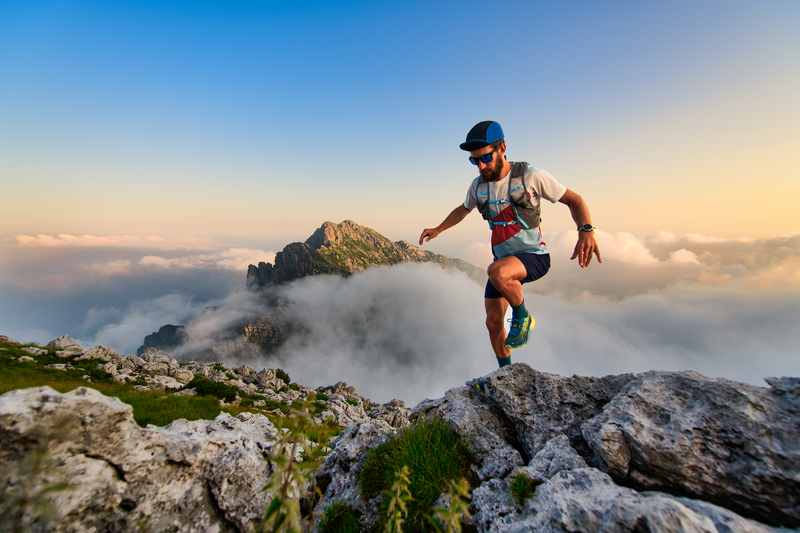
95% of researchers rate our articles as excellent or good
Learn more about the work of our research integrity team to safeguard the quality of each article we publish.
Find out more
REVIEW article
Front. Cell Dev. Biol. , 03 December 2020
Sec. Cell Growth and Division
Volume 8 - 2020 | https://doi.org/10.3389/fcell.2020.614821
This article is part of the Research Topic Cytoskeletal Dynamics and Mechanics in Cell Growth, Division, Differentiation and Aging View all 12 articles
In flowering plants, sexual reproduction involves a double fertilization event, which is facilitated by the delivery of two non-motile sperm cells to the ovule by the pollen tube. Pollen tube growth occurs exclusively at the tip and is extremely rapid. It strictly depends on an intact actin cytoskeleton, and is therefore an excellent model for uncovering the molecular mechanisms underlying dynamic actin cytoskeleton remodeling. There has been a long-term debate about the organization and dynamics of actin filaments within the apical and subapical regions of pollen tube tips. By combining state-of-the-art live-cell imaging with the usage of mutants which lack different actin-binding proteins, our understanding of the origin, spatial organization, dynamics and regulation of actin filaments within the pollen tube tip has greatly improved. In this review article, we will summarize the progress made in this area.
In flowering plants (angiosperms), seed formation requires two fertilization events. The male germ unit, called the male gametophyte, is contained within pollen grains and comprises a vegetative cell and two sperm cells that have already lost their motility (Kaul et al., 2000; Dresselhaus et al., 2016; Higashiyama, 2018). The process of double fertilization begins when pollen grains land on and adhere to the surface of the stigma. Following hydration of the pollen grain, the vegetative cell generates a pollen tube (Chapman and Goring, 2010). Pollen tubes then grow through the transmitting tissue of the style and serve as an active vehicle to transport the two immotile sperm cells into the ovule under the attraction of various female molecules (Higashiyama and Takeuchi, 2015; Zhang et al., 2017; Johnson et al., 2019). Therefore, pollen tube growth represents a critical stage during the extended journey that is required for double fertilization in flowering plants.
Similar to the filamentous protonemata of mosses and the root hairs of high plants, pollen tubes are tip-growing cells, with growth strictly occurring within the apical region (Rounds and Bezanilla, 2013). Pollen tubes grow rapidly both in vivo and in vitro. For instance, the growth rate of lily (Lilium longiflorum and Lilium formosanum) pollen tubes can reach up to 12–18 μm min–1 (Hepler et al., 2001). Although Arabidopsis thaliana pollen tubes and Nicotiana tabacum pollen tubes grow comparatively slowly when compared to lily pollen tubes, their growth rate can reach up to 2 and 1.5–6 μm min–1, respectively (Cheung and Wu, 2008). The rapidity of pollen tube growth greatly shortens the time required for the delivery of sperm cells to the ovules, thus favoring fertilization. Plant biologists have been fascinated by this remarkable growth phenomenon. The rapid growth of pollen tubes requires the availability of a huge amount of materials for plasma membrane expansion and cell wall synthesis within the pollen tube growth region. In line with this, pollen tubes harbor an active intracellular transport system to enable the efficient delivery of materials to the growth region, which subsequently coordinate with tightly regulated endocytotic and exocytotic events to support pollen tube tip growth (Rounds and Bezanilla, 2013). The actin cytoskeleton plays an essential role in driving the growth and morphogenesis of pollen tubes by choreographing endo- and exocytotic vesicle traffic (Taylor and Hepler, 1997; Cole and Fowler, 2006; Cheung and Wu, 2008; Yang, 2008; Qin and Yang, 2011; Guan et al., 2013). As such, the role and mechanism of action of the actin cytoskeleton in the regulation of polarized pollen tube growth have been subjected to intensive scrutiny in the past few decades. Careful examination of the organization of actin filaments in living and fixed pollen tubes has provided significant insights into the spatial organization of actin filaments in pollen tubes (for reviews see Hepler et al., 2001; Vidali and Hepler, 2001; Samaj et al., 2006; Ren and Xiang, 2007; Chen et al., 2009; Staiger et al., 2010; Cai et al., 2015; Fu, 2015; Qu et al., 2015; Stephan, 2017). In this review, we will describe our current understanding of the organization, dynamics and regulation of the actin cytoskeleton in pollen tubes, with the focus on the apical and subapical regions.
Experimental treatments with actin-based pharmacological agents showed that the actin cytoskeleton is absolutely required for pollen germination and pollen tube growth (Franke et al., 1972; Herth et al., 1972; Mascarenhas and Lafountain, 1972; Speranza and Calzoni, 1989; Heslopharrison and Heslopharrison, 1991; Mascarenhas, 1993; Gibbon et al., 1999; Vidali et al., 2001). As the building block of the actin cytoskeleton, actin is a very abundant protein in pollen, accounting for about 2–20% of the total soluble protein in pollen grains (Liu and Yen, 1992; Ren et al., 1997; Vidali and Hepler, 1997; Gibbon et al., 1999; Snowman et al., 2002). Therefore, plant scientists have used pollen as the starting material to isolate polymerization-competent plant actin (Liu and Yen, 1992; Ren et al., 1997). Different methods have been used to determine the cellular concentration of actin monomers in pollen from maize (Gibbon et al., 1999), poppy (Snowman et al., 2002), lily (Vidali and Hepler, 1997), and Arabidopsis (Jiang et al., 2019). These investigations showed that the total actin concentration can reach up to about 200 μM in pollen. In fact, there are five reproductive actin isovariants (ACT1, ACT3, ACT4, ACT11, and ACT12) expressed in Arabidopsis pollen, and simultaneous silence of ACT1, ACT3, ACT4, and ACT12 by RNA interference (RNAi) causes obvious reproductive defects (Pawloski et al., 2006). The direct evidence for the involvement of actin in regulating pollen germination and pollen tube growth came from the analysis of the mutant lacking ACT11, showing that pollen germination was inhibited (Chang and Huang, 2015). Surprisingly, loss of function of ACT11 upregulates pollen tube growth (Chang and Huang, 2015), which is presumably due to the increase in actin dynamics in pollen tubes. Given that the local concentration of actin monomers directly impacts their assembly and disassembly, researchers in this field have tried to reveal the intracellular localization of actin monomers in pollen tubes. They showed that there exists a tip-focused gradient of monomeric G-actin in pollen tubes (Li et al., 2001; Cardenas et al., 2005). One interesting study showed that actin monomers actually distribute uniformly within the cytoplasm of Arabidopsis pollen tubes and are rapidly redistributed via cytoplasmic streaming (Chang et al., 2017), which suggests that actin monomers are readily available to assemble within the pollen tube. Given that most actin-based functions are carried out by the filamentous form (F-actin), plant scientists have tried different methods to uncover the organization of actin filaments in pollen tubes. These approaches include labeling actin filaments with fluorescently-tagged phalloidin or immunostaining with an anti-actin antibody in fixed pollen tubes (Tang et al., 1989; Gibbon et al., 1999; Geitmann et al., 2000; Lovy-Wheeler et al., 2005; Thomas et al., 2006; Wilsen et al., 2006; Ye et al., 2009; Wu et al., 2010; Zhang et al., 2010; Qu et al., 2020), or using actin markers including GFP-mTalin or YFP-mTalin, GFP-ABD2, GFP-ADF, GFP-LIM, and Lifeact-GFP or YFP-Lifeact (Kost et al., 1998; Fu et al., 2001; Wilsen et al., 2006; Cheung et al., 2008; Vidali et al., 2009; Zhang et al., 2009; Zhang et al., 2010; Qu et al., 2013; Stephan et al., 2014) to decorate actin filaments in living pollen tubes. These investigations have resulted in a consensus view that actin filaments are arranged into longitudinally aligned bundles in the shank region of pollen tubes (Figure 1A). Shank actin filaments are important for a transport mechanism in angiosperm pollen tubes called reverse-fountain cytoplasmic streaming. The flow of cytoplasm is generated by the movement of barbed-end directed myosin motors along the shank-localized actin filaments. In the cortex of the pollen tube, cytoplasm flows toward the tip, while in the middle of the pollen tube, it flows back toward the bottom. Based on the determination of the polarity of shank-localized actin bundles in root hairs (Tominaga et al., 2000), which also generate reverse-fountain cytoplasmic streaming, cortical actin bundles and inner actin bundles likely have their barbed ends facing toward the tip and base of pollen tubes, respectively. Indeed, this has been verified by visualizing actin filaments decorated with myosin II subfragment 1 in pollen tubes by electron microscopy (Lenartowska and Michalska, 2008). Both cortical and inner actin bundles terminate at the subapex of pollen tubes. By comparison, determining the organization of the actin cytoskeleton within the apical and subapical regions has been problematic, as different configurations have been reported in pollen tubes from different species using different methods. In the following sections, we will focus on describing our understanding of the organization, dynamics and regulation of the actin cytoskeleton within the apical and subapical regions of pollen tubes.
Figure 1. Actin Filaments are Continuously Polymerized from the Plasma Membrane within the Apical and Subapical Regions of the Pollen Tube. (A) Schematic diagram depicting our previous understanding of the spatial distribution of actin filaments in the pollen tube. This model refers to the models shown in previous review articles with slight modifications (Vidali and Hepler, 2001; Lovy-Wheeler et al., 2005; Ren and Xiang, 2007; Cheung and Wu, 2008; Yang, 2008; Cai and Cresti, 2009; Qin and Yang, 2011; Guan et al., 2013; Rounds and Bezanilla, 2013; Cai et al., 2015; Fu, 2015; Bascom et al., 2018). Specifically, actin filaments are arrayed into longitudinally oriented actin bundles in the shank and in the actin fringe structure at the subapex. In terms of the polarity of actin filaments within the actin fringe, the models in (a,b) were drawn with reference to Ren and Xiang (2007); Cheung and Wu (2008), and Qin and Yang (2011), respectively. By comparison, actin filaments at the apex are short, less abundant and disorganized. (B) Actin filaments are polymerized from the plasma membrane in an Arabidopsis pollen tube tip. The right panel shows the kymograph analysis of apical actin filaments decorated with Lifeact-eGFP in the growing wild-type (WT) Arabidopsis pollen tube shown in the left panel. Scale bar = 5 μm. (C) Time-lapse images of apical actin filaments in the pollen tube shown in (B). Red dots indicate an actin filament that is polymerized from the plasma membrane, then grows into the inner region of the pollen tube. The yellow scissors indicate a severing event of the same actin filament. Scale bar = 5 μm. The lower panel shows a schematic depiction of the events in the upper panel. (D,E) Actin filaments polymerized from the plasma membrane at the tip of a growing lily (D) and tobacco (E) pollen tube. In each figure, the left panel shows the Z-projection image of actin filaments in the pollen tube. The middle panel shows kymograph analysis of actin filaments growing from the plasma membrane at the pollen tube tip, and the right panel shows some time-lapse images of actin filaments in the growing pollen tube shown in the left panel. Red dots indicate an actin filament that was polymerized from the plasma membrane, then grew into the inner region of the pollen tube. Scale bar = 5 μm. (F) Visualization of RabA4b-positive transport vesicles (left panel) and actin filaments (right panel) in WT Arabidopsis pollen tubes. Transport vesicles accumulate within the region corresponding to the clear zone at the pollen tube tip (left panel). Actin filaments at the base of the clear zone, which polymerize from the plasma membrane, correspond to the actin fringe at the subapex shown in (A). Scale bar = 5 μm. (G) Schematic depiction of our current view of the organization of actin filaments in the Arabidopsis pollen tube. Similar to the model shown in (A), actin filaments are organized into actin bundles oriented longitudinally in the shank region. Within the apical and subapical regions of the pollen tube, actin filaments are polymerized from the plasma membrane. These filaments can be viewed as a whole and defined as the “apical actin structure.” Membrane-originated actin filaments within this “apical actin structure” assume a distinct spatial distribution, with some cortical actin filaments forming thick actin bundles, while some inner actin filaments are comparatively fine and extend toward the inner region of the cytoplasm.
Previous studies suggested that pollen tube growth is more sensitive to the treatment of actin destabilizing reagents than cytoplasmic streaming (Gibbon et al., 1999; Vidali et al., 2001), which suggests that the actin cytoskeleton within the pollen tube growth region is highly dynamic. This is also the reason why the actin cytoskeleton within the apical and subapical regions cannot be fixed instantly, thus preventing us from reaching a consensus view about the organization of the actin cytoskeleton within the pollen tube growth region. In the past, efforts have been made to describe the organization of the actin cytoskeleton within the apical and subapical regions separately. Although there is some argument about whether actin filaments exist within the apical region of pollen tubes, researchers in the field believe that the apical region does contain actin filaments, but they are short, less abundant and randomly distributed (Yang, 2008; Staiger et al., 2010). Different organizational patterns of subapical actin filaments within pollen tubes have been revealed by different actin labeling approaches and, as such, different names have been provided to describe the organization of subapical actin filaments in pollen tubes from different species. These names include actin collar (Gibbon et al., 1999; Qu et al., 2013), actin fringe (Lovy-Wheeler et al., 2005; Dong et al., 2012; Rounds et al., 2014), actin ring or actin mesh (Kost et al., 1998; Chen et al., 2002). The variation in the subapical actin structure might be due to the employment of different actin labeling approaches or to true differences among pollen tubes from different species. Different schematic models had been generated to describe the organization of actin filaments within the apical and subapical regions of pollen tubes. One typical schematic model, presented in Figure 1A, shows that actin filaments are arranged into an actin fringe structure at the subapex, and are shorter, less abundant and more disorganized at the extreme apex. However, the polarity of actin filaments within the actin fringe remains the subject of debate. Different models are presented in the literature in terms of the polarity of actin filaments within the actin fringe structure. One model showed that actin filaments at the cortex and in the inner region within the actin fringe have their barbed ends facing toward the tip and base of the pollen tube, respectively (Figure 1Aa; Ren and Xiang, 2007; Cheung and Wu, 2008). Another model showed that actin filaments within the actin fringe have their barbed ends facing toward the tip of the pollen tube (Figure 1Ab; Qin and Yang, 2011). Therefore, the origin and exact organization of subapical and apical actin filaments are unclear.
In this regard, live-cell imaging of Lifeact-GFP-decorated actin filaments in growing wild-type pollen tubes and in mutant Arabidopsis pollen tubes with loss of function of specific actin-binding proteins (ABPs) has revolutionized our view about the origin, polymerization and organization of actin filaments within the apical and subapical regions of pollen tubes. Specifically, this approach has shown that actin filaments are continuously polymerized from the plasma membrane at the growing Arabidopsis pollen tube tips (Figures 1B,C; Qu et al., 2013). A similar phenomenon was also noticed in lily and tobacco pollen tubes (Figures 1D,E; Vidali et al., 2009; Rounds et al., 2014), which suggests that the polymerization of actin filaments from the apical plasma membrane is a common design in angiosperm pollen tubes. In support of this notion, loss of function of class I formins, which are important actin nucleating factors in pollen tubes, impairs the polymerization of actin filaments from the plasma membrane at pollen tube tips (Cheung et al., 2010; Lan et al., 2018). In line with this finding, loss of function of profilins, the functional partners of formins, impaired the polymerization of actin filaments from the plasma membrane at the extreme apex of pollen tubes (Liu et al., 2015). Simultaneous visualization of actin filaments, the clear zone (which corresponds to the previously defined actin fringe) and transport vesicles showed that the actin structure at the base of the clear zone (Lovy-Wheeler et al., 2005, 2006) is made up of actin filaments polymerized from the plasma membrane (Figure 1F; Qu et al., 2017). These findings allow us to propose a schematic model describing the spatial organization of apical and subapical actin filaments in the pollen tube (Figure 1G). In this model, actin filaments within both the apical and subapical regions of pollen tubes are generated from the plasma membrane, and the actin filaments within the two regions can be viewed as a whole, which is defined as the “apical actin structure” (Figure 1G; Qu et al., 2017). Consequently, actin filaments can be viewed as forming two notable structures in pollen tubes: the shank-localized longitudinal actin bundles and the “apical actin structure” (Qu et al., 2017).
Live-cell imaging of the dynamics of actin filaments revealed that actin polymerization continuously occurs from the plasma membrane at pollen tube tips, and this polymerization is required for and concurrent with pollen tube growth (Qu et al., 2017). How apical actin polymerization is regulated during pollen tube growth is an interesting question. Actin polymerization is dictated by specific actin nucleation factors, and Arp2/3 complex and formins are two major types of actin nucleation factors that have been characterized in plants (Blanchoin and Staiger, 2010). Both Arp2/3 complex and formins have received widespread attention in the context of plasma membrane-originated actin polymerization. The role of Arp2/3 complex in regulating the morphogenesis of trichome and epidermal pavement cells has been studied extensively (Le et al., 2003; Li et al., 2003; Mathur et al., 2003a,b; El-Din El-Assal et al., 2004), but the role of Arp2/3 complex in regulating actin polymerization in pollen is not clear. The tips of wild-type pollen tubes do not contain dense branched F-actin networks (Qu et al., 2015), and loss of function of Arp2/3 complex does not affect fertility in Arabidopsis (Szymanski, 2005), which indicates that the Arp2/3 complex is not essential for pollen tube growth. Therefore, there is no direct evidence that Arp2/3 complex is involved in the regulation of actin polymerization in pollen tubes. Formin proteins contain the characteristic formin homology (FH) domains, FH1 and FH2, and are able to nucleate actin assembly from actin monomers or actin-profilin complexes (Kovar, 2006; Goode and Eck, 2007). Based on the sequence of their FH2 domains, plant formins are divided into three classes, class I, class II, and class III (Cvrckova et al., 2004). Only two class III formins have been identified, and they are found in land plants that contain flagellate sperm (Grunt et al., 2008; van Gisbergen and Bezanilla, 2013). Class I and class II formins are common in plants. Most of the class I formins contain a transmembrane (TM) domain at their N-terminus, which enables them to target to the plasma membrane or endomembrane systems. The N-terminus of class II formins is quite variable. Some of them have a phosphatase and tensin homolog (PTEN)-like domain at their N-terminus (Blanchoin and Staiger, 2010). Considering that actin is buffered by an almost equimolar amount of profilin (Vidali and Hepler, 1997; Gibbon et al., 1999; Snowman et al., 2002; Jiang et al., 2019), and actin-profilin complexes are favored by formins rather than the Arp2/3 complex (Rotty et al., 2015; Suarez et al., 2015), it is easy to imagine the important role of formins in controlling actin polymerization in pollen. Accordingly, it was shown that treatment with the formin inhibitor SMIFH2 (Rizvi et al., 2009), which inhibits plant formins in vitro (Cao et al., 2016), impairs actin polymerization from the plasma membrane at pollen tube tips (Qu et al., 2017). As actin polymerization continuously occurs from the plasma membrane at the pollen tube tip (Qu et al., 2013), the TM-containing class I formins are particularly relevant. Indeed, two class I formins, Arabidopsis formin 3 (AtFH3), and AtFH5, have been shown to nucleate actin assembly from actin monomers or actin bound to profilin (Ingouff et al., 2005; Ye et al., 2009), and are redundantly required for actin polymerization from the plasma membrane in pollen tubes (Lan et al., 2018). Accordingly, reducing the expression of Nicotiana tabacum homolog of AtFH5, NtFH5, in tobacco pollen impairs the actin polymerization from the plasma membrane (Cheung et al., 2010). In line with this finding, overexpression of Arabidopsis formin 1 induces the formation of supernumerary actin cables from the plasma membrane and causes membrane deformation (Cheung and Wu, 2004). The importance of class I formins in regulating actin polymerization at pollen tube tips is also supported by the finding that the pollen-specific Lilium longiflorum Formin 1 (LlFH1) controls the construction of the actin fringe in pollen tubes (Li et al., 2017). These findings together suggest that class I formins play important roles in controlling actin polymerization within the apical and subapical regions of pollen tubes.
Functional characterization of Arabidopsis profilins in pollen also provides evidence that formin is a major player in controlling actin polymerization at the tip of pollen tubes. Profilin is a low molecular weight protein, ranging from 12 to 15 kDa, and it can bind to G-actin to form high affinity 1:1 profilin-actin complexes (Carlsson et al., 1977; Vidali and Hepler, 1997). It was shown that profilin has a dual role in regulating actin dynamics. When the barbed ends of actin filaments are capped, profilin acts as a simple actin monomer sequestering protein to promote actin depolymerization (Huang et al., 2004). In support of this notion, it was shown that microinjection of profilin into Tradescantia blossfeldiana stamen hair cells causes the disappearance of transvacuole strands and displacement of nuclei (Staiger et al., 1994). However, when the barbed ends of actin filaments are free, actin-profilin complexes can add onto the barbed ends to elongate actin filaments and thus promote actin polymerization (Pantaloni and Carlier, 1993). Evidence for such a functional role of profilin was strengthened by the finding that the presence of formin can facilitate the addition of actin-profilin complexes onto the barbed ends of actin filaments to accelerate their elongation (Romero et al., 2004; Kovar et al., 2006). Within this framework, formin facilitates the addition of actin-profilin complexes onto the barbed end of actin filaments through its proline-rich FH1 domain. Consistent with this, it was shown that the function of profilin depends on its interaction with proline-rich motifs (Gibbon et al., 1998). Based on the fact that actin binds to profilin with high affinity (Gibbon et al., 1997; Kovar et al., 2000) and they exist in roughly equimolar amounts in pollen (Vidali and Hepler, 1997; Gibbon et al., 1999; Snowman et al., 2002; Jiang et al., 2019), it was predicted that actin mainly exists in the form of actin-profilin complexes in pollen (Staiger and Blanchoin, 2006; Chen et al., 2009). In support of the role of profilin in promoting actin polymerization, it was shown that loss of function of profilins impairs actin polymerization from the plasma membrane at the tip of Arabidopsis pollen tubes (Liu et al., 2015). Importantly, it was shown that the mutant PRF5Y6A, which is defective in binding to PLP but retains normal G-actin binding activity, has impaired function in actin polymerization at pollen tube tips (Liu et al., 2015). This strongly suggests that formin and profilin work as a module in controlling actin polymerization from the plasma membrane at the tip of pollen tubes.
To support continuous actin polymerization during pollen tube growth, a pool of polymerizable actin monomers must be available within pollen tubes. Given that actin is assumed to be buffered by equimolar profilin in pollen, and plant profilins lack or have weak actin nucleotide exchange activity (Kovar et al., 2001; Chaudhry et al., 2007; Liu et al., 2015), a mechanism is required to recharge the dissociated ADP-G-actin before the formation of actin-profilin complexes. Adenylyl cyclase-associated protein 1 (CAP1), also known as Srv2p in budding yeast, is a protein shown to have actin nucleotide exchange activity in Arabidopsis (Chaudhry et al., 2007). It is assumed to take on the role of recharging ADP-G-actin in plants. CAP1 is an abundant protein and its cellular concentration falls between that of ADF and profilin in Arabidopsis pollen (Jiang et al., 2019). It can coordinate with ADF and profilin to promote actin turnover and enhance actin nucleotide exchange in vitro (Chaudhry et al., 2007; Jiang et al., 2019). CAP1 distributes uniformly in pollen tubes and depletion of CAP1 impairs membrane-originated actin polymerization at pollen tube tips. Furthermore, CAP1 protein that is defective in actin nucleotide exchange activity cannot fully rescue the apical actin polymerization defects in cap1 mutant pollen tubes (Jiang et al., 2019), which suggests that the actin nucleotide exchange activity of CAP1 is biologically significant. These findings together allow us to propose a model for the regulation of actin polymerization from the plasma membrane at pollen tube tips (Figure 2; Jiang et al., 2019). Specifically, the membrane-anchored class I formins initiate actin polymerization from the pool of actin-profilin complexes within the cytoplasm, and ADF drives the turnover of membrane-originated actin filaments and controls their length via its actin severing and depolymerizing activity (see the detailed description below). CAP1 works as the shuttle molecule between ADF and profilin to promote actin turnover and maintain the pool of polymerizable actin monomers to drive formin-mediated actin polymerization from the plasma membrane (Figure 2). These findings provide significant insights into the molecular mechanism that controls actin polymerization from the plasma membrane at the pollen tube tip.
Figure 2. Schematic depiction of the regulation of actin polymerization and dynamics in the pollen tube. (A) Schematic depiction of the intracellular localization pattern and function of various ABPs in pollen tube growth domain. The model is mainly based on data from Arabidopsis. (B) An enlarged picture of the boxed region in (A) is presented in order to show more details about the regulation of actin polymerization and dynamics in the tip of pollen tube. In brief, within the pollen tube, actin predominantly exists in the monomeric form. It is buffered by an equimolar amount of profilin to form actin-profilin complexes. Actin polymerization is initiated by membrane-anchored formins, which utilize actin-profilin complexes within the cytoplasm. The membrane-originated actin filaments assume distinct distributions in space as described in Figure 1G, and they are turned over by ADF and its cofactors, including AIP1 and CAP1, and several Ca2+-responsive actin severing proteins, which promote the dynamics and control the length of actin filaments. Under the action of various actin bundling/crosslinking proteins, including villins (Qu et al., 2013), LIMs (Wang et al., 2008; Papuga et al., 2010; Zhang et al., 2019), and fimbrins (Su et al., 2012; Zhang et al., 2016), membrane-originated actin filaments are organized into distinct structures and assume distinct distributions in the cortical and inner regions of the pollen tube.
The mechanisms that regulate the turnover of apical and subapical actin filaments have been the subject of intensive studies in the past. Given that actin filaments are mainly generated from the membrane-anchored class I formins (Cheung et al., 2010; Lan et al., 2018), the ends of actin filaments facing toward the cytoplasm are pointed ends that should be favored by actin-depolymerizing factors (ADFs). ADFs are extremely relevant players in trimming actin filaments to control their length and drive their turnover. Indeed, ADFs have been implicated in the regulation of actin dynamics in pollen grains and pollen tubes (Smertenko et al., 2001; Chen et al., 2002), but the precise mechanism underlying their action remains largely unknown. With the employment of the Arabidopsis genetic approach, our understanding of the role and mechanism of action of ADFs in pollen has improved substantially. Besides Arabidopsis ADF5, which regulates the actin cytoskeleton via stabilizing and bundling actin filaments in pollen tubes (Zhu et al., 2017), ADF7 and ADF10, which are expressed specifically in Arabidopsis pollen (Bou Daher et al., 2011; Daher and Geitmann, 2012), are two major typical actin depolymerizing factors that promote the turnover of the actin cytoskeleton in pollen via severing and depolymerizing actin filaments (Zheng et al., 2013; Jiang et al., 2017). The role of ADF7 in promoting the turnover of shank-localized actin bundles was demonstrated several years ago (Zheng et al., 2013), but its role in regulating the dynamics of apical and subapical actin filaments remains to be characterized. ADF10 was demonstrated to sever and depolymerize subapical actin filaments to promote their turnover and ordering (Jiang et al., 2017). In line with these findings, loss of function of actin-interacting protein 1 (AIP1), the cofactor of ADF (Allwood et al., 2002; Shi et al., 2013; Diao et al., 2020), reduces the rate of actin turnover and induces disorganization of subapical actin filaments in Arabidopsis pollen tubes (Diao et al., 2020). In addition, it was shown that depletion of CAP1 decreases ADF-mediated actin depolymerization and severing, which reduces the rate of actin turnover in pollen tubes (Jiang et al., 2019). These data together identify ADF as an essential player in promoting the turnover of actin filaments in pollen tubes.
In addition, as pollen tubes harbor a tip-high Ca2+ gradient (HoldawayClarke et al., 1997; Diao et al., 2018), several Ca2+-responsive actin severing proteins are involved in regulating the turnover of apical and subapical actin filaments. In this regard, the Ca2+-responsive villin/gelsolin/fragmin members are extremely relevant (Yamashiro et al., 2001; Huang et al., 2004; Xiang et al., 2007; Wang et al., 2008; Khurana et al., 2010; Zhang et al., 2010; Zhang et al., 2011; Bao et al., 2012; Wu et al., 2015). Most of the in vivo functional data about villin/gelsolin/fragmin family members have come from the analysis of villins using the reverse genetic approach, as the plant genome only encodes genes for full-length villins (Klahre et al., 2000; Huang et al., 2015). The villin homologs were originally identified from pollen by biochemical means and demonstrated to be bona fide actin bundlers (Yokota et al., 1998, 2003). Although it was subsequently confirmed that villin can bind to G-actin and promote actin depolymerization in the presence of Ca2+/Calmodulin (Yokota et al., 2005), the direct evidence supporting the role of villins in severing actin filaments came from biochemical analyses of villins from Arabidopsis and rice (Khurana et al., 2010; Zhang et al., 2010; Zhang et al., 2011; Bao et al., 2012; Wu et al., 2015). In support of the role of villins in promoting actin turnover in pollen tubes, it was shown that loss of function of Arabidopsis villin2 (VLN2) and VLN5 causes accumulation of filamentous actin at pollen tube tips (Qu et al., 2013). The reduction in the frequency of actin filament severing in vln2 vln5 double mutant pollen tubes suggests that the severing activity of villins likely contributes to their role in promoting actin turnover (Qu et al., 2013). In line with this finding, it was shown that the severing activity of villin is involved in the formation of actin foci triggered by elevation of the cytosolic Ca2+ concentration in pollen tubes (Zhao et al., 2020). Within this framework, several other Ca2+-responsive actin severing proteins were also shown to be involved in the regulation of actin turnover at pollen tube tips, such as MAP18, MDP25, and ROP-interactive CRIB motif-containing protein 1 (RIC1) (Zhu et al., 2013; Qin et al., 2014; Zhou et al., 2015). In addition, although there is no evidence showing the direct interaction of RIC3 with the actin cytoskeleton, it was shown that RIC3 promotes the release of free Ca2+, which induces actin depolymerization in pollen tubes (Gu et al., 2005). How exactly RIC3 promotes actin turnover in pollen tubes remains to be determined. Nonetheless, these data suggest that the Ca2+-responsive actin severing proteins act in concert with the Ca2+ gradient to promote actin turnover in pollen tubes.
It remains largely unknown how actin functions within the apical and subapical regions of pollen tubes. This is partly because we lack a unified view about the organization of actin filaments within that region. As discussed above, one common view is that actin filaments are arrayed into an actin fringe structure at the subapex (Figure 1A; Lovy-Wheeler et al., 2005, 2006). Different hypotheses were raised to explain the function of the actin fringe. The proposed functions include: organizing endomembranes and controlling the location of endo- and exocytotic events; acting as a physical barrier to exclude large organelles; structurally supporting the plasma membrane to facilitate turgor driven extension; and generating the force to drive cell growth (Stephan, 2017). Among the different functions, the actin cytoskeleton plays an obvious role in regulating tip-directed vesicle traffic, which leads to the accumulation of vesicles at the pollen tube tip to support pollen tube growth. Different hypotheses were proposed to explain the role of apical and subapical actin filaments in regulating vesicle traffic in pollen tubes (Geitmann and Emons, 2000). These include spatially constraining the distribution of vesicles (Kroeger et al., 2009), acting as a filter for small vesicles (Kost et al., 1998; Cheung et al., 2008), and acting as the tracks for myosin motors to transport vesicles to the tip (Lovy-Wheeler et al., 2005; Daher and Geitmann, 2011; Chebli et al., 2013). As described above, studies in Arabidopsis pollen tubes have revealed more details about the spatial organization and dynamics of the actin cytoskeleton within the apical and subapical regions, which provides an opportunity to understand how actin regulates vesicle traffic in pollen tubes. Within the apical and subapical regions, membrane-originated actin filaments assume distinct spatial distributions, including thick actin bundles in the cortex and relatively fine actin filaments in the middle (Figure 1G; Qu et al., 2017). Further analysis revealed that the cortical actin bundles act as tracks for myosin motors, allowing the transportation of vesicles to the pollen tube tip, while the inner fine actin filaments act as the physical barrier to prevent the backward movement of vesicles from the tip (Qu et al., 2017). This leads to the generation of a “V” shape of vesicle distribution (Figures 3A,C). The apical actin structure as a whole also acts as a physical barrier to prevent the invasion of large organelles into the pollen tube tip (Figures 3B,C). Therefore, cooperation between the apical actin structure and the shank-localized actin bundles leads to the generation of reverse-fountain cytoplasmic streaming and the “V”-shaped vesicle distribution in the pollen tube (Figure 3; Qu et al., 2017). These studies provide significant insights into the functional role of actin in regulating vesicle traffic in pollen tubes.
Figure 3. The Role of the Actin Cytoskeleton in Regulating Vesicle Traffic in the Pollen Tube. (A) Dual visualization of RabA4b-positive transport vesicles (green) and actin filaments (pink) in a WT Arabidopsis pollen tube. The small RabA4b-positive transport vesicles accumulate at the pollen tube tip. Scale bar = 5μm. (B) Dual visualization of ARA7-positive endosomes (green) and actin filaments (pink) in a WT Arabidopsis pollen tube. The large ARA7-positive endosomes are absent at the pollen tube tip. Scale bar = 5 μm. (C) Schematic depiction of the function of the actin cytoskeleton in regulating vesicle traffic in the pollen tube. Both ARA7-positive large endosomes and RabA4b-positive small vesicles are transported along cortical actin bundles in the shank region. Upon reaching the subapex, ARA7-positive large endosomes reverse their direction of movement and return to the base along the inner actin bundles. This is the basis for the generation of reverse fountain cytoplasmic streaming. However, after reaching the subapex, RabA4b-positive small vesicles run straight to the tip along cortical actin bundles within the “apical actin structure,” which leads to the accumulation of vesicles at the pollen tube tip. After reaching the extreme tip, some RabA4b-positive small vesicles will start to move toward the base of the pollen tube. The inner actin filaments within the “apical actin structure” function as physical barrier to prevent their return, which leads to the formation of the “V”-shaped distribution pattern of small vesicles.
Although the essential role of actin in regulating pollen tube growth is well-recognized, the cellular mechanisms underlying the function of actin during pollen tube growth remain to be uncovered. Our understanding of how actin performs its function has been hindered by the lack of a unified view about the origin, spatial organization and dynamics of actin filaments within the growth domain of pollen tubes. Recently, with the introduction of appropriate actin markers and state-of-the-art live cell imaging technologies, along with the usage of mutants lacking different ABPs, our understanding of the origin, polymerization, dynamics, and spatial organization of actin filaments within the growth domain of pollen tubes has improved substantially. Specifically, it is clear that actin filaments are continuously polymerized from the plasma membrane at the extreme apex of pollen tubes during their extension, which answers the long-standing question about whether actin filaments exist at the extreme apex. In addition, actin filaments are polymerized from the plasma membrane at the subapex, where they generate the actin fringe structure reported in pollen tubes (Lovy-Wheeler et al., 2005, 2006). This work provides insights into the origin, polarity and organization of actin filaments within the actin fringe. Together, these findings allow us to conclude that actin filaments within the apical and subapical regions of pollen tubes can be viewed as a whole in terms of their origin, and can be collectively defined as the “apical actin structure” (Qu et al., 2017). Consequently, the pollen tube actin cytoskeleton can be viewed as consisting of two structures: the shank-localized actin bundles and the “apical actin structure” (Figure 1G; Qu et al., 2017). The polymerization of actin filaments from the plasma membrane also occurs in lily and tobacco pollen tubes (Figures 1D,E; Rounds et al., 2014; Qu et al., 2017). This implies that the polymerization of actin filaments from the plasma membrane and formation of the distinct “apical actin structure” might represent a common design for angiosperm pollen tubes.
Careful observations revealed that membrane-originated actin filaments within the pollen tube growth domain assume distinct spatial distributions: they form comparatively thick actin bundles at the cortex and fine actin filaments extending toward the inner region of the cytoplasm (Figure 1G; Qu et al., 2017). The functional coordination of those spatially distinct apical and subapical actin filaments leads to the formation of a “V”-shaped vesicle distribution pattern (Figure 3C; Qu et al., 2017). In addition, the apical actin structure acts as a physical barrier to prevent the apical invasion of large organelles, which facilitates the generation of reverse fountain cytoplasmic streaming (Figure 3C; Qu et al., 2017). However, it remains to be resolved how subapical actin filaments coordinate spatially and functionally with shank-localized actin bundles. Furthermore, given that actin filaments are continuously generated from the plasma membrane at the extreme apex during pollen tube growth, it will definitely be worth exploring how those actin filaments might be involved in the control of exo- and endocytotic events.
As actin polymerization is concurrent with and required for pollen tube growth (Qu et al., 2017), a key area for future research is how growing pollen tubes perceive the upstream signals to control the polymerization and dynamics of actin filaments. Within this framework, another outstanding question is how the activity of membrane-anchored class I formins is precisely regulated. In particular, it will be interesting to investigate how the signaling mediated by ROPs (Li et al., 1999; Gu et al., 2005), phospholipids (Zhang and McCormick, 2010; Zonia, 2010) and the receptor-like kinases (RLKs) (Muschietti and Wengier, 2018) might influence the activity of formins. In particular, as ROPs and RLKs have been implicated in pollen tube guidance (Takeuchi and Higashiyama, 2016; Wang et al., 2016; Luo et al., 2017), it remains to be documented how actin reorganization is involved in the turning of pollen tubes in response to female-derived signals. Establishment of a semi-in vivo pollen tube growth system that enables the imaging of actin dynamics at high spatiotemporal resolution might allow us to understand how actin undergoes reorganization during pollen tube turning in response to female-derived attractants. Furthermore, pollen tubes have distinct distributions of ions, such as Ca2+ and H+ (HoldawayClarke et al., 1997; Messerli and Robinson, 1997; Feijo et al., 1999; Diao et al., 2018), which will influence the activity of ABPs and will in turn impact the dynamics and organization of actin filaments. How actin structures adapt to the cytosolic microenvironment at the pollen tube tip is another interesting question. In summary, plant biologists have made great progress in understanding the dynamics, organization and function of the actin cytoskeleton in pollen tube tips, but many questions still remain to be answered. This promises to be an exciting area of research for many years to come.
YX drafted this manuscript. SH conceived this manuscript and revised the writing of this manuscript. Both authors contributed to the article and approved the submitted version.
This work was supported by grants from the National Natural Science Foundation of China (31671390 and 31970180). The research in the Huang Lab was also supported by funding from Tsinghua-Peking Joint Center for Life Sciences.
The authors declare that the research was conducted in the absence of any commercial or financial relationships that could be construed as a potential conflict of interest.
We thank the members of the Huang lab for constructive comments.
Allwood, E. G., Anthony, R. G., Smertenko, A. P., Reichelt, S., Drobak, B. K., Doonan, J. H., et al. (2002). Regulation of the pollen-specific actin-depolymerizing factor LlADF1. Plant Cell 14, 2915–2927. doi: 10.1105/tpc.005363
Bao, C., Wang, J., Zhang, R., Zhang, B., Zhang, H., Zhou, Y., et al. (2012). Arabidopsis VILLIN2 and VILLIN3 act redundantly in sclerenchyma development via bundling of actin filaments. Plant J. 71, 962–975. doi: 10.1111/j.1365-313X.2012.05044.x
Bascom, C. S. Jr., Hepler, P. K., and Bezanilla, M. (2018). Interplay between ions, the cytoskeleton, and cell wall properties during tip growth. Plant Physiol. 176, 28–40. doi: 10.1104/pp.17.01466
Blanchoin, L., and Staiger, C. J. (2010). Plant formins: diverse isoforms and unique molecular mechanism. Biochim. Biophys. Acta 1803, 201–206. doi: 10.1016/j.bbamcr.2008.09.015
Bou Daher, F., van Oostende, C., and Geitmann, A. (2011). Spatial and temporal expression of actin depolymerizing factors ADF7 and ADF10 during male gametophyte development in Arabidopsis thaliana. Plant Cell Physiol. 52, 1177–1192. doi: 10.1093/pcp/pcr068
Cai, G., and Cresti, M. (2009). Organelle motility in the pollen tube: a tale of 20 years. J. Exp. Bot. 60, 495–508. doi: 10.1093/jxb/ern321
Cai, G., Parrotta, L., and Cresti, M. (2015). Organelle trafficking, the cytoskeleton, and pollen tube growth. J. Integr. Plant Biol. 57, 63–78. doi: 10.1111/jipb.12289
Cao, L., Henty-Ridilla, J. L., Blanchoin, L., and Staiger, C. J. (2016). Profilin-dependent nucleation and assembly of actin filaments controls cell elongation in Arabidopsis. Plant Physiol. 170, 220–233. doi: 10.1104/pp.15.01321
Cardenas, L., Lovy-Wheeler, A., Wilsen, K. L., and Hepler, P. K. (2005). Actin polymerization promotes the reversal of streaming in the apex of pollen tubes. Cell Motil. Cytoskeleton 61, 112–127. doi: 10.1002/cm.20068
Carlsson, L., Nystrom, L. E., Sundkvist, I., Markey, F., and Lindberg, U. (1977). Actin polymerizability is influenced by PROFILIN, a low-molecular weight protein in non-muscle cells. J. Mol. Biol. 115, 465–483. doi: 10.1016/0022-2836(77)90166-8
Chang, M., and Huang, S. (2015). Arabidopsis ACT11 modifies actin turnover to promote pollen germination and maintain the normal rate of tube growth. Plant J. 83, 515–527. doi: 10.1111/tpj.12910
Chang, M., Li, Z., and Huang, S. (2017). Monomeric G-actin is uniformly distributed in pollen tubes and is rapidly redistributed via cytoplasmic streaming during pollen tube growth. Plant J. 92, 509–519. doi: 10.1111/tpj.13668
Chapman, L. A., and Goring, D. R. (2010). Pollen-pistil interactions regulating successful fertilization in the Brassicaceae. J. Exp. Bot. 61, 1987–1999. doi: 10.1093/jxb/erq021
Chaudhry, F., Guerin, C., von Witsch, M., Blanchoin, L., and Staiger, C. J. (2007). Identification of Arabidopsis cyclase-associated protein 1 as the first nucleotide exchange factor for plant actin. Mol. Biol. Cell 18, 3002–3014. doi: 10.1091/mbc.e06-11-1041
Chebli, Y., Kroeger, J., and Geitmann, A. (2013). Transport logistics in pollen tubes. Mol. Plant 6, 1037–1052. doi: 10.1093/mp/sst073
Chen, C. Y., Wong, E. I., Vidali, L., Estavillo, A., Hepler, P. K., Wu, H. M., et al. (2002). The regulation of actin organization by actin-depolymerizing factor in elongating pollen tubes. Plant Cell 14, 2175–2190. doi: 10.1105/tpc.003038
Chen, N., Qu, X., Wu, Y., and Huang, S. (2009). Regulation of actin dynamics in pollen tubes: control of actin polymer level. J. Integr. Plant Biol. 51, 740–750. doi: 10.1111/j.1744-7909.2009.00850.x
Cheung, A. Y., Duan, Q. H., Costa, S. S., de Graaf, B. H., Di Stilio, V. S., Feijo, J., et al. (2008). The dynamic pollen tube cytoskeleton: live cell studies using actin-binding and microtubule-binding reporter proteins. Mol. Plant 1, 686–702. doi: 10.1093/mp/ssn026
Cheung, A. Y., Niroomand, S., Zou, Y., and Wu, H. M. (2010). A transmembrane formin nucleates subapical actin assembly and controls tip-focused growth in pollen tubes. Proc. Natl. Acad. Sci. U.S.A. 107, 16390–16395. doi: 10.1073/pnas.1008527107
Cheung, A. Y., and Wu, H. M. (2004). Overexpression of an Arabidopsis formin stimulates supernumerary actin cable formation from pollen tube cell membrane. Plant Cell 16, 257–269. doi: 10.1105/tpc.016550
Cheung, A. Y., and Wu, H.-M. (2008). Structural and signaling networks for the polar cell growth machinery in pollen tubes. Annu. Rev. Plant Biol. 59, 547–572. doi: 10.1146/annurev.arplant.59.032607.092921
Cole, R. A., and Fowler, J. E. (2006). Polarized growth: maintaining focus on the tip. Curr. Opin. Plant Biol. 9, 579–588. doi: 10.1016/j.pbi.2006.09.014
Cvrckova, F., Novotny, M., Pickova, D., and Zarsky, V. (2004). Formin homology 2 domains occur in multiple contexts in angiosperms. BMC Genomics 5:44. doi: 10.1186/1471-2164-5-44
Daher, F. B., and Geitmann, A. (2011). Actin is involved in pollen tube tropism through redefining the spatial targeting of secretory vesicles. Traffic 12, 1537–1551. doi: 10.1111/j.1600-0854.2011.01256.x
Daher, F. B., and Geitmann, A. (2012). Actin depolymerizing factors ADF7 and ADF10 play distinct roles during pollen development and pollen tube growth. Plant Signal. Behav. 7, 879–881. doi: 10.4161/psb.20436
Diao, M., Li, X., and Huang, S. J. (2020). Arabidopsis AIP1-1 regulates the organization of apical actin filaments by promoting their turnover in pollen tubes. Sci. China Life Sci. 63, 239–250. doi: 10.1007/s11427-019-9532-0
Diao, M., Qu, X., and Huang, S. (2018). Calcium imaging in Arabidopsis pollen cells using G-CaMP5. J. Integr. Plant Biol. 60, 897–906. doi: 10.1111/jipb.12642
Dong, H., Pei, W., and Ren, H. (2012). Actin fringe is correlated with tip growth velocity of pollen tubes. Mol. Plant 5, 1160–1162. doi: 10.1093/mp/sss073
Dresselhaus, T., Sprunck, S., and Wessel, G. M. (2016). Fertilization mechanisms in flowering plants. Curr. Biol. 26, R125–R139. doi: 10.1016/j.cub.2015.12.032
El-Din El-Assal, S., Le, J., Basu, D., Mallery, E. L., and Szymanski, D. B. (2004). DISTORTED2 encodes an ARPC2 subunit of the putative Arabidopsis ARP2/3 complex. Plant J. 38, 526–538. doi: 10.1111/j.1365-313X.2004.02065.x
Feijo, J. A., Sainhas, J., Hackett, G. R., Kunkel, J. G., and Hepler, P. K. (1999). Growing pollen tubes possess a constitutive alkaline band in the clear zone and a growth-dependent acidic tip. J. Cell Biol. 144, 483–496. doi: 10.1083/jcb.144.3.483
Franke, W. W., Herth, W., Vanderwoude, W. J., and Morre, D. J. (1972). Tubular and filamentous structures in pollen tubes: possible involvement as guide elements in protoplasmic streaming and vectorial migration of secretory vesicles. Planta 105, 317–341. doi: 10.1007/BF00386769
Fu, Y. (2015). The cytoskeleton in the pollen tube. Curr. Opin. Plant Biol. 28, 111–119. doi: 10.1016/j.pbi.2015.10.004
Fu, Y., Wu, G., and Yang, Z. B. (2001). Rop GTPase-dependent dynamics of tip-localized F-actin controls tip growth in pollen tubes. J. Cell Biol. 152, 1019–1032. doi: 10.1083/jcb.152.5.1019
Geitmann, A., and Emons, A. M. C. (2000). The cytoskeleton in plant and fungal cell tip growth. J. Microsc. 198, 218–245. doi: 10.1046/j.1365-2818.2000.00702.x
Geitmann, A., Snowman, B. N., Emons, A. M. C., and Franklin-Tong, V. E. (2000). Alterations in the actin cytoskeleton of pollen tubes are induced by the self-incompatibility reaction in Papaver rhoeas. Plant Cell 12, 1239–1251. doi: 10.1105/tpc.12.7.1239
Gibbon, B. C., Kovar, D. R., and Staiger, C. J. (1999). Latrunculin B has different effects on pollen germination and tube growth. Plant Cell 11, 2349–2363. doi: 10.1105/tpc.11.12.2349
Gibbon, B. C., Ren, H., and Staiger, C. J. (1997). Characterization of maize (Zea mays) pollen profilin function in vitro and in live cells. Biochem. J. 327(Pt 3), 909–915. doi: 10.1042/bj3270909
Gibbon, B. C., Zonia, L. E., Kovar, D. R., Hussey, P. J., and Staiger, C. J. (1998). Pollen profilin function depends on interaction with proline-rich motifs. Plant Cell 10, 981–993. doi: 10.1105/tpc.10.6.981
Goode, B. L., and Eck, M. J. (2007). Mechanism and function of formins in the control of actin assembly. Annu. Rev. Biochem. 76, 593–627. doi: 10.1146/annurev.biochem.75.103004.142647
Grunt, M., Zarsky, V., and Cvrckova, F. (2008). Roots of angiosperm formins: the evolutionary history of plant FH2 domain-containing proteins. BMC Evol. Biol. 8:115. doi: 10.1186/1471-2148-8-115
Gu, Y., Fu, Y., Dowd, P., Li, S. D., Vernoud, V., Gilroy, S., et al. (2005). A Rho family GTPase controls actin dynamics and tip growth via two counteracting downstream pathways in pollen tubes. J. Cell Biol. 169, 127–138. doi: 10.1083/jcb.200409140
Guan, Y., Guo, J., Li, H., and Yang, Z. (2013). Signaling in pollen tube growth: crosstalk, feedback, and missing links. Mol. Plant 6, 1053–1064. doi: 10.1093/mp/sst070
Hepler, P. K., Vidali, L., and Cheung, A. Y. (2001). Polarized cell growth in higher plants. Annu. Rev. Cell Dev. Biol. 17, 159–187. doi: 10.1146/annurev.cellbio.17.1.159
Herth, W., Franke, W. W., and Vanderwoude, W. J. (1972). Cytochalasin stops tip growth in plants. Naturwissenschaften 59, 38–39. doi: 10.1007/bf00594629
Heslopharrison, J., and Heslopharrison, Y. (1991). Restoration of movement and apical growth in the angiosperm pollen-tube following cytochalasin-induced paralysis. Philos. Trans. R. Soc. Lond. Ser. B Biol. Sci. 331, 225–235. doi: 10.1098/rstb.1991.0011
Higashiyama, T. (2018). Plant reproduction: autocrine machinery for the long journey of the pollen tube. Curr. Biol. 28, R266–R269. doi: 10.1016/j.cub.2018.01.067
Higashiyama, T., and Takeuchi, H. (2015). The mechanism and key molecules involved in pollen tube guidance. Annu. Rev. Plant Biol. 66, 393–413. doi: 10.1146/annurev-arplant-043014-115635
HoldawayClarke, T. L., Feijo, J. A., Hackett, G. R., Kunkel, J. G., and Hepler, P. K. (1997). Pollen tube growth and the intracellular cytosolic calcium gradient oscillate in phase while extracellular calcium influx is delayed. Plant Cell 9, 1999–2010. doi: 10.1105/tpc.9.11.1999
Huang, S., Blanchoin, L., Chaudhry, F., Franklin-Tong, V. E., and Staiger, C. J. (2004). A gelsolin-like protein from Papaver rhoeas pollen (PrABP80) stimulates calcium-regulated severing and depolymerization of actin filaments. J. Biol. Chem. 279, 23364–23375. doi: 10.1074/jbc.M312973200
Huang, S., Qu, X., and Zhang, R. (2015). Plant villins: versatile actin regulatory proteins. J. Integr. Plant Biol. 57, 40–49. doi: 10.1111/jipb.12293
Ingouff, M., Fitz Gerald, J. N., Guerin, C., Robert, H., Sorensen, M. B., Van Damme, D., et al. (2005). Plant formin AtFH5 is an evolutionarily conserved actin nucleator involved in cytokinesis. Nat. Cell Biol. 7, 374–380. doi: 10.1038/ncb1238
Jiang, Y., Chang, M., Lan, Y., and Huang, S. (2019). Mechanism of CAP1-mediated apical actin polymerization in pollen tubes. Proc. Natl. Acad. Sci. U.S.A. 116, 12084–12093. doi: 10.1073/pnas.1821639116
Jiang, Y., Wang, J., Xie, Y., Chen, N., and Huang, S. (2017). ADF10 shapes the overall organization of apical actin filaments by promoting their turnover and ordering in pollen tubes. J. Cell Sci. 130, 3988–4001. doi: 10.1242/jcs.207738
Johnson, M. A., Harper, J. F., and Palanivelu, R. (2019). A fruitful journey: pollen tube navigation from germination to fertilization. Annu. Rev. Plant Biol. 70, 809–837. doi: 10.1146/annurev-arplant-050718-100133
Kaul, S., Koo, H. L., Jenkins, J., Rizzo, M., Rooney, T., Tallon, L. J., et al. (2000). Analysis of the genome sequence of the flowering plant Arabidopsis thaliana. Nature 408, 796–815. doi: 10.1038/35048692
Khurana, P., Henty, J. L., Huang, S., Staiger, A. M., Blanchoin, L., and Staiger, C. J. (2010). Arabidopsis VILLIN1 and VILLIN3 have overlapping and distinct activities in actin bundle formation and turnover. Plant Cell 22, 2727–2748. doi: 10.1105/tpc.110.076240
Klahre, U., Friederich, E., Kost, B., Louvard, D., and Chua, N. H. (2000). Villin-like actin-binding proteins are expressed ubiquitously in Arabidopsis. Plant Physiol. 122, 35–47. doi: 10.1104/pp.122.1.35
Kost, B., Spielhofer, P., and Chua, N. H. (1998). A GFP-mouse talin fusion protein labels plant actin filaments in vivo and visualizes the actin cytoskeleton in growing pollen tubes. Plant J. 16, 393–401. doi: 10.1046/j.1365-313x.1998.00304.x
Kovar, D. R. (2006). Molecular details of formin-mediated actin assembly. Curr. Opin. Cell Biol. 18, 11–17. doi: 10.1016/j.ceb.2005.12.011
Kovar, D. R., Drobak, B. K., and Staiger, C. J. (2000). Maize profilin isoforms are functionally distinct. Plant Cell 12, 583–598. doi: 10.1105/tpc.12.4.583
Kovar, D. R., Harris, E. S., Mahaffy, R., Higgs, H. N., and Pollard, T. D. (2006). Control of the assembly of ATP- and ADP-actin by formins and profilin. Cell 124, 423–435. doi: 10.1016/j.cell.2005.11.038
Kovar, D. R., Yang, P., Sale, W. S., Drobak, B. K., and Staiger, C. J. (2001). Chlamydomonas reinhardtii produces a profilin with unusual biochemical properties. J. Cell Sci. 114(Pt 23), 4293–4305.
Kroeger, J. H., Daher, F. B., Grant, M., and Gieitmann, A. (2009). Microfilament orientation constrains vesicle flow and spatial distribution in growing pollen tubes. Biophys. J. 97, 1822–1831. doi: 10.1016/j.bpj.2009.07.038
Lan, Y., Liu, X., Fu, Y., and Huang, S. (2018). Arabidopsis class I formins control membrane-originated actin polymerization at pollen tube tips. PLoS Genet. 14:e1007789. doi: 10.1371/journal.pgen.1007789
Le, J., El-Assal Sel, D., Basu, D., Saad, M. E., and Szymanski, D. B. (2003). Requirements for Arabidopsis ATARP2 and ATARP3 during epidermal development. Curr. Biol. 13, 1341–1347. doi: 10.1016/s0960-9822(03)00493-7
Lenartowska, M., and Michalska, A. (2008). Actin filament organization and polarity in pollen tubes revealed by myosin II subfragment 1 decoration. Planta 228, 891–896. doi: 10.1007/s00425-008-0802-5
Li, H., Lin, Y. K., Heath, R. M., Zhu, M. X., and Yang, Z. B. (1999). Control of pollen tube tip growth by a pop GTPase-dependent pathway that leads to tip-localized calcium influx. Plant Cell 11, 1731–1742. doi: 10.1105/tpc.11.9.1731
Li, S., Blanchoin, L., Yang, Z., and Lord, E. M. (2003). The putative Arabidopsis arp2/3 complex controls leaf cell morphogenesis. Plant Physiol. 132, 2034–2044. doi: 10.1104/pp.103.028563
Li, S., Dong, H., Pei, W., Liu, C., Zhang, S., Sun, T., et al. (2017). LlFH1-mediated interaction between actin fringe and exocytic vesicles is involved in pollen tube tip growth. New Phytol. 214, 745–761. doi: 10.1111/nph.14395
Li, Y., Zee, S. Y., Liu, Y. M., Huang, B. Q., and Yen, L. F. (2001). Circular F-actin bundles and a G-actin gradient in pollen and pollen tubes of Lilium davidii. Planta 213, 722–730. doi: 10.1007/s004250100543
Liu, X., Qu, X., Jiang, Y., Chang, M., Zhang, R., Wu, Y., et al. (2015). Profilin regulates apical actin polymerization to control polarized pollen tube growth. Mol. Plant 8, 1694–1709. doi: 10.1016/j.molp.2015.09.013
Liu, X., and Yen, L. F. (1992). Purification and characterization of actin from maize pollen. Plant Physiol. 99, 1151–1155. doi: 10.1104/pp.99.3.1151
Lovy-Wheeler, A., Kunkel, J. G., Allwood, E. G., Hussey, P. J., and Hepler, P. K. (2006). Oscillatory increases in alkalinity anticipate growth and may regulate actin dynamics in pollen tubes of lily. Plant Cell 18, 2182–2193. doi: 10.1105/tpc.106.044867
Lovy-Wheeler, A., Wilsen, K. L., Baskin, T. I., and Hepler, P. K. (2005). Enhanced fixation reveals the apical cortical fringe of actin filaments as a consistent feature of the pollen tube. Planta 221, 95–104. doi: 10.1007/s00425-004-1423-2
Luo, N., Yan, A., Liu, G., Guo, J., Rong, D., Kanaoka, M. M., et al. (2017). Exocytosis-coordinated mechanisms for tip growth underlie pollen tube growth guidance. Nat. Commun. 8:1687.
Mascarenhas, J. P. (1993). Molecular mechanism of pollen-tube growth and differentiation. Plant Cell 5, 1303–1314. doi: 10.1105/tpc.5.10.1303
Mascarenhas, J. P., and Lafountain, J. (1972). Protoplasmic streaming, cytochalasin B, and growth of the pollen tube. Tissue Cell 4, 11–14. doi: 10.1016/s0040-8166(72)80002-8
Mathur, J., Mathur, N., Kernebeck, B., and Hulskamp, M. (2003a). Mutations in actin-related proteins 2 and 3 affect cell shape development in Arabidopsis. Plant Cell 15, 1632–1645. doi: 10.1105/tpc.011676
Mathur, J., Mathur, N., Kirik, V., Kernebeck, B., Srinivas, B. P., and Hulskamp, M. (2003b). Arabidopsis CROOKED encodes for the smallest subunit of the ARP2/3 complex and controls cell shape by region specific fine F-actin formation. Development 130, 3137–3146. doi: 10.1242/dev.00549
Messerli, M., and Robinson, K. R. (1997). Tip localized Ca2+ pulses are coincident with peak pulsatile growth rates in pollen tubes of Lilium longiflorum. J. Cell Sci. 110(Pt 11), 1269–1278.
Muschietti, J. P., and Wengier, D. L. (2018). How many receptor-like kinases are required to operate a pollen tube. Curr. Opin. Plant Biol. 41, 73–82. doi: 10.1016/j.pbi.2017.09.008
Pantaloni, D., and Carlier, M. F. (1993). How profilin promotes actin filament assembly in the presence of thymosin beta 4. Cell 75, 1007–1014. doi: 10.1016/0092-8674(93)90544-z
Papuga, J., Hoffmann, C., Dieterle, M., Moes, D., Moreau, F., Tholl, S., et al. (2010). Arabidopsis LIM proteins: a family of actin bundlers with distinct expression patterns and modes of regulation. Plant Cell 22, 3034–3052. doi: 10.1105/tpc.110.075960
Pawloski, L. C., Kandasamy, M. K., and Meagher, R. B. (2006). The late pollen actins are essential for normal male and female development in Arabidopsis. Plant Mol. Biol. 62, 881–896. doi: 10.1007/s11103-006-9063-5
Qin, T., Liu, X., Li, J., Sun, J., Song, L., and Mao, T. (2014). Arabidopsis microtubule-destabilizing protein 25 functions in pollen tube growth by severing actin filaments. Plant Cell 26, 325–339. doi: 10.1105/tpc.113.119768
Qin, Y., and Yang, Z. (2011). Rapid tip growth: insights from pollen tubes. Semin. Cell Dev. Biol. 22, 816–824. doi: 10.1016/j.semcdb.2011.06.004
Qu, X., Jiang, Y., Chang, M., Liu, X., Zhang, R., and Huang, S. (2015). Organization and regulation of the actin cytoskeleton in the pollen tube. Front. Plant Sci. 5:786. doi: 10.3389/fpls.2014.00786
Qu, X., Wang, Q., Wang, H., and Huang, S. (2020). Visualization of actin organization and quantification in fixed Arabidopsis pollen grains and tubes. Bio Protoc. 10:e3509. doi: 10.21769/BioProtoc.3509
Qu, X., Zhang, H., Xie, Y., Wang, J., Chen, N., and Huang, S. (2013). Arabidopsis villins promote actin turnover at pollen tube tips and facilitate the construction of actin collars. Plant Cell 25, 1803–1817. doi: 10.1105/tpc.113.110940
Qu, X., Zhang, R., Zhang, M., Diao, M., Xue, Y., and Huang, S. (2017). Organizational innovation of apical actin filaments drives rapid pollen tube growth and turning. Mol. Plant 10, 930–947. doi: 10.1016/j.molp.2017.05.002
Ren, H., and Xiang, Y. (2007). The function of actin-binding proteins in pollen tube growth. Protoplasma 230, 171–182. doi: 10.1007/s00709-006-0231-x
Ren, H. Y., Gibbon, B. C., Ashworth, S. L., Sherman, D. M., Yuan, M., and Staiger, C. J. (1997). Actin purified from maize pollen functions in living plant cells. Plant Cell 9, 1445–1457. doi: 10.1105/tpc.9.8.1445
Rizvi, S. A., Neidt, E. M., Cui, J., Feiger, Z., Skau, C. T., Gardel, M. L., et al. (2009). Identification and characterization of a small molecule inhibitor of formin-mediated actin assembly. Chem. Biol. 16, 1158–1168. doi: 10.1016/j.chembiol.2009.10.006
Romero, S., Le Clainche, C., Didry, D., Egile, C., Pantaloni, D., and Carlier, M. F. (2004). Formin is a processive motor that requires profilin to accelerate actin assembly and associated ATP hydrolysis. Cell 119, 419–429. doi: 10.1016/j.cell.2004.09.039
Rotty, J. D., Wu, C., Haynes, E. M., Suarez, C., Winkelman, J. D., Johnson, H. E., et al. (2015). Profilin-1 serves as a gatekeeper for actin assembly by Arp2/3-dependent and -independent pathways. Dev. Cell 32, 54–67. doi: 10.1016/j.devcel.2014.10.026
Rounds, C. M., and Bezanilla, M. (2013). “Growth mechanisms in tip-growing plant cells,” in Annual Review of Plant Biology, Vol. 64, ed. S. S. Merchant (Palo Alto, CA: Annual Reviews), 243–265. doi: 10.1146/annurev-arplant-050312-120150
Rounds, C. M., Hepler, P. K., and Winship, L. J. (2014). The apical actin fringe contributes to localized cell wall deposition and polarized growth in the Lily pollen tube. Plant Physiol. 166, 139–151. doi: 10.1104/pp.114.242974
Samaj, J., Muller, J., Beck, M., Bohm, N., and Menzel, D. (2006). Vesicular trafficking, cytoskeleton and signalling in root hairs and pollen tubes. Trends Plant Sci. 11, 594–600. doi: 10.1016/j.tplants.2006.10.002
Shi, M., Xie, Y., Zheng, Y., Wang, J., Su, Y., Yang, Q., et al. (2013). Oryza sativa actin-interacting protein 1 is required for rice growth by promoting actin turnover. Plant J. 73, 747–760. doi: 10.1111/tpj.12065
Smertenko, A. P., Allwood, E. G., Khan, S., Jiang, C. J., Maciver, S. K., Weeds, A. G., et al. (2001). Interaction of pollen-specific actin-depolymerizing factor with actin. Plant J. 25, 203–212. doi: 10.1046/j.1365-313x.2001.00954.x
Snowman, B. N., Kovar, D. R., Shevchenko, G., Franklin-Tong, V. E., and Staiger, C. J. (2002). Signal-mediated depolymerization of actin in pollen during the self-incompatibility response. Plant Cell 14, 2613–2626. doi: 10.1105/tpc.002998
Speranza, A., and Calzoni, G. L. (1989). Cytochalasin-B affects protein secretion in germinating pollen of malus-domestica borkh. J. Plant Physiol. 133, 719–726. doi: 10.1016/s0176-1617(89)80079-3
Staiger, C. J., and Blanchoin, L. (2006). Actin dynamics: old friends with new stories. Curr. Opin. Plant Biol. 9, 554–562. doi: 10.1016/j.pbi.2006.09.013
Staiger, C. J., Poulter, N. S., Henty, J. L., Franklin-Tong, V. E., and Blanchoin, L. (2010). Regulation of actin dynamics by actin-binding proteins in pollen. J. Exp. Bot. 61, 1969–1986. doi: 10.1093/jxb/erq012
Staiger, C. J., Yuan, M., Valenta, R., Shaw, P. J., Warn, R. M., and Lloyd, C. W. (1994). Microinjected profilin affects cytoplasmic streaming in plant cells by rapidly depolymerizing actin microfilaments. Curr. Biol. 4, 215–219. doi: 10.1016/s0960-9822(00)00050-6
Stephan, O., Cottier, S., Fahlen, S., Montes-Rodriguez, A., Sun, J., Eklund, D. M., et al. (2014). RISAP is a TGN-associated RAC5 effector regulating membrane traffic during polar cell growth in Tobacco. Plant Cell 26, 4426–4447. doi: 10.1105/tpc.114.131078
Stephan, O. O. H. (2017). Actin fringes of polar cell growth. J. Exp. Bot. 68, 3303–3320. doi: 10.1093/jxb/erx195
Su, H., Zhu, J., Cai, C., Pei, W., Wang, J., Dong, H., et al. (2012). FIMBRIN1 is involved in lily pollen tube growth by stabilizing the actin fringe. Plant Cell 24, 4539–4554. doi: 10.1105/tpc.112.099358
Suarez, C., Carroll, R. T., Burke, T. A., Christensen, J. R., Bestul, A. J., Sees, J. A., et al. (2015). Profilin regulates F-actin network homeostasis by favoring formin over Arp2/3 complex. Dev. Cell 32, 43–53. doi: 10.1016/j.devcel.2014.10.027
Szymanski, D. B. (2005). Breaking the WAVE complex: the point of Arabidopsis trichomes. Curr. Opin. Plant Biol. 8, 103–112. doi: 10.1016/j.pbi.2004.11.004
Takeuchi, H., and Higashiyama, T. (2016). Tip-localized receptors control pollen tube growth and LURE sensing in Arabidopsis. Nature 531, 245–248. doi: 10.1038/nature17413
Tang, X. J., Lancelle, S. A., and Hepler, P. K. (1989). Fluorescence microscopic localization of actin in pollen tubes: comparison of actin antibody and phalloidin staining. Cell Motil. Cytoskeleton 12, 216–224. doi: 10.1002/cm.970120404
Taylor, L. P., and Hepler, P. K. (1997). Pollen germination and tube growth. Annu. Rev. Plant Physiol. Plant Mol. Biol. 48, 461–491.
Thomas, S. G., Huang, S., Li, S., Staiger, C. J., and Franklin-Tong, V. E. (2006). Actin depolymerization is sufficient to induce programmed cell death in self-incompatible pollen. J. Cell Biol. 174, 221–229. doi: 10.1083/jcb.200604011
Tominaga, M., Yokota, E., Vidali, L., Sonobe, S., Hepler, P. K., and Shimmen, T. (2000). The role of plant villin in the organization of the actin cytoskeleton, cytoplasmic streaming and the architecture of the transvacuolar strand in root hair cells of Hydrocharis. Planta 210, 836–843. doi: 10.1007/s004250050687
van Gisbergen, P. A. C., and Bezanilla, M. (2013). Plant formins: membrane anchors for actin polymerization. Trends Cell Biol. 23, 227–233. doi: 10.1016/j.tcb.2012.12.001
Vidali, L., and Hepler, P. K. (1997). Characterization and localization of profilin in pollen grains and tubes of Lilium longiflorum. Cell Motil. Cytoskeleton 36, 323–338. doi: 10.1002/(sici)1097-0169(1997)36:4<323::aid-cm3>3.0.co;2-6
Vidali, L., and Hepler, P. K. (2001). Actin and pollen tube growth. Protoplasma 215, 64–76. doi: 10.1007/bf01280304
Vidali, L., McKenna, S. T., and Hepler, P. K. (2001). Actin polymerization is essential for pollen tube growth. Mol. Biol. Cell 12, 2534–2545. doi: 10.1091/mbc.12.8.2534
Vidali, L., Rounds, C. M., Hepler, P. K., and Bezanilla, M. (2009). Lifeact-mEGFP reveals a dynamic apical F-actin network in tip growing plant cells. PLoS One 4:e5744. doi: 10.1371/journal.pone.0005744
Wang, H. J., Wan, A. R., and Jauh, G. Y. (2008). An actin-binding protein, LlLIM1, mediates calcium and hydrogen regulation of actin dynamics in pollen tubes. Plant Physiol. 147, 1619–1636. doi: 10.1104/pp.108.118604
Wang, T., Liang, L., Xue, Y., Jia, P.-F., Chen, W., Zhang, M.-X., et al. (2016). A receptor heteromer mediates the male perception of female attractants in plants. Nature 531, 241–244. doi: 10.1038/nature16975
Wang, T., Xiang, Y., Hou, J., and Ren, H. Y. (2008). ABP41 is involved in the pollen tube development via fragmenting actin filaments. Mol. Plant 1, 1048–1055. doi: 10.1093/mp/ssn073
Wilsen, K. L., Lovy-Wheeler, A., Voigt, B., Menzel, D., Kunkel, J. G., and Hepler, P. K. (2006). Imaging the actin cytoskeleton in growing pollen tubes. Sex. Plant Reprod. 19, 51–62. doi: 10.1007/s00497-006-0021-9
Wu, S., Xie, Y., Zhang, J., Ren, Y., Zhang, X., Wang, J., et al. (2015). VLN2 regulates plant architecture by affecting microfilament dynamics and polar auxin transport in Rice. Plant Cell 27, 2829–2845. doi: 10.1105/tpc.15.00581
Wu, Y., Yan, J., Zhang, R., Qu, X., Ren, S., Chen, N., et al. (2010). Arabidopsis FIMBRIN5, an actin bundling factor, is required for pollen germination and pollen tube growth. Plant Cell 22, 3745–3763. doi: 10.1105/tpc.110.080283
Xiang, Y., Huang, X., Wang, T., Zhang, Y., Liu, Q., Hussey, P. J., et al. (2007). ACTIN BINDING PROTEIN 29 from Lilium pollen plays an important role in dynamic actin remodeling. Plant Cell 19, 1930–1946. doi: 10.1105/tpc.106.048413
Yamashiro, S., Kameyama, K., Kanzawa, N., Tamiya, T., Mabuchi, I., and Tsuchiya, T. (2001). The gelsolin/fragmin family protein identified in the higher plant Mimosa pudica. J. Biochem. 130, 243–249. doi: 10.1093/oxfordjournals.jbchem.a002978
Yang, Z. (2008). Cell polarity signaling in Arabidopsis. Annu. Rev. Cell Dev. Biol. 24, 551–575. doi: 10.1146/annurev.cellbio.23.090506.123233
Ye, J., Zheng, Y., Yan, A., Chen, N., Wang, Z., Huang, S., et al. (2009). Arabidopsis formin3 directs the formation of actin cables and polarized growth in pollen tubes. Plant Cell 21, 3868–3884. doi: 10.1105/tpc.109.068700
Yokota, E., Takahara, K., and Shimmen, T. (1998). Actin-bundling protein isolated from pollen tubes of lily - biochemical and immunocytochemical characterization. Plant Physiol. 116, 1421–1429. doi: 10.1104/pp.116.4.1421
Yokota, E., Tominaga, M., Mabuchi, I., Tsuji, Y., Staiger, C. J., Oiwa, K., et al. (2005). Plant villin, lily P-135-ABP, possesses G-actin binding activity and accelerates the polymerization and depolymerization of actin in a Ca2+-sensitive manner. Plant Cell Physiol. 46, 1690–1703. doi: 10.1093/pcp/pci185
Yokota, E., Vidali, L., Tominaga, M., Tahara, H., Orii, H., Morizane, Y., et al. (2003). Plant 115-kDa actin-filament bundling protein, P-115-ABP, is a homologue of plant villin and is widely distributed in cells. Plant Cell Physiol. 44, 1088–1099. doi: 10.1093/pcp/pcg132
Zhang, H., Qu, X., Bao, C., Khurana, P., Wang, Q., Xie, Y., et al. (2010). Arabidopsis VILLIN5, an actin filament bundling and severing protein, is necessary for normal pollen tube growth. Plant Cell 22, 2749–2767. doi: 10.1105/tpc.110.076257
Zhang, J., Huang, Q., Zhong, S., Bleckmann, A., Huang, J., Guo, X., et al. (2017). Sperm cells are passive cargo of the pollen tube in plant fertilization. Nat. Plants 3:17079. doi: 10.1038/nplants.2017.79
Zhang, M., Zhang, R., Qu, X., and Huang, S. (2016). Arabidopsis FIM5 decorates apical actin filaments and regulates their organization in the pollen tube. J. Exp. Bot. 67, 3407–3417. doi: 10.1093/jxb/erw160
Zhang, R., Qu, X., Zhang, M., Jiang, Y., Dai, A., Zhao, W., et al. (2019). The balance between actin-bundling factors controls actin architecture in pollen tubes. iScience 16, 162–176. doi: 10.1016/j.isci.2019.05.026
Zhang, Y., He, J., Lee, D., and McCormick, S. (2010). Interdependence of endomembrane trafficking and actin dynamics during polarized growth of Arabidopsis pollen tubes. Plant Physiol. 152, 2200–2210. doi: 10.1104/pp.109.142349
Zhang, Y., He, J., and McCormick, S. (2009). Two Arabidopsis AGC kinases are critical for the polarized growth of pollen tubes. Plant J. 58, 474–484. doi: 10.1111/j.1365-313X.2009.03792.x
Zhang, Y., and McCormick, S. (2010). The regulation of vesicle trafficking by small GTPases and phospholipids during pollen tube growth. Sex. Plant Reprod. 23, 87–93. doi: 10.1007/s00497-009-0118-z
Zhang, Y., Xiao, Y., Du, F., Cao, L., Dong, H., and Ren, H. (2011). Arabidopsis VILLIN4 is involved in root hair growth through regulating actin organization in a Ca2+-dependent manner. New Phytol. 190, 667–682. doi: 10.1111/j.1469-8137.2010.03632.x
Zhao, W., Qu, X., Zhuang, Y., Wang, L., Bosch, M., Franklin-Tong, V. E., et al. (2020). Villin controls the formation and enlargement of punctate actin foci in pollen tubes. J. Cell Sci. 133:jcs237404. doi: 10.1242/jcs.237404
Zheng, Y., Xie, Y., Jiang, Y., Qu, X., and Huang, S. (2013). Arabidopsis actin-depolymerizing factor7 severs actin filaments and regulates actin cable turnover to promote normal pollen tube growth. Plant Cell 25, 3405–3423. doi: 10.1105/tpc.113.117820
Zhou, Z., Shi, H., Chen, B., Zhang, R., Huang, S., and Fu, Y. (2015). Arabidopsis RIC1 severs actin filaments at the apex to regulate pollen tube growth. Plant Cell 27, 1140–1161. doi: 10.1105/tpc.114.135400
Zhu, J., Nan, Q., Qin, T., Qian, D., Mao, T., Yuan, S., et al. (2017). Higher-ordered actin structures remodeled by Arabidopsis ACTIN-DEPOLYMERIZING FACTOR5 are important for pollen germination and pollen tube growth. Mol. Plant 10, 1065–1081. doi: 10.1016/j.molp.2017.06.001
Zhu, L., Zhang, Y., Kang, E., Xu, Q., Wang, M., Rui, Y., et al. (2013). MAP18 regulates the direction of pollen tube growth in Arabidopsis by modulating F-actin organization. Plant Cell 25, 851–867. doi: 10.1105/tpc.113.110528
Keywords: pollen tube growth, cytoplasmic streaming, actin dynamics, apical actin structure, actin-binding proteins, formin, ADF, villin
Citation: Xu Y and Huang S (2020) Control of the Actin Cytoskeleton Within Apical and Subapical Regions of Pollen Tubes. Front. Cell Dev. Biol. 8:614821. doi: 10.3389/fcell.2020.614821
Received: 07 October 2020; Accepted: 13 November 2020;
Published: 03 December 2020.
Edited by:
Yi Zhang, Beijing Normal University, ChinaCopyright © 2020 Xu and Huang. This is an open-access article distributed under the terms of the Creative Commons Attribution License (CC BY). The use, distribution or reproduction in other forums is permitted, provided the original author(s) and the copyright owner(s) are credited and that the original publication in this journal is cited, in accordance with accepted academic practice. No use, distribution or reproduction is permitted which does not comply with these terms.
*Correspondence: Shanjin Huang, c2podWFuZ0BtYWlsLnRzaW5naHVhLmVkdS5jbg==
Disclaimer: All claims expressed in this article are solely those of the authors and do not necessarily represent those of their affiliated organizations, or those of the publisher, the editors and the reviewers. Any product that may be evaluated in this article or claim that may be made by its manufacturer is not guaranteed or endorsed by the publisher.
Research integrity at Frontiers
Learn more about the work of our research integrity team to safeguard the quality of each article we publish.