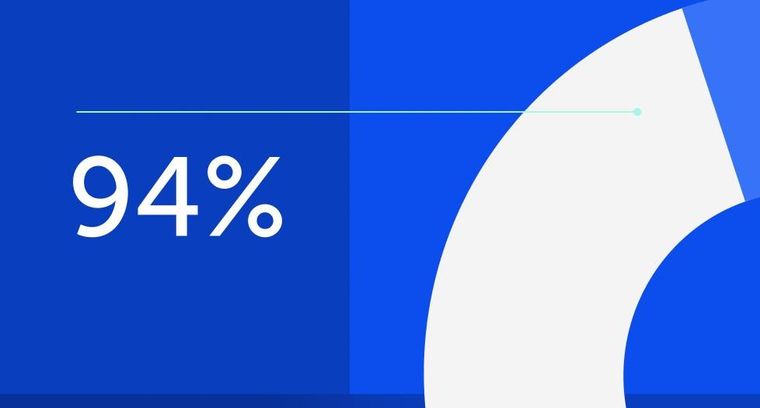
94% of researchers rate our articles as excellent or good
Learn more about the work of our research integrity team to safeguard the quality of each article we publish.
Find out more
REVIEW article
Front. Cell Dev. Biol., 09 December 2020
Sec. Signaling
Volume 8 - 2020 | https://doi.org/10.3389/fcell.2020.605379
This article is part of the Research TopicThe Fertilization Success from the Oocyte's PerspectiveView all 15 articles
Ovulation is a unique physiological phenomenon that is essential for sexual reproduction. It refers to the entire process of ovarian follicle responses to hormonal stimulation resulting in the release of mature fertilization-competent oocytes from the follicles and ovaries. Remarkably, ovulation in different species can be reproduced out-of-body with high fidelity. Moreover, most of the molecular mechanisms and signaling pathways engaged in this process have been delineated using in vitro ovulation models. Here, we provide an overview of the major molecular and cytological events of ovulation observed in frogs, primarily in the African clawed frog Xenopus laevis, using mainly ex vivo approaches, with the focus on meiotic oocyte maturation and follicle rupture. For the purpose of comparison and generalization, we also refer extensively to ovulation in other biological species, most notoriously, in mammals.
Oocytes of most vertebrate species, including frog oocytes, reside and grow in the ovaries while arrested in the first meiotic prophase. At the advanced stages of growth, oocytes resting in ovarian follicles are surrounded by the follicle envelope, consisting of several layers, such as theca/epithelial layer, granulosa cell layer, and vitelline envelope. Direct contacts between the oocyte and somatic cells in the follicle are formed via terminal gap junctions connecting the oocyte and follicle cell plasma membranes (Browne et al., 1979). Communication and paracrine interactions between the oocyte and somatic cells in the follicle were found to be necessary for normal ovulation. The fully grown frog oocytes are not competent for fertilization. They are characterized by a low activity of the main meiotic regulators, the cytostatic factor (CSF) and maturation-promoting factor (MPF) (Masui and Markert, 1971; Smith and Ecker, 1971). MPF was originally defined by Masui and Markert as a cytoplasmic activity from mature oocytes that causes complete maturation upon injection into immature oocytes, and CSF, as a cytoplasmic activity from unfertilized eggs that promotes metaphase arrest upon transfer to early dividing embryos. It was found subsequently that MPF represents a complex of cyclin B and Cdk1 kinase (Hunt, 1989), and CSF was identified as a multicomponent system comprising the meiotic protein kinase Mos and the MAPK pathway (reviewed in Tunquist and Maller, 2003; Schmidt et al., 2006; Wu and Kornbluth, 2008).
The fully grown frog oocytes acquire fertilization competence in the process of meiotic maturation. They progress through the meiotic cell cycle and arrest again before fertilization in the second meiotic metaphase with high activity of CSF and MSF. Hormonal stimuli release the prophase arrest and initiate oocyte maturation when oocytes rest in ovarian follicles. The maturing frog oocytes leave their follicles, advance from the ovary into the open coelomic body cavity, pass through the oviduct, and accumulate, for a short time, in the uterus. Finally, they are deposited outside the body into aquatic environments where fertilization occurs (Figure 1). Thus, the process of meiotic maturation is accompanied or immediately followed by oocyte release from the ovary, and the entire process of follicle responses to hormonal stimulation, which produces mature follicle-free fertilization-competent oocytes, is called “ovulation” (of note, in some studies the term “ovulation” is used narrowly, only for oocyte liberation from the ovarian follicles). Therefore, ovulation engages, as the major events, oocyte maturation and oocyte release from ovarian follicles. In addition, egg oviposition may also be considered as an integral event of ovulation in frogs because most amphibians are oviparous species with external fertilization.
Figure 1. In vivo and in vitro ovulation of frog oocytes. In vivo, favorable environmental factors initiate a hormonal cascade via the hypothalamic-pituitary-gonadal axis, to cause oocyte ovulation resulting in egg spawning. In vitro, oocyte ovulation can be induced in the ovarian fragments or isolated ovarian follicles by pituitary extracts, or luteinizing hormone (LH), or human Chorionic Gonadotropin (hCG), a functional analog of LH, or multiple steroid hormones.
In nature, the environmental factors stimulate production of luteinizing hormone releasing hormone (LHRH), also known as gonadotropin-releasing hormone (GnRH), by the hypothalamus (Figure 1). In response to LHRH, the pituitary produces gonadotropins, such as luteinizing hormone (LH) and follicle stimulating hormone (FSH). The hormones upregulate various genes involved in ovulation and stimulate steroidogenic ovarian follicle cells (granulosa and theca cells), surrounding an oocyte within a follicle, to produce various steroid hormones, prostaglandins (PGs), and multiple proteolytic enzymes (Figure 1). LH activates Gαs protein-coupled LH receptors (LHCGRs) expressed in follicular cells, leading to increased cAMP production and activation of PKA (Simoni et al., 1997; Wood and Strauss, 2002). In addition, LHCGR also activates Gq/11 and stimulates phospholipase C (PLC) and its downstream signaling in granulosa cells (Breen et al., 2013). Thus, LHCGR activation triggers multiple intracellular signal transduction pathways mediated by different signaling molecules. Involvement of PKA, PKC, PI3K, PLC, Src, EGFR, MAPK and Ras in response to LH and gonadotropin has been documented in mammalian follicles (Figure 2) (Jamnongjit and Hammes, 2006; Richards and Ascoli, 2018). In mammals, several transcription factors, such as PGR, PRARG, HIFs, CEBPA, RUNX1, RUNX2, NRIP1, NR5A2, become activated in follicular cells, resulting in the increased production of the enzymes that play important roles in the ovulatory process (Duffy et al., 2019). These enzymes cover the three crucial processes indispensable for successful ovulation, such as steroidogenesis, prostaglandin synthesis, and proteolysis.
Follicular steroid production and steroid-mediated signaling are critical for the initiation of oocyte maturation during the ovulatory process. Early studies in frogs demonstrated that the treatment of isolated amphibian ovarian follicles with frog pituitary homogenate (FPH) increases follicular progesterone (P4) levels, which, in turn, initiate oocyte maturation (Petrino and Schuetz, 1987). It was found using in vitro cultures of Rana pipiens and Rana dybowskii ovarian follicles, that steroid production in ovarian follicle cells is mediated by activation of the cAMP/PKA steroidogenic pathway. A marked increase in intrafollicular P4 levels to those produced by FPH occurred when frog ovarian follicles were exposed to exogenous dibutyryl cAMP and a phosphodiesterase inhibitor IBMX. The observed elevation of follicular levels of P4 caused by FPH administration or cAMP stimulation required the presence of somatic follicular cells (Kwon and Schuetz, 1986). It was concluded, that FPH increases the intracellular level of cAMP by modulating the relative activity of adenylyl cyclase and phosphodiesterase (Kwon and Schuetz, 1986; Kwon et al., 1990). The production of the three main steroids, such as P4, testosterone and estradiol, was reported to occur in vitro in the isolated Xenopus follicles stimulated by gonadotropin hormones (El-Zein et al., 1988; Lutz et al., 2001). Markedly, multiple steroid hormones were found to induce maturation of frog oocytes (Baulieu et al., 1978; Masui and Clarke, 1979). Their contribution into the steroid signaling during maturation is discussed further in the section “Meiotic resumption.”
Similarly to frogs, it was demonstrated that in mammals too the cAMP/PKA signaling cascade is the major pathway regulating steroid biosynthesis. In addition, it was found that, the steroidogenic acute regulatory protein (StAR), highly expressed in both theca and granulosa cells of mammalian follicles, is involved in steroidogenesis. Increased expression of StAR and upregulation of StaR activity via phosphorylation were shown to promote steroidogenesis, whereas inhibition of StAR expression led to a dramatic fall in steroid biosynthesis (Wood and Strauss, 2002; Jamnongjit and Hammes, 2006). This labile mitochondrial phosphoprotein mediates the transfer of cholesterol, the precursor for all steroid hormones, from the outer to inner mitochondrial membrane. This reaction represents the rate-limiting step in steroid hormone biosynthesis. A tight correlation between expression of the StAR protein and steroid synthesis has been reported (Manna et al., 2009). The cAMP/PKA pathway was established as a major signaling pathway for hormone-stimulated StAR expression, and the MAPK signaling cascade was demonstrated to mediate this process (Manna and Stocco, 2011). MAPK activation was found to elevate StAR expression in some steroidogenic cell lines (Gyles et al., 2001). Membrane-permeable cAMP analogs induce MAPK activation in granulosa cells of mammalian follicles, suggesting that gonadotropin-dependent activation of MAPK occurs downstream of cAMP/PKA signaling (Cameron et al., 1996; Das et al., 1996; Su et al., 2002). Notably, in contrast to frogs, synthesis of steroids is sufficient but not ultimately necessary for maturation of mammalian oocytes, indicating that higher vertebrates have developed redundant systems to trigger this process (Lieberman et al., 1976; Yamashita et al., 2003; Jamnongjit and Hammes, 2006).
Simultaneously with the elevation of follicular steroid production, which initiate meiotic oocyte maturation in frogs, LH upregulates a plethora of genes related to follicular rupture and oocyte liberation from the ovarian follicle. It was demonstrated using isolated ovarian fragments of Xenopus laevis that oocyte release from the follicles is a transcription-dependent process; inhibition of transcription with actinomycin D blocked hormone-induced follicle rupture, so that mature oocytes remained entrapped in the follicles (Liu et al., 2005; Sena and Liu, 2008). The major classes of genes related to follicle rupture and induced during ovulation via different transcription factors include the enzymes involved in PG synthesis and multiple ovarian proteases involved in decomposition of the follicle wall. The fact that inhibitors of eicosanoid synthesis can block ovarian collagenolysis and suppress gonadotropin-induced elevation in the content of interstitial collagenase in preovulatory mammalian follicles (Reich et al., 1991) strongly suggests involvement of prostaglandins upstream of follicular rupture. Indeed, prostaglandins were identified as the universal intrafollicular signaling molecules critical for ovulation in different biological species, including frogs and mammals (Schuetz, 1986; Chang et al., 1995; Sena and Liu, 2008; Niringiyumukiza et al., 2018; Takahashi et al., 2018).
Oocyte liberation from the ovary requires physical rupture of the ovarian follicle. This event is highly synchronized with meiotic resumption in oocytes. It was initially found that pre-ovulatory follicles contain a dense meshwork of collagen fibers, suggesting that collagenolysis might be a prerequisite for follicular rupture. Indeed, early morphological studies have revealed a decrease in collagen fiber contents in the apical wall of mammalian follicles prior to ovulation (Espey, 1967; Tsafriri et al., 1990). It was demonstrated that intrabursal injections of broad specificity protease inhibitors inhibited ovulation and degradation of ovarian collagen in a dose-dependent fashion, and that intrafollicular injection of metalloproteinase inhibitors completely blocked ovulation (Reich et al., 1985; Peluffo et al., 2011). Subsequently, a number of in vivo and in vitro studies have demonstrated that various follicular metalloproteinases and serine proteases are upregulated during ovulation (Figure 2). Synergetic action of multiple proteases with different substrate specificities was found to be necessary for effective degradation of the follicular wall. Involvement of proteolytic enzymes in follicle rupture has been well established in mammalian, fly and fish species, however little is known about the molecular mechanisms of follicle rupture in frogs.
Following follicle rupture, liberated frog oocytes migrate from the ovary into the oviduct, where they complete maturation and acquire a jelly layer that is necessary for successful fertilization (Figure 1). In addition, the vitelline membrane surrounding the oocytes is crippled by proteolytic enzymes within the first part of the oviduct, making this membrane penetrable to sperm at the time of fertilization (Heasman et al., 1991). It was demonstrated that frog eggs have to pass through the oviduct in order to become fertilizable (Diakow et al., 1988). Three distinct jelly coats are found in Xenopus laevis and as many as six in Rana pipiens (Freeman, 1968; Bonnell and Chandler, 1996). The jelly coats are synthesized in the oviduct and deposited sequentially on the egg during its travel through the oviduct (Bakos et al., 1990). Constituents of the egg jelly layers are essential for egg fertilization by incoming sperm. Eggs which are stripped of their jelly layers could not be fertilized, but the addition of solubilized jelly was found to partially restore fertilization capacity (Olson and Chandler, 1999). Finally, an oxytocin family peptide hormone (mesotocin in frogs), which is released by the posterior pituitary, contributes at the stage of oviposition (Schmidt, 1984; Browne and Figiel, 2019; Figure 1). In frogs, the hormone is involved in the physical process of spawning and mating behavior. Oxytocin family hormones have uterus-contracting (uterotonic) activity that causes muscle contractions via a G protein- and calcium-mediated pathway and promotes oviposition (Jurek and Neumann, 2018). It was found in monkey and cow that follicular granulosa cells produce oxytocin in response to pre-ovulatory upsurge in LH (Voss and Fortune, 1991; Einspanier et al., 1997).
In the wild, favorable environmental factors, such as balmy temperature, ample rainfall, protracted daytime, presence of males, etc., initiate oocyte ovulation in frogs via a hormonal cascade involving the hypothalamus, the pituitary, and ovarian follicle cells, as described in detail in the previous section (Figure 1). Normally, during the reproductive season, egg deposition and fertilization occur within several hours of hormonal stimulation. However, some eggs can be retained in the frog genital tract for much longer time and degrade there by an apoptotic process (Iguchi et al., 2013; Tokmakov et al., 2018). It was observed that aging frogs retained a larger number of ovulated eggs for a longer period than the young animals (Iguchi et al., 2013). It was also reported that decreasing temperatures could cause retention of mature eggs in the uterus for several days (Witschi, 1952). Thus, seasonal, environmental, and individual-specific factors make it difficult to control ovulation of frog oocytes in vivo. Of note, the same factors were also demonstrated to control the ovulatory process in different fish species, most notably, teleost fishes that have external fertilization similarly to frogs (Munakata and Kobayashi, 2010).
To facilitate dissection of the ovulatory process, various in vitro growth and ovulation models have been developed in different animals. Most of them involve the mammalian species, such as rodents, cows, pigs, horses, primates and even humans, where the studies would have apparent applications for assisted reproduction (Picton et al., 2003; Gerard and Robin, 2019; Telfer, 2019; Telfer et al., 2019). The development of technologies to grow and mature oocytes is very attractive for research, animal production technology and clinical practice. The ultimate goal of this approach is to grow, mature, ovulate and fertilize oocytes in vitro with the following production of viable embryos. The in vitro reproductive technologies were very successful in mammals. For instance, it was reported that the whole ovarian cell cycle can be reconstructed in vitro using the follicle culture of murine and human follicles (Skory et al., 2015). In vitro systems that support full development of murine oocytes starting from primordial germ cells or from induced pluripotent cells have been recently described (Hikabe et al., 2016; Morohaku et al., 2016). However, in frogs, it has proved difficult to grow early stage follicles to maturity in vitro. In fact, no report has been provided so far about the successful in vitro growth of frog oocytes. However, in vitro maturation and/or ovulation of frog oocytes have been consistently observed in the ovarian fragments and isolated fully grown follicles treated with homologous pituitary extracts (Heilbrunn et al., 1939; Wright, 1945; Wright, 1961; Dettlaff et al., 1964). Also, LH and human Chorionic Gonadotropin (hCG), the hormones that activate the same heptahelical Luteinizing Hormone/Choriogonadotropin Receptor (LHCGR), as well as various steroid hormones were found to be effective in inducing in vitro maturation of frog oocytes in ovarian fragments (Wright, 1961; Subtelny et al., 1968; Figure 1). Furthermore, it was reported that a number of streroids could induce GVBD in isolated Rana pipiens follicles even though follicle rupture did not occur (Schuetz, 1967). In addition, it was found that physiological meiotic maturation can be induced in vitro with some steroid hormones in Rana pipiens oocytes removed from their ovarian follicles. It was noted that the hormones work equally well with ovarian fragments or with isolated follicles (Smith et al., 1968). The early studies of in vitro ovulation and maturation in frogs were summarized and extensively reviewed by Smith and Ecker (1970).
Of note, frogs of the genus Rana, such as Rana pipiens, Rana dybowskii and Rana japonica, had been used most often during the early investigations of frog oocyte maturation. However, more recently, highly aquatic frogs of the Xenopus genus, such as Xenopus laevis and Xenopus tropicalis, have been employed as the main biological models in oocyte maturation and ovulation studies, focusing on signaling pathways and molecular machinery of these processes. In fact, the Xenopus pregnancy test, also called the Hogben test, based on the experiments suggested by Hogben and successively carried out by his students and co-workers in the 1930s (Shapiro and Zwarenstein, 1934), was used around the world for about three decades, until immunological test kits replaced it in the 1960s. In the test, female frogs were injected with the woman’s urine, and if the frogs had ovulated due to a high level of hCG in the urine, that meant the woman who provided the urine was pregnant.
Importantly, in vitro matured and ovulated frog eggs still cannot be fertilized efficiently because they are not penetrable to sperm. These eggs have the intact vitelline membrane, which is naturally degraded during physiological ovulation by proteolytic enzymes within the first part of the oviduct. In addition, in vitro ovulated frog eggs miss the jelly layer synthesized in the oviduct and deposited sequentially on the egg during its travel through the oviduct (Figure 1). Two general approaches have been developed that allow successful fertilization of in vitro matured frog eggs. One of them involves transferring investigated eggs into the body cavity of a host female frog, to let them pass through the frog’s oviducts (Lavin, 1964; Brun, 1975). Evidently, this approach represents a hybrid in vitro/in vivo technique, and it was extensively used in the studies of maternally inherited molecules by specifically removing or overexpressing particular mRNAs and proteins. For instance, the host-transfer technique was successfully used by the group of Janet Heasman to establish the essential role of Wnt signaling in Xenopus axis formation (Zuck et al., 1998; Mir and Heasman, 2008). Another approach to obtain in vitro matured fertilizable frog eggs involves enzymatic and/or manual removal of the vitelline membrane. Complete removal of the vitelline membrane can be carried out by combined treatment of eggs with pepsin and cysteine followed by manual removal of the loosened membrane (Kloc et al., 1989; Heasman et al., 1991). Extracts of diffusible components of Xenopus eggs jelly layers prepared by incubation of freshly ovulated eggs in high-salt buffers were shown to promote sperm’s ability to fertilize dejellied eggs in a dose-dependent manner (Olson and Chandler, 1999). It was reported that removal of the vitelline membrane and addition of the conditioned medium containing solubilized jelly obtained by soaking jellied eggs in buffer and Ficoll can vastly boost success rates of fertilization (Heasman et al., 1991; Olson and Chandler, 1999). For example, injection of oligonucleotides into isolated oocytes, followed by their in vitro maturation and fertilization using the abovementioned approach allowed to investigate the role played by xlgv7 mRNA in early development of the Xenopus embryo (Kloc et al., 1989).
Frog oocytes have been extensively used to study maturation and meiotic progression. In fact, most of the control mechanisms that operate in meiosis, including MPF and CSF, were first established in frogs. A number of key signaling molecules, such as membrane receptors, protein kinases, protein phosphatases, their substrates, inhibitors and activators, adaptor proteins, etc., have been characterized. Many of these studies were carried out in vitro, using isolated fully grown Xenopus oocytes treated with gonadotropins or steroid hormones (Figure 1). Especially, denuded frog oocytes, i.e., oocytes devoid of the follicle layer, proved to be very helpful in the maturation studies. Defolliculation could be accomplished by either enzymatic treatment or by manual dissection of oocytes from their ovarian follicles (Masui, 1967; Smith and Ecker, 1969). Also, the techniques of oocyte denucleation, as well as cytoplasmic and nuclear transfer (Dettlaff et al., 1964; Smith and Ecker, 1970; Masui and Markert, 1971), contributed greatly to the studies of molecular mechanisms of hormonal action, identification of MPF and CSF, and establishing their role in meiotic maturation.
Before maturation, fully grown Xenopus oocytes of the stage VI are naturally arrested at the diplotene stage of the first meiotic prophase at the G2/M boundary. They have an intact nuclear envelope, partially decondensed chromatin, high activity of transcription, high level of intracellular cAMP, and low activity of MPF and CSF. Several studies have implicated a constitutively activated G protein-coupled receptor 3, GPR3, as one of the key molecules responsible for elevated intracellular cAMP and maintaining meiotic prophase arrest in frog and mammalian oocytes (Mehlmann et al., 2004; Hinckley et al., 2005; Deng et al., 2008; Rios-Cardona et al., 2008). Both Gαs and Gβγ - mediated signaling were found to be involved in maintaining elevated cAMP levels and diplotene arrest in immature oocytes (Gallo et al., 1995; Lutz et al., 2000; Freudzon et al., 2005; Sheng et al., 2005). More recent studies in mammals demonstrated that cGMP too is a key regulator of meiotic transition in mammalian follicular oocytes (Jaffe and Egbert, 2017). It was found that mural granulosa cells of mammalian follicles contain a high content of cGMP in a quiescent stage due to the high expression levels of the transmembrane guanylyl cyclase natriuretic peptide receptor 2 (NPR2) and its ligand the natriuretic peptide C (NPPC) (Vaccari et al., 2009; Zhang et al., 2010). cGMP was found to diffuse from the somatic compartment into oocytes through gap junctions and inhibit phosphodiesterase 3A (PDE3A) to maintain a high level of cAMP and meiotic arrest in the oocytes (Norris et al., 2009; Vaccari et al., 2009). It should be noted that involvement of NPPC-NRP2 autocrine regulatory mechanism in frog ovulation has not been demonstrated yet.
In prophase-arrested oocytes, the major bulk of Cdk1, a key component of MPF, is present in a free monomeric and low-activity form, and some part of the kinase is stored as an inactive Cdk1/Cyclin B complex, called pre-MPF. The inhibitory kinase Myt1, which phosphorylate Cdk1 on Thr14 and Tyr15, plays a major role in maintaining pre-MPF in the inactive state (Nakajo et al., 2000). In addition, Chk1 kinase, contributes to maintaining low activity of MPF in immature oocytes. The kinase inhibits the MPF-activating phosphatase Cdc25C through direct phosphorylation on Ser28 (Nakajo et al., 1999; Figure 3). Markedly, a direct link between PKA activated by a high level of intraoocyte cAMP and Cdc25 has been reported, indicating that PKA phosphorylates and inactivates Cdc25 in frog oocytes (Duckworth et al., 2002; Shibuya, 2003). Besides the low activity of MPF, the lack of active CSF is also crucial for maintaining prophase arrest. Low activity of CSF, and specifically of the MAPK cascade, in immature prophase-arrested oocytes is ensured by the absence of Mos protein (Figure 3) that is synthesized from maternal mRNA later during maturation. Notably, CSF and MPF are embedded in a loop of positive feedback, so they can mutually affect each other’s activity (Ferrell, 2002). Low activity of the two key meiotic regulators and the existence of positive feedback between them secure stability of the prophase I arrest in immature oocytes.
Figure 3. Signaling pathways of Xenopus oocyte maturation. Molecular components of CSF and MPF are colored green and orange, correspondingly. The components of MPF auto-amplification loops mediated by polo-like kinase (Plx1) and Greatwall kinase (Gwl) are presented in light blue, and the factors involved in the initiation of egg maturation are shown in gray. Detailed explanations are provided in the text (see section “Maturation”).
Stimulation of isolated Xenopus follicles by gonadotropins was reported to increase production and release of several steroids, such as P4, testosterone, and estradiol, by steroidogenic follicle cells (Fortune and Tsang, 1981; Fortune, 1983; El-Zein et al., 1988). Importantly, either P4 or testosterone, but not estradiol and other estrogens, were shown to be able to induce in vitro maturation of frog oocytes. P4 was suggested to play a major role in triggering maturation of frog oocytes because its intra-oocyte concentration increases robustly to micromolar levels during meiosis re-entry (Haccard et al., 2012), and because it can induce meiotic maturation of isolated denuded frog oocytes at submicromolar and low micromolar concentrations (Jacobelli et al., 1974; Reynhout et al., 1975). It was noted that induction of maturation by exogenous P4 is significantly faster and more efficient in defolliculated oocytes than in the follicles, suggesting that follicle layer may not be well permeable for the hormone (Haccard et al., 2012; Tokmakov et al., 2019). In addition, evidence has been presented that androgens, rather than progestogens, might be the bona fide effectors that promote maturation of Xenopus oocytes via classical androgen receptor (Lutz et al., 2001; Hammes, 2004; Evaul et al., 2007; Sen et al., 2011). Testosterone was identified as the main steroid produced quantitatively in response to LH and capable of inducing in vitro maturation as efficiently as P4 (Baulieu et al., 1978; Fortune and Tsang, 1981; El-Zein et al., 1988; Lutz et al., 2001). It was also suggested that both types of steroids can be involved in meiotic maturation of Xenopus oocytes, considering high intra-oocyte levels of CYP17, an enzyme converting progestogens to androgens (Yang et al., 2003; Deng et al., 2009).
One of the earliest responses of oocytes to P4 is a decrease in the intracellular level of cAMP due to inhibition of plasma membrane adenylate cyclase (AC) (Schorderet-Slatkine et al., 1978; Finidori-Lepicard et al., 1981). The reduction of cAMP concentration and subsequent decline in PKA activity are crucial for initiating maturation. Microinjections of PKA catalytic subunit block P4-induced maturation, and injecting PKA inhibitors induce oocyte maturation in the absence of P4 (Maller and Krebs, 1977; Huchon et al., 1981). The finding that P4 can initiate oocyte maturation only when applied externally but not when microinjected into the cytoplasm or the nucleus, and the fact that MPF activation is observed in enucleated oocytes (Masui and Markert, 1971; Smith and Ecker, 1971) indicated that P4 receptor (PGR) is not a conventional nuclear steroid receptor but rather a non-transcriptional membrane-associated signaling receptor. Multiple studies suggest that inhibition of AC is mediated by the G-protein coupled transmembrane receptor GPR3 via a “release of inhibition” mechanism. It was shown that overexpression of GPR3 suppressed steroid-induced maturation of isolated Xenopus oocytes and gonadotropin-induced maturation of follicle-enclosed oocytes, whereas depletion of GPR3 lowered intracellular cAMP levels and enhanced oocyte maturation. hCG treatment of isolated Xenopus ovarian follicles triggers metalloproteinase-mediated cleavage and inactivation of GPR3 (Deng et al., 2008, 2009). Also, it was found that a fraction of the classical PGR displays membrane localization (Martinez et al., 2007) and complexes with Gβγ subunits via the scaffold protein called the modulator of non-genomic steroid responses (NMAR), which may mediate steroid-triggered oocyte maturation (Haas et al., 2005). Furthermore, evidence has been presented that a G protein-coupled membrane progestin receptor XmPRβ expressed on the oocyte plasma membrane may be a physiological PGR involved in initiating the resumption of meiosis during maturation of Xenopus oocytes (Zhu et al., 2003; Josefsberg Ben-Yehoshua et al., 2007). Also, it was found that the classical androgen receptor (AR) is expressed in Xenopus oocytes. It can bind both androgens and P4 with high affinity. A minor fraction of the receptor is membrane-localized and suggested to mediate non-genomic effects of various steroid during ovulation (Lutz et al., 2001, 2003; Evaul et al., 2007).
In mammals, it was reported that the fastest effect of LHCGR activation observed in vitro is a decrease in cGMP in somatic follicular cells. This leads to cGMP diffusion out of oocytes and releases PDE3A inhibition, resulting in a decrease of intra-oocyte cAMP content and triggering oocyte maturation (Norris et al., 2009; Vaccari et al., 2009; Shuhaibar et al., 2015). Another early effect of LH that occurs within minutes in cultured mouse follicles is the closure of gap junctions. This is thought to be necessary for meiotic resumption because it restrains intercellular communications and ensures autonomous mode of oocyte maturation. It was demonstrated in mammals that EGFR and the MAPK pathway participate in gap junction closure (Prochazka and Blaha, 2015).
The synthesis of several proteins from maternal mRNA is required for MPF activation during progesterone-induced oocyte maturation. It was found that protein synthesis is triggered by cytoplasmic polyadenylation of maternal mRNAs mediated by the cytoplasmic polyadenylation element (CPE) present in their 3′ UTRs (Stebbins-Boaz et al., 1996; Mendez et al., 2000; Belloc and Méndez, 2008). A recent genome-wide analysis of the Xenopus oocyte transcriptome indicated that a family of U-rich sequence elements was enriched near the polyadenylation signal of transcriptionally activated mRNAs (Yang et al., 2020). The main newly synthesized proteins include cyclins, RINGO and Mos. Site-specific phosphorylation of CPEB catalyzed by Eg2, a member of the Aurora family protein kinases, was shown to be essential for the polyadenylation of c-mos mRNA (Andresson and Ruderman, 1998; Mendez et al., 2000). Cyclins and RINGO directly bind and activate Cdk1 kinase, whereas Mos induces MPF activation by activating the MAPK cascade (Figure 3). Notably, inhibition of protein synthesis prevents MPF activation induced by PKA inhibitors, that places protein synthesis downstream of PKA and upstream of MPF. The molecular link between PKA inactivation and de novo protein synthesis remains elusive. Although Mos synthesis starts soon after progesterone stimulation, the amount of Mos protein remains low until pre-GVBD, when it is stabilized by direct phosphorylation on Ser3 by activated MAPK (Matten et al., 1996). Several enzymes of protein phosphorylation, which represent two major signaling pathways, become prominently activated at that time, leading to a dramatic shift in the equilibrium between inactive pre-MPF and active MPF. A balance between the dual-specific Cdk1-activating protein phosphatase Cdc25C and Cdk1-inactivating protein kinases Myt1 and Wee1 controls this equilibrium through the phosphorylation state of the regulatory residues Thr14 and Tyr15 in Cdk1. Markedly, CSF activation precedes MPF activation during progesterone-induced oocyte maturation, suggesting that the activated MAPK pathway operates early in maturation. MAPK response to progesterone or Mos in living oocytes displays a feature of ultrasensitivity, corresponding to the extraordinarily high Hill coefficient of at least 35 (Ferrell and Machleder, 1998). The MAPK cascade, including Mos, MAPKK and MAPK, responds little to a weak stimulus and becomes robustly activated over a narrow range of stimulus concentration, resulting in a sigmoid stimulus-response curve, resembling that of a cooperative enzyme. This feature helps to maintain the prophase diplotene arrest in immature oocytes and ensures highly efficient all-or-none physiological response observed upon oocyte maturation (Ferrell, 1999).
Cytostatic factor activity first appears in progesterone-treated oocytes at the time of Mos accumulation. Activated MAPK phosphorylates and activates the downstream Ser/Thr-specific kinase Rsk, which downregulates Cdk1 inhibitory kinase Myt1, promoting MPF activation (Mueller et al., 1995b; Palmer et al., 1998). Mos was also shown to target Myt1 independently of MAPK (Peter et al., 2002). Another Cdk1-inactivating kinase, Wee1, is absent from immature oocytes and is synthesized later during maturation (Nakajo et al., 2000). In addition, activated MAPK promotes phosphorylation and activation of Plx1 kinase and Cdc25 phosphatase in the polo-like kinase pathway mediated by xPlkk1 and Plx1 protein kinases (Kumagai and Dunphy, 1996; Qian et al., 1998b). It was found that Plx1 is activated during oocyte maturation simultaneously with Cdc25 (Qian et al., 1998a). Once activated above a threshold level, MPF accumulates rapidly even in the absence of protein synthesis by an autocatalytic mechanism that involves several positive feedback loops (Figure 2). First, in the polo-like kinase Cdk1-activating pathway, Plx1 and Cdc25C can be directly phosphorylated and upregulated by Cdk1 (Abrieu et al., 1998). Second, active MPF was shown to increase Mos stability by direct phosphorylation on Ser3 (Castro et al., 2001). Third, MPF activates the MAPK cascade by upregulating Mos synthesis, probably, by increasing polyadenylation of maternal Mos RNA (Paris et al., 1991). Of note, activated MAPK is also capable of stimulating polyadenylation of Mos mRNA (Howard et al., 1999). It was found that the adaptor protein paxillin in cooperation with embryonic PolyAdenylation Binding Protein (ePABP) enhance, in an MAPK-dependent fashion, Mos translation and promote oocyte maturation (Miedlich et al., 2017). Thus, numerous interlocking positive feedback loops contribute to MPF amplification and stabilization in maturing Xenopus oocytes.
Soon after the complete MPF activation, dissolution of the oocyte nuclear membrane, or GVBD, occurs that can be easily detected by appearance of a white spot on the oocyte’s pigmented animal hemisphere. Breakdown of the nuclear envelope, a hallmark of M-phase entry, is triggered by Cdk1-mediated phosphorylation of nuclear lamins and other components of the nuclear envelope, resulting in their disassembly (Marshall and Wilson, 1997). Besides, MAPK is involved in M-phase chromosome condensation and cytoskeleton reorganization. Active MAPK localizes to spindle and centrosomes and induces interphase-metaphase transition of microtubule arrays (Gotoh et al., 1991; Verlhac et al., 1993). There is no real M-phase exit between the two meiotic divisions in Xenopus oocytes; interphase nuclei are not formed, and chromatin remains condensed. This prevents DNA replication between meiosis I and meiosis II and leads to generation of haploid gametes by the end of meiosis. The active MAPK cascade plays a key role in the meiotic suppression of DNA replication because ablation of endogenous Mos or pharmacological inhibition of MAPK activity in maturing oocytes allow premature DNA replication after meiosis I (Furuno et al., 1994; Gross et al., 2000). Although Cdk1 activity decreases after meiosis I due to a partial degradation of cyclin B, this decrease is incomplete and transient (Iwabuchi et al., 2000). The remaining activity of Cdk1 is required for normal meiotic transition, and inhibition of MPF assembly brings about premature DNA replication after the first meiotic M-phase (Picard et al., 1996; Iwabuchi et al., 2000). The activated MAPK cascade inhibits cyclin B degradation after MI and helps to maintain MPF activity between the two meiotic divisions by suppressing the ubiquitin-dependent anaphase-promoting complex (APC/C) via Rsk (Gross et al., 2000; Figure 3).
Maturing frog oocytes enter meiosis II with high activity of MPF and CSF and arrest again in the metaphase of the second meiotic division. These completely matured Xenopus oocytes, conventionally called “eggs” in frog and some other species, remain arrested at metaphase II by the high activity of MPF and CSF. Multiple intracellular signaling pathways assure stability of the meiotic metaphase arrest.
Cyclin degradation is greatly inhibited in the mature oocytes (Murray et al., 1989; Sagata et al., 1989). In the activated MAPK pathway, the MAPK downstream target, Rsk, directly phosphorylates and activates the inhibitors of the APC/C ubiquitin ligase, Emi2/Erp1 and Bub1, controlling cyclin B degradation (Schwab et al., 2001; Inoue et al., 2007; Nishiyama et al., 2007; Figure 3). Although the Emi2/Erp1 protein is absent from immature prophase-arrested oocytes, it accumulates in mature metaphase-arrested eggs, due to cytoplasmic polyadenylation and translational unmasking of its mRNA (Tung et al., 2007). The phosphorylated inhibitor proteins suppress ligase activity of the APC/C ubiquitin ligase by sequestering the Cdc20 activator subunit of APC/C (Shoji et al., 2006). The Cdk1-inactivating kinase Myt1 is kept inhibited by high activity of the MAPK pathway, and the Wee1 kinase is also suppressed through a phosphorylation-dependent mechanism in the metaphase-arrested eggs (Mueller et al., 1995a,b; Palmer et al., 1998). On the other hand, active MPF upregulates Mos synthesis via polyadenylation of mos mRNA (Howard et al., 1999) and increases stability of the Mos protein by direct phosphorylation on Ser3 (Castro et al., 2001).
Furthermore, both Cdk1 and MAPK activate the polo-like kinase pathway related to upregulation of the MPF-activating phosphatase Cdc25C (Kumagai and Dunphy, 1996; Qian et al., 1998b; Figure 3). In addition, the major anti-Cdk1 phosphatase PP2A-B55, which dephosphorylates Cdk1-phosphorylated substrates, is suppressed via the Greatwall kinase (Gwl) activated downstream of Cdk1 (Yu et al., 2006; Castilho et al., 2009; Vigneron et al., 2009; Figure 3). Activated Gwl phosphorylates the inhibitor proteins of PP2A-B55, such as ARPP-19 and/or α-endosulfine, which then directly interact with the phosphatase (Gharbi-Ayachi et al., 2010; Mochida et al., 2010). It was found that the Gwl/ARP19/PP2A-B55 pathway acts as a major component of MPF auto-amplification, that contributes to upregulation of MPF activity (Hara et al., 2012; Dupre et al., 2013). Notably, transcriptome-wide analysis demonstrated that massive deadenylation and degradation of mRNA occurs in Xenopus eggs at meiosis II (Meneau et al., 2020; Yang et al., 2020). It was found that a significant fraction of egg transcripts contained, in addition to CPEs, (A + U)-rich elements (ARE), characteristic of the mRNAs regulated by deadenylation, and suggested that opposing activities of CPEs and AREs define the fate of egg mRNAs (Belloc and Méndez, 2008).
Thus, after completion of ovulation, frog eggs are matured and arrested at metaphase II. Multiple mechanisms stabilize the meiotic metaphase arrest. The arrest allows eggs to await fertilization, preventing parthenogenesis after completion of meiosis. It was reported that unfertilized Xenopus eggs spontaneously exit the metaphase arrest and degrade by a well-defined apoptotic process within 48–72 h after completion of ovulation (Du Pasquier et al., 2011; Tokmakov et al., 2011).
Liberation of oocytes from ovarian follicles due to follicular rupture is one of the main and indispensable events of ovulation. It was found, using the frog model, that in contrast to hormone-mediated maturation, which is non-genomic, i.e., transcription-independent, de novo transcription seems to be necessary for follicular rupture (Bayaa et al., 2000; Lutz et al., 2003; Liu et al., 2005). It was demonstrated in different animals that follicular rupture is synchronized with maturation and it occurs within several hours after meiotic resumption (reviewed in Richards and Ascoli, 2018). The following section of our review focuses on the mechanisms of follicular rupture. The process has been investigated in different species, most thoroughly in mammals, with a help of various in vitro and in vivo growth and ovulation models and in vitro follicle cultures capable of reproducing main events of the ovarian cell cycle.
Three principal theories on the mechanism of follicular rupture were under consideration since long ago, including the smooth muscle theory, the intra-follicular pressure theory, and the proteolytic enzyme theory (Rondell, 1970; Espey, 1974). The involvement of protease activity in ovulation was initially suggested by Schochet (1916). Afterward, the proteolytic mechanism has been proved to play a predominant role in follicular rupture based on numerous experimental observations, including degradation of extracellular matrix at the follicular apex, LH or hCG-induced up-regulation of protease activity, and impeding oocyte release from ovarian follicles with protease inhibitors.
The role of proteolytic activity in follicular rupture was corroborated in mammals. It was clearly demonstrated that collagenous layers at the follicular apex become loosened and degrade before follicle rupture (Espey, 1967). Correspondingly, subsequent biochemical analyses revealed a preovulatory increase in ovarian collagenolysis in vivo and an increase of collagenase activity in vitro (Tsafriri et al., 1990; Reich et al., 1991; Murdoch and McCormick, 1992). It was found that multiple metalloproteinases and serine proteases are involved in decomposition of the follicle wall during ovulation. Specifically, members of the matrix metalloproteinase (MMP) family, including collagenases, gelatinases, stromelysins and membrane-type MMPs, tissue inhibitors of metalloproteinases (TIMPs), plasmin/plasminogen activator (PA) system, and the ADAM/ADAMTS family were implicated in follicle rupture during ovulation in mammals (reviewed by Duffy et al., 2019). Gonadotropins, such as LH and hCG, were demonstrated to induce both expression and activity of MMP and ADAMTS in the ovarian follicles. In monkeys and humans, upregulation of collagenases (MMP1), gelatinases (MMP2 and MMP9), stromelysins (MMP7 and MMP10), ADAMTSs (ADAMTS1, ADAMTS4, ADAMTS9, ADAMTS15), as well as the expression of TIMPs, such as TIMP1 and TIMP2, were shown to be induced by LH/hCG (Chaffin and Stouffer, 1999; Curry and Osteen, 2003; Peluffo et al., 2011). Several studies have proposed that the PA system also plays a key role in the degradation of the follicular wall during ovulation in mammals (Ny et al., 2002; Liu et al., 2004; Ohnishi et al., 2005). Plasminogen activators of the two types, urokinase type (PLAU) and tissue type (PLAT), are thought to be involved in the ovulatory process (Tsafriri et al., 1990; Blasi, 1993; Ebisch et al., 2008). In addition, the specific inhibitors of PA system, such as SERPINE3, SERPINB2, SERPINA5 and nexin I, are also induced in the ovary during ovulation (Aertgeerts et al., 1994; Ebisch et al., 2008). It was noted that proteolytic enzymes involved in follicular rupture are largely redundant; their substrate specificities are greatly overlapping, and no single proteolytic enzyme seems to be indispensable for this process (Duffy et al., 2019).
The primary targets of LH and gonadropin are the follicle cells expressing LHCGR, such as granulosa and theca cells, which surround an oocyte within a follicle. Multiple intracellular signal transduction pathways are triggered in mammalian follicle cells by activation of LHCGR (Figure 2, see section “Overview of Ovulation”). The signaling pathways involved in the activation of proteolytic enzymes during ovulation are not clearly understood. It was found that activation of the MAPK pathway in granulosa cells early in the ovulatory process is absolutely required for both oocyte maturation and follicle rupture, as demonstrated by the infertility phenotype of mice with ERK1 and ERK2 knockout in granulosa cells. The ERK1/2 knockout leads to a complete failure to ovulate and synthesize P4 (Fan et al., 2009). ERK activation in granulosa cells was shown to occur due to the release of tonic inhibition imposed by DUSP6 phosphotyrosine phosphatase on MEK1 (Law et al., 2017). It appears that ERK activation promotes steroidogenesis primarily via the StAR. Accordingly, ERK activation was shown to increase StAR expression in some steroidogenic cell lines (Gyles et al., 2001). Several downstream targets of ERK1/2 were found to be essential for follicular rupture. Especially, induction of the two transcription factors, CCAAT enhancer–binding protein (CEBP) α and β, that are highly important for ovulation, has been shown to depend on the MAPK pathway (Fan et al., 2009). Similarly to the ERK1/2 knockout, the combination of the granulosa-specific CEBPaα and CEBPβ knockouts leads to a complete failure to ovulate (Fan et al., 2011).
Another important signaling molecule that is engaged downstream of the LH receptor is the EGF receptor (EGFR). Several studies demonstrated that EGFR signaling can promote steroidogenesis in gonadal cells, including Leydig cells and ovarian follicles, by upregulating StAR (Manna et al., 2002; Jamnongjit and Hammes, 2006). Activation of EGFR seems to be necessary for gonadotropin-induced steroid production in preovulatory follicles, as inhibition of the EGFR protein kinase activity completely abolishes LH-triggered production of P4 (Jamnongjit et al., 2005). In addition, inhibition of EGFR kinase activity significantly suppresses hCG-induced meiotic maturation and follicle rupture, so that oocytes remain arrested at the diplotene stage of the first meiotic prophase in intact ovarian follicles (Ashkenazi et al., 2005). It appears that EGFR activation during ovulation is mediated by the MAPK pathway. In mammalian follicles, activated ERK1/2 mediate transcriptional induction of the EGF–like ligands, such as amphiregulin, epiregulin, and betacellulin, in granulosa cells (Park et al., 2004). These factors function as autocrine and paracrine activators of EGFR on theca and granulosa cells, promoting increased StAR activity, presumably via phosphorylation, and subsequent steroid production. Remarkably, incubation of follicles with the EGF-like ligands evokes the morphological and biochemical events of ovulation, such as oocyte maturation and cumulus expansion (Park et al., 2004).
Progesterone receptor, a nuclear receptor transcription factor, also plays an important role in follicular rupture. It is robustly induced in mural granulosa cells via protein kinase A/cAMP response element-binding protein (CREB)-mediated transactivation and MAPK pathway activation after the LH surge (Park and Mayo, 1991). The key role of PGR in follicular rupture was confirmed in rodents, monkeys, and humans, where inhibition of P4 synthesis or its activity prevented ovulation (Espey et al., 1990; Lydon et al., 1995; Hibbert et al., 1996; Bishop et al., 2016). The precise mechanism by which PGR promotes follicle rupture is unknown, however there is mounting evidence for a link between P4 and upregulation of MMPs and other proteases (Robker et al., 2000; Chaffin and Stouffer, 2002). During ovulation, PGR is acutely activated in the presence of high local concentrations of P4 and translocated into the nucleus, where it initiates transcription of the proteins, such as ADAMTS-1 and cathepsin L, essential for follicular rupture. Knockout of PGR gene results in a complete and specific block of ovulation, however, cumulus expansion and oocyte meiotic maturation occur normally, and oocytes extracted from the knockout follicles can be fertilized (Robker et al., 2000), suggesting a specific role of PGR in mediating follicle rupture. Accordingly, pharmacological inhibition of PGR was reported to block ovulation (Jesam et al., 2016; Schutt et al., 2018). These findings are consistent with the idea that P4 is required for follicular rupture in mammalian species.
Several findings suggested involvement of prostaglandins (PGs) upstream of follicular rupture. It was demonstrated that inhibitors of eicosanoid synthesis could block ovulation and the LH/hCG-induced rise in ovarian collagenolysis (Reich et al., 1991). Synthesis of prostaglandin precursors during ovulation is governed by cyclooxygenase 2 (COX2), also known as prostaglandin synthase 2 (PTGS2). The enzyme is strongly induced in rat, primate and human preovulatory follicles after the LH surge (Sirois et al., 1992, 2004; Stouffer et al., 2007). It was shown that, COX2 knockout mice, which produce no PGs, have reduced rates of ovulation, defected cumulus expansion, and infertility. Ovulation can be restored in the COX2-deficient mice by prostaglandin E2 (PGE2) (Lim et al., 1997; Davis et al., 1999). The inhibitors of COX2 activity, such as meloxicam, also prevent ovulation, suggesting their use as emergency contraceptives (McCann et al., 2013). Although the predominant prostaglandin produced by granulosa cells is PGE2, some other PGs, such as PGF2α, have been shown to mediate certain aspects of follicular rupture (Robker et al., 2018).
Recent studies demonstrated that vasoconstriction at the follicle apex plays an essential role in mammalian follicle rupture. Vasoconstriction of apical vessels was shown to occur within several hours preceding follicle rupture in wild-type mice, and the ovulatory follicles from the mice with defective vasoconstriction failed to rupture (Migone et al., 2016). It was found that endothelin-2 (Edn2), a short vasoconstrictor peptide that is transiently produced by granulose cells before follicle rupture (Palanisamy et al., 2006), is indispensable for ovarian contraction. Edn2 knockout resulted in a significant reduction in the number of ovulated oocytes (Cacioppo et al., 2017). Also, pharmacological inhibition of the preovulatory Edn2 induction, that can be reversed with the exogenous peptide, or blocking Edn2 receptors were shown to prevent vasoconstriction and follicle rupture (Migone et al., 2016).
Of note, in contrast to frogs, mammalian oocytes are ovulated in a complex with cumulus cells, a specific sublineage of granulosa cells directly wrapping the oocyte. Expansion of cumulus cells that occurs after initiation of meiosis but before follicular rupture represents an important step of mammalian ovulation regulated via a distinct intracellular pathway (Figure 2). It involves MAPK-mediated transcriptional induction of the EGF-like ligands, such as amphiregulin, epiregulin, and betacellulin in granulosa cells (Park et al., 2004). The EGF-Ls secreted by granulosa cells transactivate EGFR on cumulus cells, inducing the production of cumulus matrix proteins, such as hyaluronan synthase 2 (HAS2) and pentraxin 3 (PTX3), which cause cumulus oocyte complex (COC) expansion. It was found that inhibition of cumulus cells expansion also impedes follicular rupture, suggesting that the two processes engage overlapping signaling pathways with similar changes in gene expression. For instance, PGs were reported to affect mammalian ovulation through their influence on cumulus expansion (Sirois et al., 2004). In detail, molecular mechanisms of coordination through the cumulus complex are reviewed elsewhere (Russel and Robker, 2007; Robker et al., 2018).
Several parallels were revealed between Drosophila melanogaster and mammalian ovulation, including follicle rupture, at both cellular and molecular levels. An ex vivo follicle rupture assay has been developed that allowed direct quantification of follicles’ capacity to respond to ovulation stimuli and rupture in Drosophila (Deady and Sun, 2015). It was found, using both in vivo and in vitro approaches, that posterior follicle cells surrounding mature oocytes are selectively degraded during ovulation in Drosophila. Like in mammals, follicle rupture also depends on MMP2 activity localized at the posterior end of mature follicles (Deady et al., 2015). It was shown that MMP2 activity is regulated by the octopaminergic signaling in mature follicle cells. Exogenous octopamine is sufficient to induce follicle rupture when isolated mature follicles are cultured ex vivo, in the absence of the oviduct or ovarian muscle sheath. Accordingly, knocking down the octoampine receptor Oamb in mature follicle cells prevented OA-induced follicle rupture ex vivo and ovulation in vivo. It was further found that follicular octopamine signaling induces MMP2 enzymatic activation but not MMP2 protein expression, likely via intracellular Ca2+ (Deady and Sun, 2015). The developed ex vivo follicle rupture assay was proposed to query enzymatic activity and to perform genetic or pharmacological screens to identify genes or small molecules involved in follicle rupture in Drosophila (Knapp et al., 2018). It was further demonstrated that steroid signaling in mature follicle cells is important for Drosophila ovulation. Knocking down the shade monooxygenase that converts ecdysone to its active analog 20-hydroxyecdysone or disruption of the ecdysone receptor specifically in mature follicle cells, was shown to block follicle rupture (Knapp and Sun, 2017). Furthermore, it was demonstrated that ecdysteroid signaling is essential for proper activation of the MMP MMP2 and follicle rupture. The zinc-finger transcription factor Hindsight (Hnt) has been implicated in the development of ovulation competency of Drosophila follicles. This factor is upregulated in mature follicle cells. It was found that Hnt elevates MMP2 expression in posterior follicle cells and expression of the adrenergic receptor Oamb in all follicle cells, which is essential for follicle rupture and ovulation (Deady et al., 2017).
Follicle rupture during ovulation has been thoroughly investigated in fish models, most notably, in teleosts (bony fishes) (reviewed by Takahashi et al., 2019). In teleost fishes, ovulation results in discharge of fertilizable oocytes from ovarian follicles into the ovarian or abdominal cavity. Several studies focused on the involvement of proteases and protease inhibitors in this process, and it was found that robust proteolytic events accompany ovulation in various teleost fishes. For instance, it was shown that collagenolytic activity is present and it increases prior to ovulation in follicle walls of brook trout (Salvelinus fontinalis) and yellow perch (Perca flavescens) follicles, suggesting involvement of the MMP activity in digesting the follicle wall (Berndtson and Goetz, 1988, 1990). Involvement of kallikrein-like serine protease KT-14 in trout ovulation was also demonstrated (Hajnik et al., 1998). It was further demonstrated that LH-induced in vitro ovulation of brook trout follicles involves follicle contraction and activation of multiple proteolytic genes (Crespo et al., 2013). LH increased mRNA expression levels of MMPs, such as MMP2 and MMP19, and other enzymes with proteolytic action during ovulation, such as disintegrin, ADAMTS1 and plasminogen, in brook trout preovulatory follicles and during the progression of LH-induced ovulation. Of note, the expression of tissue inhibitor of the MMP TIMP2 paralleled that of MMP2, suggesting the existence of a controlled mechanism of MMP action (Crespo et al., 2013). More recently, genome-wide studies identified the genes upregulated and downregulated in the ovulatory follicle during ovulation in fish (Bobe et al., 2006; Klangnurak and Tokumoto, 2017; Liu et al., 2017). Serine protease 23 and metalloprotease ADAM22 were found to be induced in the periovulatory follicle of rainbow trout (Bobe et al., 2006). A significant change in the expression of ADAMTS8b, ADAMTS9 and MMP9 was observed in preovulatory follicles of zebra fish (Liu et al., 2017). It was accompanied by upregulation of the intrinsic proteinase inhibitors, such as Serpine 1 and TIMP2.
The detailed studies on follicle rupture during ovulation in fishes were carried out using the freshwater teleost medaka (Oryzias latipes). In vitro ovulation models developed for medaka allowed identification of the hydrolytic enzymes responsible for oocyte liberation during ovulation. Furthermore, similarly to mammals, the involvement of prostaglandin E2, PGE2, cyclooxygenase-2, and PGE2 receptor subtype EP4b in medaka ovulation has also been demonstrated (Takahashi et al., 2013). It was found that follicle rupture in the medaka ovary involves the cooperation of at least three different MMPs, and the tissue inhibitor of MMP-2b protein (Ogiwara et al., 2005). The discrete roles of each of these proteins in follicle rupture has been elucidated. It was found indicated that gelatinase A (MMP2) induces the hydrolysis of type IV collagen constituting the basement membrane, membrane-type 2 MMP (MMP15) degrades type I collagen present in the theca cell layer, whereas MT1-MMP (MMP14) and the tissue inhibitor of MMP-2b TIMP2b are involved in production and regulation of gelatinase A (Ogiwara et al., 2005). Consistently, it was found, using an in vitro ovulation model based on the whole ovary culture, that medaka ovulation could be inhibited by the addition of metalloproteinase inhibitors to the ovarian culture (Ogiwara et al., 2010). In addition, involvement of a plasmin-like protease in early stage follicle rupture during ovulation in medaka has been demonstrated (Ogiwara et al., 2012). The enzyme was shown to participate in the rupture several hours before the activation of MMP-mediated hydrolysis. Furthermore, involvement of urokinase plasminogen activator and plasminogen activator inhibitor-1 in follicle rupture during ovulation in medaka has been shown (Ogiwara et al., 2015). It was found, using an in vitro ovulation assay, that various serine protease inhibitors, including a specific plasmin inhibitor, significantly reduce ovulation rates. A follicle rupture model involving two different proteolytic enzyme systems, serine protease and MMP, and sequential two-step hydrolysis of extracellular matrix has been proposed in medaka ovulation (Ogiwara et al., 2012).
Presently, molecular mechanisms leading to upregulation of the multiple proteolytic enzymes in teleosts during ovulation have not been well investigated. It was reported that ovarian prostaglandin synthesis greatly increases in several teleost species during ovulation, and that a non-selective cyclooxygenase inhibitor indomethacin blocks both in vivo and in vitro ovulation, suggesting that prostaglandins play an important role during ovulation in these oviparous species (Takahashi et al., 2013). Most recently, the involvement of Cdk activity in degradation of the extracellular matrix during ovulation in medaka has been demonstrated (Ogiwara and Takahashi, 2019). It was found that the Cdk inhibitor roscovitine inhibited both follicle ovulation and follicular expression of MMP15, implicating Cdk activity in the expression of this protease. It was further suggested that the nuclear progestin receptor, PGR, which is induced by the LH surge, serves as a functional transcription factor for MMP15 expression after Cdk9/Cyclin I-mediated phosphorylation (Ogiwara and Takahashi, 2019). Notably, in contrast to mammalian species, the importance of MAPK activation for follicle rupture in teleosts has not been established. Moreover, it was reported that incubating medaka follicles with a MEK inhibitor did not lead to significant changes in PGR phosphorylation (Ogiwara and Takahashi, 2019).
Paradoxically, although oocyte maturation was most thoroughly investigated in amphibians, little is known about the mechanisms of follicle rupture in frog species, including Xenopus laevis. Most notably, the involvement of proteolytic enzymes in follicle rupture has not been established and intracellular signal transduction pathways leading to their activation were not identified. In fact, relatively few studies have addressed mechanisms of follicle rupture during ovulation in frogs. This situation can be attributed, in part, to the lack of reliable in vitro reconstitution of follicle rupture in the frog model and to difficulties of dissecting this process in living animals.
In frogs, in vitro maturation and ovulation of Rana pipiens and Rana dybowskii oocytes was observed in the ovarian fragments and isolated follicles treated with homologous pituitary extracts (Wright, 1961; Dettlaff et al., 1964; Smith et al., 1968). It was reported that oocyte liberation can be achieved more readily from ovarian fragments than from isolated ovarian follicles and hypothesized that attachment of follicles to a site in the ovarian fragment is beneficial for contraction of follicle wall during oocyte extrusion (Schuetz, 1986; Kwon et al., 1992). The efficiency of pituitary preparations varied considerably, exhibiting seasonal dependence; ovarian fragments from some animals failed to ovulate in response to any treatment and spontaneous follicular rupture occurred in cultured ovarian fragments collected during some seasons of the year (Smith et al., 1968; Kwon et al., 1992). It was also reported that maturation proceeded in the absence of follicle rupture in follicles isolated from some animals. Moreover, follicle rupture in the in vitro cultured ovarian fragments lagged greatly behind GVBD, whereas these two events occurred almost simultaneously during natural ovulation. Furthermore, although various steroid hormones were found to be effective in inducing in vitro maturation of isolated frog oocytes, the hormones failed to promote follicular rupture (Subtelny et al., 1968; Schuetz and Lessman, 1982). Early studies of in vitro frog ovulation demonstrated that intact follicles of Rana pipiens ovulated in response to pituitary homogenate (FPH) due to, in part, mechanical contractions of the follicle wall (Schuetz and Lessman, 1982). Ovulation and follicular contractions could not be observed following removal of the surface epithelium in the presence of the intact thecal layer. Treatment of isolated amphibian ovarian follicles with FPH was shown to increase follicular P4 levels and initiate oocyte maturation (Petrino and Schuetz, 1987). However, it was found that oocytes, regardless of the removal of any or all follicular wall layers, matured but did not ovulate in response to P4. These results provided functional evidence for a role of the ovarian surface epithelium and demonstrated that P4 alone is not sufficient to initiate follicle rupture during ovulation in frogs. Nevertheless, in the later studies using cultured ovarian fragments to investigate release of frog oocytes from follicles during ovulation, follicle rupture has been observed in the vigorously shaken ovarian fragments after extensive overnight incubation in the presence of P4 or hCG (Liu et al., 2005; Sena and Liu, 2008).
At present, signaling pathways involved in the process of follicle rupture in frogs have not been established. Importance of MAPK activation in follicle cells, which is a prerequisite for follicle rupture in mammals, was not explicitly demonstrated, and the transcription factors involved in the upregulation of follicular protease activity during ovulation were not identified. Involvement of PKC was suggested by the findings that TPA effectively stimulated PGF2α secretion and ovulation, and that PKC inhibition dramatically suppressed FPH- or TPA-induced PGF2α secretion and ovulation observed in the isolated ovarian fragments of Rana pipiens. It was further demonstrated that PKC mediates gonadotropin-induced production of PGF2α but not steroid synthesis in the frog ovaries (Chang et al., 1995). It was reported that exposure of in vitro cultured ovarian follicles to PGF2α stimulated low levels of follicle rupture in the absence of maturation (Schuetz, 1986; Chang et al., 1995). Addition of PGF2α, but not PGE, to cultured follicles markedly enhanced the incidence of ovulation in follicles exposed to P4 or pituitary homogenates. Ovulatory effects of PGF2α were suggested to be mediated through the follicular epithelium. Similarly, in vitro studies of oocyte ovulation using ovarian fragments isolated from the toad Bufo arenarum revealed that PGF2α, but not PGE1, increased ovulatory responses to either pituitary homogenate or P4 at suboptimal doses, indicating that PGF2α exerted a synergistic potentiating effect (Ramos et al., 2008). Furthermore, it was found that the transcriptional inhibitor actinomycin D blocked P4-induced follicle rupture, however, PGF2α was found to overcome the inhibitory effect of the drug, indicating that this prostaglandin has the ability to induce follicle rupture in the absence of protein synthesis (Liu et al., 2005; Sena and Liu, 2008). Notably, the follicular levels of both PGF2α and PGE2 increase during the preovulatory period, however PGF2α levels are much higher than that of PGE2. It was found that expression of COX2 but not COX1 is up-regulated during hCG- or P4-induced ovulation in vitro, leading to increased synthesis of PGF2α during preovulatory period (Sena and Liu, 2008). It was found that a PGR antagonist RU486 inhibits COX2 expression and PGF2α synthesis, suppressing, in a dose-dependent manner, P4-induced ovulation observed in the isolated Xenopus ovarian follicles (Dhillon et al., 2010). It was suggested that although PGF2α is necessary for P4-induced ovulation of Xenopus oocytes, it may not be essential for hCG-induced ovulation (Sena and Liu, 2008). Based on the above findings, it has been hypothesized that ovulation and maturation of amphibian oocytes can be mediated by separate classes of hormones, and that normal synchronization of ovulation and maturation requires the combined action of prostaglandins and steroids within different follicular compartments.
Our recent study using a new in vitro ovulation model presented the first direct evidence for involvement of MAPK and MMP activities in follicle rupture during Xenopus oocyte ovulation (Tokmakov et al., 2019). It was found that both meiotic maturation and follicle rupture can be reproduced in vitro using isolated ovarian follicles shortly pretreated with collagenase before P4 administration. Oocyte maturation and follicle rupture could also be observed in the hCG-treated follicles, albeit with somewhat lower rates. Notably, follicular rupture occurred at about the same time as GVBD (Figure 4), and it didn’t occur in the absence of P4, indicating physiological relevance of the developed model. Indeed, the two processes are highly coordinated in vivo: mature oocytes are not retained in the ovary, and immature oocytes don’t ovulate during natural ovulation in frogs. Importantly, oocyte release from ovarian follicles, but not oocyte maturation, was inhibited in this model in the presence of a wide-specificity MMP inhibitor GM6001. In addition, pharmacological inhibition of the MAPK pathway using a specific MEK inhibitor U0126 was demonstrated to suppress both meiotic maturation and follicular rupture (Tokmakov et al., 2019). These findings prove that MAPK and MMP activities are involved in the process of oocyte liberation from ovarian follicles in frogs. Further studies are necessary to identify proteolytic enzymes engaged in follicle rupture and to delineate the pathway of MAPK activation in follicle cells during ovulation in frogs.
Figure 4. Timing of maturation and follicle rupture during Xenopus oocyte ovulation. Progression of meiotic maturation in the developed in vitro ovulation model (Tokmakov et al., 2019) was judged by appearance of a white spot (WS) on the animal hemisphere of oocytes, reflecting germinal vesicle breakdown (GVBD), and occurrence of follicular rapture (FR) was defined by the loss of the follicle layer (FL). (A) Theoretically, two alternative scenarios, GVBD-FR and FR-GVBD, can occur during ovulation, as indicated in the figure. To distinguish between them, the four morphologically different types of cells were counted during hormone-induced ovulation. They included oocytes without WS surrounded by FL (WS-FL+), oocytes with WS and FL (WS + FL+), oocytes without WS and FL (WS–FL–), and oocytes with WS and without FL (WS + FL). The WS-FL + phenotype characterizes original oocyte population, and the WS + FL– phenotype corresponds to ovulated mature eggs. (B) Experimentally observed relative frequencies of the two intermediate phenotypes, WS + FL + and WS–FL–, are very close, indicating that GVBD and FR occur almost simultaneously.
Oocyte liberation from the ovarian follicles and oocyte maturation are interdependent, tightly linked, and coordinated processes. All naturally ovulated frog eggs are matured and arrested in metaphase II with high activity of MPF and CSF, and all non-ovulated oocytes retained in the frog ovaries after hormonal stimulation remain immature and arrested in prophase I with low activity of MPF and CSF. Communication between the oocyte and follicular cells is necessary to coordinate the two major ovulation processes, meiotic maturation and follicle rupture, during normal physiological ovulation. However, overall coordination of ovulatory process in ovarian follicles of frogs is poorly studied. The complex intercellular communication follicular networks have been most thoroughly investigated in mammalian models and comprehensively reviewed in several recent publications (Richards and Ascoli, 2018; Robker et al., 2018; Duffy et al., 2019). Here, we provide just a bird’s-eye view of intrafollicular coordination during ovulation.
In the resting ovarian follicle, the oocyte and cumulus cells are directly connected by terminal gap junctions. The terminal gap junctions allow transit of small (<1 kDa) molecules between the oocyte and cumulus cell cytoplasm. Energy metabolites, such as glucose, pyruvate, and ATP can be transported from granulosa cells via gap junctions (Edry et al., 2006; Johnson et al., 2007). Gap junctions between oocyte and follicle cells have also been observed in frog follicles (Konduktorova and Luchinskaia, 2013), however, their role in the ovulatory process was not thoroughly investigated in this model. Importantly, cAMP and cGMP, which are needed to maintain diplotene arrest in immature oocytes (see section “Diplotene Arrest in Immature Oocytes” for details), are transported into oocytes from cumulus cells through the terminal gap junctions (Edry et al., 2006; Shuhaibar et al., 2015). The importance of direct communication between the oocyte and somatic cells in the follicle was revealed by knockout of connexin 37, a gap junction protein involved in the direct transfer of small molecules. It was found that connexin 37-deficient mice can produce large preovulatory follicles but fail to ovulate, indicating that normal ovulatory response is not possible without sustained communication between oocytes and surrounding somatic cells (Simon et al., 1997). It was demonstrated that activation of Gαs-coupled LH receptors stimulates adenylyl cyclase, elevates intracellular cAMP levels, activates PKA in follicular cells, and promotes steroidogenesis by increasing expression of the StAR in both theca and granulosa cells of mammalian follicle, as well as by StAR activation via its phosphorylation on serine 195 (Wood and Strauss, 2002; Jamnongjit and Hammes, 2006). Importantly, the LH surge causes closure of gap junctions, presumably through phosphorylation of the connexin proteins (Granot and Dekel, 1994). Involvement of the MAPK pathway and EGFR in the gap junction closure has been demonstrated in mammals (Norris et al., 2008, 2010; Prochazka and Blaha, 2015). MAPK activation and MAPK-mediated EGFR signaling in granulosa cells early in ovulation are indispensable for both oocyte maturation and follicle rupture, as detailed in the sections “Meiotic Resumption” and “Mammals” of this article. The MAPK pathway (Ras/Raf/MEK/ERK pathway) is triggered in granulosa cells by Src kinase via Gβγ proteins activated by G-protein coupled receptors (Jamnongjit and Hammes, 2006).
Discontinuation of junctional communications within the ovarian follicle promotes oocyte maturation (Sela-Abramovich et al., 2006). It is assumed that maturation proceeds in an entirely autonomous fashion after gap junction closure. The closure of gap junctions terminates transport of cAMP and cGMP from cumulus cells into the oocyte, leading to a decline in intraoocyte cAMP and cGMP levels. The decreased level of cGMP releases inhibition of phosphodiesterase 3A, further promoting accelerated degradation of cAMP and triggering meiotic resumption of the oocyte. In addition, high follicular levels of progestogens and/or androgens, which are synthesized by granulosa cells in response to LH, lead to further decrease in the intraoocyte level of cAMP due to G protein-coupled receptor-mediated inhibition of plasma membrane AC (see section “Meiotic Resumption” for details). Although the latter mechanism seems to be a major cause triggering meiotic resumption in frogs, P4 can also influence oocyte maturation in some mammalian species. For example, treatment with P4 agonists in the absence of gonadotropin was shown to promote oocyte maturation to metaphase II, but not ovulation, in rhesus macaque follicles (Borman et al., 2004). It should be emphasized that the steroid stimulates transcription-independent oocyte maturation via the membrane P4 receptor and transcription-dependent follicle rupture via the classical nuclear receptor.
Evidence is presented that P4 mediates follicular rupture in mammals. Inhibition of P4 synthesis or its activity prevents ovulation (Espey et al., 1990; Hibbert et al., 1996) and mice lacking nuclear P4 receptor do not ovulate (Lydon et al., 1995). Interestingly, the role of PGR in mammals seems to be confined mainly to mediating follicular rupture, because activation of cumulus expansion and oocyte meiosis occur normally, and the oocytes obtained from the P4 receptor-knockout follicles are fertilizable (Robker et al., 2000). Antagonists of PGR, such as mifepristone (RU486), valaprisan, ulipristal, and some others can effectively block ovulation and are used as contraceptives in clinics (Jesam et al., 2016; Schutt et al., 2018). The observation that P4 alone is able to promote the entire ovulation process in the isolated ovarian follicles of Xenopus laevis (Tokmakov et al., 2019) suggests that PGR may also be engaged in follicular rupture in frogs. Of interest, it was reported that in amphibians, unlike in mammals, RU486 acts as an agonist of P4 (Skoblina, 2002).
It appears that oocyte maturation and follicle rupture unfold completely independently after steroid production and gap junction closure following the gonadotropin surge. Indeed, the well-established fact that meiotic maturation can readily be induced by P4 and some other steroids in defolliculated frog oocytes, as well as the recent finding that blocking follicle rupture by means of MMP inhibition does not affect Xenopus oocyte maturation (Tokmakov et al., 2019), highlight autonomous character of oocyte maturation. However, it cannot be ruled out that oocytes affect vice versa ovulatory processes in follicle cells. Notably, oocytes and follicle cells are known to communicate via paracrine signals in addition to gap junctions (Eppig, 2001). For example, it was reported that expansion of the mouse cumulus oophorus requires a factor secreted by the oocyte, so called, the cumulus expansion enabling factor, CEEF (Buccione et al., 1990). Also, it was found that co-incubation of defolliculated mouse oocytes with isolated cumulus cells markedly increases MAPK activity in the cumulus cells stimulated by FSH, while MAPK activity remains low in the absence of oocytes, indicating that oocytes secrete some paracrine factors promoting MAPK activation in cumulus cells (Su et al., 2003). Oocyte-derived paracrine factors GDF9 and BMP15 were found to function in a cooperative manner to stimulate signaling pathways in preovulatory cumulus granulosa cells and to maintain the integrity of the cumulus-oocyte complex (Matzuk et al., 2002). Further evidence for the existence of an oocyte-granulosa cell regulatory loop has been provided by the observation that the BMP15 and GDF9 growth factors regulate MAPK activation in the cumulus cells and cumulus expansion, as well as meiotic oocyte resumption after the preovulatory LH surge (Su et al., 2004). A hypothesis of bidirectional communication between the oocyte and follicle cells has been proposed to corroborate coordination of meiotic maturation and cumulus expansion in the isolated cumulus cell-enclosed mouse oocytes (Su et al., 2003).
The existence of bidirectional communication between oocytes and follicle cells can easily explain the fact that follicular rupture is well synchronized with maturation and meiotic resumption, so that both processes naturally occur in vivo within several hours of hormonal stimulation. Moreover, it was demonstrated, using an in vitro model of Xenopus oocyte ovulation, that follicle rupture develops simultaneously with GVBD (Tokmakov et al., 2019; Figure 4), which takes place during the first meiotic metaphase. Paracrine signals from maturing oocytes to follicle cells would potentially prevent the failed reproductive scenario, where follicle rupture is initiated in the absence of meiotic maturation, leading to ovulation of immature and unfertilizable oocytes. On the other hand, this kind of feedback would help to avoid the situation where matured oocytes remain entrapped inside ovarian follicles in the ovary. It has been reported previously that unfertilized matured Xenopus oocytes die by a well-defined apoptotic process within 48–72 h of hormonal stimulation (Du Pasquier et al., 2011; Tokmakov et al., 2011; Iguchi et al., 2013). Eggs from different species, including mammals, have been shown to die by apoptosis in the absence of fertilization (Tokmakov et al., 2017, 2018). Clearance of large follicle-entrapped apoptotic eggs from the ovaries may represent a formidable homeostatic challenge that would potentially overload the immune system. In frogs, no matured follicular oocytes can be observed in the ovary after ovulation, and all oocytes found in the genital tract during ovulation have completed GVBD, suggesting that GVBD and oocyte liberation from ovarian follicles are remarkably synchronized and coordinated in vivo. However, a great number of eggs dying by an apoptotic process are retained in the frog genital tract after ovulation (Iguchi et al., 2013; Tokmakov et al., 2018; Sato and Tokmakov, 2020), indicating that oviposition rather than ovulation may be a bottleneck of the reproductive process in oviparous species.
Using follicular fragments, isolated follicles, and denuded oocytes greatly facilitates dissection of the ovulatory process in different animals. A remarkable progress has been achieved in depicting intricate molecular mechanisms of meiotic maturation using frog oocytes and eggs. The studies of follicular rupture and the entire process of ovulation have been successful in several other species. The crucial follicular processes indispensable for successful ovulation, such as steroidogenesis, prostaglandin synthesis, and proteolysis, have been most thoroughly investigated in mammals and teleost fishes. However, coordination of maturation and follicle rupture, the two interdependent and highly synchronized ovulatory processes, as well as other follicular events, remains largely unexplained. Multiple endocrine, paracrine, and autocrine signaling pathways regulate ovulation, and their coordinated cooperation requires further investigation.
AT, VS, and K-IS conceived the review topic and discussed its content. AT wrote the manuscript. AT, VS, and K-IS revised the work. All the authors approved the final submitted version.
This publication is a product of research activity of the Institute for Comprehensive Research “Special Research Project” financially supported by the Kyoto Sangyo University Research Grant E2013. The work was supported in part by the Collaboration Research Grant 281027 from the Kobe University, Japan, (to AT) and by the Grant-in-Aid for Scientific Research 15K07083 from the Ministry of Education, Culture, Sports, Science, and Technology of Japan (to K-IS).
The authors declare that the research was conducted in the absence of any commercial or financial relationships that could be construed as a potential conflict of interest.
The authors thank Dr. Elena Loboda for critical reading and commenting on the manuscript.
Abrieu, A., Brassac, T., Galas, S., Fisher, D., Labbe, J. C., and Doree, M. (1998). The polo-like kinase plx1 is a component of the MPF amplification loop at the G2/M-phase transition of the cell cycle in Xenopus eggs. J. Cell Sci. 111, 1751–1757.
Aertgeerts, K., De Bondt, H. L., De Ranter, C., and Declerck, P. J. (1994). A model of the reactive form of plasminogen activator inhibitor-1. J. Struct. Biol. 113, 239–245. doi: 10.1006/jsbi.1994.1058
Andresson, T., and Ruderman, J. V. (1998). The kinase Eg2 is a component of the Xenopus oocyte progesterone-activated signaling pathway. EMBO J. 17, 5627–5637. doi: 10.1093/emboj/17.19.5627
Ashkenazi, H., Cao, X., Motola, S., Popliker, M., Conti, M., and Tsafriri, A. (2005). Epidermal growth factor family members: endogenous mediators of the ovulatory response. Endocrinology 146, 77–84. doi: 10.1210/en.2004-0588
Bakos, M. A., Kurosky, A., and Hedrick, J. L. (1990). Physicochemical characterization of progressive changes in the Xenopus laevis egg envelope following oviductal transport and fertilization. Biochemistry 29, 609–615. doi: 10.1021/bi00455a003
Baulieu, E. E., Godeau, F., Schorderet, M., and Schorderet-Slatkine, S. (1978). Steroid-induced meiotic division in Xenopus laevis oocytes: surface and calcium. Nature 275, 593–598. doi: 10.1038/275593a0
Bayaa, M., Booth, R. A., Sheng, Y., and Liu, X. J. (2000). The classical progesterone receptor mediates Xenopus oocyte maturation through a nongenomic mechanism. Proc. Natl. Acad. Sci. U.S.A. 97, 12607–12612. doi: 10.1073/pnas.220302597
Belloc, E., and Méndez, R. (2008). A deadenylation negative feedback mechanism governs meiotic metaphase arrest. Nature 452, 1017–1021. doi: 10.1038/nature06809
Berndtson, A. K., and Goetz, F. W. (1988). Protease activity in brook trout (Salvelinus fontinalis) follicle walls demonstrated by substrate-polyacrylamide gel electrophoresis. Biol. Reprod. 38, 511–516. doi: 10.1095/biolreprod38.2.511
Berndtson, A. K., and Goetz, F. W. (1990). Metallo-protease activity increases prior to ovulation in brook trout (Salvelinus fontinalis) and yellow perch (Perca flavescens) follicle walls. Biol. Reprod. 42, 391–398. doi: 10.1095/biolreprod42.2.391
Bishop, C. V., Hennebold, J. D., Kahl, C. A., and Stouffer, R. L. (2016). Knockdown of progesterone receptor (PGR) in macaque granulosa cells disrupts ovulation and progesterone production. Biol. Reprod. 94:109. doi: 10.1095/biolreprod.115.134981
Blasi, F. (1993). Urokinase and urokinase receptor: a paracrine/autocrine system regulating cell migration and invasiveness. BioEssays 15, 105–111. doi: 10.1002/bies.950150206
Bobe, J., Montfort, J., Nguyen, T., and Fostier, A. (2006). Identification of new participants in the rainbow trout (Oncorhynchus mykiss) oocyte maturation and ovulation processes using cDNA microarrays. Reprod. Biol. Endocrinol. 4:39. doi: 10.1186/1477-7827-4-39
Bonnell, B. S., and Chandler, D. E. (1996). Egg jelly layers of Xenopus laevisare unique in ultrastructure and sugar distribution. Mol. Reprod. Dev 44, 212–220. doi: 10.1002/(sici)1098-2795(199606)44:2<212::aid-mrd10>3.0.co;2-4
Borman, S. M., Chaffin, C. L., Schwinof, K. M., Stouffer, R. L., and Zelinski-Wooten, M. B. (2004). Progesterone promotes oocyte maturation, but not ovulation, in nonhuman primate follicles without a gonadotropin surge. Biol. Reprod. 71, 366–373. doi: 10.1095/biolreprod.103.023390
Breen, S. M., Andric, N., Ping, T., Xie, F., Offermans, S., Gossen, J. A., et al. (2013). Ovulation involves the luteinizing hormone-dependent activation of G(q/11) in granulosa cells. Mol. Endocrinol. 27, 1483–1491. doi: 10.1210/me.2013-1130
Browne, C. L., Wiley, H. S., and Dumont, J. N. (1979). Oocyte-follicle cell gap junctions in Xenopus laevis and the effects of gonadotropin on their permeability. Science 203, 182–183. doi: 10.1126/science.569364
Browne, R. K., and Figiel, C. R. Jr. (2019). Amphibian Hormone Cycle. Apple Valley, MN: Amphibian Ark organization.
Brun, R. (1975). Oocyte maturation in vitro: contribution of the oviduct to total maturation in Xenopus laevis. Experientia 31, 1275–1276. doi: 10.1007/BF01945777
Buccione, R., Vanderhyden, B. C., Caron, P. J., and Eppig, J. J. (1990). FSH induced expansion of the mouse cumulus oophorus in vitro is dependent upon a specific factor(s) secreted by the oocyte. Dev. Biol. 138, 16–25. doi: 10.1016/0012-1606(90)90172-f
Cacioppo, J. A., Lin, P. P., Hannon, P. R., McDougle, D. R., Gal, A., and Ko, C. (2017). Granulosa cell endothelin-2 expression is fundamental for ovulatory follicle rupture. Sci. Rep. 7:817. doi: 10.1038/s41598-017-00943-w
Cameron, M. R., Foster, J. S., Bukovsky, A., and Wimalasena, J. (1996). Activation of mitogen-activated protein kinases by gonadotropins and cyclic adenosine 5-monophosphates in porcine granulosa cells. Biol. Reprod. 55, 111–119. doi: 10.1095/biolreprod55.1.111
Castilho, P. V., Williams, B. C., Mochida, S., Zhao, Y., and Goldberg, M. L. (2009). The M phase kinase Greatwall (Gwl) promotes inactivation of PP2A/B55delta, a phosphatase directed against CDK phosphosites. Mol. Biol. Cell. 20, 4777–4789. doi: 10.1091/mbc.e09-07-0643
Castro, A., Peter, M., Magnaghi-Jaulin, L., Vigneron, S., Galas, S., Lorca, T., et al. (2001). Cyclin B/cdc2 Induces c-Mos Stability by Direct Phosphorylation in Xenopus Oocytes. Mol. Biol. Cell. 12, 2660–2671. doi: 10.1091/mbc.12.9.2660
Chaffin, C. L., and Stouffer, R. L. (1999). Expression of matrix metalloproteinases and their tissue inhibitor messenger ribonucleic acids in macaque periovulatory granulosa cells: time course and steroid regulation. Biol. Reprod. 61, 14–21. doi: 10.1095/biolreprod61.1.14
Chaffin, C. L., and Stouffer, R. L. (2002). Local role of progesterone in the ovary during the periovulatory interval. Rev. Endocr. Metab. Disord. 3, 65–72. doi: 10.1023/a:1012704903128
Chang, K. J., Kim, J. W., Lee, J., Im, W. B., Kwon, H. B., and Schuetz, A. W. (1995). Prostaglandin production and ovulation during exposure of amphibian ovarian follicles to gonadotropin or phorbol ester in vitro. Gen. Comp. Endocrinol. 100, 257–266. doi: 10.1006/gcen.1995.1156
Crespo, D., Pramanick, K., Goetz, F. W., and Planas, J. V. (2013). Luteinizing hormone stimulation of in vitro ovulation in brook trout (Salvelinus fontinalis) involves follicle contraction and activation of proteolytic genes. Gen. Comp. Endocrinol. 188, 175–182. doi: 10.1016/j.ygcen.2013.02.031
Curry, T. E. Jr., and Osteen, K. G. (2003). The matrix metalloproteinase system: changes, regulation, and impact throughout the ovarian and uterine reproductive cycle. Endocr. Rev. 24, 428–465. doi: 10.1210/er.2002-0005
Das, S., Maizels, E. T., DeManno, D., St Clair, E., Adam, S. A., and Hunzicker-Dunn, M. (1996). A stimulatory role of cyclic adenosine 3,5-monophosphate in follicle-stimulating hormone-activated mitogen-activated protein kinase signaling pathway in rat ovarian granulosa cells. Endocrinology 137, 967–974. doi: 10.1210/endo.137.3.8603610
Davis, B. J., Lennard, D. E., Lee, C. A., Tiano, H. F., Morham, S. G., Wetsel, W. C., et al. (1999). Anovulation in cyclooxygenase-2-deficient Mice Is Restored by Prostaglandin E2 and interleukin-1beta. Endocrinology 140, 2685–2695. doi: 10.1210/endo.140.6.6715
Deady, L. D., Li, W., and Sun, J. (2017). The zinc-finger transcription factor hindsight regulates ovulation competency of Drosophila follicles. eLife 6:e29887. doi: 10.7554/eLife.29887
Deady, L. D., Shen, W., Mosure, S. A., Spradling, A. C., and Sun, J. (2015). Matrix Metalloproteinase 2 is required for ovulation and corpus luteum formation in Drosophila. PLoS Genet. 11:e1004989. doi: 10.1371/journal.pgen.1004989
Deady, L. D., and Sun, J. (2015). A follicle rupture assay reveals an essential role for follicular adrenergic signaling in Drosophila ovulation. PLoS Genet. 11:e1005604. doi: 10.1371/journal.pgen.1005604
Deng, J., Carbajal, L., Evaul, K., Rasar, M., Jamnongjit, M., and Hammes, S. R. (2009). Nongenomic steroid-triggered oocyte maturation: of mice and frogs. Steroids 74, 595–601. doi: 10.1016/j.steroids.2008.11.010
Deng, J., Lang, S., Wylie, C., and Hammes, S. R. (2008). The Xenopus laevis isoform of G protein-coupled receptor 3 (GPR3) is a constitutively active cell surface receptor that participates in maintaining meiotic arrest in X. laevis oocytes. Mol. Endocrinol. 22, 1853–1865. doi: 10.1210/me.2008-0124
Dettlaff, T. A., Nikitina, L. A., and Stroeva, O. G. (1964). The role of the germinal vesicle in ooecyte maturation in anurans as revealed by the removal and transplantation of nuclei. J. Embryol. Exp. Morphol. 12, 851–873.
Dhillon, J. K., Su, X., and Liu, Z. (2010). Effects of RU486 on cyclooxygenase-2 gene expression, prostaglandin F2alpha synthesis and ovulation in Xenopus laevis. Gen. Comp. Endocrinol. 165, 78–82. doi: 10.1016/j.ygcen.2009.06.005
Diakow, C., Scharff, C., and Aronow, L. (1988). Egg-oviduct interaction initiates reproductive behavior. Horm. Behav. 22, 131–138. doi: 10.1016/0018-506x(88)90036-0
Du Pasquier, D., Dupre, A., and Jessus, C. (2011). Unfertilized Xenopus eggs die by bad-dependent apoptosis under the control of Cdk1 and JNK. PLoS One 6:e23672. doi: 10.1371/journal.pone.0023672
Duckworth, B. C., Weaver, J. S., and Ruderman, J. V. (2002). G2arrest in Xenopus oocytes depends on phosphorylation of cdc25 by protein kinase A. Proc. Natl. Acad. Sci. U.S.A. 99, 16794–16799. doi: 10.1073/pnas.222661299
Duffy, D. M., Ko, C., Jo, M., Brannstrom, M., and Curry, T. E. (2019). Ovulation: Parallels With Inflammatory Processes. Endocr. Rev. 40, 369–416. doi: 10.1210/er.2018-00075
Dupre, A., Buffin, E., Roustan, C., Nairn, A. C., Jessus, C., and Haccard, O. (2013). The phosphorylation of ARPP19 by Greatwall renders the auto-amplification of MPF independently of PKA in Xenopus oocytes. J. Cell. Sci. 126, 3916–3926. doi: 10.1242/jcs.126599
Ebisch, I. M., Thomas, C. M., Wetzels, A. M., Willemsen, W. N., Sweep, F. C., and Steegers-Theunissen, R. P. (2008). Review of the role of the plasminogen activator system andvascular endothelial growth factor in subfertility. Fertil. Steril. 90, 2340–2350. doi: 10.1016/j.fertnstert.2007.10.026
Edry, I., Sela-Abramovich, S., and Dekel, N. (2006). Meiotic arrest of oocytes depends on cell-to-cell communication in the ovarian follicle. Mol. Cell. Endocrinol. 252, 102–106. doi: 10.1016/j.mce.2006.03.009
Einspanier, A., Jurdzinski, A., and Hodges, J. K. (1997). A local oxytocin system is part of the luteinization process in the preovulatory follicle of the marmoset monkey (Callithrix jacchus). Biol. Reprod. 57, 16–26. doi: 10.1095/biolreprod57.1.16
El-Zein, G., Boujard, D., Garnier, D. H., and Joly, J. (1988). The dynamics of the steroidogenic response of perifused Xenopus ovarian explants to gonadotropins. Gen. Comp. Endocrinol. 71, 132–140. doi: 10.1016/0016-6480(88)90304-8
Eppig, J. J. (2001). Oocyte control of ovarian follicular development and function in mammals. Reproduction 122, 829–838. doi: 10.1530/rep.0.1220829
Espey, L. L. (1967). Ultrastructure of the apex of the rabbit graafian follicle during the ovulatory process. Endocrinology 81, 267–276. doi: 10.1210/endo-81-2-267
Espey, L. L. (1974). Ovarian proteolytic enzymes and ovulation. Biol. Reprod. 10, 216–235. doi: 10.1095/biolreprod10.2.216
Espey, L. L., Adams, R. F., Tanaka, N., and Okamura, H. (1990). Effects of epostane on ovarian levels of progesterone, 17 beta-estradiol, prostaglandin E2, and prostaglandin F2 alpha during ovulation in the gonadotropin-primed immature rat. Endocrinology 127, 259–263. doi: 10.1210/endo-127-1-259
Evaul, K., Jamnongjit, M., Bhagavath, B., and Hammes, S. R. (2007). Testosterone and progesterone rapidly attenuate plasma membrane Gbetagamma-mediated signaling in Xenopus laevis oocytes by signaling through classical steroid receptors. Mol. Endocrinol. 21, 186–196. doi: 10.1210/me.2006-0301
Fan, H. Y., Liu, Z., Johnson, P. F., and Richards, J. S. (2011). CCAAT/enhancer-binding proteins (C/EBP)-α and -β?. are essential for ovulation, luteinization, and the expression of key target genes. Mol. Endocrinol. 25, 253–268. doi: 10.1210/me.2010-0318
Fan, H. Y., Liu, Z., Shimada, M., Sterneck, E., Johnson, P. F., Hedrick, S. M., et al. (2009). MAPK3/1 (ERK1/2) in ovarian granulosa cells are essential for female fertility. Science 324, 938–941. doi: 10.1126/science.1171396
Ferrell, J. E. Jr. (1999). Building a cellular switch: more lessons from a good egg. Bioessays 21, 866–870. doi: 10.1002/(sici)1521-1878(199910)21:10<866::aid-bies9>3.0.co;2-1
Ferrell, J. E. Jr. (2002). Self-perpetuating states in signal transduction: positive feedback, double-negative feedback and bistability. Curr. Opin. Cell Biol. 14, 140–148. doi: 10.1016/s0955-0674(02)00314-9
Ferrell, J. E. Jr., and Machleder, E. M. (1998). The biochemical basis of an all-or-none cell fate switch in Xenopus oocytes. Science 280, 895–898. doi: 10.1126/science.280.5365.895
Finidori-Lepicard, J., Schorderet-Slatkine, S., Hanoune, J., and Baulieu, E. E. (1981). Progesterone inhibits membrane-bound adenylate cyclase in Xenopus laevis oocytes. Nature 292, 255–257. doi: 10.1038/292255a0
Fortune, J. E. (1983). Steroid production by Xenopus ovarian follicles at different developmental stages. Dev. Biol. 99, 502–509. doi: 10.1016/0012-1606(83)90299-3
Fortune, J. E., and Tsang, P. C. (1981). Production of androgen and estradiol-17 beta by Xenopus ovaries treated with gonadotropins in vitro. Gen. Comp. Endocrinol. 43, 234–242. doi: 10.1016/0016-6480(81)90317-8
Freeman, S. B. (1968). A study of the jelly envelopes surrounding the egg of the amphibian, Xenopus laevis. Biol. Bull. 135, 501–513. doi: 10.2307/1539712
Freudzon, L., Norris, R. P., Hand, A. R., Tanaka, S., Saeki, Y., Jones, T. L., et al. (2005). Regulation of meiotic prophase arrest in mouse oocytes by GPR3, a constitutive activator of the Gs G protein. J. Cell Biol. 171, 255–265. doi: 10.1083/jcb.200506194
Furuno, N., Nishizawa, M., Okazaki, K., Tanaka, H., Iwashita, J., Nakajo, N., et al. (1994). Suppression of DNA replication via Mos function during meiotic divisions in Xenopus Oocytes. EMBO J. 13, 2399–2410. doi: 10.1002/j.1460-2075.1994.tb06524.x
Gallo, C. J., Hand, A. R., Jones, T. L., and Jaffe, L. A. (1995). Stimulation of Xenopus oocyte maturation by inhibition of the G-protein alpha S subunit, a component of the plasma membrane and yolk platelet membranes. J. Cell Biol. 130, 275–284. doi: 10.1083/jcb.130.2.275
Gerard, N., and Robin, E. (2019). Cellular and molecular mechanisms of the preovulatory follicle differenciation and ovulation: what do we know in the mare relative to other species. Theriogenology 130, 163–176. doi: 10.1016/j.theriogenology.2019.03.007
Gharbi-Ayachi, A., Labb, J. C., Burgess, A., Vigneron, S., and Strub, J. M. B. (2010). The substrate of Greatwall kinase, Arpp19, controls mitosis, by, inhibiting, protein, phosphatase, 2A. Science 330, 1673–1677. doi: 10.1126/science.1197048
Gotoh, Y., Nishida, E., Matsuda, S., Shiina, N., Kosako, H., Shiokawa, K., et al. (1991). In vitro effects on microtubule dynamics of purified Xenopus M phase-activated MAP Kinase. Nature 349, 251–254. doi: 10.1038/349251a0
Granot, I., and Dekel, N. (1994). Phosphorylation and expression of connexin-43 ovarian gap junction protein are regulated by luteinizing hormone. J. Biol. Chem. 269, 30502–30509.
Gross, S. D., Schwab, M. S., Taieb, F. E., Lewellyn, A. L., Qian, Y. W., and Maller, J. L. (2000). The Critical Role of the MAP Kinase Pathway in Meiosis II in Xenopus Oocytes Is Mediated by p90(Rsk). Curr. Biol. 10, 430–438. doi: 10.1016/s0960-9822(00)00425-5
Gyles, S. L., Burns, C. J., Whitehouse, B. J., Sugden, D., Marsh, P. J., Persaud, S. J., et al. (2001). ERKs regulate cyclic AMP-induced steroid synthesis through transcription of the steroidogenic acute regulatory (StAR) gene. J. Biol. Chem. 276, 34888–34895. doi: 10.1074/jbc.M102063200
Haas, D., White, S. N., Lutz, L. B., Rasar, M., and Hammes, S. R. (2005). The modulator of nongenomic actions of the estrogen receptor (MNAR) regulates transcription-independent androgen receptor-mediated signaling: evidence that MNAR participates in G protein-regulated meiosis in Xenopus laevis oocytes. Mol. Endocrinol. 19, 2035–2046. doi: 10.1210/me.2004-0531
Haccard, O., Dupr, A., Lier, P., Pianos, A., Eychenne, B., Jessus, C., et al. (2012). Naturally occurring steroids in Xenopus oocyte during meiotic maturation. Unexpected presence and role of steroid sulfates. Mol. Cell. Endocrinol. 362, 110–119. doi: 10.1016/j.mce.2012.05.019
Hajnik, C. A., Goetz, F. W., Hsu, S. Y., and Sokal, N. (1998). Characterization of a ribonucleic acid transcript from the brook trout (Salvelinus fontinalis) ovary with structural similarities to mammalian adipsin/complement factor D and tissue kallikrein, and the effects of kallikrein-like serine proteases on follicle contraction. Biol. Reprod. 58, 887–897. doi: 10.1095/biolreprod58.4.887
Hammes, S. R. (2004). Steroids and oocyte maturation a new look at an old story. Mol. Endocrinol. 18, 769–775. doi: 10.1210/me.2003-0317
Hara, M., Abe, Y., Tanaka, T., Yamamoto, T., Okumura, E., and Kishimoto, T. (2012). Greatwall kinase andcyclin B-Cdk1 are both critical constituents of M-phase-promoting factor. Nat. Commun. 3:1059. doi: 10.1038/ncomms2062
Heasman, J., Holwill, S., and Wylie, C. C. (1991). Fertilization of cultured Xenopus oocytes and use in studies of maternally inherited molecules. Methods Cell Biol. 36, 213–230. doi: 10.1016/s0091-679x(08)60279-4
Heilbrunn, I. V., Daugherty, K., and Wilbur, K. M. (1939). Initiation of maturation in the frog eggs. Physiol Zool. 1939, 97–100. doi: 10.1086/physzool.12.2.30151487
Hibbert, M. L., Stouffer, R. L., Wolf, D. P., and Zelinski-Wooten, M. B. (1996). Midcycle administration of a progesterone synthesis inhibitor prevents ovulation in primates. Proc. Natl. Acad. Sci. U.S.A. 93, 1897–1901. doi: 10.1073/pnas.93.5.1897
Hikabe, O., Hamazaki, N., Nagamatsu, G., Obata, Y., Hirao, Y., Hamada, N., et al. (2016). Reconstitution in vitro of the entire cycle of the mouse female germ line. Nature 539, 299–303. doi: 10.1038/nature20104
Hinckley, M., Vaccari, S., Horner, K., Chen, R., and Conti, M. (2005). The G-protein-coupled receptors GPR3 and GPR12 are involved in cAMP signaling and maintenance of meiotic arrest in rodent oocytes. Dev. Biol. 287, 249–261. doi: 10.1016/j.ydbio.2005.08.019
Howard, E. L., Charlesworth, A., Welk, J., and MacNicol, A. M. (1999). The Mitogen-Activated Protein Kinase Signaling Pathway Stimulates Mos mRNA Cytoplasmic Polyadenylation During Xenopus Oocyte Maturation. Mol. Cell. Biol. 19, 1990–1999. doi: 10.1128/mcb.19.3.1990
Huchon, D., Ozon, R., Fischer, E. H., and Demaille, J. G. (1981). The pure inhibitor of cAMP-dependent protein kinase initiates Xenopus laevis meiotic maturation, A 4-step scheme for meiotic maturation. Mol. Cell. Endocrinol. 22, 211–222. doi: 10.1016/0303-7207(81)90092-7
Hunt, T. (1989). Maturation promoting factor, cyclin and the control of M-phase. Curr. Opin. Cell Biol. 1, 268–274. doi: 10.1016/0955-0674(89)90099-9
Iguchi, S., Iwasaki, T., Fukami, Y., and Tokmakov, A. A. (2013). Unlaid Xenopus eggs degrade by apoptosis in the genital tract. BMC Cell Biol. 14:11. doi: 10.1186/1471-2121-14-11
Inoue, D., Ohe, M., Kanemori, Y., Nobui, T., and Sagata, N. (2007). A direct link of the Mos MAPK pathwayto Erp1/Emi2 in meiotic arrest of Xenopus laevis eggs. Nature 446, 1100–1104. doi: 10.1038/nature05688
Iwabuchi, M., Ohsumi, K., Yamamoto, T. M., Sawada, W., and Kishimoto, T. (2000). Residual Cdc2 activity remaining at meiosis I exit is essential for meiotic M M transition in Xenopus oocyte extracts. EMBO J. 19, 4513–4523. doi: 10.1093/emboj/19.17.4513
Jacobelli, S., Hanocq, J., Baltus, E., and Brachet, J. (1974). Hormone-induced maturation of Xenopus laevis oocytes: effects of dierent steroids and study of the properties of a progesterone receptor. Differentiation 2, 129–135. doi: 10.1111/j.1432-0436.1974.tb00346.x
Jaffe, L. A., and Egbert, J. R. (2017). Regulation of Mammalian Oocyte Meiosis by Intercellular Communication Within the Ovarian Follicle. Annu. Rev. Physiol. 79, 237–260. doi: 10.1146/annurev-physiol-022516-034102
Jamnongjit, M., Gill, A., and Hammes, S. R. (2005). Epidermal growth factor receptor signaling is required for normal ovarian steroidogenesis and oocyte maturation. Proc. Natl. Acad. Sci. U.S.A. 102, 16257–16261. doi: 10.1073/pnas.0508521102
Jamnongjit, M., and Hammes, S. R. (2006). Ovarian steroids: the good, the bad, and the signals that raise them. Cell Cycle 5, 1178–1183. doi: 10.4161/cc.5.11.2803
Jesam, C., Cochon, L., Salvatierra, A. M., Williams, A., Kapp, N., Levy-Gompel, D., et al. (2016). A prospective, open-label, multicenter study to assess the pharmacodynamics and safety of repeated use of30 mg ulipristal acetate. Contraception 93, 310–316. doi: 10.1016/j.contraception.2015.12.015
Johnson, M. T., Freeman, E. A., Gardner, D. K., and Hunt, P. A. (2007). Oxidative metabolism of pyruvate is required for meiotic maturation of murine oocytes in vivo. Biol. Reprod. 77, 2–8. doi: 10.1095/biolreprod.106.059899
Josefsberg Ben-Yehoshua, L., Lewellyn, A. L., Thomas, P., and Maller, J. L. (2007). The role of Xenopus membrane progesterone receptor beta in mediating the effect of progesterone on oocyte maturation. Mol. Endocrinol. 21, 664–673. doi: 10.1210/me.2006-0256
Jurek, B., and Neumann, I. D. (2018). The Oxytocin Receptor: from intracellular signaling to behavior. Physiol. Rev. 98, 1805–1908. doi: 10.1152/physrev.00031.2017
Klangnurak, W., and Tokumoto, T. (2017). Fine selection of up-regulated genes during ovulation by in vivo induction of oocyte maturation and ovulation in zebrafish. Zool. Lett. 3:2. doi: 10.1186/s40851-017-0065-8
Kloc, M., Miller, M., Carrasco, A. E., Eastman, E., and Etkin, L. (1989). The maternal store of the xlgv7 mRNA in full-grown Oocytes is not required for normal development in Xenopus. Development 107, 899–907.
Knapp, E. M., Deady, L. D., and Sun, J. (2018). Ex vivo Follicle Rupture and in situ Zymography in Drosophila. Bio Protoc. 8:e2846. doi: 10.21769/BioProtoc.2846
Knapp, E. M., and Sun, J. (2017). Steroid signaling in mature follicles is important for Drosophila ovulation. Proc. Natl. Acad. Sci. U.S.A. 114, 699–704. doi: 10.1073/pnas.1614383114
Konduktorova, V. V., and Luchinskaia, N. N. (2013). Follicular cells of the amphibian ovary: origin. Struct. Funct. Ontogenez 44, 316–330.
Kumagai, A., and Dunphy, W. G. (1996). Purification and molecular cloning of Plx1, a Cdc25-regulatory kinase from Xenopus egg extracts. Science 273, 1377–1380. doi: 10.1126/science.273.5280.1377
Kwon, H. B., Chang, K. J., Yoo, Y. R., Lee, C. C., and Schuetz, A. W. (1992). Induction of Ovulation and Oocyte Maturation of Amphibian (Rana Dybowskii) Ovarian Follicles by Protein Kinase C Activation in Vitro. Biol. Reprod. 47, 169–176. doi: 10.1095/biolreprod47.2.169
Kwon, H. B., Park, H. J., and Schuetz, A. W. (1990). Induction and inhibition of meiotic maturation of amphibian (Rana dybowskii) follicular oocytes by forskolin and cAMP in vitro. Mol. Reprod. Dev. 25, 147–154. doi: 10.1002/mrd.1080250207
Kwon, H. B., and Schuetz, A. W. (1986). Role of cAMP in modulating intrafollicular progesterone levels and oocyte maturation in amphibians (Rana pipiens). Dev. Biol. 117, 354–364. doi: 10.1016/0012-1606(86)90305-2
Lavin, L. H. (1964). The transfer of coelomic eggs between frogs. J. Embryol. Exp. Morphol. 12, 457–463.
Law, N. C., Donaubauer, E. M., Zeleznik, A. J., and Hunzicker-Dunn, M. (2017). How protein kinase a activates canonical tyrosine kinase signaling pathways to promote granulosa cell differentiation. Endocrinology 158, 2043–2051. doi: 10.1210/en.2017-00163
Lieberman, M. E., Tsafriri, A., Bauminger, S., Collins, W. P., Ahren, K., and Lindner, H. R. (1976). Oocyticmeiosis in cultured rat follicles during inhibition of steroidogenesis. Acta Endocrinol. (Copenh) 83, 151–157. doi: 10.1530/acta.0.0830151
Lim, H., Paria, B. C., Das, S. K., Dinchuk, J. E., Langenbach, R., Trzaskos, J. M., et al. (1997). Multiple female reproductive failures in cyclooxygenase 2-deficient Mice. Cell 91, 197–208. doi: 10.1016/s0092-8674(00)80402-x
Liu, D. T., Brewer, M. S., Chen, S., Hong, W., and Zhu, Y. (2017). Transcriptomic signatures for ovulation in vertebrates. Gen. Comp. Endocrinol. 247, 74–86. doi: 10.1016/j.ygcen.2017.01.019
Liu, X. S., Ma, C., Hamam, A. W., and Liu, X. J. (2005). Transcription-dependent and transcription-independent functions of the classical progesterone receptor in Xenopus ovaries. Dev. Biol. 283, 180–190. doi: 10.1016/j.ydbio.2005.04.011
Liu, Y. X., Liu, K., Feng, Q., Hu, Z. Y., Liu, H. Z., Fu, G. Q., et al. (2004). Tissue-type plasminogen activator and its inhibitor plasminogen activator inhibitor type 1 are coordinately expressed during ovulation in the rhesus monkey. Endocrinology 145, 1767–1775. doi: 10.1210/en.2003-1327
Lutz, L. B., Cole, L. M., Gupta, M. K., Kwist, K. W., Auchus, R. J., and Hammes, S. R. (2001). Evidence that androgens are the primary steroids produced by Xenopus laevis ovaries and may signal through the classical androgen receptor to promote oocyte maturation. Proc. Natl. Acad. Sci. U.S.A. 98, 13728–13733. doi: 10.1073/pnas.241471598
Lutz, L. B., Jamnongjit, M., Yang, W. H., Jahani, D., Gill, A., and Hammes, S. R. (2003). Selective modulation of genomic and nongenomic androgen responses by androgen receptor ligands. Mol. Endocrinol. 17, 1106–1116. doi: 10.1210/me.2003-0032
Lutz, L. B., Kim, B., Jahani, D., and Hammes, S. R. (2000). G protein beta gamma subunits inhibit nongenomic progesterone-induced signaling and maturation in Xenopus laevis oocytes, Evidence for a release of inhibition mechanism for cell cycle progression. J. Biol. Chem. 275, 41512–41520. doi: 10.1074/jbc.M006757200
Lydon, J. P., DeMayo, F. J., Funk, C. R., Mani, S. K., Hughes, A. R., Montgomery, C. A., et al. (1995). Mice lacking progesterone receptor exhibit pleiotropic reproductive abnormalities. Genes Dev. 9, 2266–2278. doi: 10.1101/gad.9.18.2266
Maller, J. L., and Krebs, E. G. (1977). Progesterone-stimulated meiotic cell division in Xenopus oocytes. Induction by regulatory subunit and inhibition by catalytic subunit of adenosine 3’:5’-monophosphate-dependent protein kinase. J. Biol. Chem. 252, 1712–1718.
Manna, P. R., Dyson, M. T., and Stocco, D. M. (2009). Regulation of the steroidogenic acute regulatory protein gene expression: present and future perspectives. Mol. Hum. Reprod. 15, 321–333. doi: 10.1093/molehr/gap025
Manna, P. R., Huhtaniemi, I. T., Wang, X. J., Eubank, D. W., and Stocco, D. M. (2002). Mechanisms of epidermal growth factor signaling: regulation of steroid biosynthesis and the steroidogenic acute regulatory protein in mouse Leydig tumor cells. Biol. Reprod. 67, 1393–1404. doi: 10.1095/biolreprod.102.007179
Manna, P. R., and Stocco, D. M. (2011). The Role of Specific Mitogen-Activated Protein Kinase Signaling Cascades in the Regulation of Steroidogenesis. J. Signal Transduct. 22011:821615. doi: 10.1155/2011/821615
Marshall, I. C., and Wilson, K. L. (1997). Nuclear Envelope Assembly After Mitosis. Trends Cell Biol. 7, 69–74. doi: 10.1016/S0962-8924(96)10047-7
Martinez, S., Pasten, P., Suarez, K., Garcia, A., Nualart, F., Montecino, M., et al. (2007). Classical Xenopus laevis progesterone receptor associates to the plasma membrane through its ligand-binding domain. J. Cell. Physiol. 211, 560–567. doi: 10.1002/jcp.20964
Masui, Y. (1967). Relative roles of the pituitary, follicle cells, and progesterone in the induction of oocyte maturation in Rana pipiens. J. Exp. Zool. 166, 365–375. doi: 10.1002/jez.1401660309
Masui, Y., and Clarke, H. J. (1979). Oocyte maturation. Int. Rev. Cytol. 57, 185–282. doi: 10.1016/s0074-7696(08)61464-3
Masui, Y., and Markert, C. L. (1971). Cytoplasmic control of nuclear behavior during meiotic maturation of frog oocytes. J. Exp. Zool. 177, 129–145. doi: 10.1002/jez.1401770202
Matten, W. T., Copeland, T. D., Ahn, N. G., and Vande Woude, G. F. (1996). Positive feedback between MAP kinase and Mos during Xenopus oocyte maturation. Dev. Biol. 179, 485–492. doi: 10.1006/dbio.1996.0277
Matzuk, M. M., Burns, K. H., Viveiros, M. M., and Eppig, J. J. (2002). Intercellular communication in the mammalian ovary: oocytes carry the conversation. Science 296, 2178–2180. doi: 10.1126/science.1071965
McCann, N. C., Lynch, T. J., Kim, S. O., and Duffy, D. M. (2013). The COX-2 inhibitor meloxicam prevents pregnancy when administered as an emergency contraceptive to nonhuman primates. Contraception 88, 744–748. doi: 10.1016/j.contraception.2013.09.006
Mehlmann, L. M., Saeki, Y., Tanaka, S., Brennan, T. J., Evsikov, A. V., Pendola, F. L., et al. (2004). The Gs-linked receptor GPR3 maintains meiotic arrest in mammalian oocytes. Science 306, 1947–1950. doi: 10.1126/science.1103974
Mendez, R., Hake, L. E., Andresson, T., Littlepage, L. E., Ruderman, J. V., and Richter, J. D. (2000). Phosphorylation of CPE binding factor by Eg2 regulates translation of c-mos mRNA. Nature 404, 302–307. doi: 10.1038/35005126
Meneau, F., Dupré, A., Jessus, C., and Daldello, E. M. (2020). Translational control of Xenopus Oocyte Meiosis: toward the Genomic Era. Cells 9:1502. doi: 10.3390/cells9061502
Miedlich, S. U., Taya, M., Young, M. R., and Hammes, S. R. (2017). Paxillin and embryonic polyAdenylation binding protein (ePABP) engage to regulate androgen-dependent Xenopus laevis oocyte maturation - A model of kinase-dependent regulation of protein expression. Mol. Cell. Endocrinol. 448, 87–97. doi: 10.1016/j.mce.2017.03.028
Migone, F. F., Cowan, R. G., Williams, R. M., Gorse, K. J., Zipfel, W. R., and Quirk, S. M. (2016). In vivo imaging reveals an essential role of vasoconstriction in rupture of the ovarian follicle at ovulation. Proc. Natl. Acad. Sci. U.S.A. 113, 2294–2299. doi: 10.1073/pnas.1512304113
Mir, A., and Heasman, J. (2008). How the mother can help: studying maternal Wnt signaling by anti-sense-mediated depletion of maternal mRNAs and the host transfer technique. Methods Mol. Biol. 469, 417–429. doi: 10.1007/978-1-60327-469-2_26
Mochida, S., Maslen, S. L., Skehel, M., and Hunt, T. (2010). Greatwall phosphorylates an inhibitor of protein phosphatase 2A that is essential for mitosis. Science 330, 1670–1673. doi: 10.1126/science.1195689
Morohaku, K., Tanimoto, R., Sasaki, K., Kawahara-Miki, R., Kono, T., Hayashi, K., et al. (2016). Complete in vitro generation of fertile oocytes from mouse primordial germ cells. Proc. Natl. Acad. Sci. U.S.A. 113, 9021–9026. doi: 10.1073/pnas.1603817113
Mueller, P. R., Coleman, T. R., and Dunphy, W. G. (1995a). Cell cycle regulation of a Xenopus Wee1-like kinase. Mol. Biol. Cell 6, 119–134. doi: 10.1091/mbc.6.1.119
Mueller, P. R., Coleman, T. R., Kumagai, A., and Dunphy, W. G. (1995b). Myt1: a membrane-associated inhibitory kinase that phosphorylates Cdc2 on both threonine-14 and tyrosine-15. Science 270, 86–90. doi: 10.1126/science.270.5233.86
Munakata, A., and Kobayashi, M. (2010). Endocrine control of sexual behavior in teleost fish. Gen. Comp. Endocrinol. 165, 456–468. doi: 10.1016/j.ygcen.2009.04.011
Murdoch, W. J., and McCormick, R. J. (1992). Enhanced degradation of collagen within apical vs. basal wall of ovulatory ovine follicle. Am. J. Physiol. 263, 221–225. doi: 10.1152/ajpendo.1992.263.2.E221
Murray, A. W., Solomon, M. J., and Kirschner, M. W. (1989). The role of cyclin synthesis and degradation in the control of maturation promoting factor activity. Nature 339, 280–286. doi: 10.1038/339280a0
Nakajo, N., Oe, T., Uto, K., and Sagata, N. (1999). Involvement of Chk1 kinase in prophase I arrest of Xenopus oocytes. Dev. Biol. 207, 432–444. doi: 10.1006/dbio.1998.9178
Nakajo, N., Yoshitome, S., Iwashita, J., Iida, M., Uto, K., Ueno, S., et al. (2000). Absence of Wee1 ensures the meiotic cell cycle in Xenopus oocytes. Genes Dev. 14, 328–338.
Niringiyumukiza, J. D., Cai, H., and Xiang, W. (2018). Prostaglandin E2 involvement in mammalian female fertility: ovulation, fertilization, embryo development and early implantation. Reprod. Biol. Endocrinol. 16:43. doi: 10.1186/s12958-018-0359-5
Nishiyama, T., Ohsumi, K., and Kishimoto, T. (2007). Phosphorylation of Erp1 by p90rsk is required for cytostatic factor arrest in Xenopus laevis eggs. Nature 446, 1096–1099. doi: 10.1038/nature05696
Norris, R. P., Freudzon, M., Mehlmann, L. M., Cowan, A. E., Simon, A. M., Paul, D. L., et al. (2008). Luteinizing hormone causes MAP kinase-dependent phosphorylation and closure of connexin 43 gap junctions in mouse ovarian follicles: one of two paths to meiotic resumption. Development 135, 3229–3238. doi: 10.1242/dev.025494
Norris, R. P., Freudzon, M., Nikolaev, V. O., and Jaffe, L. A. (2010). Epidermal growth factor receptor kinase activity is required for gap junction closure and for part of the decrease in ovarian follicle cGMP in response to LH. Reproduction 140, 655–662. doi: 10.1530/REP-10-0288
Norris, R. P., Ratzan, W. J., Freudzon, M., Mehlmann, L. M., Krall, J., Movsesian, M. A., et al. (2009). Cyclic GMP from the surrounding somatic cells regulates cyclic AMP and meiosis in the mouse oocyte. Development 136, 1869–1878. doi: 10.1242/dev.035238
Ny, T., Wahlberg, P., and Brändström, I. J. (2002). Matrix remodeling in the ovary: regulation and functional role of the plasminogen activator and matrix metalloproteinase systems. Mol. Cell. Endocrinol. 187, 29–38. doi: 10.1016/s0303-7207(01)00711-0
Ogiwara, K., Hagiwara, A., Rajapakse, S., and Takahashi, T. (2015). The role of urokinase plasminogen activator and plasminogen activator inhibitor-1 in follicle rupture during ovulation in the teleost medaka. Biol. Reprod. 92:10. doi: 10.1095/biolreprod.114.121442
Ogiwara, K., Ikeda, T., and Takahashi, T. (2010). A new in vitro ovulation model for medaka based on whole ovary culture. Zool. Sci. 27, 762–767. doi: 10.2108/zsj.27.762
Ogiwara, K., Minagawa, K., Takano, N., Kageyama, T., and Takahashi, T. (2012). Apparent involvement of plasmin in early-stage follicle rupture during ovulation in medaka. Biol. Reprod. 86:113. doi: 10.1095/biolreprod.111.093880
Ogiwara, K., and Takahashi, T. (2019). Nuclear progestin receptor phosphorylation by Cdk9 is required for the expression of Mmp15, a protease indispensable for ovulation in medaka. Cells 8:215. doi: 10.3390/cells8030215
Ogiwara, K., Takano, N., Shinohara, M., Murakami, M., and Takahashi, T. (2005). Gelatinase A and membrane-type matrix metalloproteinases 1 and 2 are responsible for follicle rupture during ovulation in the medaka. Proc. Natl. Acad. Sci. U.S.A. 102, 8442–8447. doi: 10.1073/pnas.0502423102
Ohnishi, J., Ohnishi, E., Shibuya, H., and Takahashi, T. (2005). Functions for proteinases in the ovulatory process. Biochim. Biophys. Acta 1751, 95–109. doi: 10.1016/j.bbapap.2005.05.002
Olson, J. H., and Chandler, D. E. (1999). Xenopus laevis egg jelly contains small proteins that are essential to fertilization. Dev. Biol. 210, 401–410. doi: 10.1006/dbio.1999.9281
Palanisamy, G. S., Cheon, Y. P., Kim, J., Kannan, A., Li, Q., Sato, M., et al. (2006). A novel pathway involving progesterone receptor, endothelin-2, and endothelin receptor B controls ovulation in mice. Mol. Endocrinol. 20, 2784–2795. doi: 10.1210/me.2006-0093
Palmer, A., Gavin, A. C., and Nebreda, A. R. (1998). A link between MAP kinase and p34(cdc2)/cyclin B during oocyte maturation: p90(rsk) phosphorylates and inactivates the p34(cdc2) inhibitory kinase Myt1. EMBO J. 17, 5037–5047. doi: 10.1093/emboj/17.17.5037
Paris, J., Swenson, K., Piwnica-Worms, H., and Richter, J. D. (1991). Maturation-specific polyadenylation: in vitro activation by p34cdc2 and phosphorylation of a 58-kD CPE-binding protein. Genes Dev. 5, 1697–1708. doi: 10.1101/gad.5.9.1697
Park, J. Y., Su, Y. Q., Ariga, M., Law, E., Jin, S. L., and Conti, M. (2004). EGF-like growth factors as mediators of LH action in the ovulatory follicle. Science 303, 682–684. doi: 10.1126/science.1092463
Park, O. K., and Mayo, K. E. (1991). Transient expression of progesterone receptor messenger RNA in ovarian granulosa cells after the preovulatory luteinizing hormone surge. Mol. Endocrinol. 5, 967–978. doi: 10.1210/mend-5-7-967
Peluffo, M. C., Murphy, M. J., Baughman, S. T., Stouffer, R. L., and Hennebold, J. D. (2011). Systematic analysis of protease gene expression in the rhesus macaque ovulatory follicle: metalloproteinase involvement in follicle rupture. Endocrinology 152, 3963–3974. doi: 10.1210/en.2011-1172
Peter, M., Labbe, J.-C., Doree, M., and Mandart, E. (2002). A new role for Mos in Xenopus oocyte maturation: targeting Myt1 independently of MAPK. Development 129, 2129–2139.
Petrino, T., and Schuetz, A. W. (1987). Cholesterol mediation of progesterone production and oocyte maturation in cultured amphibian (Rana pipiens) ovarian follicles. Biol. Reprod. 36, 1219–1228. doi: 10.1095/biolreprod36.5.1219
Picard, A., Galas, S., Peaucellier, G., and Doree, M. (1996). Newly assembled cyclin B-cdc2 kinase is required to suppress DNA replication between meiosis I and meiosis II in starfish oocytes. EMBO J. 15, 3590–3598. doi: 10.1002/j.1460-2075.1996.tb00728.x
Picton, H. M., Danfour, M. A., Harris, S. E., Chambers, E. L., and Huntriss, J. (2003). Growth and maturation of oocytes in vitro. Reprod. Suppl. 61, 445–462.
Prochazka, R., and Blaha, M. (2015). Regulation of mitogen-activated protein kinase 3/1 activity during meiosis resumption in mammals. J. Reprod. Dev. 61, 495–502. doi: 10.1262/jrd.2015-069
Qian, Y. W., Erikson, E., Li, C., and Maller, J. L. (1998a). Activated polo-like kinase Plx1 is required at multiple points during mitosis in Xenopus laevis. Mol. Cell. Biol. 18, 4262–4271. doi: 10.1128/mcb.18.7.4262
Qian, Y. W., Erikson, E., and Maller, J. L. (1998b). Purification and cloning of a protein kinase that phosphorylates and activates the polo-like kinase Plx1. Science 282, 1701–1704. doi: 10.1126/science.282.5394.1701
Ramos, I., Cisint, S. B., Crespo, C. A., Medina, M. F., and Fernandez, S. N. (2008). Modulators of Bufo arenarum ovulation. Zygote 16, 65–72. doi: 10.1017/S0967199407004510
Reich, R., Daphna-Iken, D., Chun, S. Y., Popliker, M., Slager, R., Adelmann-Grill, B. C., et al. (1991). Preovulatory changes in ovarian expression of collagenases and tissue metalloproteinase inhibitor messenger ribonucleic acid: role of eicosanoids. Endocrinology 129, 1869–1875. doi: 10.1210/endo-129-4-1869
Reich, R., Tsafriri, A., and Mechanic, G. L. (1985). The involvement of collagenolysis in ovulation in the rat. Endocrinology 1985, 522–527. doi: 10.1210/endo-116-2-522
Reynhout, J. K., Taddei, C., Smith, L. D., and LaMarca, M. J. (1975). Response of large oocytes of Xenopus laevis to progesterone in vitro in relation to oocyte size and time after previous HCG-induced ovulation. Dev. Biol 44, 375–379. doi: 10.1016/0012-1606(75)90408-x
Richards, J. S., and Ascoli, M. (2018). Endocrine, paracrine, and autocrine signaling pathways that regulate ovulation. Trends Endocrinol. Metab. 29, 313–325. doi: 10.1016/j.tem.2018.02.012
Rios-Cardona, D., Ricardo-Gonzalez, R. R., Chawla, A., and Ferrell, J. E. Jr. (2008). A role for GPRx, a novel GPR3/6/12-related G-protein coupled receptor, in the maintenance of meiotic arrest in Xenopus laevis oocytes. Dev. Biol. 317, 380–388. doi: 10.1016/j.ydbio.2008.02.047
Robker, R. L., Hennebold, J. D., and Russell, D. L. (2018). Coordination of ovulation and Oocyte maturation: a good egg at the right time. Endocrinology 159, 3209–3218. doi: 10.1210/en.2018-00485
Robker, R. L., Russell, D. L., Espey, L. L., Lydon, J. P., O’Malley, B. W., and Richards, J. S. (2000). Progesterone-regulated genes in the ovulation process: ADAMTS-1 and cathepsin L proteases. Proc. Natl. Acad. Sci. U.S.A. 97, 4689–4694. doi: 10.1073/pnas.080073497
Rondell, P. (1970). Biological aspects of ovulation. Biol. Reprod. Suppl. 2, 64–89. doi: 10.1095/biolreprod2.supplement_2.64
Russel, D. L., and Robker, R. L. (2007). Molecular mechanisms of ovulation: co-ordination through the cumulus complex. Hum. Reprod. Update 13, 289–312. doi: 10.1093/humupd/dml062
Sagata, N., Watanabe, N., Vande Woude, G. F., and Ikawa, Y. (1989). The C-Mos proto-oncogene product is a cytostatic factor responsible for meiotic arrest in vertebrate eggs. Nature 342, 512–518. doi: 10.1038/342512a0
Sato, K.-I., and Tokmakov, A. A. (2020). Toward the understanding of biology of oocyte life cycle in Xenopus Laevis: No oocytes left behind. Reprod. Med. Biol. 19, 114–119. doi: 10.1002/rmb2.12314
Schmidt, A., Rauh, N. R., Nigg, E. A., and Mayer, T. U. (2006). Cytostatic factor: an activity that puts the cell cycle on hold. J. Cell Sci. 119, 1213–1218. doi: 10.1242/jcs.02919
Schmidt, R. S. (1984). Mating call phonotaxis in the female American toad: induction by hormones. Gen. Comp. Endocrinol. 55, 150–156. doi: 10.1016/0016-6480(84)90139-4
Schochet, S. S. (1916). A suggestion as to the process of ovulation and ovarian cyst formation. Anatom. Rec. 10, 447–457. doi: 10.1002/ar.1090100605
Schorderet-Slatkine, S., Schorderet, M., Boquet, P., Godeau, F., and Baulieu, E. E. (1978). Progesterone-induced meiosis in Xenopus laevis oocytes: a role for cAMP at the “maturation-promoting factor” level. Cell 15, 1269–1275. doi: 10.1016/0092-8674(78)90052-1
Schuetz, A. W. (1967). Effect of steroids on germinal vesicle of oocytes of the frog (Rana pipiens) in vitro. Proc. Soc. Exp. Biol. Med. 124, 1307–1310. doi: 10.3181/00379727-124-31993
Schuetz, A. W. (1986). Hormonal dissociation of ovulation and maturation of oocytes: ovulation of immature amphibian oocytes by prostaglandin. Gamete Res 15, 99–113. doi: 10.1002/mrd.1120150202
Schuetz, A. W., and Lessman, C. (1982). Evidence for follicle wall involvement in ovulation and progesterone production by frog (Rana pipiens) follicles in vitro. Differentiation 22, 79–84. doi: 10.1111/j.1432-0436.1982.tb01229.x
Schutt, B., Schultze-Mosgau, M. H., Draeger, C., Chang, X., Lowen, S., Kaiser, A., et al. (2018). Effect of the novel selective progesterone receptor modulator vilaprisan on ovarian activity in healthy women. J. Clin. Pharmacol. 58, 228–239. doi: 10.1002/jcph.998
Schwab, M. S., Roberts, B. T., Gross, S. D., Tunquist, B. J., Taieb, F. E., Lewellyn, A. L., et al. (2001). Bub1 is activated by the protein kinase p90 (Rsk) during Xenopus oocyte maturation. Curr. Biol. 11, 141–150. doi: 10.1016/s0960-9822(01)00045-8
Sela-Abramovich, S., Edry, I., Galiani, D., Nevo, N., and Dekel, N. (2006). Disruption of gap junctional communication within the ovarian follicle induces oocyte maturation. Endocrinology 147, 2280–2286. doi: 10.1210/en.2005-1011
Sen, A., Prizant, H., and Hammes, S. R. (2011). Understanding extranuclear (nongenomic) androgen signaling: what a frog oocyte can tell us about human biology. Steroids 76, 822–828. doi: 10.1016/j.steroids.2011.02.016
Sena, J., and Liu, Z. (2008). Expression of cyclooxygenase genes and production of prostaglandins during ovulation in the ovarian follicles of Xenopus laevis. Gen. Comp. Endocrinol. 157, 165–173. doi: 10.1016/j.ygcen.2008.04.012
Shapiro, B. G., and Zwarenstein, H. A. (1934). A rapid test for pregnancy on Xenopus laevis. Nature 133:762. doi: 10.1038/133762a0
Sheng, Y., Montplaisir, V., and Liu, X. J. (2005). Co-operation of Gsalpha and Gbetagamma in maintaining G2 arrest in Xenopus oocytes. J. Cell. Physiol. 202, 32–40. doi: 10.1002/jcp.20084
Shibuya, E. K. (2003). G2cell cycle arrest - A direct link between PKA and Cdc25C. Cell Cycle 2, 39–41. doi: 10.4161/cc.2.1.291
Shoji, S., Yoshida, N., Amanai, M., Ohgishi, M., Fukui, T., Fujimoto, S., et al. (2006). Mammalian Emi2 mediates cytostatic arrest and transduces the signal for meiotic exit via Cdc20. EMBO J. 25, 834–845. doi: 10.1038/sj.emboj.7600953
Shuhaibar, L. C., Egbert, J. R., Norris, R. P., Lampe, P. D., Nikolaev, V. O., Thunemann, M., et al. (2015). Intercellular signaling via cyclic GMP diffusion through gap junctions restarts meiosis in mouse ovarian follicles. Proc. Natl. Acad. Sci. U.S.A. 112, 5527–5532. doi: 10.1073/pnas.1423598112
Simon, A. M., Goodenough, D. A., Li, E., and Paul, D. L. (1997). Female infertility in mice lacking connexin 37. Nature 385, 525–529. doi: 10.1038/385525a0
Simoni, M., Gromoll, J., and Nieschlag, E. (1997). The follicle-stimulating hormone receptor: biochemistry, molecular biology, physiology, and pathophysiology. Endocr. Rev. 18, 739–773. doi: 10.1210/edrv.18.6.0320
Sirois, J., Sayasith, K., Brown, K. A., Stock, A. E., Bouchard, N., and Doree, M. (2004). Cyclooxygenase-2 and its role in ovulation: a 2004 account. Hum. Reprod. Update 10, 373–385. doi: 10.1093/humupd/dmh032
Sirois, J., Simmons, D. L., and Richards, J. S. (1992). Hormonal regulation of messenger ribonucleic acid encoding a novel isoform of prostaglandin endoperoxide H synthase in rat preovulatory follicles, Induction in vivo and in vitro. J. Biol. Chem. 267, 11586–11592.
Skoblina, M. N. (2002). Effects of the antagonist of the classical progesterone receptor on ovulation and maturation of common frog (Rana Temporaria L.) oocytes in vitro. Ontogenez 33, 465–470.
Skory, R. M., Xu, Y., Shea, L. D., and Woodru, T. K. (2015). Engineering the ovarian cycle using in vitro follicle culture. Hum. Reprod. 30, 1386–1395. doi: 10.1093/humrep/dev052
Smith, L. D., and Ecker, R. E. (1969). Role of the oocyte nucleus in physiological maturation in Rana pipiens. Dev. Biol. 19, 281–309. doi: 10.1016/0012-1606(69)90065-7
Smith, L. D., and Ecker, R. E. (1970). Regulatory processes in the maturation and early cleavage of amphibian eggs. Curr. Top. Dev. Biol. 5, 1–38. doi: 10.1016/s0070-2153(08)60051-4
Smith, L. D., and Ecker, R. E. (1971). The interaction of steroids with Rana pipiens oocytes in the induction of maturation. Dev. Biol. 25, 232–247. doi: 10.1016/0012-1606(71)90029-7
Smith, L. D., Ecker, R. E., and Subtelny, S. (1968). In vitro induction of physiological maturation in Rana pipiens oocytes removed from their ovarian follicles. Dev. Biol. 17, 627–643. doi: 10.1016/0012-1606(68)90010-9
Stebbins-Boaz, B., Hake, L. E., and Richter, J. D. (1996). CPEB controls the cytoplasmic polyadenylation of cyclin, Cdk2 and c-mos mRNAs and is necessary for oocyte maturation in Xenopus. EMBO J. 15, 2582–2592. doi: 10.1002/j.1460-2075.1996.tb00616.x
Stouffer, R. L., Xu, F., and Duffy, D. M. (2007). Molecular control of ovulation and luteinization in the primate follicle. Front. Biosci. 12:297–307. doi: 10.2741/2065
Su, Y., Wu, X., O’brien, M. J., Pendola, F. L., Denegre, J. N., Matzuk, M. M., et al. (2004). Synergistic roles of BMP15 and GDF9 in the development and function of the oocyte-cumulus cell complex in mice: genetic evidence for an oocyte-granulosa cell regulatory loop. Dev. Biol. 276, 64–73. doi: 10.1016/j.ydbio.2004.08.020
Su, Y. Q., Denegre, J. M., Wigglesworth, K., Pendola, F. L., O’Brien, M. J., and Eppig, J. J. (2003). Oocyte-dependent activation of mitogen-activated protein kinase (ERK1/2) in cumulus cells is required for the maturation of the mouse oocyte-cumulus cell complex. Dev. Biol. 263, 126–138. doi: 10.1016/s0012-1606(03)00437-8
Su, Y. Q., Wigglesworth, K., Pendola, F. L., O’Brien, M. J., and Eppig, J. J. (2002). Mitogen-activated protein kinase (MAPK) activity in cumulus cells is essential for gonadotropin-induced oocyte meiotic resumption and cumulus expansion in the mouse. Endocrinology 143, 2221–2232. doi: 10.1210/endo.143.6.8845
Subtelny, S., Smith, L. D., and Ecker, R. E. (1968). Maturation of ovarian frog eggs without ovulation. J. Exp. Zool. 168, 39–47. doi: 10.1002/jez.1401680104
Takahashi, T., Fujimori, C., Hagiwara, A., and Ogiwara, K. (2013). Recent advances in the understanding of teleost medaka ovulation: the roles of proteases and prostaglandins. Zool. Sci. 30, 239–247. doi: 10.2108/zsj.30.239
Takahashi, T., Hagiwara, A., and Ogiwara, K. (2018). Prostaglandins in teleost ovulation: a review of the roles with a view to comparison with prostaglandins in mammalian ovulation. Mol. Cell. Endocrinol. 461, 236–247. doi: 10.1016/j.mce.2017.09.019
Takahashi, T., Hagiwara, A., and Ogiwara, K. (2019). Follicle Rupture During Ovulation With an Emphasis on Recent Progress in Fish Models. Reproduction 157, R1–R13.
Telfer, E. E. (2019). FERTILITY PRESERVATION: progress and prospects for developing human immature oocytes in vitro. Reproduction 158, F45–F54. doi: 10.1530/REP-19-0077
Telfer, E. E., Sakaguchi, K., Clarkson, Y. L., and McLaughlin, M. (2019). In vitro growth of immature bovine follicles and oocytes. Reprod. Fertil. Dev. 32, 1–6. doi: 10.1071/RD19270
Tokmakov, A. A., Iguchi, S., Iwasaki, T., and Fukami, Y. (2011). Unfertilized frog eggs die by apoptosis following meiotic exit. BMC Cell Biol. 12:56. doi: 10.1186/1471-2121-12-56
Tokmakov, A. A., Iguchi, S., Iwasaki, T., Fukami, Y., and Sato, K.-I. (2017). Global decay of mRNA is a hallmark of apoptosis in aging Xenopus eggs. RNA Biol. 14, 339–346. doi: 10.1080/15476286.2016.1276695
Tokmakov, A. A., Matsumoto, Y., Isobe, T., and Sato, K.-I. (2019). In Vitro Reconstruction of Xenopus Oocyte Ovulation. Int. J. Mol. Sci. 20:4766. doi: 10.3390/ijms20194766
Tokmakov, A. A., Sato, K. I., and Stefanov, V. E. (2018). Postovulatory cell death: why eggs die via apoptosis in biological species with external fertilization. J. Reprod. Dev. 64, 1–6. doi: 10.1262/jrd.2017-100
Tsafriri, A., Daphna-Iken, D., Abisogun, A. O., and Reich, R. (1990). “Follicular rupture during ovulation: activation of collagenolysis,” in Advances in Assisted Reproductive Technologies, ed. S. Mashiach (New York, NY: Plenum Press), doi: 10.1007/978-1-4613-0645-0_12
Tung, J. J., Padmanabhan, K., Hansen, D. V., Richter, J. D., and Jackson, P. K. (2007). Translational unmasking of Emi2 directs cytostatic factor arrest in meiosis II. Cell Cycle 6, 725–731. doi: 10.4161/cc.6.6.3936
Tunquist, B. J., and Maller, J. L. (2003). Under arrest: cytostatic factor (CSF)-mediated metaphase arrest in vertebrate eggs. Genes Dev. 17, 683–691. doi: 10.1101/gad.1071303
Vaccari, S., Weeks, J. L. II, Hsieh, M., Menniti, F. S., and Conti, M. (2009). Cyclic GMP signaling is involved in the luteinizing hormone-dependent meiotic maturation of mouse oocytes. Biol. Reprod. 81, 595–604. doi: 10.1095/biolreprod.109.077768
Verlhac, M. H., de Pennart, H., Maro, B., Cobb, M. H., and Clarke, H. J. (1993). MAP kinase becomes stably activated at metaphase and is associated with microtubule-organizing centers during meiotic maturation of mouse oocytes. Dev. Biol. 158, 330–340. doi: 10.1006/dbio.1993.1192
Vigneron, S., Brioudes, E., Burgess, A., Labb, J. C., Lorca, T., and Castro, A. (2009). Greatwall maintains mitosis through regulation of PP2A. EMBO J. 28, 2786–2793. doi: 10.1038/emboj.2009.228
Voss, A. K., and Fortune, J. E. (1991). Oxytocin secretion by bovine granulosa cells: effects of stage of follicular development, gonadotropins, and coculture with theca interna. Endocrinology 128, 1991–1999. doi: 10.1210/endo-128-4-1991
Witschi, E. (1952). Overripeness of the egg as a cause of twinning and teratogenesis: a review. Cancer Res. 1952, 763–786.
Wood, J. R., and Strauss, J. F. III (2002). Multiple signal transduction pathways regulate ovarian steroidogenesis. Rev. Endocr. Metab. Disord. 3, 33–46. doi: 10.1023/a:1012748718150
Wright, P. A. (1945). Factors affecting in vitro ovulation in the frog. J. Exp. Zool. 100, 565–575. doi: 10.1002/jez.1401000316
Wright, P. A. (1961). Influence of estrogens on induction of ovulation in vitro in Rana pipiens. Gen. Comp. Endocrinol. 1, 381–385. doi: 10.1016/0016-6480(61)90001-6
Wu, J. Q., and Kornbluth, S. (2008). Across the meiotic divide - CSF activity in the post-Emi2/XErp1 era. Cell Sci. 121, 3509–3514. doi: 10.1242/jcs.036855
Yamashita, Y., Shimada, M., Okazaki, T., Maeda, T., and Terada, T. (2003). Production of progesterone from de novo-synthesized cholesterol in cumulus cells and its physiological role during meiotic resumption of porcine oocytes. Biol. Reprod. 68, 1193–1198. doi: 10.1095/biolreprod.102.010934
Yang, F., Wang, W., Cetinbas, M., Sadreyev, R. I., and Blower, M. D. (2020). Genome-wide analysis identifies cis-acting elements regulating mRNA polyadenylation and translation during vertebrate oocyte maturation. RNA 26, 324–344. doi: 10.1261/rna.073247.119
Yang, W. H., Lutz, L. B., and Hammes, S. R. (2003). Xenopus laevis ovarian CYP17 is a highly potent enzyme expressed exclusively in oocytes. Evidence that oocytes play a critical role in Xenopus ovarian androgen production. J. Biol. Chem. 278, 9552–9559. doi: 10.1074/jbc.M212027200
Yu, J., Zhao, Y., Li, Z., Galas, S., and Goldberg, M. L. (2006). Greatwall kinase participates in the Cdc2 autoregulatory loop in Xenopus egg extracts. Mol. Cell. 22, 83–91. doi: 10.1016/j.molcel.2006.02.022
Zhang, M., Su, Y. Q., Sugiura, K., Xia, G., and Eppig, J. J. (2010). Granulosa cell ligand NPPC and its receptor NPR2 maintain meiotic arrest in mouse oocytes. Science 330, 366–369. doi: 10.1126/science.1193573
Zhu, Y., Bond, J., and Thomas, P. (2003). Identification, classification, and partial characterization of genes in humans and other vertebrates homologous to a fish membrane progestin receptor. Proc. Natl. Acad. Sci. U.S.A. 100, 2237–2242. doi: 10.1073/pnas.0436133100
Keywords: oocyte, ovulation, maturation, follicle rupture, in vitro
Citation: Tokmakov AA, Stefanov VE and Sato K-I (2020) Dissection of the Ovulatory Process Using ex vivo Approaches. Front. Cell Dev. Biol. 8:605379. doi: 10.3389/fcell.2020.605379
Received: 12 September 2020; Accepted: 19 November 2020;
Published: 09 December 2020.
Edited by:
Rafael A. Fissore, University of Massachusetts Amherst, United StatesReviewed by:
Matteo Avella, University of Tulsa, United StatesCopyright © 2020 Tokmakov, Stefanov and Sato. This is an open-access article distributed under the terms of the Creative Commons Attribution License (CC BY). The use, distribution or reproduction in other forums is permitted, provided the original author(s) and the copyright owner(s) are credited and that the original publication in this journal is cited, in accordance with accepted academic practice. No use, distribution or reproduction is permitted which does not comply with these terms.
*Correspondence: Alexander A. Tokmakov, dG9rbWFrQGNjLmt5b3RvLXN1LmFjLmpw
Disclaimer: All claims expressed in this article are solely those of the authors and do not necessarily represent those of their affiliated organizations, or those of the publisher, the editors and the reviewers. Any product that may be evaluated in this article or claim that may be made by its manufacturer is not guaranteed or endorsed by the publisher.
Research integrity at Frontiers
Learn more about the work of our research integrity team to safeguard the quality of each article we publish.