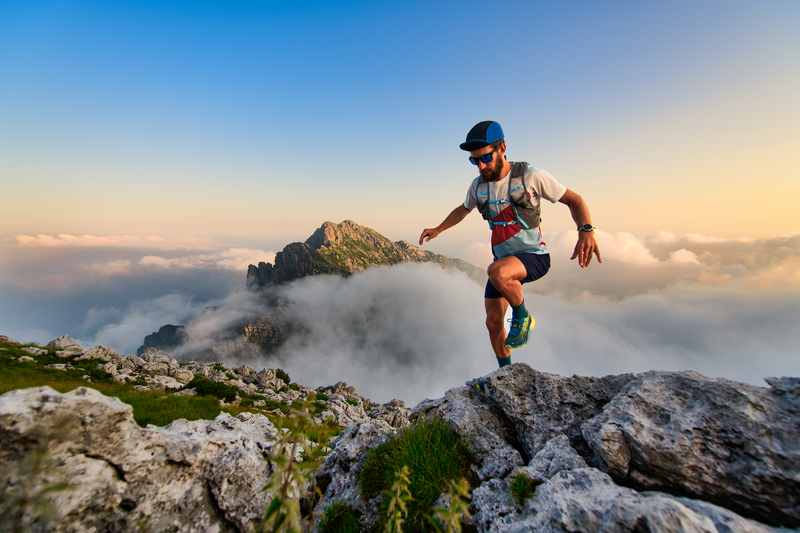
94% of researchers rate our articles as excellent or good
Learn more about the work of our research integrity team to safeguard the quality of each article we publish.
Find out more
ORIGINAL RESEARCH article
Front. Cell Dev. Biol. , 25 January 2021
Sec. Cellular Biochemistry
Volume 8 - 2020 | https://doi.org/10.3389/fcell.2020.609493
This article is part of the Research Topic Mitochondrial Remodeling and Dynamic Inter-Organellar Contacts in Cardiovascular Physiopathology View all 12 articles
Besides skeletal muscle dysfunction, Duchenne muscular dystrophy (DMD) exhibits a progressive cardiomyopathy characterized by an impaired calcium (Ca2+) homeostasis and a mitochondrial dysfunction. Here we aimed to determine whether sarco-endoplasmic reticulum (SR/ER)–mitochondria interactions and mitochondrial function were impaired in dystrophic heart at the early stage of the pathology. For this purpose, ventricular cardiomyocytes and mitochondria were isolated from 3-month-old dystrophin-deficient mice (mdx mice). The number of contacts points between the SR/ER Ca2+ release channels (IP3R1) and the porine of the outer membrane of the mitochondria, VDAC1, measured using in situ proximity ligation assay, was greater in mdx cardiomyocytes. Expression levels of IP3R1 as well as the mitochondrial Ca2+ uniporter (MCU) and its regulated subunit, MICU1, were also increased in mdx heart. MICU2 expression was however unchanged. Furthermore, the mitochondrial Ca2+ uptake kinetics and the mitochondrial Ca2+ content were significantly increased. Meanwhile, the Ca2+-dependent pyruvate dehydrogenase phosphorylation was reduced, and its activity significantly increased. In Ca2+-free conditions, pyruvate-driven complex I respiration was decreased whereas in the presence of Ca2+, complex I-mediated respiration was boosted. Further, impaired complex I-mediated respiration was independent of its intrinsic activity or expression, which remains unchanged but is accompanied by an increase in mitochondrial reactive oxygen species production. Finally, mdx mice were treated with the complex I modulator metformin for 1 month. Metformin normalized the SR/ER-mitochondria interaction, decreased MICU1 expression and mitochondrial Ca2+ content, and enhanced complex I-driven respiration. In summary, before any sign of dilated cardiomyopathy, the DMD heart displays an aberrant SR/ER-mitochondria coupling with an increase mitochondrial Ca2+ homeostasis and a complex I dysfunction. Such remodeling could be reversed by metformin providing a novel therapeutic perspective in DMD.
Duchenne muscular dystrophy (DMD) is the most common X-linked disorder (1/3,500 newborn male affected) caused by non-sense mutations in dystrophin gene and resulting in the absence of the large protein dystrophin (427 kDa) (Hoffman, 2020). Dystrophin links the cytoskeleton to a complex of proteins at the cell surface, which, in turn, interacts with the extracellular matrix. Dystrophin deficiency causes progressive muscle weakness and cardiac failure. Cardiac involvement is inevitable and progresses with age toward a dilated cardiomyopathy (DCM) with an increased frequency of ventricular arrhythmia and sudden cardiac death. Among DMD patients, the cardiac phenotype varies with age from no discernible cardiac remodeling or dysfunction to early onset of DCM with heart failure (Sasaki et al., 1998; Amedro et al., 2019; Segawa et al., 2020). Whereas more than 90% of DMD patients display echocardiographic features of left ventricular remodeling and contractile dysfunction by the age of 18 years 11/26/20 5:34:00 PM, in the murine model of DMD, the mdx mice, similar defects are evident at 42 weeks of age (Quinlan et al., 2004).
At the cellular level, altered calcium (Ca2+) homeostasis is one of the first pathophysiological features associated with dystrophin deficiency. Before any sign of DCM and cardiomyopathy, the calcium channels of the sarcoplasmic reticulum (SR), the type 2 ryanodine receptors (RyR2), are leaky due to posttranslational remodeling (Fauconnier et al., 2010). Associated with an increased Ca2+ influx due to sarcolemmal damages and overactivation of stretch-activated channels and/or L-type Ca2+ current, these defects increase the diastolic Ca2+ level and promote fatal cardiac arrhythmias (Williams and Allen, 2007a; Jung et al., 2008; Ullrich et al., 2009; Fauconnier et al., 2010; Prosser et al., 2011). In parallel, DMD is associated with a progressive deterioration of the mitochondrial ultrastructure and function (Kyrychenko et al., 2015). Mitochondrial defects were mainly characterized in skeletal muscle where a significant uncoupling of the oxidative phosphorylation, defects in complex I, and a reduction of ATP synthesis were observed (Sperl et al., 1997; Kuznetsov et al., 1998; Percival et al., 2013; Rybalka et al., 2014; Moore et al., 2020). In heart muscle, a metabolic shift from fatty acid oxidation to carbohydrate oxidation has also been observed prior to the onset of DCM and heart failure (Khairallah et al., 2007, 2008; Burelle et al., 2010). Furthermore, mitochondrial Ca2+ uptake as well as mitochondrial reactive oxygen species (ROS) production and oxidative damages are increased in the DMD mdx mouse model and contribute to the pathogenesis of heart failure in DMD (Williams and Allen, 2007b; Dubinin et al., 2020; Hughes et al., 2020). Importantly, the first signs of mitochondrial and bioenergetic deficiencies precede the decline in myocardial function. Therefore, targeting mitochondrial function and/or metabolism in DMD has become a therapeutic issue over the last decade. Among the therapeutic candidates, the antidiabetic drug metformin has recently been evaluated in DMD both in preclinical studies and in clinical trials (Ljubicic and Jasmin, 2015; Hafner et al., 2016, 2019; Mantuano et al., 2018; Vitiello et al., 2019). This N,N-dimethylbiguanide interferes with the activity of mitochondrial complex I and activates AMP-activated protein kinase (AMPK), a critical hub for metabolic-mediated signaling pathways (for a review, see Foretz et al., 2014). Although the variability of the severity profile and the different stages of the pathology will require larger-scale clinical trials, treatment with metformin in combination with nitric oxide precursors has shown encouraging positive effects, particularly on motor function (Hafner et al., 2019). In mdx mice, long-term treatment with metformin alone also improves diaphragm function and limits muscle damage and the development of fibrosis due to exercise (Mantuano et al., 2018). To date, the only evidence of a beneficial effect of metformin in the development of cardiomyopathy associated with DMD is a decrease in the mass index of the heart (Mantuano et al., 2018).
The tight connection between the sarco-endoplasmic reticulum (SR/ER) and the mitochondria, also called mitochondria-associated ER membrane (MAM), is essential for the maintenance of energy metabolism, Ca2+ homeostasis, and cell fate (Rossini and Filadi, 2020). The tethering is maintained by mitofusin-2 (MFN2) as well as by a macromolecular complex comprising the SR/ER Ca2+ channel IP3 receptor 1 (IP3R1) and the porine of the outer membrane of the mitochondria (OMM), VDAC1 (voltage-dependent anion channel 1). These two channels are linked by a chaperone, GRP75, forming a direct Ca2+ channeling between the SR/ER and the mitochondria (Lee and Min, 2018). To cross the inner mitochondrial membrane (IMM), Ca2+ passes through the mitochondrial Ca2+ uniporter (MCU) complex creating an IP3R1–GRP75–VDAC–MCU Ca2+ transfer axis (Lee and Min, 2018). MCU is a highly selective Ca2+ channel which comprises a membrane-spanning 40-kDa protein that forms a low-conductance Ca2+-selective pore. MCU exists as a large protein complex (~480 kDa) comprising MICU1 and MICU2 which give it its Ca2+ sensitivity (Tarasova et al., 2019). This SR/ER–mitochondria interaction and contact points have never been studied in DMD hearts. However, at the early stage of the pathology, we have recently demonstrated an impaired SR/ER–mitochondria interaction in mdx skeletal muscles associated with an alteration of Ca2+ homeostasis, and increase in the unfolding protein response (UPR or ER stress) and muscle weakness (Pauly et al., 2017).
The aim of this study was thus to establish whether, in mdx mice that show no signs of DCM, the interconnection between SR/ER and mitochondria is modified and whether it may interfere with myocardial mitochondrial function. Our results demonstrate that increased SR/ER–mitochondria interaction is associated with increased mitochondrial Ca2+ content and a disrupted mitochondrial function. One-month metformin treatment reversed these defects providing a novel therapeutic perspective in DMD.
All experiments were conformed to the institutional ethics committee for animal experiments and received the agreement from the national Ministère de l'enseignement supérieur et de la recherche (N° #16473-2018082016141320). Male mdx or WT (wild-type, C57bl/10ScSn) mice (CNRS, IGMM, France) were used at 3 months of age. According to the animal-to-human translation factor for drug treatments and as previously shown, a daily dose of metformin of 200 mg/kg/day was given for 1 month in 50 ml of drinking water (Reagan-Shaw et al., 2008; Mantuano et al., 2018). Mice receiving metformin treatment were housed in a single cage to ensure homogeneity between groups. Left ventricular myocytes were enzymatically dissociated as previously described (Fauconnier et al., 2005). In brief, after removal, the heart was retrogradely perfused at 37°C with a modified enzyme solution [0.1 mg.mL−1 of Liberase™ (Roche)] containing 113 mM NaCl, 4.7 mM KCl, 0.6 mM KH2PO4, 0.6 mM Na2HPO4, 1.2 mM MgSO4, 12 mM NaHCO3, 10 mM KHCO3, 10 mM Hepes, 30 mM taurine (pH 7.4). Isolated myocytes were then transferred to the same enzyme-free solution containing 1 mM CaCl2 prior experiments.
Twenty to thirty micrograms of total or mitochondrial protein was separated on SDS-polyacrylamide gel and electro-blotted onto a nitrocellulose membrane (Bio-Rad). Membranes were saturated with blocking buffer for 1 h at room temperature and incubated overnight at 4°C with monoclonal mouse anti-VDAC (1:1000, Abcam), anti-OXPHOS (1:5000, Abcam), anti-IP3R1 (1:1000, Santa Cruz), anti-NDUFA13 (1:1000, Abcam), anti-GAPDH (1:10 000, Abcam) or with polyclonal rabbit anti-MCU (1:500, Abcam), anti-MICU1 (1:500, Thermo Fisher), anti-MICU2 (1:500, Sigma Aldrich), anti-PDH-E1α (1:1000, Abcam), anti-PDHE1α phosphor Ser 293 (1:1000, Abcam), anti-PDHE1α phosphor Ser 300 (1:1000, Millipore), anti-PDHE1α phosphor Ser 232 (1:1000, Calbiochem), anti-PDK4 (1:1000 Novus Biotech), anti-GRP75 (1:1000, Santa Cruz), anti-SIGMA1R (1:1000, Cell Signaling), anti-MFN2 (1:1000, Abcam), and anti-Hsp60 (1:1000, Abcam). Hsp60 and GAPDH were used as loading controls. All immunoblots were developed and quantified using the Odyssey infrared imaging system (LICOR Biosystems) and infrared-labeled secondary antibodies. Band intensities were quantified with ImageJ.
Isolated cardiomyocyte RNA was extracted with the TRIzol Reagent (Life Technologies). The level of target mRNAs was measured by reverse transcription (Superscript II, Invitrogen, 1 μg total RNA) followed by quantitative real-time PCR using a RotorGene (Corbett Research). TATA-binding protein (TBP) mRNA was used as a housekeeping gene Tbp (forward 5′-TGGTGTGCACAGGAGCCAAG-3′, reverse 5′-TTCACATCACAGCTCCCCAC-3′). The primer sequences of ER stress-related genes are Hspa5 forward 5′-CCACCTCCAATATCAACTTG-3′, Hspa5 reverse 5′-ACGATCAGGGCAACCGCATCA-3′; Ddit3 forward 5′-CTGGAAGCCTGGTATGAGGA-3′, Ddit3 reverse 5′-CTCTGACTGGAATCTGGAGA-3′; total Xbp1 forward 5′-GTGCAGGCCCAGTTGTCACC-3′, Xbp1 reverse 5′-TCTGGGTAGACCTCTGGGAG-3′; U-Xbp1 forward 5′-CAGACTATGTGCACCTCTGC-3′, U Xbp1 reverse 5′-TCTGGGTAGACCTCTGGGAG-3′; Atf3 forward 5′-CCAGGTCTCTGCCTCAGAAG-3′, Atf3 reverse 5′-CATCTCCCAGGGGTCTGTTGT-3′; Atf4 forward 5′-TCGATGCTCTGTTTCGAATG-3′, Atf4 reverse 5′-AGAATGTAAAGGGGGCAACC-3′; Atf6 forward 5′-TCGAGGCTGGGTTCATAGAC-3′, Atf6 reverse 5′-CTGTGTACTGGACAGCCATC-3′.
The Proximity Ligation Assay (PLA) was performed as previously described (Paillard et al., 2013; Pauly et al., 2017). Cells were fixed with paraformaldehyde 4% and permeabilized at RT with 0.1% Triton-X100. After washing, they were incubated with blocking buffer for 30 min at 37°C. The blocking solution was removed before incubation of primary antibodies (anti-VDAC Abcam, ab1734, 1:200, and anti-IP3R1, Santa Cruz, sc-28614, 1:200, or anti-GRP75, Santa Cruz, sc-13967) overnight at 4°C. The cells were washed two times using PBS with 0.01% Tween. The two PLA probes 1:5 were prepared in antibody diluent 20 min before incubation for 1 h at 37°C. Next, cells were incubated with mix containing 5× ligation stock (diluted 1:5 in water) and 1× ligation solution (diluted 1:40) for 30 min at 37°C. Next, the cells were incubated with mix containing 5× amplification stock (diluted 1:5 in water) and polymerase (diluted 1:80) for 100 min at 37°C. Finally, the cells were washed with Dapi (diluted 1:500) in wash buffer B 1× and mounted using Dako fluorescent mounting medium (S3023) and analyzed using a fluorescence microscope (excitation: 594 nm, emission: 624 nm, magnification: 40×).
Mitochondrial isolation protocols were performed and adapted from Frezza et al. (2007). Briefly, left ventricles (LV) or isolated cardiomyocytes were homogenized with Teflon pestle in cold isolation buffer (pH 7.4, 225 mM Mannitol, 75 mM Sucrose, and 30 mM Tris with anti-protease 1×) and homogenized by 10 strokes with a motorized Potter Elvejhem (1500 rpm). Nuclei and cellular debris were pelleted at 800 g for 10 min at 4°C. Supernatants containing mitochondria were centrifuged twice at 9000 g for 10 min to pellet mitochondria. Mitochondria were resuspended in isolation buffer, and protein concentration was determined by a bicinchoninic assay kit. Isolated mitochondria pellets were diluted in HCl 0.6 N, homogenized, and sonicated. After incubation during 30 min in boiling water, mitochondria were centrifuged for 5 min at 10 000 g, and the supernatant was recovered. Ca2+ content in supernatant was determined spectrophotometrically (Tecan) using an o-cresolphthalein complexone assay according to the manufacturer's instructions (TECO Diagnostics). Results are expressed in mM of Ca2+ per μg of protein.
Respiration was measured on digitonin-permeabilized cardiomyocytes (15 μg per million cells) in the respiratory buffer containing 10 mM KH2PO4, 3 mM MgCl2·6H2O, 0.5 mM EGTA, 20 mM taurine, 60 mM K-lactobionate, 20 mM HEPES, 110 mM sucrose, and 1 g/L BSA, pH 7.1. The respiratory rates of 10 000 cardiomyocytes were recorded at 37°C using a high-resolution Oxygraph respirometer (Oroboros, Innsbruck, Austria) after addition of different substrates. Briefly, the aerobic glycolysis pathway was stimulated by addition of pyruvate and malate. Pyruvate, the last substrate generated by glycolysis, and malate activate the Krebs cycle after their conversion in acetyl coenzyme A and oxaloacetic acid, respectively. Under these conditions, the complex I of the mitochondrial respiratory chain was activated by NADH produced. Complex II-driven respiration was obtained by addition of succinate and rotenone (to inhibit the complex I), and complex IV-driven respiration was permitted by addition of antimycin A (to inhibit the complex III), ascorbate and N,N,N′,N′-tetramethyl-p-phenylenediamine dihydrochloride (TMPD) to reduce cytochrome c. In some experiments, the free Ca2+ was set to 400 nM in the respiratory buffer using the Maxchelator program (https://somapp.ucdmc.ucdavis.edu/pharmacology/bers/maxchelator/). The respiratory coupling ratio (RCR) was measured in all conditions, and no differences between groups were observed (Supplementary Figure 1). Mitochondrial Ca2+ uptake was measured with Calcium Green (Invitrogen) with the O2k-Fluorescence LED2-Module on isolated mitochondria in the presence of thaspigargin (10 μM). The decay time constant of the calcium green fluorescence was measured after application of 25 μM extramitochondrial Ca2+ and reflects mitochondrial Ca2+ uptake.
Activity of the pyruvate dehydrogenase (PDH) was obtained by using Pyruvate Dehydrogenase Enzyme Activity Microplate Assay (Abcam ab109902) and 100 μg of mitochondria isolated from heart homogenates according to the manufacturer's instruction. Mitochondrial enzymatic activities of complexes I and IV and citrate synthase were measured on mitochondria isolated from heart homogenates at 37°C using a SAFAS spectrophotometer and standard method (Angebault et al., 2018).
Mitochondrial anion superoxide production was monitored using the fluorescent indicator MitoSOX Red (Invitrogen) and confocal microscopy as described previously (Fauconnier et al., 2007). Briefly, cardiomyocytes were loaded with MitoSOX Red (5 μM) for 30 min at room temperature, followed by washout. Confocal images were obtained after 5 min of 1-Hz stimulation in standard Tyrode solution and subsequently after addition of antimycin A (50 μM) to estimate the maximal complex I superoxide production capacity. MitoSOX Red fluorescence was measured in the same region of the cell at each time point. The signal from each cell was normalized to that immediately before pacing.
All data are presented as mean ± SEM. Normality of distribution, controlled by Agostino–Person omnibus normality test, not being respected; the Mann–Whitney test was used for all experiments. For multiple comparisons, the non-parametric Kruskal–Wallis test was applied. The significance level was set at α = 0.05.
We first determined whether SR/ER–mitochondria interactions are altered in mdx mouse cardiomyocytes as previously reported in skeletal muscle, namely, a decrease in SR/ER–mitochondria interaction (Pauly et al., 2017). For this purpose, we first measured the expression level of proteins involved in the SR/ER-mitochondria tethering. Although the MFN2 expression as well as VDAC1 and GRP75 levels were similar in both groups, IP3R1 and its regulatory subunits SIGMA-1R (Sig-1R) were significantly increased in mdx cardiomyocytes (Figures 1A,B). The expression of sarco/endoplasmic reticulum Ca2+-ATPase type 2a (SERCA2a) and its regulatory protein, phospholamban (PLB), and its phosphorylation were also unchanged, although the Ca2+ load of the SR was reduced (Supplementary Figure 2). We next evaluated the contact points between the two organelles using the in situ PLA assay. The IP3R1–VDAC1 interaction was significantly enhanced as well as the GRP75–VDAC1 connections, indicating that the physical SR/ER–mitochondria interconnection via IP3R1 and VDAC is enhanced in mdx cardiomyocytes (Figure 1C). However, the immunoprecipitation of IP3R1 did not show a difference in the VDAC/IP3R1 and GRP75/IP3R1 ratio meaning that the increase is at least in part related to the increase in the expression of IP3R1 (Supplementary Figure 3). In addition, except Atf3 gene expression which increases, mRNA levels of ER stress markers were unchanged in mdx heart (Supplementary Figure 4). The increased IP3R1–GRP75–VDAC1 interaction is supposed to enhance the direct channeling for Ca2+ from the SR/ER to the mitochondria. This is reinforced by an increase in both MCU and MICU1 expression level as reported recently (Dubinin et al., 2020). Importantly, the MICU2 expression remained unchanged (Figures 2A,B). This structural remodeling was associated with a faster mitochondrial Ca2+ uptake indicating functional changing of the MCU complex (Figure 2C). Similarly, mitochondrial Ca2+ content was also significantly elevated compared to WT mitochondria (Figure 2D). Unlike skeletal muscle, these data indicate an increase in SR/ER–mitochondria interactions and mitochondrial Ca2+ uptake in the mdx heart.
Figure 1. Increase in interconnection between SR/ER and mitochondria in mdx cardiomyocytes. All quantifications of proteins were normalized either to GAPDH when heart homogenates were used or to Hsp60 when Western blot were performed on isolated mitochondria and expressed relative to WT. (A) Representative immunoblots and quantification (B) of IP3R1, GRP75, Sig-1R, and VDAC. Quantification of IP3R1, GRP75, MFN2, and Sig-1R was carried out from N = 6 mdx and WT hearts and from N = 5 mdx and WT hearts for VDAC. Data are mean ± SEM. For IP3R1 *p = 0.0317 and for Sig-1R *p = 0.0043 mdx vs. WT. (C) Representative images and quantitative analysis of IP3R1-VDAC and GRP75-VDAC1 interaction measured by in situ PLA on isolated cardiomyoctytes from mdx (N = 3, n > 49) and WT (N = 3; n > 44). The numbers of dots were normalized to the number of nucleus per cells. Data are mean ± SEM. *p = 0.0057 mdx vs. WT for IP3R1-VDAC and p < 0.0001 mdx vs. WT for GRP75-VDAC1.
Figure 2. Increase MCU/MICU1 expression and mitochondrial Ca2+ in mdx cardiomyocytes. All Western blots were performed on isolated mitochondria and quantifications of proteins were normalized to Hsp60 and expressed relative to WT. (A) Representative immunoblots and quantification (B) of MCU, MICU1, and MICU2. Data are mean ± SEM, *p = 0.0043 and *p = 0.0022 for MCU and MICU1, respectively, mdx (N = 6) vs. WT (N = 6). (C) The mean rate of mitochondrial Ca2+ uptake measured as the decay time constant of calcium green fluorescence in presence of 25 μM of Ca2+. Data are mean ± SEM, *p = 0.0476 mdx (N = 6) vs. WT (N = 5). (D) Mean of the absolute mitochondrial Ca2+ content in mdx (N = 6) and WT (N = 6) hearts. Data are mean ± SEM, *p = 0.0152 mdx vs. WT.
Once in the mitochondrial matrix, Ca2+ regulates several processes that interfere with the mitochondrial function and the metabolic flux. Among them, Ca2+ controls the activity of the PDH, which is the entry point for the glycolytic product pyruvate into the oxidative metabolism. The PDH phosphorylation by the PDH kinase (PDK) inhibits its activity whereas its dephosphorylation by the Ca2+-dependent PDH phosphatase (PDP) increases its activity. We thus measured the PDH phosphorylation in mdx LV mitochondria. The total PDH content and the PDK4 expression were comparable in both groups; however, the phosphorylation levels of the three phosphorylable serines (pPDH-ser232, pPDH-ser293, and pPDH-ser 300) were significantly reduced (Figures 3A,B; Supplementary Figure 5). Furthermore, the PDH activity was significantly increased in LV mdx mitochondria consistent with the decrease in PDH phosphorylation (Figures 3B,C).
Figure 3. Increase PDH activity in mdx hearts. All Western blots were performed on isolated mitochondria and quantifications of proteins were normalized to Hsp60 and expressed relative to WT. (A) Representative immunoblots and (B) quantification of total pyruvate dehydrogenase subunit E1α (PDH-E1α), PDH phosphorylated on Serine 232 (*p = 0.0262), 293 (*p = 0.0499), and 300 (*p = 0.0281). Data are mean ± SEM, mdx (N = 8) vs. WT (N = 8). (C) Mean PDH activity measured on isolated mitochondria. Data are mean ± SEM, *p = 0.0043 mdx (N = 6) vs. WT (N = 6).
Increased PDH activity may impact carbohydrate metabolic flux and pyruvate-mediated oxidative phosphorylation. We next measured maximal mitochondrial respiration rates in a Ca2+-free conditions using pyruvate/malate as carbohydrate substrates to feed the complex I. In the presence of ADP, the complex I-mediated oxygen consumption was significantly reduced whereas complex II- and complex IV-related respiration and the RCR were comparable in both groups (Figure 4A; Supplementary Figure 1). These results indicate that only the complex I-driven oxygen consumption is altered in mdx mice. In order to determine whether the decrease in the respiration mediated by complex I is related to Ca2+, we measured complex I-driven oxygen consumption in the presence of 400 nM Ca2+. In this condition, the complex I-mediated respiration rate was significantly enhanced in mdx mitochondria compared to WT (Figure 4B), while the expression of complex I subunits is unchanged (Figures 4C,D). It is noteworthy that the expression of the other complexes is also unchanged compared to WT (Supplementary Figure 6). Another parameter which could explain the decrease in complex I-driven oxygen consumption is the capacity of the complex I to transfer electron. We performed quantification of enzymatic activity, but we did not observe any modification in enzymatic activities of complexes I and IV nor of the activity of citrate synthase in mdx mice (Figure 4E). A preserved complex I activity and a reduced complex I-mediated respiration could indicate an electron leak at the complex I level and superoxide anion (O2.−) production. We have indeed observed an increase in Mitosox red fluorescence in paced mdx cardiomyocytes but also in the presence of the complex III inhibitor antimycin A, demonstrating an increase in the production of O2.− at the level of complex I compared to WT (Figure 4F). Altogether, these data indicate an impaired carbohydrate-mediated mitochondrial respiration at the level of complex I which is partly compensated by the increase in mitochondrial Ca2+.
Figure 4. Impaired complex I-mediated mitochondrial respiration in mdx hearts. (A) Respiration rates under glycolysis protocols in permeabilized isolated ventricular cardiomyocytes from WT and mdx mice (N = 5–7). Complex I-dependent respiration State 3 (EIII) is determined in presence of malate and pyruvate (CxI). Complex II and IV-dependent respiration State 3 (EIII) is obtained by addition of succinate and rotenone (CxII) and ascorbate/TMPD (CxIV), respectively. Data are mean ± SEM, *p = 0.0379 mdx vs. WT. (B) Mean complex I-dependent respiration in the presence of 400 nM extramitochondrial Ca2+ relative to 0 nM Ca2+. Data are mean ± SEM, *p = 0.0260 mdx (N = 6) vs. WT (N = 6). (C) Representative immunoblots and (D) quantification of two subunits of complex I (NDUFA13: CxI 13 and NDUFB8: CxI 20). Isolated mitochondria from N = 6 hearts were tested in mdx and WT mice, and Hsp60 was used as loading control and expressed relative to WT. Data are mean ± SEM. (E) Enzymatic activities of complexes I and IV and citrate synthase measured on isolated mitochondria from Data are means ± SEM, p > 0.05 mdx (N = 5) vs. WT (N = 5). (F) Mitochondrial ROS production is evaluated under confocal microscopy with MitoSOX red and expressed as percentage of baseline after electric stimulation at 1 Hz (*p = 0.0071) or Antimycin A (*p = 0.0435) addition. Data are mean ± SEM, mdx (N = 10) vs. WT (N = 9).
We next investigated the effects of a treatment with metformin, the antidiabetic drugs targeting mitochondrial complex I. After 1 month in drinking water, the level of phosphorylation of AMPK and acetyl-CoA carboxylase (ACC), one of the downstream targets of AMPK, was significantly increased validating the efficacy treatment with metformin (Supplementary Figure 7). We next evaluated the SR/ER–mitochondria interaction using in situ PLA assay. As shown in Figure 5, metformin treatment decreased IP3R1/VDAC contacts (Figures 5A,B) whereas the expression levels of IP3R1, VDAC, GRP75, or Sig-1R remained similar (Supplementary Figure 7). Although MCU expression is also unchanged, MICU1 level is decreased and this is accompanied by a decrease in the mitochondrial Ca2+ content (Figures 5C,D). The decrease in mitochondrial Ca2+ content is accompanied by an increase of the phosphorylation level of the PDH but only on the Ser232 site (Figures 6A,B). However, this is not sufficient to affect the PDH activity which remained comparable to untreated mdx mice (Figure 6C). Finally, the complex I-mediated oxygen consumption under pyruvate/malate substrate is significantly enhanced (Figure 6D), indicating that metformin treatment in addition to restoration of SR/ER-mitochondrial interactions optimizes mitochondrial function in mdx ventricular cardiomyocytes.
Figure 5. Chronic metformin treatment decreases SR/ER and mitochondria interaction and mitochondrial Ca2+ content in mdx cardiomyocytes. All Western blots were performed on isolated mitochondria, and quantifications of proteins were normalized to Hsp60 and expressed relative to mdx. (A, B) Representative images and quantitative analysis of IP3R1–VDAC interaction measured by in situ PLA on isolated cardiomyocytes from mdx (N = 3, n = 49) and mdx + metformin (N = 2; n = 33). The numbers of dots were normalized to the number of nucleus per cells and expressed relative to mdx mean value. Mean ± SEM, *p = 0.046 mdx vs. mdx + metformin. (C) Representative immunoblots and quantification of MCU and MICU1. Data are mean ± SEM, *p = 0.0303 mdx (N = 6) vs. mdx + metformin (N = 6). (D) Mean of the absolute mitochondrial Ca2+ content in mdx (N = 4) and mdx + metformin (N = 6) hearts. Mean ± SEM, *p = 0.0095 mdx vs. mdx + metformin.
Figure 6. Chronic metformin treatment increases PDH Ser232 phosphorylation and complex I-mediated respiration in mdx hearts. All Western blots were performed on isolated mitochondria and quantifications of proteins were normalized to Hsp60 and expressed relative to mdx. (A) Representative immunoblots and (B) quantification of total pyruvate dehydrogenase subunit E1α (PDH-E1α), PDH phosphorylated on Serine 232, 293, and 300. Data are mean ± SEM, *p = 0.0317 mdx (N = 4–6) vs. mdx + metformin (N = 4–6). (C) Mean PDH activity measured on isolated mitochondria. Data are mean ± SEM, p > 0.05 mdx (N = 6) vs. mdx + metformin (N = 7). (D) Complex I-dependent respiration State 3 (EIII) is determined in the presence of malate and pyruvate (CxI). Complex II- and IV-dependent respiration State 3 (EIII) is obtained by addition of succinate and rotenone (CxII) and ascorbate/TMPD (CxIV), respectively. Data are mean ± SEM, *p = 0.036 mdx (N = 6) vs. mdx + metformin (N = 9).
Dystrophin deficiency causes profound striated skeletal muscle lesions which lead to major muscle weakness at the early stage of the pathology and ultimately to fatal respiratory failure. Secondary to peripheral muscle deficiencies, a progressive cardiomyopathy develops with left ventricle dilatation, fibrosis, and arrhythmias (Sasaki et al., 1998; Fauconnier et al., 2010; Amedro et al., 2019; Segawa et al., 2020). Over the last decade, due to the improvement of the management of patients' respiratory failure, cardiac failure has become a major cause of death. Although cardiac and skeletal muscles share similar pathophysiological mechanisms, which are more or less shifted in time, some of these processes appear to be regulated differently. Indeed, despite an increase of IP3R1 expression in both tissues, here we demonstrated an increase in SR/ER–mitochondria interactions, characterized by an increase in IP3R1–GRP75–VDAC contact points, whereas in skeletal fibers such interactions were decreased (Pauly et al., 2017). Interestingly, the IP3R1/GRP75 or IP3R1/VDAC1 protein–protein binding remained unchanged, indicating that the elevation in PLA signals is most likely related to an increase in IP3R1 expression. Although the expression of MFN2 is unchanged, electron microscopy would be useful to establish whether the SR/ER–mitochondria tethering, and the physical distances between the two organelles, is disturbed in mdx hearts prior to the development of DCM. IP3Rs are involved in many cellular processes like metabolism, secretion, gene transcription, cell fate, and ER stress (Kiviluoto et al., 2013), but compared to RyR2 which is 50–100 times more expressed in left ventricles, the contribution of the IP3Rs to the cardiac beat-to-beat Ca2+ homeostasis and SR Ca2+ leak is unlikely (Kockskämper et al., 2008).
Increase of IP3R1 expression is accompanied by an elevation in Sig-1R, a transmembrane chaperone located in MAMs interacting with IP3Rs and ER stress sensors (for review Delprat et al., 2020). Sig-1R has been shown to (i) stabilize IP3R1 in MAMs contributing to the strengthening of ER–mitochondria contact and ER–mitochondria Ca2+ transfer and (ii) bind the protein chaperone BiP in the ER lumen stabilizing the ER stress response proteins (Hayashi and Su, 2007). Although a prolonged disrupted IP3R1–GRP75–VDAC interaction promotes ER stress (Rieusset et al., 2016; Pauly et al., 2017), an increase in contact points and Sig-1R expression has been reported in the early stage of the UPR response to sustain cell homeostasis and bioenergetics and alleviate ER stress (Hayashi and Su, 2007; Bravo et al., 2011; Delprat et al., 2020). Consistently, in contrast to skeletal muscle and with the exception of Atf3, UPR-inducible genes and ER sensor expression remained unchanged in the present study. Of note, the absence of ER stress response in the mdx heart does not exclude a mitochondrial stress response per se.
As indicated, the mitigation of ER stress might be related to an enhancement of Ca2+ transfer and mitochondrial function (Bravo et al., 2011). Here, the increase in the IP3R1–GRP75–VDAC1 juxtaposition was associated with an increase in the MCU–MICU1 complex suggesting a reinforcement of the IP3R1–GRP75–VDAC–MCU Ca2+ transfer axis. As shown recently by Dubinin et al. (2020), we also observed an increase expression of MICU1. MICU1/2 subunits form heterodimers located in the mitochondrial intermembrane space regulating the Ca2+-dependent gating and threshold properties of the MCU. At low Ca2+ concentrations, the MICU1/MICU2 dimer keeps MCU in the close state, and at higher Ca2+ level, MICU2-dependent inhibition is released and MICU1 activates MCU allosterically (Payne et al., 2017; Tarasova et al., 2019). An increase in the MICU1/MICU2 ratio reduces the Ca2+ threshold for MCU activation and increases the Ca2+ sensitivity of the MCU complex (Payne et al., 2017). Here, increased expression of MCU and MICU1 is not associated with a change in MICU2, which may in addition to decreased expression of the dominant negative isoform of MCU (MCUb) enhanced the mitochondrial Ca2+ uptake and content as shown in mdx ventricular mitochondria [Figure 2; (Dubinin et al., 2020)]. This change in MICU1 expression may affect the pharmacology of MCU and should be considered for future therapeutic strategies aimed at directly targeting mitochondrial Ca2+ uptake (Kon et al., 2017; Márta et al., 2020). The elevation of mitochondrial Ca2+ content is therefore the result of a remodeling of the MCU complex associated with the increase in the leakage of Ca2+ from the SR, the elevation of diastolic Ca2+, and the strengthening of the contact points between the two organelles.
More generally, the enhancement of mitochondrial Ca2+ uptake and content has several functional consequences. It increases the metabolic and respiration rate by stimulating the respiratory chain and the activities of several enzymes involved in the metabolic flux and the Krebs cycle. Among them, the PDH is the entry point for the glycolytic product pyruvate into the oxidative metabolism. In the mitochondrial matrix, Ca2+ activates the PDH phosphatase 1 that dephosphorylates PDH to increase its activity, and thus the use of carbohydrates for energy production. Here PDH phosphorylation at three serine residues (pSer232, pSer293, pSer300 in the mouse) on the alpha chain of the E1 subunit is significantly decreased in conjunction with an increase in PDH activity. This increases in PDH activity may account for the previously described elevation in the pyruvate decarboxylation and the shift from fatty acid to carbohydrate oxidation in the heart of both mdx and DMD patients (Perloff et al., 1984; Quinlivan et al., 1996; Momose et al., 2001; Naruse et al., 2004; Khairallah et al., 2007). PDH catalyzes the irreversible step of oxidative decarboxylation of pyruvate to produce acetyl-CoA and fuel the tricarboxylic acid cycle and electron transport chain. The increased PDH activity thus enhances the glycolytic flux, and pyruvate becomes the privileged substrate for the oxidative phosphorylation (Sun et al., 2015). However, in the absence of Ca2+ and as recently reported, the pyruvate-driven complex I respiration is reduced independently of any change in complex I expression level (Hughes et al., 2020). Such impairment might be related to posttranslational modifications; however, intrinsic complex I activity remained unchanged. It is noteworthy that mitochondrial respiration analyzed with substrates other than pyruvate, such as glutamate, does not appear to show any difference in mdx heart (Ascah et al., 2011; Viola et al., 2013). Remarkably, in the mdx diaphragm, the mitochondrial rate of ATP production was partly improved by directly stimulating Complex II, suggesting that Kreb's-driven NADH-dependent complex I function is defective (Rybalka et al., 2014). In addition, in the presence of Ca2+, complex I-mediated mitochondrial respiration is boosted, suggesting adaptive mechanisms to sustain the energy demand. On the one hand, it can increase the rate of pyruvate consumption to improve NADH production but, on the other hand, enhance electron leakage and mitochondrial ROS production (Williams and Allen, 2007b; Viola et al., 2013). Although an increase in mitochondrial ROS production is commonly observed in the heart of mdx (Williams and Allen, 2007b; Viola et al., 2013; Kuno et al., 2018; Hughes et al., 2020), the exact mechanisms linking complex I to ROS production remain to be established. It would be interesting to determine whether ROS are produced in the forward direction or in the reverse direction of electron transfer (Hirst and Roessler, 2016). Although we have not explored these mechanisms in detail, electron leakage from complex II is somewhat unlikely because the succinate-induced respiration is comparable in mdx and WT hearts. In the forward mode hypothesis, targeting the function of complex I may improve mitochondrial activity and metabolism.
We here tested metformin, an antidiabetic drug with pleiotropic properties that are related to its mitochondrial effects (for review see Foretz et al., 2014; Vial et al., 2019). Long-term treatment with metformin has already demonstrated some beneficial effects on motor function in stable DMD patients with encouraging evidence regarding muscle degeneration and histopathology (Hafner et al., 2016, 2019). The exact mechanisms of action of metformin have yet to be elucidated; however, at high doses, metformin inhibits the oxidation of NADH at the complex I level. Consequently, the ADP:ATP or AMP:ATP ratios increase which is thought to activate AMPK, a hub for major metabolic and energy sensing pathways (Horman et al., 2012; Foretz et al., 2014; He and Wondisford, 2015). In addition, at low doses, metformin has also been shown to activate AMPK independent of direct inhibition of complex I (He and Wondisford, 2015). In all cases, activation of AMPK demonstrated significant beneficial effects on the dystrophic phenotype in skeletal and cardiac muscles. Importantly, it improves mechanical signaling, muscle strength, and force; limits muscle necrosis, fibrosis, and inflammation; and stimulates the oxidative phenotype, mitochondrial function, and autophagy processes (Ljubicic et al., 2011, 2012; Pauly et al., 2012; Garbincius and Michele, 2015; Juban et al., 2018). Metformin has also been shown to increase PGC1-α expression in mdx muscle fibers, a central transcriptional coactivator regulating a wide range of biological processes such as mitochondrial biogenesis, oxidative phosphorylation, and muscle regeneration (Scarpulla, 2011; Ljubicic and Jasmin, 2015; Suntar et al., 2020). In addition, increased activation of AMPK stimulates autophagic clearance of defective mitochondria and may thus improve mitochondrial function (De Palma et al., 2012; Pauly et al., 2012). More generally, the maintenance of cell fate and cell proteostasis are emerging therapeutic targets in DMD (De Palma et al., 2012), but depending on the phase, severity, and inflammatory state of the pathology, the therapeutic window is critical for such emerging strategies (Farini et al., 2019). Here, in line with other studies, treatment with metformin also improves pyruvate-mediated mitochondrial respiration (Wang et al., 2019) and increasing the level of ACC phosphorylation would also facilitate fatty acid oxidation (Saddik et al., 1993). In parallel, MICU1 expression decreased but MCU did not, which is consistent with a recent report demonstrating a causal link between the pyruvate fluxe and consumption and MICU1 expression, suggesting that MICU1 could serve as a metabolic sensor (Nemani et al., 2020). Moreover, the IP3R1–VDAC1 interactions also decrease, indicating a reduction in the SR/ER–mitochondria contact points. Although the mechanism remains to be established, changes in SR/ER–mitochondria interaction are causally related to energy metabolism. AMPK activation has recently been shown to reduce the formation of cardiac MAM in hyperglycemia and decrease IP3-induced Ca2+ release (Arias-del-Val et al., 2019; Wu et al., 2019). Remodeling of the IP3R1–VDAC1–MCU axis under metformin treatment also reduced mitochondrial Ca2+ content and enhanced the phosphorylation of the Ser232 site of the PDH-E1α subunit, which was not sufficient to reduce PDH activity in mdx cardiomyocytes. All three phosphorylation sites can restrain enzyme activity; however, Ser293 phosphorylation has a higher inhibitory impact than Ser300 and even more than Ser232 phosphorylation (Korotchkina and Patel, 1995; Gray et al., 2014). Finally, metformin can also impact ROS production in a different way, first of all as a modulator of complex I activity and then by its effect on mitochondrial Ca2+ content. AMPK activation has also been reported to decrease mitochondrial production of ROS (Foretz et al., 2014).
Myocardial metabolic and mitochondrial impairments as well as mitochondrial and cellular Ca2+ handling have been extensively studied in DMD during the last decade. All the studies agree that these disorders, which precede the onset of structural remodeling and deterioration of myocardial macroscopic function, play a central role in the progression of the pathology to heart failure (for review: Esposito and Carsana, 2019). Here, for the first time, our data suggests that the enhancement of SR/ER–mitochondria contact points and increasing mitochondrial Ca2+ uptake and content would enhance glycolytic flux and complex I respiration. However, the downside of this scheme is that increasing mitochondrial Ca2+ would increase ROS production, sensitize mPTP, further impair metabolic flexibility, and alter ATP production and Ca2+ handling creating an amplification loop with all the ingredients toward contractile dysfunction, arrhythmia, fibrosis, and finally heart failure. Accordingly, long-term metformin treatment has already proved to have some beneficial effects on the development of DCM linked to mutations in the dystrophin–glycoprotein complex (Mantuano et al., 2018; Kanamori et al., 2019). Although more studies are needed to understand and characterize the beneficial effects of chronic treatment with metformin on the development of the cardiomyopathy associated with DMD, in the present study, metformin already demonstrates beneficial effects on the aberrant SR/ER-mitochondria interaction and increased mitochondrial Ca2+ as well as mitochondrial function. It will therefore be very important in the near future to determine whether treatment with metformin can improve dystrophic cardiomyopathy in the advanced stage of the pathology. To conclude, our data support the concept that metformin alone or in combination with other drugs might be a potential therapeutic strategy to ameliorate the dystrophinopathies (Casteels et al., 2010; Ljubicic and Jasmin, 2015; Hafner et al., 2016, 2019; Mantuano et al., 2018; Vitiello et al., 2019).
The original contributions presented in the study are included in the article/Supplementary Material, further inquiries can be directed to the corresponding author/s.
The animal study was reviewed and approved by Comité d'éthique régional en expérimentation animale languedoc Roussillon–Ministère de l'enseignement supérieur de la recherche et de l'innovation (N° #16473-2018082016141320).
CA and MP performed and analyzed the experiments. ML and JR performed the experiments. AL designed the experiments, discussed the data, and read the manuscript. JF designed the experiments, collected and discussed the data, and wrote the manuscript. All authors contributed to the article and approved the submitted version.
CA was supported by Association Française contre les Myopathies (# AFM UM181704) and the Lefoulon Delalande fondation (#170612), MP was supported by “Fondation pour la recherche médical” (FRM-R180551 FF), and JF was supported by ANR-18-CE17-0003-CALMOS.
The authors declare that the research was conducted in the absence of any commercial or financial relationships that could be construed as a potential conflict of interest.
We thank Annick Bourret and Fabien Dagnac-Lagrange for administrative assistance and Patrice Bideaux for the animal care.
The Supplementary Material for this article can be found online at: https://www.frontiersin.org/articles/10.3389/fcell.2020.609493/full#supplementary-material
Supplementary Figure 1. Respiratory control ratio (RCR) was measured in (A) WT (N = 7), mdx (N = 13) and mdx + met (N = 9) hearts and (B) in WT (N = 6) and mdx (N = 6) in presence of in presence of 0 nM or 400 nM extramitochondrial Ca2+. Data are mean ± SEM, p > 0.05 mdx vs. WT.
Supplementary Figure 2. SERCA2a, PLB expression, and SR Ca2+ load. (A) Full length immunoblots and quantification of SERCA2a, phospholamban (PLB), and Phospho-Phospholamban (Ser16/Thr17) (pPLB-Ser16; pPLB-Thr17) were normalized to GAPDH. Data are mean ± SEM, p > 0.05 mdx (N = 4-6) vs. WT (N = 4-6). (B) Mean values of the amplitude of caffeine-induced SR Ca2+ release, estimating the SR Ca2+ load. *p = 0.0182 WT (N = 3; N = 9) vs. mdx (N = 3; N = 10).
Supplementary Figure 3. IP3R1 immunoprecipitation. Anti-IP3R1 antibody (1:200) was used to immunoprecipitate IP3R1 from heart homogenate. Samples were incubated with an anti-IP3R1 antibody in 0.5 ml of a modified RIPA buffer (10 mM Tris–HCl, pH 7.4; 150 mM NaCl; 1% Triton; 5 mM NaF and protease inhibitor cocktail) for 2 h at 4°C. The immune complex was incubated with protein A/G magnetic beads (Pierce 88802) at 4°C for 2 h, after which the beads were washed out three times with RIPA buffer. Proteins were separated on SDS/PAGE gels and transferred onto nitrocellulose membranes for 1 h at 100 V. The immunoblots were prepared using antibodies against IP3R1 (1:1,000), anti-GRP75 antibody (1:1,000, Santa Cruz) and anti-VDAC (1:300). All immunoblots were developed and quantified using the Odyssey infrared imaging system (LICOR Biosystems) and infrared-labeled secondary antibodies. After immunoprecipitation (IP) of IP3R1 from heart muscle of WT or mdx mice, immunoblots were used to detect IP3R1, Grp75, and VDAC. Data are mean ± SEM, p > 0.05 mdx (N = 3) vs. WT (N = 3).
Supplementary Figure 4. (A) Transcript levels of UPR response genes quantified by 30 reverse transcription quantitative polymerase chain reaction (RT-qPCR) in WT (N = 4) and mdx (N = 4) hearts. The mRNA levels were normalized to the reference gene TBP. Data are means ± SEM, *p = 0.0286 mdx vs. WT. (B) PLA technical negative control obtained in absence of primary antibody.
Supplementary Figure 5. Pyruvate dehydrogenase kinase 4 (PDK4) expression. Full length immunoblots and quantification of PDK4 were normalized to Hsp60. Data are mean ± SEM, p > 0.05 mdx (N = 5) vs. WT (N = 5).
Supplementary Figure 6. Protein expression of mitochondrial respiratory chain complexes. All western blots were performed on isolated mitochondria and quantifications of proteins are normalized to Hsp60. (A) Representative immunoblots and (B) quantification of complex I (CxI), complex 40 II(CxII), complex III (CxIII), complex IV (CxIV), complex V (Cx V). Data are mean ± SEM, mdx (N = 6) vs. WT (N = 6).
Supplementary Figure 7. Phosphorylation level of AMPK and ACC, and proteins expression of SR/ER and mitochondria contacts points. All quantifications of proteins are normalized either to GAPDH or Actin when heart homogenates were used or to Hsp60 when western blot were performed on isolated mitochondria. (A) Representative immunoblots and quantification of IP3R1, GRP75, Sig-1R, and VDAC. Quantification of IP3R1, GRP75, MFN2, and Sig-1R was carried out from N = 6 mdx and mdx +metformin hearts. Data are mean ± SEM. (B) Mean value of pAMPK/AMPK and pACC/ACC ratio. Ratio were established after proteins normalization to Actin from N = 6 WT, mdx and mdx +metformin hearts. Full lengths gels are in supplemental information. WT vs. mdx+ metformin #p = 0.0101 and mdx vs. mdx+metformin *p = 0.0419.
Supplementary Information. All full lengths gel for all protein probed.
Amedro, P., Vincenti, M., De La Villeon, G., Lavastre, K., Barrea, C., Guillaumont, S., et al. (2019). Speckle-tracking echocardiography in children with Duchenne muscular dystrophy: a prospective multicenter controlled cross-sectional study. J. Am. Soc. Echocardiogr. Off. Publ. Am. Soc. Echocardiogr. 32, 412–422. doi: 10.1016/j.echo.2018.10.017
Angebault, C., Fauconnier, J., Patergnani, S., Rieusset, J., Danese, A., Affortit, C. A., et al. (2018). ER-mitochondria cross-talk is regulated by the Ca2+ sensor NCS1 and is impaired in Wolfram syndrome. Sci. Signal. 11:eaaq1380. doi: 10.1126/scisignal.aaq1380
Arias-del-Val, J., Santo-Domingo, J., García-Casas, P., Alvarez-Illera, P., Núñez Galindo, A., Wiederkehr, A., et al. (2019). Regulation of inositol 1,4,5-trisphosphate-induced Ca2+ release from the endoplasmic reticulum by AMP-activated kinase modulators. Cell Calcium 77, 68–76. doi: 10.1016/j.ceca.2018.12.004
Ascah, A., Khairallah, M., Daussin, F., Bourcier-Lucas, C., Godin, R., Allen, B. G., et al. (2011). Stress-induced opening of the permeability transition pore in the dystrophin-deficient heart is attenuated by acute treatment with sildenafil. Am. J. Physiol. Heart Circ. Physiol. 300, H144–H153. doi: 10.1152/ajpheart.00522.2010
Bravo, R., Vicencio, J. M., Parra, V., Troncoso, R., Munoz, J. P., Bui, M., et al. (2011). Increased ER-mitochondrial coupling promotes mitochondrial respiration and bioenergetics during early phases of ER stress. J. Cell Sci. 124, 2143–2152. doi: 10.1242/jcs.080762
Burelle, Y., Khairallah, M., Ascah, A., Allen, B. G., Deschepper, C. F., Petrof, B. J., et al. (2010). Alterations in mitochondrial function as a harbinger of cardiomyopathy: lessons from the dystrophic heart. J. Mol. Cell. Cardiol. 48, 310–321. doi: 10.1016/j.yjmcc.2009.09.004
Casteels, K., Fieuws, S., van Helvoirt, M., Verpoorten, C., Goemans, N., Coudyzer, W., et al. (2010). Metformin therapy to reduce weight gain and visceral adiposity in children and adolescents with neurogenic or myogenic motor deficit. Pediatr. Diabetes 11, 61–69. doi: 10.1111/j.1399-5448.2009.00512.x
De Palma, C., Morisi, F., Cheli, S., Pambianco, S., Cappello, V., Vezzoli, M., et al. (2012). Autophagy as a new therapeutic target in Duchenne muscular dystrophy. Cell Death Dis. 3:e418. doi: 10.1038/cddis.2012.159
Delprat, B., Crouzier, L., Su, T.-P., and Maurice, T. (2020). At the crossing of ER stress and MAMs: a key role of sigma-1 receptor? Adv. Exp. Med. Biol. 1131, 699–718. doi: 10.1007/978-3-030-12457-1_28
Dubinin, M. V., Talanov, E., Yu, Tenkov, K. S., Starinets, V. S., Mikheeva, I. B., et al. (2020). Transport of Ca2+ and Ca2+-dependent permeability transition in heart mitochondria in the early stages of Duchenne muscular dystrophy. Biochim. Biophys. Acta Bioenerg. 1861:148250. doi: 10.1016/j.bbabio.2020.148250
Esposito, G., and Carsana, A. (2019). Metabolic alterations in cardiomyocytes of patients with Duchenne and Becker muscular dystrophies. J. Clin. Med. 8:2151. doi: 10.3390/jcm8122151
Farini, A., Gowran, A., Bella, P., Sitzia, C., Scopece, A., Castiglioni, E., et al. (2019). Fibrosis rescue improves cardiac function in dystrophin-deficient mice and Duchenne patient–specific cardiomyocytes by immunoproteasome modulation. Am. J. Pathol. 189, 339–353. doi: 10.1016/j.ajpath.2018.10.010
Fauconnier, J., Andersson, D. C., Zhang, S.-J., Lanner, J. T., Wibom, R., Katz, A., et al. (2007). Effects of palmitate on Ca(2+) handling in adult control and ob/ob cardiomyocytes: impact of mitochondrial reactive oxygen species. Diabetes 56, 1136–1142. doi: 10.2337/db06-0739
Fauconnier, J., Lanner, J. T., Zhang, S.-J., Tavi, P., Bruton, J. D., Katz, A., et al. (2005). Insulin and inositol 1,4,5-trisphosphate trigger abnormal cytosolic Ca2+ transients and reveal mitochondrial Ca2+ handling defects in cardiomyocytes of ob/ob mice. Diabetes 54, 2375–2381. doi: 10.2337/diabetes.54.8.2375
Fauconnier, J., Thireau, J., Reiken, S., Cassan, C., Richard, S., Matecki, S., et al. (2010). Leaky RyR2 trigger ventricular arrhythmias in Duchenne muscular dystrophy. Proc. Natl. Acad. Sci. U.S.A. 107, 1559–1564. doi: 10.1073/pnas.0908540107
Foretz, M., Guigas, B., Bertrand, L., Pollak, M., and Viollet, B. (2014). Metformin: from mechanisms of action to therapies. Cell Metab. 20, 953–966. doi: 10.1016/j.cmet.2014.09.018
Frezza, C., Cipolat, S., and Scorrano, L. (2007). Organelle isolation: functional mitochondria from mouse liver, muscle, and cultured fibroblasts. Nat. Protoc. 2, 287–295. doi: 10.1038/nprot.2006.478
Garbincius, J. F., and Michele, D. E. (2015). Dystrophin-glycoprotein complex regulates muscle nitric oxide production through mechanoregulation of AMPK signaling. Proc. Natl. Acad. Sci. U.S.A. 112, 13663–13668. doi: 10.1073/pnas.1512991112
Gray, L. R., Tompkins, S. C., and Taylor, E. B. (2014). Regulation of pyruvate metabolism and human disease. Cell. Mol. Life Sci. 71, 2577–2604. doi: 10.1007/s00018-013-1539-2
Hafner, P., Bonati, U., Erne, B., Schmid, M., Rubino, D., Pohlman, U., et al. (2016). Improved muscle function in Duchenne muscular dystrophy through L-arginine and metformin: an investigator-initiated, open-label, single-center, proof-of-concept-study. PLoS ONE 11:e0147634. doi: 10.1371/journal.pone.0147634
Hafner, P., Bonati, U., Klein, A., Rubino, D., Gocheva, V., Schmidt, S., et al. (2019). Effect of combination l-citrulline and metformin treatment on motor function in patients with Duchenne muscular dystrophy: a randomized clinical trial. JAMA Netw. Open 2:e1914171. doi: 10.1001/jamanetworkopen.2019.14171
Hayashi, T., and Su, T.-P. (2007). Sigma-1 receptor chaperones at the ER- mitochondrion interface regulate Ca2+ signaling and cell survival. Cell 131, 596–610. doi: 10.1016/j.cell.2007.08.036
He, L., and Wondisford, F. E. (2015). Metformin action: concentrations matter. Cell Metab. 21, 159–162. doi: 10.1016/j.cmet.2015.01.003
Hirst, J., and Roessler, M. M. (2016). Energy conversion, redox catalysis, and generation of reactive oxygen species by respiratory complex I. Biochim. Biophys. Acta Bioenerg. 1857, 872–883. doi: 10.1016/j.bbabio.2015.12.009
Hoffman, E. P. (2020). The discovery of dystrophin, the protein product of the Duchenne muscular dystrophy gene. FEBS J. 287, 3879–3887. doi: 10.1111/febs.15466
Horman, S., Beauloye, C., Vanoverschelde, J.-L., and Bertrand, L. (2012). AMP-activated protein kinase in the control of cardiac metabolism and remodeling. Curr. Heart Fail. Rep. 9, 164–173. doi: 10.1007/s11897-012-0102-z
Hughes, M. C., Ramos, S. V., Turnbull, P. C., Edgett, B. A., Huber, J. S., Polidovitch, N., et al. (2020). Impairments in left ventricular mitochondrial bioenergetics precede overt cardiac dysfunction and remodelling in Duchenne muscular dystrophy. J. Physiol. 598, 1377–1392. doi: 10.1113/JP277306
Juban, G., Saclier, M., Yacoub-Youssef, H., Kernou, A., Arnold, L., Boisson, C., et al. (2018). AMPK activation regulates LTBP4-dependent TGF-β1 secretion by pro-inflammatory macrophages and controls fibrosis in Duchenne muscular dystrophy. Cell Rep. 25, 2163–2176. doi: 10.1016/j.celrep.2018.10.077
Jung, C., Martins, A. S., Niggli, E., and Shirokova, N. (2008). Dystrophic cardiomyopathy: amplification of cellular damage by Ca2+ signalling and reactive oxygen species-generating pathways. Cardiovasc. Res. 77, 766–773. doi: 10.1093/cvr/cvm089
Kanamori, H., Naruse, G., Yoshida, A., Minatoguchi, S., Watanabe, T., Kawaguchi, T., et al. (2019). Metformin enhances autophagy and provides cardioprotection in δ-sarcoglycan deficiency-induced dilated cardiomyopathy. Circ. Heart Fail. 12:e005418. doi: 10.1161/CIRCHEARTFAILURE.118.005418
Khairallah, M., Khairallah, R., Young, M. E., Dyck, J. R. B., Petrof, B. J., and Des Rosiers, C. (2007). Metabolic and signaling alterations in dystrophin-deficient hearts precede overt cardiomyopathy. J. Mol. Cell. Cardiol. 43, 119–129. doi: 10.1016/j.yjmcc.2007.05.015
Khairallah, M., Khairallah, R. J., Young, M. E., Allen, B. G., Gillis, M. A., Danialou, G., et al. (2008). Sildenafil and cardiomyocyte-specific cGMP signaling prevent cardiomyopathic changes associated with dystrophin deficiency. Proc. Natl. Acad. Sci. U.S.A. 105, 7028–7033. doi: 10.1073/pnas.0710595105
Kiviluoto, S., Vervliet, T., Ivanova, H., Decuypere, J.-P., De Smedt, H., Missiaen, L., et al. (2013). Regulation of inositol 1,4,5-trisphosphate receptors during endoplasmic reticulum stress. Biochim. Biophys. Acta 1833, 1612–1624. doi: 10.1016/j.bbamcr.2013.01.026
Kockskämper, J., Zima, A. V., Roderick, H. L., Pieske, B., Blatter, L. A., and Bootman, M. D. (2008). Emerging roles of inositol 1,4,5-trisphosphate signaling in cardiac myocytes. J. Mol. Cell. Cardiol. 45, 128–147. doi: 10.1016/j.yjmcc.2008.05.014
Kon, N., Murakoshi, M., Isobe, A., Kagechika, K., Miyoshi, N., and Nagayama, T. (2017). DS16570511 is a small-molecule inhibitor of the mitochondrial calcium uniporter. Cell Death Discov. 3:17045. doi: 10.1038/cddiscovery.2017.45
Korotchkina, L. G., and Patel, M. S. (1995). Mutagenesis studies of the phosphorylation sites of recombinant human pyruvate dehydrogenase. Site-specific regulation. J. Biol. Chem. 270, 14297–14304. doi: 10.1074/jbc.270.24.14297
Kuno, A., Hosoda, R., Sebori, R., Hayashi, T., Sakuragi, H., Tanabe, M., et al. (2018). Resveratrol ameliorates mitophagy disturbance and improves cardiac pathophysiology of dystrophin-deficient mdx mice. Sci. Rep. 8:15555. doi: 10.1038/s41598-018-33930-w
Kuznetsov, A. V., Winkler, K., Wiedemann, F. R., von Bossanyi, P., Dietzmann, K., and Kunz, W. S. (1998). Impaired mitochondrial oxidative phosphorylation in skeletal muscle of the dystrophin-deficient mdx mouse. Mol. Cell. Biochem. 183, 87–96. doi: 10.1023/A:1006868130002
Kyrychenko, V., Poláková, E., Janíček, R., and Shirokova, N. (2015). Mitochondrial dysfunctions during progression of dystrophic cardiomyopathy. Cell Calcium 58, 186–195. doi: 10.1016/j.ceca.2015.04.006
Lee, S., and Min, K.-T. (2018). The interface between ER and mitochondria: molecular compositions and functions. Mol. Cells 41, 1000–1007. doi: 10.14348/molcells.2018.0438
Ljubicic, V., and Jasmin, B. J. (2015). Metformin increases peroxisome proliferator-activated receptor γ Co-activator-1α and utrophin a expression in dystrophic skeletal muscle. Muscle Nerve 52, 139–142. doi: 10.1002/mus.24692
Ljubicic, V., Khogali, S., Renaud, J.-M., and Jasmin, B. J. (2012). Chronic AMPK stimulation attenuates adaptive signaling in dystrophic skeletal muscle. Am. J. Physiol. Cell Physiol. 302, C110–C121. doi: 10.1152/ajpcell.00183.2011
Ljubicic, V., Miura, P., Burt, M., Boudreault, L., Khogali, S., Lunde, J. A., et al. (2011). Chronic AMPK activation evokes the slow, oxidative myogenic program, and triggers beneficial adaptations in mdx mouse skeletal muscle. Hum. Mol. Genet. 20, 3478–3493. doi: 10.1093/hmg/ddr265
Mantuano, P., Sanarica, F., Conte, E., Morgese, M. G., Capogrosso, R. F., Cozzoli, A., et al. (2018). Effect of a long-term treatment with metformin in dystrophic mdx mice: a reconsideration of its potential clinical interest in Duchenne muscular dystrophy. Biochem. Pharmacol. 154, 89–103. doi: 10.1016/j.bcp.2018.04.022
Márta, K., Hasan, P., Rodríguez-Prados, M., Paillard, M., and Hajnóczky, G. (2020). Pharmacological inhibition of the mitochondrial Ca2+ uniporter: relevance for pathophysiology and human therapy. J. Mol. Cell. Cardiol. doi: 10.1016/j.yjmcc.2020.09.014. [Epub ahead of print].
Momose, M., Iguchi, N., Imamura, K., Usui, H., Ueda, T., Miyamoto, K., et al. (2001). Depressed myocardial fatty acid metabolism in patients with muscular dystrophy. Neuromuscul. Disord. 11, 464–469. doi: 10.1016/S0960-8966(01)00191-2
Moore, T. M., Lin, A. J., Strumwasser, A. R., Cory, K., Whitney, K., Ho, T., et al. (2020). Mitochondrial dysfunction is an early consequence of partial or complete dystrophin loss in mdx mice. Front. Physiol. 11:690. doi: 10.3389/fphys.2020.00690
Naruse, H., Miyagi, J., Arii, T., Ohyanagi, M., Iwasaki, T., and Jinnai, K. (2004). The relationship between clinical stage, prognosis, and myocardial damage in patients with Duchenne-type muscular dystrophy: five-year follow-up study. Ann. Nucl. Med. 18, 203–208. doi: 10.1007/BF02985001
Nemani, N., Dong, Z., Daw, C. C., Madaris, T. R., Ramachandran, K., Enslow, B. T., et al. (2020). Mitochondrial pyruvate and fatty acid flux modulate MICU1-dependent control of MCU activity. Sci. Signal. 13:eaaz6206. doi: 10.1126/scisignal.aaz6206
Paillard, M., Tubbs, E., Thiebaut, P.-A., Gomez, L., Fauconnier, J., Da Silva, C. C., et al. (2013). Depressing mitochondria-reticulum interactions protects cardiomyocytes from lethal hypoxia-reoxygenation injury. Circulation 128, 1555–1565. doi: 10.1161/CIRCULATIONAHA.113.001225
Pauly, M., Angebault-Prouteau, C., Dridi, H., Notarnicola, C., Scheuermann, V., Lacampagne, A., et al. (2017). ER stress disturbs SR/ER-mitochondria Ca2+ transfer: implications in Duchenne muscular dystrophy. Biochim. Biophys. Acta Mol. Basis Dis. 1863, 2229–2239. doi: 10.1016/j.bbadis.2017.06.009
Pauly, M., Daussin, F., Burelle, Y., Li, T., Godin, R., Fauconnier, J., et al. (2012). AMPK activation stimulates autophagy and ameliorates muscular dystrophy in the mdx mouse diaphragm. Am. J. Pathol. 181, 583–592. doi: 10.1016/j.ajpath.2012.04.004
Payne, R., Hoff, H., Roskowski, A., and Foskett, J. K. (2017). MICU2 restricts spatial crosstalk between InsP 3 R and MCU channels by regulating threshold and gain of micu1-mediated inhibition and activation of MCU. Cell Rep. 21, 3141–3154. doi: 10.1016/j.celrep.2017.11.064
Percival, J. M., Siegel, M. P., Knowels, G., and Marcinek, D. J. (2013). Defects in mitochondrial localization and ATP synthesis in the mdx mouse model of Duchenne muscular dystrophy are not alleviated by PDE5 inhibition. Hum. Mol. Genet. 22, 153–167. doi: 10.1093/hmg/dds415
Perloff, J. K., Henze, E., and Schelbert, H. R. (1984). Alterations in regional myocardial metabolism, perfusion, and wall motion in Duchenne muscular dystrophy studied by radionuclide imaging. Circulation 69, 33–42. doi: 10.1161/01.CIR.69.1.33
Prosser, B. L., Ward, C. W., and Lederer, W. J. (2011). X-ROS signaling: rapid mechano-chemo transduction in heart. Science 333, 1440–1445. doi: 10.1126/science.1202768
Quinlan, J. G., Hahn, H. S., Wong, B. L., Lorenz, J. N., Wenisch, A. S., and Levin, L. S. (2004). Evolution of the mdx mouse cardiomyopathy: physiological and morphological findings. Neuromuscul. Disord. 14, 491–496. doi: 10.1016/j.nmd.2004.04.007
Quinlivan, R. M., Lewis, P., Marsden, P., Dundas, R., Robb, S. A., Baker, E., et al. (1996). Cardiac function, metabolism, and perfusion in Duchenne and Becker muscular dystrophy. Neuromuscul. Disord. 6, 237–246. doi: 10.1016/0960-8966(96)00007-7
Reagan-Shaw, S., Nihal, M., and Ahmad, N. (2008). Dose translation from animal to human studies revisited. FASEB J. 22, 659–661. doi: 10.1096/fj.07-9574LSF
Rieusset, J., Fauconnier, J., Paillard, M., Belaidi, E., Tubbs, E., Chauvin, M.-A., et al. (2016). Disruption of calcium transfer from ER to mitochondria links alterations of mitochondria-associated ER membrane integrity to hepatic insulin resistance. Diabetologia 59, 614–623. doi: 10.1007/s00125-015-3829-8
Rossini, M., and Filadi, R. (2020). Sarcoplasmic reticulum-mitochondria kissing in cardiomyocytes: Ca2+, ATP, and undisclosed secrets. Front. Cell Dev. Biol. 8:532. doi: 10.3389/fcell.2020.00532
Rybalka, E., Timpani, C. A., Cooke, M. B., Williams, A. D., and Hayes, A. (2014). Defects in mitochondrial ATP synthesis in dystrophin-deficient mdx skeletal muscles may be caused by complex I insufficiency. PLoS ONE 9:e115763. doi: 10.1371/journal.pone.0115763
Saddik, M., Gamble, J., Witters, L. A., and Lopaschuk, G. D. (1993). Acetyl-CoA carboxylase regulation of fatty acid oxidation in the heart. J. Biol. Chem. 268, 25836–25845.
Sasaki, K., Sakata, K., Kachi, E., Hirata, S., Ishihara, T., and Ishikawa, K. (1998). Sequential changes in cardiac structure and function in patients with Duchenne type muscular dystrophy: a two-dimensional echocardiographic study. Am. Heart J. 135, 937–944. doi: 10.1016/S0002-8703(98)70057-2
Scarpulla, R. C. (2011). Metabolic control of mitochondrial biogenesis through the PGC-1 family regulatory network. Biochim. Biophys. Acta 1813, 1269–1278. doi: 10.1016/j.bbamcr.2010.09.019
Segawa, K., Sugawara, N., Maruo, K., Kimura, K., Komaki, H., Takahashi, Y., et al. (2020). Left ventricular end-diastolic diameter and cardiac mortality in Duchenne muscular dystrophy. Neuropsychiatr. Dis. Treat. 16, 171–178. doi: 10.2147/NDT.S235166
Sperl, W., Skladal, D., Gnaiger, E., Wyss, M., Mayr, U., Hager, J., et al. (1997). High resolution respirometry of permeabilized skeletal muscle fibers in the diagnosis of neuromuscular disorders. Mol. Cell. Biochem. 174, 71–78. doi: 10.1023/A:1006880529195
Sun, W., Liu, Q., Leng, J., Zheng, Y., and Li, J. (2015). The role of Pyruvate Dehydrogenase Complex in cardiovascular diseases. Life Sci. 121, 97–103. doi: 10.1016/j.lfs.2014.11.030
Suntar, I., Sureda, A., Belwal, T., Sanches Silva, A., Vacca, R. A., Tewari, D., et al. (2020). Natural products, PGC-1α, and Duchenne muscular dystrophy. Acta Pharm. Sin. B 10, 734–745. doi: 10.1016/j.apsb.2020.01.001
Tarasova, N. V., Vishnyakova, P. A., Logashina, Y. A., and Elchaninov, A. V. (2019). Mitochondrial calcium uniporter structure and function in different types of muscle tissues in health and disease. Int. J. Mol. Sci. 20:4823. doi: 10.3390/ijms20194823
Ullrich, N. D., Fanchaouy, M., Gusev, K., Shirokova, N., and Niggli, E. (2009). Hypersensitivity of excitation-contraction coupling in dystrophic cardiomyocytes. Am. J. Physiol. Heart Circ. Physiol. 297, H1992–H2003. doi: 10.1152/ajpheart.00602.2009
Vial, G., Detaille, D., and Guigas, B. (2019). Role of mitochondria in the mechanism(s) of action of metformin. Front. Endocrinol. 10:294. doi: 10.3389/fendo.2019.00294
Viola, H. M., Davies, S. M. K., Filipovska, A., and Hool, L. C. (2013). L-type Ca(2+) channel contributes to alterations in mitochondrial calcium handling in the mdx ventricular myocyte. Am. J. Physiol. Heart Circ. Physiol. 304, H767–H775. doi: 10.1152/ajpheart.00700.2012
Vitiello, L., Tibaudo, L., Pegoraro, E., Bello, L., and Canton, M. (2019). Teaching an old molecule new tricks: drug repositioning for Duchenne muscular dystrophy. Int. J. Mol. Sci. 20:6053. doi: 10.3390/ijms20236053
Wang, Y., An, H., Liu, T., Qin, C., Sesaki, H., Guo, S., et al. (2019). Metformin improves mitochondrial respiratory activity through activation of AMPK. Cell Rep. 29, 1511–1523. doi: 10.1016/j.celrep.2019.09.070
Williams, I. A., and Allen, D. G. (2007a). Intracellular calcium handling in ventricular myocytes from mdx mice. Am. J. Physiol. Heart Circ. Physiol. 292, H846–H855. doi: 10.1152/ajpheart.00688.2006
Williams, I. A., and Allen, D. G. (2007b). The role of reactive oxygen species in the hearts of dystrophin-deficient mdx mice. Am. J. Physiol. Heart Circ. Physiol. 293, H1969–H1977. doi: 10.1152/ajpheart.00489.2007
Wu, S., Lu, Q., Ding, Y., Wu, Y., Qiu, Y., Wang, P., et al. (2019). Hyperglycemia-driven inhibition of AMP-activated protein kinase α2 induces diabetic cardiomyopathy by promoting mitochondria-associated endoplasmic reticulum membranes in vivo. Circulation 139, 1913–1936. doi: 10.1161/CIRCULATIONAHA.118.033552
Keywords: Duchenne muscular dystrophy cardiomyopathy, mitochondria-associated ER membrane, mitochondrial calcium uniporter, MICU1, calcium
Citation: Angebault C, Panel M, Lacôte M, Rieusset J, Lacampagne A and Fauconnier J (2021) Metformin Reverses the Enhanced Myocardial SR/ER–Mitochondria Interaction and Impaired Complex I-Driven Respiration in Dystrophin-Deficient Mice. Front. Cell Dev. Biol. 8:609493. doi: 10.3389/fcell.2020.609493
Received: 23 September 2020; Accepted: 08 December 2020;
Published: 25 January 2021.
Edited by:
Gaetano Santulli, Columbia University, United StatesReviewed by:
Clementina Sitzia, University of Milan, ItalyCopyright © 2021 Angebault, Panel, Lacôte, Rieusset, Lacampagne and Fauconnier. This is an open-access article distributed under the terms of the Creative Commons Attribution License (CC BY). The use, distribution or reproduction in other forums is permitted, provided the original author(s) and the copyright owner(s) are credited and that the original publication in this journal is cited, in accordance with accepted academic practice. No use, distribution or reproduction is permitted which does not comply with these terms.
*Correspondence: Jérémy Fauconnier, amVyZW15LmZhdWNvbm5pZXJAaW5zZXJtLmZy
Disclaimer: All claims expressed in this article are solely those of the authors and do not necessarily represent those of their affiliated organizations, or those of the publisher, the editors and the reviewers. Any product that may be evaluated in this article or claim that may be made by its manufacturer is not guaranteed or endorsed by the publisher.
Research integrity at Frontiers
Learn more about the work of our research integrity team to safeguard the quality of each article we publish.