- 1Department of Molecular Oncology, British Columbia Cancer Research Centre, Vancouver, BC, Canada
- 2Department Drug Discovery and Development, Harrison School of Pharmacy, Auburn University, Auburn, AL, United States
- 3National Laboratory Astana, Nazarbayev University, Nur-Sultan, Kazakhstan
- 4Groupe “Mechanisms of DNA Repair and Carcinogenesis”, Equipe Labellisée LIGUE 2016, CNRS UMR 9019, Université Paris-Saclay, Villejuif, France
The nucleosome is a stretch of DNA wrapped around a histone octamer. Electrostatic interactions and hydrogen bonds between histones and DNA are vital for the stable organization of nucleosome core particles, and for the folding of chromatin into more compact structures, which regulate gene expression via controlled access to DNA. As a drawback of tight association, under genotoxic stress, DNA can accidentally cross-link to histone in a covalent manner, generating a highly toxic DNA-histone cross-link (DHC). DHC is a bulky lesion that can impede DNA transcription, replication, and repair, often with lethal consequences. The chemotherapeutic agent cisplatin, as well as ionizing and ultraviolet irradiations and endogenously occurring reactive aldehydes, generate DHCs by forming either stable or transient covalent bonds between DNA and side-chain amino groups of histone lysine residues. The mechanisms of DHC repair start to unravel, and certain common principles of DNA-protein cross-link (DPC) repair mechanisms that participate in the removal of cross-linked histones from DNA have been described. In general, DPC is removed via a two-step repair mechanism. First, cross-linked proteins are degraded by specific DPC proteases or by the proteasome, relieving steric hindrance. Second, the remaining DNA-peptide cross-links are eliminated in various DNA repair pathways. Delineating the molecular mechanisms of DHC repair would help target specific DNA repair proteins for therapeutic intervention to combat tumor resistance to chemotherapy and radiotherapy.
Introduction
Cellular DNA is constantly altered by endogenous and exogenous factors, resulting in tens of thousands of lesions in a human cell every day (Lindahl, 1993). This damage may be classified into two types according to size: non-bulky DNA and bulky DNA. Non-bulky DNA lesions include base mismatches, abasic sites, and small base modifications, which in general are repaired by mismatch repair (MMR), base excision repair (BER), nucleotide incision repair (NIR), direct reversal repair (DRR), and translesion DNA synthesis (TLS) (Gros et al., 2004; Fortini and Dogliotti, 2007; Sharma et al., 2013; Yi and He, 2013; Ignatov et al., 2017). Bulky DNA lesions include, among other types of damage: double-strand breaks, DNA-protein cross-links (DPCs), and intra- and inter-strand DNA cross-links. The structural complexity of certain bulky DNA lesions requires the use of several DNA repair pathways acting in a coordinated manner, including homologous recombination (HR), non-homologous DNA end-joining (NHEJ), nucleotide excision repair (NER), TLS and BER; Fanconi anemia (FA) signaling system and complex proteolytic machinery (Ishchenko et al., 2006; Ho and Schärer, 2010; Duxin et al., 2014; Tretyakova et al., 2015; Martin et al., 2017). Non-bulky DNA lesions cause limited and local DNA perturbations, whereas bulky ones induce significant distortions in the overall DNA helix structure (Ide et al., 2011). DNA-protein cross-links (DPCs) are formed when a protein covalently binds to DNA (Tretyakova et al., 2015). They are difficult to repair because of their super-bulky character compared with known voluminous, helix-distorting DNA lesions, such as UV-induced pyrimidine dimers. These super-bulky adducts can be generated by exposure of cells to endogenous and exogenous cross-linking agents (Stingele et al., 2017; Zhang et al., 2020). The presence of protein covalently attached to DNA strongly interferes with DNA replication, transcription, repair, and chromatin remodeling (Kuo et al., 2007; Klages-Mundt and Li, 2017; Yudkina et al., 2018; Ji et al., 2019). DPCs may be classified into five types, according to the nature of the covalent link in the DNA-protein complex and the presence of DNA strand breaks (Ide et al., 2015, 2018; Nakano et al., 2017). Type 1, the most common type of DPC, is formed when proteins covalently link to a nitrogenous base in undisrupted DNA. Type 2-4 cross-links occur when DNA-cleaving enzymes are trapped in a covalent intermediate with a DNA strand (Ide et al., 2015, 2018; Nakano et al., 2017). Type 2 is formed when bi-functional DNA glycosylases and repair enzymes containing β-lyase activity such as DNA polymerase β and Parp1 irreversibly bind to a cleaved apurinic/apyrimidinic (AP) site (Ide et al., 2015, 2018; Nakano et al., 2017). Type 3 is generated during abortive DNA strand cleavage by topoisomerase 1 (Top1) and formation of a covalent tyrosinyl–phosphodiester bond between the protein and the 3′-terminal DNA phosphate moiety of SSB (Ide et al., 2015, 2018; Nakano et al., 2017). The abortive action of topoisomerase 2 (Top2) generates type 4 DPC, in which tyrosine is linked to the 5′-terminal phosphates of double-strand breaks (DSB) (Ide et al., 2015, 2018; Nakano et al., 2017). Recently, a new type of DPC emerged after the discovery of HMCES, a 5-hydroxymethylcytosine (5hmC) binding protein which can recognize abasic sites in single stranded DNA (ssDNA) and form a covalent ssDNA-HMCES crosslink to prevent error-prone translesion synthesis past the lesion (Mohni et al., 2019). Because of the differences in structure and composition between these five groups, each type of DPC is processed by a distinct repair mechanism. It seems difficult to remove super-bulky Type 1 DPC in the canonical linear DNA excision repair pathways because the presence of a protein molecule blocks access to DNA. Nevertheless, recent studies have revealed that nucleotide excision repair (NER) and homologous recombination (HR) can remove certain types of DPCs in a nuclease-dependent manner (Zhang et al., 2020). However, it is still not clear whether these repair pathways could deal with other types of DPC. Stingele et al. (2017) have proposed that each constituent of DPC: DNA, protein, and the covalent linkage between them might be processed by three different repair mechanisms. A recent paper by Kühbacher and Duxin (2020) provides comprehensive review on the formation and repair of DPCs. In this review, we summarize the current knowledge regarding the repair mechanisms involved in removal of DHCs induced by various genotoxic agents. Covalent cross-linking to DNA occurs more often with DNA binding proteins, such as histones, transcription factors, and DNA metabolizing enzymes including repair factors and topoisomerases (Klages-Mundt and Li, 2017). In the cell nucleus, histones are assembled into an octamer forming the nucleosome core with 147 bp of DNA wrapped around and tightly bound to it (Luger et al., 1997, 2012). This basic chromatin structure makes histones primary targets of DNA cross-linking agents, leading to the formation of DNA-histone cross-links (DHC) (Solomon and Varshavsky, 1985). Currently, the repair mechanisms counteracting DHCs generated by various factors only started to unravel.
DNA-Histone Cross-Links (DHCs)
Nucleosomal DNA is packaged into compact units referred as chromosomes, in which core nucleosome particles are connected by stretches of linker DNA up to 80 bp length. A nucleosome core particle (NCP) is composed of two copies each of histones H2A, H2B, H3, and H4. The molecular weight of individual histones range from 11 to 22 KDa, whereas the molecular weight of histone octamer in NCP is 210 KDa (Eickbush and Moudrianakis, 1978; Luger et al., 1997). The stability of the nucleosome is based on various protein-protein interactions, and numerous non-covalent electrostatic and hydrogen bonds between histones and the DNA duplex (Luger et al., 1997, 2012; Davey et al., 2002; Rohs et al., 2009). The primary structure of chromatin can be depicted as a beads-on-a-string organization of individual nucleosomes, which can be further folded into compact secondary and tertiary structures, with the help of histone variants present in certain nucleosomes and post-translational modifications (PTMs) situated in disordered histone tails (Woodcock and Dimitrov, 2001; Luger et al., 2012). The folding of chromatin into primary, secondary, and tertiary structures is crucial for regulating the accessibility of DNA to complex multi-protein machinery involved in DNA replication, transcription, and repair. Non-covalent interactions between DNA and histones enable chromatin dynamics to switch between the closed and open conformations. DHCs impair chromatin flexibility, which may subsequently affect long-distance interactions in chromatin that would indirectly disturb DNA replication, transcription, and repair within a topologically associating domain (TAD) (Hinz et al., 2010; Todd and Lippard, 2010; Tretyakova et al., 2015; Hauer and Gasser, 2017; Nakano et al., 2017). DHCs belong to type 1, a non-enzymatic form of DPC, in which a protein is covalently attached to an undisrupted DNA (Ide et al., 2011). Several comprehensive studies describing the mechanisms of formation of DHCs have been published recently (Ming et al., 2017; Shang et al., 2019; Yang and Greenberg, 2019), nevertheless, it is not known whether specific repair mechanisms for the removal of DHCs exist. In this review, we focus mainly on the repair pathways of DHCs and briefly describe their formation.
Formation of DHCs
A water-soluble covalent complex of DNA and histones (H2A and H2B) was first identified in a UV cross-linking assay (Smith, 1966; Sperling and Sperling, 1978). With this finding, it became evident that UV irradiation can induce DHCs in addition to well-known pyrimidine dimers. It was then discovered that exogenous and endogenous aldehydes could also form DHCs in cells (Lam et al., 1985; Kuykendall and Bogdanffy, 1992). More than 10% of amino acid residues in histones are lysines, whereas, aldehydes preferentially react with ε-amino groups of lysine side-chains with the formation of a Schiff base, which further reacts with exocyclic amino groups of guanine, adenine, and cytosine DNA bases, creating methylene linkage. Many cross-linking agents, such as chromate, metal ions, and cisplatin (cis-diaminedichloroplatinum-II), also induce DHCs in cells (Zhitkovich and Costa, 1992). Platinum compounds not only cause DNA-DNA cross-links but also covalently link DNA-protein complexes. In the case of histones (Figure 1A), these compounds cross-link ε-amino-groups of lysines and N7 atoms of guanosines (Tretyakova et al., 2015; Ming et al., 2017). Cross-links between DNA and methionine residues were also observed in an X-ray structure of nucleosomes treated with platinum compounds (Wu et al., 2008). Exposure of purified nucleosome to bi-functional alkylating agents (e.g., nitrogen mustards) also cross-links histones to guanosines in DNA (Shang et al., 2019); however, these types of cross-links in cells are much less abundant than DNA cross-links with cysteines and histidines of non-histone proteins (Loeber et al., 2009).
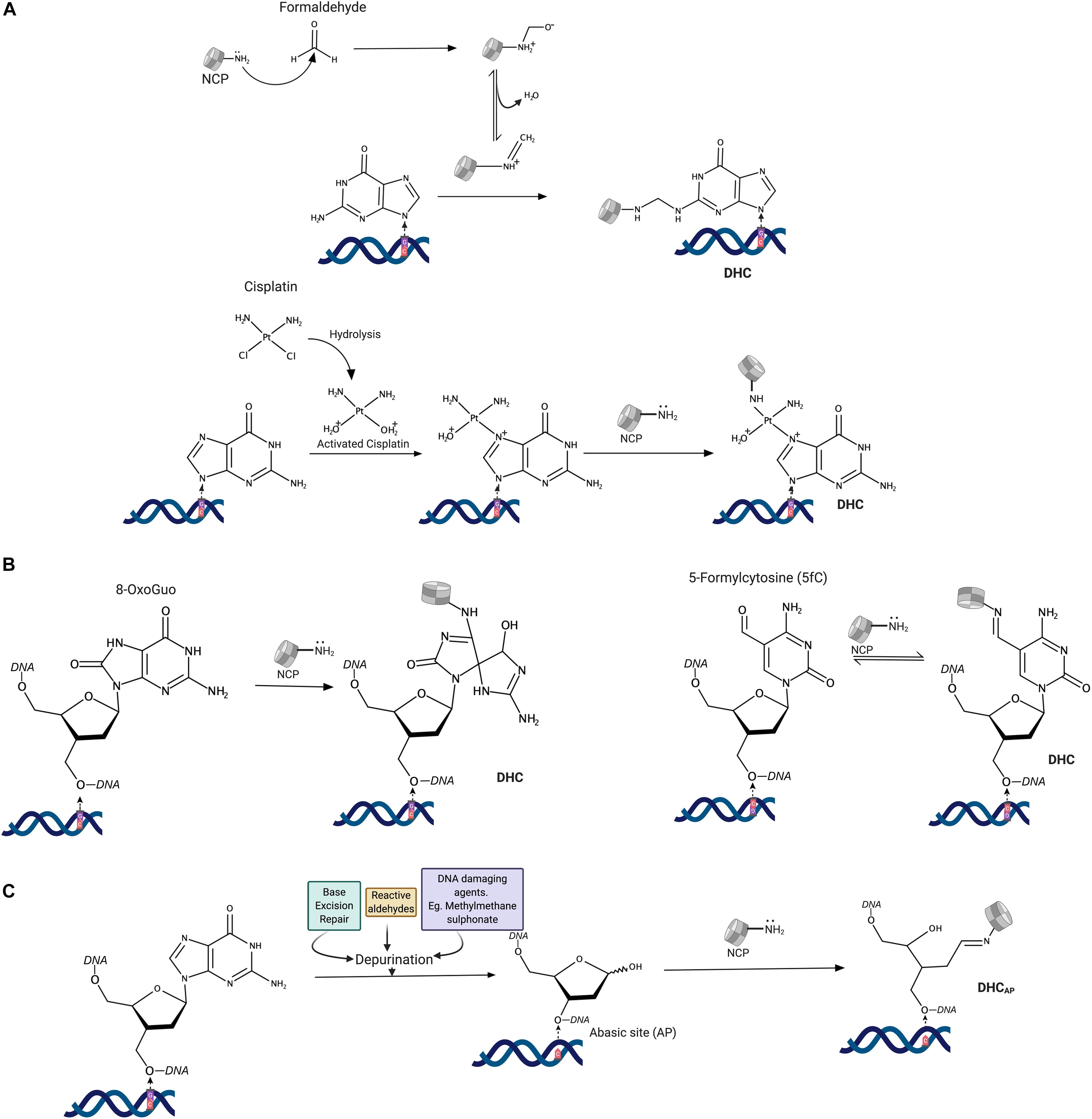
Figure 1. Mechanisms of histone-DNA cross-links formation. (A) Reaction mechanisms of DNA cross-linking agents. (B) Direct cross-linking of histones to modified DNA bases. (C) Abasic site mediated cross-linking of histones to DNA. NCP-NH2: N-terminal amine (Lysine) of histones in the nucleosome core.
Histones can also directly react with 5-formylcytosine, a naturally occurring modified DNA base, and 8-oxoguanine, a major oxidative DNA damage product. Lysine amino groups react with 5-formylcytosine (Figure 1B), with the formation of a reversible Schiff base (Li et al., 2017; Raiber et al., 2018). The reaction of lysine side-chains with 8-oxoguanosine produced a stable protein cross-linked spiroiminodihydantoin (Sp) adduct (Xu et al., 2008).
Finally, the majority of DHCs are produced by a reaction between histone lysines and an aldehyde form of the 2′-deoxyribose at apurinic/apyrimidinic (AP) sites (Figure 1C) that are either directly formed upon damage or generated during excision of damaged bases in the base excision repair pathway (Solomon and Varshavsky, 1985; Sczepanski et al., 2010). The resulting Schiff base often undergoes strand-breaking ß-elimination, followed by a reversal of a histone-DNA cross-link. Since histone emerges unaltered from the reaction, the whole process is sometimes referred to as histone-catalyzed strand cleavage at AP sites (Ren et al., 2019). It should be noted that histone PTMs and the chromatin state could have a significant impact on DHC formation at abasic sites and with DNA bases (Sczepanski et al., 2010; Bowman and Poirier, 2015).
Mechanisms of Repair of DHC
Although DPCs, especially DHCs, often occur in cells and present a constant threat to genome stability, it is presumed that, except for tyrosyl-DNA phosphodiesterases, there is no specialized DNA repair pathway dedicated to meet these super-bulky challenges. Instead, the cell employs several distinct DNA repair and protein degradation mechanisms to target cross-linked DNA and protein/histone components in a given DPC/DHC. The covalently bound protein could be detected and degraded to a small peptide by cell proteolytic machinery, such as the specialized proteases SPRTN/Wss1, Ddi1, and GCNA1, or by proteasome, an ATP-dependent multi-subunit protease complex, whereas the damaged DNA component is detected and repaired in the NER, BER, HR, NHEJ, and FA pathways.
Proteasome-Dependent Proteolysis of Histones Cross-Linked to DNA
Proteasome-mediated proteolysis is the major pathway for the degradation of damaged proteins in a cell. A 26S proteasome consists of a cylindrical 20S core particle and one or two 19S regulatory particles (Ciechanover, 1998; Lecker et al., 2006). Although 20S core can bind to different regulatory particles, only the 19S particle confers the ability to degrade ubiquitylated proteins (Coux et al., 1996; Adams, 2004; Stadtmueller and Hill, 2011). Considering the vital role of the proteasome in the degradation of damaged protein, proteasome and ubiquitin involvement in the proteolysis of DHCs or DPCs remains a topic of debate. Inhibition of proteasome in Xenopus egg extracts did not stabilize the DPCs (Nakano et al., 2007; Duxin et al., 2014). However, many studies of the repair of DPCs in mammalian cells suggest proteasome participation (Adams, 2004; Baker et al., 2007; Zecevic et al., 2010; Larsen et al., 2019). Proteasome involvement in DHC removal surfaced for the first time in the research of Quievryn and Zhitkovich (2000), who discovered that proteasome inhibitors prevent the removal of DHCs and sensitize human cells to lower levels of formaldehyde. A study in Xenopus egg extracts found that DPCs are ubiquitylated by TRAIP E3 ubiquitin ligase and are subsequently degraded by the proteasome (Duxin et al., 2014; Larsen et al., 2019). However, an earlier study clearly demonstrated that DPCs are not marked with polyubiquitin chains, but are nevertheless subjected to proteasomal degradation by a mechanism that is not well understood (Nakano et al., 2009). The 26S proteasome can degrade purified non-ubiquitylated histones (Kisselev et al., 2006), raising the possibility of proteasomal degradation of non-ubiquitylated damaged histones in cells. A couple of studies have demonstrated that during replication stress induced by genotoxic agents, histones are hyperacetylated, and then specifically degraded in a ubiquitin-independent manner by a complex of 20S proteasome with PA200 proteasome activator, a distinct regulatory particle (Qian et al., 2013; Mandemaker et al., 2018). Although these studies have demonstrated that the ubiquitin-independent degradation of acetylated histones alleviates replication stress, the additional function of PA200-20S proteasome in DHC repair cannot be excluded. Moreover, PA200 was detected in nuclear speckles, and its role in DNA repair has been proposed (Ustrell et al., 2002). Thus, more detailed understanding of the role of proteasome in DHC repair requires further investigation.
The 20S proteasome is a hollow, barrel-shaped particle composed of 28 non-identical subunits arranged into four stacked rings. The active sites are sequestered inside an internal cavity separated from regulatory 19S and PA200 complexes by a gated channel. This 13Å channel is too narrow for a folded protein to enter (Löwe et al., 1995; Groll et al., 1997). For complete degradation of a DNA-cross-linked protein, the cross-linked DNA nucleotide itself would have to enter the proteolytic chamber, pulling a DNA strand inside. However, the DNA component of a DPC might be too bulky to enter the channel. Therefore, proteasome can remove only part of a cross-linked protein, converting DHC into a smaller DNA-peptide cross-link. Alternatively, traditional proteases, in which an active site is located in a cleft on the enzyme surface, could be involved in excision of the bulk of the non-cross-linked polypeptide chain, which can then be degraded by any of these proteases and by the proteasome.
Metalloprotease-Based Proteolysis of Histones Cross-Linked to DNA
Wss1 (weak suppressor of smt3) is a DNA-dependent metalloprotease, whose role in DPC removal was unraveled in a study in which treatment of yeast cells lacking Wss1 and TDP1 with formaldehyde resulted in a synthetic sickness (Stingele et al., 2014). It was also demonstrated that SUMOylation of both enzymatic and non-enzymatic DPCs by DNA-bound SUMO ligases targets them to Wss1 (Psakhye and Jentsch, 2012; Jentsch and Psakhye, 2013; Stingele et al., 2014; Balakirev et al., 2015). Search for an ortholog of Wss1 in higher eukaryotes revealed another metalloprotease, Dvc1/Spartan, of the SprT protease family, whose function was initially thought to be a removal of Polη from chromatin during translesion DNA repair synthesis. (Davis et al., 2012; Mosbech et al., 2012). Spartan/Dvc1 has a similar domain organization and shares a common evolutionary origin with Wss1. Like Wss1, Dvc1/Spartan also repairs DPCs in Xenopus egg extracts (Duxin et al., 2014; Stingele et al., 2014, 2015). A detailed study of DPC removal by Spartan/Dvc1 in Xenopus levis revealed stepwise proteolysis of DPC during DNA replication (Larsen et al., 2019). During replication, on both the leading and lagging strands, CMG helicase bypasses the DPC lesion, followed by the stalling of DNA polymerase (Duxin et al., 2014; Larsen et al., 2019; Sparks et al., 2019). Replicative DNA polymerase stalled at DPC triggers the recruitment of Spartan protease (Figure 2A), which then degrades the DPC protein component (Larsen et al., 2019; Reinking et al., 2020). However, in the case of DHCs, CMG helicase bypass would depend on the complexity of nucleosomal damage. If the crosslink involves just a single histone within NCP, then CMG helicase can bypass the DHC due to small size of protein residue. If multiple histones within NCP are cross-linked, then the large nucleosome-size DHC should stall CMG helicase, triggering the ubiquitination of crosslinked histones by TRAIP E3 ubiquitin ligase and their subsequent degradation by 26S proteasome (Nakano et al., 2013; Larsen et al., 2019; Sparks et al., 2019). In contrast to Wss1, targeting of DPCs by Spartan/Dvc1 does not require SUMOylation of DPC (Duxin et al., 2014; Larsen et al., 2019). Several studies have demonstrated that proteasome and Spartan might work in concert to attain efficient DPC proteolysis. For proteolysis of DPC by proteasome, the active site topology in the 20S core particle makes it arduous for efficient proteolysis, leaving a larger peptide adduct, which could be further degraded by Spartan (Larsen et al., 2019; Sparks et al., 2019). The above observations support a hypothesis proposed by the Walter laboratory (Larsen et al., 2019) that initial proteolysis of DPC by proteasome may help reduce the size of a DPC for the CMG helicase bypass. This stepwise process applies for most type 1 DPCs when histones cross-linked to DNA are initially degraded either by 26S proteasome or PA200-20S proteasome, and in a second step by Spartan (Figure 2A). Validation of this hypothetical order of steps in proteolysis of DHC by the proteasome and Spartan requires more research. However, a recent study proved that Wss1 is actively involved in histone proteolysis in a NCP during replication stress (Maddi et al., 2020). Although this study showed that Wss1 removes histones non-covalently bound to DNA, it is very likely that this protease can also remove histones covalently cross-linked to DNA. Initially, it was thought that Spartan/Dvc1 could only execute DPC proteolysis in a replication-dependent manner, but later it was demonstrated that Spartan/Dvc1 could act on a single-stranded DNA to degrade DPC independently of replisome (Larsen et al., 2019). A couple of other studies have also demonstrated that in fly embryos Spartan is recruited to chromatin before replication and that its absence greatly sensitized the arrested, non-replicating L1 worm larvae to formaldehyde (Delabaere et al., 2014; Stingele et al., 2016; Reinking et al., 2020). This evidence clearly defines a role for Spartan in the replication-independent repair of DPCs, which may be required for chromatin-based transactions associated with transcription. More data are required to confirm the existence of transcription-coupled DPC repair in cells.
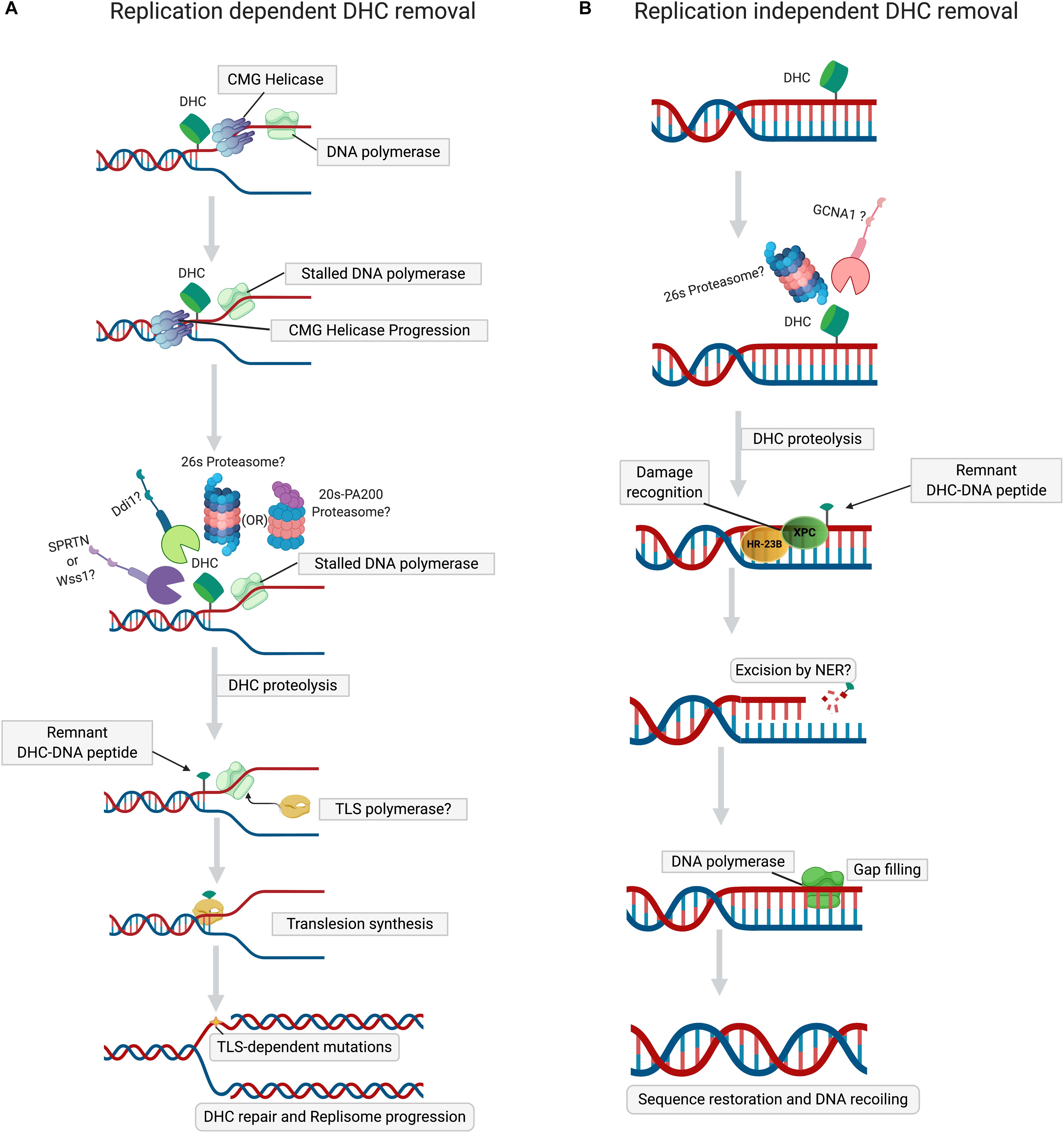
Figure 2. Mechanisms of DHC repair. (A) Replication-dependent DHC removal. Stalling of DNA polymerase at DHC during replication triggers proteolysis of DHC by the proteasome (26S or PA200), followed either by the SPRTN or Ddi1 proteases. The peptide-DNA crosslink, a product of proteolyzed DHC, is bypassed by translesion synthesis (TLS) DNA polymerases, resuming the replisome progression. (B) Replication-independent DHC removal. In non-dividing cells, when histones are cross-linked to DNA, distortion of the DNA helix induces histone PTMs (acetylation or SUMOylation) and chromatin opening, thus permitting the proteolysis of DHC by 26S proteasome or GCNA1 protease. The peptide-DNA crosslink, a remnant of proteolyzed DHC, is then excised by NER, followed by gap-filling by DNA polymerase and ligation, which lead to the restoration of primary DNA sequence and chromatin.
Germ cell nuclear acidic peptidase (GCNA), also known as germ cell nuclear antigen or acidic repeat containing (ACRC), is a metalloprotease that contains an SprT domain, such as Spartan/Dvc1 (Carmell et al., 2016; Dokshin et al., 2020). It has recently been demonstrated in Caenorhabditis elegans that GCNA/ACRC, with its C-terminal Spartan (SprT)-domain, can also be involved in the removal of formaldehyde-induced DPCs in exposed cells (Borgermann et al., 2019). Recently, another study of Drosophila embryos clearly established that the loss of GCNA resulted in the accumulation of DPCs that included histones, confirming the role of GCNA in the removal of DHCs (Bhargava et al., 2020). Like Wss1, it was proved that SUMOylation of DPC triggers the recruitment of GCNA/ACRC protease, but in a replication-independent manner (Borgermann et al., 2019). Noteworthy, the chromatin-associated proteins were highly SUMOylated (Borgermann et al., 2019) (Figure 2B), suggesting that formaldehyde induces DHCs, which are then SUMOylated to recruit GCNA/ACRC SprT protease. However, the detailed mechanism of GCNA/ACRC-mediated repair of DHC requires further investigation.
Ddi1 Protease-Based Proteolysis of Histones Cross-Linked to DNA
It was recently shown that a novel protease, Ddi1 (DNA-damage inducible-1), is involved in DPC repair (Serbyn et al., 2020). Ddi1 is an aspartic protease. Its protease domain is structurally similar to that of retroviral aspartic proteases (Krylov and Koonin, 2001; Sirkis et al., 2006). Ddi1 is involved in response to hydroxyurea (HU)-induced replication stress by facilitating the removal of replication termination factor RTF2, restarting stalled replication forks (Kottemann et al., 2018). In addition, it is well documented that Saccharomyces cerevisiae Ddi1 contains a ubiquitin-like (UBL)-ubiquitin associated (UBA) domain, which enables the protein to act as a shuttle delivering ubiquitylated proteins to the proteasome (Dantuma et al., 2009). However, a genetic screen using the tdp1wss1 mutant defective in the processing of DNA-Top1 covalent cross-link (Top1cc), revealed that Ddi1 could rescue mutant cells treated with Top1cc trapping and other genotoxic agents (Serbyn et al., 2020). Retention of Flp-Nick-Induced DPC in Ddi1-depleted cells confirmed a direct role of Ddi1 in DPC repair, due to proteolysis catalyzed by its retroviral-like protease domain (Krylov and Koonin, 2001; Serbyn et al., 2020). Previous evidence supports the idea that the proteolytic function of Ddi1 in DNA repair is replisome-dependent (Clarke et al., 2001; Kottemann et al., 2018) (Figure 2A). However, Ddi1-dependent proteolysis of stalled RNA polymerase II (Pol II) on chromatin suggests an additional function of this protease (Serbyn et al., 2020). Interestingly, it has been demonstrated that Ddi1-mediated proteolysis of DPCs can be a proteasome-independent compensatory mechanism in the case of Wss1 dysfunction (Serbyn et al., 2020). Yeast Ddi1, and the human ortholog DDI2, are more dependent on poly-ubiquitination as compared to proteasome; these proteases cannot cleave non-ubiquitylated proteins and prefer substrates bearing extra-long ubiquitin chains (Sivá et al., 2016; Dirac-Svejstrup et al., 2020; Yip et al., 2020). Serbyn et al. (2020) found that depletion of Ddi1 makes cells hypersensitive to formaldehyde, suggesting that DHC can be a major substrate of aspartyl protease-catalyzed proteolysis. However, the extension of this function of yeast Ddi1 to its human DDI2 ortholog remains unclear, since certain structural domains of Ddi1 and human DDI2 are not evolutionarily conserved (Sivá et al., 2016; Trempe et al., 2016). In conclusion, the respective roles of Ddi1 and DDI2 in the removal of DHCs in higher eukaryotes remain to be investigated.
DNA Repair Pathways in DHC Removal
As mentioned earlier, protein components (histones) of DHC are mainly targeted by specific proteases; however, partial proteolysis of cross-linked histones will still leave a small peptide covalently attached to DNA, requiring the coupling of proteases with classical DNA repair pathways to ensure complete removal of DHC and restoration of the primary DNA structure. In absence of studies on the DHC specific repair mechanisms, in this review we tried to infer the knowledge available on specific type 1 DPC repair mechanisms that are applicable to DHCs.
Nucleotide Excision Repair
Several biochemical and genetic studies showed that NER-deficient cells are sensitive to formaldehyde, suggesting direct involvement of NER in DHC removal (Nishioka, 1973; Takahashi et al., 1985). However, other studies have revealed that NER defective cells are not sensitive to 5-aza-2′-deoxycytidine (5-azadC)-based treatment, which induces cross-linking of DNA cytosine residues to DNA methyltransferase 1 (Dnmt1). This implies that NER can excise DPCs with smaller proteins (<8–12 kDa) induced by formaldehyde, but does not excise DPCs that contain proteins of larger size (>15 kDa), induced by 5-azaC (Bhagwat and Roberts, 1987; Lal et al., 1988; Nakano et al., 2007). This size limitation for excision of DPCs by bacterial NER may be attributed to the strong dependence of UvrB loading efficiency on DPC size, which subsequently influences the overall incision efficiency of the UvrABC nuclease complex. Thus, bacterial NER machinery can make bracketed incisions on DNA strands containing DPC with a small peptide, but not with a large one (Minko et al., 2002, 2005; Nakano et al., 2007). Histones are relatively small proteins ranging in size from 11 to 16 kDa; it was therefore proposed that DHCs could be removed in the NER pathway without preceding proteolysis step. Based on these observations, one may also propose that DHCs/DPCs induced by AP sites in DNA might be removed by NER (Torres-Ramos et al., 2000; Sczepanski et al., 2010). However, the role of NER in repair of AP sites induced DHCs remains to be established. A few studies have demonstrated that NER is implicated in DPC repair in mammalian cells (Fornace, 1982; Baker et al., 2007). Each histone is very small in size; they are assembled into a large NCP, which may sterically inhibit the loading of XPC/Rad23B and XPA/RPA complexes onto the DHC site (Ide et al., 2011). When the XPA/RPA complex fails to bind to the damage site, TFIIH cannot access and open up the DNA duplex around DHC to discriminate between intact and damaged DNA strands. All these render subsequent cleavage of this strand by XPG and XPF-ERCC1 nucleases unfeasible. Thus, proteolysis of DNA-cross-linked NCP might be required for efficient removal of a histone trapped on DNA by the NER machinery (Nakano et al., 2009). A study using mammalian cells has demonstrated that XPF/ERCC1 nuclease requires pre-processing of the cross-linked protein adduct by the proteasome and proteases before its removal (Nakano et al., 2009; Zhang et al., 2011; Stingele et al., 2017). In uvrA cells, plasmid cross-linked to the partially digested histone H1 (peptide sizes: 4.5 kDa and 1.8–3.5 kDa) was more efficiently repaired than a DPC containing a full-length histone (22 kDa) (Nakano et al., 2007) (Figure 2A). It is also worth noting that chromatin remodeling at a DNA damage site is required to provide access to NER machinery (Dinant et al., 2012). Covalent cross-linking of histones will impede this process, strongly suggesting that shrinkage of the bulky protein component must precede repair of the DNA component by the NER pathway. Further studies are required to understand the involvement of specific repair pathway(s) that precede and succeed NER and the sequence of steps involved in the removal of DPC/DHC (involving NER).
Homologous Recombination
The role of homologous recombination in DPC repair has been addressed in bacterial genetic studies, where it was found that Escherichia coli recA and recB mutants defective for homologous recombination (HR) are sensitized to formaldehyde and 5-azadC-induced DPCs (Nishioka, 1973; Takahashi et al., 1985). Further studies revealed that the role of HR in DPC repair is highly conserved in mammalian cells, and that clipping of DPC by the conventional MRN complex leads to strong resistance to DPC-inducing agents (Connelly et al., 2003; Neale et al., 2005; Ridpath et al., 2007; de Graaf et al., 2009; Orta et al., 2013). MRN, a heterotrimeric protein complex, is a DNA nuclease involved in the resection of a double-strand break (DSB) that initiates the HR pathway (Neale et al., 2005; Rothenberg et al., 2009). Increased sister chromatid exchange rates and accumulation of DSBs and RAD51 foci near DPC in formaldehyde-treated mammalian cells further support involvement of HR pathway in DPC removal (Shaham et al., 1997). However, the molecular mechanism of HR-mediated DPC removal is still poorly understood. The repair of DPC in E. coli is dependent on the RecBCD nuclease that initiates the HR pathway (Bidnenko et al., 2002; Nakano et al., 2007). DSB adjacent to DPC and stalled replisome could be generated by re-replication of incomplete nascent DNA strands, with the subsequent collapse of the replication fork. They can also be generated by RecG helicase-mediated fork reversal, leading to the formation of a Holliday junction, which is then processed by RecBCD nuclease to generate DSB (McGlynn and Lloyd, 2001; Michel et al., 2004; Payne et al., 2006; Nakano et al., 2007, 2009). Certain studies suggest that replisome stalling at DPC does not lead to fork collapse, because template switching via fork reversal may allow DNA synthesis (Nakano et al., 2007; Stingele et al., 2015; Klages-Mundt and Li, 2017). In addition, other studies showed that DSB formation is not observed during the replication-dependent repair of DPCs in Xenopus egg extracts and hamster cells treated with DPC-inducing agents (Speit et al., 2000; Duxin et al., 2014). Thus, studies regarding the induction of DSBs near DPC have provided conflicting results, necessitating further studies of the mechanisms of HR-mediated DPCs repair. Differences in the sensitivity of E. coli recA and uvrA mutants, deficient for HR and NER respectively, to large DPCs inducing 5-azadC, revealed that unlike NER, HR could repair large DPCs (Santi et al., 1984; Bhagwat and Roberts, 1987; Ide et al., 2011; Zhang et al., 2020). Since histones are small proteins (11–16 kDa), their size should not be an obstacle to DHC repair in the HR-pathway. However, HR may not directly repair the DHC lesion in the chromatin context until access is provided to DNA damage-sensing factors. Thus, pre-processing of NCP containing a DHC or a repair pathway that precedes HR must be required to repair DHC by HR. Further research is needed to validate this model.
Fanconi Anemia Pathway
The Fanconi anemia (FA) pathway is involved in the repair of inter-strand DNA cross-links (ICLs) and plays a pivotal role in cellular defense against reactive aldehydes (Ridpath et al., 2007; Rosado et al., 2011; Kottemann and Smogorzewska, 2013; Ceccaldi et al., 2016). However, the role of the FA pathway in DPCs repair is currently under debate (Duxin and Walter, 2015). A study conducted by Orta et al. (2013) suggests that Fanconi anemia-dependent HR is required for DPC removal in cells treated with 5-azadC. In addition, several studies have shown that cells depleted in FANC2, FANCQ/XPF, and FANCG proteins are sensitive to DPC-inducing agents, such as acetaldehyde, formaldehyde, and 5-azadC (Ridpath et al., 2007; Mechilli et al., 2008; Lorenti Garcia et al., 2009; Langevin et al., 2011; Rosado et al., 2011; Orta et al., 2013). The majority of DPCs induced by reactive aldehydes are DHCs (Solomon and Varshavsky, 1985). These results are in stark contrast to an in vitro study by Duxin et al. (2014), who demonstrated that depletion of FANCI-FANCD2 from Xenopus egg extracts inhibited ICL repair, but not DPC repair or TLS-mediated bypass. In agreement with latter observations, studies using C. elegans and mouse embryonic fibroblasts showed that depletion of FANCD2 did not affect the cells’ sensitivity to formaldehyde (Stingele et al., 2016). Despite these conflicting reports, the potential role of FA pathway components in the removal of DHCs should not be overlooked. A conserved FANCM-MHF DNA remodeling complex that recognizes a DNA lesion at the stalled replication fork, contains histone-binding sites (Yan et al., 2010). Therefore, it is possible that the FANCM-MHF complex, which recruits downstream Fanconi proteins to excise DHC, could readily recognize DNA cross-linked histones. Future studies are necessary to confirm and delineate the role of the FA pathway in DHC removal, and to determine whether the FA pathway requires pre-processing of DHC/DPC for their removal.
Base Excision Repair
DNA glycosylase-initiated base excision repair (BER) is a major pathway for removing small non-bulky base lesions resulting from deamination, oxidation, and alkylation which do not significantly distort the DNA helix (Krokan and Bjørås, 2013). However, the DNA glycosylases NEIL1 and NEIL3 can also resolve psoralen-induced bulky ICLs in three- and four-stranded DNA structures (Couvé-Privat et al., 2007; Couvé et al., 2009; Martin et al., 2017). NEIL1, a bi-functional DNA glycosylase, can also repair Sp-amine adduct-containing DN-protein cross-links (McKibbin et al., 2013). Furthermore, another study demonstrated that a DPC generated as repair intermediate of PARP1, could be processed by BER machinery (Prasad et al., 2019). Thus, the role of BER may not be limited to small non-bulky DNA lesions.
Interestingly, it has been found that an oxidized AP site formed by a reactive oxygen species (ROS) can trap DNA polymerase β (Polβ) to form a stable DNA- Polβ cross-link (Polβ-DPC) (DeMott et al., 2002). It was later found that DNA glycosylase-generated AP sites can also trap several DNA repair proteins, such as PARP1, Ku proteins, DNA polymerase λ (Polλ), and other factors (Prasad et al., 2019; Quiñones et al., 2020). Thus, under certain circumstances, instead of repairing the lesion, BER can act as a source of a DPC lesion.
It is unknown whether impairment of BER machinery affects cells’ sensitivity to various DPC inducing agents. Also, it is not clear whether BER, like NER, has a size limit for processing of DPCs. As mentioned earlier, histones in a NCP can cross-link to abasic sites. In fact, the AP site generated by a DNA glycosylase may trap histones to form a covalent DHC. A Schiff base at an AP site can lead to DNA strand scission, thus contributing to major nucleosomal DNA damage. Although detailed information on the role of BER in formation of DHC is available, at present, little is known about the role of DNA glycosylases and AP endonucleases in the repair of this chromatin damage. Past evidence suggests that NEIL1 DNA glycosylase has a flexible active site that can accommodate bulky modifications of DNA bases and efficiently remove them (Couvé et al., 2009; McKibbin et al., 2013). Thus, it is predicted that NEIL1, and possibly NEIL3, may play a role in the processing of DHC, but that remains to be confirmed. Also, several studies suggest that BER coupled to proteolysis could participate in the efficient removal of DPC/DHC (Hauer et al., 2017; Prasad et al., 2019; Quiñones et al., 2020). Hence, further studies are required to gather information on the role of BER proteins in DHC removal.
Translesion Synthesis
When a DNA replication fork stalls at unrepaired DNA damage, the cell can circumvent the obstacle through tolerance pathways, such as an HR-mediated template switch mechanism and translesion DNA synthesis (TLS). TLS is a lesion bypass mechanism that tolerates DNA damage and allows for DNA replication to proceed through unrepaired bulky nucleobase adducts (Woodgate, 1999; Friedberg et al., 2005; Lehmann et al., 2007; Sale, 2013). DPCs are super-bulky lesions that can stall replication forks and lead to their collapse. These lesions require a collaborative network of several cellular repair systems to remove them. However, removal of DPC in a stalled replication fork could provoke fork collapse and generation of DSB, with detrimental consequences to a cell, depending on the DNA repair mechanism used. On the other hand, the TLS pathway initiated by specific DNA polymerases replicates over and past the lesion in the damaged DNA template, thus providing a form of DNA damage tolerance, which avoids fork collapse and DSB. Therefore, TLS can play an important role in the management of DPCs in cells. Indeed, several studies have reported that specialized TLS DNA polymerases can bypass DPCs (Duxin et al., 2014; Wickramaratne et al., 2016; Pande et al., 2017; Larsen et al., 2019). Nevertheless, these studies demonstrated that large non-processed DPCs require the degradation of large protein adducts to smaller peptides cross-linked to DNA to allow bypass by TLS DNA polymerases. Thus, partial proteolysis of DPCs is necessary for efficient TLS bypass (Figure 2A). Larsen et al. (2019) study in Xenopus egg extracts clearly established that DPC proteolysis by Spartan/Dvc1 ensures efficient bypass by TLS-specific DNA polymerases REV1-Polζ. Although TLS DNA polymerases help bypass the DPC lesion, the extension of DNA past the lesion by the same DNA polymerases is error-prone and can lead to mutagenesis. Hence, DNA repair pathways, such as mismatch repair (MMR) and NER, could be coupled with TLS to prevent DPC-induced mutations. A study by Wickramaratne et al. (2016) demonstrated that partially digested DHC (histones H4 and H2A) can be bypassed by human TLS DNA polymerases η and κ. However, the authors did not investigate proteolysis of DHCs and the specific proteases involved in their digestion and of the DNA repair pathways that follow TLS. Thus, understanding the role of TLS and associated repair systems in counteracting the genotoxic effects of DHCs requires further investigation.
The Interplay of DHC Repair Pathways
Due to the heterogeneity and super-bulky size of DHCs, several distinct DNA repair pathways may work in concert to remove them. Although cells lack DHC-specific damage sensors, these lesions can be detected and processed by well-known classic DNA repair pathways with the help of various proteases. When a replication fork is stalled at a DHC, the cross-linked histone could be digested by Spartan/Dvc1 protease and proteasome. Proteolysis of DHC by Spartan and proteasome could be backed up with Ddi1 protease in yeast, or with DDI2 in higher eukaryotes. After proteolysis, the remnant peptide could be bypassed by TLS DNA polymerases to avoid replication fork collapse (Figure 2A). Previous evidence also suggested that partial proteolysis of a DPC/DHC coupled with TLS could be a way to repair these complex DNA lesions (Duxin et al., 2014). However, to avoid TLS induced mutations, proteolysis of DPC/DHC could be coupled to NER and HR. Data from several studies support the idea that the combination of proteolysis and NER could be a convenient error-free mechanism for cells to remove the majority of DPCs. Nonetheless, proteolytic degradation of the protein component may also be coupled with BER during removal of DHC/DPC if NER cannot remove the remnant adduct after proteolysis. Considering that most histone cross-links are formed at abasic sites, the BER pathway may have a specific role in removal of AP site-induced DPCs. This leads us to hypothesize that for the efficient removal of AP site-induced DHCs, proteolysis coupled with BER could be one of the most preferred in cells under genotoxic stress conditions that promote DNA base loss (McKibbin et al., 2013; Prasad et al., 2019; Quiñones et al., 2020). One could speculate that HR proceeds after proteolysis. However, Stingele et al. (2014) have demonstrated that HR and proteolysis are two distinct means of resolving DPCs during the S phase of the cell cycle. When there is a DNA strand incision next to a DPC, or when the DPC load is high, the FA-dependent HR or MRN complex-dependent HR, respectively, may take over the protease-mediated repair. Certain aldehyde-induced DPCs require a DNA strand incision near the lesion, which is further processed by FA-dependent HR. Since reactive aldehydes preferentially induce DHCs, it is proposed that FA-dependent HR (ICL-like repair) may serve as a back-up mechanism when proteolysis coupled TLS/NER/BER is inactive or dysfunctional.
Replication-independent repair of DHCs may involve the 26S proteasome-mediated degradation of DHC, followed by removal of the remnant peptide by the global genome or transcription-coupled (TC) NER (Quievryn and Zhitkovich, 2000; de Graaf et al., 2009) (Figure 2A). Considering that Spartan/Dvc1 protease can play a role outside DNA replication, it may mediate DHC proteolysis that blocks transcription, and the remnants are then removed in the TC-NER sub-pathway. In germ and certain quiescent cells, the GCNA1/ACRC protease may be involved in degradation of DHCs, whereas remnants of the proteolysis could be removed by NER (Figure 2B). In the absence of NER, replication-independent proteolysis by proteasome 26S/Spartan/GCNA1 may be coupled with BER to remove DHCs. These hypothetical models of the interplay of different repair pathways in DHC removal require further studies to test them.
Concluding Remarks
DNA-protein cross-links occur frequently and are the most bulky DNA lesions in living cells. Among various DPCs, DHCs occupy a special place, because histones, the most abundant DNA-binding proteins, constitute the nucleosome, a basic structural unit of chromatin. Therefore, we propose to classify DNA-histone covalent complexes as a special DHC group of DPCs. Histones cross-link either to DNA bases, mostly guanines and 5-formylcytosines, or to the AP site sugar (Ren et al., 2019). With more than 10,000 abasic sites generated every day in a cell far exceeding the number of oxidized bases (Lindahl, 1993), this lesion is the most abundant type of endogenous DNA damage. Indeed, around 10% of AP sites catalyze the formation of DPCs, among which the majority are likely to be DHCs (Ren et al., 2019). Therefore, it seems that hundreds, and perhaps even thousands of histone cross-links to abasic sites in DNA are formed every day in a cell. Even greater production of DHCs is expected in cells exposed to certain genotoxic stress, leading to significant biological consequences, such as sensitization of cancer cell to chemotherapeutic agents. It is difficult to delineate a single repair pathway for various types of DHCs. Similar to DPCs, the repair of super-bulky DHC lesions requires two steps: partial proteolysis of histone and repair of remaining cross-linked peptide via DNA excision. Cells utilize several proteases and DNA repair pathways for these purposes. Assuming that active BER can generate an excess number of AP sites as repair intermediates of DNA glycosylases, this repair pathway might be one of the major factors in the formation of DHCs in cells. A recent discovery of the role of DNA glycosylases of the Nei-family in repair of bulky ICLs suggests that the BER pathway may participate in removal of AP site-induced DHCs (Couvé-Privat et al., 2007; Semlow et al., 2016; Martin et al., 2017). In eukaryotic cells, chromatin dynamics plays an essential role in DHC removal by various DNA repair pathways. For example, it is well documented that partial and transient nucleosomal DNA unwrapping is indispensable for DNA repair and for transcription initiation. Indeed, several studies have indicated that on average, DNA unwrapping events occur several times per second (Li and Widom, 2004). DHCs should strongly interfere with natural nucleosome unwrapping, and hence with DNA lesion detection, signaling, and repair. Moreover, several laboratories have detected inter-nucleosomal cross-links, including DHCs mediated by histone tail domains. These structures should further impede accessibility of DNA lesions for the DNA repair machinery (Banerjee et al., 2018; Yang and Greenberg, 2019). This implies that PTMs of histone tails via lysine acetylation and methylation, and recruitment of chromatin remodeling complexes, may also influence DHC formation and repair (Yang et al., 2019). Blocking chromatin remodeling and DNA repair pathways involved in DHC removal should lead to the persistence of these DNA lesions and further impede chromatin-based transactions and chromatin organization. However, current knowledge regarding DNA repair pathways involved in DHC removal is far from complete. Further studies are required to delineate the mechanisms involved in the repair of DHCs.
Author Contributions
MP, BM, MS, and RG wrote the DNA repair part of the review. MP, RG, and AK wrote the proteasome part of the review and prepared figures. All authors discussed and contributed to analysis of published literature and to writing the manuscript. All authors contributed to the article and approved the submitted version.
Funding
This work was supported by grants from la Ligue Nationale Française Contre le Cancer “Equipe LNCC 2016” (https://www.ligue-cancer.net) and Electricité de France RB 2020-02 (http://www.edf.fr) to MS, by Nazarbayev University Oak Ridge Associated Universities (ORAU) grant 091019CRP2111 to BM and by grant 1R01CA213223 from the National Cancer Institute to AK. MP is supported by a U54 Moonshot grant from the National Institutes of Health (NIH) and the SBF-SU2C grant from American Association of Cancer Research/Sub-Children’s Hospital of Philadelphia to Dr. Poul Sorensen.
Conflict of Interest
AK is the founder and Chief Scientific Officer of InhiProt LLC.
The authors declare that the research was conducted in the absence of any commercial or financial relationships that could be construed as a potential conflict of interest.
Acknowledgments
We thank Biorender.com and Marvin Sketch for their assistance in preparation of the figures. We are grateful to Dr. Noah Horowitz for editing assistance.
References
Adams, J. (2004). The development of proteasome inhibitors as anticancer drugs. Cancer Cell 5, 417–421. doi: 10.1016/S1535-6108(04)00120-5
Baker, D. J., Wuenschell, G., Xia, L., Termini, J., Bates, S. E., and Riggs, A. D. (2007). Nucleotide excision repair eliminates unique dna-protein cross-links from mammalian cells. J. Biol. Chem. 282, 22592–22604. doi: 10.1074/jbc.M702856200
Balakirev, M. Y., Mullally, J. E., Favier, A., Assard, N., Sulpice, E., and Lindsey, D. F. (2015). Wss1 metalloprotease partners with Cdc48/Doa1 in processing genotoxic SUMO conjugates. eLife 4:e06763. doi: 10.7554/eLife.06763
Banerjee, D. R., Deckard, C. E., Elinski, M. B., Buzbee, M. L., Wang, W. W., and Batteas, J. D. (2018). Plug-and-play approach for preparing chromatin containing site-specific DNA modifications: the influence of chromatin structure on base excision repair. J. Am. Chem. Soc. 140, 8260–8267. doi: 10.1021/jacs.8b04063
Bhagwat, A. S., and Roberts, R. J. (1987). Genetic analysis of the 5-azacytidine sensitivity of Escherichia coli K-12. J. Bacteriol. 169, 1537–1546. doi: 10.1128/jb.169.4.1537-1546.1987
Bhargava, V., Goldstein, C. D., Russell, L., Xu, L., Ahmed, M., Li, W., et al. (2020). GCNA preserves genome integrity and fertility across species. Dev. Cell 52, 38.e10–52.e10. doi: 10.1016/j.devcel.2019.11.007
Bidnenko, V., Ehrlich, S. D., and Michel, B. (2002). Replication fork collapse at replication terminator sequences. EMBO J. 21, 3898–3907. doi: 10.1093/emboj/cdf369
Borgermann, N., Ackermann, L., Schwertman, P., Hendriks, I. A., Thijssen, K., Liu, J. C., et al. (2019). SUMOylation promotes protective responses to DNA-protein crosslinks. EMBO J. 38:e101496. doi: 10.15252/embj.2019101496
Bowman, G. D., and Poirier, M. G. (2015). Post-translational modifications of histones that influence nucleosome dynamics. Chem. Rev. 115, 2274–2295. doi: 10.1021/cr500350x
Carmell, M. A., Dokshin, G. A., Skaletsky, H., Hu, Y.-C., van Wolfswinkel, J. C., Igarashi, K. J., et al. (2016). A widely employed germ cell marker is an ancient disordered protein with reproductive functions in diverse eukaryotes. eLife 5:e19993. doi: 10.7554/eLife.19993
Ceccaldi, R., Sarangi, P., and D’Andrea, A. D. (2016). The Fanconi anaemia pathway: new players and new functions. Nat. Rev. Mol. Cell Biol. 17, 337–349. doi: 10.1038/nrm.2016.48
Ciechanover, A. (1998). The ubiquitin-proteasome pathway: on protein death and cell life. EMBO J. 17, 7151–7160. doi: 10.1093/emboj/17.24.7151
Clarke, D. J., Mondesert, G., Segal, M., Bertolaet, B. L., Jensen, S., Wolff, M., et al. (2001). Dosage suppressors of pds1 implicate ubiquitin-associated domains in checkpoint control. Mol. Cell. Biol. 21, 1997–2007. doi: 10.1128/MCB.21.6.1997-2007.2001
Connelly, J. C., de Leau, E. S., and Leach, D. R. F. (2003). Nucleolytic processing of a protein-bound DNA end by the E. coli SbcCD (MR) complex. DNA Repair. 2, 795–807. doi: 10.1016/S1568-7864(03)00063-6
Couvé, S., Macé-Aimé, G., Rosselli, F., and Saparbaev, M. K. (2009). The human oxidative DNA Glycosylase NEIL1 excises psoralen-induced interstrand DNA cross-links in a three-stranded DNA structure. J. Biol. Chem. 284, 11963–11970. doi: 10.1074/jbc.M900746200
Couvé-Privat, S., Macé, G., Rosselli, F., and Saparbaev, M. K. (2007). Psoralen-induced DNA adducts are substrates for the base excision repair pathway in human cells. Nucleic Acids Res 35, 5672–5682. doi: 10.1093/nar/gkm592
Coux, O., Tanaka, K., and Goldberg, A. L. (1996). Structure and functions of the 20S and 26S proteasomes. Annu. Rev. Biochem. 65, 801–847. doi: 10.1146/annurev.bi.65.070196.004101
Dantuma, N. P., Heinen, C., and Hoogstraten, D. (2009). The ubiquitin receptor Rad23: at the crossroads of nucleotide excision repair and proteasomal degradation. DNA Repair. 8, 449–460. doi: 10.1016/j.dnarep.2009.01.005
Davey, C. A., Sargent, D. F., Luger, K., Maeder, A. W., and Richmond, T. J. (2002). Solvent mediated interactions in the structure of the nucleosome core particle at 1.9 a resolution. J. Mol. Biol. 319, 1097–1113. doi: 10.1016/S0022-2836(02)00386-8
Davis, E. J., Lachaud, C., Appleton, P., Macartney, T. J., Näthke, I., and Rouse, J. (2012). DVC1 (C1orf124) recruits the p97 protein segregase to sites of DNA damage. Nat. Struct. Mol. Biol. 19, 1093–1100. doi: 10.1038/nsmb.2394
de Graaf, B., Clore, A., and McCullough, A. K. (2009). Cellular pathways for DNA repair and damage tolerance of formaldehyde-induced DNA-protein crosslinks. DNA Repair. 8, 1207–1214. doi: 10.1016/j.dnarep.2009.06.007
Delabaere, L., Orsi, G. A., Sapey-Triomphe, L., Horard, B., Couble, P., and Loppin, B. (2014). The spartan ortholog maternal haploid is required for paternal chromosome integrity in the drosophila zygote. Curr. Biol, 24, 2281–2287. doi: 10.1016/j.cub.2014.08.010
DeMott, M. S., Beyret, E., Wong, D., Bales, B. C., Hwang, J.-T., Greenberg, M. M., et al. (2002). Covalent trapping of human DNA Polymerase β by the Oxidative DNA Lesion 2-deoxyribonolactone. J. Biol. Chem. 277, 7637–7640. doi: 10.1074/jbc.C100577200
Dinant, C., Bartek, J., and Bekker-Jensen, S. (2012). Histone displacement during nucleotide excision repair. Int. J. Mol. Sci. 13, 13322–13337. doi: 10.3390/ijms131013322
Dirac-Svejstrup, A. B., Walker, J., Faull, P., Encheva, V., Akimov, V., Puglia, M., et al. (2020). DDI2 is a ubiquitin-directed endoprotease responsible for cleavage of transcription factor NRF1. Mol. Cell 79, 332.e7–341.e7. doi: 10.1016/j.molcel.2020.05.035
Dokshin, G. A., Davis, G. M., Sawle, A. D., Eldridge, M. D., Nicholls, P. K., Gourley, T. E., et al. (2020). GCNA interacts with spartan and topoisomerase II to regulate genome stability. Dev. Cell 52, 53.e6–68.e6. doi: 10.1016/j.devcel.2019.11.006
Duxin, J. P., Dewar, J. M., Yardimci, H., and Walter, J. C. (2014). Repair of a DNA-protein crosslink by replication-coupled proteolysis. Cell 159, 346–357. doi: 10.1016/j.cell.2014.09.024
Duxin, J. P., and Walter, J. C. (2015). What is the DNA repair defect underlying Fanconi anemia? Curr. Opin. Cell Biol. 37, 49–60. doi: 10.1016/j.ceb.2015.09.002
Eickbush, T. H., and Moudrianakis, E. N. (1978). The histone core complex: an octamer assembled by two sets of protein-protein interactions. Biochemistry 17, 4955–4964. doi: 10.1021/bi00616a016
Fornace, A. J. (1982). Detection of DNA single-strand breaks produced during the repair of damage by DNA-protein cross-linking agents. Cancer Res. 42, 145–149.
Fortini, P., and Dogliotti, E. (2007). Base damage and single-strand break repair: mechanisms and functional significance of short- and long-patch repair subpathways. DNA Repair. 6, 398–409. doi: 10.1016/j.dnarep.2006.10.008
Friedberg, E. C., Lehmann, A. R., and Fuchs, R. P. P. (2005). Trading places: how do DNA polymerases switch during translesion DNA synthesis? Mol. Cell 18, 499–505. doi: 10.1016/j.molcel.2005.03.032
Groll, M., Ditzel, L., Löwe, J., Stock, D., Bochtler, M., Bartunik, H. D., et al. (1997). Structure of 20S proteasome from yeast at 2.4Å resolution. Nature 386, 463–471. doi: 10.1038/386463a0
Gros, L., Ishchenko, A. A., Ide, H., Elder, R. H., and Saparbaev, M. K. (2004). The major human AP endonuclease (Ape1) is involved in the nucleotide incision repair pathway. Nucleic Acids Res. 32, 73–81. doi: 10.1093/nar/gkh165
Hauer, M. H., and Gasser, S. M. (2017). Chromatin and nucleosome dynamics in DNA damage and repair. Genes Dev. 31, 2204–2221. doi: 10.1101/gad.307702.117
Hauer, M. H., Seeber, A., Singh, V., Thierry, R., Sack, R., Amitai, A., et al. (2017). Histone degradation in response to DNA damage enhances chromatin dynamics and recombination rates. Nat. Struct. Mol. Biol. 24, 99–107. doi: 10.1038/nsmb.3347
Hinz, J. M., Rodriguez, Y., and Smerdon, M. J. (2010). Rotational dynamics of DNA on the nucleosome surface markedly impact accessibility to a DNA repair enzyme. PNAS 107, 4646–4651. doi: 10.1073/pnas.0914443107
Ho, T. V., and Schärer, O. D. (2010). Translesion DNA synthesis polymerases in DNA interstrand crosslink repair. Environ. Mol. Mutagen. 51, 552–566. doi: 10.1002/em.20573
Ide, H., Nakano, T., Salem, A. M. H., and Shoulkamy, M. I. (2018). DNA–protein cross-links: formidable challenges to maintaining genome integrity. DNA Repair. 71, 190–197. doi: 10.1016/j.dnarep.2018.08.024
Ide, H., Shoulkamy, M. I., Nakano, T., Miyamoto-Matsubara, M., and Salem, A. M. H. (2011). Repair and biochemical effects of DNA–protein crosslinks. Mutat. Res. Fund.Mol. Mech. Mutagen. 711, 113–122. doi: 10.1016/j.mrfmmm.2010.12.007
Ide, H., Nakano, T., Shoulkamy, M. I., and Salem, A. M. H. (2015). “Formation, repair, and biological effects of DNA–protein cross-link damage,” Adv. DNA Repair. ed C. C. Chen (INTECH), 43–80. doi: 10.5772/59683
Ignatov, A. V., Bondarenko, K. A., and Makarova, A. V. (2017). Non-bulky lesions in human DNA: the ways of formation, repair, and replication. Acta Nat. 9, 12–26. doi: 10.32607/20758251-2017-9-3-12-26
Ishchenko, A. A., Deprez, E., Maksimenko, A., Brochon, J.-C., Tauc, P., and Saparbaev, M. K. (2006). Uncoupling of the base excision and nucleotide incision repair pathways reveals their respective biological roles. PNAS 103, 2564–2569. doi: 10.1073/pnas.0508582103
Jentsch, S., and Psakhye, I. (2013). Control of nuclear activities by substrate-selective and protein-group SUMOylation. Annu. Rev. Genet. 47, 167–186. doi: 10.1146/annurev-genet-111212-133453
Ji, S., Thomforde, J., Rogers, C., Fu, I., Broyde, S., and Tretyakova, N. Y. (2019). Transcriptional bypass of DNA–protein and DNA–Peptide conjugates by T7 RNA polymerase. ACS Chem. Biol. 14, 2564–2575. doi: 10.1021/acschembio.9b00365
Kisselev, A. F., Callard, A., and Goldberg, A. L. (2006). Importance of the different proteolytic sites of the proteasome and the efficacy of inhibitors varies with the protein substrate. J. Biol. Chem. 281, 8582–8590. doi: 10.1074/jbc.M509043200
Klages-Mundt, N. L., and Li, L. (2017). Formation and repair of DNA-protein crosslink damage. Sci. China Life Sci. 60, 1065–1076. doi: 10.1007/s11427-017-9183-4
Kottemann, M. C., Conti, B. A., Lach, F. P., and Smogorzewska, A. (2018). Removal of RTF2 from Stalled Replisomes Promotes Maintenance of Genome Integrity. Mol. Cell 69, 24.e5–35.e5. doi: 10.1016/j.molcel.2017.11.035
Kottemann, M. C., and Smogorzewska, A. (2013). Fanconi anaemia and the repair of Watson and Crick DNA crosslinks. Nature 493, 356–363. doi: 10.1038/nature11863
Krokan, H. E., and Bjørås, M. (2013). Base excision repair. Cold Spring Harb. Perspect. Biol. 5:a012583. doi: 10.1101/cshperspect.a012583
Krylov, D. M., and Koonin, E. V. (2001). Correspondence: a novel family of predicted retroviral-like aspartyl proteases with a possible key role in eukaryotic cell cycle control. Curr. Biol. 11, R584–R587. doi: 10.1016/S0960-9822(01)00357-8
Kühbacher, U., and Duxin, J. P. (2020). How to fix DNA-protein crosslinks. DNA Repair 94:102924. doi: 10.1016/j.dnarep.2020.102924
Kuo, H. K., Griffith, J. D., and Kreuzer, K. N. (2007). 5-azacytidine–induced methyltransferase-DNA adducts block DNA replication in vivo. Cancer Res. 67, 8248–8254. doi: 10.1158/0008-5472.CAN-07-1038
Kuykendall, J. R., and Bogdanffy, M. S. (1992). Efficiency of DNA-histone crosslinking induced by saturated and unsaturated aldehydes in vitro. Mutat. Res. 283, 131–136. doi: 10.1016/0165-7992(92)90145-8
Lal, D., Som, S., and Friedman, S. (1988). Survival and mutagenic effects of 5-azacytidine in Escherichia coli. Mutat. Res. DNA Repair Rep. 193, 229–236. doi: 10.1016/0167-8817(88)90033-8
Lam, C. W., Casanova, M., and Heck, H. D. (1985). Depletion of nasal mucosal glutathione by acrolein and enhancement of formaldehyde-induced DNA-protein cross-linking by simultaneous exposure to acrolein. Arch. Toxicol. 58, 67–71. doi: 10.1007/BF00348311
Langevin, F., Crossan, G. P., Rosado, I. V., Arends, M. J., and Patel, K. J. (2011). Fancd2 counteracts the toxic effects of naturally produced aldehydes in mice. Nature 475, 53–58. doi: 10.1038/nature10192
Larsen, N. B., Gao, A. O., Sparks, J. L., Gallina, I., Wu, R. A., Mann, M., et al. (2019). Replication-coupled dna-protein crosslink repair by SPRTN and the proteasome in xenopus egg extracts. Mol. Cell 73, 574.e7–588.e7. doi: 10.1016/j.molcel.2018.11.024
Lecker, S. H., Goldberg, A. L., and Mitch, W. E. (2006). Protein degradation by the ubiquitin–proteasome pathway in normal and disease states. JASN 17, 1807–1819. doi: 10.1681/ASN.2006010083
Lehmann, A. R., Niimi, A., Ogi, T., Brown, S., Sabbioneda, S., Wing, J. F., et al. (2007). Translesion synthesis: Y-family polymerases and the polymerase switch. DNA Repair 6, 891–899. doi: 10.1016/j.dnarep.2007.02.003
Li, F., Zhang, Y., Bai, J., Greenberg, M. M., Xi, Z., and Zhou, C. (2017). 5-Formylcytosine Yields DNA–protein cross-links in nucleosome core particles. J. Am. Chem. Soc. 139, 10617–10620. doi: 10.1021/jacs.7b05495
Li, G., and Widom, J. (2004). Nucleosomes facilitate their own invasion. Nat. Struct. Mol. Biol. 11, 763–769. doi: 10.1038/nsmb801
Lindahl, T. (1993). Instability and decay of the primary structure of DNA. Nature 362, 709–715. doi: 10.1038/362709a0
Loeber, R. L., Michaelson-Richie, E. D., Codreanu, S. G., Liebler, D. C., Campbell, C. R., and Tretyakova, N. Y. (2009). Proteomic analysis of DNA-Protein cross-linking by antitumor nitrogen Mustards. Chem. Res. Toxicol. 22, 1151–1162. doi: 10.1021/tx900078y
Lorenti Garcia, C., Mechilli, M., Proietti De Santis, L., Schinoppi, A., Katarzyna, K., and Palitti, F. (2009). Relationship between DNA lesions, DNA repair and chromosomal damage induced by acetaldehyde. Mutat. Res. Fund. Mol. Mech. Mutagen. 662, 3–9. doi: 10.1016/j.mrfmmm.2008.11.008
Löwe, J., Stock, D., Jap, B., Zwickl, P., Baumeister, W., and Huber, R. (1995). Crystal structure of the 20S proteasome from the archaeon T. acidophilum at 3.4 A resolution. Science 268, 533–539. doi: 10.1126/science.7725097
Luger, K., Dechassa, M. L., and Tremethick, D. J. (2012). New insights into nucleosome and chromatin structure: an ordered state or a disordered affair? Nat, Rev. Mol. Cell Biol. 13, 436–447. doi: 10.1038/nrm3382
Luger, K., Mäder, A. W., Richmond, R. K., Sargent, D. F., and Richmond, T. J. (1997). Crystal structure of the nucleosome core particle at 2.8 A resolution. Nature 389, 251–260. doi: 10.1038/38444
Maddi, K., Sam, D. K., Bonn, F., Prgomet, S., Tulowetzke, E., Akutsu, M., et al. (2020). Wss1 promotes replication stress tolerance by degrading histones. Cell Rep. 30, 3117.e4–3126.e4. doi: 10.1016/j.celrep.2020.02.018
Mandemaker, I. K., Geijer, M. E., Kik, I., Bezstarosti, K., Rijkers, E., Raams, A., et al. (2018). DNA damage-induced replication stress results in PA200-proteasome-mediated degradation of acetylated histones. EMBO Rep. 19:e45566. doi: 10.15252/embr.201745566
Martin, P. R., Couvé, S., Zutterling, C., Albelazi, M. S., Groisman, R., Matkarimov, B. T., et al. (2017). The human DNA glycosylases NEIL1 and NEIL3 Excise Psoralen-Induced DNA-DNA cross-links in a four-stranded DNA structure. Sci. Rep. 7:17438. doi: 10.1038/s41598-017-17693-4
McGlynn, P., and Lloyd, R. G. (2001). Rescue of stalled replication forks by RecG: simultaneous translocation on the leading and lagging strand templates supports an active DNA unwinding model of fork reversal and Holliday junction formation. PNAS 98, 8227–8234. doi: 10.1073/pnas.111008698
McKibbin, P. L., Fleming, A. M., Towheed, M. A., Van Houten, B., Burrows, C. J., and David, S. S. (2013). Repair of hydantoin lesions and their amine adducts in DNA by base and nucleotide excision repair. J. Am. Chem. Soc. 135, 13851–13861. doi: 10.1021/ja4059469
Mechilli, M., Schinoppi, A., Kobos, K., Natarajan, A. T., and Palitti, F. (2008). DNA repair deficiency and acetaldehyde-induced chromosomal alterations in CHO cells. Mutagenesis 23, 51–56. doi: 10.1093/mutage/gem042
Michel, B., Grompone, G., Florès, M.-J., and Bidnenko, V. (2004). Multiple pathways process stalled replication forks. PNAS 101, 12783–12788. doi: 10.1073/pnas.0401586101
Ming, X., Groehler, A., Michaelson-Richie, E. D., Villalta, P. W., Campbell, C., and Tretyakova, N. Y. (2017). Mass spectrometry based proteomics study of cisplatin-induced DNA–Protein cross-linking in human fibrosarcoma (HT1080) cells. Chem. Res. Toxicol. 30, 980–995. doi: 10.1021/acs.chemrestox.6b00389
Minko, I. G., Kurtz, A. J., Croteau, D. L., Van Houten, B., Harris, T. M., and Lloyd, R. S. (2005). Initiation of repair of DNA–polypeptide cross-links by the UvrABC nuclease. Biochemistry 44, 3000–3009. doi: 10.1021/bi0478805
Minko, I. G., Zou, Y., and Lloyd, R. S. (2002). Incision of DNA–protein crosslinks by UvrABC nuclease suggests a potential repair pathway involving nucleotide excision repair. PNAS 99, 1905–1909. doi: 10.1073/pnas.042700399
Mohni, K. N., Wessel, S. R., Zhao, R., Wojciechowski, A. C., Luzwick, J. W., Layden, H., et al. (2019). HMCES maintains genome integrity by shielding abasic sites in single-strand DNA. Cell 176, 144.e13–153.e13. doi: 10.1016/j.cell.2018.10.055
Mosbech, A., Gibbs-Seymour, I., Kagias, K., Thorslund, T., Beli, P., Povlsen, L., et al. (2012). DVC1 (C1orf124) is a DNA damage–targeting p97 adaptor that promotes ubiquitin-dependent responses to replication blocks. Nat. Struct. Mol. Biol. 19, 1084–1092. doi: 10.1038/nsmb.2395
Nakano, T., Katafuchi, A., Matsubara, M., Terato, H., Tsuboi, T., Masuda, T., et al. (2009). Homologous recombination but not nucleotide excision repair plays a pivotal role in tolerance of DNA-protein cross-links in mammalian cells. J. Biol. Chem. 284, 27065–27076. doi: 10.1074/jbc.M109.019174
Nakano, T., Miyamoto-Matsubara, M., Shoulkamy, M. I., Salem, A. M. H., Pack, S. P., Ishimi, Y., et al. (2013). Translocation and stability of replicative DNA helicases upon encountering DNA-protein cross-links. J. Biol. Chem. 288, 4649–4658. doi: 10.1074/jbc.M112.419358
Nakano, T., Morishita, S., Katafuchi, A., Matsubara, M., Horikawa, Y., Terato, H., et al. (2007). Nucleotide excision repair and homologous recombination systems commit differentially to the repair of DNA-protein crosslinks. Mol. Cell 28, 147–158. doi: 10.1016/j.molcel.2007.07.029
Nakano, T., Xu, X., Salem, A. M. H., Shoulkamy, M. I., and Ide, H. (2017). Radiation-induced DNA–protein cross-links: mechanisms and biological significance. Free Radic. Biol. Med. 107, 136–145. doi: 10.1016/j.freeradbiomed.2016.11.041
Neale, M. J., Pan, J., and Keeney, S. (2005). Endonucleolytic processing of covalent protein-linked DNA double-strand breaks. Nature 436, 1053–1057. doi: 10.1038/nature03872
Nishioka, H. (1973). Lethal and mutagenic action of formaldehyde in Hcr+ and Hcr− strains of Escherichiacoli. Mutat. Res. Fund. Mol. Mech. Mutag. 17, 261–265. doi: 10.1016/0027-5107(73)90175-9
Orta, M. L., Calderón-Montaño, J. M., Domínguez, I., Pastor, N., Burgos-Morón, E., López-Lázaro, M., et al. (2013). 5-Aza-2′-deoxycytidine causes replication lesions that require Fanconi anemia-dependent homologous recombination for repair. Nucleic Acids Res. 41, 5827–5836. doi: 10.1093/nar/gkt270
Pande, P., Ji, S., Mukherjee, S., Schärer, O. D., Tretyakova, N. Y., and Basu, A. K. (2017). Mutagenicity of a model DNA-Peptide cross-link in human cells: roles of translesion synthesis DNA polymerases. Chem. Res. Toxicol. 30, 669–677. doi: 10.1021/acs.chemrestox.6b00397
Payne, B. T. I., van Knippenberg, I. C., Bell, H., Filipe, S. R., Sherratt, D. J., and McGlynn, P. (2006). Replication fork blockage by transcription factor-DNA complexes in Escherichia coli. Nucleic Acids Res. 34, 5194–5202. doi: 10.1093/nar/gkl682
Prasad, R., Horton, J. K., Dai, D.-P., and Wilson, S. H. (2019). Repair pathway for PARP-1 DNA-protein crosslinks. DNA Repair 73, 71–77. doi: 10.1016/j.dnarep.2018.11.004
Psakhye, I., and Jentsch, S. (2012). Protein group modification and synergy in the SUMO pathway as exemplified in DNA Repair. Cell 151, 807–820. doi: 10.1016/j.cell.2012.10.021
Qian, M.-X., Pang, Y., Liu, C. H., Haratake, K., Du, B.-Y., Ji, D.-Y., et al. (2013). Acetylation-mediated proteasomal degradation of core histones during DNA repair and spermatogenesis. Cell 153, 1012–1024. doi: 10.1016/j.cell.2013.04.032
Quievryn, G., and Zhitkovich, A. (2000). Loss of DNA–protein crosslinks from formaldehyde-exposed cells occurs through spontaneous hydrolysis and an active repair process linked to proteosome function. Carcinogenesis 21, 1573–1580. doi: 10.1093/carcin/21.8.1573
Quiñones, J. L., Thapar, U., Wilson, S. H., Ramsden, D. A., and Demple, B. (2020). Oxidative DNA-protein crosslinks formed in mammalian cells by abasic site lyases involved in DNA repair. DNA Repair 87:102773. doi: 10.1016/j.dnarep.2019.102773
Raiber, E.-A., Portella, G., Martínez Cuesta, S., Hardisty, R., Murat, P., Li, Z., et al. (2018). 5-Formylcytosine organizes nucleosomes and forms Schiff base interactions with histones in mouse embryonic stem cells. Nat. Chem. 10, 1258–1266. doi: 10.1038/s41557-018-0149-x
Reinking, H. K., Hofmann, K., and Stingele, J. (2020). Function and evolution of the DNA-protein crosslink proteases Wss1 and SPRTN. DNA Repair 88:102822. doi: 10.1016/j.dnarep.2020.102822
Ren, M., Bai, J., Xi, Z., and Zhou, C. (2019). DNA damage in nucleosomes. Sci. China Chem. 62, 561–570. doi: 10.1007/s11426-018-9421-5
Ridpath, J. R., Nakamura, A., Tano, K., Luke, A. M., Sonoda, E., Arakawa, H., et al. (2007). Csells deficient in the FANC/BRCA pathway are hypersensitive to plasma levels of formaldehyde. Cancer Res. 67, 11117–11122. doi: 10.1158/0008-5472.CAN-07-3028
Rohs, R., West, S. M., Sosinsky, A., Liu, P., Mann, R. S., and Honig, B. (2009). The role of DNA shape in protein-DNA recognition. Nature 461, 1248–1253. doi: 10.1038/nature08473
Rosado, I. V., Langevin, F., Crossan, G. P., Takata, M., and Patel, K. J. (2011). Formaldehyde catabolism is essential in cells deficient for the Fanconi anemia DNA-repair pathway. Nat. Struct. Mol. Biolo. 18, 1432–1434. doi: 10.1038/nsmb.2173
Rothenberg, M., Kohli, J., and Ludin, K. (2009). Ctp1 and the MRN-complex are required for endonucleolytic Rec12 removal with release of a single class of oligonucleotides in fission yeast. PLoS Genet. 5:e1000722. doi: 10.1371/journal.pgen.1000722
Sale, J. E. (2013). Translesion DNA synthesis and mutagenesis in eukaryotes. Cold Spring Harb. Perspect. Biol. 5:a012708. doi: 10.1101/cshperspect.a012708
Santi, D. V., Norment, A., and Garrett, C. E. (1984). Covalent bond formation between a DNA-cytosine methyltransferase and DNA containing 5-azacytosine. PNAS 81, 6993–6997. doi: 10.1073/pnas.81.22.6993
Sczepanski, J. T., Wong, R. S., McKnight, J. N., Bowman, G. D., and Greenberg, M. M. (2010). Rapid DNA–protein cross-linking and strand scission by an abasic site in a nucleosome core particle. PNAS 107, 22475–22480. doi: 10.1073/pnas.1012860108
Semlow, D. R., Zhang, J., Budzowska, M., Drohat, A. C., and Walter, J. C. (2016). Replication-dependent unhooking of DNA interstrand cross-links by the NEIL3 glycosylase. Cell 167, 498.e14–511.e14. doi: 10.1016/j.cell.2016.09.008
Serbyn, N., Noireterre, A., Bagdiul, I., Plank, M., Michel, A. H., Loewith, R., et al. (2020). The aspartic protease Ddi1 contributes to DNA-Protein crosslink repair in yeast. Mol. Cell 77, 1066.e9–1079.e9. doi: 10.1016/j.molcel.2019.12.007
Shaham, J., Bomstein, Y., Melzer, A., and Ribak, J. (1997). DNA–Protein crosslinks and sister chromatid exchanges as biomarkers of exposure to formaldehyde. Int. J. Occup. Environ. Health 3, 95–104. doi: 10.1179/107735297800407695
Shang, M., Ren, M., and Zhou, C. (2019). Nitrogen mustard induces formation of DNA–histone cross-links in nucleosome core particles. Chem. Res. Toxicol. 32, 2517–2525. doi: 10.1021/acs.chemrestox.9b00354
Sharma, S., Helchowski, C. M., and Canman, C. E. (2013). The roles of DNA polymerase ζ and the Y family DNA polymerases in promoting or preventing genome instability. Mutat. Res. Fund. Mol. Mech. Mutagen. 74, 97–110. doi: 10.1016/j.mrfmmm.2012.11.002
Sirkis, R., Gerst, J. E., and Fass, D. (2006). Ddi1, a eukaryotic protein with the retroviral protease fold. J. Mol. Biol. 364, 376–387. doi: 10.1016/j.jmb.2006.08.086
Sivá, M., Svoboda, M., Veverka, V., Trempe, J.-F., Hofmann, K., Kožíšek, M., et al. (2016). Human DNA-damage-inducible 2 protein is structurally and functionally distinct from its yeast Ortholog. Sci. Rep. 6:30443. doi: 10.1038/srep30443
Smith, K. C. (1966). Physical and chemical changes induced in nucleic acids by ultraviolet light. Radiat. Res. Suppl. 6, 54–79. doi: 10.2307/3583551
Solomon, M. J., and Varshavsky, A. (1985). Formaldehyde-mediated DNA-protein crosslinking: a probe for in vivo chromatin structures. PNAS 82, 6470–6474. doi: 10.1073/pnas.82.19.6470
Sparks, J. L., Chistol, G., Gao, A. O., Räschle, M., Larsen, N. B., Mann, M., et al. (2019). The CMG helicase bypasses DNA-Protein cross-links to facilitate their repair. Cell 176, 167.e21–181.e21. doi: 10.1016/j.cell.2018.10.053
Speit, G., Schütz, P., and Merk, O. (2000). Induction and repair of formaldehyde-induced DNA–protein crosslinks in repair-deficient human cell lines. Mutagenesis 15, 85–90. doi: 10.1093/mutage/15.1.85
Sperling, J., and Sperling, R. (1978). Photochemical cross-linking of histones to DNA nucleosomes. Nucleic Acids Res. 5, 2755–2773. doi: 10.1093/nar/5.8.2755
Stadtmueller, B. M., and Hill, C. P. (2011). Proteasome activators. Mol. Cell 41, 8–19. doi: 10.1016/j.molcel.2010.12.020
Stingele, J., Bellelli, R., Alte, F., Hewitt, G., Sarek, G., Maslen, S. L., et al. (2016). Mechanism and regulation of DNA-protein crosslink repair by the DNA-Dependent metalloprotease SPRTN. Mol. Cell 64, 688–703. doi: 10.1016/j.molcel.2016.09.031
Stingele, J., Bellelli, R., and Boulton, S. J. (2017). Mechanisms of DNA–protein crosslink repair. Nat. Rev. Mol. Cell Biol. 18, 563–573. doi: 10.1038/nrm.2017.56
Stingele, J., Habermann, B., and Jentsch, S. (2015). DNA–protein crosslink repair: proteases as DNA repair enzymes. Trends Biochem. Sci. 40, 67–71. doi: 10.1016/j.tibs.2014.10.012
Stingele, J., Schwarz, M. S., Bloemeke, N., Wolf, P. G., and Jentsch, S. (2014). A DNA-dependent protease involved in DNA-Protein crosslink repair. Cell 158, 327–338. doi: 10.1016/j.cell.2014.04.053
Takahashi, K., Morita, T., and Kawazoe, Y. (1985). Mutagenic characteristics of formaldehyde on bacterial systems. Mutat. Res. Genet. Toxicol. 156, 153–161. doi: 10.1016/0165-1218(85)90058-8
Todd, R. C., and Lippard, S. J. (2010). Consequences of cisplatin binding on nucleosome structure and dynamics. Chem. Biol. 17, 1334–1343. doi: 10.1016/j.chembiol.2010.10.018
Torres-Ramos, C. A., Johnson, R. E., Prakash, L., and Prakash, S. (2000). Evidence for the involvement of nucleotide excision repair in the removal of abasic sites in yeast. Mol. Cell. Biol. 20, 3522–3528. doi: 10.1128/MCB.20.10.3522-3528.2000
Trempe, J.-F., Šašková, K. G., Sivá, M., Ratcliffe, C. D. H., Veverka, V., Hoegl, A., et al. (2016). Structural studies of the yeast DNA damage-inducible protein Ddi1 reveal domain architecture of this eukaryotic protein family. Sci. Rep. 6:33671. doi: 10.1038/srep33671
Tretyakova, N. Y., Groehler, A., and Ji, S. (2015). DNA-protein cross-links: formation. structural identities, and biological outcomes. Acc. Chem. Res. 48, 1631–1644. doi: 10.1021/acs.accounts.5b00056
Ustrell, V., Hoffman, L., Pratt, G., and Rechsteiner, M. (2002). PA200, a nuclear proteasome activator involved in DNA repair. EMBO J. 21, 3516–3525. doi: 10.1093/emboj/cdf333
Wickramaratne, S., Ji, S., Mukherjee, S., Su, Y., Pence, M. G., Lior-Hoffmann, L., et al. (2016). Bypass of DNA-protein cross-links conjugated to the 7-Deazaguanine Position of DNA by translesion synthesis polymerases. J. Biol. Chem. 291, 23589–23603. doi: 10.1074/jbc.M116.745257
Woodcock, C. L., and Dimitrov, S. (2001). Higher-order structure of chromatin and chromosomes. Curr. Opin. Genet. Dev. 11, 130–135. doi: 10.1016/s0959-437x(00)00169-6
Woodgate, R. (1999). A plethora of lesion-replicating DNA polymerases. Genes Dev. 13, 2191–2195. doi: 10.1101/gad.13.17.2191
Wu, B., Dröge, P., and Davey, C. A. (2008). Site selectivity of platinum anticancer therapeutics. Nat. Chem. Biol. 4, 110–112. doi: 10.1038/nchembio.2007.58
Xu, X., Muller, J. G., Ye, Y., and Burrows, C. J. (2008). DNA–protein cross-links between guanine and lysine depend on the mechanism of oxidation for formation of C5 Vs C8 guanosine adducts. J. Am. Chem. Soc. 130, 703–709. doi: 10.1021/ja077102a
Yan, Z., Delannoy, M., Ling, C., Daee, D., Osman, F., Muniandy, P. A., et al. (2010). A histone-fold complex and FANCM form a conserved DNA-remodeling complex to maintain genome stability. Mol. Cell 37, 865–878. doi: 10.1016/j.molcel.2010.01.039
Yang, K., and Greenberg, M. M. (2019). DNA–protein cross-link formation in nucleosome core particles treated with methyl methanesulfonate. Chem. Res. Toxicol. 32, 2144–2151. doi: 10.1021/acs.chemrestox.9b00314
Yang, K., Prasse, C., and Greenberg, M. M. (2019). Effect of histone lysine methylation on DNA lesion reactivity in nucleosome core particles. Chem. Res. Toxicol. 32, 910–916. doi: 10.1021/acs.chemrestox.9b00049
Yi, C., and He, C. (2013). DNA repair by reversal of DNA damage. Cold Spring Harb. Perspect. Biol. 5:a012575. doi: 10.1101/cshperspect.a012575
Yip, M. C. J., Bodnar, N. O., and Rapoport, T. A. (2020). Ddi1 is a ubiquitin-dependent protease. PNAS 117, 7776–7781. doi: 10.1073/pnas.1902298117
Yudkina, A. V., Dvornikova, A. P., and Zharkov, D. O. (2018). Variable termination sites of DNA polymerases encountering a DNA–protein cross-link. PLoS One 13:e0198480. doi: 10.1371/journal.pone.0198480
Zecevic, A., Hagan, E., Reynolds, M., Poage, G., Johnston, T., and Zhitkovich, A. (2010). XPA impacts formation but not proteasome-sensitive repair of DNA–protein cross-links induced by chromate. Mutagenesis 25, 381–388. doi: 10.1093/mutage/geq017
Zhang, H., Xiong, Y., and Chen, J. (2020). DNA–protein cross-link repair: what do we know now? Cell Biosci. 10:3. doi: 10.1186/s13578-019-0366-z
Zhang, Y.-W., Regairaz, M., Seiler, J. A., Agama, K. K., Doroshow, J. H., and Pommier, Y. (2011). Poly(ADP-ribose) polymerase and XPF–ERCC1 participate in distinct pathways for the repair of topoisomerase I-induced DNA damage in mammalian cells. Nucleic Acids Res. 39, 3607–3620. doi: 10.1093/nar/gkq1304
Keywords: DNA-histone cross-link, nucleosome core particle, chromatin, genome instability, spartan protease, proteasome, DNA repair
Citation: Pachva MC, Kisselev AF, Matkarimov BT, Saparbaev M and Groisman R (2020) DNA-Histone Cross-Links: Formation and Repair. Front. Cell Dev. Biol. 8:607045. doi: 10.3389/fcell.2020.607045
Received: 16 September 2020; Accepted: 30 November 2020;
Published: 21 December 2020.
Edited by:
Nikita Kuznetsov, Institute of Chemical Biology and Fundamental Medicine (RAS), RussiaReviewed by:
Anna Yudkina, Institute of Chemical Biology and Fundamental Medicine (RAS), RussiaChunying Li, Georgia State University, United States
Copyright © 2020 Pachva, Kisselev, Matkarimov, Saparbaev and Groisman. This is an open-access article distributed under the terms of the Creative Commons Attribution License (CC BY). The use, distribution or reproduction in other forums is permitted, provided the original author(s) and the copyright owner(s) are credited and that the original publication in this journal is cited, in accordance with accepted academic practice. No use, distribution or reproduction is permitted which does not comply with these terms.
*Correspondence: Murat Saparbaev, bXVyYXQuc2FwYXJiYWV2QGd1c3RhdmVyb3Vzc3kuZnI=; Regina Groisman, cmVnaW5hLmdyb2lzbWFuQGd1c3RhdmVyb3Vzc3kuZnI=