- 1Department of Neurology, Center for Life Science, Beth Israel Deaconess Medical Center, Harvard Medical School, Boston, MA, United States
- 2Pharmaceutical Research Institute, Albany College of Pharmacy and Health Sciences, Albany, NY, United States
- 3Albany Medical College, Albany, NY, United States
- 4Taipei Cancer Center, Taipei Medical University, Taipei, Taiwan
- 5Graduate Institute of Cancer Biology and Drug Discovery, College of Medical Science and Technology, Taipei Medical University, Taipei, Taiwan
- 6Traditional Herbal Medicine Research Center of Taipei Medical University Hospital, Taipei Medical University, Taipei, Taiwan
- 7TMU Research Center of Cancer Translational Medicine, Taipei Medical University, Taipei, Taiwan
- 8Department of Sciences, University “Roma Tre,” Rome, Italy
- 9Department of Biology, University of Rome Tor Vergata, Rome, Italy
- 10Department of Experimental Medicine, University “La Sapienza,” Rome, Italy
- 11National Institute of Gastroenterology, Istituto di Ricovero e Cura a Carattere Scientifico (IRCCS) “S. de Bellis” Research Hospital, Castellana Grotte, Italy
The interdependence between thyroid hormones (THs), namely, thyroxine and triiodothyronine, and immune system is nowadays well-recognized, although not yet fully explored. Synthesis, conversion to a bioactive form, and release of THs in the circulation are events tightly supervised by the hypothalamic–pituitary–thyroid (HPT) axis. Newly synthesized THs induce leukocyte proliferation, migration, release of cytokines, and antibody production, triggering an immune response against either sterile or microbial insults. However, chronic patho-physiological alterations of the immune system, such as infection and inflammation, affect HPT axis and, as a direct consequence, THs mechanism of action. Herein, we revise the bidirectional crosstalk between THs and immune cells, required for the proper immune system feedback response among diverse circumstances. Available circulating THs do traffic in two distinct ways depending on the metabolic condition. Mechanistically, internalized THs form a stable complex with their specific receptors, which, upon direct or indirect binding to DNA, triggers a genomic response by activating transcriptional factors, such as those belonging to the Wnt/β-catenin pathway. Alternatively, THs engage integrin αvβ3 receptor on cell membrane and trigger a non-genomic response, which can also signal to the nucleus. In addition, we highlight THs-dependent inflammasome complex modulation and describe new crucial pathways involved in microRNA regulation by THs, in physiological and patho-physiological conditions, which modify the HPT axis and THs performances. Finally, we focus on the non-thyroidal illness syndrome in which the HPT axis is altered and, in turn, affects circulating levels of active THs as reported in viral infections, particularly in immunocompromised patients infected with human immunodeficiency virus.
Introduction
Thyrotropin-releasing hormone (TRH) and thyroid-stimulating hormone (TSH) produced by the hypothalamus and pituitary gland, respectively, are effectors of the hypothalamic–pituitary–thyroid (HPT) axis, which regulates levels of circulating thyroid hormones (THs; Kelly, 2000). TRH induces TSH release that, once in circulation, stimulates THs biosynthesis and maturation, events that take place in the thyroid. The bioactive form of THs, namely 3,5,3′-triiodo-L-thyronine (T3; Incerpi et al., 2016), in turn, acts via a negative feedback loop to control the hypothalamic–pituitary component of the HPT axis (Kelly, 2000). T3 results from deiodination of thyroxine (T4) by deiodinase (DIO) 1 and 2 enzymes, while DIO 3 activity converts T4 in reverse T3 (rT3), an inert isomer of T3 (Incerpi et al., 2016; Lanni et al., 2016). T3 and T4 may enter into the target cells through specific transporters (Hennemann et al., 2001) and act by binding to different molecules located either on plasma membrane (i.e., integrin αvβ3; Bergh et al., 2005; Davis et al., 2005; De Vito et al., 2011) or intracellularly (i.e., THα and THβ receptors: THRs; Cheng et al., 2010; Brent, 2012; Incerpi et al., 2016). These interactions activate a variety of pathways that largely signal to the nucleus (Flamant et al., 2017), or the nuclear transcription machinery by directly activating THs response elements (TREs) on gene promoters (Singh et al., 2018). T3 shows higher affinity than T4 for THRs, whereas T4 is more potent than T3 in binding integrin avβ3. Both these receptors activate signaling molecules such as phosphoinositide 3-phosphate kinase (PI3K), protein kinase B (AKT), and mitogen-activated protein kinases (MAPKs; Incerpi et al., 2016; Lanni et al., 2016; Davis et al., 2019).
The immune system can also affect THs synthesis and release, either centrally (from thyroid gland), or peripherally, from tissues or target organs. Here, we review recent findings on how THs and the immune system crosstalk. In particular, we will focus on the THs-dependent regulation of (1) Nod-like receptor protein 3 (NLRP3)-mediated inflammasome, (2) small non-coding RNAs such as microRNAs (miRNAs; Anastasiadou et al., 2018a,b), and (3) Wnt/β-catenin pathway in anti- or pro-inflammatory conditions, such as non-thyroidal illness syndrome (NTIS) and (4) during chronic viral infections, such as those caused by human immunodeficiency virus (HIV).
THs and Immune System: A Bidirectional Crosstalk
The existence of a bidirectional crosstalk between the endocrine and the immune system, in which THs and cytokines represent the key players, is well documented (Klecha et al., 2000, 2008; De Vito et al., 2011). Interestingly, immune cells’ reactivity to circulating THs (De Vito et al., 2011, 2012) as well as responsiveness of endocrine cells to available cytokines, such as interleukin-1 (IL-1), IL-6, interferon (IFN)-γ, and tumor necrosis factor-α (TFN-α), positively correlate with the expression of these molecules and to the affinity for their specific receptors (Klecha et al., 2000, 2008). A central role of THs in the modulation of immune system is confirmed by the influence of T3 and T4 in cytokine maturation and release, a process that involves the activation of MAPKs and mediated by phosphorylation of the Signal Transducer and Activator of Transcription 1α (STAT1α; Lin et al., 1999; Shih et al., 2004).
Abnormal THs secretion, hyperthyroidism, autoimmune thyroiditis, and hypothyroidism can affect immunological functions. Hyperthyroidism correlates with increased humoral and immune cell responses (De Vito et al., 2011). Opposite effects were found in hypothyroidism (Klecha et al., 2008). Moreover, levels of circulating THs positively match up with an immunological reactivity in healthy individuals, such as in physiological maintenance of lymphocyte subpopulations (Hodkinson et al., 2009). Recently, it has been shown that T3 increased the number of IL-17-expressing T lymphocytes by activating dendritic cells, in vitro (Alamino et al., 2019). In addition, T and B lymphocytes are capable of synthesizing and releasing TSH (Smith et al., 1983; Harbour et al., 1989), which might affect healthy and abnormal thyroid cells, expressing the TSH receptor. This novel and unexpected non-pituitary source of TSH could be also decisive in affecting immune response during infections and chronic inflammation (Klein, 2006). Initial reports of TSH and immune cells appeared more than 20 years ago (Smith et al., 1983; Kruger and Blalock, 1986). Bacterial toxins (Smith et al., 1983) or in vitro TRH administration (Klein, 2006) enhance TSH production and release from leukocytes. The work of Blalock et al. (1984) showed that TSH induced a strong cellular and humoral response, thus enhancing the lymphocyte proliferation by inducing the production of endogenous inflammatory factors: IL-6 and monocyte chemoattractant protein-1 (MCP-1; Gagnon et al., 2014). Moreover, in vitro and in vivo studies showed that TSH treatments significantly increased T3 levels in thymocytes and other immune cells (Csaba and Pállinger, 2009). Experiments performed in mice lacking the pituitary gland (unable to produce central TSH) showed increased THs levels during inflammation (Bagriacik et al., 2001). Conversely, unbalanced immune response may be linked to low levels of THs in the plasma, since TSH fluctuations might alter T3 and T4 release from thyroid gland. Moreover, acute infections indirectly influence THs release through the action of inflammatory molecules (like IL-1, IL-6, and TFN-α) on hypothalamus, thus minimizing TSH action on the thyroid and, consequently, reducing T3 and T4 in the circulation, promoting NTIS. This lowers the energy expenditure during illnesses, offering an alternative pathway to the HPT axis control, for central neuroendocrine–immune and metabolic fine-tuning (Klein, 2006). However, induction and regulation of NTIS may involve alterations in the HPT axis and may be relatively independent of circulating THs (de Vries et al., 2015).
The T3 and T4 are also involved in the regulation of reactive oxygen species (ROS) production through the activation of the PI3K–AKT axis in immune cells (De Vito et al., 2011; Figure 1, right panel). Moderate levels of ROS could act as a second messenger and play an important role in the leukocyte activation during immune surveillance and phlogosis (Figure 1, left panel). This process, together with actin polymerization induced by T4 and rT3, may contribute to the immune cell migration and proliferation at the sites of inflammation (Marino et al., 2006; De Vito et al., 2011, 2012).
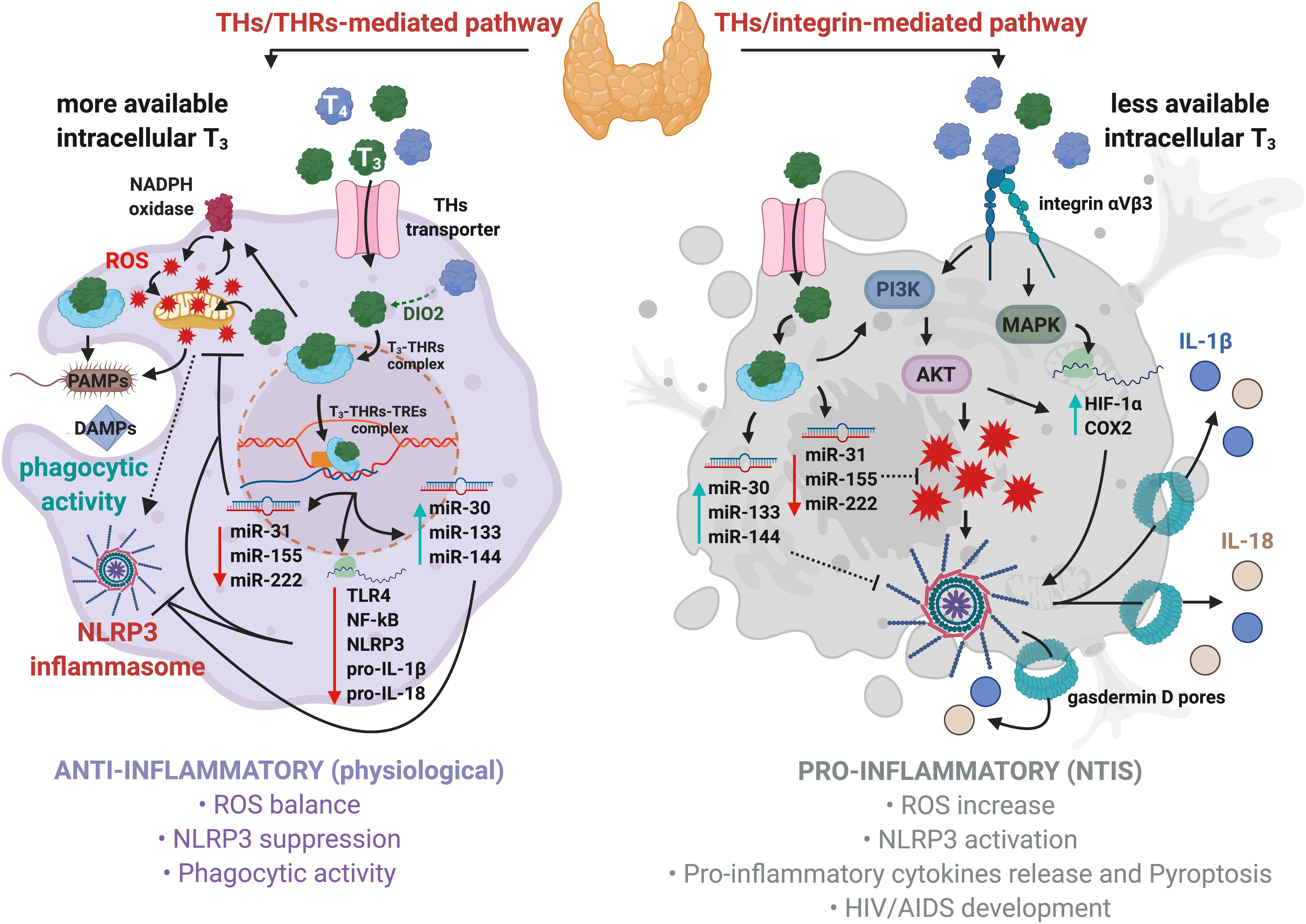
Figure 1. Regulation of NLRP3 inflammasome by thyroid hormones in macrophages. Physiological levels of T3 promote anti-inflammatory responses, bactericidal activity, and phagocytosis (left panel). Mechanistically, T3–THRs–TREs complex downregulates TLR4, NF-kB, NLRP3, pro-IL-1β, pro-IL-18, and several miRNAs, such as miR-31, -155, and -222, thus reducing ROS levels. Moreover, the T3–THRs–TREs complex upregulates miR-30, -133, and -144 that target Fasl, Ilk, Serpine1, hepatocyte growth factor (Hgf), Beta secretase 1 (Bace 1), and C-X-C motif chemokine receptor 4 (Cxcr4), thus further preventing the assembly of NLRP3 inflammasome (Forini et al., 2019). Hypothyroidism induces acute and chronic inflammatory responses, such as NTIS (right panel). High levels of T4 cause a robust production of ROS through the integrin αvβ3–PI3K–AKT signaling cascade, which ultimately triggers NLRP3 inflammasome. This is due to higher affinity displayed by T4 then T3 for integrin αvβ3 receptor on cell membrane. In addition, the T4–integrin αvβ3–MAPKs axis enhances the expression of HIF-1α and COX2 to promote NLRP3 inflammasome assembly and stability.
Role of THs on NLRP3 Inflammasome Activation
Inflammasomes are intracellular multiprotein complexes typical of immune cells, such as monocytes and macrophages, which mediate the first line of defense in response to sterile (absence of microbial particles) and non-sterile (microbial infection) threats, by activating pro-inflammatory cytokines (He et al., 2016a,b; Mangan et al., 2018). The sterile signals include damage-associated molecular patterns (DAMPs; Ahechu et al., 2018), debris from dead or dying cells (Newton and Dixit, 2012), and other organic and inorganic molecules (Allam et al., 2013; He et al., 2016a,b; Amores-Iniesta et al., 2017). The non-sterile agents encompass the pathogen-associated molecular patterns (PAMPs), lipopolysaccharide (LPS; Schroder and Tschopp, 2010), RNA (Franchi et al., 2014), and a wide range of bacterial toxins (Greaney et al., 2015).
Inflammasomes consist of a sensor protein, such as NLRP3, which recognizes the insults and activates effector proteins: Caspase-1. The active Caspase-1 cleaves the inflammatory pro-IL-1β and pro-IL-18 to generate their mature forms, as well as gasdermin D, whose N-terminus domains auto-assemble into pores on the plasma membrane for the release of bioactive cytokines, thus inducing an inflammatory form of cell death known as pyroptosis (Shi et al., 2015; Magupalli et al., 2020). Two temporally distinct events are required for the full activation of NLRP3-mediated inflammasome. The first step, priming, involves engagement of Toll-like receptors (TLRs) by pathogens or sterile particles. This is followed by recruitment of the myddosome complex, which transduce downstream signal to NF-kB, allowing an increase of NLRP3 and pro-ILs levels (Lamkanfi, 2011). The second event, activation, consists in the assembly of the inflammasome proteins into a functional active structure and includes different signal molecules, which cause an intracellular ion disbalance and activation of ROS production, culminating with NLRP3 inflammasome maturation (Wang L. et al., 2020). The amplitude of the inflammasome activation is a crucial event that controls shifts from acute to severe inflammation (Moossavi et al., 2018; Wang Z. et al., 2020). Recent evidence suggests that negative or positive modulation of the NLRP3 inflammasome could be dependent on T3 availability and uptake in the target cells, thus possibly diverting a physiological condition toward a pathological status. Therefore, T3 activity could be crucial for adequate macrophage function and tissue homeostasis. Indeed, alterations in these processes could lead to cancer, diabetes, intestinal bowel disease, or atherosclerosis (Wynn et al., 2013; Kwakkel et al., 2014).
After uptake, T3 partially migrates to the nucleus and binds to the macrophage dominant isoform of THRs (i.e., THRα; Kwakkel et al., 2014) and, subsequently, to the TREs located on promoters of the target genes. The T3–THRs–TREs complex regulates gene transcription through direct or indirect interactions with the nuclear DNA (Singh et al., 2017). It is well-established that the T3–THRs–TREs complex affects different miRNAs families, as miR-30, -133, and -144, whose expressions are increased by T3–THRs–TREs complex activity (Forini et al., 2018, 2019). These miRNAs dampen pro-inflammatory genes, such as Fast apoptosis signal Ligand (FasL; Chen et al., 2016) and Integrin-linked kinase (Ilk), two key players that trigger NLRP3 inflammasome assembly and inflammation (Boro and Balaji, 2017). Moreover, it was recently shown that the T3–THRs–TREs complex reduced cardiac-related miR-31, -155, and -222 (Forini et al., 2018). This results in an increased expression of superoxide dismutase 1 (SOD1) and 2 (SOD2; Wang et al., 2015; Forini et al., 2019), which lower the levels of ROS and inhibit the activation of NLRP3 inflammasome. In addition, the T3–THRs–TREs complex downregulates the TLR4/NF-kB pathway (Furuya et al., 2017; de Castro et al., 2018), thus reducing the levels of NLRP3, pro-IL-1β, and pro-IL-18. All this suggests that T3–THRs nuclear action may direct immune cells to an anti-inflammatory condition (Vargas and Videla, 2017; Forini et al., 2019; Figure 1, left panel). In particular, cytosolic T3–THRs complex controls nicotinamide adenine dinucleotide phosphate oxidase (NADPH)-dependent ROS production by involving the PI3K–AKT axis (Gnocchi et al., 2012). Cytosolic ROS partially contribute to the generation of mitochondrial ROS (mtROS; West et al., 2011; Pushpakumar et al., 2017), thus forming a loop between NADPH and mitochondria, which keeps intracellular levels of ROS within a physiological range (Dikalov, 2011). Finally, the cooperative interactions between the T3–THRs complex, moderate levels of ROS and mtROS, maintain the NLRP3 inflammasome activation under strict control and promote bactericidal clearance, phagocytic activity, and anti-inflammatory condition (Vernon and Tang, 2013; van der Spek et al., 2018; Figure 1, left panel).
On the other hand, more pronounced pro-inflammatory pathways might take place when levels of THs lean toward T4, a common condition diagnosed in clinical hypothyroidism, often associated with inflammation and risk of NTIS onset (Boelen et al., 2004; Mancini et al., 2016). Circulating T4 binds to integrin αvβ3, located on plasma membrane and signals to MAPKs, thus increasing levels of hypoxia-inducible factor 1-alpha (HIF-1α) and cyclooxygenase-2 (COX2; De Vito et al., 2011; Lin H. Y. et al., 2013a), both involved in the NLRP3 inflammasome activation (Hua et al., 2015; Gupta et al., 2017). In parallel, the T4–integrin αvβ3 axis activates PI3K and AKT, thus inducing a robust production of ROS (De Vito et al., 2012), as well as enhancing HIF-1α expression (Lin H. Y. et al., 2013a; Hsieh et al., 2017). All these events could ultimately lead to NLRP3 inflammasome activation (Figure 1, right panel). In support of this, it was found that excessive iodine promoted pyroptosis of thyroid follicular cells by the ROS–NF–kB–NLRP3 pathway in a model of autoimmune thyroiditis (Liu et al., 2019).
In summary, the net immunological response is determined by concentration and availability of circulating and intracellular THs, as well as by the metabolic status that could potentially promote an anti- or pro-inflammatory response by opposite regulations on NLRP3 inflammasome activation and stability.
Interplay Between THs, Wnt Pathway, and miRNAs During Inflammation
Interactions between THs and the Wnt/β-catenin pathway have been investigated in recent years (Todaro et al., 2010). The modulation of Wnt/β-catenin signaling pathway by the T3–THRs–TREs complex affects fundamental biological processes such as cell proliferation, development, tissue homeostasis, and metabolism (Ely et al., 2018). While THRα1 receptor controls gut development and homeostasis through the Wnt pathway in physiological conditions (Kress et al., 2009), in pathological conditions, such as colorectal cancer, the THRα1 receptor is thought to activate β-catenin/Tcf4 transcription, thus increasing the cell proliferation and tissue rearrangement in the gut (Kress et al., 2010). Grainyhead-like transcription factor 3 (GRHL3), essential for epidermal differentiation and morphogenesis, suppresses DIO3 activity (increasing T3 levels) and acts as a downstream signal for the Wnt/β-catenin pathway (Kimura-Yoshida et al., 2018). In addition, the T3–THRs–TREs complex induces expression of Dickkopf (DKK) 4, which antagonizes Wnt/β-catenin signaling in hepatocellular carcinoma cell lines (HCC), suggesting a role for T3 in tumor suppression and unraveling the T3/DKK4/Wnt/β-catenin pathway as a possible therapeutic target in HCC (Liao et al., 2012; Figure 2A). The T3–THRs–TREs complex also controls several epigenetic mechanisms of gene expression (Dong et al., 2010; Janssen et al., 2014; Forini et al., 2018). Dissecting T3–THRs–TREs-dependent genetic and epigenetic crosstalk could provide new insights to develop therapeutic strategies for pathologies that affect the HPT axis, as NTIS and autoimmune thyroiditis (Tomer, 2014; McDermott, 2019*). Decreased levels of THRs and THs have been found in animal models for NTIS and in NTIS patients affected by sepsis and cardiovascular disturbances (Warner and Beckett, 2010; von Hafe et al., 2019).
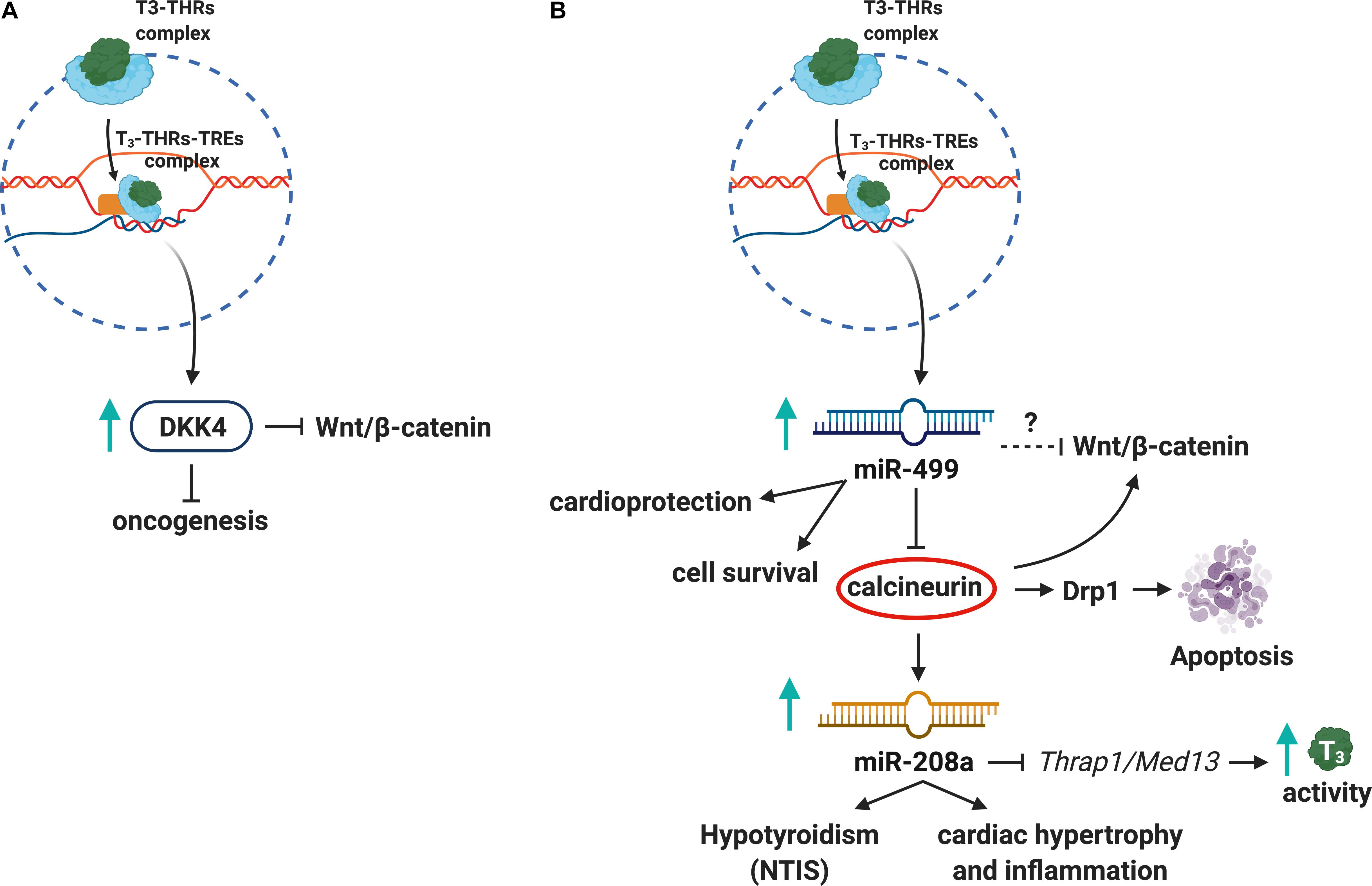
Figure 2. Thyroid hormones, Wnt pathway, and miRNAs crosstalk. (A) The T3–THRs–TREs complex upregulates DKK4, a tumor suppressor, which inhibits Wnt/β-catenin pathway. (B) Also, the T3–THRs–TREs complex increases miR-499 and downregulates calcineurin and miR208a, thus reducing apoptosis, cardiac hypertrophy, inflammation, and NTIS.
Several studies showed that T3–THRs–TREs signaling affects miRNAs expression (Dong et al., 2010; Janssen et al., 2014; Babu and Tay, 2019). For instance, T3–THRs–TREs binds to miR-17 promoter and decreases transcription and processing of mature miR-17, involved in cancer (Lin Y. H. et al., 2013b). Also, some miRNAs regulated by THs play an important role in the Wnt pathway. For instance, miR-499 related to cardioprotection (Wang et al., 2011) inhibits calcineurin (a signaling molecule of Wnt/β-catenin pathway), thus reducing the levels of Dynamin-1-like protein (Drp1), which is involved in apoptosis (Tan et al., 2008). Another cardiac-specific miRNA (Seok et al., 2014; Wang et al., 2015; Su et al., 2016), miR-208a, inhibits T3-mediated signaling pathway by repressing the THs Associated Protein/Mediator Complex Subunit 13 (THRAP1/MED13) in a mouse model of cardiac hypertrophy and hypothyroidism (van Rooij et al., 2009; Neppl and Wang, 2014; Figure 2B). Although there is ample evidence about the THs/THRs/miRNAs alterations in immune-related pathologies, it will be crucial to further explore the regulatory networks between miRNAs and THs in pathological contexts such as NTIS.
NTIS and THs During HIV Infection
Impairments in the HPT axis in the course of NTIS affect circulating levels of THs, especially T3. NTIS may result from HPT setpoint alterations that occur during prolonged hospitalization in a variety of systemic diseases (Boelen et al., 2011; de Vries et al., 2015; Yasar et al., 2015). In this context, it has been described that patients affected by severe acute respiratory syndrome (SARS) present signs of NTIS and HPT dysfunctions (Marazuela et al., 2020; Pal and Banerjee, 2020). Same alterations might also be caused by Coronavirus disease 2019 (COVID-19) as recently reported (Khoo et al., 2020; Wai Lui et al., 2020). However, other factors such as elevated levels of circulating IL-6 (Wajner et al., 2011) and TNF-α (Feelders et al., 1999) also inhibit or reduce T4 conversion to T3 in NTIS patients.
NTIS is characterized by reduced circulating levels of T3 and increased rT3, as a result of dysregulated deiodination of intracellular T4 by DIO3 and perhaps other deiodinases, which inactivate THs, preventing their excess (De Groot, 1999; Wajner et al., 2011). In particular, LPS administration is a model of NTIS that stimulates DIO2 activity, NF-κB activation, and consequently cytokine increase, whereas it decreases DIO1 levels (Boelen et al., 2011). Furthermore, many systemic and non-endocrine pathologies such as congestive heart failure, cardiorenal syndrome, and starvation/malnutrition are commonly observed in NTIS patients (Larsen et al., 2002; Lee and Farwell, 2016). It has been suggested that NTIS may represent a form of hypothyroidism linked to the oxidative stress and reduced antioxidant defense system, related to the altered function of deiodinases (Mancini et al., 2016). In fact, the administration of the antioxidant N-acetylcysteine, in order to prevent NTIS in patients with acute myocardial infarction, increased serum T3 while decreasing rT3 (Vidart et al., 2014; de Vries et al., 2015; Lee and Farwell, 2016). Interestingly, adaptive NTIS response, in terms of thyroid function, during sustained immune defense has been interpreted as an effort—in terms of reduced available T3—to decrease the energy expenditure and turnover of several proteins involved in host defenses (Klein, 2006; de Vries et al., 2015). Acute NTIS has also been interpreted as a support mechanism for the immune response because of high production of pro-inflammatory cytokines found at the early stage of the disease (Boelen et al., 2011).
Acquired immunodeficiency syndrome (AIDS), in which HIV seriously compromises immune defenses, is associated with dysfunction of HPT and endocrine organs and shows typical markers of endocrine alterations related to NTIS, such as high ROS levels (Parsa and Bhangoo, 2013). More importantly, recent findings suggest that HIV-related conditions promote NRLP3-mediated inflammasome activation (Haque et al., 2016; Bandera et al., 2018; Figure 1, right panel).
The screening of TSH is highly recommended in HIV patients and, if the levels of TSH are found altered, free T3 and T4 measurements become necessary. During such screenings, possible occurrence of NTIS must be considered for differential diagnosis related to the abnormal thyroid functionality, especially in individuals with advanced AIDS (Hoffmann and Brown, 2007). Analysis of THs metabolism on post-mortem tissues of HIV-infected patients showed alterations in the HPT axis and 27% of screened HIV patients showed abnormal TSH levels (50% had TSH < 0.5 mU/L and the remaining had >4 mU/L; Langford et al., 2011). HPT axis alterations are usually considered as NTIS and depend on the severity of HIV-related disease. TSH may change, and usually the activity of deiodinases is decreased; therefore, higher circulating T4 and rT3 levels are found, whereas circulating T3 is decreased (Hoffmann and Brown, 2007). The lack of effectiveness of T3 administration/replacement in NTIS patients (Chopra, 1997; De Groot, 2006; Warner and Beckett, 2010; de Vries et al., 2015) could be explained by the actions of rT3, which is largely inactive (Lanni et al., 1993, 2016; Moreno et al., 2008).
The autoantibodies, namely, TgAb and TPOAb, were also altered in HIV-infected individuals (Ketsamathi et al., 2006). Drug abuse, or its withdrawal, may also contribute to this (Langford et al., 2011). Interestingly, during anti-retroviral therapy (ART), a subclinical hypothyroidism is commonly observed. Indeed, isolated levels of TSH are elevated, whereas low free T4 is found (Hoffmann and Brown, 2007). Similarly, results on THs alterations have been reported in a study on HIV-infected children treated with ART. Therefore, due to the serious outcome of these pathologies and their consequences on the psychosomatic development, the thyroid dysfunctions should be carefully evaluated not only in adults but also in children (Viganò et al., 2004). Indeed, impact of NTIS in critically ill children (Jacobs et al., 2019) remains unclear.
Discussion and Future Perspectives
Herein, we have discussed the bilateral crosstalk between the immune system and THs both in physiological and patho-physiological conditions. The activation of NLRP3 inflammasome, modulation of the Wnt/β-catenin pathway, and NTIS could rely on a complex interplay that involves THs and miRNAs. Finally, how viral infections could affect NTIS and HPT functions have been discussed. Broadly, we have provided new insights into how the immune system and endocrine system interact with each other. Ultimately, it is our hope that ideas discussed here will eventually open novel avenues of research and drug development (Silverman et al., 2020). In particular, since miRNAs are involved in the crosstalk between inflammation and THs-related diseases, they might be considered not only as biomarkers but also as potential druggable targets in order to combat, with higher efficiency, NTIS. Indeed, a recent study has shown how downregulation of miR-155 by an anti-miRNA compound, cobomarsen, reduced inflammation and tumor volume in preclinical models and in a patient (Anastasiadou et al., 2020). Future studies related to how THs affect the immune system in physiological and pathological settings, including those that mimic HIV infection in vitro, will provide important insights and impetus to this exciting field.
Author Contributions
RDL, PJD, PT, EAn, RN, and SI conceptualized and wrote the manuscript. H-YL, FG, ZAP, EAf, JZP, and CM edited the manuscript and provided important insights and suggestions. All authors contributed to the article and approved the submitted version.
Conflict of Interest
The authors declare that the research was conducted in the absence of any commercial or financial relationships that could be construed as a potential conflict of interest.
Acknowledgments
Images were obtained by using BioRender software (BioRender 2020).
References
Ahechu, P., Zozaya, G., Martí, P., Hernández-Lizoáin, J. L., Baixauli, J., Unamuno, X., et al. (2018). NLRP3 Inflammasome: a possible link between obesity-associated low-grade chronic inflammation and colorectal cancer development. Front. Immunol. 9:2918. doi: 10.3389/fimmu.2018.02918
Alamino, V. A., Montesinos, M. D. M., Soler, M. F., Giusiano, L., Gigena, N., Fozzatti, L., et al. (2019). Dendritic cells exposed to triiodothyronine deliver pro-inflammatory signals and amplify IL-17-driven immune responses. Cell Physiol. Biochem. 52, 354–367. doi: 10.33594/000000025
Allam, R., Darisipudi, M. N., Tschopp, J., and Handers, H. J. (2013). Histones trigger sterile inflammation by activating the NLRP3 inflammasome. Eur. J. Immunol. 43, 3336–3342. doi: 10.1002/eji.201243224
Amores-Iniesta, J., Barberà-Cremades, M., Martiníz, C. M., Pons, J. A., Revilla-Nuin, B., Martínez- Alarcón, L., et al. (2017). Extracellular ATP activates the NLRP3 inflammasome and is an early danger signal of skin allograft rejection. Cell Rep. 21, 3414–3426. doi: 10.1016/j.celrep.2017.11.079
Anastasiadou, E., Faggioni, A., Trivedi, P., and Slack, F. J. (2018a). The nefarious nexus of noncoding RNAs in cancer. Int. J. Mol. Sci. 19:2072. doi: 10.3390/ijms19072072
Anastasiadou, E., Jacob, L. S., and Slack, F. J. (2018b). Non-coding RNA networks in cancer. Nat. Rev. Cancer 18, 5–18. doi: 10.1038/nrc.2017.99
Anastasiadou, E., Seto, A., Beatty, X., Hermreck, M., Gilles, M.-E., Stroopinsky, D., et al. (2020). Cobomarsen, an oligonucleotide inhibitor of miR-155, slows DLBCL tumor cell growth in vitro and in vivo. Clin. Cancer Res. doi: 10.1158/1078-0432.CCR-20-3139 [Epub ahead of print].
Babu, K. R., and Tay, Y. (2019). The yin-yang regulation of reactive oxygen species and microRNAs in cancer. Int. J. Mol. Sci. 20:5335. doi: 10.3390/ijms20215335
Bagriacik, E. U., Zhou, Q., Wang, H. C., and Klein, J. R. (2001). Rapid and transient reduction in circulating thyroid hormones following systemic antigen priming: implications for functional collaboration between dendritic cells and thyroid. Cell Immunol. 212, 92–100. doi: 10.1006/cimm.2001.1846
Bandera, A., Masetti, M., Fabbiani, M., Biasin, M., Muscatello, A., Squillace, N., et al. (2018). The NLRP3 inflammasome is upregulated in HIV-infected antiretroviral therapy-treated individuals with defective immune recovery. Front. Immunol. 9:214. doi: 10.3389/fimmu.2018.00214
Bergh, J. J., Lin, H. Y., Lansing, L., Mohamed, S. N., Davis, F. B., et al. (2005). Integrin (V(3 contains a cell surface receptor site for thyroid hormone that is linked to activation of mitogen-activated protein kinase and induction of angiogenesis. Endocrinology 146, 2864–2871. doi: 10.1210/en.2005-2102
Blalock, J. E., Johnson, H. M., Smith, E. M., and Torres, B. A. (1984). Enhancement of the in vitro antibody response by thyrotropin. Biochem. Biophys. Res. Commun. 125, 30–34. doi: 10.1016/s0006-291x(84)80329-80320
Boelen, A., Kwakkel, J., and Fliers, E. (2011). Beyond low plasma T3: local thyroid hormone metabolism during inflammation and infection. Endocr. Rev. 32, 670–693. doi: 10.1210/er.2011-2017
Boelen, A., Kwakkel, J., Platvoet-ter Schiphorst, M., Mentrup, B., Baur, A., Koehrle, J., et al. (2004). Interleukin-18, a proinflammatory cytokine, contributes to the pathogenesis of non-thyroidal illness mainly via the central part of the hypothalamus-pituitary-thyroid axis. Eur. J. Endocrinol. 151, 497–502. doi: 10.1530/eje.0.1510497
Boro, M., and Balaji, K. N. (2017). CXCL1 and CXCL2 regulate NLRP3 inflammasome activation via G-protein-coupled receptor CXCR2. J. Immunol. 199, 1660–1671. doi: 10.4049/jimmunol.1700129
Brent, G. A. (2012). Mechanisms of thyroid hormone action. J. Clin. Invest. 122, 3035–3043. doi: 10.1172/JCI60047
Chen, Y., Yuan, M., Xia, M., Wang, L., Zhang, Y., and Li, P. L. (2016). Instant membrane resealing in nlrp3 inflammasome activation of endothelial cells. Front. Biosci. 21:635–650. doi: 10.2741/4411
Cheng, S. Y., Leonard, J. L., and Davis, P. J. (2010). Molecular aspects of thyroid hormone actions. Endocr. Rev. 31, 139–170. doi: 10.1210/er.2009-2007
Chopra, I. J. (1997). Clinical review 86: euthyroid sick syndrome: is it a misnomer? J. Clin. Endocrinol. Metab. 82, 329–334. doi: 10.1210/jcem.82.2.3745
Csaba, G., and Pállinger, E. (2009). Thyrotropic hormone (TSH) regulation of triiodothyronine (T3) concentration in immune cells. Inflamm. Res. 58, 151–154.
Davis, P. J., Ashur-Fabian, O., Incerpi, S., and Mousa, S. A. (2019). Editorial: non genomic actions of thyroid hormones in Cancer. Front. Endocrinol. (Lausanne) 10:847. doi: 10.3389/fendo.2019.00847
Davis, P. J., Davis, F. B., and Cody, V. (2005). Membrane receptors mediating thyroid hormone action. Trends Endocrinol. Metab. 16, 429–435. doi: 10.1016/j.tem.2005.09.007
de Castro, A. L., Fernandes, R. O., Ortiz, V. D., Campos, C., Bonetto, J. H. P., Fernandes, T., et al. (2018). Thyroid hormones decrease the proinflammatory TLR4/NF-κβ pathway and improve functional parameters of the left ventricle of infarcted rats. Mol. Cell. Endocrinol. 461, 132–142. doi: 10.1016/j.mce.2017.09.003
De Groot, L. J. (1999). Dangerous dogmas in medicine: the nonthyroidal illness syndrome. J. Clin. Endocrinol. Metab. 84, 151–164. doi: 10.1210/jcem.84.1.5364
De Groot, L. J. (2006). Non-thyroidal illness syndrome is a manifestation of hypothalamicpituitary dysfunction, and in view of current evidence, should be treated with appropriate replacement therapies. Crit. Care Clin. 22, 57–86. doi: 10.1016/j.ccc.2005.10.001
De Vito, P., Balducci, V., Leone, S., Percario, Z., Mangino, G., Davis, P. J., et al. (2012). Nongenomic effects of thyroid hormones on the immune system cells: new targets, old players. Steroids 77, 988–995. doi: 10.1016/j.steroids.2012.02.018
De Vito, P., Incerpi, S., Pedersen, J. Z., Luly, P., Davis, F. B., and Davis, P. J. (2011). Thyroid hormones as modulators of immune activities at the cellular level. Thyroid 21, 879–890. doi: 10.1089/thy.2010.0429
de Vries, E. M., Fliers, E., and Boelen, A. (2015). The molecular basis of the non-thyroidal illness syndrome. J. Endocrinol. 225, R67–R81. doi: 10.1530/JOE-15-0133
Dikalov, S. (2011). Crosstalk between mitochondria and NADPH oxidases. Free Radic. Biol. Med. 51, 1289–1301. doi: 10.1016/j.freeradbiomed.2011.06.033
Dong, H., Paquette, M., Williams, A., Zoeller, R. T., Wade, M., and Yauk, C. (2010). Thyroid hormone may regulate mRNA abundance in liver by acting on microRNAs. PLoS One 5:e12136. doi: 10.1371/journal.pone.0012136
Ely, K. A., Bischoff, L. A., and Weiss, V. L. (2018). Wnt signaling in thyroid homeostasis and carcinogenesis. Genes (Basel) 9:204. doi: 10.3390/genes9040204
Feelders, R. A., Swaak, A. J., Romijn, J. A., Eggermont, A. M., Tielens, E. T., Vreugdenhil, G., et al. (1999). Characteristics of recovery from the euthyroid sick syndrome induced by tumor necrosis factor alpha in cancer patients. Metabolism 48, 324–329. doi: 10.1016/s0026-0495(99)90080-x
Flamant, F., Cheng, S. Y., Hollenberg, A. N., Moeller, L. C., Samurat, J., Wondisford, F. E., et al. (2017). Thyroid hormone signaling pathways: time for a more precise nomenclature. Endocrinology 158, 2052–2057. doi: 10.1210/en.2017-2250
Forini, F., Nicolini, G., Kusmic, C., D’Aurizio, R., Rizzo, M., Baumgart, M., et al. (2018). Integrative analysis of differentially expressed genes and miRNAs predicts complex T3-mediated protective circuits in a rat model of cardiac ischemia reperfusion. Sci. Rep. 8:13870. doi: 10.1038/s41598-018-32237-32230
Forini, F., Nicolini, G., Kusmic, C., and Iervasi, G. (2019). Protective effects of euthyroidism restoration on mitochondria function and quality control in cardiac pathophysiology. Int. J. Mol. Sci. 20:3377. doi: 10.3390/ijms20143377
Franchi, L., Eigenbrod, T., Muñoz-Panillo, R., Ozkurede, U., Kim, Y. G., and Arindam, C. (2014). Cytosolic double-stranded RNA activates the NLRP3 inflammasome via MAVS-induced membrane permeabilization and K+ efflux. J. Immunol. 193, 4214–4222. doi: 10.4049/jimmunol.1400582
Furuya, F., Ishii, T., Tamura, S., Takahashi, K., Kobayashi, H., Ichijo, M., et al. (2017). The ligand-bound thyroid hormone receptor in macrophages ameliorates kidney injury via inhibition of nuclear factor-κB activities. Sci. Rep. 7:43960. doi: 10.1038/srep43960
Gagnon, A., Langille, M. L., Chaker, S., Antunes, T. T., Durand, J., and Sorisky, A. (2014). TSH signaling pathways that regulate MCP-1 in human differentiated adipocytes. Metabolism 63, 812–821. doi: 10.1016/j.metabol.2014.02.015
Gnocchi, D., Leoni, S., Incerpi, S., and Bruscalupi, G. (2012). 3,5,3’-Triiodothyronine (T3) stimulates cell proliferation through the activation of the PI3K/Akt pathway and reactive oxygen species (ROS) production in chick embryo hepatocytes. Steroids 77, 589–595. doi: 10.1016/j.steroids.2012.01.022
Greaney, A. J., Leppla, S. H., and Moayeri, M. (2015). Bacterial exotoxins and the inflammasome. Front. Immunol. 6:570. doi: 10.3389/fimmu.2015.00570
Gupta, N., Sahu, A., Prabhakar, A., Chatterjee, T., Tyagi, T., and Kumari, B. (2017). Activation of NLRP3 inflammasome complex potentiates venous thrombosis in response to hypoxia. Proc. Natl. Acad. Sci. U S A. 114, 4763–4768. doi: 10.1073/pnas.1620458114
Haque, S., Lan, X., Wen, H., Lederman, R., Chawla, A., Attia, M., et al. (2016). HIV promotes NLRP3 inflammasome complex activation in murine HIV-associated nephropathy. Am. J. Pathol. 186, 347–358. doi: 10.1016/j.ajpath.2015.10.002
Harbour, D. V., Kruger, T. E., Coppenhaver, D., Smith, E. M., and Meyer, W. J. III (1989). Differential expression and regulation of thyrotropin (TSH) in T cell lines. Mol. Cell. Endocrinol. 64, 229–241. doi: 10.1016/0303-7207(89)90150-90150
He, Y., Hara, H., and Núñez, G. (2016a). Mechanism and regulation of NLRP3 inflammasome activation. Trends Biochem. Sci. 41, 1012–1021. doi: 10.1016/j.tibs.2016.09.002
He, Y., Zeng, M. Y., Yang, D., Motro, B., and Núñez, G. (2016b). NEK7 is an essential mediator of NLRP3 activation downstream of potassium efflux. Nature 530, 354–357. doi: 10.1038/nature16959
Hennemann, G., Docter, R., Friesema, E. C., de Jong, M., Krenning, E. P., and Visser, T. J. (2001). Plasma membrane transport of thyroid hormones and its role in thyroid hormone metabolism and bioavailability. Endocr. Rev. 22, 451–476. doi: 10.1210/edrv.22.4.0435
Hodkinson, C. F., Simpson, E. E., Beattie, J. H., O’Connor, J. M., Campbell, D. J., Strain, J. J., et al. (2009). Preliminary evidence of immune function modulation by thyroid hormones in healthy men and women aged 55-70 years. J. Endocrinol. 202, 55–63. doi: 10.1677/JOE08-0488
Hoffmann, C. J., and Brown, T. T. (2007). Thyroid function abnormalities in HIV-infected patients. Clin. Infect. Dis. 45, 488–494. doi: 10.1086/519978
Hsieh, M.-T., Wang, L.-M., Changou, C. A., Chin, Y.-T., Yang, Y.-C. S. H., Lai, H.-Y., et al. (2017). Crosstalk between integrin αvβ3 and ERα contributes to thyroid hormone induced proliferation of ovarian cancer cells. Oncotarget 8, 24237–24249. doi: 10.18632/oncotarget.10757
Hua, K. F., Chou, J. C., Ka, S. M., Tasi, Y. L., Chen, A., Wu, S. H., et al. (2015). Cyclooxygenase-2 regulates NLRP3 inflammasome-derived IL-1β production. J. Cell. Physiol. 230, 863–874. doi: 10.1002/jcp.24815
Incerpi, S., Davis, P. J., Pedersen, J. Z., and Lanni, A. (2016). “Nongenomic actions of thyroid hormones,” in Principles of Endocrinology and Hormone in Review Action, eds A. Belfiore, and D. LeRoith, (Cham: Springer International Publishing), 1–26. doi: 10.1007/978-3-319-44675-2_32
Jacobs, A., Derese, I., Vander Perre, S., van Puffelen, E., Verstraete, S., Pauwels, L., et al. (2019). Non-thyroidal illness syndrome in critically ill children: prognostic value and impact of nutritional management. Thyroid 29, 480–492. doi: 10.1089/thy.2018.0420
Janssen, R., Zuidwijk, M. J., Kuster, D. W., Muller, A., and Simonides, W. S. (2014). Thyroid hormone-regulated cardiac microRNAs are predicted to suppress pathological hypertrophic signaling. Front. Endocrinol. (Lausanne) 5:171. doi: 10.3389/fendo.2014.00171
Kelly, G. S. (2000). Peripheral metabolism of thyroid hormones: a review. Altern. Med. Rev. 5, 306–333.
Ketsamathi, C., Jongjaroenprasert, W., Chailurkit, L. O., Udomsubpayakul, U., and Kiertiburanakul, S. (2006). Prevalence of thyroid dysfunction in Thai HIV-infected patients. Curr. HIV Res. 4, 463–467. doi: 10.2174/157016206778560036
Khoo, B., Tan, T., Clarke, S. A., Mills, E. G., Patel, B., Modi, M., et al. (2020). Thyroid function before, during and after COVID-19. J. Clin. Endocrinol. Metab. doi: 10.1210/clinem/dgaa830 Online ahead of print.
Kimura-Yoshida, C., Mochida, K., Nakaya, M. A., Mizutani, T., and Matsuo, I. (2018). Cytoplasmic localization of GRHL3 upon epidermal differentiation triggers cell shape change for epithelial morphogenesis. Nat. Commun. 9:4059. doi: 10.1038/s41467-018-06171-6178
Klecha, A. J., Barreiro Arcos, M. L., Frick, L., Genaro, A. M., and Cremaschi, G. (2008). Immune-endocrine interactions in autoimmune thyroid diseases. Neuroimmunomodulation 15, 68–75. doi: 10.1159/000135626
Klecha, A. J., Genaro, A. M., Lysionek, A. E., Caro, R. A., Coluccia, A. G., and Cremaschi, G. A. (2000). Experimental evidence pointing to the bidirectional interaction between the immune system and the thyroid axis. Int. J. Immunopharmacol. 22, 491–500. doi: 10.1016/s0192-0561(00)00012-16
Klein, J. R. (2006). The immune system as a regulator of thyroid hormone activity. Exp. Biol. Med. (Maywood) 231, 229–236. doi: 10.1177/153537020623100301
Kress, E., Samarut, J., and Plateroti, M. (2009). Thyroid hormones and the control of cell proliferation or cell differentiation: paradox or duality? Mol. Cell Endocrinol. 313, 36–49.
Kress, E., Skah, S., Sirakov, M., Nadjar, J., Gadot, N., Scoazec, J. Y., et al. (2010). Cooperation between the thyroid hormone receptor TRα1 and the WNT pathway in the induction of intestinal tumorigenesis. Gastroenterology 138, 1863–1874. doi: 10.1053/j.gastro.2010.01.041
Kruger, T. E., and Blalock, J. E. (1986). Cellular requirements for thyrotropin enhancement of in vitro antibody production. J. Immunol. 137, 197–200.
Kwakkel, J., Surovtseva, O. V., de Vries, E. M., Stap, J., Fliers, E., and Boelen, A. (2014). A novel role for the thyroid hormone-activating enzyme type 2 deiodinase in the inflammatory response of macrophages. Endocrinology 155, 2725–2734. doi: 10.1210/en.2013-2066
Lamkanfi, M. (2011). Emerging inflammasome effector mechanisms. Nat. Rev. Immunol. 11, 213–220. doi: 10.1038/nri2936
Langford, D., Baron, D., Joy, J., Del Valle, L., and Shack, J. (2011). Contributions of HIV infection in the hypothalamus and substance abuse/use to HPT dysregulation. Psychoneuroendocrinology 36, 710–719. doi: 10.1016/j.psyneuen.2010.10.005
Lanni, A., Moreno, M., Cioffi, M., and Goglia, F. (1993). Effect of 3,3’-di-iodothyronine and 3,5-diiodothyronine on rat liver mitochondria. J. Endocrinol. 136, 59–64. doi: 10.1677/joe.0.1360059
Lanni, A., Moreno, M., and Goglia, F. (2016). Mitochondrial actions of thyroid hormone. Compr. Physiol. 6, 1591–1607. doi: 10.1002/cphy.c150019
Larsen, R. L., Eguchi, J. H., Mulla, N. F., Johnston, J. K., Fitts, J., Kuhn, M. A., et al. (2002). Usefulness of cardiac transplantation in children with visceral heterotaxy (asplenic and polysplenic syndromes and single right-sided spleen with levocardia) and comparison of results with cardiac transplantation in children with dilated cardiomyopathy. Am. J. Cardiol. 89, 1275–1279. doi: 10.1016/s0002-9149(02)02325-2321
Lee, S., and Farwell, A. P. (2016). Euthyroid sick syndrome. Compr. Physiol. 6, 1071–1080. doi: 10.1002/cphy.c150017
Liao, C. H., Yeh, C. T., Huang, Y. H., Wu, S. M., Chi, H. C., Tsai, M. M., et al. (2012). Dickkopf 4 positively regulated by the thyroid hormone receptor suppresses cell invasion in human hepatoma cells. Hepatology 55, 910–920. doi: 10.1002/hep.24740
Lin, H. Y., Davis, F. B., Gordinier, J. K., Martino, L. J., and Davis, P. J. (1999). Thyroid hormone induces activation of mitogen-activated protein kinase in cultured cells. Am. J. Physiol. 276, C1014–C1024. doi: 10.1152/ajpcell.1999.276.5.C1014
Lin, H. Y., Su, Y. F., Hsieh, M. T., Lin, S., Meng, R., London, D., et al. (2013a). Nuclear monomeric integrin αv in cancer cells is a coactivator regulated by thyroid hormone. FASEB J. 27, 3209–3216. doi: 10.1096/fj.12-227132
Lin, Y. H., Liao, C. J., Huang, Y. H., Wu, M. H., Chi, H. C., Wu, S. M., et al. (2013b). Thyroid hormone receptor represses miR-17 expression to enhance tumor metastasis in human hepatoma cells. Oncogene 32, 4509–4518. doi: 10.1038/onc.2013.309
Liu, J., Mao, C., Dong, L., Kang, P., Ding, C., Zheng, T., et al. (2019). Excessive iodine promotes pyroptosis of thyroid follicular epithelial cells in Hashimoto’s Thyroiditis through the ROS-NFκB-NLRP3 pathway. Front. Endocrinol. (Lausanne) 20:778. doi: 10.3389/fendo.2019.00778
Magupalli, M. G., Negro, R., Tian, Y., Hauenstein, A. V., Di Caprio, G., Skillern, W., et al. (2020). HDAC6 mediates an aggresome-like mechanism for NLRP3 and pyrin inflammasome activation. Science 369:eaas8995. doi: 10.1126/science
Mancini, A., Di Segni, C., Raimondo, S., Olivieri, G., Silvestrini, A., Meucci, E., et al. (2016). Thyroid hormones, oxidative stress, and inflammation. Mediators Inflamm. 2016:6757154. doi: 10.1155/2016/6757154
Mangan, M. S. J., Olhava, E. J., Roush, W. R., Seidel, H. M., Glick, J. D., and Latz, E. (2018). Targeting the NLRP3 inflammasome in inflammatory diseases. Nat. Rev. Drug Discov. 17, 588–606. doi: 10.1038/nrd.2018.97
Marazuela, M., Giustina, A., and Puig-Domingo, M. (2020). Endocrine and metabolic aspects of the COVID-19 pandemic. Rev. Endocr. Metab. Disord 9, 1–13. doi: 10.1007/s11154-020-09569-9562
Marino, F., Guasti, L., Cosentino, M., De Piazza, D., Simoni, C., Piantanida, E., et al. (2006). Thyroid hormone regulation of cell migration and oxidative metabolism in polymorphonuclear leukocytes: clinical evidence in thyroidectomized subjects on thyroxine replacement therapy. Life Sci. 78, 1071–1077. doi: 10.1016/j.lfs.2005.06.016
McDermott, M. T. (2019*). “Non-thyroidal illness syndrome (Euthyroid sick syndrome),” in Management of Patients With Pseudo-endocrine Disorders ed M. T. McDermott, (Cham: Springer), 331–339.
Moossavi, M., Parsamanesh, N., Bahrami, A., Atkin, S. L., and Sahebkar, A. (2018). Role of the NLRP3 inflammasome in cancer. Mol. Cancer 17, 158. doi: 10.1186/s12943-018-0900-903
Moreno, M., de Lange, P., Lombardi, A., Silvestri, E., Lanni, A., and Goglia, F. (2008). Metabolic effects of thyroid hormone derivatives. Thyroid 18, 239–253. doi: 10.1089/thy.2007.0248
Neppl, R. L., and Wang, D. Z. (2014). The myriad essential roles of microRNAs in cardiovascular homeostasis and disease. Genes Dis. 1, 18–39. doi: 10.1016/j.gendis.2014.06.003
Newton, K., and Dixit, V. M. (2012). Signaling in innate immunity and inflammation. Cold Spring Harb. Perspect. Biol. 4:a006049. doi: 10.1101/cshperspect.a006049
Pal, R., and Banerjee, M. (2020). COVID-19 and the endocrine system: exploring the unexplored. J. Endocrinol. Invest. 43, 1027–1031. doi: 10.1007/s40618-020-01276-8
Parsa, A. A., and Bhangoo, A. (2013). HIV and thyroid dysfunction. Rev. Endocr. Metab. Disord 14, 127–131. doi: 10.1007/s11154-013-9248-9246
Pushpakumar, S., Ren, L., Kundu, S., Gamon, A., Tyagi, S. C., and Sen, U. (2017). Toll-like receptor 4 deficiency reduces oxidative stress and macrophage mediated inflammation in hypertensive kidney. Sci. Rep. 7:6349. doi: 10.1038/s41598-017-06484-6486
Schroder, K., and Tschopp, J. (2010). The Inflammasomes. Cell 140, 821–832. doi: 10.1016/j.cell.2010.01.040
Seok, H. Y., Chen, J., Kataoka, M., Huang, Z. P., Ding, J., Yan, J., et al. (2014). Loss of microRNA-155 protects the heart from pathological cardiac hypertrophy. Circ. Res. 114, 1585–1595. doi: 10.1161/CIRCRESAHA.114.303784
Shi, J., Zhao, Y., Wang, K., Shi, X., Wang, Y., Huang, H., et al. (2015). Cleavage of GSDMD by inflammatory caspases determines pyroptotic cell death. Nature 526, 660–665. doi: 10.1038/nature15514
Shih, A., Zhang, S., Cao, H. J., Tang, H. Y., Davis, F. B., Davis, P. J., et al. (2004). Disparate effects of thyroid hormone on actions of epidermal growth factor and transforming growth factor-α are mediated by 3′,5′-cyclic adenosine 5′-monophosphate-dependent protein kinase II. Endocrinology 145, 1708–1717. doi: 10.1210/en.2003-2742
Silverman, E. K., Schmidt, H. H. H. W., Anastasiadou, E., Altucci, L., Angelini, M., Lina Badimon, L., et al. (2020). Molecular networks in network medicine: development and applications. Wiley Interdiscip Rev. Syst. Biol. Med. 12, e1489. doi: 10.1002/wsbm.1489
Singh, B. K., Sinha, R. A., Ohba, K., and Yen, P. M. (2017). Role of thyroid hormone in hepatic gene regulation, chromatin remodeling, and autophagy. Mol. Cell. Endocrinol. 458, 160–168. doi: 10.1016/j.mce.2017.02.018
Singh, B. K., Sinha, R. A., and Yen, P. M. (2018). Novel transcriptional mechanisms for regulating metabolism by thyroid hormone. Int. J. Mol. Sci. 19:3284. doi: 10.3390/ijms19103284
Smith, E. M., Phan, M., Kruger, T. E., Coppenhaver, D. H., and Blalock, J. E. (1983). Human lymphocyte production of immunoreactive thyrotropin. Proc. Natl. Acad. Sci. U S A. 80, 6010–6013. doi: 10.1073/pnas.80.19.6010
Su, M., Chen, Z., Wang, C., Song, L., Zou, Y., Zhang, L., et al. (2016). Cardiac-Specific overexpression of miR-222 induces heart failure and inhibits autophagy in mice. Cell Physiol. Biochem. 39, 1503–1511. doi: 10.1159/000447853
Tan, W. Q., Wang, J. X., Lin, Z. Q., Li, Y. R., Lin, Y., and Li, P. F. (2008). Novel cardiac apoptotic pathway: the dephosphorylation of apoptosis repressor with caspase recruitment domain by calcineurin. Circulation 118, 2268–2276. doi: 10.1161/CIRCULATIONAHA.107.750869
Todaro, M., Iovino, F., Eterno, V., Cammareri, P., Gambara, G., Espina, V., et al. (2010). Tumorigenic and metastatic activity of human thyroid cancer stem cells. Cancer Res. 70, 8874–8885. doi: 10.1158/0008-5472.CAN-10-1994
Tomer, Y. (2014). Mechanisms of autoimmune thyroid diseases: from genetics to epigenetics. Annu. Rev. Pathol. 9, 147–156. doi: 10.1146/annurev-pathol-012513-104713
van der Spek, A. H., Surovtseva, O. V., Jim, K. K., van Oudenaren, A., Brouwer, M. C., Vandenbroucke-Grauls, C. M. J. E., et al. (2018). Regulation of intracellular triiodothyronine is essential for optimal macrophage function. Endocrinology 159, 2241–2252. doi: 10.1210/en.2018-2053
van Rooij, E., Quiat, D., Johnson, B. A., Sutherland, L. B., Qi, X., Richardson, J. A., et al. (2009). A family of microRNAs encoded by myosin genes governs myosin expression and muscle performance. Dev. Cell 17, 662–673. doi: 10.1016/j.devcel.2009.10.013
Vargas, R., and Videla, L. A. (2017). Thyroid hormone suppresses ischemia-reperfusion-induced liver NLRP3 inflammasome activation: role of AMP-activated protein kinase. Immunol. Lett. 184, 92–97. doi: 10.1016/j.imlet.2017.01.007
Vernon, P. J., and Tang, D. (2013). Eat-me: autophagy, phagocytosis, and reactive oxygen species signaling. Antioxid. Redox. Signal. 18, 677–691. doi: 10.1089/ars.2012.4810
Vidart, J., Wajner, S. M., Leite, R. S., Manica, A., Schaan, B. D., Larsen, P. R., et al. (2014). N-acetylcysteine administration prevents nonthyroidal illness syndrome in patients with acute myocardial infarction: a randomized clinical trial. J. Clin. Endocrinol. Metab. 99, 4537–4545. doi: 10.1210/jc.2014-2192
Viganò, A., Riboni, S., Bianchi, R., Cafarelli, L., Vago, T., Manzoni, P., et al. (2004). Thyroid dysfunction in antiretroviral treated children. Pediatr. Infect. Dis. J. 23, 235–239. doi: 10.1097/01.inf.0000114903.05472.e4
von Hafe, M., Neves, J. S., Vale, C., Borges-Canha, M., and Leite-Moreira, A. (2019). The impact of thyroid hormone dysfunction on ischemic heart disease. Endocr Connect 8, R76–R90. doi: 10.1530/EC-19-0096
Wai Lui, D. T., Lee, C. H., Chow, W. S., Hong Lee, A. C., Tam, A. R., Yi Fong, C. H., et al. (2020). Thyroid dysfunction in relation to immune profile, disease status and outcome in 191 patients with COVID-19. J. Clin. Endocrinol. Metab. doi: 10.1210/clinem/dgaa813 Online ahead of print.
Wajner, S. M., Goemann, I. M., Bueno, A. L., Larsen, P. R., and Maia, A. L. (2011). IL-6 promotes nonthyroidal illness syndrome by blocking thyroxine activation while promoting thyroid hormone inactivation in human cells. J. Clin. Invest. 121, 1834–1845. doi: 10.1172/JCI44678
Wang, J. X., Jiao, J. Q., Li, Q., Long, B., Wang, K., Liu, J. P., et al. (2011). miR-499 regulates mitochondrial dynamics by targeting calcineurin and dynamin-related protein-1. Nat. Med. 17, 71–78. doi: 10.1038/nm.2282
Wang, L., Negro, R., and Wu, H. (2020). TRPM2, linking oxidative stress and Ca2+ permeation to NLRP3 inflammasome activation. Curr. Opin. Immunol. 62, 131–135. doi: 10.1016/j.coi.2020.01.005
Wang, Y., Men, M., Yang, W., Zheng, H., and Xue, S. (2015). MiR-31 downregulation protects against cardiac ischemia/reperfusion injury by targeting Protein Kinase C Epsilon (PKCε) directly. Cell Physiol. Biochem. 36, 179–190. doi: 10.1159/000374062
Wang, Z., Zhang, S., Xiao, Y., Zhang, W., Wu, S., Qin, T., et al. (2020). NLRP3 inflammasome and inflammatory diseases. Oxid. Med. Cell Longev. 17, 4063562. doi: 10.1155/2020/4063562
Warner, M. H., and Beckett, G. J. (2010). Mechanisms behind the non-thyroidal illness syndrome: an update. J. Endocrinol. 205, 1–13. doi: 10.1677/JOE-09-0412
West, P. A., Brodsky, I. E., Rahner, C., Woo, D. K., Erdjument-Bromage, H., Tempst, P., et al. (2011). TLR signaling augments macrophage bactericidal activity through mitochondrial ROS. Nature 472, 476–480. doi: 10.1038/nature09973
Wynn, T. A., Chawla, A., and Pollard, J. W. (2013). Macrophage biology in development, homeostasis and disease. Nature 496, 445–455. doi: 10.1038/nature12034
Keywords: human immunodeficiency virus, hypothalamic–pituitary–thyroid, immune system, inflammasome, microRNAs, non-thyroidal illness syndrome, thyroid hormones, Wnt/β-catenin
Citation: De Luca R, Davis PJ, Lin H-Y, Gionfra F, Percario ZA, Affabris E, Pedersen JZ, Marchese C, Trivedi P, Anastasiadou E, Negro R and Incerpi S (2021) Thyroid Hormones Interaction With Immune Response, Inflammation and Non-thyroidal Illness Syndrome. Front. Cell Dev. Biol. 8:614030. doi: 10.3389/fcell.2020.614030
Received: 05 October 2020; Accepted: 14 December 2020;
Published: 21 January 2021.
Edited by:
Isabel Merida, Consejo Superior de Investigaciones Científicas (CSIC), SpainReviewed by:
Carlos Del Fresno, Spanish National Centre for Cardiovascular Research, SpainGuadalupe Sabio, Spanish National Centre for Cardiovascular Research, Spain
Copyright © 2021 De Luca, Davis, Lin, Gionfra, Percario, Affabris, Pedersen, Marchese, Trivedi, Anastasiadou, Negro and Incerpi. This is an open-access article distributed under the terms of the Creative Commons Attribution License (CC BY). The use, distribution or reproduction in other forums is permitted, provided the original author(s) and the copyright owner(s) are credited and that the original publication in this journal is cited, in accordance with accepted academic practice. No use, distribution or reproduction is permitted which does not comply with these terms.
*Correspondence: Roberto De Luca, cmRlbHVjYUBiaWRtYy5oYXJ2YXJkLmVkdQ==; Roberto Negro, cm9iZXJ0by5uZWdyb0BpcmNjc2RlYmVsbGlzLml0; Sandra Incerpi, c2FuZHJhLmluY2VycGlAdW5pcm9tYTMuaXQ=; c2FuZHJhLmluY2VycGlAZ21haWwuY29t
†These authors have contributed equally to this work and share senior authorship