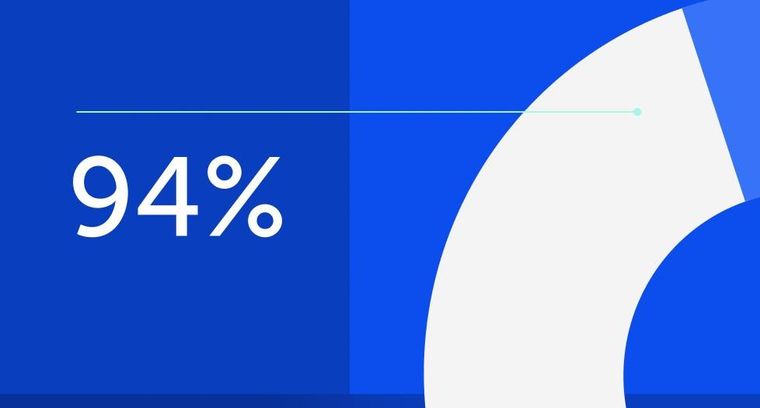
94% of researchers rate our articles as excellent or good
Learn more about the work of our research integrity team to safeguard the quality of each article we publish.
Find out more
REVIEW article
Front. Cell Dev. Biol., 16 November 2020
Sec. Signaling
Volume 8 - 2020 | https://doi.org/10.3389/fcell.2020.599281
This article is part of the Research TopicMolecular Mechanisms and Signaling in Endothelial Cell Biology and Vascular HeterogeneityView all 27 articles
The vascular endothelial growth factors (VEGFs) and their receptors (VEGFRs) play crucial roles in vasculogenesis and angiogenesis. Angiogenesis is an important mechanism in many physiological and pathological processes, and is involved in endothelial cell proliferation, migration, and survival, then leads to further tubulogenesis, and finally promotes formation of vessels. This series of signaling cascade pathways are precisely mediated by VEGF/VEGFR-2 system. The VEGF binding to the IgD2 and IgD3 of VEGFR-2 induces the dimerization of the receptor, subsequently the activation and trans-autophosphorylation of the tyrosine kinase, and then the initiation of the intracellular signaling cascades. Finally the VEGF-activated VEGFR-2 stimulates and mediates variety of signaling transduction, biological responses, and pathological processes in angiogenesis. Several crucial phosphorylated sites Tyr801, Try951, Try1175, and Try1214 in the VEGFR-2 intracellular domains mediate several key signaling processes including PLCγ-PKC, TSAd-Src-PI3K-Akt, SHB-FAK-paxillin, SHB-PI3K-Akt, and NCK-p38-MAPKAPK2/3 pathways. Based on the molecular structure and signaling pathways of VEGFR-2, the strategy of the VEGFR-2-targeted therapy should be considered to employ in the treatment of the VEGF/VEGFR-2-associated diseases by blocking the VEGF/VEGFR-2 signaling pathway, inhibiting VEGF and VEGFR-2 gene expression, blocking the binding of VEGF and VEGFR-2, and preventing the proliferation, migration, and survival of vascular endothelial cells expressing VEGFR-2.
In organism, various physiological and pathological processes are involved in vasculogenesis, angiogenesis, and formation and maintenance of new blood vessel structures, including embryonic development (Vallon et al., 2014), tissue growth and wound healing (Ivkovic et al., 2003), tumorigenesis (Yehya et al., 2018), rheumatoid arthritis (Marrelli et al., 2011), diabetic retinopathy (Crawford et al., 2009; Cheng and Ma, 2015), axon growth (Klagsbrun and Eichmann, 2005), cancer (Hanahan and Folkman, 1996; Rajabi and Mousa, 2017; Li et al., 2020), and inflammation (Alkim et al., 2015), which are stimulated by a variety of factors, including basic fibroblast growth factor (bFGF) (Iwasaki et al., 2004), vascular endothelial growth factor (VEGF) (Hoeben et al., 2004; Nilsson and Heymach, 2006), platelet-derived growth Factor (PDGF) (Lindahl et al., 1999), ephrin-Eph receptors (Zhang and Hughes, 2006), angiopoietin-1 (Yin et al., 2020), hepatocyte growth factor (HGF) (Kaga et al., 2012), transforming growth factor-β (TGF-β) (Ferrari et al., 2009), and interleukin 6 (IL-6) (Gopinathan et al., 2015), etc. VEGFs and their receptors (VEGFRs) are currently the most important and specific factors to stimulate endothelial cell proliferation, regulate both the development of blood vessels from precursor cells during early embryogenesis and the formation of blood vessels from pre-existing vessels at a later stage, and increase vascular permeability and chemotaxis of vascular endothelial cells (Ferrara and Kerbel, 2005; Benedito et al., 2012; Chen et al., 2013). The VEGF and its receptor VEGFR have been reported to play crucial roles not only in physiological but in most pathological angiogenesis.
Vascular endothelial growth factors are important signaling molecules involved in both vasculogenesis and angiogenesis that are the two distinct processes by which new vascular network are formed during embryonic development (Drake et al., 2000). The vasculogenesis is a fundamental process of blood vessel system formation in the embryo, occurring by a De novo synthesis and differentiation of endothelial precursor cells into endothelial cells, and it is the first stage of the formation of the vascular network. The angiogenesis is a vital physiological process of growth of new capillaries through which the pre-existing vasculatures formed in the earlier stage of the vasculogenesis continue to grow, sprout, split, and grow (Drake et al., 2000). VEGF is crucial to ensure normal vascular morphogenesis, especial to increase the number of capillaries in angiogenesis. The embryos lacking a single VEGF allele exhibit abnormal vascular development and lethality (Carmeliet et al., 1996; Ferrara et al., 1996). The VEGF and its receptor VEGFR have been shown to play important roles in many angiogenic processes not only in normal physiological conditions but in most pathological conditions, such as embryonic development, axon growth, cancer, and inflammation (Hanahan and Folkman, 1996; Risau, 1997; Bellon et al., 2010). VEGF is a sub-family of the cystine-knot growth factor PDGF supergene family. All members of the VEGF family can stimulate cellular responses by binding to their tyrosine kinase receptors (VEGFRs) on the cell surface.
Currently, the human VEGF/VEGFR system is composed of VEGF-A (also VEGF), VEGF-B, VEGF-C, VEGF-D, and PGF (placental growth factor), three main VEGF receptors VEGFR-1 (Flt-1), VEGFR-2 (KDR), VEGFR-3 (Flt-4), and two non-protein kinase co-receptors neuropilin-1 and neuropilin-2 (NRP-1 and -2) (Rahimi, 2006; Shibuya, 2011). The VEGFR-1 and VEGFR-2 regulate angiogenesis and vascular permeability, and the VEGFR-3 mainly regulates lymphangiogenesis (Alitalo and Carmeliet, 2002). Among them, VEGFR-2 is mainly distributed in vascular endothelial cells and acts as major signal transducer for angiogenesis by PLCγ-PKC-MAPK, PLCγ-PKC-eNOS-NO, TSAd-Src-PI3K-Akt, SHB-FAK-paxillin, SHB-PI3K-Akt, and NCK-p38-MAPKAPK2/3 pathways (Wong and Jin, 2005; Shibuya, 2011). The VEGF/VEGFR system is an important target for anti-angiogenic therapy in cancer and for pro-angiogenic therapy in neuronal degeneration and ischemic diseases (Yang et al., 2018). Here, the molecular structures, physiological functions, and pathological roles of VEGFR-2 and its regulation mechanisms of signal transduction have been analyzed and reviewed.
Vascular endothelial growth factors are main regulators in vasculogenesis and angiogenesis and play their roles by binding to VEGFRs on cell surface and activating subsequently the signaling pathways of angiogenesis. The VEGFR-2 is the major receptor of VEGF, expresses in vascular endothelial cells, and plays a major role in angiogenesis (Bellon et al., 2010; Leppanen et al., 2010).
Human VEGFR-2, a kinase insert domain containing receptor (KDR) gene, is located at chromosome locus 4q11-12 and encodes 1356 amino acids of the full-length receptor (Park et al., 2018). Mature VEGFR-2 is a transmembrane glycoprotein with a molecular weight of 230 kD (Figure 1; Takahashi and Shibuya, 1997). The other two forms of VEGFR-2 are the non-glycosylated form with a molecular weight of 150 kD and the intermediate form with a molecular weight of 200 kD (Shen et al., 1998). Only the mature glycosylated form of VEGFR-2 (KDR) can achieve intracellular signal transduction (Shibuya, 2013). Mouse VEGFR-2 is also called fetal liver kinase 1 (Flk-1), which is composed of 1367 amino acids and has 83% homology with human KDR, and has three forms, molecular weight of 180, 200, and 220 kD, respectively.
Figure 1. The molecular structure of VEGF/VEGFR-2. (A) Diagram of the VEGFR-2 structure. VEGFR-2 is composed of a signal peptide, a extracellular domain (ECD) including seven Ig-like subdomains (IgD1∼7), a TMD, a JMD, a catalytic tyrosine kinas domain (TKD) including ATP binding domain (TKD1), kinase insert domain (KID) and phosphotransferase domain (TKD2), and a flexible C-terminal domain (CTD), and many functional sites. (B) VEGF-activated VEGFR-2 homodimer. After VEGFs binding to VEGFR-2, the crucial tyrosine residues on the TKD have been phosphorylated and are involved in mediating downstream signaling pathways. (C) Molecular structure of VEGF-A binding to IgD2 and IgD3 of VEGFR-2 [PDB ID: 3V2A (Brozzo et al., 2012)]. (D) Molecular structure of TKD of VEGFR-2 including TKD1 (N-lobe), KID, and TKD2 (C-lobe) [PDB ID: 4ASD (McTigue et al., 2012)]. There are three important motifs: glycine-rich loop (blue, 841–846 aa), catalytic loop (red, 1026–1033 aa), and activation loop (green, 1045–1075 aa), and three crucial phosphorylation sites (spheres) on the TKD: Tyr951 on the KID, and Tyr1054 and Try1059 on the TKD2.
VEGFR-2 is mainly distributed in vascular endothelial cells, lymphatic endothelial cells, and embryonic precursor cells, and can bind to VEGF-A, VEGF-C, and VEGF-D. By binding and activating VEGFR-2, VEGF mediates endothelial cell proliferation, invasion and migration, and survival, and increases vascular permeability and neovascularization (Holmes et al., 2007).
The full-length VEGFR-2 is composed of 1356 amino acids including a signal peptide (1∼19 aa) and a mature protein (20∼1356 aa). As a mature transmembrane protein, the VEGFR-2 is divided into extracellular domain (ECD, 20∼764 aa), transmembrane domain (TMD, 765∼789 aa), juxtamembrane domain (JMD, 790∼833 aa), catalytic tyrosine kinas domain (TKD, 834∼1162 aa) including ATP binding domain (TKD1, 834∼930 aa), kinase insert domain (KID, 931∼998 aa) and phosphotransferase domain (TKD2, 999∼1162 aa), and a flexible C-terminal domain (CTD, 1163∼1356 aa) (Table 1 and Figure 1).
The ECD is composed of 7 immunoglobulin-like subdomains, IgD1 (46∼110 aa), IgD2 (141∼207 aa), IgD3 (224∼320 aa), IgD4 (328∼414 aa), IgD5 (421∼548 aa), IgD6 (551∼660 aa), and IgD7 (667∼753 aa) (Figure 1A; Fuh et al., 1998). Investigation have showed that the IgD1 is involved in regulating the binding of receptors to ligands; while the IgD2 and IgD3 are required for tight binding to the dimeric VEGF and VEGF-induced VEGFR-2 dimerization and activation; the IgD2 and IgD4 can affect the binding rate of the ligand; the IgD5 and IgD6 may be involved in affect the receptor molecules unbinding from the ligands; and the IgD7 plays a crucial role in receptor dimerization and regulation (Figures 1B,C; Shinkai et al., 1998; Di Stasi et al., 2019).
The TKD is the most conserved region among VEGFRs (Park et al., 2018). This protein kinase core of the VEGFR-2 has a two-lobed spatial structure that forms the active center between the both lobes. At the N-terminus of the intracellular tyrosine kinas domain, there is a hydrophobic pocket containing a glycine-rich (GXGXXG, 841∼846 aa) ATP phosphate binding loop in the β-sheet structures. At the TKD C-terminal, there are several α-helical structures, including a catalytic loop (HRDLAARN, 1026∼1033 aa), and activation loop (A-loop, 1045∼1075 aa), which play important roles for VEGFR-2 catalytic properties (Tables 1, 2 and Figures 1A,D).
In the human VEGFR-2, there are 18 N-linked glycosylation sites, 15 phosphorylation sites, and many ATP binding sites and substrate binding sites, which play important roles in post-translational modifications of VEGFR-2, protein folding, protein activation, and cellular attachment, and can further modulate the function of VEGFR-2 (Croci et al., 2014; Chandler et al., 2019; Chung et al., 2019).
VEGFR-2 is activated by VEGF-A, -C, and -D binding to its Ig-like domains 2 and 3 (Figure 1C). Similar to other receptor tyrosine kinases (RTKs), cellular signaling mediated by VEGFR-2 is stimulated and initiated upon binding of its ligand dimer to the extracellular receptor Ig-like domains 2 and 3 (Ruch et al., 2007). This ligand-receptor interaction causes VEGFR-2 homo- and hetero-dimerization followed by phosphorylation of specific tyrosine residues located in the intracellular region including juxtamembrane domain, tyrosine kinas domain, and the carboxy-terminal domain. Subsequently, a variety of signaling molecules are recruited to VEGFR-2 dimers that activate downstream signaling pathways, and ultimately affect the physiological characteristics of endothelial cells and the entire vascular environment (Figure 2A; Fuh et al., 1998; Ruch et al., 2007; Ma et al., 2011).
Figure 2. VEGF/VEGFR-2 mediated signaling pathways during angiogenesis. (A) Diagram of VEGF-activated VEGFR-2 homodimer. Several crucial tyrosine residues have been phosphorylated after VEGFs binding to VEGFR-2. The Try801 on JMD is involved in cell permeability and proliferation by mediating the PLCγ-PKC, then eNOS-NO or MEK-ERK, respectively. The Try951 on KID mediates the cell survival and permeability via the TSAd-Src-PI3K-Akt pathway. The Try1054 and Try1059 on TKD2 can increase VEGFR-2 kinase activity. The Try1175 is involved in cell permeability, proliferation, and migration by regulating PLCγ-PKC, SHB-FAK-paxillin, and SHB-PI3K-Rac pathways. The Try1214 mediates cell migration through NCK-p38-MAPKAPK2/3 pathway. (B) Structure-based sequence alignment of PDGF-derived regions of the VEGF family members, includes human VEGF-A (NP_003367), human VEGF-B (NP_003368), human VEGF-C (NP_005420), human VEGF-D (NP_004460), human PGF (placental growth factor) (NP_002623), Orf virus orfVEGF (VEGF-E) (ABA00650), and Snake venom svVEGF (BAD38844), which are composed of two α-helix and five β-sheets. There are functional sites, including receptor binding site 1 (blue arrow) and site 2 (green arrows), cysteine knot motifs (red closed circles) that form three disulfide bridges including Cys263-Cys308, Cys267-Cys310, and Cys232-Cys274 in VEGF-A, and dimerization interface sites (orange stars).
The extracellular domain (ECD) of VEGFR-2 consists of several Ig-like subdomains, the linkers connecting these subdomains, and multiple N-linked glycosylation sites, which play important roles in formation of VEGF/VEGFR-2 system, in receptor dimerization after with ligand binding, and in maintain of the monomeric VEGFR-2 in the absence of ligand (Table 1). Each VEGF monomer is composed of two α-helix and five β-sheets that forms a central antiparallel beta sheet. In VEGF-A, a canonical cysteine knot formed by three intramolecular disulfide bridges Cys263-Cys308, Cys267-Cys310, and Cys232-Cys274, and two receptor binding sites and dimerization interface sites are critical for VEGF binding to VEGFR-2 and subsequently VEGFR-2 dimerization (Figure 2B).
In the ECD of VEGFRs, the ability to bind to its ligand is best studied at the biochemical and the structural level. For VEGFR-2, the ligands binding to VEGFR-2 required two Ig-like subdomains 2 and 3 (IgD2 and IgD3), and the stabilization of VEGF-bound VEGFR-2 dimers and VEGF-mediated VEGFR activity required Ig-like subdomains 4∼7 (IgD4∼7) (Li et al., 2000; Yang et al., 2010). As a N-glycosylated RTK, VEGFR-2 has 18 potential N-glycosylation sites in the seven Ig-like subdomains, which play a central role in RTK ligand binding, trafficking, stabilizing, and pro-angiogenic signaling in physiological and pathological contexts, including cancer (Table 2 and Figure 1; Croci et al., 2014; Chandler et al., 2017, 2019).
The transmembrane domain (TMD) and juxtamembrane domain (JMD) of VEGFR-2 have been shown to play pivotal roles in regulating VEGFR-2 kinase activity (Solowiej et al., 2009; Koch and Claesson-Welsh, 2012; Table 1).
Investigations on the role of the TMD in VEGFR-2 signaling showed that the activation of VEGFR-2 may depend on specific orientation of the receptor monomers in an active dimer resulting from VEGF-induced ECD rearrangement, and the TMD is involved in dimerization of the receptor monomers with specific orientations (Moriki et al., 2001; Bennasroune et al., 2004; Holmes et al., 2007; Manni et al., 2014b). The JMD of VEGFR-2 has the first phosphorylation site Y801, which is crucial for the autophosphorylation rate of VEGFR-2 (Solowiej et al., 2009). The unphosphorylated JMD autoinhibits kinase activity by interacting with the activation loop (A-loop) in the kinase domain 2 (TKD2). Therefore, the phosphorylated JMD at specific tyrosine residue Y801 may disrupt this interaction with the A-loop, promote reorientation of the activation loop, and induce an enzymatically active conformation (Table 2 and Figures 1A,B; Wybenga-Groot et al., 2001; Walter et al., 2007; Solowiej et al., 2009).
The activation of VEGFR-2 upon its VEGFs-mediated dimerization allows the TKD transphosphorylation, and then mediates cellular signaling, and regulates endothelial cell survival, proliferation, cell migration, and the vascular tube formation (Koch et al., 2011; Table 1). The VEGFR-2 TKD contains three subdomains, TKD1, an ATP binding domain, KID, the kinase insert domain, and TKD2, a phosphotransferase domain. The TKD is involved in VEGFR-2 activation and signaling by its multiple phosphorylation sites (Figures 1B,D).
In VEGFR-2 TKD, there are several major phosphorylation sites involved in cellular signaling mediated by TKD, including Tyr951 (Y951) in the KID and Try1054 (Y1054) and Try1059 (Y1059) in the TKD2, which is phosphorylated for the kinase activation (Takahashi et al., 2001; Matsumoto et al., 2005; Table 2). The Y951 site is phosphorylated on VEGFA stimulation, and the phosphorylated Y951 is indispensable for downstream signaling by the activated kinase (Matsumoto et al., 2005). The Y1054 and Y1059 sites are located in the TKD2 A-loop and play crucial role in kinase activity, whose autophosphorylation resulted by autophosphorylation at Y801 site increases the VEGFR-2 kinase activity (Figures 1B,D; Kendall et al., 1999; Solowiej et al., 2009).
The carboxyl terminus domain (CTD) is critical for VEGFR-2 activation and signaling. In VEGFR-2 CTD, there are two important autophosphorylation sites Try1175 (Y1175) and Try1214 (Y1214) (Table 1 and Figures 1B,D). After VEGF-A binding to VEGFR-2, activation of VEGFR-2 phosphorylates the Y1175 and Y1214 (Sase et al., 2009; Table 2). Then, the VEGF-activated VEGFR-2 bind to several signaling molecules such as PLCγ (Takahashi et al., 2001), PI3K (Kim et al., 2019), and adapter proteins SHB and SCK (Warner et al., 2000; Holmqvist et al., 2004) by the phosphorylated Try1175 site (pY1175), and adapter protein NCK (Lamalice et al., 2006) by the phosphorylated Try1214 site (pY1214), which directly activate VEGFR-2 to promote the proliferation, migration, and permeability of vascular endothelial cells (Figure 2A; Koch and Claesson-Welsh, 2012; Manni et al., 2014a).
The VEGF/VEGFR-2 signaling is essential for the development and maintenance of the organ-specific vascular systems and physiological function of many tissues and plays important roles in the pathogenesis of diseases such as cardiovascular disease and cancer. The VEGFR-2 has been proved to mediate various VEGF-stimulated cellular signal transduction including endothelial cell survival, proliferation, migration, and to enhance vascular permeability (Holmes et al., 2007; Siveen et al., 2017).
The VEGFR-2 plays crucial roles in vascular endothelial cell survival and blood vessel formation in vivo (Sase et al., 2009). VEGFR-2 regulates endothelial cell survival mainly by the activation of TSAd-Src-PI3K-PKB/AKT signaling pathway (Figure 2). Depending on VEGFR-2 and subsequent activated PI3K, VEGF-A regulates the survival of human umbilical vein endothelial cells. The PI3K can catalyze creation of PIP3 from PIP2, and then phosphorylate and activate protein kinase B (PKB) and Akt pathway (PKB/Akt pathway) (Downward, 2004). The Akt directly phosphorylates two types of apoptosis proteins: Bcl-2 associated death promoter (BAD) and caspase 9, and then inhibits their apoptotic activity to ensure cell survival (Lee et al., 2014).
VEGFR-2 plays a critical role in endothelial cell proliferation during angiogenesis. Through the VEGFR-2 on the cell membrane, the VEGF-A, a mitogen of many endothelial cells, can transmit extracellular signals to the cytoplasm and activate a series of downstream signaling pathways, and regulate the proliferation of endothelial cells. VEGFR-2 is mainly through the PLCγ-PKC-Raf-MEK-MAPK signaling pathway, and transmits the VEGF signal to the nucleus to activate DNA synthesis and promote the proliferation of endothelial cells (Takahashi et al., 1999; Figure 2). The VEGFR-2 can bind and activate phospholipase C-γ (PLCγ) by phosphorylation of the C-terminal Y1175 of VEGFR-2, and then phosphorylate the PLCγ and enhance its catalytic activity (Takahashi et al., 2001; Sase et al., 2009). The activated PLCγ hydrolyzes phosphatidylinositol (4, 5)-bisphosphate (PIP2) and then produces diacylglycerol (DAG) and inositol 1, 4, 5-trisphosphate (IP3), in which IP3 can increase the intracellular Ca2+ concentration, and DAG is a physiological activator of PKC. The ERK in the PKC-Raf-MEK-ERK signaling cascade is activated by the VEGF-activated VEGFR-2, enters the nucleus, and then binds to transcription factors that induce gene expression in response to extracellular stimuli. Finally, VEGFR-2 is involved in the endothelial cell proliferation (Holmes et al., 2007; Rask-Madsen and King, 2008).
The migration of endothelial cells is crucial for angiogenesis. A variety of signal pathways mediated by VEGFR-2 are related to the migration of endothelial cells. VEGFR-2 has been proved to regulate cell migration by activating SHB, NCK, and PI3K mediated pathway via the both phosphorylation sites Y1175 and Y1214 on VEGFR-2 CTD (Laramee et al., 2007; Graupera et al., 2008). The phosphorylated Tyr1175 (pY1175) site at the CTD of VEGFR-2 can bind the Src homology domain-2 (SH2) of the adaptor protein SHB and regulate cell migration caused by VEGF (Holmqvist et al., 2004; Figure 2). The downregulated expression of SHB can prevent VEGF/VEGFR-2-mediated cell scaffold reorganization, migration, and PI3K activation (Masoumi Moghaddam et al., 2012; Park et al., 2018). The phosphorylated Tyr951 (pY951) site at the TKD of VEGFR-2 can bind T-cell-specific adapter (TSAd) in the vascular endothelial cells of tumor tissue (Matsumoto et al., 2005), activate formation of TSAd and Src complex, and subsequently regulate cell migration. Site-directed mutation of Tyr951 on VEGFR-2 can inhibit VEGF-mediated cytoskeletal reorganization and migration (Cebe-Suarez et al., 2006). While the phosphorylated Tyr1214 (pY1214) site at the C-terminus domain of VEGFR-2 is involved in the remodeling of actin by VEGFR-2 mediated NCK/Src-p21/Cdc42-SAPK2/p38-MAPK pathway (Lamalice et al., 2004, 2006).
The VEGF-A acts as a vascular permeability factor and its signal can activate endothelial nitric oxide synthase (eNOS) to create NO, and then change vascular permeability (Holmes et al., 2007). VEGF-activated VEGFR-2 can stimulate vascular endothelial cells to release NO, in which the phosphorylation of the Try801 (pY801) residue at the JMD of VEGFR-2 is necessary for the release of NO induced by VEGF, and the pY801 activates eNOS mainly by the PKC-PI3K/Akt pathway (Blanes et al., 2007; Figure 2). VEGFR-2 mediated signals can promote eNOS binding to its molecular chaperone heat shock protein 90 (Hsp90), and then enhance the release of NO by endothelial cells (Duval et al., 2007). VEGFR-2 can also mediate effect of lowering blood pressure via increased permeability of blood vessels and relaxed of blood vessels caused by VEGF-A (Gliki et al., 2001).
In organism, many angiogenic proteins are involved in the stimulation of angiogenesis including EphrinB2/EphB4 (Groppa et al., 2018), fibroblast growth factors (FGFs) (Maddaluno et al., 2017), VEGFs/VEGFR-2 (Basagiannis et al., 2016), angiopoietin/Tie receptors (Zhang et al., 2019), and platelet-derived growth factors (PDGFs/PDGFRs) (Zhang et al., 2009; Manzat Saplacan et al., 2017). Among the angiogenic proteins, VEGFs/VEGFR-2 is a crucial regulator of physiological vasculogenesis and angiogenesis in early embryonic and adult stages and pathological angiogenesis in tumorigenesis.
VEGFR-2 mediates the main physiological functions of VEGF. VEGF, the vascular permeability factor (VPF), is an essential and crucial growth factor for vascular endothelial cells, and plays various roles in cardiovascular system (Wang et al., 2019), central nervous system (Bellon et al., 2010; Luck et al., 2019), hematopoiesis (Hooper et al., 2009), development (Karaman et al., 2018), and tumorigenesis (Volz et al., 2020; Zhong et al., 2020). Despite having so many physiological functions, the main physiological functions of VEGF on endothelial cells are almost all achieved by activating VEGFR-2, including stimulating endothelial cell proliferation, increasing vascular permeability, and chemotaxis to endothelial cells, etc. Investigations showed that VEGFR-2 is the main receptor to mediate the increase in vascular permeability by VEGF (Koch and Claesson-Welsh, 2012; Smith et al., 2020). VEGFR-2 is required for VEGF-mediated signaling and regulating. The phosphorylation of VEGFR-2 can be found on the surface of whole cell. Several important phosphorylation sites, Try951, Try1054, Try1059, and Try1175 had been proved to be involved in this process by binding SHB (Holmqvist et al., 2004; Smith et al., 2020), SCK (Warner et al., 2000), PLCγ (Takahashi et al., 2001), PI3K (Blanes et al., 2007), and TASD (Matsumoto et al., 2005) and activating their respective signal pathway.
VEGFR-2 plays a very important role in embryonic development. VEGF-activated VEGFR-2 stimulates endothelial cell proliferation and is crucial to the development of the embryonic vascular system and hematopoietic system (Hooper et al., 2009; Han et al., 2018). During embryonic development, the VEGFR-2 signaling pathway is involved in the proliferation, growth, and migration of hematopoietic and early endothelial cells (angioblasts) (Dias et al., 2000; LeCouter et al., 2004). Several studies showed that the VEGF/VEGFR-2 signaling directly regulates the development and function of neurons, e.g., increased axon branching (Luck et al., 2019).
Though the VEGF/VEGFR-2 system plays important functions in normal physiological condition, de-regulation of the VEGF/VEGFR-2 implicates directly in various diseases, and dysfunctional VEGFR-2 can cause developmental disorders of the vascular system and hematopoietic system during embryonic development (Shibuya, 2001; Zeng et al., 2001).
VEGF-activated VEGFR-2 plays important roles in mediating the formation of new blood vessels under various pathological conditions and processes, including wound healing, rheumatoid arthritis (RA), diabetic retinopathy (DR), Alzheimer’s disease (AD), small vessel disease, coronary heart disease (CHD), and cancer owing to its complicated molecular and structural characteristics. The VEGFA/VEGFR-2 signal transduction leads to endothelial cell proliferation, migration, survival and new vessel formation involved in angiogenesis, and has been implicated in pathogenesis of several diseases, e.g., inflammation, cancers, ophthalmic diseases, and neurological diseases (Louveau et al., 2015; Ferrara and Adamis, 2016; Ma et al., 2017).
VEGFR-2 is required for cardiovascular system diseases. Investigations showed that VEGFR-2-deficient mice die in early embryo for an early defect in the development of hematopoietic and endothelial cells (Shalaby et al., 1995; Carmeliet et al., 1996; Ferrara et al., 1996). VEGFR-2 is also involved in tumor angiogenesis and lymphatic development via recruiting endothelial cells (Dumont et al., 1998; Rafii et al., 2002). Studies have reported that the expression of Ets-1 and Flk-1 (mouse VEGFR-2) are highly correlated in angiogenesis and tumor angiogenesis, and are involved in stem cell leukemia (Elvert et al., 2003).
VEGFR-2 is an important target of anti-tumor angiogenesis. VEGF secreted by tumor cells activates its receptor VEGFR-2, and they subsequently promote vascular growth and supply the oxygen and nutrition into the hypoxic areas of tumor tissues (Lugano et al., 2020). VEGF-activated VEGFR-2 mediate the phosphorylation of many proteins in the downstream signaling pathways, e.g., Akt (protein kinase B), mTOR (mammalian target of rapamycin), Erk1/2 (extracellular signal-regulated kinase 1/2), FAK (focal adhesion kinase), and p70S6K (ribosomal protein S6 kinase), and promotes tumor angiogenesis (Karar and Maity, 2011; Lechertier and Hodivala-Dilke, 2012; Ji et al., 2018). Therefore, VEGFR-2 functions as an important target for anti-tumor therapy (Weis and Cheresh, 2011; Fallah et al., 2019).
Vascular endothelial growth factors, as the key regulators, are involved in vasculogenesis, angiogenesis, and hematopoiesis during development. Thay play their roles by binding to and activating their tyrosine kinase receptors (VEGFRs) on the cell surface. In VEGF/VEGFR-2 system, the VEGF binding to the Ig-like subdomains 2 and 3 (IgD2 and IgD3) of VEGFR-2 induces the dimerization of the receptor, the activation and trans-autophosphorylation of the tyrosine kinase, and then the initiation of the intracellular signaling cascades, finally is involved in proliferation, survival, migration, and permeability of vascular endothelial cells.
The interaction of VEGF and VEGFR-2 is crucial for the dimerization of VEGFR-2. In endothelial cell, the signaling by VEGFR-2 requires VEGF-mediated dimerization with precise positioning of VEGFR-2 subunits in active dimers. In VEGF-A, there are a canonical cysteine knot that consists of three intramolecular disulfide bridges Cys263-Cys308, Cys267-Cys310, and Cys232-Cys274, and two receptor binding sites that bind to each VEGFR-2 of the dimer and dimerization interface sites, which are critical for the ligand binding to VEGFR-2. In VGEFR2 Ig-like subdomains, the IgD2 and IgD3 are involved in the VEGF binding to its receptor. The dimeric VEGF/VEGFR-2 complexes subsequently induce the dimerization of the receptor.
The dimerization of VEGFAR-2 is required for activation of VEGFR-2. The dimeric VEGF ligands binding to diffusing monomeric VEGFR-2 promotes dimerization of the later, and then stimulates the activation of VEGFR-2. It is reported that ligand-induced VEGFR-2 phosphorylation is increased as much as 10-fold compared to the phosphorylation in the absence of ligand (Sarabipour et al., 2016). In the active homotypic VEGFR-2-VEGFR-2, the IgD4∼7 were proved to be critical for efficient phosphorylation of the VEGFR-2 in the presence of VEGF, and the TMD dimer upon ligand binding increases VEGFR-2 phosphorylation and stabilizes the VEGFR-2 dimers, indicating that VEGF binding and VEGFR-2 dimerization are required for its activation.
The VEGFR-2-mediated intracellular signaling are involved in cell survival, proliferation, migration, and vascular permeability. VEGFR-2 is the primary mediator of the physiological effects of VEGF in angiogenesis. The VEGF binding to VEGFR-2 induces autophosphorylation of specific tyrosine residues in the cytoplasmic domain of VEGFR-2, including the Try801 on JMD involved in cell permeability and proliferation by mediating the PLCγ-PKC, then eNOS-NO or MEK-ERK, respectively, the Try951 on KID mediating the cell survival and permeability via the TSAd-Src-PI3K-Akt pathway, the Try1054 and Try1059 on TKD2 increasing VEGFR-2 kinase activity, the Try1175 involved in cell permeability, proliferation, and migration by regulating PLCγ-PKC, SHB-FAK-paxillin, and SHB-PI3K-Rac pathways, and the Try1214 mediates cell migration through NCK-p38-MAPKAPK2/3 pathway. These signaling networks mediated by VEGF/VEGFR-2 are involved in regulating the process of angiogenesis, and controlling endothelial cell survival, proliferation and motility, and vascular fenestration and permeabilization.
The VEGFR-2-targeted therapy strategies will contribution to clinical treatment of disease. So far, the in-depth study of the structure and signal transduction of VEGFR-2 has made the mechanism of related pathogenesis further elucidated and effectively treated. VEGFR-2-targeted therapy strategies have been widely explored and applied clinically in cancer treatment. VEGF/VEGFR-2 signal transduction pathway is used in anti-tumor angiogenesis. VEGF/VEGFR-2 system is involved in various pathological conditions and processes, especially in tumorigenesis, owing to its complicated molecular structure and signal transduction, indicating these diseases can be considered to employ the strategy of the VEGFR-2-targeted therapy. The tumor microenvironment stimulates the specific expression of VEGF and VEGFR-2 in tumor cells and endothelial cells around the tumor, making the expression of VEGF and VEGFR-2 significantly higher than in normal tissues, indicating that inhibiting tumor angiogenesis by blocking the VEGF/VEGFR-2 signaling pathway may be an effective anti-cancer treatment strategy, including (a) inhibiting VEGF and VEGFR-2 gene expression level by antisense oligonucleotides (ASOs), RNA interference (RNAi), and ribozyme (Rz) (Marchand et al., 2002); (b) blocking the binding of VEGF and VEGFR-2 in protein level through neutralizing antibodies (nAbs), soluble VEGFR-2 (sVEGFR-2), and small molecule inhibitor VEGF/VEGFR-2 tyrosine kinase signaling pathway (Jain et al., 2006); and (c) destroying vascular endothelial cells through directed therapy by VEGF combined with small molecule toxic substances or VEGFR-2 monoclonal antibody (mAb) cross-linked with drugs and killing or inhibiting the growth of vascular endothelial cells expressing VEGFR-2 (Stopeck et al., 2002).
XW, AMB, GS, and BM contributed to manuscript writing and language improving. BM and XW designed and made the figures and wrote and edited the review. All authors contributed to the article and approved the submitted version.
The study was funded by Discipline Construction Fund Project of Gansu Agricultural University (GAU-XKJS-2018-037)
The authors declare that the research was conducted in the absence of any commercial or financial relationships that could be construed as a potential conflict of interest.
Alitalo, K., and Carmeliet, P. (2002). Molecular mechanisms of lymphangiogenesis in health and disease. Cancer Cell 1, 219–227. doi: 10.1016/s1535-6108(02)00051-x
Alkim, C., Alkim, H., Koksal, A. R., Boga, S., and Sen, I. (2015). Angiogenesis in Inflammatory Bowel Disease. Int. J. Inflam. 2015:970890.
Basagiannis, D., Zografou, S., Murphy, C., Fotsis, T., Morbidelli, L., Ziche, M., et al. (2016). VEGF induces signalling and angiogenesis by directing VEGFR2 internalisation through macropinocytosis. J. Cell Sci. 129, 4091–4104.
Bellon, A., Luchino, J., Haigh, K., Rougon, G., Haigh, J., Chauvet, S., et al. (2010). VEGFR2 (KDR/Flk1) signaling mediates axon growth in response to semaphorin 3E in the developing brain. Neuron 66, 205–219. doi: 10.1016/j.neuron.2010.04.006
Benedito, R., Rocha, S. F., Woeste, M., Zamykal, M., Radtke, F., Casanovas, O., et al. (2012). Notch-dependent VEGFR3 upregulation allows angiogenesis without VEGF-VEGFR2 signalling. Nature 484, 110–114. doi: 10.1038/nature10908
Bennasroune, A., Fickova, M., Gardin, A., Dirrig-Grosch, S., Aunis, D., Cremel, G., et al. (2004). Transmembrane peptides as inhibitors of ErbB receptor signaling. Mol. Biol. Cell 15, 3464–3474. doi: 10.1091/mbc.e03-10-0753
Blanes, M. G., Oubaha, M., Rautureau, Y., and Gratton, J. P. (2007). Phosphorylation of tyrosine 801 of vascular endothelial growth factor receptor-2 is necessary for Akt-dependent endothelial nitric-oxide synthase activation and nitric oxide release from endothelial cells. J. Biol. Chem. 282, 10660–10669. doi: 10.1074/jbc.m609048200
Brozzo, M. S., Bjelic, S., Kisko, K., Schleier, T., Leppanen, V. M., Alitalo, K., et al. (2012). Thermodynamic and structural description of allosterically regulated VEGFR-2 dimerization. Blood 119, 1781–1788. doi: 10.1182/blood-2011-11-390922
Carmeliet, P., Ferreira, V., Breier, G., Pollefeyt, S., Kieckens, L., Gertsenstein, M., et al. (1996). Abnormal blood vessel development and lethality in embryos lacking a single VEGF allele. Nature 380, 435–439. doi: 10.1038/380435a0
Cebe-Suarez, S., Zehnder-Fjallman, A., and Ballmer-Hofer, K. (2006). The role of VEGF receptors in angiogenesis; complex partnerships. Cell Mol. Life Sci. 63, 601–615. doi: 10.1007/s00018-005-5426-3
Chandler, K. B., Leon, D. R., Kuang, J., Meyer, R. D., Rahimi, N., and Costello, C. E. (2019). N-Glycosylation regulates ligand-dependent activation and signaling of vascular endothelial growth factor receptor 2 (VEGFR2). J. Biol. Chem. 294, 13117–13130. doi: 10.1074/jbc.ra119.008643
Chandler, K. B., Leon, D. R., Meyer, R. D., Rahimi, N., and Costello, C. E. (2017). Site-Specific N-glycosylation of endothelial cell receptor tyrosine kinase VEGFR-2. J Proteome Res 16, 677–688. doi: 10.1021/acs.jproteome.6b00738
Chen, X., Yang, G., Song, J. H., Xu, H., Li, D., Goldsmith, J., et al. (2013). Probiotic yeast inhibits VEGFR signaling and angiogenesis in intestinal inflammation. PLoS One 8:e64227. doi: 10.1371/journal.pone.0064227
Cheng, R., and Ma, J. X. (2015). Angiogenesis in diabetes and obesity. Rev. Endocr. Metab. Disord. 16, 67–75.
Chung, T. W., Kim, E. Y., Choi, H. J., Han, C. W., Jang, S. B., Kim, K. J., et al. (2019). 6’-Sialylgalactose inhibits vascular endothelial growth factor receptor 2-mediated angiogenesis. Exp. Mol. Med. 51, 1–13. doi: 10.1038/s12276-019-0311-6
Crawford, T. N., Alfaro, D. V. III, Kerrison, J. B., and Jablon, E. P. (2009). Diabetic retinopathy and angiogenesis. Curr. Diabetes Rev. 5, 8–13.
Croci, D. O., Cerliani, J. P., Dalotto-Moreno, T., Mendez-Huergo, S. P., Mascanfroni, I. D., Dergan-Dylon, S., et al. (2014). Glycosylation-dependent lectin-receptor interactions preserve angiogenesis in anti-VEGF refractory tumors. Cell 156, 744–758. doi: 10.1016/j.cell.2014.01.043
Di Stasi, R., Diana, D., De Rosa, L., Fattorusso, R., and D’Andrea, L. D. (2019). Biochemical and conformational characterization of recombinant VEGFR2 domain 7. Mol. Biotechnol. 61, 860–872. doi: 10.1007/s12033-019-00211-4
Dias, S., Hattori, K., Zhu, Z., Heissig, B., Choy, M., Lane, W., et al. (2000). Autocrine stimulation of VEGFR-2 activates human leukemic cell growth and migration. J. Clin. Invest. 106, 511–521. doi: 10.1172/jci8978
Downward, J. (2004). PI 3-kinase. Akt and cell survival. Semin. Cell Dev. Biol. 15, 177–182. doi: 10.1016/j.semcdb.2004.01.002
Drake, C. J., LaRue, A., Ferrara, N., and Little, C. D. (2000). VEGF regulates cell behavior during vasculogenesis. Dev. Biol. 224, 178–188. doi: 10.1006/dbio.2000.9744
Dumont, D. J., Jussila, L., Taipale, J., Lymboussaki, A., Mustonen, T., Pajusola, K., et al. (1998). Cardiovascular failure in mouse embryos deficient in VEGF receptor-3. Science 282, 946–949. doi: 10.1126/science.282.5390.946
Duval, M., Le Boeuf, F., Huot, J., and Gratton, J. P. (2007). Src-mediated phosphorylation of Hsp90 in response to vascular endothelial growth factor (VEGF) is required for VEGF receptor-2 signaling to endothelial NO synthase. Mol. Biol. Cell 18, 4659–4668. doi: 10.1091/mbc.e07-05-0467
Elvert, G., Kappel, A., Heidenreich, R., Englmeier, U., Lanz, S., Acker, T., et al. (2003). Cooperative interaction of hypoxia-inducible factor-2alpha (HIF-2alpha) and Ets-1 in the transcriptional activation of vascular endothelial growth factor receptor-2 (Flk-1). J. Biol. Chem. 278, 7520–7530. doi: 10.1074/jbc.m211298200
Fallah, A., Sadeghinia, A., Kahroba, H., Samadi, A., Heidari, H. R., Bradaran, B., et al. (2019). Therapeutic targeting of angiogenesis molecular pathways in angiogenesis-dependent diseases. Biomed. Pharmacother. 110, 775–785. doi: 10.1016/j.biopha.2018.12.022
Ferrara, N., and Adamis, A. P. (2016). Ten years of anti-vascular endothelial growth factor therapy. Nat. Rev. Drug Discov. 15, 385–403. doi: 10.1038/nrd.2015.17
Ferrara, N., Carver-Moore, K., Chen, H., Dowd, M., Lu, L., O’Shea, K. S., et al. (1996). Heterozygous embryonic lethality induced by targeted inactivation of the VEGF gene. Nature 380, 439–442. doi: 10.1038/380439a0
Ferrari, G., Cook, B. D., Terushkin, V., Pintucci, G., and Mignatti, P. (2009). Transforming growth factor-beta 1 (TGF-beta1) induces angiogenesis through vascular endothelial growth factor (VEGF)-mediated apoptosis. J. Cell Physiol. 219, 449–458. doi: 10.1002/jcp.21706
Fuh, G., Li, B., Crowley, C., Cunningham, B., and Wells, J. A. (1998). Requirements for binding and signaling of the kinase domain receptor for vascular endothelial growth factor. J. Biol. Chem. 273, 11197–11204. doi: 10.1074/jbc.273.18.11197
Gliki, G., Abu-Ghazaleh, R., Jezequel, S., Wheeler-Jones, C., and Zachary, I. (2001). Vascular endothelial growth factor-induced prostacyclin production is mediated by a protein kinase C (PKC)-dependent activation of extracellular signal-regulated protein kinases 1 and 2 involving PKC-delta and by mobilization of intracellular Ca2+. Biochem. J. 353, 503–512. doi: 10.1042/0264-6021:3530503
Gopinathan, G., Milagre, C., Pearce, O. M., Reynolds, L. E., Hodivala-Dilke, K., Leinster, D. A., et al. (2015). Interleukin-6 stimulates defective angiogenesis. Cancer Res. 75, 3098–3107. doi: 10.1158/0008-5472.can-15-1227
Graupera, M., Guillermet-Guibert, J., Foukas, L. C., Phng, L. K., Cain, R. J., Salpekar, A., et al. (2008). Angiogenesis selectively requires the p110alpha isoform of PI3K to control endothelial cell migration. Nature 453, 662–666. doi: 10.1038/nature06892
Groppa, E., Brkic, S., Uccelli, A., Wirth, G., Korpisalo-Pirinen, P., Filippova, M., et al. (2018). EphrinB2/EphB4 signaling regulates non-sprouting angiogenesis by VEGF. EMBO Rep. 19:e45054.
Han, S., Tan, C., Ding, J., Wang, J., Ma’ayan, A., and Gouon-Evans, V. (2018). Endothelial cells instruct liver specification of embryonic stem cell-derived endoderm through endothelial VEGFR2 signaling and endoderm epigenetic modifications. Stem. Cell Res. 30, 163–170. doi: 10.1016/j.scr.2018.06.004
Hanahan, D., and Folkman, J. (1996). Patterns and emerging mechanisms of the angiogenic switch during tumorigenesis. Cell 86, 353–364. doi: 10.1016/s0092-8674(00)80108-7
Hoeben, A., Landuyt, B., Highley, M. S., Wildiers, H., Van Oosterom, A. T., and De Bruijn, E. A. (2004). Vascular endothelial growth factor and angiogenesis. Pharmacol. Rev. 56, 549–580.
Holmes, K., Roberts, O. L., Thomas, A. M., and Cross, M. J. (2007). Vascular endothelial growth factor receptor-2: structure, function, intracellular signalling and therapeutic inhibition. Cell Signal. 19, 2003–2012. doi: 10.1016/j.cellsig.2007.05.013
Holmqvist, K., Cross, M. J., Rolny, C., Hagerkvist, R., Rahimi, N., Matsumoto, T., et al. (2004). The adaptor protein shb binds to tyrosine 1175 in vascular endothelial growth factor (VEGF) receptor-2 and regulates VEGF-dependent cellular migration. J. Biol. Chem. 279, 22267–22275. doi: 10.1074/jbc.m312729200
Hooper, A. T., Butler, J. M., Nolan, D. J., Kranz, A., Iida, K., Kobayashi, M., et al. (2009). Engraftment and reconstitution of hematopoiesis is dependent on VEGFR2-mediated regeneration of sinusoidal endothelial cells. Cell Stem. Cell 4, 263–274. doi: 10.1016/j.stem.2009.01.006
Ivkovic, S., Yoon, B. S., Popoff, S. N., Safadi, F. F., Libuda, D. E., Stephenson, R. C., et al. (2003). Connective tissue growth factor coordinates chondrogenesis and angiogenesis during skeletal development. Development 130, 2779–2791. doi: 10.1242/dev.00505
Iwasaki, A., Kuwahara, M., Yoshinaga, Y., and Shirakusa, T. (2004). Basic fibroblast growth factor (bFGF) and vascular endothelial growth factor (VEGF) levels, as prognostic indicators in NSCLC. Eur. J. Cardiothorac. Surg. 25, 443–448. doi: 10.1016/j.ejcts.2003.11.031
Jain, R. K., Duda, D. G., Clark, J. W., and Loeffler, J. S. (2006). Lessons from phase III clinical trials on anti-VEGF therapy for cancer. Nat. Clin. Pract. Oncol. 3, 24–40. doi: 10.1038/ncponc0403
Ji, L., Wu, M., and Li, Z. (2018). Rutacecarpine inhibits angiogenesis by targeting the VEGFR2 and VEGFR2-Mediated Akt/mTOR/p70s6k Signaling Pathway. Molecules 23:2047. doi: 10.3390/molecules23082047
Kaga, T., Kawano, H., Sakaguchi, M., Nakazawa, T., Taniyama, Y., and Morishita, R. (2012). Hepatocyte growth factor stimulated angiogenesis without inflammation: differential actions between hepatocyte growth factor, vascular endothelial growth factor and basic fibroblast growth factor. Vascul. Pharmacol. 57, 3–9. doi: 10.1016/j.vph.2012.02.002
Karaman, S., Leppanen, V. M., and Alitalo, K. (2018). Vascular endothelial growth factor signaling in development and disease. Development 145:dev151019. doi: 10.1242/dev.151019
Karar, J., and Maity, A. (2011). PI3K/AKT/mTOR Pathway in Angiogenesis. Front. Mol. Neurosci. 4:51. doi: 10.3389/fnmol.2011.00051
Kendall, R. L., Rutledge, R. Z., Mao, X., Tebben, A. J., Hungate, R. W., and Thomas, K. A. (1999). Vascular endothelial growth factor receptor KDR tyrosine kinase activity is increased by autophosphorylation of two activation loop tyrosine residues. J. Biol. Chem. 274, 6453–6460. doi: 10.1074/jbc.274.10.6453
Kim, D. Y., Park, J. A., Kim, Y., Noh, M., Park, S., Lie, E., et al. (2019). SALM4 regulates angiogenic functions in endothelial cells through VEGFR2 phosphorylation at Tyr1175. FASEB J. 33, 9842–9857. doi: 10.1096/fj.201802516rr
Klagsbrun, M., and Eichmann, A. (2005). A role for axon guidance receptors and ligands in blood vessel development and tumor angiogenesis. Cytokine Growth Factor. Rev. 16, 535–548. doi: 10.1016/j.cytogfr.2005.05.002
Koch, S., and Claesson-Welsh, L. (2012). Signal transduction by vascular endothelial growth factor receptors. Cold Spring Harb. Perspect. Med. 2:a006502. doi: 10.1101/cshperspect.a006502
Koch, S., Tugues, S., Li, X., Gualandi, L., and Claesson-Welsh, L. (2011). Signal transduction by vascular endothelial growth factor receptors. Biochem. J. 437, 169–183. doi: 10.1042/bj20110301
Lamalice, L., Houle, F., and Huot, J. (2006). Phosphorylation of Tyr1214 within VEGFR-2 triggers the recruitment of Nck and activation of Fyn leading to SAPK2/p38 activation and endothelial cell migration in response to VEGF. J. Biol. Chem. 281, 34009–34020. doi: 10.1074/jbc.m603928200
Lamalice, L., Houle, F., Jourdan, G., and Huot, J. (2004). Phosphorylation of tyrosine 1214 on VEGFR2 is required for VEGF-induced activation of Cdc42 upstream of SAPK2/p38. Oncogene 23, 434–445. doi: 10.1038/sj.onc.1207034
Laramee, M., Chabot, C., Cloutier, M., Stenne, R., Holgado-Madruga, M., Wong, A. J., et al. (2007). The scaffolding adapter Gab1 mediates vascular endothelial growth factor signaling and is required for endothelial cell migration and capillary formation. J. Biol. Chem. 282, 7758–7769. doi: 10.1074/jbc.m611327200
Lechertier, T., and Hodivala-Dilke, K. (2012). Focal adhesion kinase and tumour angiogenesis. J. Pathol. 226, 404–412. doi: 10.1002/path.3018
LeCouter, J., Zlot, C., Tejada, M., Peale, F., and Ferrara, N. (2004). Bv8 and endocrine gland-derived vascular endothelial growth factor stimulate hematopoiesis and hematopoietic cell mobilization. Proc. Natl. Acad. Sci. U.S.A. 101, 16813–16818. doi: 10.1073/pnas.0407697101
Lee, M. Y., Luciano, A. K., Ackah, E., Rodriguez-Vita, J., Bancroft, T. A., Eichmann, A., et al. (2014). Endothelial Akt1 mediates angiogenesis by phosphorylating multiple angiogenic substrates. Proc. Natl. Acad. Sci. U.S.A. 111, 12865–12870. doi: 10.1073/pnas.1408472111
Leppanen, V. M., Prota, A. E., Jeltsch, M., Anisimov, A., Kalkkinen, N., Strandin, T., et al. (2010). Structural determinants of growth factor binding and specificity by VEGF receptor 2. Proc. Natl. Acad. Sci. U.S.A. 107, 2425–2430. doi: 10.1073/pnas.0914318107
Li, B., Fuh, G., Meng, G., Xin, X., Gerritsen, M. E., Cunningham, B., et al. (2000). Receptor-selective variants of human vascular endothelial growth factor. Generation and characterization. J. Biol. Chem. 275, 29823–29828. doi: 10.1074/jbc.m002015200
Li, X., He, S., and Ma, B. (2020). Autophagy and autophagy-related proteins in cancer. Mol. Cancer 19:12.
Lindahl, P., Bostrom, H., Karlsson, L., Hellstrom, M., Kalen, M., and Betsholtz, C. (1999). Role of platelet-derived growth factors in angiogenesis and alveogenesis. Curr. Top. Pathol. 93, 27–33. doi: 10.1007/978-3-642-58456-5_4
Louveau, A., Smirnov, I., Keyes, T. J., Eccles, J. D., Rouhani, S. J., Peske, J. D., et al. (2015). Structural and functional features of central nervous system lymphatic vessels. Nature 523, 337. doi: 10.1038/nature14432
Luck, R., Urban, S., Karakatsani, A., Harde, E., Sambandan, S., Nicholson, L., et al. (2019). VEGF/VEGFR2 signaling regulates hippocampal axon branching during development. eLife 8:e49818.
Lugano, R., Ramachandran, M., and Dimberg, A. (2020). Tumor angiogenesis: causes, consequences, challenges and opportunities. Cell Mol. Life Sci. 77, 1745–1770. doi: 10.1007/s00018-019-03351-7
Ma, B., Xiang, Y., and An, L. (2011). Structural bases of physiological functions and roles of the vacuolar H(+)-ATPase. Cell Signal 23, 1244–1256. doi: 10.1016/j.cellsig.2011.03.003
Ma, Q. L., Ineichen, B. V., Detmar, M., and Proulx, S. T. (2017). Outflow of cerebrospinal fluid is predominantly through lymphatic vessels and is reduced in aged mice. Nat. Commun. 8:1434.
Maddaluno, L., Urwyler, C., and Werner, S. (2017). Fibroblast growth factors: key players in regeneration and tissue repair. Development 144, 4047–4060. doi: 10.1242/dev.152587
Manni, S., Kisko, K., Schleier, T., Missimer, J., and Ballmer-Hofer, K. (2014a). Functional and structural characterization of the kinase insert and the carboxy terminal domain in VEGF receptor 2 activation. FASEB J. 28, 4914–4923. doi: 10.1096/fj.14-256206
Manni, S., Mineev, K. S., Usmanova, D., Lyukmanova, E. N., Shulepko, M. A., Kirpichnikov, M. P., et al. (2014b). Structural and functional characterization of alternative transmembrane domain conformations in VEGF receptor 2 activation. Structure 22, 1077–1089. doi: 10.1016/j.str.2014.05.010
Manzat Saplacan, R. M., Balacescu, L., Gherman, C., Chira, R. I., Craiu, A., Mircea, P. A., et al. (2017). The role of PDGFs and PDGFRs in colorectal cancer. Mediat. Inflamm. 2017, 4708076.
Marchand, G. S., Noiseux, N., Tanguay, J. F., and Sirois, M. G. (2002). Blockade of in vivo VEGF-mediated angiogenesis by antisense gene therapy: role of Flk-1 and Flt-1 receptors. Am. J. Physiol. Heart Circ. Physiol. 282, H194–H204.
Marrelli, A., Cipriani, P., Liakouli, V., Carubbi, F., Perricone, C., Perricone, R., et al. (2011). Angiogenesis in rheumatoid arthritis: a disease specific process or a common response to chronic inflammation? Autoimmun. Rev. 10, 595–598. doi: 10.1016/j.autrev.2011.04.020
Masoumi Moghaddam, S., Amini, A., Morris, D. L., and Pourgholami, M. H. (2012). Significance of vascular endothelial growth factor in growth and peritoneal dissemination of ovarian cancer. Cancer Metastasis Rev. 31, 143–162. doi: 10.1007/s10555-011-9337-5
Matsumoto, T., Bohman, S., Dixelius, J., Berge, T., Dimberg, A., Magnusson, P., et al. (2005). VEGF receptor-2 Y951 signaling and a role for the adapter molecule TSAd in tumor angiogenesis. EMBO J. 24, 2342–2353. doi: 10.1038/sj.emboj.7600709
McTigue, M., Murray, B. W., Chen, J. H., Deng, Y. L., Solowiej, J., and Kania, R. S. (2012). Molecular conformations, interactions, and properties associated with drug efficiency and clinical performance among VEGFR TK inhibitors. Proc. Natl. Acad. Sci. U.S.A. 109, 18281–18289. doi: 10.1073/pnas.1207759109
Moriki, T., Maruyama, H., and Maruyama, I. N. (2001). Activation of preformed EGF receptor dimers by ligand-induced rotation of the transmembrane domain. J. Mol. Biol. 311, 1011–1026. doi: 10.1006/jmbi.2001.4923
Nilsson, M., and Heymach, J. V. (2006). Vascular endothelial growth factor (VEGF) pathway. J. Thorac. Oncol. 1, 768–770. doi: 10.1097/01243894-200610000-00003
Park, S. A., Jeong, M. S., Ha, K. T., and Jang, S. B. (2018). Structure and function of vascular endothelial growth factor and its receptor system. BMB Rep. 51, 73–78. doi: 10.5483/bmbrep.2018.51.2.233
Rafii, S., Meeus, S., Dias, S., Hattori, K., Heissig, B., Shmelkov, S., et al. (2002). Contribution of marrow-derived progenitors to vascular and cardiac regeneration. Semin. Cell Dev. Biol. 13, 61–67. doi: 10.1006/scdb.2001.0285
Rahimi, N. (2006). VEGFR-1 and VEGFR-2: two non-identical twins with a unique physiognomy. Front. Biosci. 11:818–829. doi: 10.2741/1839
Rajabi, M., and Mousa, S. A. (2017). The role of angiogenesis in cancer treatment. Biomedicines 5:34. doi: 10.3390/biomedicines5020034
Rask-Madsen, C., and King, G. L. (2008). Differential regulation of VEGF signaling by PKC-alpha and PKC-epsilon in endothelial cells. Arterioscler. Thromb. Vasc. Biol. 28, 919–924. doi: 10.1161/atvbaha.108.162842
Ruch, C., Skiniotis, G., Steinmetz, M. O., Walz, T., and Ballmer-Hofer, K. (2007). Structure of a VEGF-VEGF receptor complex determined by electron microscopy. Nat. Struct. Mol. Biol. 14, 249–250. doi: 10.1038/nsmb1202
Sarabipour, S., Ballmer-Hofer, K., and Hristova, K. (2016). VEGFR-2 conformational switch in response to ligand binding. eLife 5:e13876.
Sase, H., Watabe, T., Kawasaki, K., Miyazono, K., and Miyazawa, K. (2009). VEGFR2-PLCgamma1 axis is essential for endothelial specification of VEGFR2+ vascular progenitor cells. J. Cell Sci. 122, 3303–3311. doi: 10.1242/jcs.049908
Shalaby, F., Rossant, J., Yamaguchi, T. P., Gertsenstein, M., Wu, X. F., Breitman, M. L., et al. (1995). Failure of blood-island formation and vasculogenesis in Flk-1-deficient mice. Nature 376, 62–66. doi: 10.1038/376062a0
Shen, B. Q., Lee, D. Y., Gerber, H. P., Keyt, B. A., Ferrara, N., and Zioncheck, T. F. (1998). Homologous up-regulation of KDR/Flk-1 receptor expression by vascular endothelial growth factor in vitro. J. Biol. Chem. 273, 29979–29985. doi: 10.1074/jbc.273.45.29979
Shibuya, M. (2001). Structure and function of VEGF/VEGF-receptor system involved in angiogenesis. Cell Struct. Funct. 26, 25–35. doi: 10.1247/csf.26.25
Shibuya, M. (2011). Vascular endothelial growth factor (VEGF) and its receptor (VEGFR) signaling in angiogenesis: a crucial target for anti- and pro-angiogenic therapies. Genes Cancer 2, 1097–1105. doi: 10.1177/1947601911423031
Shibuya, M. (2013). VEGFR and type-V RTK activation and signaling. Cold Spring Harb. Perspect. Biol. 5, a009092. doi: 10.1101/cshperspect.a009092
Shinkai, A., Ito, M., Anazawa, H., Yamaguchi, S., Shitara, K., and Shibuya, M. (1998). Mapping of the sites involved in ligand association and dissociation at the extracellular domain of the kinase insert domain-containing receptor for vascular endothelial growth factor. J. Biol. Chem. 273, 31283–31288. doi: 10.1074/jbc.273.47.31283
Siveen, K. S., Prabhu, K., Krishnankutty, R., Kuttikrishnan, S., Tsakou, M., Alali, F. Q., et al. (2017). Vascular endothelial growth factor (VEGF) signaling in tumour vascularization: potential and challenges. Curr. Vasc. Pharmacol. 15, 339–351.
Smith, R. O., Ninchoji, T., Gordon, E., Andre, H., Dejana, E., Vestweber, D., et al. (2020). Vascular permeability in retinopathy is regulated by VEGFR2 Y949 signaling to VE-cadherin. eLife 9:e54056.
Solowiej, J., Bergqvist, S., McTigue, M. A., Marrone, T., Quenzer, T., Cobbs, M., et al. (2009). Characterizing the effects of the juxtamembrane domain on vascular endothelial growth factor receptor-2 enzymatic activity, autophosphorylation, and inhibition by axitinib. Biochemistry 48, 7019–7031. doi: 10.1021/bi900522y
Stopeck, A., Sheldon, M., Vahedian, M., Cropp, G., Gosalia, R., and Hannah, A. (2002). Results of a Phase I dose-escalating study of the antiangiogenic agent, SU5416, in patients with advanced malignancies. Clin. Cancer Res. 8, 2798–2805.
Takahashi, T., and Shibuya, M. (1997). The 230 kDa mature form of KDR/Flk-1 (VEGF receptor-2) activates the PLC-gamma pathway and partially induces mitotic signals in NIH3T3 fibroblasts. Oncogene 14, 2079–2089. doi: 10.1038/sj.onc.1201047
Takahashi, T., Ueno, H., and Shibuya, M. (1999). VEGF activates protein kinase C-dependent, but Ras-independent Raf-MEK-MAP kinase pathway for DNA synthesis in primary endothelial cells. Oncogene 18, 2221–2230. doi: 10.1038/sj.onc.1202527
Takahashi, T., Yamaguchi, S., Chida, K., and Shibuya, M. (2001). A single autophosphorylation site on KDR/Flk-1 is essential for VEGF-A-dependent activation of PLC-gamma and DNA synthesis in vascular endothelial cells. EMBO J. 20, 2768–2778. doi: 10.1093/emboj/20.11.2768
Vallon, M., Chang, J., Zhang, H., and Kuo, C. J. (2014). Developmental and pathological angiogenesis in the central nervous system. Cell Mol. Life Sci. 71, 3489–3506. doi: 10.1007/s00018-014-1625-0
Volz, C., Breid, S., Selenz, C., Zaplatina, A., Golfmann, K., Meder, L., et al. (2020). Inhibition of tumor VEGFR2 induces serine 897 EphA2-dependent tumor cell invasion and metastasis in NSCLC. Cell Rep. 31:107568. doi: 10.1016/j.celrep.2020.107568
Walter, M., Lucet, I. S., Patel, O., Broughton, S. E., Bamert, R., Williams, N. K., et al. (2007). The 2.7 A crystal structure of the autoinhibited human c-Fms kinase domain. J. Mol. Biol. 367, 839–847.
Wang, L., Ge, H., Peng, L., and Wang, B. (2019). A meta-analysis of the relationship between VEGFR2 polymorphisms and atherosclerotic cardiovascular diseases. Clin. Cardiol. 42, 860–865. doi: 10.1002/clc.23233
Warner, A. J., Lopez-Dee, J., Knight, E. L., Feramisco, J. R., and Prigent, S. A. (2000). The Shc-related adaptor protein, Sck, forms a complex with the vascular-endothelial-growth-factor receptor KDR in transfected cells. Biochem. J. 347, 501–509. doi: 10.1042/0264-6021:3470501
Weis, S. M., and Cheresh, D. A. (2011). Tumor angiogenesis: molecular pathways and therapeutic targets. Nat. Med. 17, 1359–1370. doi: 10.1038/nm.2537
Wong, C., and Jin, Z. G. (2005). Protein kinase C-dependent protein kinase D activation modulates ERK signal pathway and endothelial cell proliferation by vascular endothelial growth factor. J. Biol. Chem. 280, 33262–33269. doi: 10.1074/jbc.m503198200
Wybenga-Groot, L. E., Baskin, B., Ong, S. H., Tong, J., Pawson, T., and Sicheri, F. (2001). Structural basis for autoinhibition of the Ephb2 receptor tyrosine kinase by the unphosphorylated juxtamembrane region. Cell 106, 745–757. doi: 10.1016/s0092-8674(01)00496-2
Yang, J., Yan, J., and Liu, B. (2018). Targeting VEGF/VEGFR to modulate antitumor immunity. Front. Immunol. 9:978.
Yang, Y., Xie, P., Opatowsky, Y., and Schlessinger, J. (2010). Direct contacts between extracellular membrane-proximal domains are required for VEGF receptor activation and cell signaling. Proc. Natl. Acad. Sci. U.S.A. 107, 1906–1911. doi: 10.1073/pnas.0914052107
Yehya, A. H. S., Asif, M., Petersen, S. H., Subramaniam, A. V., Kono, K., Majid, A., et al. (2018). Angiogenesis: managing the culprits behind tumorigenesis and metastasis. Medicina 54:8. doi: 10.3390/medicina54010008
Yin, Z., Gong, G., Zhu, C., Wang, B., Sun, C., Liu, X., et al. (2020). Angiopoietin-1 protects neurons by inhibiting autophagy after neuronal oxygen-glucose deprivation/recovery injury. Neuroreport 31, 825–832.
Zeng, H., Dvorak, H. F., and Mukhopadhyay, D. (2001). Vascular permeability factor (VPF)/vascular endothelial growth factor (VEGF) peceptor-1 down-modulates VPF/VEGF receptor-2-mediated endothelial cell proliferation, but not migration, through phosphatidylinositol 3-kinase-dependent pathways. J. Biol. Chem. 276, 26969–26979. doi: 10.1074/jbc.m103213200
Zhang, J., Cao, R., Zhang, Y., Jia, T., Cao, Y., and Wahlberg, E. (2009). Differential roles of PDGFR-alpha and PDGFR-beta in angiogenesis and vessel stability. FASEB J. 23, 153–163. doi: 10.1096/fj.08-113860
Zhang, J., and Hughes, S. (2006). Role of the ephrin and Eph receptor tyrosine kinase families in angiogenesis and development of the cardiovascular system. J. Pathol. 208, 453–461. doi: 10.1002/path.1937
Zhang, Y., Kontos, C. D., Annex, B. H., and Popel, A. S. (2019). Angiopoietin-tie signaling pathway in endothelial cells: a computational model. iScience 20, 497–511. doi: 10.1016/j.isci.2019.10.006
Keywords: VEGF, VEGFR-2, structure, function and role, vasculogenesis, angiogenesis
Citation: Wang X, Bove AM, Simone G and Ma B (2020) Molecular Bases of VEGFR-2-Mediated Physiological Function and Pathological Role. Front. Cell Dev. Biol. 8:599281. doi: 10.3389/fcell.2020.599281
Received: 26 August 2020; Accepted: 21 October 2020;
Published: 16 November 2020.
Edited by:
Xiaofeng Yang, Temple University, United StatesReviewed by:
Qian Yang, Fourth Military Medical University, ChinaCopyright © 2020 Wang, Bove, Simone and Ma. This is an open-access article distributed under the terms of the Creative Commons Attribution License (CC BY). The use, distribution or reproduction in other forums is permitted, provided the original author(s) and the copyright owner(s) are credited and that the original publication in this journal is cited, in accordance with accepted academic practice. No use, distribution or reproduction is permitted which does not comply with these terms.
*Correspondence: Binyun Ma, YmlueXVubWFAdXNjLmVkdQ==; Xinrong Wang, d2FuZ3hyQGdzYXUuZWR1LmNu
Disclaimer: All claims expressed in this article are solely those of the authors and do not necessarily represent those of their affiliated organizations, or those of the publisher, the editors and the reviewers. Any product that may be evaluated in this article or claim that may be made by its manufacturer is not guaranteed or endorsed by the publisher.
Research integrity at Frontiers
Learn more about the work of our research integrity team to safeguard the quality of each article we publish.