. H. (1988). Drosophila basement membrane procollagen IV. I. Protein characterization and distribution. J. Biol. Chem. 263, 18318–18327.
Ma, W., and Goldberg, J. (2016). TANGO1/cTAGE5 receptor as a polyvalent template for assembly of large COPII coats. Proc. Natl. Acad. Sci. U S A. 113, 10061–10066. doi: 10.1073/pnas.1605916113
Maeda, M., Katada, T., and Saito, K. (2017). TANGO1 recruits Sec16 to coordinately organize ER exit sites for efficient secretion. J. Cell Biol. 216, 1731–1743. doi: 10.1083/jcb.201703084
Maeda, M., Komatsu, Y., and Saito, K. (2020). Mitotic ER exit site disassembly and reassembly are regulated by the phosphorylation status of TANGO1. Dev. Cell. 55, 237–250. doi: 10.1016/j.devcel.2020.07.017
Maeda, M., Saito, K., and Katada, T. (2016). Distinct isoform-specific complexes of TANGO1 cooperatively facilitate collagen secretion from the endoplasmic reticulum. Mol. Biol. Cell. 27, 2688–2696. doi: 10.1091/mbc.E16-03-0196
Malhotra, V., and Erlmann, P. (2011). Protein export at the ER: loading big collagens into COPII carriers. EMBO J. 30, 3475–3480. doi: 10.1038/emboj.2011.255
Malhotra, V., and Erlmann, P. (2015). The pathway of collagen secretion. Annu. Rev. Cell Dev. Biol. 31, 109–124. doi: 10.1146/annurev-cellbio-100913-013002
Matsui, Y., Hirata, Y., Wada, I., and Hosokawa, N. (2020). Visualization of procollagen IV reveals ER-to-golgi transport by ERGIC-independent carriers. Cell Struct. Funct. 45, 107–119. doi: 10.1247/csf.20025
McCaughey, J., Miller, V. J., Stevenson, N. L., Brown, A. K., Budnik, A., Heesom, K. J., et al. (2016). TFG promotes organization of transitional er and efficient collagen secretion. Cell Rep. 15, 1648–1659. doi: 10.1016/j.celrep.2016.04.062
McCaughey, J., Stevenson, N. L., Cross, S., and Stephens, D. J. (2019). ER-to-Golgi trafficking of procollagen in the absence of large carriers. J. Cell Biol. 218, 929–948. doi: 10.1083/jcb.201806035
Melville, D., Gorur, A., and Schekman, R. (2019). Fatty-acid binding protein 5 modulates the SAR1 GTPase cycle and enhances budding of large COPII cargoes. Mol. Biol. Cell 30, 387–399. doi: 10.1091/mbc.E18-09-0548
Meyer, F., and Moussian, B. (2009). Drosophila multiplexin (Dmp) modulates motor axon pathfinding accuracy. Dev. Growth Differ. 51, 483–498. doi: 10.1111/j.1440-169X.2009.01111.x
Mironov, A. A., and Beznoussenko, G. V. (2019). Models of intracellular transport: pros and cons. Front. Cell Dev. Biol. 7:146. doi: 10.3389/fcell.2019.00146
Muschalik, N., and Munro, S. (2018). Golgins. Curr. Biol. 28, R374–R376. doi: 10.1016/j.cub.2018.01.006
Natzle, J. E., Monson, J. M., and McCarthy, B. J. (1982). Cytogenetic location and expression of collagen-like genes in Drosophila. Nature 296, 368–371. doi: 10.1038/296368a0
Nogueira, C., Erlmann, P., Villeneuve, J., Santos, A. J., Martinez-Alonso, E., Martinez-Menarguez, J. A., et al. (2014). SLY1 and Syntaxin 18 specify a distinct pathway for procollagen VII export from the endoplasmic reticulum. Elife 3:e02784. doi: 10.7554/eLife.02784
Omari, S., Makareeva, E., Gorrell, L., Jarnik, M., Lippincott-Schwartz, J., and Leikin, S. (2020). Mechanisms of procollagen and HSP47 sorting during ER-to-Golgi trafficking. Matrix Biol. 93, 79–94. doi: 10.1016/j.matbio.2020.06.002
Omari, S., Makareeva, E., Roberts-Pilgrim, A., Mirigian, L., Jarnik, M., Ott, C., et al. (2018). Noncanonical autophagy at ER exit sites regulates procollagen turnover. Proc. Natl. Acad. Sci. U S A. 115, E10099–E10108. doi: 10.1073/pnas.1814552115
Palade, G. (1975). Intracellular aspects of the process of protein synthesis. Science 189, 347–358. doi: 10.1126/science.1096303
Pastor-Pareja, J. C. (2020). Atypical basement membranes and basement membrane diversity - what is normal anyway? J. Cell. Sci. 133:jcs241794. doi: 10.1242/jcs.241794
Pastor-Pareja, J. C., and Xu, T. (2011). Shaping cells and organs in Drosophila by opposing roles of fat body-secreted Collagen IV and perlecan. Dev. Cell. 21, 245–256. doi: 10.1016/j.devcel.2011.06.026
Petley-Ragan, L. M., Ardiel, E. L., Rankin, C. H., and Auld, V. J. (2016). Accumulation of laminin monomers in drosophila glia leads to glial endoplasmic reticulum stress and disrupted larval locomotion. J. Neurosci. 36, 1151–1164. doi: 10.1523/JNEUROSCI.1797-15.2016
Raote, I., Ernst, A. M., Campelo, F., Rothman, J. E., Pincet, F., and Malhotra, V. (2020). TANGO1 membrane helices create a lipid diffusion barrier at curved membranes. Elife 9:e57822. doi: 10.7554/eLife.57822
Raote, I., and Malhotra, V. (2019). Protein transport by vesicles and tunnels. J. Cell Biol. 218, 737–739. doi: 10.1083/jcb.201811073
Raote, I., Ortega Bellido, M., Pirozzi, M., Zhang, C., Melville, D., Parashuraman, S., et al. (2017). TANGO1 assembles into rings around COPII coats at ER exit sites. J. Cell Biol. 216, 901–909. doi: 10.1083/jcb.201608080
Raote, I., Ortega-Bellido, M., Santos, A. J., Foresti, O., Zhang, C., Garcia-Parajo, M. F., et al. (2018). TANGO1 builds a machine for collagen export by recruiting and spatially organizing COPII, tethers and membranes. Elife 7:e32723. doi: 10.7554/eLife.32723
Reynolds, H. M., Zhang, L., Tran, D. T., and Ten Hagen, K. G. (2019). Tango1 coordinates the formation of endoplasmic reticulum/Golgi docking sites to mediate secretory granule formation. J. Biol. Chem. 294, 19498–19510. doi: 10.1074/jbc.RA119.011063
Rios-Barrera, L. D., Sigurbjornsdottir, S., Baer, M., and Leptin, M. (2017). Dual function for tango1 in secretion of bulky cargo and in ER-golgi morphology. Proc. Natl. Acad. Sci. U S A. 114, E10389–E10398. doi: 10.1073/pnas.1711408114
Ripoche, J., Link, B., Yucel, J. K., Tokuyasu, K., and Malhotra, V. (1994). Location of Golgi membranes with reference to dividing nuclei in syncytial drosophila embryos. Proc. Natl. Acad. Sci. U S A. 91, 1878–1882. doi: 10.1073/pnas.91.5.1878
Roy Chowdhury, S., Bhattacharjee, C., Casler, J. C., Jain, B. K., Glick, B. S., and Bhattacharyya, D. (2020). ER arrival sites associate with ER exit sites to create bidirectional transport portals. J. Cell. Biol. 219:e201902114. doi: 10.1083/jcb.201902114
Saito, K., Chen, M., Bard, F., Chen, S., Zhou, H., Woodley, D., et al. (2009). TANGO1 facilitates cargo loading at endoplasmic reticulum exit sites. Cell 136, 891–902. doi: 10.1016/j.cell.2008.12.025
Saito, K., Yamashiro, K., Ichikawa, Y., Erlmann, P., Kontani, K., Malhotra, V., et al. (2011). cTAGE5 mediates collagen secretion through interaction with TANGO1 at endoplasmic reticulum exit sites. Mol. Biol. Cell 22, 2301–2308. doi: 10.1091/mbc.E11-02-0143
Saito, K., Yamashiro, K., Shimazu, N., Tanabe, T., Kontani, K., and Katada, T. (2014). Concentration of Sec12 at ER exit sites via interaction with cTAGE5 is required for collagen export. J. Cell Biol. 206, 751–762. doi: 10.1083/jcb.201312062
Santos, A. J., Nogueira, C., Ortega-Bellido, M., and Malhotra, V. (2016). TANGO1 and Mia2/cTAGE5 (TALI) cooperate to export bulky pre-chylomicrons/VLDLs from the endoplasmic reticulum. J. Cell. Biol. 213, 343–354. doi: 10.1083/jcb.201603072
Santos, A. J., Raote, I., Scarpa, M., Brouwers, N., and Malhotra, V. (2015). TANGO1 recruits ERGIC membranes to the endoplasmic reticulum for procollagen export. Elife 4:e10982. doi: 10.7554/eLife.10982
Schekman, R. (2010). Charting the secretory pathway in a simple eukaryote. Mol. Biol. Cell 21, 3781–3784. doi: 10.1091/mbc.e10-05-0416
Sepulveda, D., Rojas-Rivera, D., Rodríguez, D. A., Groenendyk, J., Köhler, A., Lebeaupin, C., et al. (2018). Interactome screening identifies the ER luminal chaperone Hsp47 as a regulator of the unfolded protein response transducer IRE1α. Mol. Cell 69, 238–252. doi: 10.1016/j.molcel.2017.12.028
Sparkes, I. A., Ketelaar, T., de Ruijter, N. C., and Hawes, C. (2009). Grab a golgi: laser trapping of golgi bodies reveals in vivo interactions with the endoplasmic reticulum. Traffic 10, 567–571. doi: 10.1111/j.1600-0854.2009.00891.x
Sprangers, J., and Rabouille, C. (2015). SEC16 in COPII coat dynamics at ER exit sites. Biochem. Soc. Transac. 43, 97–103. doi: 10.1042/bst20140283
Stoll, R., and Bosserhoff, A. (2008). Extracellular SH3 domain containing proteins–features of a new protein family. Curr. Protein Pept. Sci. 9, 221–226. doi: 10.2174/138920308784534014
Stoll, R., Renner, C., Zweckstetter, M., Brüggert, M., Ambrosius, D., Palme, S., et al. (2001). The extracellular human melanoma inhibitory activity (MIA) protein adopts an SH3 domain-like fold. Embo j. 20, 340–349. doi: 10.1093/emboj/20.3.340
Suisse, A., and Treisman, J. E. (2019). Reduced SERCA function preferentially affects wnt signaling by retaining E-cadherin in the endoplasmic reticulum. Cell Rep. 26, 322–329. doi: 10.1016/j.celrep.2018.12.049
Sun, T., Song, Y., Dai, J., Mao, D., Ma, M., Ni, J. Q., et al. (2019). Spectraplakin shot maintains perinuclear microtubule organization in drosophila polyploid cells. Dev. Cell 49:e737. doi: 10.1016/j.devcel.2019.03.027
Tanabe, T., Maeda, M., Saito, K., and Katada, T. (2016). Dual function of cTAGE5 in collagen export from the endoplasmic reticulum. Mol. Biol. Cell 27, 2008–2013. doi: 10.1091/mbc.E16-03-0180
Thomas, G. (2002). Furin at the cutting edge: from protein traffic to embryogenesis and disease. Nat. Rev. Mol. Cell Biol. 3, 753–766. doi: 10.1038/nrm934
Tiwari, P., Kumar, A., Das, R. N., Malhotra, V., and VijayRaghavan, K. (2015). A tendon cell specific RNAi screen reveals novel candidates essential for muscle tendon interaction. PLoS One 10:e0140976. doi: 10.1371/journal.pone.0140976
Usener, D., Schadendorf, D., Koch, J., Dubel, S., and Eichmuller, S. (2003). cTAGE: a cutaneous T cell lymphoma associated antigen family with tumor-specific splicing. J. Invest. Dermatol. 121, 198–206. doi: 10.1046/j.1523-1747.2003.12318.x
Venditti, R., Scanu, T., Santoro, M., Di Tullio, G., Spaar, A., Gaibisso, R., et al. (2012). Sedlin controls the ER export of procollagen by regulating the Sar1 cycle. Science 337, 1668–1672. doi: 10.1126/science.1224947
Wendler, F., Gillingham, A. K., Sinka, R., Rosa-Ferreira, C., Gordon, D. E., Franch-Marro, X., et al. (2010). A genome-wide RNA interference screen identifies two novel components of the metazoan secretory pathway. EMBO J. 29, 304–314. doi: 10.1038/emboj.2009.350
Wilson, D. G., Phamluong, K., Li, L., Sun, M., Cao, T. C., Liu, P. S., et al. (2011). Global defects in collagen secretion in a Mia3/TANGO1 knockout mouse. J. Cell Biol. 193, 935–951. doi: 10.1083/jcb.201007162
Witte, K., Schuh, A. L., Hegermann, J., Sarkeshik, A., Mayers, J. R., Schwarze, K., et al. (2011). TFG-1 function in protein secretion and oncogenesis. Nat. Cell Biol. 13, 550–558. doi: 10.1038/ncb2225
Yuan, L., Kenny, S. J., Hemmati, J., Xu, K., and Schekman, R. (2018). TANGO1 and SEC12 are copackaged with procollagen I to facilitate the generation of large COPII carriers. Proc. Natl. Acad. Sci. U S A. 115, E12255–E12264. doi: 10.1073/pnas.1814810115
Yurchenco, P. D. (2011). Basement membranes: cell scaffoldings and signaling platforms. Cold Spring Harb. Perspect. Biol. 3:a004911. doi: 10.1101/cshperspect.a004911
Zang, Y., Wan, M., Liu, M., Ke, H., Ma, S., Liu, L. P., et al. (2015). Plasma membrane overgrowth causes fibrotic collagen accumulation and immune activation in Drosophila adipocytes. Elife 4:e07187. doi: 10.7554/eLife.07187
Zhang, L., Syed, Z. A., van Dijk Hard, I., Lim, J. M., Wells, L., and Ten Hagen, K. G. (2014). O-glycosylation regulates polarized secretion by modulating Tango1 stability. Proc. Natl. Acad. Sci. U S A. 111, 7296–7301. doi: 10.1073/pnas.1322264111
Keywords: traffic, secretion, ER exit site, COPII, collagen, extracellular matrix
Citation: Feng Z, Yang K and Pastor-Pareja JC (2021) Tales of the ER-Golgi Frontier: Drosophila-Centric Considerations on Tango1 Function. Front. Cell Dev. Biol. 8:619022. doi: 10.3389/fcell.2020.619022
Received: 19 October 2020; Accepted: 14 December 2020;
Published: 11 January 2021.
Edited by:
Yasunori Saheki, Nanyang Technological University, SingaporeReviewed by:
Vivek Malhotra, Centre for Genomic Regulation (CRG), SpainJaakko Saraste, University of Bergen, Norway
Copyright © 2021 Feng, Yang and Pastor-Pareja. This is an open-access article distributed under the terms of the Creative Commons Attribution License (CC BY). The use, distribution or reproduction in other forums is permitted, provided the original author(s) and the copyright owner(s) are credited and that the original publication in this journal is cited, in accordance with accepted academic practice. No use, distribution or reproduction is permitted which does not comply with these terms.
*Correspondence: José C. Pastor-Pareja, am9zZXBhc3RvckB0c2luZ2h1YS5lZHUuY24=