- 1Marcus Center for Therapeutic Cell Characterization and Manufacturing, Parker H. Petit Institute for Bioengineering and Bioscience, Georgia Institute of Technology, Atlanta, GA, United States
- 2NSF Engineering Research Center (ERC) for Cell Manufacturing Technologies (CMaT), Georgia Institute of Technology, Atlanta, GA, United States
- 3The Wallace H. Coulter Department of Biomedical Engineering, Georgia Institute of Technology, Emory University, Atlanta, GA, United States
- 4Center for ImmunoEngineering, Georgia Institute of Technology, Atlanta, GA, United States
Mesenchymal stromal cells (MSCs) have been widely investigated for regenerative medicine applications, from treating various inflammatory diseases as a cell therapy to generating engineered tissue constructs. Numerous studies have evaluated the potential effects of MSCs following therapeutic administration. By responding to their surrounding microenvironment, MSCs may mediate immunomodulatory effects through various mechanisms that directly (i.e., contact-dependent) or indirectly (i.e., paracrine activity) alter the physiology of endogenous cells in various disease pathologies. More specifically, a pivotal crosstalk between MSCs and tissue-resident macrophages and monocytes (TMφ) has been elucidated using in vitro and in vivo preclinical studies. An improved understanding of this crosstalk could help elucidate potential mechanisms of action (MOAs) of therapeutically administered MSCs. TMφ, by nature of their remarkable functional plasticity and prevalence within the body, are uniquely positioned as critical modulators of the immune system – not only in maintaining homeostasis but also during pathogenesis. This has prompted further exploration into the cellular and molecular alterations to TMφ mediated by MSCs. In vitro assays and in vivo preclinical trials have identified key interactions mediated by MSCs that polarize the responses of TMφ from a pro-inflammatory (i.e., classical activation) to a more anti-inflammatory/reparative (i.e., alternative activation) phenotype and function. In this review, we describe physiological and pathological TMφ functions in response to various stimuli and discuss the evidence that suggest specific mechanisms through which MSCs may modulate TMφ phenotypes and functions, including paracrine interactions (e.g., secretome and extracellular vesicles), nanotube-mediated intercellular exchange, bioenergetics, and engulfment by macrophages. Continued efforts to elucidate this pivotal crosstalk may offer an improved understanding of the immunomodulatory capacity of MSCs and inform the development and testing of potential MOAs to support the therapeutic use of MSCs and MSC-derived products in various diseases.
Introduction
Mesenchymal stromal cells (MSCs), also referred to as Mesenchymal Stem Cells or Medicinal Signaling Cells, have garnered attention as cell therapies against various diseases, including graft versus host disease (GvHD) (Ball et al., 2013), neurological disorders (Riordan et al., 2019; Sun et al., 2020), and cardiovascular disease (Hare et al., 2009). For decades, MSCs have been investigated for their immunomodulatory, anti-inflammatory, and regenerative functions revealing several potential modalities for mediating therapeutic effects. A large body of literature has demonstrated that MSCs are highly responsive to environmental cues and elicit their effects through direct [i.e., cell–cell contact (Quaedackers et al., 2009; Galipeau and Sensebe, 2018)] and indirect (i.e., paracrine signaling (Caplan and Correa, 2011; Salgado and Gimble, 2013; Serejo et al., 2019)] interactions resulting in suppression of pathogenic cells (Ren et al., 2008; Quaedackers et al., 2009), induction of regulatory cells (Luz-Crawford et al., 2013; Lee et al., 2017), cytoprotection (Block et al., 2009), trophic support (Zhang et al., 2007), and tissue repair (Wu et al., 2007). Thus, the therapeutic capacity of exogenously administered MSCs relies on their innate ability to respond to surrounding pathophysiological cues and orchestrate cellular and molecular changes to restore local and systemic homeostasis.
Although MSCs hold promise for clinical use, our knowledge of MSCs has been gained primarily through in vitro assays and pre-clinical animal studies, leaving gaps in translation and an inability to demonstrate definitive efficacy in human clinical trials (Galipeau and Sensebe, 2018). How we characterize MSCs and test their therapeutic “potencies” ex vivo may be the reason for these disparities that are observed between preclinical and clinical trial results. Further elucidations into cellular and molecular interactions mediated by MSCs will better inform future investigations of key endogenous cellular targets and, ultimately, bridge the gaps to advance clinical use of MSCs by understanding how, when, and where to deliver therapeutic MSCs.
MSCs have been isolated from various tissues of the body including bone marrow, adipose, and umbilical cord tissue. By harnessing the plastic-adherence property of MSCs and with the addition of a tailored media formulation for sustaining their growth, the residual tissue-resident cells can be eliminated and MSCs can be obtained for investigational use (Secunda et al., 2015; Palumbo et al., 2018). Culture systems enable expansion of MSCs for acquiring the necessary cell numbers (i.e., yield) for therapeutic dosages in humans or animal studies. Regardless of the tissue source, plastic-adherent MSCs are further characterized by a specific set of criteria such as their expression profile of positive (e.g., CD73, CD90, and CD105) and negative (e.g., CD11b, CD14, CD19, CD34, CD45, CD79a, and HLA-DR) surface markers and in vitro multi-lineage differentiation capacity (i.e., induced osteogenesis, adipogenesis, and chondrogenesis) (Dominici et al., 2006). The caveat, however, is that ex vivo manipulation to isolate, expand, and interrogate MSCs may introduce transcriptional, epigenetic, metabolomic, and proteomic changes – and these characteristics of cultured MSCs likely do not parallel those of endogenous stromal cells (Caplan, 2008). Moreover, delivery of therapeutic MSCs back to an in vivo environment makes it additionally challenging to anticipate outcomes and demonstrate reproducible results. For these reasons, we will primarily focus on MSCs infused as therapy and the consequential effects on endogenous cells.
Autologous or allogeneic MSCs delivered as therapy may exert multiple effects to mitigate local and systemic pathologies. These potential therapeutic modalities are a result of the dynamic ability of MSCs to respond to various stimuli (Caplan and Correa, 2011). The caveat, however, is that these multimodal effects of MSCs make it challenging to identify specific mechanisms of action (MOA) that, if realized, can then be exploited in developed testing platforms. In fact, there is a strong need to develop and test a multivariate set of assays to evaluate mechanistic outcomes of MSCs using co-cultures with immune cells. Not only would understanding biological variation, evaluating manufacturing processes, and evaluating tissue sources be improved, these assays would help predict in vivo “therapeutic potencies” of infused MSCs, ultimately facilitating the translation and standardization necessary to advance cell manufacturing and regulatory approval for clinical use (Bianco et al., 2008; Chinnadurai et al., 2018). In vitro and in vivo preclinical studies have, thus far, provided compelling evidence for key interactions, referred to as molecular crosstalk, between MSCs and immune cells, most notably monocytes, macrophages, and T lymphocytes (Bianco et al., 2008; Chinnadurai et al., 2018; Antebi et al., 2019). These vital immune cells are at the crux of immune system functions, transmitting information in the form of molecular signals from a site of pathology to the rest of the body. Here, we take a more in-depth look into evidence that suggests an integral crosstalk between MSCs and specifically monocytes and tissue-resident macrophages (TMφ) to get us one step closer to identifying potential MOAs by MSCs.
Changing Dogma Delineate Monocyte and Macrophage Populations
First, understanding the physiological roles of TMφ during steady-state (i.e., homeostasis) and pathology is necessary to realize the alterations mediated by MSCs, and vice versa. Monocytes and macrophages, as well as dendritic cells, comprise the mononuclear phagocytic system (MPS). More in-depth physiological roles will be further described herein, but the most simplified term that captures the functions of MPS cells is “SHIP” – Sample, Heal, Inhibit, and Present (antigen) (Mills et al., 2014). Together, MPS cells are essential cells of the innate immune system that acquire information from their surroundings (e.g., by phagocytosis) and communicate the information (e.g., by antigen-presentation) to the adaptive immune system for a coordinated resolution of pathology. Thus, physiological plasticity (i.e., functional heterogeneity) is the underlying propensity of these cells to regulate tissue microenvironments (Wynn et al., 2013). With the dynamic nature of both MSCs and TMφ, determining the mechanistic effects resulting from this integral crosstalk continues to be an ongoing exploration.
The literature depicting the physiology of macrophages often pays homage to the pioneering work of Ilya Metchnikoff, a Russian zoologist turned immunologist and Nobel Prize laureate. He not only described these “large devouring cells” in the late 19th century but, more importantly, suggested the role of these cells as part of the host’s defenses, the first implications of the innate immune system, deeming him the father of cellular immunity (Hoeffel and Ginhoux, 2015). Discoveries after that showed commonalities in cellular responses and phagocytic functions between bone marrow-derived monocytes and tissue-resident macrophages that led many to believe that monocytes were the predecessors of macrophages. Although these physiological similarities are still appreciated, fate mapping and lineage tracing technologies have more recently delineated their ontogenies. Hematopoiesis in the bone marrow generates myeloid precursors that differentiate into monocytes upon emigration to the bloodstream. TMφ can develop from circulating monocytes that have infiltrated into tissues. However, most of them originate from either yolk sac-derived erythro-myeloid or fetal liver progenitors during embryogenesis, i.e., hematopoietic stem cell-independent precursors (Dey et al., 2014; Hoeffel and Ginhoux, 2015). Further delineation of monocytes and macrophages describes differentiation into subsets and transitional phenotypes, respectively, with distinct functions influenced by spatiotemporal cues.
Monocytes are generally categorized into subsets corresponding to surface markers and functional activities, some of which are shared with TMφ. Interestingly, the frequency of each monocyte subset is inversely related to their lifespan during a steady state. Classical monocytes (CD14highCD16–) make up about 85% of the circulating monocytes, and 15% consist of both intermediate (CD14highCD16+) and non-classical monocytes (CD14lowCD16high) (Patel et al., 2017; Ong et al., 2019). Classical monocytes are considered less mature and emerge from the bone marrow, where they enter the circulatory system with a propensity for phagocytosis of debris or foreign invaders, with the shortest lifespan of 1 day. The majority of these cells die or extravasate into tissues, whereas a small portion transition into intermediate monocytes. Intermediate monocytes are generated in response to an initial stimulus and function to propagate inflammatory signaling over a lifespan of a little over 4 days. Intermediate monocytes then transition to non-classical monocytes, with have an extended lifespan of approximately 7.5 days, allowing them to patrol the vasculature and potentially infiltrate affected tissues to resolve the inflammatory stimulus (Thomas et al., 2015; Patel et al., 2017). Thus, intermediate and non-classical monocytes are considered the mature “inflammatory” subsets as their frequency is elevated in the blood during inflammation or pathogenesis (Hamilton and Tak, 2009; Thomas et al., 2015). Although circulating monocytes have been observed to extravasate into tissues, especially during pathological activities, the majority provide short-term surveillance as a host’s first line of defense and are then replenished by continued hematopoiesis (van Furth and Cohn, 1968).
In contrast, tissue-resident macrophages possess dynamic phenotypes and functions, some of which exhibit tissue-specific functions. Here, TMφ self-renew and persist for months to even years in steady-state. Upon activation by inflammation or other pathological stimuli, naïve macrophages (M0) differentiate into classical or alternative activation macrophages, formerly M1 or M2 phenotypes, respectively, according to their surrounding microenvironment. Initially, the M1 or M2 phenotypes denoted a pro- or anti-inflammatory function, respectively, and this paradigm was synonymous with the polarized responses of toll-like receptor (TLR) signaling observed with T lymphocyte subset (i.e., type 1 or 2 helper T cells) (Mills et al., 2000). Although this macrophage nomenclature is still used, emergent evidence now suggests a spectrum of spatiotemporal identifiers, ultimately relinquishing these finite conventions (Nahrendorf and Swirski, 2016). The dynamic phenotypes of the classical or alternative activation macrophage subsets have been identified to capture physiological functions closely correlated to metabolic programs (Mills et al., 2000; Vasandan et al., 2016). A burgeoning research area endeavors to ascertain their spatiotemporal role, however, this review will collectively consider macrophages regardless of the nomenclatures describing subsets, phenotypes, and tissue-specific names to describe their crosstalk with MSCs (Table 1).
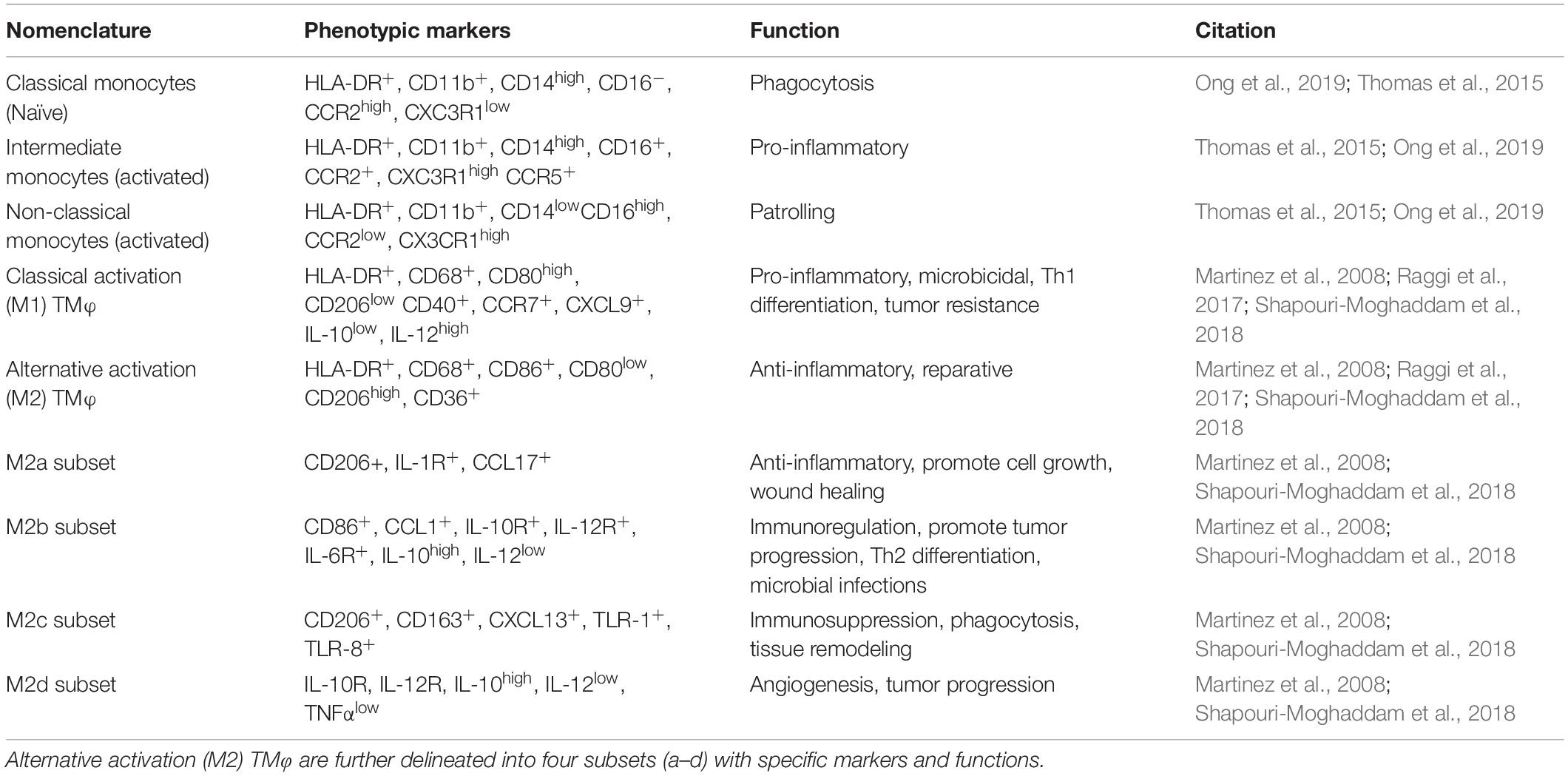
Table 1. Nomenclature used to denote monocyte and macrophage subsets with associated phenotypic markers and functions found in humans.
Functional Heterogeneity of Macrophages
TMφ are vital cells of the body that are integral to the development and homeostatic maintenance of all tissues (Figure 1). These professional phagocytes have shared roles and responses across tissues, including clearance of dead cells and debris, presenting antigen, remodeling of tissue, and metabolic regulation but also functions that serve tissue-specific demands related to each organ. Interestingly, some physiological functions enable others. For instance, one of the primary TMφ of the bone, osteoclasts, are responsible for bone resorption which is fundamental to bone remodeling during development; thus, osteoclasts sculpt the bone cavity, enabling hematopoiesis to ensue. Consequently, the generation of monocytes and subsequently immune system functions rely on these bone resident TMφ. Throughout the body, these shared roles act specifically to maintain steady state functions of resident cells and organ system functions.
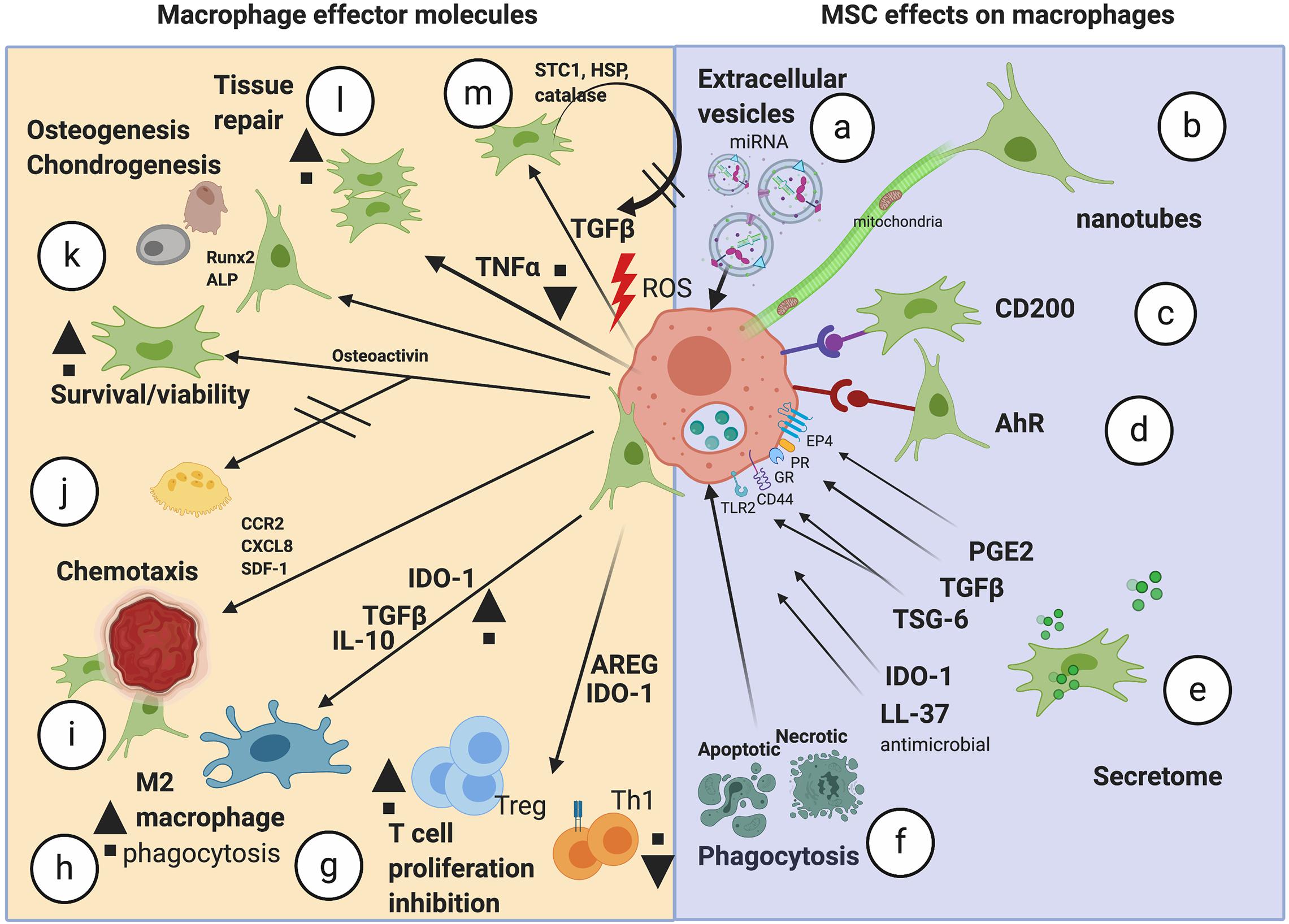
Figure 1. Schematic of the potential mechanisms of action mediated by MSCs on TMφ. The range of mechanisms important in MSC effects on macrophages (a–f) and the resulting effector molecules/effects seen in macrophages (g–m). miRNA, microRNA; AhR, aryl hydrocarbon receptor; PGE2, prostaglandin E2; TGFβ, transforming growth factor beta; TSG-6, TNF alpha-stimulated gene 6; IDO-1, indoleamine 2,3 dioxygenase; LL-37, antimicrobial peptide; AREG, amphiregulin; Tregs, regulatory T cells; IL-10, interleukin-10; CCR2, monocyte chemotactic protein −1 receptor; CXCL8, chemokine C-X-C motif chemokine ligand 8; SDF-1, stromal cell derived factor 1; Runx2, Runt-related transcription factor 2; ALP, alkaline phosphatase; LPS, lipopolysaccharide; TNFα, tumor necrosis factor alpha; ROS, reactive oxygen species; HSP, heat shock protein; STC1, stanniocalcin-1; Image created with BioRender.
During erythropoiesis, macrophages surround maturing erythroblasts, ingest extruding nuclei and essentially permit formation of erythrocytes, or red blood cells. The depletion of erythrocytes for natural turnover is also a steady state function of splenic and hepatic TMφ. The liver, pancreas, and adipose tissue are organs that maintain metabolic homeostasis. TMφ of the liver, called Kupffer cells, facilitate the metabolism of hepatocytes during caloric intake, regulating the uptake, synthesis, and oxidation of fatty acids. Similarly, TMφ support β-cell function in the pancreas, although their precise role during steady state remains to be determined since discoveries so far appear to be a consequence of pancreatic dysfunction, such as insulin resistance. Insulin and other hormone sensitivities are maintained by TMφ in adipose tissue for systemic metabolic regulation and thermogenic control of the body (Hamilton and Tak, 2009; Wynn et al., 2013).
Microglia are the main brain-resident TMφ responsible for neuronal patterning, survival, and function while other TMφ in the brain are localized to key areas for maintaining fluid balance and the integrity of the blood-brain barrier (Chen et al., 2015). Understandably, TMφ are fundamental constituents of the heart, lungs, and other organ systems, playing key roles during development and throughout adulthood; however, it is mostly through inflammatory and pathological conditions that their roles are elucidated. It is of no surprise that TMφ are involved in almost every disease and crosstalk with neighboring stromal cells serves as a vital connection to restore homeostasis of tissues.
Cellular Crosstalk With MSCs
Several of the proposed MOAs that have been implicated as the underlying therapeutic effects of administered MSCs directly or indirectly acts on TMφ (Figure 2). Initial pathogenesis or physical insult mount a “SOS” response, recruiting cells (e.g., TMφ) that are proximal and distal to the site of pathology by chemotactic and tropic factors. MSCs, too, have demonstrated an ability to home to sites of pathology once infused as therapy (Shi et al., 2007). However, the most common route of delivery for MSCs is currently by intravenous infusion, rendering MSCs lodged in the capillaries of the lungs soon after and no evidence of engraftment to date (Giri and Galipeau, 2020). This evidence has markedly shaped approaches to investigate and exploit alternative mechanisms that may be employed by MSCs to mediate therapeutic effects. We now recognize that the effects of MSCs following infusion could be largely paracrine mediated, and not by direct cell–cell contact (Caplan and Dennis, 2006; Caplan and Correa, 2011).
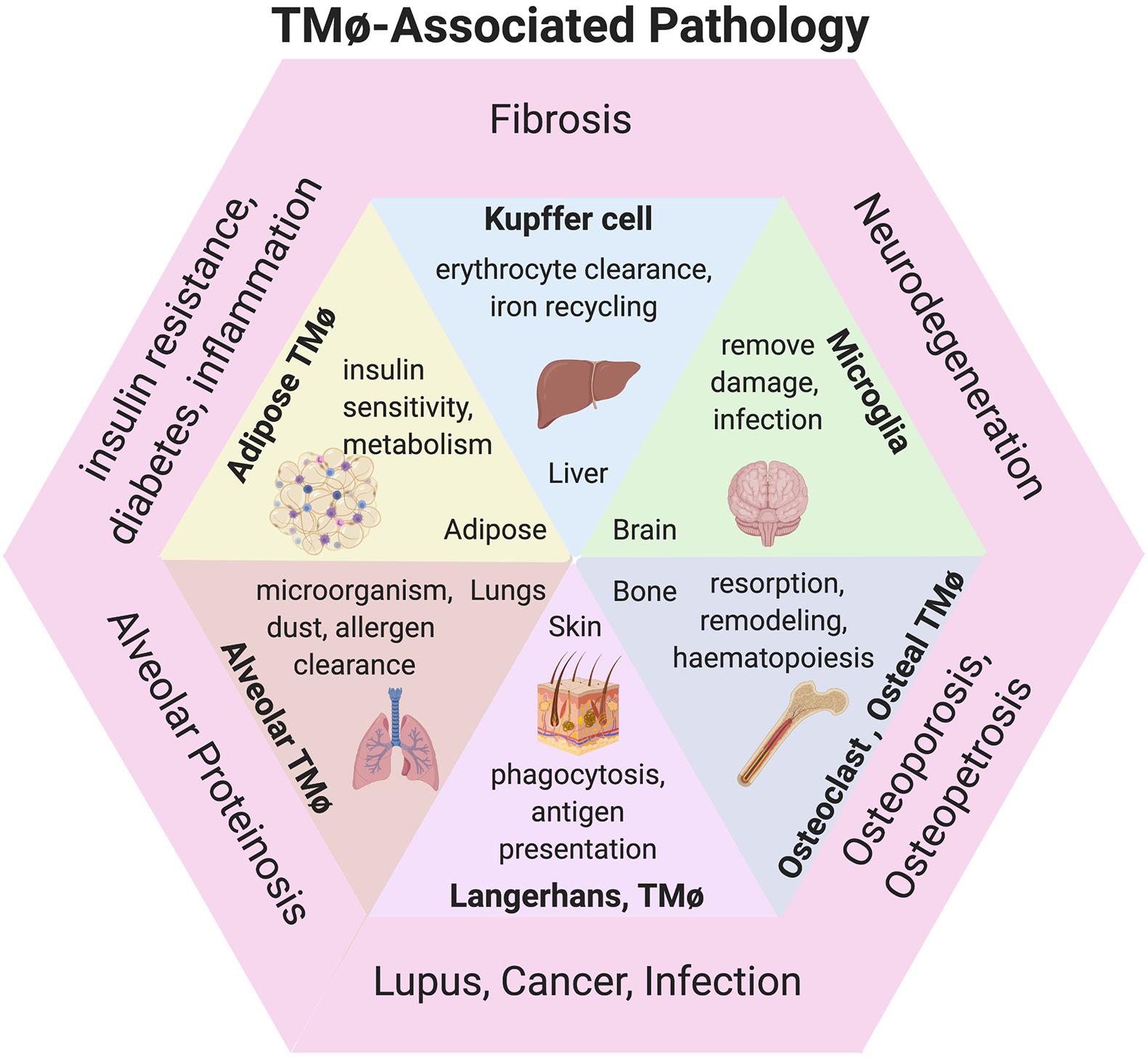
Figure 2. TMφ-associated pathologies. Diagram of TMφ functions described during physiological (inside) and pathological presentations of disease (outer) corresponding to organs. Image created with BioRender.
Soluble mediators may play more than one role in resolving tissue damage or pathology. These signals can be released directly into extracellular spaces to incite local responses or packaged in extracellular vesicles, e.g., exosomes, which travel to distal sites for systemic responses. The constituents of this cellular crosstalk described here are, in many cases, likely produced by MSCs that then directly influence the metabolic program and, in turn, the physiological function of TMφ. Alterations in macrophages are detected as skewed phenotypes as a result of metabolic reprogramming, although the duration of these temporal changes, downstream targets, and overall in vivo effects during pathology remain to be fully elucidated locally and systemically.
COX/PGE2/EP4 Axis
One of the most well-known soluble mediators that has been attributed to the therapeutic effects of MSCs is prostaglandin E2 (PGE2). PGE2 is a homeostatic factor derived from the metabolism of arachidonic acid by prostaglandin synthases and cyclooxygenases (constitutively active COX1 and inducible COX2) in both myeloid and stromal cells (Kalinski, 2012). Both human and mouse MSCs constitutively produce PGE2, and upon pro-inflammatory challenge with interferon-γ (IFNγ), tumor necrosis factor-α (TNFα) or interleukin (IL)-1β, induced elevation of PGE2 has been demonstrated (Noronha et al., 2019). PGE2 promotes the production of interleukin-10 (IL-10) from TMφ and has a synergistic effect with indoleamine 2,3-dioxygenase (IDO) to elicit MSC-induced immunosuppression on various immune cells in vitro (Spaggiari et al., 2008; Nemeth et al., 2009). An MSC-dependent PGE2 has been demonstrated to alter monocyte-to-macrophage differentiation, promoting the survival of monocytes activated by macrophage colony stimulating factor (M-CSF), by trans-activation of the M-CSF receptor (Digiacomo et al., 2015) and, more importantly, polarized to an alternative activation (M2-like) phenotype (increased CD163 and CD206 and reduced MHCII/HLA-DR expressions) of TMφ (Figure 3F). Alternative activation macrophages upregulated secretion of amphiregulin (AREG) (Ko et al., 2020), showed bolstered functions of scavenging and phagocytic activities and enhanced production of immunomodulatory cytokines IL-10 and transforming growth factor-β (TGFβ) in vitro (Chiossone et al., 2016). MSCs from multiple tissues have reproducibly polarized macrophages of various sources by this PGE2-dependent mechanism resulting in the suppression of pro-inflammatory factors, e.g., tumor necrosis factor α (TNFα), IL-12p70, and IL-17, while promoting anti-inflammatory IL-10, ultimately inhibiting perpetuation of immune responses by antigen presentation (Melief et al., 2013; Deng et al., 2016; Manferdini et al., 2017). PGE2 binding to EP4 activates adenylate cyclase and intracellular cAMP levels are elevated. This in turn activates PKA and this has been shown to phosphorylate CREB (cyclic AMP-responsive element binding). Phosphorylated CREB leads to transcription of C/EBP-β which promotes anti-inflammatory gene expression (Na et al., 2015). Of all the PGE2 receptors, only EP4 was found to facilitate the production of IL-10 and suppression of TNFα (Yasui et al., 2015). Furthermore, the secreted molecules released by the M2-like macrophages induced regulatory T cells (Tregs), a T cell subset essential for immune tolerance (Schmidt et al., 2016).
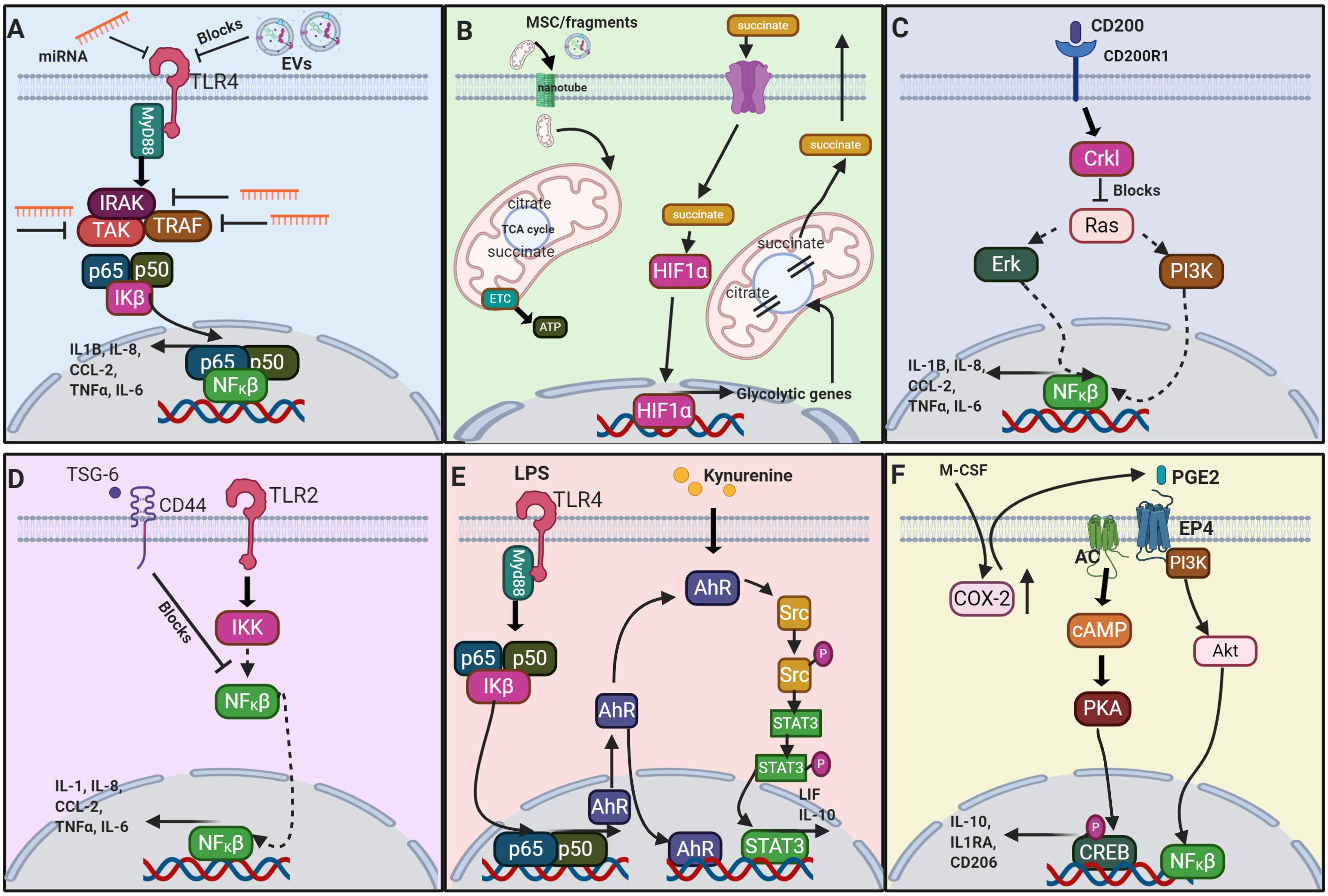
Figure 3. Schematic of a selection of the proposed signaling pathways which result from MSC or MSC derived factors interacting with macrophages. (A) The signaling of extracellular vesicles (EV) via negative regulation of the TLR4 (toll-like receptor 4) (Abdi et al., 2018); (B) metabolite signaling via the TCA cycle (Viola et al., 2019); (C) CD200-CD200R1 interaction (Manich et al., 2019); (D) TSG-6 via negative regulation of TLR2 (Choi et al., 2011); (E) AhR via TLR4 signaling (Hinden et al., 2015; Zhu et al., 2018); and (F) PGE2 (Na et al., 2015; Xu et al., 2017). miRNA, microRNA; TLR4, toll-like receptor 4; EV, extracellular vesicle; MyD88, myeloid differentiation primary response 88; IRAK, interleukin-1 receptor-associated kinase; TAK, TGF-β activated kinase; TRAF, TNF receptor-associated factor; p65, NF-κβ p65 subunit; p50, NF-κβ p50 subunit; IKβ, NFKβ inhibitor; NFKβ, nuclear factor kappa light chain enhancer of activated B cells; IL1β, interleukin-1 β; IL-8, interleukin-8; CCL-2, C-C motif chemokine ligand 2; TNFα, tumor necrosis factor α; IL-6, interleukin-6; MSC, mesenchymal stromal cell; HIF1α, hypoxia-inducible factor 1-α; TCA, tricarboxylic acid cycle; ETC, electron transport chain; ATP, adenosine triphosphate; CD200, cluster of differentiation 200; CD200R1, CD200 receptor 1; Crkl, Crk-like protein; Erk, extracellular signal regulated kinase; PI3K, phosphoinositide 3-kinase; TSG-6, TNFα-stimulated gene 6; CD44, cluster of differentiation 44; TLR2, toll-like receptor 2; IKK, inhibitor of nuclear factor NF-κβ kinase, LPS, lipopolysaccharide; AhR, aryl hydrocarbon receptor; Src, proto-oncogene c-Src; Src-P, phosphorylated Src; STAT3, signal transducer and activator of transcription 3; LIF, leukemia inhibitory factor; IL-10, interleukin-10; M-CSF, macrophage colony-stimulating factor; COX-2, cyclooxygenase 2; PGE2, prostaglandin E2; EP4, E-type prostanoid receptor 4; AC, adenylate cyclase; Akt, protein kinase B; cAMP, cyclic adenosine monophosphate; PKA, protein kinase A; CREB, cAMP response element binding protein; IL-1RA, interleukin-1 receptor antagonist; CD206 (mannose receptor). Image created with BioRender.
Tregs and AREG
MSCs skew TMφ phenotype via the secretome, with the resulting pro-regenerative macrophages demonstrating heightened release of IL10 and TGFβ (Francois et al., 2012; Mittal et al., 2016; Vasandan et al., 2016). However, MSC-conditioned macrophages are known to influence T cell activation in vitro, by inducing the differentiation of FoxP3+ Tregs from CD4+ helper T cells (Schmidt et al., 2016). Tregs are mediators of self-tolerance, essential to prevention of autoimmunity, and are immunosuppressive of inflammatory and allergic responses to infection. Tregs are recognized as significant contributors to immunomodulatory responses mediated by MSCs and in-depth descriptions of these interactions are described by Burr et al. (2013) and English (2013). MSC-primed macrophages, utilize TGFβ, IL-10 and other immunomodulatory factors that alter the differentiation of T cell subsets, dendritic cells and B cells in vitro as well as new in vitro and in vivo evidence points to epidermal growth factor receptor ligand AREG as another signaling moiety (Ko et al., 2020).
AREG has been implicated in the resolution of inflammation, regeneration of tissues, and restoration of homeostasis after injury. There are several ways that MSCs can promote the secretion of AREG from macrophages. These include the uptake of mitochondria from MSCs and the use of the COX-2/PGE2/EP4 signaling axis (Ko et al., 2020). In a mouse model of retinal inflammation, AREG suppressed immune responses by upregulating Tregs and downregulating Th1 cells. Recombinant AREG, administered alongside MSCs in macrophage depleted mice, showed some level of recovery of retinal pathology (Ko et al., 2020).
Metabolic Reprogramming of TMφ by MSCs
The metabolic profile of the macrophage could be a key determinant of phenotype and function. Steady state macrophages exhibit a metabolism that utilizes glucose and oxygen for mitochondrial oxidative phosphorylation to generate energy in the form of ATP. Stimulation toward the classical activation phenotype in vitro demonstrated that a metabolic switch to glycolysis facilitated pro-inflammatory functions in vitro (El Kasmi and Stenmark, 2015). Classical activation (M1-like) macrophages stimulated by lipopolysaccharide treatment in vitro, indicated an upregulation of 31 metabolic enzyme/transporter-related genes which confirmed increased glycolysis, the citric acid cycle intermediate succinate, and release of pro-inflammatory IL-1β. Succinate was inferred as a key metabolite that enhances pro-inflammatory signaling during inflammation (Tannahill et al., 2013). This switch can be mitigated by the anti-inflammatory cytokine IL-10 (El Kasmi and Stenmark, 2015), suggesting multiple ways that MSCs modulate macrophage metabolism and thus phenotypic function. The reliance of M1 macrophages on glycolysis and the pentose phosphate pathway (PPP) appears to be related to two interruptions in the tricarboxylic acid (TCA)/Krebs cycle, which cause accumulation and exit of itaconate, succinate and citrate from the cycle. These metabolites are released from mitochondria, which both limits coupling of TCA to the electron transport chain (ETC) and also renders them capable of regulating cell metabolism. Succinate can stabilize HIF1α (Figure 3B) and thereby activate transcription of glycolytic genes, such that glycolysis is favored. Conversely, M2 macrophages appear to have an intact TCA cycle and so ROS are kept low and metabolites are not released to the cytoplasm (Viola et al., 2019). Upon receiving mitochondria from MSCs, M1-like (classical activation) macrophages polarized to M2-like (alternative activation) which resulted in a switch from glycolysis to oxidative phosphorylation. Therefore the exposure of MSCs to macrophages, and subsequent polarization to M2, is concomitant with a lower bioenergetic state with emphasis on catabolic pathways in vitro (El Kasmi and Stenmark, 2015; Vasandan et al., 2016). These catabolic pathways, involving β-oxidation of fatty acids, enhanced activity of 5′ AMP-activated kinase and reduced mTOR phosphorylation, are thought to rescue the macrophage from low tryptophan levels but have the obvious advantage of switching to energy conservation as well as a pro-regenerative TMφ state in vitro and in vivo (Phinney et al., 2015; Vasandan et al., 2016). During pathology, neural stem cells scavenge extracellular succinate, thwarting its utility by macrophages, in order to reduce infiltration of mononuclear phagocytes in neuroinflammation (Peruzzotti-Jametti et al., 2018). MSCs releasing insulin-like growth factor-2 under hypoxic conditions reprogram maturing macrophages to OXPHOS metabolism to improve neuroinflammation in a mouse model of Multiple Sclerosis (Du et al., 2019). These studies allude to targeting the metabolic programs of macrophages to regulate or restore the homeostatic balance of the milieu as potential therapeutic approaches.
Macrophages are in large part responsible for the development of atherosclerotic plaques since their activation by IFNγ produces foam cells, which will proceed to form unstable lesions in the intima of arteries. The elevated expression of scavenger receptors and CD36 on foam cells allows for increased uptake of low density lipoprotein (LDL) (Murray and Wynn, 2011) and release of cytokines locally that influence atherosclerosis pathology in vivo (Tedgui and Mallat, 2006). Other macrophage phenotypes have been identified in the plaque and can be atheroprotective (i.e., Mhem) (Boyle et al., 2009), pro-atherogenic (i.e., Mox) (Kadl et al., 2010) or both (i.e., M4) (Erbel et al., 2015). In an atherosclerotic mouse model, the use of skin-derived or human amnion-derived MSCs decreased plaque size in the arteries in vivo (Li et al., 2015; Wei et al., 2019). MSCs are implicated in reducing the aggregation of TMφ in the arterial intima (Shoji et al., 2011), inhibiting the formation of foam cells by elevating the number and function of Tregs in vivo (Wang et al., 2015) and by decreasing TNFα release (Li et al., 2015). All of these steps require regulation of TMφ polarization and modulation of the phenotypes in the plaque (Yang et al., 2020). In this way, MSCs sense the inflammatory environment and attempt to mitigate TMφ inflammatory responses.
Oxidative Stress
MSCs counter oxidative insult by expressing antioxidant enzymes and heat shock proteins and upregulating redox-sensitive factors, such that lipid peroxidation and hydrogen peroxide (H2O2) and superoxide () radical species are decreased in vitro (Oh et al., 2014). If free radical quenching, antioxidant production, switching of TMφ bioenergetics and mitochondrial transfer is all viewed as management of oxidative stress then this constitutes a significant feature of the interaction of MSCs with TMφ at sites of inflammation. Stanniocalcin-1, secreted by MSCs, decreases reactive oxygen species (ROS) generation, including mitochondrial ROS and suppresses the activation of the nucleotide-binding domain and leucine-rich repeat pyrin 3 (NLRP3) inflammasome in vitro (Oh et al., 2014). The NLRP3 inflammasome in activated macrophages senses damage-associated molecular patterns (DAMPS) and generates IL-1β to initiate the inflammatory cascade. Its activity can be quenched by co-culture with umbilical cord blood MSCs (Shin et al., 2016). Furthermore, when these MSCs were incubated with recombinant human IL-1β, their COX-2 expression was upregulated, and this suggests the idea of a feedback loop between the IL-1β from the inflammasome and MSC immunosuppression. In this case direct COX-2/PGE2 signaling is responsible for immunosuppressive effects on the inflammasome, in the absence of NO and IDO effects (Shin et al., 2016).
Reactive oxygen species also play a role in macrophage polarization in the heart. Resident cardiac TMφ are thought to be lost with age or after myocardial infarction (MI) and replacement may be inferior, due to lack of resident cells to engage in proliferation or the pro-inflammatory activity of monocytes recruited from the bone marrow (Weinberger and Schulz, 2015). In an MI study in mice, less apoptotic cardiomyocytes were observed in the infarct zone, after MSC infusion, and although both M2 and M1 macrophage levels were decreased, M2 was proportionately increased in the heart but not bone marrow in vivo (Dayan et al., 2011). Rat and mouse models of MI, with MSC administration, observed increases in alternative activation (M2-like) TMφ at the transplant site in vivo (Ben-Mordechai et al., 2013; Ishikane et al., 2013). CD146+ MSCs performed better than MSCs alone in a model of myocardial regeneration and this was attributed to a reduction in reactive oxygen species by the expression of CD146, an integral perivascular marker (Zhang et al., 2019a). In a follow up study, the total number of macrophages in the hearts of the mice did not vary after MSC transplantation but the ratio of M2:M1 increased (Zhang et al., 2019b). Injection of TNFα alongside the MSC transplantation abrogated the reparative effects of the MSCs in vivo. Although much is still unknown about macrophage subsets in the heart, MSCs appear to drive regeneration by reprogramming TMφ phenotypes.
Message in a Bottle – MSC-Derived Extracellular Vesicles
The paracrine-mediated immunomodulatory factors secreted by MSCs are not solely attributed to the release of soluble molecules that act directly on cells in the local environment but also by uptake of those packaged in extracellular vesicles (EVs). MSC-derived EVs (MSC-EVs) are implicated as a cell-free product with, in some cases, comparable effects to infused MSCs, thus rendering MSC-EVs attractive candidates as therapy. Cultured MSCs secrete EVs that can be collected and subsequently isolated from the conditioned media (CM), enabling studies to perform comparisons using MSC-CM and MSC-EVs to determine the direct influences of MSC-EVs. Together, the ability to harness the therapeutic effects of MSCs without the need to deliver the cells make MSC-EVs attractive treatment alternatives under growing investigations in pre-clinical and clinical trials (Harrell et al., 2019a).
Apoptotic bodies (>1,000 nm in diameter) are the largest of the EVs which bud from MSCs during apoptosis. Microvesicles (MVs) are typically 100-1,000 nm in diameter and bud from the plasma membrane, and exosomes are the smallest EVs with diameters measuring 30–200 nm and result from budding of the late endosome membranes (Harrell et al., 2019b). The cargo packaged into MSC-EVs include many of the soluble cytokines and molecules discussed herein as well as other proteins, enzymes, organelles, lipids, metabolites, nucleic acids, and non-coding RNAs, all of which are comprehensively discussed in the context of inflammatory disease by Harrell et al. (2019b). The cargo that relays the “messages” to distant sites is still elusive yet is suggested to be highly specific (Baek et al., 2019).
MSC-derived exosomes are capable of inducing macrophage polarization to the alternative activation phenotype by several proposed mechanisms. MSC-derived exosomes have been attributed to macrophage polarization to the alternative activation phenotype with increased production and secretion of AREG by the described PGE2-dependent mechanism in vitro (Ko et al., 2020), suppression of the infiltration of classical activation macrophages and associated pro-inflammatory signaling (Mao et al., 2017; Willis et al., 2018a), and improved histoarchitecture by TMφ remodeling in vivo (Willis et al., 2018a) demonstrating alternative immunomodulatory delivery modalities that too can be supplied by MSCs (Figure 3A). Although the MSC-derived exosome-mediated effects are the result of the comprehensive mediators contained, depicting specific microRNA (miRNA) (Essandoh et al., 2016), cytokines, metabolites, and other molecules (Willis et al., 2018b) of which the EVs are comprised, may serve to identify other targeted therapeutics.
MSC-derived exosomes have been widely investigated in a number of pre-clinical studies of inflammatory diseases. TMφ induced to a classical activation phenotype and pro-inflammatory function that perpetuates inflammatory signaling were altered by MSC-derived exosomes to the alternative activation phenotype, resulting in attenuation of pathological severity in lung injury (Morrison et al., 2017; Wang et al., 2020), colitis (Mao et al., 2017), cardiomyopathy (Sun et al., 2018), retinal damage (Yu et al., 2016a), musculoskeletal conditions (Zhang et al., 2016; Cosenza et al., 2017), chronic wounds (Lo Sicco et al., 2017) and spinal cord injury (Lankford et al., 2018). MSC-derived exosomes not only mediated cellular improvements through TMφ, but also promoted the survival (Sun et al., 2018) and cytoprotection (Cosenza et al., 2017) of other vital tissue-specific cells in vivo. The mechanisms of action for MSC-derived exosomes remain to be fully elucidated, as multiple mediators are involved, and thus presumably more than one mechanistic effect, exerting comprehensive benefits during pathology.
Organelle Donation and Bioenergetics
Organelles such as mitochondria too can be shuttled from MSCs to TMφ to support higher demands of macrophage physiological functions. For example, stimulation of TMφ phagocytosis in acute respiratory distress syndrome (ARDS) was elicited via nanotube transfer of MSC mitochondria to macrophages in vivo (Jackson et al., 2016). In addition, MSCs under intracellular oxidative stress in vitro will shuttle damaged mitochondria (containing an excess of oxidized and nitrosylated proteins) into microvesicles for extrusion to improve their bioenergetics in vitro. Simultaneous de-sensitization via miRNA-containing exosomes from the MSCs mitigates the activation of TMφ, which permits phagocytosis and re-use of the donated mitochondria by TMφ, a mechanism that promotes the survival of MSCs by outsourcing mitophagy (Phinney et al., 2015). Macrophages co-cultured with MSCs have shown enhanced phagocytic activity and this may be orchestrated by nanotube/EV-mediated mitochondrial transfer from MSCs (Ibrahim et al., 2014; Phinney et al., 2015; Ko et al., 2020). Although more evidence is necessary to determine the advantage of using MSC-EVs over MSCs, alternative strategies to elicit the comprehensive effects from MSC-macrophage crosstalk are promising.
Contact-Dependent Communication
The complexities of the intercommunication between MSCs and TMφ may never be completely teased apart, and therefore we should expect possibilities of both indirect and direct mechanisms working in tandem to promote improvements to a pathological milieu. Moreover, the spatiotemporal microenvironment will continue to be altered to resolve inflammation and restore homeostasis, necessitating multiple functions of both the macrophages and MSCs. The dynamic heterogeneity of functions of both cell types are no coincidence; the integral crosstalk is the forefront to not only resolving pathology but improving our understanding of prophylactic measures to prevent disease. The observations of a number of in vivo investigations are presented in Table 2.
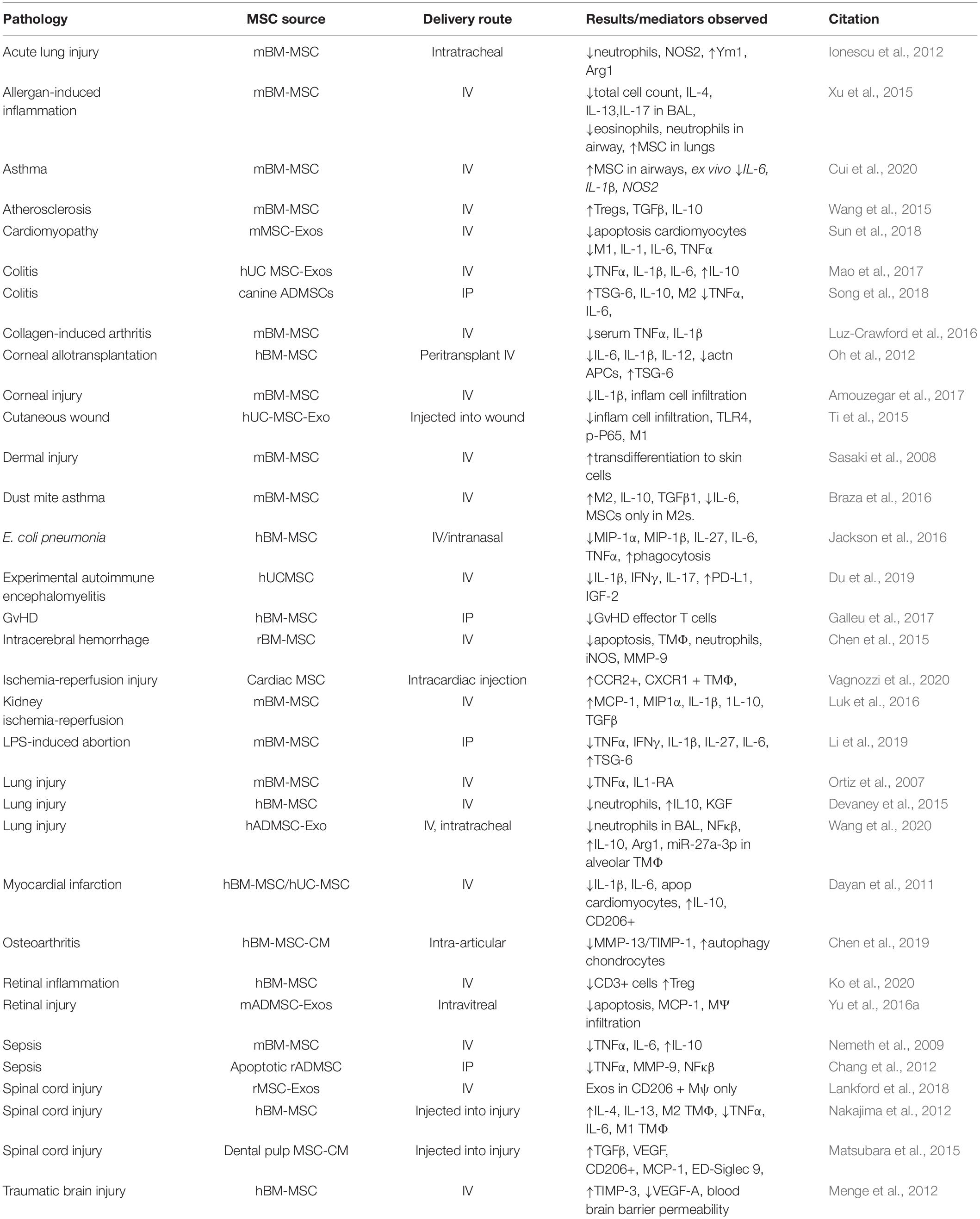
Table 2. A selection of in vivo studies using therapeutic MSCs with described pathology, source of MSCs, delivery route and outcomes.
Phagocytosis of Dead/Apoptotic Cells
Mechanisms involved in recognition of apoptotic cells and the consequential removal by phagocytosis, involve receptors classed as C-type lectin, vitronectin (with assistance from CD36 and thrombospondin on apoptotic cells), phosphatidylserine, scavenger proteins (bind apoptotic, necrotic cells, opsonized pathogens and cell debris), TLRs and macrophage antigens. Phagocytosis of apoptotic cells has been shown to inhibit macrophage production of a range of cytokines, except TGFβ1, PGE2 and platelet activating factor (PAF) in vitro (Fadok et al., 1998). In general, the result of phagocytosis appears to be immunosuppression. Recently, a novel cell contact-dependent mechanism demonstrated a lipoprotein receptor protein mediated uptake of MSC-derived cytoplasmic components, or processing bodies, by monocytes and macrophages resulted in reprogramming to an anti-inflammatory function. Reprogrammed monocytes and macrophages were able to significantly suppress activated helper T cells proliferation in vitro and mitigate inflammation in a small animal model of LPS-induced lung inflammation (Min et al., 2020). In a pre-clinical model of asthma, polarization of alveolar TMφ was accompanied by phagocytosis of PKH26 + MSC and upregulation of TGFβ and IL-10 mRNA (Braza et al., 2016) and M2 markers ex vivo were only expressed on macrophages which had ingested MSCs.
Indeed the daily clearance of fetal apoptotic stromal cells by maternal resident lung macrophages, stimulating IL-10 release and IL-1β suppression, facilitates immunotolerance in vivo (Abumaree et al., 2006; Galipeau and Sensebe, 2018). Efferocytosis, a term originally coined in 2003, is the targeted removal of apoptotic cells, as well as cells dying through the many other forms of cell death (Henson, 2017). Phagocytosing macrophages clear apoptotic MSCs, and this process precipitates intracellular signaling in macrophages to downregulate TNFα and NO production, in favor of TGFβ1 and IL-10 in vitro (Braza et al., 2016; de Witte et al., 2018). Therefore, MSCs may take part in immunoregulation and macrophage polarization even after becoming apoptotic.
These studies allude to the state of MSCs when delivered in vivo. Freshly thawed cells, in general, have a higher metabolic activity, greater percentage of apoptotic cells and a higher necrotic fraction than culture rescued cells (Antebi et al., 2019). MSCs used within 24 h of thawing, show compromised T cell suppression, increased susceptibility to lysis by complement or immune cells and shortened persistence in vivo with intravenous (IV) administration (Moll et al., 2016). It has also been shown that roughly 50% of IV transfused mouse MSCs become trapped in the lung and are ultimately phagocytosed by lung resident macrophages (Nemeth et al., 2009). In an ischemia-reperfusion injury model in mice, cardiac MSC injection improved heart function, not by production of new cardiomyocytes but by induction of CCR2+ and CX3CR1+ TMφ. Changes in the local extracellular matrix content of the peri-infarct border zone in vivo occurred whether the MSCs were live or freeze-thawed (non-viable) and could be substituted by a chemical inducer of the innate immune response (zymosan) (Vagnozzi et al., 2020). Therefore, this may be an indirect effect of the MSCs and not paracrine-mediated, but nevertheless, MSCs whether viable, intact or even apoptotic, cell signaling still produces a beneficial effect on the resulting macrophage functional output.
It is therefore possible that MSCs can be apoptotic, metabolically inactivated or fragmented even (membrane particles) and still exert immunomodulation (Luk et al., 2016; Goncalves et al., 2017). Apoptotic adipose-derived MSCs were able to improve survival of rats in a model of sepsis by decreasing TNFα levels in circulations as well as the frequencies of systemic and splenic helper and cytotoxic T cells (Chang et al., 2012). Evidence supports that apoptotic cells were more potent than viable cells in lung, kidney injury and ischemia-reperfusion models (Sung et al., 2013). Furthermore, the deliberate perforin-mediated induction of apoptosis of MSCs by cytotoxic cells in a murine model of GvHD, appeared necessary for the success of the MSC infusion (Galleu et al., 2017). Induction of caspase 8 and apoptosis was necessary for immunosuppression in vivo and patients with high cytoxicity were more likely to respond to MSCs, indicating a bifurcation of patient responses. Intraperitoneally (IP)-delivered MSCs were sequestered in the phagocytes in lymph nodes and IV-delivered MSCs homed to the lungs. These apoptotic MSCs only improved GvHD outcomes when delivered IP, and not IV, and no IDO was induced by IV-administered MSCs. Regulation of pro-inflammatory Th1 and Th17 cells by MSC-derived PGE2 only occurred in the presence of CD14+ cells in PBMC cultures, therefore indicating a reliance on myeloid cells for immunoregulatory mechanisms (Rozenberg et al., 2016).
The heat inactivation (HI) protocol of Luk et al. (2016) introduced in 2016 incubated MSCs for 30 min at 50°C (HI MSCs) and this resulted in the lack of a secretory profile, no proliferative or metabolic activity and a disintegration of the cell without heat shock protein release. HI MSCs did not inhibit T cell proliferation but were able to reduce TNFα release by monocytes challenged with LPS in vitro (Luk et al., 2016). This points to a non-specific immunosuppression, i.e., independent of cell viability, by the reticuloendothelial system of the host (Poon et al., 2014). Indeed, studies in our laboratory, using MSC suppression of TNFα release by THP-1 macrophages, have found both freshly thawed and cultured MSCs able to exert immunosuppressive effects and yet the culture-rescued cells generally have few apoptotic and necrotic cells post harvest, as mentioned earlier (Pradhan P et al. BioRxiv doi: https://doi.org/10.1101/2020.09.12.294850).
Aryl Hydrocarbon Receptor on MSCs
Aside from the secretome and engulfment of MSCs, a proposed mechanism mediated by MSCs resulting in macrophage polarization, or macrophage phenotype “plasticity,” is by the activation of the aryl hydrocarbon receptor (AhR). This MSC receptor responds to environmental stimuli and contributes to both physiological cell development and immune regulation (Abney and Galipeau, 2020). The AhR, when bound by ligands of environmental pollutants, translocates from the cytoplasm to the nucleus and facilitates AhR-related transcription of genes, which typically elicit immunotoxicological effects. For example, MSCs upregulate cytochrome P450 isoforms, cyp1a1 and cyp1b2 genes in response to cockroach allergen in vitro (Xu et al., 2015). This AhR receptor activation is kynurenine-mediated and results in immunosuppressive alterations [decreased IL-6 expression and enhanced leukemia inhibitory factor (LIF) ex vivo (Hinden et al., 2015)] (Figure 3E). This suggests an immunomodulatory potential of MSC directly regulated by AhR.
In mice treated with MSCs prior to intratracheal cockroach extract (CRE) challenge, there was a significant decrease in bronchial inflammation and goblet cell hyperplasia. Isolated lung TMφ showed a significant increase in alternative activation (M2-like) marker expressions (e.g., Arg1, FIZZ1, and Ym1) relative to CRE treatment alone, suggesting treatment with MSCs polarized macrophages to an alternative activation phenotype under allergen-induced pulmonary inflammation in vivo (Cui et al., 2020). The IDO inhibitor 1-methyl tryptophan can activate AhR in MSCs in vitro (Lewis et al., 2017) and the AhR-Src-STAT3-IL10 signaling pathway has been pivotal to controlling inflammatory macrophages in vitro (Zhu et al., 2018). The correlation between this signaling pathway and immunomodulatory mechanisms by MSCs may be a key axis for targeted approaches.
CD200, TSG-6, and Hormone Receptors
Another contact-dependent interaction implicated in macrophage polarization is the CD200/CD200R1 receptor complex. CD200 (OX-2) is a transmembrane glycoprotein and its counterpart, CD200-R1, is found on myeloid cells and T cells. Interestingly, the role of CD200 cannot be fully extricated from soluble tumor necrosis factor stimulated gene-6 (TSG-6) signaling. In an LPS-induced abortion mouse model, TSG-6-silenced or CD200-silenced MSCs exhibited a higher embryo resorption rate and both had higher levels of TNFα, IFNγ, and induced nitric oxide synthase (iNOS) in the decidua than non-silenced control MSCs supporting the CD200- (cell-mediated) and TSG-6-dependent (i.e., paracrine-mediated) mechanism (Li et al., 2019) (Figure 3C). This evidence gives credence to the idea that MSCs exert immune tolerance by both cell-contact as well as paracrine-mediated mechanisms.
The ability of bone marrow MSCs to suppress TNFα release by IFNγ-primed THP-1 macrophages appeared correlated to the levels of CD200 (Pietila et al., 2012). High expression of CD200 on umbilical cord derived MSCs was associated with improved immunomodulatory effects in vitro and lack of CD200 expression was correlated to poor suppressive capacity of the MSCs, suggesting a link between CD200 expression on MSCs and suppression of pro-inflammatory macrophage signaling (Pietila et al., 2012). TSG-6 released by MSCs is a signaling molecule that has been the focus of several pathological conditions. TSG-6 deletion in MSCs abrogated the ability to repair corneal damage, myocardial infarct and aid in corneal allograft survival (Oh et al., 2012). The role of TSG-6 signaling has been explored in inflammatory bowel disease (IBD), where canine adipose tissue-derived MSCs induced polarization of TMφ in murine IBD, resulting in more M2 TMφ released into the colon and improvements in disease activity index (Song et al., 2018). TSG-6, released by the MSCs, prevented blood brain barrier (BBB) disruption in intracerebral hemorrhage in rats and reduced the density of microglia/macrophages at the hemorrhage site (Chen et al., 2015). TSG-6 has a known interaction with the CD44 receptor on TMφ, which blocks TLR2-mediated translocation of nuclear factor kappa κβ (NFκβ) to the nucleus alleviating inflammatory signaling in vitro (Choi et al., 2011).
In pathological conditions, MSCs have been shown to block the translocation of NFκβ to the nucleus, indicative of the TSG6/TLR2/NFκβ pathway. LPS and IFNγ trigger intracellular signaling pathways, via degradation of Iκβ, which frees NFκβ to translocate to the nucleus to bind promoters of pro-inflammatory mediators (Figure 3D). LPS-induced lung injury was lessened by treatment with MSCs or MSC-CM, with alveolar macrophages showing heightened Ym1 and decreased iNOS (NOS2) compared to untreated controls ex vivo (Ionescu et al., 2012). However, the signaling pathways of activated resident macrophages are complex and difficult to study in vivo, with considerable heterogeneity of response to different stimuli. The transcriptome of activated macrophages revealed nine distinct activation programs, a spectrum of activation much more advanced than the M1/M2 classification conventions (Xue et al., 2014).
Apart from modulation of TMφ phenotypes, MSCs also play a role in blocking the differentiation of steady state myeloid progenitors under inflammatory conditions and the subsequent infiltration of inflammatory effector cells at the site of inflammation. Under homeostatic conditions, bone marrow-derived MSCs support hematopoiesis, maintaining hematopoietic stem cells (HSCs) in an undifferentiated state via trophic factor release. Under inflammatory conditions (i.e., high levels of IFNγ, IL1β, and TNFα), however, HSCs undergo myelopoiesis, resulting in their differentiation to macrophages and neutrophils. CD200 expressed on MSCs is proposed to be responsible for the suppression of inflammation and the maintenance of myeloid progenitors in an undifferentiated state in vitro and in vivo (Amouzegar et al., 2017). In a mouse model of corneal injury, systemically administered control MSCs showed a fivefold induction of myeloid progenitors in the cornea and a concomitant reduction in inflammatory cells and IL-1β, compared to mice injected with (silenced) CD200-shRNA-treated MSCs (Amouzegar et al., 2017).
Besides PGE2-EP4, TSG-6-CD44, and CD200-CD200R1, signaling between progesterone receptors (PR) and glucocorticoid receptors (GR) on microglia, the macrophages of the CNS, has been implicated in the triggering of microglial polarization in vivo (Xu et al., 2017). Progesterone has been shown to be neuroprotective in pre-clinical models of traumatic brain injury, by inhibition of microglial activation and prevention of inflammatory cytokine release (Lopez-Rodriguez et al., 2015). Inhibition of PR and GR by mifepristone partly blocked human placental MSC-driven polarization of macrophages. In this study, the basal release of soluble factors by MSCs suggested TGFβ as a key mediator of the resulting immunomodulation (Abumaree et al., 2013).
MSC-macrophage interactions in bone (re)modeling appear to be paracrine- and contact-mediated via CD200/CD200R in vitro (Varin et al., 2013). Osteoclasts (bone resorbing cells) can differentiate from hematopoietic precursor cells or other macrophage lineage cells. Activated TMφ, which release pro-inflammatory cytokines, can disrupt the balance of osteoclast-mediated bone resorption and osteoblast-mediated bone formation, resulting in bone loss (Yang and Yang, 2019). However, depletion of TMφ during intramembranous bone deposition in fracture repair led to impaired healing (Alexander et al., 2011). Soluble CD200 can inhibit differentiation of osteoclast precursors and inhibit receptor activator of nuclear factor kappa-β ligand (RANKL) signaling. MSCs expressing CD200 can block osteoclast formation and resorption pit activity in vitro (Varin et al., 2013) and CD200R inhibition can result in hyperactivation of macrophages and increased susceptibility to autoimmune diseases (Wright et al., 2003). Future research should unravel the reliance on contact dependent vs. soluble mediators for bone regulation and pathology.
MSCs Altered by Macrophages
Macrophage conditioned media, as well as co-culture with MSCs, can influence MSCs viability and secretome (Freytes et al., 2013). M2 macrophages are reported to produce more osteoactivin/gpnmb and thereby activate the ERK/JNK signaling pathway to assist MSC survival, proliferation and migration (Yu et al., 2016b; Xu et al., 2017). LPS-induced TNFα release by macrophages can stimulate MSCs to secrete growth factors that promote tissue repair (Crisostomo et al., 2008) and drive MSCs to release inflammatory cytokines (Abumaree et al., 2013).
It is notable that M1 macrophage-MSC co-cultures demonstrated markedly higher upregulated genes compared to than equivalent M2 macrophage-MSC co-cultures (Espagnolle et al., 2017), verifying that the macrophage program can specify gene expression and cell-mediated immune responses. Upregulated genes in M1-MSCs cultures included IDO, COX2 (immunosuppressive genes), PDL-1, CD54 (MSC and T lymphocytes), CXCL9 and CXCL10 (involved in T cell trafficking). M1-primed MSCs showed stronger inhibition of T cell proliferation, likely through a homotypic CD54 synapse between M1 macrophage and MSC (Espagnolle et al., 2017).
Other evidence of the effects of macrophage-primed MSCs can be found in orthopedic research, and relate to the multi-tissue compartment of the joint. M2 macrophages, co-cultured with MSCs, drive the expression of alkaline phosphatase, osteogenic markers and bone mineralization to regenerate bone (Champagne et al., 2002) and the expression of chondrogenic and clonogenic genes, to aid cartilage formation (Sesia et al., 2015). Similarly, exosomes isolated from LPS-treated monocytes increased gene expression of Runx2 and BMP-2 in human MSCs upon exposure in vitro (Ekstrom et al., 2013). Synovial M1 macrophages promote upregulation of proteolytic enzymes in osteoarthritis and negatively impact MSC chondrogenic effects on chondroprogenitors (Fahy et al., 2014). These key investigations shed light on the potential MOAs of MSCs in musculoskeletal indications, however, the dark side of the molecular crosstalk between MSCs and TMφ reveals how these potential MOAs can be exploited during carcinogenesis.
Although this review is intended to focus on MSCs administered as therapy, several studies have also reported a central crosstalk between MSCs and TMφ in the context of cancer. Given that MSCs are defined as a cultured cell type and their in vivo identity prior to isolation still remains unclear [i.e., pericyte-like (Caplan, 2008)], explorations to reveal the influences of MSCs within the tumor microenvironment are limited. Often, culture expanded MSCs are co-cultured with cancer cells and TAMs isolated from tumors or injected directly into the in vivo tumor microenvironment to investigate the influences of MSCs. Furthermore, it is challenging to elucidate the phenotypes, functions, and crosstalk attributed to the various cells within the in vivo tumor microenvironment that may, collectively, promote or mitigate cancer progression.
Like TMφ, the paradigm of polarized responses resulting from TLR signaling has also been described for MSCs (i.e., MSC1 and MSC2), suggesting pro- or anti-inflammatory effects (Tomchuck et al., 2008; Waterman et al., 2010). Waterman et al. (2012) suggested that MSC1 attenuated tumor growth in vitro and in vivo, whereas MSC2 had the opposite effect of promoting tumor growth and metastasis, linking the secretory profiles of MSCs directly to alterations to cancer cells. The changing tumor microenvironment likely alters, “educates,” or even “hijacks” (Quail and Joyce, 2013) MSCs as well as tumor-associated macrophages (TAMs). For example, key findings have linked inflammation and cancer progression by elucidating the roles of polarized TAMs and their activation of MSCs. Anti-tumor effects have been attributed to M1-like TAMs and, in contrast, multiple aspects of tumor progression are correlated with the suppressive program of M2-like TAMs. Inflammation in the tumor microenvironment produced an M1 phenotype of TAMS which, in turn, induced an immunosuppressive profile of MSCs, expressing high levels of iNOS and MCP1. Further recruitment of TAMs mediated by MCP1 secreted from MSCs along with IL-6 led to polarization into an M2-like phenotype which promoted tumor growth (Jia et al., 2016). Our contextual understanding regarding the crosstalk between TAMs and endogenous cells during carcinogenesis is far from being fully understood. A greater appreciation of the crosstalk between MSCs and TAMs as well as the development of cancer stem cells in cancer research can be found in more focused reviews by Papaccio et al. (2017) and Ridge et al. (2017).
Conclusion
Scientific understanding is continually enriched and reshaped by technological advancements, research methodologies and new discoveries. As our understanding of monocytes and macrophages has evolved recently, so has our viewpoint about MSCs. There is now broad agreement that MSCs are not in fact stem cells and likely do not exhibit multipotency when delivered in vivo; rather, they are potent signaling cells with great plasticity, that interact dynamically with their microenvironment, e.g., with TMφ, to modulate and control immune homeostasis and produce, or help produce, various pro-regenerative signals.
As with current assays that evaluate in vitro functional or therapeutic potencies of MSCs, a developed assay based on an identified MOA needs to account for many considerations that may obscure the reproducibility of results – highlighting the importance of standardization of all processes from harvest to delivery of MSCs. One must consider the biological variation of each donor and alterations imparted by different manufacturing processes including, how the cells were isolated, stored, shipped, cultured, expanded, and delivered (e.g., route, timing, and dose). A major realization is that MSCs are mainly administered by intravenous infusion, destined for entrapment in the lungs – a tissue that may be far from the site of pathology. Thus, the applicability of a given MOA with respect to the route of delivery and site of pathology for treatment must be considered, although the predominant therapeutic effects of MSCs could be via paracrine activities (Giri and Galipeau, 2020). Furthermore, we refer to therapeutic MSCs as a culture-based cell type confined by an identity characterized ex vivo that are then re-introduced to an in vivo milieu that is highly variable from patient to patient. This alludes to the difficulty in developing in vitro assays that are predictive of in vivo outcomes. The complexity of identifying and validating potential MOAs mediated by therapeutic MSCs bolster the need for deep and broad characterization of the cells especially using multi-omic analyses, better understanding of the critical process parameters that can help produce cells with consistent and reproducible quality, identification of the critical quality attributes that are predictive of the product quality and patient outcomes, standardization of processes and analytical methods, pertinent in vitro potency and safety assays, appropriate animal models for in vivo pre-clinical validation, and well-designed randomized controlled trials to evaluate clinical efficacy.
The evidences from pre-clinical studies, to date, suggest that MSC-macrophage crosstalk may play a critical role in their in vivo function and can be a potential MOA. These interactions are largely a result of the MSC secretome, including soluble factors, mitochondrial donation, mediating complex pathological milieus characterized by pro-inflammatory, metabolic, proliferative, differentiative, hypoxic, REDOX mediators, along with some cell contact-dependent mechanisms. Together, these studies shed light on the various modes in which MSCs alter macrophage phenotype and, in so doing, can modulate local and systemic immunopathology to promote repair and restore homeostasis.
As mentioned earlier, the majority of mechanisms by which macrophages and MSCs interact have been discovered in vitro and much more in vivo studies are needed to tie in these ex vivo observations to those occurring in the body upon administration. Through correlation of in vitro functional assays to qualitative and quantitative in vivo effects, we should be able to identify potency assays which are more representative of in vivo performance and employ these to inform the manufacturing of MSCs for mainstream clinical therapy. The potential for MSC therapeutics lies in the ability to improve our understanding of how we can best harness their key communication mechanisms with other cells, and reproducibly promote the beneficial effects, ultimately translating benchtop discoveries to bedside MOAs to advance these promising therapies into clinic and the industry.
Author Contributions
HS and AB contributed to the preparation, writing, and review of the manuscript. CY and KR contributed to the writing and review of the manuscript. All authors approved the submitted version.
Funding
This work was supported by The Marcus Foundation, Georgia Research Alliance, and the National Science Foundation Engineering Research Center for Cell Manufacturing Technologies (NSF EEC 1648035).
Conflict of Interest
The authors declare that the research was conducted in the absence of any commercial or financial relationships that could be construed as a potential conflict of interest.
Acknowledgments
The authors would like to thank supporters of The Marcus Center for Therapeutic Cell Characterization and Manufacturing and funding entities for allowing us to extend their reach in advancing cell therapies.
References
Abdi, J., Rashedi, I., and Keating, A. (2018). Concise review: TLR pathway-miRNA interplay in mesenchymal stromal cells: regulatory roles and therapeutic directions. Stem Cells 36, 1655–1662. doi: 10.1002/stem.2902
Abney, K. K., and Galipeau, J. (2020). Aryl hydrocarbon receptor in mesenchymal stromal cells: new frontiers in AhR biology. FEBS J. doi: 10.1111/febs.15599
Abumaree, M. H., Al Jumah, M. A., Kalionis, B., Jawdat, D., Al Khaldi, A., Abomaray, F. M., et al. (2013). Human placental mesenchymal stem cells (pMSCs) play a role as immune suppressive cells by shifting macrophage differentiation from inflammatory M1 to anti-inflammatory M2 macrophages. Stem Cell Rev. Rep. 9, 620–641. doi: 10.1007/s12015-013-9455-2
Abumaree, M. H., Stone, P. R., and Chamley, L. W. (2006). The effects of apoptotic, deported human placental trophoblast on macrophages: possible consequences for pregnancy. J. Reprod. Immunol. 72, 33–45. doi: 10.1016/j.jri.2006.03.001
Alexander, K. A., Chang, M. K., Maylin, E. R., Kohler, T., Muller, R., Wu, A. C., et al. (2011). Osteal macrophages promote in vivo intramembranous bone healing in a mouse tibial injury model. J. Bone. Miner. Res. 26, 1517–1532. doi: 10.1002/jbmr.354
Amouzegar, A., Mittal, S. K., Sahu, A., Sahu, S. K., and Chauhan, S. K. (2017). Mesenchymal stem cells modulate differentiation of myeloid progenitor cells during inflammation. Stem Cells. 35, 1532–1541. doi: 10.1002/stem.2611
Antebi, B., Asher, A. M., Rodriguez, L. A. II, Moore, R. K., Mohammadipoor, A., and Cancio, L. C. (2019). Cryopreserved mesenchymal stem cells regain functional potency following a 24-h acclimation period. J Transl Med. 17:297. doi: 10.1186/s12967-019-2038-5
Baek, G., Choi, H., Kim, Y., Lee, H. C., and Choi, C. (2019). Mesenchymal stem cell-derived extracellular vesicles as therapeutics and as a drug delivery platform. Stem Cells Transl. Med. 8, 880–886. doi: 10.1002/sctm.18-0226
Ball, L. M., Bernardo, M. E., Roelofs, H., van Tol, M. J., Contoli, B., Zwaginga, J. J., et al. (2013). Multiple infusions of mesenchymal stromal cells induce sustained remission in children with steroid-refractory, grade III-IV acute graft-versus-host disease. Br. J. Haematol. 163, 501–509. doi: 10.1111/bjh.12545
Ben-Mordechai, T., Holbova, R., Landa-Rouben, N., Harel-Adar, T., Feinberg, M. S., Abd Elrahman, I., et al. (2013). Macrophage subpopulations are essential for infarct repair with and without stem cell therapy. J. Am. Coll. Cardiol. 62, 1890–1901. doi: 10.1016/j.jacc.2013.07.057
Bianco, P., Robey, P. G., and Simmons, P. J. (2008). Mesenchymal stem cells: revisiting history, concepts, and assays. Cell Stem Cell 2, 313–319. doi: 10.1016/j.stem.2008.03.002
Block, G. J., Ohkouchi, S., Fung, F., Frenkel, J., Gregory, C., Pochampally, R., et al. (2009). Multipotent stromal cells are activated to reduce apoptosis in part by upregulation and secretion of stanniocalcin-1. Stem Cells 27, 670–681. doi: 10.1002/stem.20080742
Boyle, J. J., Harrington, H. A., Piper, E., Elderfield, K., Stark, J., Landis, R. C., et al. (2009). Coronary intraplaque hemorrhage evokes a novel atheroprotective macrophage phenotype. Am. J. Pathol. 174, 1097–1108. doi: 10.2353/ajpath.2009.080431
Braza, F., Dirou, S., Forest, V., Sauzeau, V., Hassoun, D., Chesne, J., et al. (2016). Mesenchymal stem cells induce suppressive macrophages through phagocytosis in a mouse model of Asthma. Stem Cells 34, 1836–1845. doi: 10.1002/stem.2344
Burr, S. P., Dazzi, F., and Garden, O. A. (2013). Mesenchymal stromal cells and regulatory T cells: the Yin and Yang of peripheral tolerance? Immunol Cell Biol. 91, 12–18. doi: 10.1038/icb.2012.60
Caplan, A. I. (2008). All MSCs are pericytes? Cell Stem Cell 3, 229–230. doi: 10.1016/j.stem.2008.08.008
Caplan, A. I., and Correa, D. (2011). The MSC: an injury drugstore. Cell Stem Cell. 9, 11–15. doi: 10.1016/j.stem.2011.06.008
Caplan, A. I., and Dennis, J. E. (2006). Mesenchymal stem cells as trophic mediators. J. Cell. Biochem. 98, 1076–1084. doi: 10.1002/jcb.20886
Champagne, C. M., Takebe, J., Offenbacher, S., and Cooper, L. F. (2002). Macrophage cell lines produce osteoinductive signals that include bone morphogenetic protein-2. Bone 30, 26–31. doi: 10.1016/S8756-3282(01)00638-X
Chang, C. L., Leu, S., Sung, H. C., Zhen, Y. Y., Cho, C. L., Chen, A., et al. (2012). Impact of apoptotic adipose-derived mesenchymal stem cells on attenuating organ damage and reducing mortality in rat sepsis syndrome induced by cecal puncture and ligation. J Transl Med. 10:244. doi: 10.1186/1479-5876-10-244
Chen, M., Li, X., Zhang, X., He, X., Lai, L., Liu, Y., et al. (2015). The inhibitory effect of mesenchymal stem cell on blood-brain barrier disruption following intracerebral hemorrhage in rats: contribution of TSG-6. J. Neuroinflammation 12:61. doi: 10.1186/s12974-015-0284-x
Chen, W., Sun, Y., Gu, X., Hao, Y., Liu, X., Lin, J., et al. (2019). Conditioned medium of mesenchymal stem cells delays osteoarthritis progression in a rat model by protecting subchondral bone, maintaining matrix homeostasis, and enhancing autophagy. J. Tissue Eng. Regen. Med. 13, 1618–1628. doi: 10.1002/term.2916
Chinnadurai, R., Rajan, D., Qayed, M., Arafat, D., Garcia, M., Liu, Y., et al. (2018). Potency analysis of mesenchymal stromal cells using a combinatorial assay matrix approach. Cell Rep. 22, 2504–2517. doi: 10.1016/j.celrep.2018.02.013
Chiossone, L., Conte, R., Spaggiari, G. M., Serra, M., Romei, C., Bellora, F., et al. (2016). Mesenchymal stromal cells induce peculiar alternatively activated macrophages capable of dampening both innate and adaptive immune responses. Stem Cells 34, 1909–1921. doi: 10.1002/stem.2369
Choi, H., Lee, R. H., Bazhanov, N., Oh, J. Y., and Prockop, D. J. (2011). Anti-inflammatory protein TSG-6 secreted by activated MSCs attenuates zymosan-induced mouse peritonitis by decreasing TLR2/NF-kappaB signaling in resident macrophages. Blood 118, 330–338. doi: 10.1182/blood-2010-12-327353
Cosenza, S., Ruiz, M., Toupet, K., Jorgensen, C., and Noel, D. (2017). Mesenchymal stem cells derived exosomes and microparticles protect cartilage and bone from degradation in osteoarthritis. Sci Rep. 7:16214. doi: 10.1038/s41598-017-15376-8
Crisostomo, P. R., Wang, Y., Markel, T. A., Wang, M., Lahm, T., and Meldrum, D. R. (2008). Human mesenchymal stem cells stimulated by TNF-alpha, LPS, or hypoxia produce growth factors by an NF kappa B- but not JNK-dependent mechanism. Am. J. Physiol. Cell Physiol. 294, C675–C682. doi: 10.1152/ajpcell.00437.2007
Cui, Z., Feng, Y., Li, D., Li, T., Gao, P., and Xu, T. (2020). Activation of aryl hydrocarbon receptor (AhR) in mesenchymal stem cells modulates macrophage polarization in asthma. J Immunotoxicol. 17, 21–30. doi: 10.1080/1547691X.2019.1706671
Dayan, V., Yannarelli, G., Billia, F., Filomeno, P., Wang, X. H., Davies, J. E., et al. (2011). Mesenchymal stromal cells mediate a switch to alternatively activated monocytes/macrophages after acute myocardial infarction. Basic Res. Cardiol. 106, 1299–1310. doi: 10.1007/s00395-011-0221-9
de Witte, S. F. H., Luk, F., Sierra Parraga, J. M., Gargesha, M., Merino, A., Korevaar, S. S., et al. (2018). Immunomodulation by therapeutic mesenchymal stromal Cells (MSC) is triggered through phagocytosis of MSC by monocytic cells. Stem Cells. 36, 602–615. doi: 10.1002/stem.2779
Deng, Y., Zhang, Y., Ye, L., Zhang, T., Cheng, J., Chen, G., et al. (2016). umbilical cord-derived mesenchymal stem cells instruct monocytes towards an IL10-producing phenotype by secreting IL6 and HGF. Sci Rep. 6:37566. doi: 10.1038/srep37566
Devaney, J., Horie, S., Masterson, C., Elliman, S., Barry, F., O’Brien, T., et al. (2015). Human mesenchymal stromal cells decrease the severity of acute lung injury induced by E. coli in the rat. Thorax 70, 625–635. doi: 10.1136/thoraxjnl-2015-206813
Dey, A., Allen, J., and Hankey-Giblin, P. A. (2014). Ontogeny and polarization of macrophages in inflammation: blood monocytes versus tissue macrophages. Front. Immunol. 5:683. doi: 10.3389/fimmu.2014.00683
Digiacomo, G., Ziche, M., Dello Sbarba, P., Donnini, S., and Rovida, E. (2015). Prostaglandin E2 transactivates the colony-stimulating factor-1 receptor and synergizes with colony-stimulating factor-1 in the induction of macrophage migration via the mitogen-activated protein kinase ERK1/2. FASEB J. 29, 2545–2554. doi: 10.1096/fj.14-258939
Dominici, M., Le Blanc, K., Mueller, I., Slaper-Cortenbach, I., Marini, F., Krause, D., et al. (2006). Minimal criteria for defining multipotent mesenchymal stromal cells. The international society for cellular therapy position statement. Cytotherapy 8, 315–317. doi: 10.1080/14653240600855905
Du, L., Lin, L., Li, Q., Liu, K., Huang, Y., Wang, X., et al. (2019). IGF-2 Preprograms maturing macrophages to acquire oxidative phosphorylation-dependent anti-inflammatory properties. Cell Metab. 29, 1363.e8–1375.e8. doi: 10.1016/j.cmet.2019.01.006
Ekstrom, K., Omar, O., Graneli, C., Wang, X., Vazirisani, F., and Thomsen, P. (2013). Monocyte exosomes stimulate the osteogenic gene expression of mesenchymal stem cells. PLoS One 8:e75227. doi: 10.1371/journal.pone.0075227
El Kasmi, K. C., and Stenmark, K. R. (2015). Contribution of metabolic reprogramming to macrophage plasticity and function. Semin. Immunol. 27, 267–275. doi: 10.1016/j.smim.2015.09.001
English, K. (2013). Mechanisms of mesenchymal stromal cell immunomodulation. Immunol. Cell Biol. 91, 19–26. doi: 10.1038/icb.2012.56
Erbel, C., Wolf, A., Lasitschka, F., Linden, F., Domschke, G., Akhavanpoor, M., et al. (2015). Prevalence of M4 macrophages within human coronary atherosclerotic plaques is associated with features of plaque instability. Int. J. Cardiol. 186, 219–225. doi: 10.1016/j.ijcard.2015.03.151
Espagnolle, N., Balguerie, A., Arnaud, E., Sensebe, L., and Varin, A. (2017). CD54-Mediated interaction with pro-inflammatory macrophages increases the immunosuppressive function of human mesenchymal stromal cells. Stem Cell Rep. 8, 961–976. doi: 10.1016/j.stemcr.2017.02.008
Essandoh, K., Li, Y., Huo, J., and Fan, G. C. (2016). MiRNA-mediated macrophage polarization and its potential role in the regulation of inflammatory response. Shock 46, 122–131. doi: 10.1097/SHK.0000000000000604
Fadok, V. A., Bratton, D. L., Konowal, A., Freed, P. W., Westcott, J. Y., and Henson, P. M. (1998). Macrophages that have ingested apoptotic cells in vitro inhibit proinflammatory cytokine production through autocrine/paracrine mechanisms involving TGF-beta, PGE2, and PAF. J. Clin. Invest. 101, 890–898. doi: 10.1172/JCI1112
Fahy, N., de Vries-van Melle, M. L., Lehmann, J., Wei, W., Grotenhuis, N., Farrell, E., et al. (2014). Human osteoarthritic synovium impacts chondrogenic differentiation of mesenchymal stem cells via macrophage polarisation state. Osteoarthritis Cartilage 22, 1167–1175. doi: 10.1016/j.joca.2014.05.021
Francois, M., Romieu-Mourez, R., Li, M., and Galipeau, J. (2012). Human MSC suppression correlates with cytokine induction of indoleamine 2,3-dioxygenase and bystander M2 macrophage differentiation. Mol. Ther. 20, 187–195. doi: 10.1038/mt.2011.189
Freytes, D. O., Kang, J. W., Marcos-Campos, I., and Vunjak-Novakovic, G. (2013). Macrophages modulate the viability and growth of human mesenchymal stem cells. J. Cell. Biochem. 114, 220–229. doi: 10.1002/jcb.24357
Galipeau, J., and Sensebe, L. (2018). Mesenchymal stromal cells: clinical challenges and therapeutic opportunities. Cell Stem Cell. 22, 824–833. doi: 10.1016/j.stem.2018.05.004
Galleu, A., Riffo-Vasquez, Y., Trento, C., Lomas, C., Dolcetti, L., Cheung, T. S., et al. (2017). Apoptosis in mesenchymal stromal cells induces in vivo recipient-mediated immunomodulation. Sci. Transl. Med. 9:eaam7828. doi: 10.1126/scitranslmed.aam7828
Giri, J., and Galipeau, J. (2020). Mesenchymal stromal cell therapeutic potency is dependent upon viability, route of delivery, and immune match. Blood Adv. 4, 1987–1997. doi: 10.1182/bloodadvances.2020001711
Goncalves, F. D. C., Luk, F., Korevaar, S. S., Bouzid, R., Paz, A. H., Lopez-Iglesias, C., et al. (2017). Membrane particles generated from mesenchymal stromal cells modulate immune responses by selective targeting of pro-inflammatory monocytes. Sci Rep. 7:12100. doi: 10.1038/s41598-017-12121-z
Hamilton, J. A., and Tak, P. P. (2009). The dynamics of macrophage lineage populations in inflammatory and autoimmune diseases. Arthritis Rheum. 60, 1210–1221. doi: 10.1002/art.24505
Hare, J. M., Traverse, J. H., Henry, T. D., Dib, N., Strumpf, R. K., Schulman, S. P., et al. (2009). A randomized, double-blind, placebo-controlled, dose-escalation study of intravenous adult human mesenchymal stem cells (prochymal) after acute myocardial infarction. J. Am. Coll. Cardiol. 54, 2277–2286. doi: 10.1016/j.jacc.2009.06.055
Harrell, C. R., Fellabaum, C., Jovicic, N., Djonov, V., Arsenijevic, N., and Volarevic, V. (2019a). Molecular mechanisms responsible for therapeutic potential of mesenchymal stem cell-derived secretome. Cells 8:467. doi: 10.3390/cells8050467
Harrell, C. R., Jovicic, N., Djonov, V., Arsenijevic, N., and Volarevic, V. (2019b). Mesenchymal stem cell-derived exosomes and other extracellular vesicles as new remedies in the therapy of inflammatory diseases. Cells 8:1605. doi: 10.3390/cells8121605
Henson, P. M. (2017). Cell removal: efferocytosis. Annu. Rev. Cell Dev. Biol. 33, 127–144. doi: 10.1146/annurev-cellbio-111315-125315
Hinden, L., Shainer, R., Almogi-Hazan, O., and Or, R. (2015). Ex vivo induced regulatory human/murine mesenchymal stem cells as immune modulators. Stem Cells. 33, 2256–2267. doi: 10.1002/stem.2026
Hoeffel, G., and Ginhoux, F. (2015). Ontogeny of tissue-resident macrophages. Front. Immunol. 6:486. doi: 10.3389/fimmu.2015.00486
Ibrahim, A. G., Cheng, K., and Marban, E. (2014). Exosomes as critical agents of cardiac regeneration triggered by cell therapy. Stem Cell Rep. 2, 606–619. doi: 10.1016/j.stemcr.2014.04.006
Ionescu, L., Byrne, R. N., van Haaften, T., Vadivel, A., Alphonse, R. S., Rey-Parra, G. J., et al. (2012). Stem cell conditioned medium improves acute lung injury in mice: in vivo evidence for stem cell paracrine action. Am. J. Physiol. Lung. Cell Mol. Physiol. 303, L967–L977. doi: 10.1152/ajplung.00144.2011
Ishikane, S., Hosoda, H., Yamahara, K., Akitake, Y., Kyoungsook, J., Mishima, K., et al. (2013). Allogeneic transplantation of fetal membrane-derived mesenchymal stem cell sheets increases neovascularization and improves cardiac function after myocardial infarction in rats. Transplantation 96, 697–706. doi: 10.1097/TP.0b013e31829f753d
Jackson, M. V., Morrison, T. J., Doherty, D. F., McAuley, D. F., Matthay, M. A., Kissenpfennig, A., et al. (2016). Mitochondrial transfer via tunneling nanotubes is an important mechanism by which mesenchymal stem cells enhance macrophage phagocytosis in the in vitro and in vivo models of ARDS. Stem Cells 34, 2210–2223. doi: 10.1002/stem.2372
Jia, X. H., Feng, G. W., Wang, Z. L., Du, Y., Shen, C., Hui, H., et al. (2016). Activation of mesenchymal stem cells by macrophages promotes tumor progression through immune suppressive effects. Oncotarget 7, 20934–20944. doi: 10.18632/oncotarget.8064
Kadl, A., Meher, A. K., Sharma, P. R., Lee, M. Y., Doran, A. C., Johnstone, S. R., et al. (2010). Identification of a novel macrophage phenotype that develops in response to atherogenic phospholipids via Nrf2. Circ. Res. 107, 737–746. doi: 10.1161/CIRCRESAHA.109.215715
Kalinski, P. (2012). Regulation of immune responses by prostaglandin E2. J. Immunol. 188, 21–28. doi: 10.4049/jimmunol.1101029
Ko, J. H., Kim, H. J., Jeong, H. J., Lee, H. J., and Oh, J. Y. (2020). Mesenchymal stem and stromal cells harness macrophage-derived amphiregulin to maintain tissue homeostasis. Cell Rep. 30, 3806.e6–3820.e6. doi: 10.1016/j.celrep.2020.02.062
Lankford, K. L., Arroyo, E. J., Nazimek, K., Bryniarski, K., Askenase, P. W., and Kocsis, J. D. (2018). Intravenously delivered mesenchymal stem cell-derived exosomes target M2-type macrophages in the injured spinal cord. PLoS One 13:e0190358. doi: 10.1371/journal.pone.0190358
Lee, H. J., Kim, S. N., Jeon, M. S., Yi, T., and Song, S. U. (2017). ICOSL expression in human bone marrow-derived mesenchymal stem cells promotes induction of regulatory T cells. Sci Rep. 7:44486. doi: 10.1038/srep44486
Lewis, H. C., Chinnadurai, R., Bosinger, S. E., and Galipeau, J. (2017). The IDO inhibitor 1-methyl tryptophan activates the aryl hydrocarbon receptor response in mesenchymal stromal cells. Oncotarget 8, 91914–91927. doi: 10.18632/oncotarget.20166
Li, Q., Sun, W., Wang, X., Zhang, K., Xi, W., and Gao, P. (2015). Skin-derived mesenchymal stem cells alleviate atherosclerosis via modulating macrophage function. Stem Cells Transl. Med. 4, 1294–1301. doi: 10.5966/sctm.2015-0020
Li, Y., Zhang, D., Xu, L., Dong, L., Zheng, J., Lin, Y., et al. (2019). Cell-cell contact with proinflammatory macrophages enhances the immunotherapeutic effect of mesenchymal stem cells in two abortion models. Cell Mol. Immunol. 16, 908–920. doi: 10.1038/s41423-019-0204-6
Lo Sicco, C., Reverberi, D., Balbi, C., Ulivi, V., Principi, E., Pascucci, L., et al. (2017). Mesenchymal stem cell-derived extracellular vesicles as mediators of anti-inflammatory effects: endorsement of macrophage polarization. Stem Cells Transl Med. 6, 1018–1028. doi: 10.1002/sctm.16-0363
Lopez-Rodriguez, A. B., Acaz-Fonseca, E., Giatti, S., Caruso, D., Viveros, M. P., Melcangi, R. C., et al. (2015). Correlation of brain levels of progesterone and dehydroepiandrosterone with neurological recovery after traumatic brain injury in female mice. Psychoneuroendocrinology 56, 1–11. doi: 10.1016/j.psyneuen.2015.02.018
Luk, F., de Witte, S. F., Korevaar, S. S., Roemeling-van Rhijn, M., Franquesa, M., Strini, T., et al. (2016). Inactivated mesenchymal stem cells maintain immunomodulatory capacity. Stem Cells Dev. 25, 1342–1354. doi: 10.1089/scd.2016.0068
Luz-Crawford, P., Djouad, F., Toupet, K., Bony, C., Franquesa, M., Hoogduijn, M. J., et al. (2016). Mesenchymal stem cell-derived interleukin 1 receptor antagonist promotes macrophage polarization and inhibits B cell differentiation. Stem Cells 34, 483–492. doi: 10.1002/stem.2254
Luz-Crawford, P., Kurte, M., Bravo-Alegria, J., Contreras, R., Nova-Lamperti, E., Tejedor, G., et al. (2013). Mesenchymal stem cells generate a CD4+CD25+Foxp3+ regulatory T cell population during the differentiation process of Th1 and Th17 cells. Stem Cell Res Ther. 4:65. doi: 10.1186/scrt216
Manferdini, C., Paolella, F., Gabusi, E., Gambari, L., Piacentini, A., Filardo, G., et al. (2017). Adipose stromal cells mediated switching of the pro-inflammatory profile of M1-like macrophages is facilitated by PGE2: in vitro evaluation. Osteoarthritis Cartilage 25, 1161–1171. doi: 10.1016/j.joca.2017.01.011
Manich, G., Recasens, M., Valente, T., Almolda, B., Gonzalez, B., and Castellano, B. (2019). Role of the CD200-CD200R axis during homeostasis and neuroinflammation. Neuroscience 405, 118–136. doi: 10.1016/j.neuroscience.2018.10.030
Mao, F., Wu, Y., Tang, X., Kang, J., Zhang, B., Yan, Y., et al. (2017). Exosomes derived from human umbilical cord mesenchymal stem cells relieve inflammatory bowel disease in mice. Biomed Res Int. 2017:5356760. doi: 10.1155/2017/5356760
Martinez, F. O., Sica, A., Mantovani, A., and Locati, M. (2008). Macrophage activation and polarization. Front. Biosci. 13:453–461. doi: 10.2741/2692
Matsubara, K., Matsushita, Y., Sakai, K., Kano, F., Kondo, M., Noda, M., et al. (2015). Secreted ectodomain of sialic acid-binding Ig-like lectin-9 and monocyte chemoattractant protein-1 promote recovery after rat spinal cord injury by altering macrophage polarity. J. Neurosci. 35, 2452–2464. doi: 10.1523/JNEUROSCI.4088-14.2015
Melief, S. M., Geutskens, S. B., Fibbe, W. E., and Roelofs, H. (2013). Multipotent stromal cells skew monocytes towards an anti-inflammatory interleukin-10-producing phenotype by production of interleukin-6. Haematologica 98, 888–895. doi: 10.3324/haematol.2012.078055
Menge, T., Zhao, Y., Zhao, J., Wataha, K., Gerber, M., Zhang, J., et al. (2012). Mesenchymal stem cells regulate blood-brain barrier integrity through TIMP3 release after traumatic brain injury. Sci. Transl. Med. 4:161ra50. doi: 10.1126/scitranslmed.3004660
Mills, C. D., Kincaid, K., Alt, J. M., Heilman, M. J., and Hill, A. M. (2000). M-1/M-2 macrophages and the Th1/Th2 paradigm. J. Immunol. 164, 6166–6173. doi: 10.4049/jimmunol.164.12.6166
Mills, C. D., Thomas, A. C., Lenz, L. L., and Munder, M. (2014). Macrophage: SHIP of immunity. Front Immunol. 5:620. doi: 10.3389/fimmu.2014.00620
Min, H., Xu, L., Parrott, R., Overall, C. C., Lillich, M., Rabjohns, E. M., et al. (2020). Mesenchymal stromal cells reprogram monocytes and macrophages with processing bodies. Stem Cells J. doi: 10.1002/stem.3292
Mittal, M., Tiruppathi, C., Nepal, S., Zhao, Y. Y., Grzych, D., Soni, D., et al. (2016). TNFalpha-stimulated gene-6 (TSG6) activates macrophage phenotype transition to prevent inflammatory lung injury. Proc. Natl. Acad. Sci. U.S.A. 113, E8151–E8158. doi: 10.1073/pnas.1614935113
Moll, G., Geissler, S., Catar, R., Ignatowicz, L., Hoogduijn, M. J., Strunk, D., et al. (2016). Cryopreserved or fresh mesenchymal stromal cells: only a matter of taste or key to unleash the full clinical potential of MSC therapy? Adv Exp Med Biol. 951, 77–98. doi: 10.1007/978-3-319-45457-3_7
Morrison, T. J., Jackson, M. V., Cunningham, E. K., Kissenpfennig, A., McAuley, D. F., O’Kane, C. M., et al. (2017). Mesenchymal stromal cells modulate macrophages in clinically relevant lung injury models by extracellular vesicle mitochondrial transfer. Am. J. Respir. Crit. Care Med. 196, 1275–1286. doi: 10.1164/rccm.201701-0170OC
Murray, P. J., and Wynn, T. A. (2011). Protective and pathogenic functions of macrophage subsets. Nat. Rev. Immunol. 11, 723–737. doi: 10.1038/nri3073
Na, Y. R., Jung, D., Yoon, B. R., Lee, W. W., and Seok, S. H. (2015). Endogenous prostaglandin E2 potentiates anti-inflammatory phenotype of macrophage through the CREB-C/EBP-beta cascade. Eur. J. Immunol. 45, 2661–2671. doi: 10.1002/eji.201545471
Nahrendorf, M., and Swirski, F. K. (2016). Abandoning M1/M2 for a network model of macrophage function. Circ. Res. 119, 414–417. doi: 10.1161/CIRCRESAHA.116.309194
Nakajima, H., Uchida, K., Guerrero, A. R., Watanabe, S., Sugita, D., Takeura, N., et al. (2012). Transplantation of mesenchymal stem cells promotes an alternative pathway of macrophage activation and functional recovery after spinal cord injury. J. Neurotrauma 29, 1614–1625. doi: 10.1089/neu.2011.2109
Nemeth, K., Leelahavanichkul, A., Yuen, P. S., Mayer, B., Parmelee, A., Doi, K., et al. (2009). Bone marrow stromal cells attenuate sepsis via prostaglandin E(2)-dependent reprogramming of host macrophages to increase their interleukin-10 production. Nat Med. 15, 42–49. doi: 10.1038/nm.1905
Noronha, N. C., Mizukami, A., Caliari-Oliveira, C., Cominal, J. G., Rocha, J. L. M., Covas, D. T., et al. (2019). Priming approaches to improve the efficacy of mesenchymal stromal cell-based therapies. Stem Cell Res Ther. 10:131. doi: 10.1186/s13287-019-1259-0
Oh, J. Y., Ko, J. H., Lee, H. J., Yu, J. M., Choi, H., Kim, M. K., et al. (2014). Mesenchymal stem/stromal cells inhibit the NLRP3 inflammasome by decreasing mitochondrial reactive oxygen species. Stem Cells. 32, 1553–1563. doi: 10.1002/stem.1608
Oh, J. Y., Lee, R. H., Yu, J. M., Ko, J. H., Lee, H. J., Ko, A. Y., et al. (2012). Intravenous mesenchymal stem cells prevented rejection of allogeneic corneal transplants by aborting the early inflammatory response. Mol. Ther. 20, 2143–2152. doi: 10.1038/mt.2012.165
Ong, S. M., Teng, K., Newell, E., Chen, H., Chen, J., Loy, T., et al. (2019). A novel, five-marker alternative to CD16-CD14 gating to identify the three human monocyte subsets. Front. Immunol. 10:1761. doi: 10.3389/fimmu.2019.01761
Ortiz, L. A., Dutreil, M., Fattman, C., Pandey, A. C., Torres, G., Go, K., et al. (2007). Interleukin 1 receptor antagonist mediates the antiinflammatory and antifibrotic effect of mesenchymal stem cells during lung injury. Proc. Natl. Acad. Sci. U.S.A. 104, 11002–11007. doi: 10.1073/pnas.0704421104
Palumbo, P., Lombardi, F., Siragusa, G., Cifone, M. G., Cinque, B., and Giuliani, M. (2018). Methods of isolation, characterization and expansion of human adipose-derived stem cells (ASCs): an overview. Int J Mol Sci. 19:1897. doi: 10.3390/ijms19071897
Papaccio, F., Paino, F., Regad, T., Papaccio, G., Desiderio, V., and Tirino, V. (2017). Concise review: cancer cells, cancer stem cells, and mesenchymal stem cells: influence in cancer development. Stem Cells Transl. Med. 6, 2115–2125. doi: 10.1002/sctm.17-0138
Patel, A. A., Zhang, Y., Fullerton, J. N., Boelen, L., Rongvaux, A., Maini, A. A., et al. (2017). The fate and lifespan of human monocyte subsets in steady state and systemic inflammation. J Exp Med. 214, 1913–1923. doi: 10.1084/jem.20170355
Peruzzotti-Jametti, L., Bernstock, J. D., Vicario, N., Costa, A. S. H., Kwok, C. K., Leonardi, T., et al. (2018). Macrophage-derived extracellular succinate licenses neural stem cells to suppress chronic neuroinflammation. Cell Stem Cell 22, 355.e13–368.e13. doi: 10.1016/j.stem.2018.01.020
Phinney, D. G., Di Giuseppe, M., Njah, J., Sala, E., Shiva, S., St Croix, C. M., et al. (2015). Mesenchymal stem cells use extracellular vesicles to outsource mitophagy and shuttle microRNAs. Nat. Commun. 6:8472. doi: 10.1038/ncomms9472
Pietila, M., Lehtonen, S., Tuovinen, E., Lahteenmaki, K., Laitinen, S., Leskela, H. V., et al. (2012). CD200 positive human mesenchymal stem cells suppress TNF-alpha secretion from CD200 receptor positive macrophage-like cells. PLoS One 7:e31671. doi: 10.1371/journal.pone.0031671
Poon, I. K., Lucas, C. D., Rossi, A. G., and Ravichandran, K. S. (2014). Apoptotic cell clearance: basic biology and therapeutic potential. Nat. Rev. Immunol. 14, 166–180. doi: 10.1038/nri3607
Quaedackers, M. E., Baan, C. C., Weimar, W., and Hoogduijn, M. J. (2009). Cell contact interaction between adipose-derived stromal cells and allo-activated T lymphocytes. Eur. J. Immunol. 39, 3436–3446. doi: 10.1002/eji.200939584
Quail, D. F., and Joyce, J. A. (2013). Microenvironmental regulation of tumor progression and metastasis. Nat. Med. 19, 1423–1437. doi: 10.1038/nm.3394
Raggi, F., Pelassa, S., Pierobon, D., Penco, F., Gattorno, M., Novelli, F., et al. (2017). Regulation of human macrophage M1-M2 polarization balance by hypoxia and the triggering receptor expressed on myeloid cells-1. Front Immunol. 8:1097. doi: 10.3389/fimmu.2017.01097
Ren, G., Zhang, L., Zhao, X., Xu, G., Zhang, Y., Roberts, A. I., et al. (2008). Mesenchymal stem cell-mediated immunosuppression occurs via concerted action of chemokines and nitric oxide. Cell Stem Cell 2, 141–150. doi: 10.1016/j.stem.2007.11.014
Ridge, S. M., Sullivan, F. J., and Glynn, S. A. (2017). Mesenchymal stem cells: key players in cancer progression. Mol. Cancer 16:31. doi: 10.1186/s12943-017-0597-8
Riordan, N. H., Hincapie, M. L., Morales, I., Fernandez, G., Allen, N., Leu, C., et al. (2019). Allogeneic human umbilical cord mesenchymal stem cells for the treatment of autism spectrum disorder in children: safety profile and effect on cytokine levels. Stem Cells Transl. Med. 8, 1008–1016. doi: 10.1002/sctm.19-0010
Rozenberg, A., Rezk, A., Boivin, M. N., Darlington, P. J., Nyirenda, M., Li, R., et al. (2016). Human mesenchymal stem cells impact th17 and th1 responses through a prostaglandin e2 and myeloid-dependent mechanism. Stem Cells Transl. Med. 5, 1506–1514. doi: 10.5966/sctm.2015-0243
Salgado, A. J., and Gimble, J. M. (2013). Secretome of mesenchymal stem/stromal cells in regenerative medicine. Biochimie 95:2195. doi: 10.1016/j.biochi.2013.10.013
Sasaki, M., Abe, R., Fujita, Y., Ando, S., Inokuma, D., and Shimizu, H. (2008). Mesenchymal stem cells are recruited into wounded skin and contribute to wound repair by transdifferentiation into multiple skin cell type. J. Immunol. 180, 2581–2587. doi: 10.4049/jimmunol.180.4.2581
Schmidt, A., Zhang, X. M., Joshi, R. N., Iqbal, S., Wahlund, C., Gabrielsson, S., et al. (2016). Human macrophages induce CD4(+)Foxp3(+) regulatory T cells via binding and re-release of TGF-beta. Immunol. Cell Biol. 94, 747–762. doi: 10.1038/icb.2016.34
Secunda, R., Vennila, R., Mohanashankar, A. M., Rajasundari, M., Jeswanth, S., and Surendran, R. (2015). Isolation, expansion and characterisation of mesenchymal stem cells from human bone marrow, adipose tissue, umbilical cord blood and matrix: a comparative study. Cytotechnology 67, 793–807. doi: 10.1007/s10616-014-9718-z
Serejo, T. R. T., Silva-Carvalho, A. E., Braga, L., Neves, F. A. R., Pereira, R. W., Carvalho, J. L., et al. (2019). Assessment of the immunosuppressive potential of INF-gamma licensed adipose mesenchymal stem cells, their secretome and extracellular vesicles. Cells 8:22. doi: 10.3390/cells8010022
Sesia, S. B., Duhr, R., Medeiros da Cunha, C., Todorov, A., Schaeren, S., Padovan, E., et al. (2015). Anti-inflammatory/tissue repair macrophages enhance the cartilage-forming capacity of human bone marrow-derived mesenchymal stromal cells. J. Cell. Physiol. 230, 1258–1269. doi: 10.1002/jcp.24861
Shapouri-Moghaddam, A., Mohammadian, S., Vazini, H., Taghadosi, M., Esmaeili, S. A., Mardani, F., et al. (2018). Macrophage plasticity, polarization, and function in health and disease. J. Cell. Physiol. 233, 6425–6440. doi: 10.1002/jcp.26429
Shi, M., Li, J., Liao, L., Chen, B., Li, B., Chen, L., et al. (2007). Regulation of CXCR4 expression in human mesenchymal stem cells by cytokine treatment: role in homing efficiency in NOD/SCID mice. Haematologica 92, 897–904. doi: 10.3324/haematol.10669
Shin, T. H., Kim, H. S., Kang, T. W., Lee, B. C., Lee, H. Y., Kim, Y. J., et al. (2016). Human umbilical cord blood-stem cells direct macrophage polarization and block inflammasome activation to alleviate rheumatoid arthritis. Cell Death Dis. 7:e2524. doi: 10.1038/cddis.2016.442
Shoji, M., Oskowitz, A., Malone, C. D., Prockop, D. J., and Pochampally, R. (2011). Human mesenchymal stromal cells (MSCs) reduce neointimal hyperplasia in a mouse model of flow-restriction by transient suppression of anti-inflammatory cytokines. J. Atheroscler. Thromb. 18, 464–474. doi: 10.5551/jat.6213
Song, W. J., Li, Q., Ryu, M. O., Ahn, J. O., Bhang, D. H., Jung, Y. C., et al. (2018). TSG-6 released from intraperitoneally injected canine adipose tissue-derived mesenchymal stem cells ameliorate inflammatory bowel disease by inducing M2 macrophage switch in mice. Stem Cell Res Ther. 9:91. doi: 10.1186/s13287-018-0841-1
Spaggiari, G. M., Capobianco, A., Abdelrazik, H., Becchetti, F., Mingari, M. C., and Moretta, L. (2008). Mesenchymal stem cells inhibit natural killer-cell proliferation, cytotoxicity, and cytokine production: role of indoleamine 2,3-dioxygenase and prostaglandin E2. Blood 111, 1327–1333. doi: 10.1182/blood-2007-02-074997
Sun, J. M., Dawson, G., Franz, L., Howard, J., McLaughlin, C., Kistler, B., et al. (2020). Infusion of human umbilical cord tissue mesenchymal stromal cells in children with autism spectrum disorder. Stem Cells Transl. Med. 9, 1137–1146. doi: 10.1002/sctm.19-0434
Sun, X., Shan, A., Wei, Z., and Xu, B. (2018). Intravenous mesenchymal stem cell-derived exosomes ameliorate myocardial inflammation in the dilated cardiomyopathy. Biochem. Biophys. Res. Commun. 503, 2611–2618. doi: 10.1016/j.bbrc.2018.08.012
Sung, P. H., Chang, C. L., Tsai, T. H., Chang, L. T., Leu, S., Chen, Y. L., et al. (2013). Apoptotic adipose-derived mesenchymal stem cell therapy protects against lung and kidney injury in sepsis syndrome caused by cecal ligation puncture in rats. Stem Cell Res Ther. 4:155. doi: 10.1186/scrt385
Tannahill, G. M., Curtis, A. M., Adamik, J., Palsson-McDermott, E. M., McGettrick, A. F., Goel, G., et al. (2013). Succinate is an inflammatory signal that induces IL-1beta through HIF-1alpha. Nature 496, 238–242. doi: 10.1038/nature11986
Tedgui, A., and Mallat, Z. (2006). Cytokines in atherosclerosis: pathogenic and regulatory pathways. Physiol. Rev. 86, 515–581. doi: 10.1152/physrev.00024.2005
Thomas, G., Tacke, R., Hedrick, C. C., and Hanna, R. N. (2015). Nonclassical patrolling monocyte function in the vasculature. Arterioscler. Thromb. Vasc. Biol. 35, 1306–1316. doi: 10.1161/ATVBAHA.114.304650
Ti, D., Hao, H., Tong, C., Liu, J., Dong, L., Zheng, J., et al. (2015). LPS-preconditioned mesenchymal stromal cells modify macrophage polarization for resolution of chronic inflammation via exosome-shuttled let-7b. J. Transl. Med. 13:308. doi: 10.1186/s12967-015-0642-6
Tomchuck, S. L., Zwezdaryk, K. J., Coffelt, S. B., Waterman, R. S., Danka, E. S., and Scandurro, A. B. (2008). Toll-like receptors on human mesenchymal stem cells drive their migration and immunomodulating responses. Stem Cells. 26, 99–107. doi: 10.1634/stemcells.2007-0563
Vagnozzi, R. J., Maillet, M., Sargent, M. A., Khalil, H., Johansen, A. K. Z., Schwanekamp, J. A., et al. (2020). An acute immune response underlies the benefit of cardiac stem cell therapy. Nature 577, 405–409. doi: 10.1038/s41586-019-1802-2
van Furth, R., and Cohn, Z. A. (1968). The origin and kinetics of mononuclear phagocytes. J. Exp. Med. 128, 415–435. doi: 10.1084/jem.128.3.415
Varin, A., Pontikoglou, C., Labat, E., Deschaseaux, F., and Sensebe, L. (2013). CD200R/CD200 inhibits osteoclastogenesis: new mechanism of osteoclast control by mesenchymal stem cells in human. PLoS One 8:e72831. doi: 10.1371/journal.pone.0072831
Vasandan, A. B., Jahnavi, S., Shashank, C., Prasad, P., Kumar, A., and Prasanna, S. J. (2016). Human mesenchymal stem cells program macrophage plasticity by altering their metabolic status via a PGE2-dependent mechanism. Sci Rep. 6:38308. doi: 10.1038/srep38308
Viola, A., Munari, F., Sanchez-Rodriguez, R., Scolaro, T., and Castegna, A. (2019). The metabolic signature of macrophage responses. Front Immunol. 10:1462. doi: 10.3389/fimmu.2019.01462
Wang, J., Huang, R., Xu, Q., Zheng, G., Qiu, G., Ge, M., et al. (2020). Mesenchymal stem cell-derived extracellular vesicles alleviate acute lung injury via transfer of miR-27a-3p. Crit. Care Med. 48, e599–e610. doi: 10.1097/CCM.0000000000004315
Wang, Z. X., Wang, C. Q., Li, X. Y., Feng, G. K., Zhu, H. L., Ding, Y., et al. (2015). Mesenchymal stem cells alleviate atherosclerosis by elevating number and function of CD4(+)CD25 (+)FOXP3 (+) regulatory T-cells and inhibiting macrophage foam cell formation. Mol. Cell. Biochem. 400, 163–172. doi: 10.1007/s11010-014-2272-3
Waterman, R. S., Henkle, S. L., and Betancourt, A. M. (2012). Mesenchymal stem cell 1 (MSC1)-based therapy attenuates tumor growth whereas MSC2-treatment promotes tumor growth and metastasis. PLoS One 7:e45590. doi: 10.1371/journal.pone.0045590
Waterman, R. S., Tomchuck, S. L., Henkle, S. L., and Betancourt, A. M. (2010). A new mesenchymal stem cell (MSC) paradigm: polarization into a pro-inflammatory MSC1 or an Immunosuppressive MSC2 phenotype. PLoS One 5:e10088. doi: 10.1371/journal.pone.0010088
Wei, X., Sun, G., Zhao, X., Wu, Q., Chen, L., Xu, Y., et al. (2019). Human amnion mesenchymal stem cells attenuate atherosclerosis by modulating macrophage function to reduce immune response. Int. J. Mol. Med. 44, 1425–1435. doi: 10.3892/ijmm.2019.4286
Weinberger, T., and Schulz, C. (2015). Myocardial infarction: a critical role of macrophages in cardiac remodeling. Front Physiol. 6:107. doi: 10.3389/fphys.2015.00107
Willis, G. R., Fernandez-Gonzalez, A., Anastas, J., Vitali, S. H., Liu, X., Ericsson, M., et al. (2018a). Mesenchymal stromal cell exosomes ameliorate experimental bronchopulmonary dysplasia and restore lung function through macrophage immunomodulation. Am. J. Respir. Crit. Care Med. 197, 104–116. doi: 10.1164/rccm.201705-0925OC
Willis, G. R., Fernandez-Gonzalez, A., Reis, M., Mitsialis, S. A., and Kourembanas, S. (2018b). Macrophage immunomodulation: the gatekeeper for mesenchymal stem cell derived-exosomes in pulmonary arterial hypertension? Int. J. Mol. Sci. 19:2534. doi: 10.3390/ijms19092534
Wright, G. J., Cherwinski, H., Foster-Cuevas, M., Brooke, G., Puklavec, M. J., Bigler, M., et al. (2003). Characterization of the CD200 receptor family in mice and humans and their interactions with CD200. J. Immunol. 171, 3034–3046. doi: 10.4049/jimmunol.171.6.3034
Wu, Y., Chen, L., Scott, P. G., and Tredget, E. E. (2007). Mesenchymal stem cells enhance wound healing through differentiation and angiogenesis. Stem Cells 25, 2648–2659. doi: 10.1634/stemcells.2007-0226
Wynn, T. A., Chawla, A., and Pollard, J. W. (2013). Macrophage biology in development, homeostasis and disease. Nature 496, 445–455. doi: 10.1038/nature12034
Xu, C., Fu, F., Li, X., and Zhang, S. (2017). Mesenchymal stem cells maintain the microenvironment of central nervous system by regulating the polarization of macrophages/microglia after traumatic brain injury. Int. J. Neurosci. 127, 1124–1135. doi: 10.1080/00207454.2017.1325884
Xu, T., Zhou, Y., Qiu, L., Do, D. C., Zhao, Y., Cui, Z., et al. (2015). Aryl hydrocarbon receptor protects lungs from cockroach allergen-induced inflammation by modulating mesenchymal stem cells. J. Immunol. 195, 5539–5550. doi: 10.4049/jimmunol.1501198
Xue, J., Schmidt, S. V., Sander, J., Draffehn, A., Krebs, W., Quester, I., et al. (2014). Transcriptome-based network analysis reveals a spectrum model of human macrophage activation. Immunity 40, 274–288. doi: 10.1016/j.immuni.2014.01.006
Yang, D. H., and Yang, M. Y. (2019). The role of macrophage in the pathogenesis of osteoporosis. Int. J. Mol. Sci. 20:2093. doi: 10.3390/ijms20092093
Yang, S., Yuan, H. Q., Hao, Y. M., Ren, Z., Qu, S. L., Liu, L. S., et al. (2020). Macrophage polarization in atherosclerosis. Clin. Chim. Acta 501, 142–146. doi: 10.1016/j.cca.2019.10.034
Yasui, M., Tamura, Y., Minami, M., Higuchi, S., Fujikawa, R., Ikedo, T., et al. (2015). The prostaglandin E2 receptor EP4 regulates obesity-related inflammation and insulin sensitivity. PLoS One 10:e0136304. doi: 10.1371/journal.pone.0136304
Yu, B., Shao, H., Su, C., Jiang, Y., Chen, X., Bai, L., et al. (2016a). Exosomes derived from MSCs ameliorate retinal laser injury partially by inhibition of MCP-1. Sci. Rep. 6:34562. doi: 10.1038/srep34562
Yu, B., Sondag, G. R., Malcuit, C., Kim, M. H., and Safadi, F. F. (2016b). Macrophage-associated Osteoactivin/GPNMB mediates mesenchymal stem cell survival, proliferation, and migration Via a CD44-dependent mechanism. J. Cell. Biochem. 117, 1511–1521. doi: 10.1002/jcb.25394
Zhang, B., Zhang, J., Zhu, D., and Kong, Y. (2019a). Mesenchymal stem cells rejuvenate cardiac muscle after ischemic injury. Aging 11, 63–72. doi: 10.18632/aging.101718
Zhang, B., Zhao, N., Zhang, J., Liu, Y., Zhu, D., and Kong, Y. (2019b). Mesenchymal stem cells rejuvenate cardiac muscle through regulating macrophage polarization. Aging 11, 3900–3908. doi: 10.18632/aging.102009
Zhang, M., Mal, N., Kiedrowski, M., Chacko, M., Askari, A. T., Popovic, Z. B., et al. (2007). SDF-1 expression by mesenchymal stem cells results in trophic support of cardiac myocytes after myocardial infarction. FASEB J. 21, 3197–3207. doi: 10.1096/fj.06-6558com
Zhang, S., Chu, W. C., Lai, R. C., Lim, S. K., Hui, J. H., and Toh, W. S. (2016). Exosomes derived from human embryonic mesenchymal stem cells promote osteochondral regeneration. Osteoarthritis Cartilage 24, 2135–2140. doi: 10.1016/j.joca.2016.06.022
Keywords: macrophages (M1/M2), mechanism of action (MOA), immunomodulation, cell therapy, mesenchymal stromal (or stem) cells
Citation: Stevens HY, Bowles AC, Yeago C and Roy K (2020) Molecular Crosstalk Between Macrophages and Mesenchymal Stromal Cells. Front. Cell Dev. Biol. 8:600160. doi: 10.3389/fcell.2020.600160
Received: 28 August 2020; Accepted: 05 November 2020;
Published: 09 December 2020.
Edited by:
Antonio Salgado, University of Minho, PortugalReviewed by:
Gianandrea Pasquinelli, University of Bologna, ItalyLudmila Buravkova, Institute of Biomedical Problems, Russian Academy of Sciences, Russia
Charles Samuel Cox, The University of Texas Health Science Center at Houston, United States
Copyright © 2020 Stevens, Bowles, Yeago and Roy. This is an open-access article distributed under the terms of the Creative Commons Attribution License (CC BY). The use, distribution or reproduction in other forums is permitted, provided the original author(s) and the copyright owner(s) are credited and that the original publication in this journal is cited, in accordance with accepted academic practice. No use, distribution or reproduction is permitted which does not comply with these terms.
*Correspondence: Krishnendu Roy, a3Jpc2gucm95QGdhdGVjaC5lZHU=