- 1Department of Nutritional Physiology, Institute of Agricultural and Nutritional Sciences, Martin-Luther University Halle-Wittenberg, Halle, Germany
- 2Department of Physiology, University of Hohenheim, Stuttgart, Germany
Together with fibroblast growth factors (FGFs) 19 and 21, FGF23 is an endocrine member of the family of FGFs. Mainly secreted by bone cells, FGF23 acts as a hormone on the kidney, stimulating phosphate excretion and suppressing formation of 1,25(OH)2D3, active vitamin D. These effects are dependent on transmembrane protein αKlotho, which enhances the binding affinity of FGF23 for FGF receptors (FGFR). Locally produced FGF23 in other tissues including liver or heart exerts further paracrine effects without involvement of αKlotho. Soluble Klotho (sKL) is an endocrine factor that is cleaved off of transmembrane Klotho or generated by alternative splicing and regulates membrane channels, transporters, and intracellular signaling including insulin growth factor 1 (IGF-1) and Wnt pathways, signaling cascades highly relevant for tumor progression. In mice, lack of FGF23 or αKlotho results in derangement of phosphate metabolism and a syndrome of rapid aging with abnormalities affecting most organs and a very short life span. Conversely, overexpression of anti-aging factor αKlotho results in a profound elongation of life span. Accumulating evidence suggests a major role of αKlotho as a tumor suppressor, at least in part by inhibiting IGF-1 and Wnt/β-catenin signaling. Hence, in many malignancies, higher αKlotho expression or activity is associated with a more favorable outcome. Moreover, also FGF23 and phosphate have been revealed to be factors relevant in cancer. FGF23 is particularly significant for those forms of cancer primarily affecting bone (e.g., multiple myeloma) or characterized by bone metastasis. This review summarizes the current knowledge of the significance of FGF23 and αKlotho for tumor cell signaling, biology, and clinically relevant parameters in different forms of cancer.
Fibroblast Growth Factor 23 (FGF23)
The human fibroblast growth factor 23 (FGF23) gene localized on chromosome 12p13 was discovered in 2000 (Autosomal dominant hypophosphataemic rickets is associated with mutations in FGF23, 2000, ADHR Consortium, 2000). FGF23 is a member of the family of fibroblast growth factors (FGFs) and a proteohormone of 32 kDa (Yamashita et al., 2000; Yamazaki et al., 2002). It is characterized by endocrine and paracrine effects in contrast to most other FGFs, which do not act as classical hormones (Angelin et al., 2012). Endocrine FGF23 is primarily produced by bone cells and released into the bloodstream (Riminucci et al., 2003; Yoshiko et al., 2007). Low Fgf23 expression was detected in other tissues, such as spleen, thymus, small intestine, liver, kidney, heart, and brain (Yamashita et al., 2000; Yoshiko et al., 2007). The secretion of the biologically active hormone into the blood is controlled by proteolytic cleavage of the full-length, intact FGF23 molecule by a furin/furin-like proprotein convertase between 179Arg and 180Ser (Shimada et al., 2001). The susceptibility of FGF23 to proteolytic degradation is regulated by UDP-N-acetyl-alpha-D galactosamine: polypeptide N-acetylgalactosaminyltransferase 3 (GalNT3)-mediated O-glycosylation at threonine 178 and phosphorylation at serine 180 by the enzyme family with sequence similarity 20 member C (FAM20C) (Tagliabracci et al., 2014). Target organs of FGF23 include kidney and parathyroid glands (Ben-Dov et al., 2007; Gattineni et al., 2009). In the former, FGF23 inhibits the reabsorption of phosphate by down-regulating the membrane abundance of NaPiIIa, the major Na+-coupled phosphate transporter of the proximal tubule (Gattineni et al., 2009). Moreover, FGF23 suppresses the synthesis of 1,25(OH)2D3, active vitamin D, by inhibiting key enzyme 1-α-hydroxylase (encoded by Cyp27b1) in the kidney (Chanakul et al., 2013). In the parathyroid glands, FGF23 down-regulates the production and secretion of parathyroid hormone (PTH) (Ben-Dov et al., 2007). This way, FGF23 is part of a hormone circuit additionally involving PTH and 1,25(OH)2D3 and regulating phosphate and vitamin D metabolism, as well as impacting on Ca2+ (Blau and Collins, 2015). These endocrine effects of FGF23 are mediated by FGF receptors (FGFRs) including FGFR1c, FGFR3c, and FGFR4 with αKlotho (KL) serving as a scaffolding protein, which is needed to enhance the binding affinity of FGF23 (Gattineni et al., 2009, 2011; Chen G. et al., 2018). Other effects of locally produced FGF23 are, at least in part, paracrine and include the regulation of inflammation in hepatocytes (Singh et al., 2016), the induction of cardiac hypertrophy (Faul et al., 2011), or inhibition of neutrophils (Rossaint et al., 2016). At least some of these effects are independent of KL (Quarles, 2019). The plasma concentration of FGF23 goes up in many acute and chronic diseases (Gutierrez et al., 2005). In chronic kidney disease (CKD), high FGF23 plasma levels are observed prior to hyperparathyroidism or hyperphosphatemia (Isakova et al., 2011). FGF23 predicts progression and outcome in CKD (Hasegawa et al., 2010). Independently of kidney disease, FGF23 is associated with carotid atherosclerosis (Rodríguez-Ortiz et al., 2020), fibrosis, and poorer prognosis in heart failure (Roy et al., 2020) and prognosis in heart failure with preserved ejection fraction (Kanagala et al., 2020). In another cohort, however, the role of FGF23 for patients with heart failure was less clear (Stöhr et al., 2020). Dyslipidemia is associated with higher FGF23 levels (Mirza et al., 2011). Inflammatory conditions also up-regulate FGF23 (Czaya and Faul, 2019). Hence, FGF23 is discussed as a biomarker correlating with progression and outcome in some significant diseases of high burden (Schnedl et al., 2015).
αKlotho
The αKlotho (referred to as KL) gene was identified in 1997. In mice, a mutation of the Kl gene causes a syndrome of rapid aging including a drastically shortened life span and further age-associated diseases and symptoms affecting most organs and tissues such as atherosclerosis, osteoporosis, skin atrophy, infertility, or emphysema (Kuro-O et al., 1997). KL is mainly expressed in the kidney but also in the central nervous system (cerebellum, cerebral cortex, spinal cord) and in other tissues with detectable but lower expression such as thyroid gland, aorta, urinary bladder, ovary, skeletal muscle, pancreas, prostate gland, testis, or the adrenal gland (Kuro-O et al., 1997; Lim et al., 2015). However, it has not been clear for a long time how KL develops its function until it was discovered that the phenotype of the Kl knockout mouse is similar to the Fgf23 knockout mouse. The mice exhibit high serum phosphate levels, soft tissue and vascular calcification, increased expression of renal sodium phosphate cotransporter NaPiIIa, and 1-α-hydroxylase, accompanied by high serum levels of 1,25(OH)2D3 (Tsujikawa et al., 2003; Nakatani et al., 2009; Razzaque, 2009a). Moreover, it could be shown that the ablation of 1,25(OH)2D3 signaling in mice lacking a functional vitamin D receptor prevents the premature aging phenotype in Kl–/– mice (Anour et al., 2012; Andrukhova et al., 2017). Deficiency of both 1-α-hydroxylase and Kl prevents soft tissue and vascular calcification and normalizes the high Fgf23 and low PTH levels paralleled by Kl deficiency in mice (Ohnishi et al., 2009). These findings assign KL an important physiological role in the regulation and maintenance of phosphate homeostasis (Razzaque, 2009b). The human KL gene is located on chromosome 13q12 and ranges over 50 kb with 5 exons and 4 introns (Matsumura et al., 1998). It encodes the KL protein, which shows homology with family I β-glycosidases and is a 135-kDa single-pass transmembrane protein (Kuro-O et al., 1997; Chen et al., 2007; Xu and Sun, 2015; Dalton et al., 2017). The protein comprises a N-terminal short signal sequence, the large ectodomain containing two internal repeats termed KL1 and KL2 mediating KL activity and function, the transmembrane domain, and a short intracellular domain (Kuro-O et al., 1997; Kuro-O, 2008; Xu and Sun, 2015) (Figure 1). Three different isoforms can be distinguished: full-length transmembrane KL, the 130-kDa shed soluble form (sKL), and the shorter truncated secreted variant of KL (65 kDa) (Kuro-O et al., 1997; Shiraki-Iida et al., 1998; Dalton et al., 2017). sKL consists of the KL1 and KL2 domain but lacks the transmembrane and intracellular domain. It arises because of proteolytic cleavage, termed α-cut, of full-length transmembrane KL on the cell surface by α-secretases A disintegrin and metalloproteinase domain-containing proteins 10 and 17 (ADAM10 and ADAM 17) and the β-APP cleaving enzyme 1 (BACE1) (Chen et al., 2007; Bloch et al., 2009; Xu and Sun, 2015). The residual transmembrane fragment undergoes an intramembrane proteolytic degradation by γ-secretases (Bloch et al., 2009). Moreover, another cleavage mechanism of KL by ADAM10 and ADAM17, termed β-cut, generates the two 65-kDa fragments KL1 and KL2 (Chen et al., 2007). Therefore, after shedding, sKL protein enters blood, urine, or cerebrospinal fluid as KL1 or KL2 only or both KL1 and KL2 and exerts its functions in other tissues and organs (Imura et al., 2004; Akimoto et al., 2012; Xu and Sun, 2015; Dalton et al., 2017). sKL inhibits insulin growth factor 1 receptor (IGF-1R)/phosphoinositide 3-kinase (PI3K)/AKT serine/threonine kinase (AKT) signaling and activates forkhead box O (FOXO) (Kurosu et al., 2005; Yamamoto et al., 2005). It increases glucose uptake and glycogen storage and reduces lipid accumulation and insulin resistance through PPARα expression (Gu et al., 2020) corroborating the role of KL and underlying signaling in glucose metabolism and adipocyte maturation as discussed recently (Razzaque, 2012). Other KL downstream effects are the activation of extracellular signal–related kinase 1/2 (ERK1/2) (Maekawa et al., 2011), inhibition of Wnt signaling (Liu et al., 2007), or reduction of inflammation (Maekawa et al., 2009). Moreover, sKL is involved in the stimulation of ion channels and transporters including transient receptor potential ion channel TRPV5 (Chang et al., 2005; Cha et al., 2008) or renal outer medullary potassium channel 1 (ROMK1) (Cha et al., 2009). The secreted isoform of KL is processed by alternative RNA splicing in the internal splice donor site of exon 3, containing a N-terminal signal sequence and KL1 only (Matsumura et al., 1998). In contrast to KL and sKL, the secreted isoform has not been detected in vivo yet (Kuro-O, 2019).
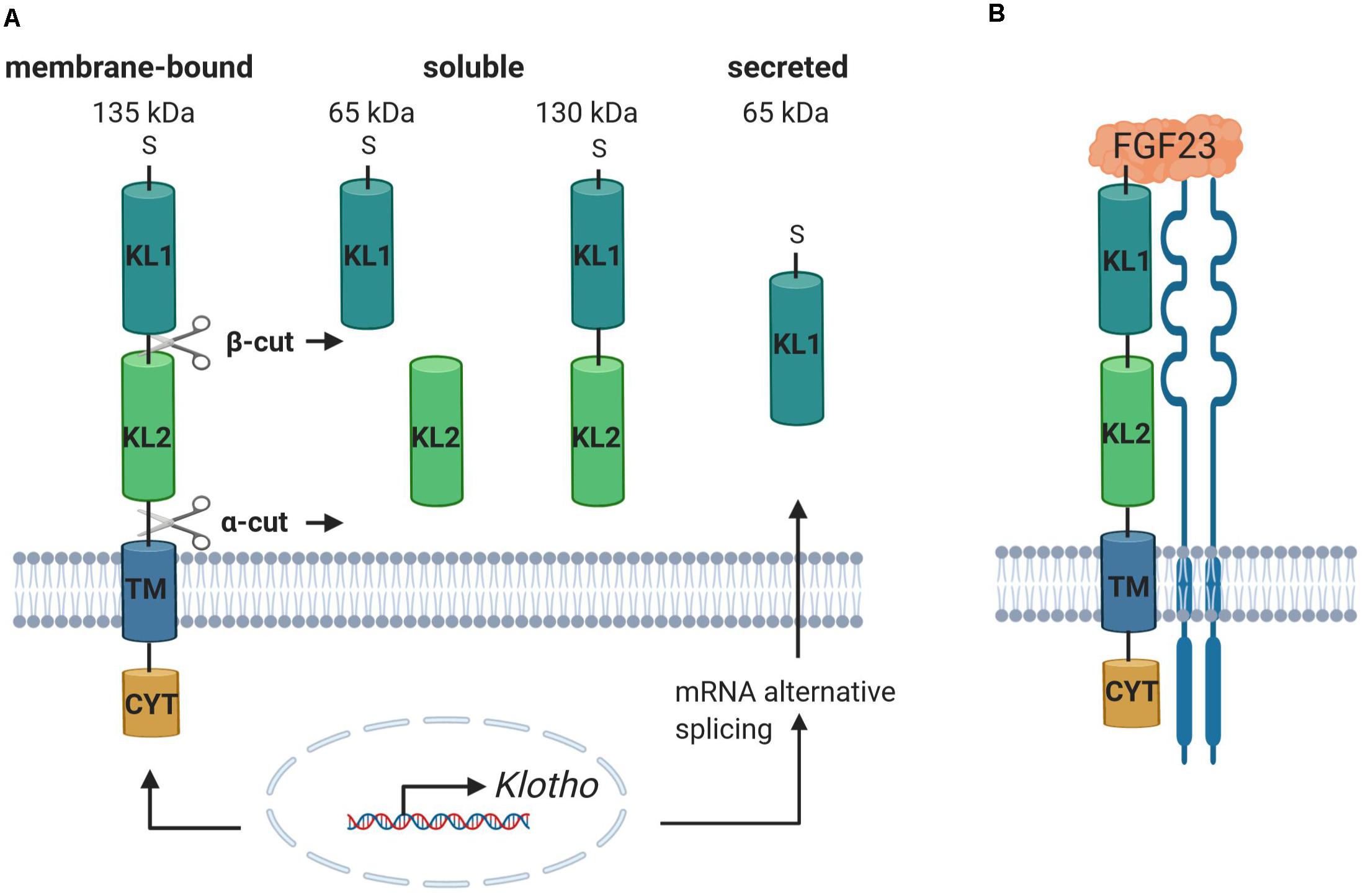
Figure 1. Klotho includes different isoforms and binds to FGFR, facilitating the binding and subsequent signal transduction of FGF23. (A) The KL gene encodes a single-pass transmembrane protein, comprising an N-terminal short signal sequence (S), the ectodomain, containing the internal repeats KL1 and KL2, the transmembrane domain (TM), and a short cytoplasmic domain (CYT). It exists as full-length 135-kDa membrane-bound KL, the 130-kDa shed soluble KL (sKL) isoform, and the truncated secreted variant of KL. Proteolytic cleavage of full-length KL due to α-cut and/or β-cut produces sKL, containing either KL1, KL2, or both. Alternative RNA splicing of KL mRNA generates the secreted isoform, containing an N-terminal signal sequence and KL1 only. (B) The complexation of KL with FGFR enables the binding of FGF23, resulting in the formation of a trimeric complex, which activates the downstream signaling pathway. Created with BioRender.com.
Progressing CKD is associated with decreased renal KL expression and loss of renal function (Koh et al., 2001; Komaba et al., 2010; Hu et al., 2011). Lower KL expression correlates with more cardiovascular events in patients on hemodialysis (Memmos et al., 2019). In addition, KL inhibits inflammation (Maekawa et al., 2009; Zhao et al., 2011) and oxidative stress (Kimura et al., 2018; Qian et al., 2018), conditions enhanced in CKD (Mihai et al., 2018) and cardiovascular diseases (Dhiman et al., 2015).
In addition, the KL family includes two other members, termed βKlotho and γKlotho (referred to as KLB and KLG hereinafter). The Klb gene, identified in 2000, shows sequence similarity to Kl and encodes a single-pass transmembrane protein (Ito et al., 2000). KLB is localized in the cell membrane and mainly expressed in the liver and adipose tissue, where it forms a complex with FGFR1 and FGFR4, and mediates metabolic functions of FGF19 and FGF21 (Kurosu et al., 2007; Ogawa et al., 2007; Xu and Sun, 2015). FGF19 controls bile acid synthesis through suppression of Cyp7a1 (Kurosu et al., 2007). Thus, Klb–/–, Fgf15–/–, and also Fgfr4–/– mice lack Cyp7a1 suppression, resulting in increased bile acid production and excretion (Inagaki et al., 2005; Ito et al., 2005). Moreover, KLB is necessary for FGF21 signaling, which is expressed mainly in the liver, where it is involved as downstream target of peroxisome proliferator–activated receptor α (PPARα) in metabolic adaptation to fasting but also in adipose tissue, where it modulates lipolysis and glucose uptake (Kurosu et al., 2007; Arner et al., 2008; Suzuki et al., 2008; Dolegowska et al., 2019). The Klph gene was found in mice, encoding the Klotho lactase-phlorizin hydrolase-related protein, which is mainly expressed in the eyes but also in the kidney, adipose tissue, and skin (Ito et al., 2002; Fon Tacer et al., 2010). This novel member of the KL family is also termed KLG. KLG interacts with FGFR1b, 1c, 2c, and 4 and promotes activation of FGF signaling by FGF19 in HEK293 cells (Fon Tacer et al., 2010).
FGF23 and Cancer
As detailed below and summarized in Table 1, the implications of FGF23 in cancer biology are thus far sparser than the known role of its coreceptor KL in tumor diseases. This may, in large part, be due to the fact that KL acts as a tumor suppressor in various types of cancer, whereas such a function is not established for FGF23. A role of FGF23 in malignancies is most clearly proven in the case of tumor-induced osteomalacia (TIO) or oncogenic hypophosphatemic osteomalacia (Larsson et al., 2003). This is a rare paraneoplastic syndrome due to a tumor excessively producing FGF23, which, in line with its main endocrine effects, induces renal phosphate excretion, as well as reduction of 1,25(OH)2D3. As a consequence of both, the patients suffer from osteomalacia, demineralized bone (Yamazaki et al., 2002; Larsson et al., 2003). Benign soft tissue (mesenchymal) tumors are most frequently responsible for TIO (Boland et al., 2018), but also malignancies including colon adenocarcinoma (Leaf et al., 2013), ovarian cancer (Lin et al., 2014), small cell carcinoma of the lung (Sauder et al., 2016), anaplastic thyroid carcinoma (Abate et al., 2016), B-cell non-Hodgkin lymphoma (Elderman et al., 2016), breast cancer (Savva et al., 2019), and intracranial tumors (Colazo et al., 2020) can produce FGF23. If the causative tumor cannot be identified, the anti-FGF23 monoclonal antibody KRN23 may be therapeutically useful in TIO (Minisola et al., 2017).
Hematologic Malignancies
Because bone is the main site of FGF23 production, malignancies typically affecting or arising from bone may have a link to FGF23. In patients with bone metastasis due to different solid tumors, a higher FGF23 plasma concentration is associated with shorter survival and shorter time to skeletal-related events (Mansinho et al., 2019). Patients with myelodysplastic syndrome (MDS) characterized by impaired hematopoiesis in the bone marrow have a higher FGF23 plasma concentration that is associated with anemia and lower bone mineralization (Weidner et al., 2020). In mice, MDS is paralleled by Fgf23 expression in erythroid precursor cells (Weidner et al., 2020). Multiple myeloma (MM) is characterized by painful bone lesions. MM cells exhibit KL-dependent FGF23 signaling, and intact FGF23 plasma levels are elevated in MM patients (Suvannasankha et al., 2015).
Prostate Cancer
FGF23 single-nucleotide polymorphisms (SNPs) are associated with increased risk of prostate cancer (Kim et al., 2014a). FGF23 expression is enhanced in patients with castration-resistant prostate cancer, as well as FGF23/FGFR1/KL in different prostate cancer cell lines (Lee et al., 2014). FGF23 acts as an autocrine factor in prostate cancer cells stimulating tumor invasion and cell proliferation (Feng et al., 2015). According to another study, KL expression is reduced due to promoter hypermethylation (Seo et al., 2017). FGF23 down-regulation suppresses tumor growth in vivo (Feng et al., 2015). FGF23 production may be subject to autocrine stimulation through FGFR in prostate cancer (Feng et al., 2012; Wu et al., 2013; Lee et al., 2014). According to one study, the FGF23 plasma level is unchanged in prostate cancer (Vlot et al., 2018), although prostate cancer cells may stimulate FGF23 expression in osteocytes (Choudhary et al., 2018). Bone metastasis may account for the high FGF23 levels and symptoms of TIO observed in patients with prostate cancer according to other studies (Nakahama et al., 1995; Cotant and Rao, 2007; Chiam et al., 2013).
Gynecologic Tumors
In endometrial cancer, no change in the FGF23 plasma concentration is observed (Cymbaluk-Płoska et al., 2020), whereas the FGF23 plasma concentration goes up in advanced-stage epithelial ovarian cancer (EOC) (Tebben et al., 2005), and a defined FGF23 SNP is associated with better prognosis in this tumor entity (Meng et al., 2014). Breast cancer may be associated with oncogenic osteomalacia and raised FGF23 levels (Savva et al., 2019). FGF23 mRNA expression is high in breast cancer cells, and FGF produced by tumor cells contributes to metastatic lesions (Aukes et al., 2017). Furthermore, FGFR signaling may be highly relevant for breast cancer oncogenesis (Navid et al., 2020). According to a phase 0/1 clinical trial, combined aromatase and FGFR1 inhibition in breast cancer results in a surge in the FGF23 plasma concentration (Quintela-Fandino et al., 2019).
FGF23 in Other Forms of Cancer
The plasma FGF23 concentration may rise in colorectal adenoma (Jacobs et al., 2011), and FGF23 excretion is enhanced in the stool from patients with colorectal carcinoma (Wang H.-P. et al., 2014). In urothelial carcinoma, an increase in the FGF23 plasma concentration is reported (Li et al., 2019). In patients with prolactinoma, the FGF23 plasma concentration is unaltered, and there is only a minor effect of FGF23 on bone loss in these patients, if any (Arslan et al., 2017). Progression of hepatocellular carcinoma (HCC) is not linked to altered FGF23 expression (Zou et al., 2018).
It is important to keep in mind that most of the aforementioned studies on FGF23 and different types of cancer report associations, not necessarily causative relationships.
αKlotho Signaling Pathways Relevant for Cancer
The development of cancer, its progression, and metastasis are a complex process. Initially, cells are exposed to harmful genetic or epigenetic alterations resulting in dysregulated signaling pathways. Subsequently, the modified cells escape homeostatic checks and elimination (Sever and Brugge, 2015). Typical dysregulated pathways in cancer include IGF-1R, PI3K/AKT1/mammalian target of rapamycin (mTOR), mitogen-activated protein kinase (MAPK)/ERK, glycogen synthase kinase-3β (GSK-3β), or Wnt/β-catenin signaling. Many of them are controlled by KL (Sopjani et al., 2015; Badve and Kumar, 2019). Moreover, aging is a major driver of cancer (Aunan et al., 2017). Also in view of rapid aging of Kl-deficient mice (Kuro-O et al., 1997), it is intriguing to speculate that KL signaling in many tissues is implicated in cancer development and may be a possible target in cancer prevention or therapy. The role of KL in different forms of cancer is summarized in Table 2.
The Role of αKlotho in Cancer
Breast Cancer
In 2008, KL was revealed as a tumor suppressor in breast cancer (Wolf et al., 2008). According to this study, normal breast tissue exhibits higher KL expression than ductal carcinoma in situ or invasive ductal carcinoma. Also, in less-differentiated breast cancer cell lines, KL expression is lower than in the non-tumor breast cell line MCF-12A or in well-differentiated MCF-7 breast cancer cells. KL overexpression reduces, whereas RNAi-mediated KL down-regulation enhances breast cancer cell proliferation. KL overexpression activates the FGF pathway, whereas KL overexpression and sKL attenuate IGF-1R activation and its downstream targets AKT1, GSK-3β, and ERK1/2 (Wolf et al., 2008). In vitro and ex vivo, methylation of the KL promoter in breast cancer cells is negatively correlated with KL mRNA abundance, suggesting a role of epigenetic silencing of KL in breast cancer (Rubinek et al., 2012; Dallol et al., 2015). Also dietary methyltransferase inhibition with green tea polyphenols and histone deacetylase inhibition with sulforaphane up-regulate epigenetically silenced KL in breast cancer cells (Sinha et al., 2015). sKL may exert further antitumor effects in breast cancer by regulating endoplasmic reticulum (ER) Ca2+ storage, as well as inner mitochondrial membrane potential and Ca2+ transport (Shmulevich et al., 2020). Heterozygosity for a certain KL gene variant (KL-VS) is associated with an even higher breast cancer risk of patients with BRCA1 mutation prone to developing breast cancer (Wolf et al., 2010).
Colorectal Cancer
Epigenetic silencing through KL promoter hypermethylation is observed in different colon cancer cell lines (Pan et al., 2011). Also, in human colorectal cancer (CRC) specimens, KL promoter methylation with reduced KL mRNA is frequent (Gan et al., 2011; Pan et al., 2011; Li et al., 2014; Yang et al., 2014; Perveez et al., 2015; Arbel Rubinstein et al., 2019; Liu et al., 2019; Son et al., 2020). According to some studies, methylation status and reduced KL expression are independent of age, gender, TNM stage, histological grade, or tumor differentiation (Pan et al., 2011; Yang et al., 2014; Perveez et al., 2015). Others found an association of KL expression with decreased survival of CRC patients (Liu et al., 2019) or TNM stage, invasiveness, and lymph node metastasis (Li et al., 2016; Arbel Rubinstein et al., 2019). Moreover, a recent study observed an association between KL variants and an increased risk of CRC (Kamal et al., 2020). Overexpression of KL or KL1 fragment or treatment with sKL decreases surviving colonies and cell proliferation and induces cell cycle arrest and apoptosis of colon cancer cells (Pan et al., 2011; Arbel Rubinstein et al., 2019). Mice colon cancer cells transfected with KL exhibit lower tumor growth, weight, and volume (Li et al., 2014). The same holds true after treatment with sKL1 (Arbel Rubinstein et al., 2019). Similar to breast cancer, KL might be tumor-suppressing by inhibiting IGF-1R–dependent PI3K/AKT signaling (Li et al., 2014) or aerobic glycolysis via ERK/hypoxia-inducible factor 1α (HIF-1α) (Li et al., 2018) in CRC. Also, down-regulation of Wnt3a/β-catenin signaling and apoptosis are induced by KL in CRC cells (Bordonaro and Lazarova, 2015; Arbel Rubinstein et al., 2019; Xie et al., 2020). miR-15b may contribute to reduced KL expression in CRC because higher miR-15b levels in CRC patients compared to healthy subjects, those with metastasis than without, and those with cancer recurrence than without are described (Li et al., 2016). In CRC cells, inflammation-inherent nuclear factor κB (NF-κB) and IGF-1R activity further lowers KL expression, increasing cell proliferation and invasion (Xie et al., 2019). Conversely, KL blocks NF-κB activation (Liu et al., 2019).
Lung Cancer
KL is down-regulated in lung cancer cells and tissues and even more so in chemotherapy-resistant lung cancer (Chen et al., 2012, 2016; Chen B. et al., 2018). KL inhibits lung cancer cell proliferation, growth, invasiveness, and migration and fosters apoptosis (Chen et al., 2010, 2012, 2016, 2019; Wang X. et al., 2011; Wang et al., 2013), effects, at least in part, dependent on IGF-1R/AKT (Chen et al., 2010; Wang et al., 2013) and Wnt3a/β-catenin signaling (Chen et al., 2012, 2019) and on reduced interleukin 6 (IL-6) and IL-8 production (Chen B. et al., 2018). MiR-10b lowers, Ras-related GTPase Ras8 up-regulates KL expression in non–small-cell lung cancer cells (Huang et al., 2015). Patients with large-cell neuroendocrine lung carcinoma or small-cell lung cancer with KL expression have better outcome than those without KL expression pointing to KL being a potential biomarker (Usuda et al., 2011a; Vanoirbeek et al., 2011; Brominska et al., 2019). This could not be confirmed for sKL in lung cancer (Pako et al., 2020). KL may sensitize lung cancer cells to apoptosis induction by cisplatin via PI3K/AKT signaling (Wang et al., 2013) or due to decreased autophagy (Chen et al., 2016).
Hepatocellular Cancer
HCC cells and HCC tissue exhibit reduced KL expression (Shu et al., 2013; Xie et al., 2013b; Sun et al., 2015; Tang et al., 2016b), a phenomenon again explained by epigenetic silencing of the KL promoter through hypermethylation and acetylation (Xie et al., 2013b). KL promoter methylation is associated with a poorer prognosis (Xie et al., 2013b), whereas KL expression is inversely related to histological grade and clinical stage in HCC (Tang et al., 2016b). KL overexpression or treatment with recombinant KL or sKL decreases colony formation, cell proliferation, migration, and tumor invasion while inducing apoptosis and autophagy through inhibition of Wnt/β-catenin (Sun et al., 2015; Tang et al., 2016b) and IGF-1R/AKT/ERK signaling (Shu et al., 2013). According to another study, however, KL activates vascular endothelial growth factor receptor 2/p21-activated kinase 1, resulting in cell death resistance and favoring tumor migration and invasion (Chen et al., 2013). Thus, higher KL expression is associated with cirrhosis, venous invasion, tumor multiplicity, and a lower overall survival in HCC patients according to this study (Chen et al., 2013).
Squamous Cell Carcinoma
Lower KL and higher DNA methyltransferase 3a (enzyme required for epigenetic alteration of KL promoter activity) are typical of the transition from normal tissue to oral dysplastic lesions to oral squamous cell carcinoma (SCC) (Adhikari et al., 2017). KL promoter methylation may predict survival prognosis in head and neck SCC with conflicting results (Alsofyani et al., 2017; Zhu et al., 2019). Higher KL gene expression is again associated with better survival, and KL methylation with gender, tumor grade, and site (Zhu et al., 2019). Survival of patients with esophageal SCC is better if the tumor expresses KL (Tang et al., 2016a). Moreover, KL expression is inversely correlated with invasion depth, histological grade, clinical stage, and lymph node metastasis in esophageal SCC (Tang et al., 2016a). In lung SCC, KL expression is associated with invasiveness (Ibi et al., 2017). KL inhibits N-cadherin and regulates epithelial–mesenchymal transition (EMT) (Ibi et al., 2017). Also, in cervix SCC, KL is reduced (Aviel-Ronen et al., 2016).
Pancreatic Cancer
Pancreatic adenocarcinoma tissue or human pancreatic adenocarcinoma cell lines Panc1, MiaPaCa2, and Colo357 are characterized by reduced KL expression compared to normal pancreatic tissue (Abramovitz et al., 2011). Epigenetic silencing due to a hemimethylated KL promoter may account for this (Abramovitz et al., 2011). Overexpression of KL or recombinant sKL reduce survival and size of the cancer cell colonies and potentiates chemotherapeutic effects (Abramovitz et al., 2011). They inhibit IGF-1R and its downstream signaling effectors IRS-1, AKT1, and ERK1/2 as well as FGF2 pathway activation (Abramovitz et al., 2011). sKL injection also reduces tumor growth in mice (Abramovitz et al., 2011). KL expression is positively, p-IGF-1R abundance negatively, correlated with lower TNM stage and pathological grade (Jiang et al., 2014). Higher methylation of the KL promoter in pancreatic ductal adenocarcinoma compared to normal pancreatic tissue worsens outcome (Jiang et al., 2014). miR-199a lowers KL expression in pancreatic adenocarcinoma Panc1 cells (Zhang et al., 2020). KL inhibits mTOR as downstream target of AKT1 and MEK/ERK signaling in Panc1 cells (Zhang et al., 2020).
Gastric Carcinoma
KL promoter hypermethylation with decreased gene expression is typical of gastric carcinomas and gastric carcinoma cell lines (Wang L. et al., 2011). KL overexpression inhibits growth and ERK1/2 activity, resulting in apoptosis of AGS and MKN28 gastric carcinoma cells (Wang L. et al., 2011). Promoter hypermethylation correlates with poorer survival of patients with gastric cancer, making it an independent prognosis factor (Wang L. et al., 2011). Restoration of KL expression reduces p-IGF-1R, p-PI3K, and p-mTOR in GC-7901 cells (Xie et al., 2013a). Similar to pancreatic cancer (Zhang et al., 2020), miR-199a influences KL expression in gastric cancer (He et al., 2014). The human sex determining region Y (SRY)–related high-mobility-group (HMG) box protein family member 17 (SOX17) protein also binds to the KL promoter in gastric cancer cells, thereby inducing KL expression (Yang et al., 2020).
Prostate Cancer
A KL single-nucleotide polymorphism (rs3752472) is associated with the risk of prostate cancer (odds ratio = 1.85) (Kim et al., 2014b). Methylation in the KL CpG island region KL-M3, including −593 to −406 bp, accounts for the down-regulation of KL mRNA in prostate cancer cell lines DU145 and PC-3 (Seo et al., 2017). The same region is unmethylated in 22Rv1 prostate cancer cells exhibiting KL mRNA expression (Seo et al., 2017). The KL promoter in 22Rv1 cells is hypomethylated, and in DU145 and PC-3 cells hypermethylated (Seo et al., 2017).
Renal Cell Carcinoma
In renal cell carcinoma (RCC) tissue and cell lines, KL protein and mRNA expression are reduced (Zhu et al., 2013; Gigante et al., 2015; Kim et al., 2016; Dehghani et al., 2018). KL expression is negatively associated with TNM stage, tumor size, shorter overall and progression-free survival (Zhu et al., 2013; Gigante et al., 2015). KL overexpression in RCC cells down-regulates PI3K/AKT/GSK3-β/Snail signaling, thereby inhibiting cell migration, invasion, and EMT (Zhu et al., 2013). Moreover, KL inhibits epidermal growth factor 1–dependent p38MAPK activation and IGF-1R signaling in Caki-1 cells compromising cell motility and proliferation (Zhu et al., 2013; Kim et al., 2016; Dehghani et al., 2018).
Ovarian Cancer
Results regarding the role of KL in ovarian cancer are controversial. According to a clinical study of 189 EOC patients, 73.5% of patients exhibit detectable KL expression. sKL is associated with high tumor grade, suboptimal tumor debulking results, disease progression [hazard ratio (HR) = 1.97], and death (HR = 2.09), possibly due to KL supporting the tumor with energy and angiogenesis (Lu et al., 2008). Others found reduced KL expression in different human EOC cell lines and specimens, as well as inhibition of proliferation of different EOC cell lines upon sKL treatment or KL overexpression (Lojkin et al., 2015; Yan et al., 2017). KL suppresses IGF-1–induced ERK 1/2 phosphorylation in OVCA-432 and SKOV-3 cells (Lojkin et al., 2015). KL expression is lower in ovarian cancer and is associated with decreased survival (Yan et al., 2017). In mice, KL-expressing A2780 tumor cells grow more slowly than KL-negative tumor cells (Yan et al., 2017). KL suppresses a tumor-associated inflammatory response in mice with ovarian cancer, thereby contributing to a more favorable outcome (Yan et al., 2017).
Melanoma
In different melanoma cell lines, a mutually inhibitory effect of Wnt5a and KL expression is established impacting on metastasis (Camilli et al., 2011). The effect of KL on Wnt5a internalization and signaling is dependent on heparan sulfate proteoglycans (Camilli et al., 2011). Moreover, KL inhibits Wnt5a-mediated filamin A cleavage through calpain, an effect contributing to reduced motility of melanoma cell lines (Camilli et al., 2011). Melanoma cells exhibit KL expression, depending on the age of surrounding fibroblasts (Behera et al., 2017). Older patients’ melanoma cells show lower KL expression (Behera et al., 2017). Treatment of melanoma cells with media of aged fibroblasts results in increased Wnt5a expression and less KL mRNA expression, compared to incubation with media of young fibroblasts (Behera et al., 2017). KL expression in melanoma cells is enhanced by PPARγ, and KL or PPARγ agonist rosiglitazone treatment reduce melanoma growth in mice (Behera et al., 2017). HMG protein B1 (HMGB1) activates NF-κB and inhibits KL expression melanoma cell lines (Xie et al., 2016).
Thyroid Cancer
KL overexpression and sKL induce apoptosis and compromise proliferation of thyroid cancer cell lines FTC133 and FTC238, an effect presumably dependent on stanniocalcin-1 (Dai et al., 2016). Low differentiation is paralleled by reduced KL expression in human thyroid cancer (Pawlikowski et al., 2019).
Other Forms of Cancer
KL is a possible tumor suppressor in urothelial carcinoma of the bladder (Hori et al., 2016, 2018). KL expression in glioblastoma multiforme (GBM) correlates with outcome (Trošt et al., 2016; Peshes-Yeloz et al., 2019). sKL decreases viability of GBM cell lines, and reduced KL expression is due to epigenetic KL promoter methylation in these cells (Peshes-Yeloz et al., 2019). Similar epigenetic mechanisms of down-regulation of KL expression are effective in human specimens of invasive cervical carcinoma and cell lines (Lee et al., 2010). Secreted KL acts as a tumor suppressor in CaSki cervical carcinoma cells by inhibiting canonical Wnt signaling and c-MYC and Cyclin D1 expression (Lee et al., 2010). KL overexpression in SiHa cells down-regulates β-catenin, c-MYC, and cyclin D1 signaling, as well as EMT (Lee et al., 2010; Chang et al., 2012).
KL expression correlates with overall survival and is lower in dedifferentiated liposarcoma (DDLPS) than in adipose tissue (Delcroix et al., 2018). KL-overexpressing DDLPS blunts IGF-1–induced Ca2+ and ERK1/2 signaling, reducing proliferation, inducing apoptosis, and sensitizing cells to ER stress (Delcroix et al., 2018).
Also in T-cell lymphoma and diffuse large B-cell lymphoma (DLBLC), KL overexpression attenuates IGF-1R, ERK1/2 and AKT signaling (Zhou et al., 2017a,b). Moreover, in biopsies and cell lines of T-cell lymphoma and DLBLC, KL expression is reduced correlating with shorter survival. KL overexpression in T-cell lymphoma and DLBLC cell lines lowers proliferation and enhances apoptosis (Zhou et al., 2017a,b).
FGF23/KL and the Cancer Microenvironment
As summarized in Figure 2, KL is a potent regulator of IGF-1R and Wnt/β-catenin signaling, and these pathways are highly relevant for the cancer microenvironment (Huang and Du, 2008; Sanchez-Lopez et al., 2016). Local hypoxia is typical of advanced cancers activating HIF-1 (Petrova et al., 2018). KL inhibits HIF-1α in CRC (Li et al., 2018). Conversely, HIF-1α increases ectopic FGF23 expression in patients with TIO (Zhang et al., 2016). Hypoxia fosters accumulation of tumor-associated macrophages in the tumor microenvironment and mediates inflammation (Lewis and Murdoch, 2005). Interestingly, cultured macrophages express FGF23, which up-regulates cell number and their tumor necrosis factor α expression (Masuda et al., 2015; Han et al., 2016). Thus, FGF23 production and local inflammation may be interdependent in the microenvironment of the tumor depending on hypoxia, HIF-1α activation, and tumor-associated macrophages. Furthermore, FGF23 possibly contributes to a bone-like microenvironment in phosphaturic mesenchymal tumor, mixed connective tissue variant (PMTMCT), through FGFR1c/KL, inducing enhanced FGF23 production by the tumor cells and worsening TIO (Kinoshita et al., 2019). Clearly, further studies are warranted to address this important issue.
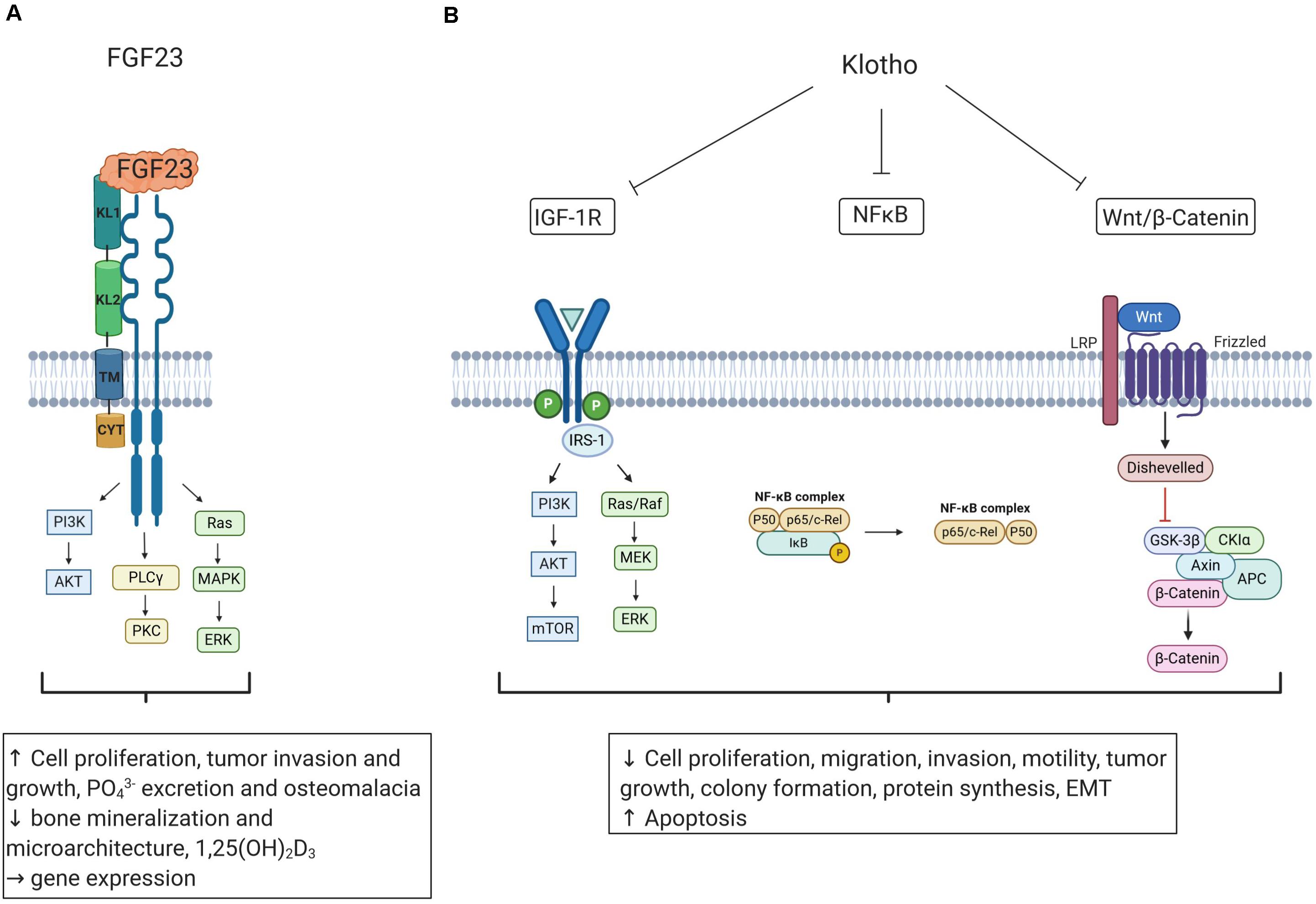
Figure 2. The influence of FGF23 and Klotho on oncogenic and tumor-suppressing pathways. (A) FGF23 binds to FGFRs and coreceptor KL and may impact cell proliferation, tumor growth, and bone gene transcription. Tumor-induced elevation of FGF23 production may cause phosphate wasting, 1,25(OH)2D3 reduction, and osteomalacia. (B) Klotho (KL) is a tumor-suppressor inhibiting pathways relevant for tumorigenesis including IGF-1R, Wnt/β-catenin, and NF-κB signaling, resulting in decreased cell proliferation, invasion, migration, tumor growth, protein synthesis, and EMT and inducing apoptosis. Figure according to Sachdeva et al. (2020). Created with BioRender.com. Insulin-like growth factor 1 receptor (IGF-1R); phosphoinositide 3-kinase (PI3K); mammalian target of rapamycin (mTOR); mitogen-activated protein kinase (MAPK); mitogen-activated protein kinase kinase (MEK); extracellular receptor signal-related kinase (ERK); insulin receptor substrate 1 (IRS-1); nuclear factor ‘kappa-light-chain-enhancer’ of activated B-cells (NF-κB); iκappaB kinase (IκB); low density lipoprotein receptor-related protein (LRP); glycogen synthase kinase-3β (GSK-3β); adenomatous polyposis coli (APC); phospholipase Cγ (PLCγ), protein kinase C (PKC); epithelial to mesenchymal transition (EMT); phosphate (PO43−), 1,25(OH)2D3 (active vitamin D).
FGF23/KL, Phosphate Homeostasis, and Cancer
FGF23/FGFR/KL regulate renal phosphate handling (Gattineni et al., 2009). Moreover, FGF23 indirectly impacts on phosphate by inhibiting 1,25(OH)2D3 formation (Chanakul et al., 2013) and by affecting PTH (Krajisnik et al., 2007; Kawakami et al., 2017). Hence, FGF23/KL have a central role in the interaction of bone, kidney, small intestine, and parathyroid gland, maintaining phosphate homeostasis (Razzaque, 2009b). Serum phosphate levels are higher in patients with cancer than in healthy individuals (Papaloucas et al., 2014). Higher phosphate concentrations in men are related to a higher overall cancer risk (Wulaningsih et al., 2013), and higher phosphate intake accelerates tumorigenesis in mice (Lee et al., 2015), uncovering phosphate as a possible factor in cancer (Brown and Razzaque, 2018). Accordingly, CKD patients, often exhibiting hyperphosphatemia and 1,25(OH)2D3 deficiency, have an increased risk of cancer (Wong et al., 2009, 2016; Park et al., 2019). 1,25(OH)2D3 may have anti-cancer activity (Vanoirbeek et al., 2011). According to Brown’s hypothesis, hyperphosphatemia is an important factor in tumorigenesis and at the same time causes an endocrine reduction of 1,25(OH)2D3, which in turn is associated with an increased risk of cancer (Brown, 2019). For this hypothesis, FGF23/KL plays an important role due to its pivotal function in phosphate handling. Definitely, further research on pathological derangements of phosphate homeostasis is warranted to uncover the relationship between FGF23/KL dysregulation, disturbed phosphate homeostasis, and cancer development.
Conclusion
KL seems to be an universal tumor suppressor in many different tumor entities owing to its inhibitory effect on pro-survival intracellular pathways including IGF-1R/PI3K/AKT or Wnt signaling. Often, cell culture studies revealed similar actions of sKL and overexpression of transmembrane KL in different types of cancer. Whether targeting KL can be therapeutically exploited in cancer must be investigated in future trials. In most studies and types of cancer, higher abundance of sKL is associated with a more favorable prognosis, presumably due to its down-regulatory effect on major prosurvival signaling cascades required for cancer progression. The investigations into the role of FGF23 in cancer have so far revealed two important aspects in general: In those forms of cancer affecting bone or originating from it such as MM or prostate cancer, FGF23 signaling may directly contribute to cancer biology/progression. In many other tumor entities, the biological role of an elevation of the plasma FGF23 concentration is still enigmatic, but FGF23 may serve as a (tumor) biomarker. In TIO, treatment with anti-FGF23 monoclonal antibody offers a beneficial therapeutic intervention. In other malignancies affecting bone including prostate cancer or MM, an anti-FGF23 approach may also be useful as enhanced FGF23 or FGF23 signaling is typical of these tumor entities. Clearly, this and the role of FGF23-dependent phosphate metabolism in cancer require further studies.
Author Contributions
All authors listed have made a substantial, direct and intellectual contribution to the work, and approved it for publication.
Funding
MFö’s work in the field of FGF23 was supported by the Deutsche Forschungsgemeinschaft (Fo 695/2-2 and Fo 695/6-1).
Conflict of Interest
The authors declare that the research was conducted in the absence of any commercial or financial relationships that could be construed as a potential conflict of interest.
Abbreviations
1,25(OH)2D3, active vitamin D; ADAM10, A disintegrin and metalloproteinase domain-containing proteins 10; ADAM17, A disintegrin and metalloproteinase domain-containing proteins 17; ALPL, alkaline phosphatase; BACE1, β-APP cleaving enzyme 1; CKD, chronic kidney disease; CRC, colorectal cancer; CYP, cytochrome P 450; DDLPS, dedifferentiated liposarcoma; DLBLC, diffuse large B-cell lymphoma; EGR-1, early gene response transcription factor 1; EMT, epithelial to mesenchymal transition; EOC, epithelial ovarian cancer; ER, endoplasmic reticulum; ERK1/2, extracellular receptor signal-related kinase ½; FAM20C, family with sequence similarity 20 member C; FGF, fibroblast growth factor; FGFR, fibroblast growth factor receptor; FOXO, forkhead box O; GalNT3, UDP-N-acetyl-alpha-D galactosamine:polypeptide N acetylgalactosaminyltransferase 3; GFR, glomerular filtration rate; GBM, glioblastoma multiforme; GSK-3 β, glycogen synthase kinase-3 β; HCC, hepatocellular carcinoma; HIF-1 α, hypoxia-inducible factor 1 α; HMGB1, high-mobility group protein B 1; HPSE, heparanase; HR, hazard ratio; IGF-1, insulin-like growth factor 1; IL, interleukin; KL, α Klotho; KLB, β Klotho; KLG, γ Klotho; Klph, Klotho lactase-phlorizin hydrolase-related protein; MAPK, mitogen-activated protein kinase; MDS, myelodysplastic syndromes; miR, micro ribonucleic acid; MM, multiple myeloma; mTOR, mammalian target of rapamycin; NaPiIIa, sodium phosphate cotransporter 2a; NF- κ B, nuclear factor ‘kappa-light-chain-enhancer’ of activated B-cells; PDAC, pancreatic ductal adenocarcinoma; PPAR, peroxisome proliferator–activated receptor; PI3K, phosphoinositide 3-kinase; PTH, parathyroid hormone; RCC, renal cell carcinoma; ROMK1, renal outer medullary potassium channel 1; sKL, soluble Klotho; SCC, squamous cell carcinoma; SNP, single-nucleotide polymorphism; SOX17, sex determining region Y (SRY) – related high-mobility group (HMG) box protein family member 17; TNM, tumor nodes metastasis; TIO, tumor-induced osteomalacia; TRPV5, transient receptor potential ion channel 5; VDR, vitamin D receptor; Wnt, wingless-related integration site.
References
Abate, E. G., Bernet, V., Cortese, C., and Garner, H. W. (2016). Tumor induced osteomalacia secondary to anaplastic thyroid carcinoma: a case report and review of the literature. Bone Rep. 5, 81–85. doi: 10.1016/j.bonr.2015.11.004.
Abramovitz, L., Rubinek, T., Ligumsky, H., Bose, S., Barshack, I., Avivi, C., et al. (2011). KL1 internal repeat mediates klotho tumor suppressor activities and inhibits bFGF and IGF-I signaling in pancreatic cancer. Clin. Cancer Res. 17, 4254–4266. doi: 10.1158/1078-0432.CCR-10-2749.
Adhikari, B. R., Uehara, O., Matsuoka, H., Takai, R., Harada, F., Utsunomiya, M., et al. (2017). Immunohistochemical evaluation of Klotho and DNA methyltransferase 3a in oral squamous cell carcinomas. Med. Mol. Morphol. 50, 155–160. doi: 10.1007/s00795-017-0156-9.
ADHR Consortium (2000). Autosomal dominant hypophosphataemic rickets is associated with mutations in FGF23. Nat. Genet. 26, 345–348. doi: 10.1038/81664.
Akimoto, T., Yoshizawa, H., Watanabe, Y., Numata, A., Yamazaki, T., Takeshima, E., et al. (2012). Characteristics of urinary and serum soluble Klotho protein in patients with different degrees of chronic kidney disease. BMC Nephrol. 13:155. doi: 10.1186/1471-2369-13-155.
Alsofyani, A. A., Alsiary, R. A., Samkari, A., Alhaj-Hussain, B. T., Khan, J. A., Al-Maghrabi, J., et al. (2017). Prognostic potential of KLOTHO and SFRP1 promoter methylation in head and neck squamous cell carcinoma. J. Appl. Genet. 58, 459–465. doi: 10.1007/s13353-017-0404-7.
Andrukhova, O., Bayer, J., Schüler, C., Zeitz, U., Murali, S. K., Ada, S., et al. (2017). Klotho Lacks an FGF23-independent role in mineral homeostasis. J. Bone Min. Res. 32, 2049–2061. doi: 10.1002/jbmr.3195.
Angelin, B., Larsson, T. E., and Rudling, M. (2012). Circulating fibroblast growth factors as metabolic regulators–a critical appraisal. Cell Metab. 16, 693–705. doi: 10.1016/j.cmet.2012.11.001.
Anour, R., Andrukhova, O., Ritter, E., Zeitz, U., and Erben, R. G. (2012). Klotho lacks a vitamin D independent physiological role in glucose homeostasis, bone turnover, and steady-state PTH secretion in vivo. PLoS One 7:e31376. doi: 10.1371/journal.pone.0031376.
Arbel Rubinstein, T., Shahmoon, S., Zigmond, E., Etan, T., Merenbakh-Lamin, K., Pasmanik-Chor, M., et al. (2019). Klotho suppresses colorectal cancer through modulation of the unfolded protein response. Oncogene 38, 794–807. doi: 10.1038/s41388-018-0489-4.
Arner, P., Pettersson, A., Mitchell, P. J., Dunbar, J. D., Kharitonenkov, A., and Rydén, M. (2008). FGF21 attenuates lipolysis in human adipocytes - a possible link to improved insulin sensitivity. FEBS Lett. 582, 1725–1730. doi: 10.1016/j.febslet.2008.04.038.
Arslan, M. S., Sahin, M., Karakose, M., Tutal, E., Topaloglu, O., Ucan, B., et al. (2017). Serum Levels Of Fibroblast Growth Factor-23, Osteoprotegerin, And Receptor Activator Of nuclear factor kappa B ligand in patients with prolactinoma. Endocr. Pract. 23, 266–370. doi: 10.4158/EP161440.OR
Aukes, K., Forsman, C., Brady, N. J., Astleford, K., Blixt, N., Sachdev, D., et al. (2017). Breast cancer cell-derived fibroblast growth factors enhance osteoclast activity and contribute to the formation of metastatic lesions. PLoS One 12:e0185736. doi: 10.1371/journal.pone.0185736.
Aunan, J. R., Cho, W. C., and Søreide, K. (2017). The biology of aging and cancer: a brief overview of shared and divergent molecular hallmarks. Aging Dis. 8, 628–642. doi: 10.14336/AD.2017.0103.
Aviel-Ronen, S., Rubinek, T., Zadok, O., Vituri, A., Avivi, C., Wolf, I., et al. (2016). Klotho expression in cervical cancer: differential expression in adenocarcinoma and squamous cell carcinoma. J. Clin. Pathol. 69, 53–57. doi: 10.1136/jclinpath-2015-202929.
Badve, S., and Kumar, G. L. eds (2019). Predictive Biomarkers in Oncology. Cham: Springer International Publishing.
Behera, R., Kaur, A., Webster, M. R., Kim, S., Ndoye, A., Kugel, C. H., et al. (2017). Inhibition of age-related therapy resistance in melanoma by rosiglitazone-mediated induction of klotho. Clin. Cancer Res. 23, 3181–3190. doi: 10.1158/1078-0432.CCR-17-0201.
Ben-Dov, I. Z., Galitzer, H., Lavi-Moshayoff, V., Goetz, R., Kuro-O, M., Mohammadi, M., et al. (2007). The parathyroid is a target organ for FGF23 in rats. J. Clin. Invest. 117, 4003–4008. doi: 10.1172/JCI32409.
Blau, J. E., and Collins, M. T. (2015). The PTH-Vitamin D-FGF23 axis. Rev. Endocr. Metab. Disord. 16, 165–174. doi: 10.1007/s11154-015-9318-z.
Bloch, L., Sineshchekova, O., Reichenbach, D., Reiss, K., Saftig, P., Kuro-O, M., et al. (2009). Klotho is a substrate for alpha-, beta- and gamma-secretase. FEBS Lett. 583, 3221–3224. doi: 10.1016/j.febslet.2009.09.009.
Boland, J. M., Tebben, P. J., and Folpe, A. L. (2018). Phosphaturic mesenchymal tumors: what an endocrinologist should know. J. Endocrinol. Invest. 41, 1173–1184. doi: 10.1007/s40618-018-0849-5.
Bordonaro, M., and Lazarova, D. L. (2015). Hypothesis: cell signalling influences age-related risk of colorectal cancer. J. Cell. Mol. Med. 19, 74–81. doi: 10.1111/jcmm.12366.
Brominska, B., Gabryel, P., Jarmołowska-Jurczyszyn, D., Janicka-Jedyńska, M., Kluk, A., Trojanowski, M., et al. (2019). Klotho expression and nodal involvement as predictive factors for large cell lung carcinoma. Arch. Med. Sci. 15, 1010–1016. doi: 10.5114/aoms.2018.75889.
Brown, R. B. (2019). Vitamin D, cancer, and dysregulated phosphate metabolism. Endocrine 65, 238–243. doi: 10.1007/s12020-019-01985-y.
Brown, R. B., and Razzaque, M. S. (2018). Phosphate toxicity and tumorigenesis. Biochim. Biophys. Acta Rev. Cancer 1869, 303–309. doi: 10.1016/j.bbcan.2018.04.007.
Camilli, T. C., Xu, M., O’Connell, M. P., Chien, B., Frank, B. P., Subaran, S., et al. (2011). Loss of Klotho during melanoma progression leads to increased filamin cleavage, increased Wnt5A expression, and enhanced melanoma cell motility. Pigment Cell Melan. Res. 24, 175–186. doi: 10.1111/j.1755-148X.2010.00792.x.
Cha, S. -K., Hu, M. -C., Kurosu, H., Kuro-O, M., Moe, O., and Huang, C. -L. (2009). Regulation of renal outer medullary potassium channel and renal K(+) excretion by Klotho. Mol. Pharmacol. 76, 38–46. doi: 10.1124/mol.109.055780.
Cha, S. -K., Ortega, B., Kurosu, H., Rosenblatt, K. P., Kuro-O, M., and Huang, C. L. (2008). Removal of sialic acid involving Klotho causes cell-surface retention of TRPV5 channel via binding to galectin-1. Proc. Natl. Acad. Sci. U.S.A. 105, 9805–9810. doi: 10.1073/pnas.0803223105.
Chanakul, A., Zhang, M. Y. H., Louw, A., Armbrecht, H. J., Miller, W. L., Portale, A. A., et al. (2013). FGF-23 regulates CYP27B1 transcription in the kidney and in extra-renal tissues. PLoS One 8:e72816. doi: 10.1371/journal.pone.0072816.
Chang, B., Kim, J., Jeong, D., Jeong, Y., Jeon, S., Jung, S. I., et al. (2012). Klotho inhibits the capacity of cell migration and invasion in cervical cancer. Oncol. Rep. 28, 1022–1028. doi: 10.3892/or.2012.1865.
Chang, Q., Hoefs, S., van der Kemp, A. W., Topala, C. N., Bindels, R. J., and Hoenderop, J. G. (2005). The beta-glucuronidase klotho hydrolyzes and activates the TRPV5 channel. Science 310, 490–493. doi: 10.1126/science.1114245.
Chen, B., Huang, S., Pisanic Ii, T. R., Stark, A., Tao, Y., Cheng, B., et al. (2019). Rab8 GTPase regulates Klotho-mediated inhibition of cell growth and progression by directly modulating its surface expression in human non-small cell lung cancer. eBio Med. 49, 118–132. doi: 10.1016/j.ebiom.2019.10.040.
Chen, B., Liang, Y., Chen, L., Wei, Y., Li, Y., Zhao, W., et al. (2018). Overexpression of Klotho Inhibits HELF Fibroblasts SASP-related protumoral effects on non-small cell lung cancer cells. J. Cancer 9, 1248–1258. doi: 10.7150/jca.23967.
Chen, B., Ma, X., Liu, S., Zhao, W., and Wu, J. (2012). Inhibition of lung cancer cells growth, motility and induction of apoptosis by Klotho, a novel secreted Wnt antagonist, in a dose-dependent manner. Cancer Biol. Ther. 13, 1221–1228. doi: 10.4161/cbt.21420.
Chen, B., Wang, X., Zhao, W., and Wu, J. (2010). Klotho inhibits growth and promotes apoptosis in human lung cancer cell line A549. J. Exp. Clin. Cancer Res. 29, 99. doi: 10.1186/1756-9966-29-99.
Chen, C. -D., Podvin, S., Gillespie, E., Leeman, S. E., and Abraham, C. R. (2007). Insulin stimulates the cleavage and release of the extracellular domain of Klotho by ADAM10 and ADAM17. Proc. Natl. Acad. Sci. U.S.A. 104, 19796–19801. doi: 10.1073/pnas.0709805104.
Chen, G., Liu, Y., Goetz, R., Fu, L., Jayaraman, S., Hu, M. C., et al. (2018). α-Klotho is a non-enzymatic molecular scaffold for FGF23 hormone signalling. Nature 553, 461–466. doi: 10.1038/nature25451.
Chen, L., Liu, H., Liu, J., Zhu, Y., Le, X., He, H., et al. (2013). Klotho Endows Hepatoma Cells with Resistance to Anoikis via VEGFR2/PAK1 Activation in Hepatocellular Carcinoma. PLoS One 8:e0058413. doi: 10.1371/journal.pone.0058413.
Chen, T., Ren, H., Thakur, A., Yang, T., Li, Y., Zhang, S., et al. (2016). Decreased level of klotho contributes to drug resistance in lung cancer cells: involving in klotho-mediated cell autophagy. DNA Cell Biol. 35, 751–757. doi: 10.1089/dna.2016.3437.
Chiam, P., Tan, H. C., Bee, Y. M., and Chandran, M. (2013). Oncogenic osteomalacia – hypophosphataemic spectrum from “benignancy” to “malignancy”. Bone 53, 182–187. doi: 10.1016/j.bone.2012.11.040.
Choudhary, S., Ramasundaram, P., Dziopa, E., Mannion, C., Kissin, Y., Tricoli, L., et al. (2018). Human ex vivo 3D bone model recapitulates osteocyte response to metastatic prostate cancer. Sci. Rep. 8:17975. doi: 10.1038/s41598-018-36424-x.
Colazo, J. M., Thompson, R. C., Covington, N. V., and Dahir, K. M. (2020). An intracranial mass causing tumor-induced osteomalacia (TIO): rapid and complete resolution of severe osteoporosis after surgical resection. Radiol. Case Rep. 15, 492–497. doi: 10.1016/j.radcr.2020.01.039.
Cotant, C. L., and Rao, P. S. (2007). Elevated fibroblast growth factor 23 in a patient with metastatic prostate cancer and hypophosphatemia. Am. J. Kidney Dis. 50, 1033–1036. doi: 10.1053/j.ajkd.2007.07.031.
Cymbaluk-Płoska, A., Gargulińska, P., Chudecka-Głaz, A., Kwiatkowski, S., Pius-Sadowska, E., and Machaliński, B. (2020). The suitability of FGF21 and FGF23 as new biomarkers in endometrial cancer patients. Diagnostics 10:414. doi: 10.3390/diagnostics10060414.
Czaya, B., and Faul, C. (2019). The role of fibroblast growth Factor 23 in inflammation and anemia. Int. J Mol. Sci. 20:4195. doi: 10.3390/ijms20174195.
Dai, D., Wang, Q., Li, X., Liu, J., Ma, X., and Xu, W. (2016). Klotho inhibits human follicular thyroid cancer cell growth and promotes apoptosis through regulation of the expression of stanniocalcin-1. Oncol. Rep. 35, 552–558. doi: 10.3892/or.2015.4358.
Dallol, A., Buhmeida, A., Merdad, A., Al-Maghrabi, J., Gari, M. A., Abu-Elmagd, M. M., et al. (2015). Frequent methylation of the KLOTHO gene and overexpression of the FGFR4 receptor in invasive ductal carcinoma of the breast. Tumour Biol. 36, 9677–9683. doi: 10.1007/s13277-015-3733-3733.
Dalton, G. D., Xie, J., An, S. -W., and Huang, C. L. (2017). New Insights into the Mechanism of Action of Soluble Klotho. Front. Endocrinol. 8:323. doi: 10.3389/fendo.2017.00323.
Dehghani, M., Brobey, R. K., Wang, Y., Souza, G., Amato, R. J., and Rosenblatt, K. P. (2018). Klotho inhibits EGF-induced cell migration in Caki-1 cells through inactivation of EGFR and p38 MAPK signaling pathways. Oncotarget 9, 26737–26750. doi: 10.18632/oncotarget.25481.
Delcroix, V., Mauduit, O., Tessier, N., Montillaud, A., Lesluyes, T., Ducret, T., et al. (2018). The role of the anti-aging protein klotho in IGF-1 signaling and reticular calcium leak: impact on the chemosensitivity of dedifferentiated liposarcomas. Cancers 10:439. doi: 10.3390/cancers10110439.
Dhiman, M., Thakur, S., Upadhyay, S., Kaur, A., and Mantha, A. K. (2015). “Oxidative Stress and Inflammation in Cardiovascular Diseases: Two Sides of the Same Coin,” in Free Radicals in Human Health and Disease, ed. V. Rani and U. C. S. Yadav (New Delhi: Springer), 259–278.
Dolegowska, K., Marchelek-Mysliwiec, M., Nowosiad-Magda, M., Slawinski, M., and Dolegowska, B. (2019). FGF19 subfamily members: FGF19 and FGF21. J. Physiol. Biochem. 75, 229–240. doi: 10.1007/s13105-019-00675-7.
Elderman, J. H., Wabbijn, M., and de Jongh, F. (2016). Hypophosphataemia due to FGF-23 producing B cell non-Hodgkin’s lymphoma. BMJ case reports 2016:bcr2015213954. doi: 10.1136/bcr-2015-213954.
Faul, C., Amaral, A. P., Oskouei, B., Hu, M. -C., Sloan, A., Isakova, T., et al. (2011). FGF23 induces left ventricular hypertrophy. J. Clin. Invest. 121, 4393–4408. doi: 10.1172/JCI46122.
Feng, S., Shao, L., Yu, W., Gavine, P., and Ittmann, M. (2012). Targeting fibroblast growth factor receptor signaling inhibits prostate cancer progression. Clin. Cancer Res. 18, 3880–3888. doi: 10.1158/1078-0432.CCR-11-3214.
Feng, S., Wang, J., Zhang, Y., Creighton, C. J., and Ittmann, M. (2015). FGF23 promotes prostate cancer progression. Oncotarget 6, 17291–17301. doi: 10.18632/oncotarget.4174.
Fon Tacer, K., Bookout, A. L., Ding, X., Kurosu, H., John, G. B., Wang, L., et al. (2010). Research resource: comprehensive expression atlas of the fibroblast growth factor system in adult mouse. Mol. Endocrinol. 24, 2050–2064. doi: 10.1210/me.2010-2142.
Gan, L. H., Pan, J., Chen, S. J., Zhong, J., and Wang, L. J. (2011). DNA methylation of ZIC1 and KLOTHO gene promoters in colorectal carcinomas and its clinicopathological significance. J. Zhejiang Univ. Med. Sci. 40, 309–314.
Gattineni, J., Bates, C., Twombley, K., Dwarakanath, V., Robinson, M. L., Goetz, R., et al. (2009). FGF23 decreases renal NaPi-2a and NaPi-2c expression and induces hypophosphatemia in vivo predominantly via FGF receptor 1. Am. J. Physiol. Renal Physiol. 297, F282–F291. doi: 10.1152/ajprenal.90742.2008.
Gattineni, J., Twombley, K., Goetz, R., Mohammadi, M., and Baum, M. (2011). Regulation of serum 1,25(OH)2 vitamin D3 levels by fibroblast growth factor 23 is mediated by FGF receptors 3 and 4. Am. J. Physiol. Renal Physiol. 301, F371–F377. doi: 10.1152/ajprenal.00740.2010.
Gigante, M., Lucarelli, G., Divella, C., Netti, G. S., Pontrelli, P., Cafiero, C., et al. (2015). Soluble Serum αKlotho is a potential predictive marker of disease progression in clear cell renal cell carcinoma. Medicine 94:e1917. doi: 10.1097/MD.0000000000001917.
Gu, H., Jiang, W., You, N., Huang, X., Li, Y., Peng, X., et al. (2020). Soluble Klotho Improves Hepatic Glucose and Lipid homeostasis in type 2 diabetes. Mol. Ther. Methods Clin. Dev. 18, 811–823. doi: 10.1016/j.omtm.2020.08.002.
Gutierrez, O., Isakova, T., Rhee, E., Shah, A., Holmes, J., Collerone, G., et al. (2005). Fibroblast growth factor-23 mitigates hyperphosphatemia but accentuates calcitriol deficiency in chronic kidney disease. J. Am. Soc. Nephrol. 16, 2205–2215. doi: 10.1681/ASN.2005010052.
Han, X., Li, L., Yang, J., King, G., Xiao, Z., and Quarles, L. D. (2016). Counter-regulatory paracrine actions of FGF-23 and 1,25(OH)2 D in macrophages. FEBS Lett. 590, 53–67. doi: 10.1002/1873-3468.12040.
Hasegawa, H., Nagano, N., Urakawa, I., Yamazaki, Y., Iijima, K., Fujita, T., et al. (2010). Direct evidence for a causative role of FGF23 in the abnormal renal phosphate handling and vitamin D metabolism in rats with early-stage chronic kidney disease. Kidney Int. 78, 975–980. doi: 10.1038/ki.2010.313.
He, X. -J., Ma, Y. -Y., Yu, S., Jiang, X. -T., Lu, Y. -D., Tao, L., et al. (2014). Up-regulated miR-199a-5p in gastric cancer functions as an oncogene and targets klotho. BMC Cancer 14:218. doi: 10.1186/1471-2407-14-218.
Hori, S., Miyake, M., Onishi, S., Tatsumi, Y., Morizawa, Y., Nakai, Y., et al. (2016). Clinical significance of α- and β-Klotho in urothelial carcinoma of the bladder. Oncol. Rep. 36, 2117–2125. doi: 10.3892/or.2016.5053.
Hori, S., Miyake, M., Tatsumi, Y., Morizawa, Y., Nakai, Y., Onishi, S., et al. (2018). Gamma-Klotho exhibits multiple roles in tumor growth of human bladder cancer. Oncotarget 9, 19508–19524. doi: 10.18632/oncotarget.24628.
Hu, M. C., Shi, M., Zhang, J., Quiñones, H., Griffith, C., Kuro-O, M., et al. (2011). Klotho deficiency causes vascular calcification in chronic kidney disease. J. Am. Soc. Nephrol. 22, 124–136. doi: 10.1681/ASN.2009121311.
Huang, D., and Du, X. (2008). Crosstalk between tumor cells and microenvironment via Wnt pathway in colorectal cancer dissemination. World J. Gastroenterol. 14, 1823–1827. doi: 10.3748/wjg.14.1823.
Huang, J., Sun, C., Wang, S., He, Q., and Li, D. (2015). microRNA miR-10b inhibition reduces cell proliferation and promotes apoptosis in non-small cell lung cancer (NSCLC) cells. Mol. Biosyst. 11, 2051–2059. doi: 10.1039/c4mb00752b.
Ibi, T., Usuda, J., Inoue, T., Sato, A., and Takegahara, K. (2017). Klotho expression is correlated to molecules associated with epithelial-mesenchymal transition in lung squamous cell carcinoma. Oncol. Lett. 14, 5526–5532. doi: 10.3892/ol.2017.6862.
Imura, A., Iwano, A., Tohyama, O., Tsuji, Y., Nozaki, K., Hashimoto, N., et al. (2004). Secreted Klotho protein in sera and CSF: implication for post-translational cleavage in release of Klotho protein from cell membrane. FEBS Lett. 565, 143–147. doi: 10.1016/j.febslet.2004.03.090.
Inagaki, T., Choi, M., Moschetta, A., Peng, L., Cummins, C. L., McDonald, J. G., et al. (2005). Fibroblast growth factor 15 functions as an enterohepatic signal to regulate bile acid homeostasis. Cell Metab. 2, 217–225. doi: 10.1016/j.cmet.2005.09.001.
Isakova, T., Wahl, P., Vargas, G. S., Gutiérrez, O. M., Scialla, J., Xie, H., et al. (2011). Fibroblast growth factor 23 is elevated before parathyroid hormone and phosphate in chronic kidney disease. Kidney Int. 79, 1370–1378. doi: 10.1038/ki.2011.47.
Ito, S., Fujimori, T., Furuya, A., Satoh, J., Nabeshima, Y., and Nabeshima, Y. I. (2005). Impaired negative feedback suppression of bile acid synthesis in mice lacking betaKlotho. J. Clin. Invest. 115, 2202–2208. doi: 10.1172/JCI23076.
Ito, S., Fujimori, T., Hayashizaki, Y., and Nabeshima, Y. I. (2002). Identification of a novel mouse membrane-bound family 1 glycosidase-like protein, which carries an atypical active site structure. Biochimica et Biophysica Acta 1576, 341–345. doi: 10.1016/s0167-4781(02)00281-6.
Ito, S., Kinoshita, S., Shiraishi, N., Nakagawa, S., Sekine, S., Fujimori, T., et al. (2000). Molecular cloning and expression analyses of mouse βklotho, which encodes a novel Klotho family protein. Mechanisms of Development 98, 115–119. doi: 10.1016/S0925-4773(00)00439-1.
Jacobs, E., Martinez, M. E., Buckmeier, J., Lance, P., May, M., and Jurutka, P. (2011). Circulating fibroblast growth factor-23 is associated with increased risk for metachronous colorectal adenoma. J. Carcinogen. 10:3. doi: 10.4103/1477-3163.76723.
Jiang, B., Gu, Y., and Chen, Y. (2014). Identification of novel predictive markers for the prognosis of pancreatic ductal adenocarcinoma. Cancer Invest. 32, 218–225. doi: 10.3109/07357907.2014.905586.
Kamal, A., Salama, M., Kamal, A., Mohsen, A., and Siam, I. (2020). Klotho (rs1207568 and rs564481) gene variants and colorectal cancer risk. Turkish J. Gastroenterol. 31, 497–502. doi: 10.5152/tjg.2020.19235.
Kanagala, P., Arnold, J. R., Khan, J. N., Singh, A., Gulsin, G. S., Eltayeb, M., et al. (2020). Fibroblast-growth-factor-23 in heart failure with preserved ejection fraction: relation to exercise capacity and outcomes. ESC Heart Fail. doi: 10.1002/ehf2.13020
Kawakami, K., Takeshita, A., Furushima, K., Miyajima, M., Hatamura, I., Kuro-O, M., et al. (2017). Persistent fibroblast growth factor 23 signalling in the parathyroid glands for secondary hyperparathyroidism in mice with chronic kidney disease. Sci. Rep. 7:40534. doi: 10.1038/srep40534.
Kim, H. J., Kim, K. -H., Lee, J., Oh, J. J., Cheong, H. S., Wong, E. L., et al. (2014a). Single nucleotide polymorphisms in fibroblast growth factor 23 gene, FGF23, are associated with prostate cancer risk. BJU Int. 114, 303–310. doi: 10.1111/bju.12396.
Kim, H. J., Lee, J., Lee, S. Y., Cheong, H. S., Kye, Y. -S., Kim, W., et al. (2014b). The association between KL polymorphism and prostate cancer risk in Korean patients. Mol. Biol. Rep. 41, 7595–7606. doi: 10.1007/s11033-014-3647-y.
Kim, J. -H., Hwang, K. -H., Lkhagvadorj, S., Jung, J. H., Chung, H. C., Park, K. -S., et al. (2016). Klotho plays a critical role in clear cell renal cell carcinoma progression and clinical outcome. Korean J. Physiol. Pharmacol. 20, 297–304. doi: 10.4196/kjpp.2016.20.3.297.
Kimura, T., Shiizaki, K., Akimoto, T., Shinzato, T., Shimizu, T., Kurosawa, A., et al. (2018). The impact of preserved Klotho gene expression on antioxidative stress activity in healthy kidney. Am. J. Physiol. Renal Physiol. 315, F345–F352. doi: 10.1152/ajprenal.00486.2017.
Kinoshita, Y., Takashi, Y., Ito, N., Ikegawa, S., Mano, H., Ushiku, T., et al. (2019). Ectopic expression of Klotho in fibroblast growth factor 23 (FGF23)-producing tumors that cause tumor-induced rickets/osteomalacia (TIO). Bone Rep. 10:100192. doi: 10.1016/j.bonr.2018.100192.
Koh, N., Fujimori, T., Nishiguchi, S., Tamori, A., Shiomi, S., Nakatani, T., et al. (2001). Severely reduced production of klotho in human chronic renal failure kidney. Biochem. Biophys. Res. Commun. 280, 1015–1020. doi: 10.1006/bbrc.2000.4226.
Komaba, H., Goto, S., Fujii, H., Hamada, Y., Kobayashi, A., Shibuya, K., et al. (2010). Depressed expression of Klotho and FGF receptor 1 in hyperplastic parathyroid glands from uremic patients. Kidney Int. 77, 232–238. doi: 10.1038/ki.2009.414.
Krajisnik, T., Björklund, P., Marsell, R., Ljunggren, O., Akerström, G., Jonsson, K. B., et al. (2007). Fibroblast growth factor-23 regulates parathyroid hormone and 1alpha-hydroxylase expression in cultured bovine parathyroid cells. J. Endocrinol. 195, 125–131. doi: 10.1677/JOE-07-0267.
Kuro-O, M. (2008). Klotho as a regulator of oxidative stress and senescence. Biol. Chem. 389, 233–241. doi: 10.1515/BC.2008.028.
Kuro-O, M. (2019). The Klotho proteins in health and disease. Nat. Rev. Nephrol. 15, 27–44. doi: 10.1038/s41581-018-0078-3.
Kuro-O, M., Matsumura, Y., Aizawa, H., Kawaguchi, H., Suga, T., Utsugi, T., et al. (1997). Mutation of the mouse klotho gene leads to a syndrome resembling ageing. Nature 390, 45–51. doi: 10.1038/36285.
Kurosu, H., Choi, M., Ogawa, Y., Dickson, A. S., Goetz, R., Eliseenkova, A. V., et al. (2007). Tissue-specific expression of betaKlotho and fibroblast growth factor (FGF) receptor isoforms determines metabolic activity of FGF19 and FGF21. J. Biol. Chem. 282, 26687–26695. doi: 10.1074/jbc.M704165200.
Kurosu, H., Yamamoto, M., Clark, J. D., Pastor, J. V., Nandi, A., Gurnani, P., et al. (2005). Suppression of aging in mice by the hormone Klotho. Science 309, 1829–1833. doi: 10.1126/science.1112766.
Larsson, T., Zahradnik, R., Lavigne, J., Ljunggren, O., Jüppner, H., and Jonsson, K. B. (2003). Immunohistochemical detection of FGF-23 protein in tumors that cause oncogenic osteomalacia. Eur. J. Endocrinol. 148, 269–276. doi: 10.1530/eje.0.1480269.
Leaf, D. E., Pereira, R. C., Bazari, H., and Jüppner, H. (2013). Oncogenic osteomalacia due to FGF23-expressing colon adenocarcinoma. J. Clin. Endocrinol. Metab. 98, 887–891. doi: 10.1210/jc.2012-3473.
Lee, E. K., Martinez, M. C. R., Blakely, K., Santos, K. D., van Hoang, C., Chow, A., et al. (2014). FGF23: mediator of poor prognosis in a sizeable subgroup of patients with castration-resistant prostate cancer presenting with severe hypophosphatemia? Med. Hypotheses 83, 482–487. doi: 10.1016/j.mehy.2014.08.005.
Lee, J., Jeong, D. -J., Kim, J., Lee, S., Park, J. -H., Chang, B., et al. (2010). The anti-aging gene KLOTHO is a novel target for epigenetic silencing in human cervical carcinoma. Mol. Cancer 9:109. doi: 10.1186/1476-4598-9-109.
Lee, S., Kim, J. -E., Hong, S. -H., Lee, A. -Y., Park, E. -J., Seo, H. W., et al. (2015). High inorganic phosphate intake promotes tumorigenesis at early stages in a mouse model of lung cancer. PLoS One 10:e0135582. doi: 10.1371/journal.pone.0135582.
Lewis, C., and Murdoch, C. (2005). Macrophage responses to hypoxia. Am. J. Pathol. 167, 627–635. doi: 10.1016/S0002-9440(10)62038-X.
Li, J., Chen, Y., Guo, X., Zhou, L., Jia, Z., Tang, Y., et al. (2016). Inhibition of miR-15b decreases cell migration and metastasis in colorectal cancer. Tumour Biol. 37, 8765–8773. doi: 10.1007/s13277-015-4396-9.
Li, J. -R., Chiu, K. -Y., Ou, Y. -C., Wang, S. -S., Chen, C. -S., Yang, C. -K., et al. (2019). Alteration in serum concentrations of FGF19, FGF21, and FGF23 in patients with urothelial carcinoma. BioFactors 45, 62–68. doi: 10.1002/biof.1460.
Li, Q., Li, Y., Liang, L., Li, J., Luo, D., Liu, Q., et al. (2018). Klotho negatively regulated aerobic glycolysis in colorectal cancer via ERK/HIF1α axis. Cell Commun. Signal. 16:26. doi: 10.1186/s12964-018-0241-2.
Li, X. -X., Huang, L. -Y., Peng, J. -J., Liang, L., Shi, D. -B., Zheng, H. -T., et al. (2014). Klotho suppresses growth and invasion of colon cancer cells through inhibition of IGF1R-mediated PI3K/AKT pathway. Int. J. Oncol. 45, 611–618. doi: 10.3892/ijo.2014.2430.
Lim, K., Groen, A., Molostvov, G., Lu, T., Lilley, K. S., Snead, D., et al. (2015). α-Klotho Expression in Human Tissues. J. Clin. Endocrinol. Metab. 100, E1308–E1318. doi: 10.1210/jc.2015-1800.
Lin, H. -A., Shih, S. -R., Tseng, Y. -T., Chen, C. -H., Chiu, W. -Y., Hsu, C. -Y., et al. (2014). Ovarian cancer-related hypophosphatemic osteomalacia–a case report. J. Clin. Endocrinol. Metab. 99, 4403–4407. doi: 10.1210/jc.2014-0.
Liu, H., Fergusson, M. M., Castilho, R. M., Liu, J., Cao, L., Chen, J., et al. (2007). Augmented Wnt signaling in a mammalian model of accelerated aging. Science 317, 803–806. doi: 10.1126/science.1143578.
Liu, Y., Pan, J., Pan, X., Wu, L., Bian, J., Lin, Z., et al. (2019). Klotho-mediated targeting of CCL2 suppresses the induction of colorectal cancer progression by stromal cell senescent microenvironments. Mol. Oncol. 13, 2460–2475. doi: 10.1002/1878-0261.12577.
Lojkin, I., Rubinek, T., Orsulic, S., Schwarzmann, O., Karlan, B. Y., Bose, S., et al. (2015). Reduced expression and growth inhibitory activity of the aging suppressor klotho in epithelial ovarian cancer. Cancer Lett. 362, 149–157. doi: 10.1016/j.canlet.2015.03.035.
Lu, L., Katsaros, D., Wiley, A., La Longrais, I. A. R., de Puopolo, M., and Yu, H. (2008). Klotho expression in epithelial ovarian cancer and its association with insulin-like growth factors and disease progression. Cancer Invest. 26, 185–192. doi: 10.1080/07357900701638343.
Maekawa, Y., Ishikawa, K., Yasuda, O., Oguro, R., Hanasaki, H., Kida, I., et al. (2009). Klotho suppresses TNF-alpha-induced expression of adhesion molecules in the endothelium and attenuates NF-kappaB activation. Endocrine 35, 341–346. doi: 10.1007/s12020-009-9181-3.
Maekawa, Y., Ohishi, M., Ikushima, M., Yamamoto, K., Yasuda, O., Oguro, R., et al. (2011). Klotho protein diminishes endothelial apoptosis and senescence via a mitogen-activated kinase pathway. Geriatr. Gerontol. Int. 11, 510–516. doi: 10.1111/j.1447-0594.2011.00699.x.
Mansinho, A., Ferreira, A. R., Casimiro, S., Alho, I., Vendrell, I., Costa, A. L., et al. (2019). Levels of circulating fibroblast growth factor 23 (FGF23) and prognosis in cancer patients with bone metastases. Int. J. Mol. Sci. 20:695. doi: 10.3390/ijms20030695.
Masuda, Y., Ohta, H., Morita, Y., Nakayama, Y., Miyake, A., Itoh, N., et al. (2015). Expression of Fgf23 in activated dendritic cells and macrophages in response to immunological stimuli in mice. Biol. Pharm. Bull. 38, 687–693. doi: 10.1248/bpb.b14-00276.
Matsumura, Y., Aizawa, H., Shiraki-Iida, T., Nagai, R., Kuro-O, M., and Nabeshima, Y. (1998). Identification of the human klotho gene and its two transcripts encoding membrane and secreted klotho protein. Biochem. Biophys. Res. Commun. 242, 626–630. doi: 10.1006/bbrc.1997.8019.
Memmos, E., Sarafidis, P., Pateinakis, P., Tsiantoulas, A., Faitatzidou, D., Giamalis, P., et al. (2019). Soluble Klotho is associated with mortality and cardiovascular events in hemodialysis. BMC Nephrol. 20:217. doi: 10.1186/s12882-019-1391-1.
Meng, Q. H., Xu, E., Hildebrandt, M. A. T., Liang, D., Lu, K., Ye, Y., et al. (2014). Genetic variants in the fibroblast growth factor pathway as potential markers of ovarian cancer risk, therapeutic response, and clinical outcome. Clin. Chem. 60, 222–232. doi: 10.1373/clinchem.2013.211490.
Mihai, S., Codrici, E., Popescu, I. D., Enciu, A. -M., Albulescu, L., Necula, L. G., et al. (2018). Inflammation-related mechanisms in chronic kidney disease prediction, progression, and outcome. J. Immunol. Res. 2018:2180373. doi: 10.1155/2018/2180373.
Minisola, S., Peacock, M., Fukumoto, S., Cipriani, C., Pepe, J., Tella, S. H., et al. (2017). Tumour-induced osteomalacia. Nat. Rev. Dis. Prim. 3:17044. doi: 10.1038/nrdp.2017.44.
Mirza, M. A. I., Alsiö, J., Hammarstedt, A., Erben, R. G., Michaëlsson, K., Tivesten, A., et al. (2011). Circulating fibroblast growth factor-23 is associated with fat mass and dyslipidemia in two independent cohorts of elderly individuals. Arteriosc. Thromb. Vascu. Biol. 31, 219–227. doi: 10.1161/ATVBAHA.110.214619.
Nakahama, H., Nakanishi, T., Uno, H., Takaoka, T., Taji, N., Uyama, O., et al. (1995). Prostate cancer-induced oncogenic hypophosphatemic osteomalacia. Urol. Int. 55, 38–40. doi: 10.1159/000282746.
Nakatani, T., Sarraj, B., Ohnishi, M., Densmore, M. J., Taguchi, T., Goetz, R., et al. (2009). In vivo genetic evidence for klotho-dependent, fibroblast growth factor 23 (Fgf23) -mediated regulation of systemic phosphate homeostasis. FASEB J. 23, 433–441. doi: 10.1096/fj.08-114397.
Navid, S., Fan, C. O., Flores-Villanueva, P., Generali, D., and Li, Y. (2020). The fibroblast growth factor receptors in breast cancer: from oncogenesis to better treatments. Int. J. Mol. Sci. 21:2011. doi: 10.3390/ijms21062011.
Ogawa, Y., Kurosu, H., Yamamoto, M., Nandi, A., Rosenblatt, K. P., Goetz, R., et al. (2007). BetaKlotho is required for metabolic activity of fibroblast growth factor 21. Proc. Natl. Acad. Sci. U.S.A. 104, 7432–7437. doi: 10.1073/pnas.0701600104.
Ohnishi, M., Nakatani, T., Lanske, B., and Razzaque, M. S. (2009). Reversal of mineral ion homeostasis and soft-tissue calcification of klotho knockout mice by deletion of vitamin D 1alpha-hydroxylase. Kidney Int. 75, 1166–1172. doi: 10.1038/ki.2009.24.
Pako, J., Bikov, A., Barta, I., Matsueda, H., Puskas, R., Galffy, G., et al. (2020). Assessment of the circulating klotho protein in lung cancer patients. Pathol. Oncol. Res. 26, 233–238. doi: 10.1007/s12253-018-0441-5.
Pan, J., Zhong, J., Gan, L. H., Chen, S. J., Jin, H. C., Wang, X., et al. (2011). Klotho, an anti-senescence related gene, is frequently inactivated through promoter hypermethylation in colorectal cancer. Tumour Biol. 32, 729–735. doi: 10.1007/s13277-011-0174-5.
Papaloucas, C. D., Papaloucas, M. D., Kouloulias, V., Neanidis, K., Pistevou-Gompaki, K., Kouvaris, J., et al. (2014). Measurement of blood phosphorus: a quick and inexpensive method for detection of the existence of cancer in the body. Too good to be true, or forgotten knowledge of the past? Med. Hypoth. 82, 24–25. doi: 10.1016/j.mehy.2013.10.028.
Park, S., Lee, S., Kim, Y., Lee, Y., Kang, M. W., Han, K., et al. (2019). Risk of cancer in pre-dialysis chronic kidney disease: a nationwide population-based study with a matched control group. Kidney Res. Clin. Pract. 38, 60–70. doi: 10.23876/j.krcp.18.0131.
Pawlikowski, M., Pisarek, H., Borkowska, M., and Winczyk, K. (2019). Expression of α-Klotho protein in human thyroid cancers - an immunohistochemical study. Endokrynologia Polska 70, 237–240. doi: 10.5603/EP.a2019.0004.
Perveez, M., Ajaz, M., and Afroze, D. (2015). Promoter hypermethylation of KLOTHO; an anti-senescence related gene in colorectal cancer patients of Kashmir valley. Mol. Biol. Res. Commun. 4, 217–224.
Peshes-Yeloz, N., Ungar, L., Wohl, A., Jacoby, E., Fisher, T., Leitner, M., et al. (2019). Role of klotho protein in tumor genesis, cancer progression, and prognosis in patients with high-grade glioma. World Neurosurg. 130, e324–e332. doi: 10.1016/j.wneu.2019.06.082.
Petrova, V., Annicchiarico-Petruzzelli, M., Melino, G., and Amelio, I. (2018). The hypoxic tumour microenvironment. Oncogenesis 7:10. doi: 10.1038/s41389-017-0011-9.
Qian, Y., Guo, X., Che, L., Guan, X., Wu, B., Lu, R., et al. (2018). Klotho reduces necroptosis by targeting oxidative stress involved in renal ischemic-reperfusion injury. Cell. Physiol. Biochem. 45, 2268–2282. doi: 10.1159/000488172.
Quarles, L. D. (2019). Fibroblast growth factor 23 and α-Klotho co-dependent and independent functions. Curr. Opin. Nephrol. Hyperten. 28, 16–25. doi: 10.1097/MNH.0000000000000467.
Quintela-Fandino, M., Apala, J. V., Malon, D., Mouron, S., Hornedo, J., Gonzalez-Cortijo, L., et al. (2019). Nintedanib plus letrozole in early breast cancer: a phase 0/I pharmacodynamic, pharmacokinetic, and safety clinical trial of combined FGFR1 and aromatase inhibition. Breast Cancer Res. 21:69. doi: 10.1186/s13058-019-1152-x.
Razzaque, M. S. (2009b). The FGF23-Klotho axis: endocrine regulation of phosphate homeostasis. Nat. Rev. Endocrinol. 5, 611–619. doi: 10.1038/nrendo.2009.196.
Razzaque, M. S. (2009a). FGF23-mediated regulation of systemic phosphate homeostasis: is Klotho an essential player? Am. J. Physiol. Renal Physiol. 296, F470-6. doi: 10.1152/ajprenal.90538.2008.
Razzaque, M. S. (2012). The role of Klotho in energy metabolism. Nat. Rev. Endocrinol. 8, 579–587. doi: 10.1038/nrendo.2012.75.
Riminucci, M., Collins, M. T., Fedarko, N. S., Cherman, N., Corsi, A., White, K. E., et al. (2003). FGF-23 in fibrous dysplasia of bone and its relationship to renal phosphate wasting. J. Clin. Invest. 112, 683–692. doi: 10.1172/JCI18399.
Rodríguez-Ortiz, M. E., Alcalá-Díaz, J. F., Canalejo, A., Torres-Peña, J. D., Gómez-Delgado, F., Muñoz-Castañeda, J. R., et al. (2020). Fibroblast growth factor 23 predicts carotid atherosclerosis in individuals without kidney disease. The CORDIOPREV study Eur. J. Int. Med. 74, 79–85. doi: 10.1016/j.ejim.2019.12.008.
Rossaint, J., Oehmichen, J., van Aken, H., Reuter, S., Pavenstädt, H. J., Meersch, M., et al. (2016). FGF23 signaling impairs neutrophil recruitment and host defense during CKD. J. Clin. Invest. 126, 962–974. doi: 10.1172/JCI83470
Roy, C., Lejeune, S., Slimani, A., Meester, C., de Ahn As, S. A., Rousseau, M. F., et al. (2020). Fibroblast growth factor 23: a biomarker of fibrosis and prognosis in heart failure with preserved ejection fraction. ESC Heart Fail. 7, 2494–2507. doi: 10.1002/ehf2.12816
Rubinek, T., Shulman, M., Israeli, S., Bose, S., Avraham, A., Zundelevich, A., et al. (2012). Epigenetic silencing of the tumor suppressor klotho in human breast cancer. Breast Cancer Res. Treat. 133, 649–657. doi: 10.1007/s10549-011-1824-4.
Sachdeva, A., Gouge, J., Kontovounisios, C., Nikolaou, S., Ashworth, A., Lim, K., et al. (2020). Klotho and the Treatment of Human Malignancies. Cancers 12:1665. doi: 10.3390/cancers12061665
Sanchez-Lopez, E., Flashner-Abramson, E., Shalapour, S., Zhong, Z., Taniguchi, K., Levitzki, A., et al. (2016). Targeting colorectal cancer via its microenvironment by inhibiting IGF-1 receptor-insulin receptor substrate and STAT3 signaling. Oncogene 35, 2634–2644. doi: 10.1038/onc.2015.326
Sauder, A., Wiernek, S., Dai, X., Pereira, R., Yudd, M., Patel, C., et al. (2016). FGF23-Associated Tumor-Induced Osteomalacia in a patient with small cell carcinoma: a case report and regulatory mechanism study. Int. J. Surg. Pathol. 24, 116–120. doi: 10.1177/1066896915617828.
Savva, C., Adhikaree, J., Madhusudan, S., and Chokkalingam, K. (2019). Oncogenic osteomalacia and metastatic breast cancer: a case report and review of the literature. J. Diabetes Metab. Disord. 18, 267–272. doi: 10.1007/s40200-019-00398-y.
Schnedl, C., Fahrleitner-Pammer, A., Pietschmann, P., and Amrein, K. (2015). FGF23 in acute and chronic Illness. Disease Mark. 2015:358086. doi: 10.1155/2015/358086
Seo, M., Kim, M. S., Jang, A., Chung, H. J., Noh, Y., Kim, D. -H., et al. (2017). Epigenetic suppression of the anti-aging gene KLOTHO in human prostate cancer cell lines. Anim. Cells Syst. 21, 223–232. doi: 10.1080/19768354.2017.1336112.
Sever, R., and Brugge, J. S. (2015). Signal transduction in cancer. Cold Spring Harbor Perspect. Med. 5:a006098. doi: 10.1101/cshperspect.a006098
Shimada, T., Mizutani, S., Muto, T., Yoneya, T., Hino, R., Takeda, S., et al. (2001). Cloning and characterization of FGF23 as a causative factor of tumor-induced osteomalacia. Proc. Natl. Acad. Sci. U.S.A. 98, 6500–6505. doi: 10.1073/pnas.101545198
Shiraki-Iida, T., Aizawa, H., Matsumura, Y., Sekine, S., Iida, A., Anazawa, H., et al. (1998). Structure of the mouse klotho gene and its two transcripts encoding membrane and secreted protein 1. FEBS Lett. 424, 6–10. doi: 10.1016/S0014-5793(98)00127-6
Shmulevich, R., Nissim, T. B.-K., Wolf, I., Merenbakh-Lamin, K., Fishman, D., Sekler, I., et al. (2020). Klotho rewires cellular metabolism of breast cancer cells through alteration of calcium shuttling and mitochondrial activity. Oncogene 39, 4636–4649. doi: 10.1038/s41388-020-1313-5
Shu, G., Xie, B., Ren, F., Liu, D. -C., Zhou, J., Li, Q., et al. (2013). Restoration of klotho expression induces apoptosis and autophagy in hepatocellular carcinoma cells. Cell. Oncol. 36, 121–129. doi: 10.1007/s13402-012-0118-0
Singh, S., Grabner, A., Yanucil, C., Schramm, K., Czaya, B., Krick, S., et al. (2016). Fibroblast growth factor 23 directly targets hepatocytes to promote inflammation in chronic kidney disease. Kidney Int. 90, 985–996. doi: 10.1016/j.kint.2016.05.019
Sinha, S., Shukla, S., Khan, S., Tollefsbol, T. O., and Meeran, S. M. (2015). Epigenetic reactivation of p21CIP1/WAF1 and KLOTHO by a combination of bioactive dietary supplements is partially ERα-dependent in ERα-negative human breast cancer cells. Mol. Cell. Endocrinol. 406, 102–114. doi: 10.1016/j.mce.2015.02.020
Son, H. J., Choi, E. J., Yoo, N. J., and Lee, S. H. (2020). Inactivating mutations of tumor suppressor genes KLOTHO and DTWD1 in colorectal cancers. Pathol. Res. Pract. 216:152816. doi: 10.1016/j.prp.2020.152816
Sopjani, M., Rinnerthaler, M., Kruja, J., and Dermaku-Sopjani, M. (2015). Intracellular signaling of the aging suppressor protein Klotho. Curr. Mol. Med. 15, 27–37. doi: 10.2174/1566524015666150114111258
Stöhr, R., Brandenburg, V. M., Heine, G. H., Maeder, M. T., Leibundgut, G., Schuh, A., et al. (2020). Limited role for fibroblast growth factor 23 in assessing prognosis in heart failure patients: data from the TIME-CHF trial. Eur. J. Heart Fail. 22, 701–709. doi: 10.1002/ejhf.1749
Sun, H., Gao, Y., Lu, K., Zhao, G., Li, X., Li, Z., et al. (2015). Overexpression of Klotho suppresses liver cancer progression and induces cell apoptosis by negatively regulating wnt/β-catenin signaling pathway. World J. Surg. Oncol. 13, 307. doi: 10.1186/s12957-015-0717-0
Suvannasankha, A., Tompkins, D. R., Edwards, D. F., Petyaykina, K. V., Crean, C. D., Fournier, P. G., et al. (2015). FGF23 is elevated in multiple myeloma and increases heparanase expression by tumor cells. Oncotarget 6, 19647–19660. doi: 10.18632/oncotarget.3794
Suzuki, M., Uehara, Y., Motomura-Matsuzaka, K., Oki, J., Koyama, Y., Kimura, M., et al. (2008). betaKlotho is required for fibroblast growth factor (FGF) 21 signaling through FGF receptor (FGFR) 1c and FGFR3c. Mol. Endocrinol. 22, 1006–1014. doi: 10.1210/me.2007-2313
Tagliabracci, V. S., Engel, J. L., Wiley, S. E., Xiao, J., Gonzalez, D. J., Nidumanda Appaiah, H., et al. (2014). Dynamic regulation of FGF23 by Fam20C phosphorylation, GalNAc-T3 glycosylation, and furin proteolysis. Proc. Natl. Acad. Sci. U.S.A. 111, 5520–5525. doi: 10.1073/pnas.1402218111
Tang, X., Fan, Z., Wang, Y., Ji, G., Wang, M., Lin, J., et al. (2016a). Expression of klotho and β-catenin in esophageal squamous cell carcinoma, and their clinicopathological and prognostic significance. Dis. Esophagus 29, 207–214. doi: 10.1111/dote.12289
Tang, X., Wang, Y., Fan, Z., Ji, G., Wang, M., Lin, J., et al. (2016b). Klotho: a tumor suppressor and modulator of the Wnt/β-catenin pathway in human hepatocellular carcinoma. Lab. Invest. 96, 197–205. doi: 10.1038/labinvest.2015.86.
Tebben, P. J., Kalli, K. R., Cliby, W. A., Hartmann, L. C., Grande, J. P., Singh, R. J., et al. (2005). Elevated fibroblast growth factor 23 in women with malignant ovarian tumors. Mayo Clinic proceedings 80, 745–751. doi: 10.1016/S0025-6196(11)61528-0
Trošt, N., Peña-Llopis, S., Koirala, S., Stojan, J., Potts, P. R., Fon Tacer, K., et al. (2016). γKlotho is a novel marker and cell survival factor in a subset of triple negative breast cancers. Oncotarget 7, 2611–2628. doi: 10.18632/oncotarget.6006
Tsujikawa, H., Kurotaki, Y., Fujimori, T., Fukuda, K., and Nabeshima, Y. I. (2003). Klotho, a gene related to a syndrome resembling human premature aging, functions in a negative regulatory circuit of vitamin D endocrine system. Mol. Endocrinol. 17, 2393–2403. doi: 10.1210/me.2003-2048
Usuda, J., Ichinose, S., Ishizumi, T., Ohtani, K., Inoue, T., Saji, H., et al. (2011b). Klotho predicts good clinical outcome in patients with limited-disease small cell lung cancer who received surgery. Lung Cancer 74, 332–337. doi: 10.1016/j.lungcan.2011.03.004
Usuda, J., Ichinose, S., Ishizumi, T., Ohtani, K., Inoue, T., Saji, H., et al. (2011a). Klotho is a novel biomarker for good survival in resected large cell neuroendocrine carcinoma of the lung. Lung Cancer 72, 355–359. doi: 10.1016/j.lungcan.2010.10.008
Vanoirbeek, E., Krishnan, A., Eelen, G., Verlinden, L., Bouillon, R., Feldman, D., et al. (2011). The anti-cancer and anti-inflammatory actions of 1,25(OH)2D3. Best Pract. Res. Clin. Endocrinol. Metab. 25, 593–604. doi: 10.1016/j.beem.2011.05.001
Vlot, M. C., Bijnsdorp, I., den Heijer, M., Jonge, R., de van Moorselaar, R. J. A., and Heijboer, A. C. (2018). Plasma FGF23 is not elevated in prostate cancer. Clin. Chim. Acta 478, 129–131. doi: 10.1016/j.cca.2017.12.035
Wang, H. -P., Wang, Y. -Y., Pan, J., Cen, R., and Cai, Y. -K. (2014). Evaluation of specific fecal protein biochips for the diagnosis of colorectal cancer. World J. Gastroenterol. 20, 1332–1339. doi: 10.3748/wjg.v20.i5.1332
Wang, L., Wang, X., Wang, X., Jie, P., Lu, H., Zhang, S., et al. (2011). Klotho is silenced through promoter hypermethylation in gastric cancer. Am. J. Cancer Res. 1, 111–119.
Wang, X., Chen, B., Xu, W., Liu, S., Zhao, W., and Wu (2011). Combined effects of klotho and soluble CD40 ligand on A549 lung cancer cells. Oncol. Rep. 25, 1465–1472. doi: 10.3892/or.2011.1178
Wang, Y., Chen, L., Huang, G., He, D., He, J., Xu, W., et al. (2013). Klotho sensitizes human lung cancer cell line to cisplatin via PI3k/Akt pathway. PLoS One 8:e57391. doi: 10.1371/journal.pone.0057391
Weidner, H., Baschant, U., Lademann, F., Ledesma Colunga, M. G., Balaian, E., Hofbauer, C., et al. (2020). Increased FGF-23 levels are linked to ineffective erythropoiesis and impaired bone mineralization in myelodysplastic syndromes. JCI Insight 5:e137062. doi: 10.1172/jci.insight.137062
Wolf, I., Laitman, Y., Rubinek, T., Abramovitz, L., Novikov, I., Beeri, R., et al. (2010). Functional variant of KLOTHO: a breast cancer risk modifier among BRCA1 mutation carriers of Ashkenazi origin. Oncogene 29, 26–33. doi: 10.1038/onc.2009.301
Wolf, I., Levanon-Cohen, S., Bose, S., Ligumsky, H., Sredni, B., Kanety, H., et al. (2008). Klotho: a tumor suppressor and a modulator of the IGF-1 and FGF pathways in human breast cancer. Oncogene 27, 7094–7105. doi: 10.1038/onc.2008.292
Wong, G., Hayen, A., Chapman, J. R., Webster, A. C., Wang, J. J., Mitchell, P., et al. (2009). Association of CKD and cancer risk in older people. J. Am. Soc. Nephrol. 20, 1341–1350. doi: 10.1681/ASN.2008090998
Wong, G., Staplin, N., Emberson, J., Baigent, C., Turner, R., Chalmers, J., et al. (2016). Chronic kidney disease and the risk of cancer: an individual patient data meta-analysis of 32,057 participants from six prospective studies. BMC Cancer 16:488. doi: 10.1186/s12885-016-2532-6
Wu, A. -L., Feng, B., Chen, M. Z., Kolumam, G., Zavala-Solorio, J., Wyatt, S. K., et al. (2013). Antibody-mediated activation of FGFR1 induces FGF23 production and hypophosphatemia. PLoS One 8:e57322. doi: 10.1371/journal.pone.0057322
Wulaningsih, W., Michaelsson, K., Garmo, H., Hammar, N., Jungner, I., Walldius, G., et al. (2013). Inorganic phosphate and the risk of cancer in the Swedish AMORIS study. BMC Cancer 13:257. doi: 10.1186/1471-2407-13-257
Xie, B., Cao, K., Li, J., Chen, J., Tang, J., Chen, X., et al. (2016). Hmgb1 inhibits Klotho expression and malignant phenotype in melanoma cells by activating NF-κB. Oncotarget 7, 80765–80782. doi: 10.18632/oncotarget.12623
Xie, B., Hu, F., Li, M., Mo, L., Xu, C., Xiao, Y., et al. (2020). FLI-1 mediates tumor suppressor function via Klotho signaling in regulating CRC. Cell Biol. Int. 44, 1514–1522. doi: 10.1002/cbin.11347
Xie, B., Nie, S., Hu, G., Xiong, L., Hu, F., Li, M., et al. (2019). The involvement of NF-κB/Klotho signaling in colorectal cancer cell survival and invasion. Pathol. Oncol. Res. 25, 1553–1565. doi: 10.1007/s12253-018-0493-6
Xie, B., Zhou, J., Shu, G., Liu, D. -C., Zhou, J., Chen, J., et al. (2013a). Restoration of klotho gene expression induces apoptosis and autophagy in gastric cancer cells: tumor suppressive role of klotho in gastric cancer. Cancer Cell Int. 13:18. doi: 10.1186/1475-2867-13-8
Xie, B., Zhou, J., Yuan, L., Ren, F., Liu, D. -C., Li, Q., et al. (2013b). Epigenetic silencing of Klotho expression correlates with poor prognosis of human hepatocellular carcinoma. Hum. Pathol. 44, 795–801. doi: 10.1016/j.humpath.2012.07.023
Xu, Y., and Sun, Z. (2015). Molecular basis of Klotho: from gene to function in aging. Endocr. Rev. 36, 174–193. doi: 10.1210/er.2013-1079
Yamamoto, M., Clark, J. D., Pastor, J. V., Gurnani, P., Nandi, A., Kurosu, H., et al. (2005). Regulation of oxidative stress by the anti-aging hormone klotho. J. Biol. Chem. 280, 38029–38034. doi: 10.1074/jbc.M509039200
Yamashita, T., Yoshioka, M., and Itoh, N. (2000). Identification of a novel fibroblast growth factor, FGF-23, preferentially expressed in the ventrolateral thalamic nucleus of the brain. Biochem. Biophys. Res. Commun. 277, 494–498. doi: 10.1006/bbrc.2000.3696
Yamazaki, Y., Okazaki, R., Shibata, M., Hasegawa, Y., Satoh, K., Tajima, T., et al. (2002). Increased circulatory level of biologically active full-length FGF-23 in patients with hypophosphatemic rickets/osteomalacia. J. Clin. Endocrinol. Metab. 87, 4957–4960. doi: 10.1210/jc.2002-021105
Yan, Y., Wang, Y., Xiong, Y., Lin, X., Zhou, P., and Chen, Z. (2017). Reduced Klotho expression contributes to poor survival rates in human patients with ovarian cancer, and overexpression of Klotho inhibits the progression of ovarian cancer partly via the inhibition of systemic inflammation in nude mice. Mol. Med. Rep. 15, 1777–1785. doi: 10.3892/mmr.2017.6172
Yang, L., Wu, Y., He, H., Hu, F., Li, M., Mo, L., et al. (2020). Delivery of BR2-SOX17 fusion protein can inhibit cell survival, proliferation, and invasion in gastric cancer cells through regulating Klotho gene expression. Cell Biol. Int. 44, 2011–2020. doi: 10.1002/cbin.11407
Yang, W., Wang, X., Li, X., Wang, M., Chen, X., Wu, X., et al. (2014). The specific methylation characteristics of cancer related genes in Chinese colorectal cancer patients. Tumour Biol. 35, 8267–8279. doi: 10.1007/s13277-014-2100-0
Yoshiko, Y., Wang, H., Minamizaki, T., Ijuin, C., Yamamoto, R., Suemune, S., et al. (2007). Mineralized tissue cells are a principal source of FGF23. Bone 40, 1565–1573. doi: 10.1016/j.bone.2007.01.017
Zhang, G., Zhai, N., and Zhang, X. (2020). Alkannin represses growth of pancreatic cancer cells based on the down regulation of miR-199a. BioFactors. 46, 849–859. doi: 10.1002/biof.1613
Zhang, Q., Doucet, M., Tomlinson, R. E., Han, X., Quarles, L. D., Collins, M. T., et al. (2016). The hypoxia-inducible factor-1α activates ectopic production of fibroblast growth factor 23 in tumor-induced osteomalacia. Bone Res. 4:16011. doi: 10.1038/boneres.2016.11
Zhao, Y., Banerjee, S., Dey, N., LeJeune, W. S., Sarkar, P. S., Brobey, R., et al. (2011). Klotho depletion contributes to increased inflammation in kidney of the db/db mouse model of diabetes via RelA (Serine)536 Phosphorylation. Diabetes 60, 1907–1916. doi: 10.2337/db10-1262
Zhou, X., Fang, X., Jiang, Y., Geng, L., Li, X., Li, Y., et al. (2017a). Klotho, an anti-aging gene, acts as a tumor suppressor and inhibitor of IGF-1R signaling in diffuse large B cell lymphoma. J. Hematol. Oncol. 10:37. doi: 10.1186/s13045-017-0391-5
Zhou, X., Zhang, Y., Li, Y., Xu, Y., Zhang, L., and Wang, X. (2017b). Klotho suppresses tumor progression via inhibiting IGF-1R signaling in T-cell lymphoma. Oncol. Rep. 38, 967–974. doi: 10.3892/or.2017.5744
Zhu, Y., Cao, X., Zhang, X., Chen, Q., Wen, L., and Wang, P. (2019). DNA methylation-mediated Klotho silencing is an independent prognostic biomarker of head and neck squamous carcinoma. Cancer Manag. Res. 11, 1383–1390. doi: 10.2147/CMAR.S188415
Zhu, Y., Le Xu Zhang, J., Xu, W., Liu, Y., Yin, H., et al. (2013). Klotho suppresses tumor progression via inhibiting PI3K/Akt/GSK3β/Snail signaling in renal cell carcinoma. Cancer Sci. 104, 663–671. doi: 10.1111/cas.12134
Keywords: Ca2+, calcitriol, inflammation, malignancies, phosphate
Citation: Ewendt F, Feger M and Föller M (2021) Role of Fibroblast Growth Factor 23 (FGF23) and αKlotho in Cancer. Front. Cell Dev. Biol. 8:601006. doi: 10.3389/fcell.2020.601006
Received: 31 August 2020; Accepted: 15 October 2020;
Published: 14 January 2021.
Edited by:
Marzia Di Donato, University of Campania Luigi Vanvitelli, ItalyReviewed by:
Gabriella Castoria, Second University of Naples, ItalyMohammed S. Razzaque, Lake Erie College of Osteopathic Medicine, United States
Copyright © 2021 Ewendt, Feger and Föller. This is an open-access article distributed under the terms of the Creative Commons Attribution License (CC BY). The use, distribution or reproduction in other forums is permitted, provided the original author(s) and the copyright owner(s) are credited and that the original publication in this journal is cited, in accordance with accepted academic practice. No use, distribution or reproduction is permitted which does not comply with these terms.
*Correspondence: Michael Föller, bWljaGFlbC5mb2VsbGVyQHVuaS1ob2hlbmhlaW0uZGU=