- 1Orthopaedic Research Laboratory, Feinstein Institutes for Medical Research, Northwell Health, Manhasset, NY, United States
- 2Department of Orthopaedic Surgery, Northwell Health, New Hyde Park, NY, United States
Background: Microfracture is one of the most widely used techniques for the repair of articular cartilage. However, microfracture often results in filling of the chondral defect with fibrocartilage, which exhibits poor durability and sub-optimal mechanical properties. Stromal cell-derived factor-1 (SDF-1) is a potent chemoattractant for mesenchymal stem cells (MSCs) and is expressed at high levels in bone marrow adjacent to developing cartilage during endochondral bone formation. Integrating SDF-1 into an implantable collagen scaffold may provide a chondro-conductive and chondro-inductive milieu via chemotaxis of MSCs and promotion of chondrogenic differentiation, facilitating more robust hyaline cartilage formation following microfracture.
Objective: This work aimed to confirm the chemoattractive properties of SDF-1 in vitro and develop a one-step method for incorporating SDF-1 in vivo to enhance cartilage repair using a rat osteochondral defect model.
Methods: Bone marrow-derived MSCs (BMSCs) were harvested from the femurs of Sprague–Dawley rats and cultured in low-glucose Dulbecco’s modified Eagle’s medium containing 10% fetal bovine serum, with the medium changed every 3 days. Passage 1 MSCs were analyzed by flow cytometry with an S3 Cell Sorter (Bio-Rad). In vitro cell migration assays were performed on MSCs by labeling cells with carboxyfluorescein diacetate, succinimidyl ester (CFDA-SE; Bio-Rad). For the microfracture model, a 1.6-mm-diameter osteochondral defect was created in the femoral trochleae of 20 Sprague–Dawley rats bilaterally until bone marrow spillage was seen under saline irrigation. One knee was chosen at random to receive implantation of the scaffold, and the contralateral knee was left unfilled as an empty control. Type I collagen scaffolds (Kensey Nash) were coated with either gelatin only or gelatin and SDF-1 using a dip coating process. The rats received implantation of either a gelatin-only scaffold (N = 10) or gelatin-and-SDF-1 scaffold (N = 10) at the site of the microfracture. Femurs were collected for histological analyses at 4- and 8-week time points post-operatively, and sections were stained with Safranin O/Fast Green. The samples were graded blindly by two observers using the Modified O’Driscoll score, a validated scoring system for chondral repair. A minimum of 10 separate grading scores were made per sample and averaged. Quantitative comparisons of cell migration in vitro were performed with one-way ANOVA. Cartilage repair in vivo was also compared among groups with one-way ANOVA, and the results were presented as mean ± standard deviation, with P-values < 0.05 considered as statistically significant.
Results: MSC migration showed a dose–response relationship with SDF-1, with an optimal dosage for chemotaxis between 10 and 100 ng/ml. After scaffold implantation, the SDF-1-treated group demonstrated complete filling of the cartilage defect with mature cartilage tissue, exhibiting strong proteoglycan content, smooth borders, and good incorporation into marginal cartilage. Modified O’Driscoll scores after 8 weeks showed a significant improvement of cartilage repair in the SDF-1 group relative to the empty control group (P < 0.01), with a trend toward improvement when compared with the gelatin-only-scaffold group (P < 0.1). No significant differences in scores were found between the empty defect group and gelatin-only group.
Conclusion: In this study, we demonstrated a simple method for improving the quality of cartilage defect repair in a rat model of microfracture. We confirmed the chemotactic properties of SDF-1 on rat MSCs and found an optimized dosage range for chemotaxis between 10 and 100 ng/ml. Furthermore, we demonstrated a strategy to incorporate SDF-1 into gelatin–collagen I scaffolds in vivo at the site of an osteochondral defect. SDF-1-treated defects displayed robust hyaline cartilage resurfacing of the defect with minimal fibrous tissue, in contrast to the empty control group. The results of the in vitro and in vivo studies together suggest that SDF-1-mediated signaling may significantly improve the quality of cartilage regeneration in an osteochondral defect.
Introduction
While the etiology of joint degeneration and osteoarthritis occurs at the molecular, cellular, and tissue level, current treatments of isolated chondral defects are primarily surgical (Furman et al., 2006; Daher et al., 2009; Anderson et al., 2011). Regeneration of hyaline cartilage is limited by the lack of chondral vascularity, diminishing the recruitment of renewable cells to the site of injury (Csaki et al., 2008; Fong et al., 2011). Thus, penetration of the subchondral bone with techniques such as microfracture emerged and served to recruit mesenchymal stem cells (MSCs) from the bone marrow to infiltrate the overlying chondral lesion. However, long-term durability was inadequate, as the native hyaline cartilage was repaired with fibrocartilage consisting of primarily type I collagen, which is prone to rapid degeneration and less adaptable than its hyaline counterpart (Mithoefer et al., 2009; Gomoll and Minas, 2014; Richter et al., 2016). It is thought that microfracture alone does not recruit a sufficient amount of reparative cells and growth factors to promote adequate native tissue repair (Richter, 2009). One potential therapeutic strategy to augment the chondrogenic milieu following microfracture is by incorporating homing factors into cell-free scaffolds to promote the recruitment of MSCs to the site of injury (Mishima and Lotz, 2008; Chanda et al., 2010; Fong et al., 2011; Eseonu and De Bari, 2015; Truong et al., 2017; Lu et al., 2018).
Stromal cell-derived factor-1 (SDF-1) is a potent chemoattractant for MSCs and has associations with bone repair (Hwang et al., 2015; Liu et al., 2015). SDF-1 attracts MSCs to the site of bone fractures and is expressed in marrow adjacent to developing cartilage during endochondral bone formation (Wei et al., 2010; Murata et al., 2012; Toupadakis et al., 2012). In addition, the receptor for SDF-1, C-X-C chemokine receptor type 4 (CXCR4), plays a significant role in chondrocyte differentiation and proliferation (Mazzetti et al., 2004). Integrating SDF-1 into an implantable collagen scaffold may improve the quality of cartilage repair by recruiting MSCs from the underlying bone marrow and promoting a chondroinductive milieu. We seek to evaluate a novel method of improving the quality of chondral repair following microfracture by incorporating SDF-1 into an implantable, cell-free scaffold and directly comparing it to microfracture alone.
Materials and Methods
Preparation of BMSCs
Rat bone marrow-derived MSCs (BMSCs) were isolated from the femurs of Sprague–Dawley rats as previously described (Haynesworth et al., 1992). Briefly, the femurs were harvested using an aseptic technique. After removing the soft tissue and periosteum, the bones were cut using a bone rongeur. Bone marrow was washed out with low-glucose Dulbecco’s modified Eagle’s medium (Fisher Scientific, Pittsburgh, PA, United States) containing 10% fetal bovine serum (Fisher Scientific, Pittsburgh, PA, United States) using a syringe. The bone marrow was then mixed with medium and cultured at 37°C with 5% CO2 in an incubator, with the medium changed every 3 days.
Flow Cytometry Study
Rat BMSCs at passage 1 were detached from the cell culture flask by Accutase (Innovative Cell Technologies, San Diego, CA, United States). After washing with phosphate-buffered saline (PBS), the cells were stained with antibodies against CD45 (AbD Serotec, Raleigh, NC, United States), CD73 (BD Biosciences, San Jose, CA, United States), CD90 (BioLegend, San Diego, CA, United States), and CD106 (BioLegend, San Diego, CA, United States). The stained cells were analyzed by flow cytometry with an S3 Cell Sorter (Bio-Rad, Hercules, CA, United States).
In vitro Cell Migration Assay
Rat BMSCs at passage 1 were serum-starved for 2 h at 37°C. A 24-well plate was loaded with Transwell inserts (8.0 μm in pore size; Fisher Scientific, Pittsburgh, PA, United States). The lower chambers contained 600 μl of either serum-free medium (negative control), medium with serum (positive control), or SDF-1 (1, 10, or 100 ng/ml; R&D Systems, Minneapolis, MN, United States). Each condition was set up in triplicate. The serum-starved BMSCs (105 cells/well) were loaded on the upper chamber and incubated at 37°C for 4 h. The membranes were fixed with formalin for 10 min and washed with PBS before staining with Wright–Giemsa. The migrated cells were counted under a light microscope (×400), with 30 random fields analyzed per membrane. The results are represented as the average number of migrated cells counted per ×400 magnification field.
For morphological observation, BMSCs were labeled with carboxyfluorescein diacetate, succinimidyl ester (CFDA-SE; Bio-Rad, Hercules, CA, United States) following the manufacturer’s instructions prior to loading on Transwell inserts. Fluorescence blocking inserts were used; thus, fluorescence was visible only when cells migrated to the observation side of the membrane.
Scaffold Preparation
Type I collagen was selected as the scaffold biomaterial given its proven safety and biocompatibility profile and widespread availability, making it an ideal agent for translation into clinical application (Tampieri et al., 2008; Kon et al., 2010). Collagen I scaffolds (Kensey Nash, Exton, PA, United States) were coated with either gelatin only (control) or gelatin and SDF-1 using a dip coating process previously described (Dines et al., 2007, 2011; Uggen et al., 2010; Cummings et al., 2012). Briefly, gelatin was prepared by heating a 10% (wt) solution of medical-grade soluble bovine collagen (Semed-S, Kensey-Nash, Exton PA) to 80°C for 10 min, followed by incubation at 37°C. SDF-1 (50 ng/ml) was reconstituted in 10 mM HCl and mixed with the gelatin at a ratio of 2:1 (gelatin/SDF). Collagen scaffolds were pre-treated in 70% EtOH for 10 min, washed with PBS, and then dip-coated in the gelatin solution at 37°C for 30 min with gentle agitation. The scaffolds were removed from the solution and air-dried overnight in a sterile laminar flow hood.
Surgical Procedure
The usage of animals in this study was approved by the Institutional Animal Care and Use Committee of Feinstein Institutes for Medical Research. A medial parapatellar approach to the knee joint was performed bilaterally in Sprague–Dawley rats (N = 20; weight, 500 g). A 1.6-mm-diameter full-thickness osteochondral defect was created in each femoral trochlea until bone marrow spillage was visualized under saline irrigation as a model of microfracture. One knee was chosen at random with a coin toss to receive scaffold implantation (1.8 mm in diameter, 1.5 mm in thickness) and the contralateral knee served as an empty control. The rats received implantation of a scaffold dip-coated either with gelatin only (N = 10) or with gelatin and SDF-1 (N = 10). Femurs were collected for histological analysis at 4- and 8-week time points post-operatively, with the endpoints defined based upon current literature (Lee and Im, 2012; Dahlin et al., 2014; Mahmoud et al., 2017; Hayashi et al., 2018).
Histological Evaluation
The distal femurs were dissected free of soft tissue and fixed in 10% Millonig’s buffered formalin (Fisher Scientific, Pittsburgh, PA, United States). The samples were decalcified with formic acid decalcification solution (Fisher Scientific, Pittsburgh, PA, United States) for 5 days with shaking, then embedded in paraffin, and cut in the coronal plane (5 μm in thickness). Serial sections were cut at 100-μm intervals through an area approximately 30% of the total surface area of the defect. The sections were mounted and stained with Safranin-O/Fast Green. The samples were graded blindly by two observers using the Modified O’Driscoll Score, a validated scoring system for chondral repair (Qi et al., 2014). A minimum of 10 separate grading scores were made per sample and averaged.
Statistical Analyses
The samples were tested for normality using the Shapiro–Wilk test. Quantitative comparisons of cell migration in vitro were performed with one-way ANOVA. The results are presented as mean ± standard deviation, with P-values less than 0.05 considered as statistically significant. Cartilage repair in vivo was compared among groups with one-way ANOVA. The results are presented as mean ± standard deviation, with P-values less than 0.05 considered as statistically significant.
Results
Flow Cytometric Characteristics of Rat BMSCs
Bone marrow-derived MSCs at passage 1 were 43% CD90-positive and 21% CD45-negative. Among the CD45-negative cells, 95% were CD90-positive, 19% of CD45-negative cells were positive for both CD90 and CD106, and 38% of CD45-negative cells were positive for both CD90 and CD73 (Figure 1).
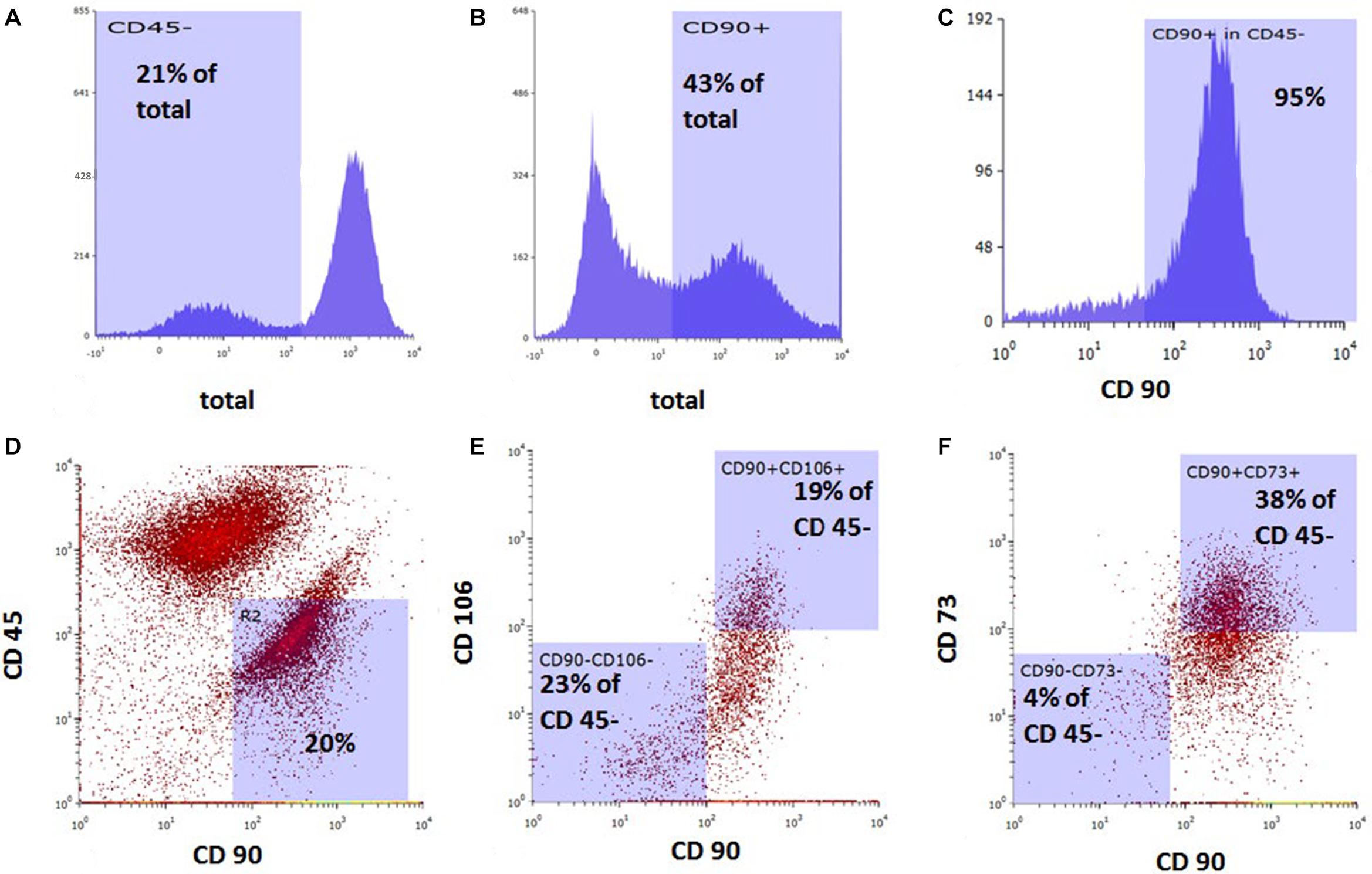
Figure 1. Flow cytometric analysis of rat bone marrow-derived mesenchymal stem cells prior to in vitro cell migration studies. Cell population stained with (A) anti-CD45, (B) anti-CD90, and (C) anti-CD90 among CD45-negative cells. Dot plot of combined staining for (D) CD45 and CD90, (E) CD106 and CD90 among CD45-negative population, and (F) CD73 and CD90 among CD45-negative population.
In vitro Cell Migration
Chemoattraction of BMSCs by SDF-1 was tested with the Transwell culture system in vitro. After 4 h in culture, the CFDA-SE-stained cells were found to have migrated in great quantities toward the SDF-1-containing chamber (100 ng/ml). Morphologically, these cells were round and larger than the pore size (Figure 2A).
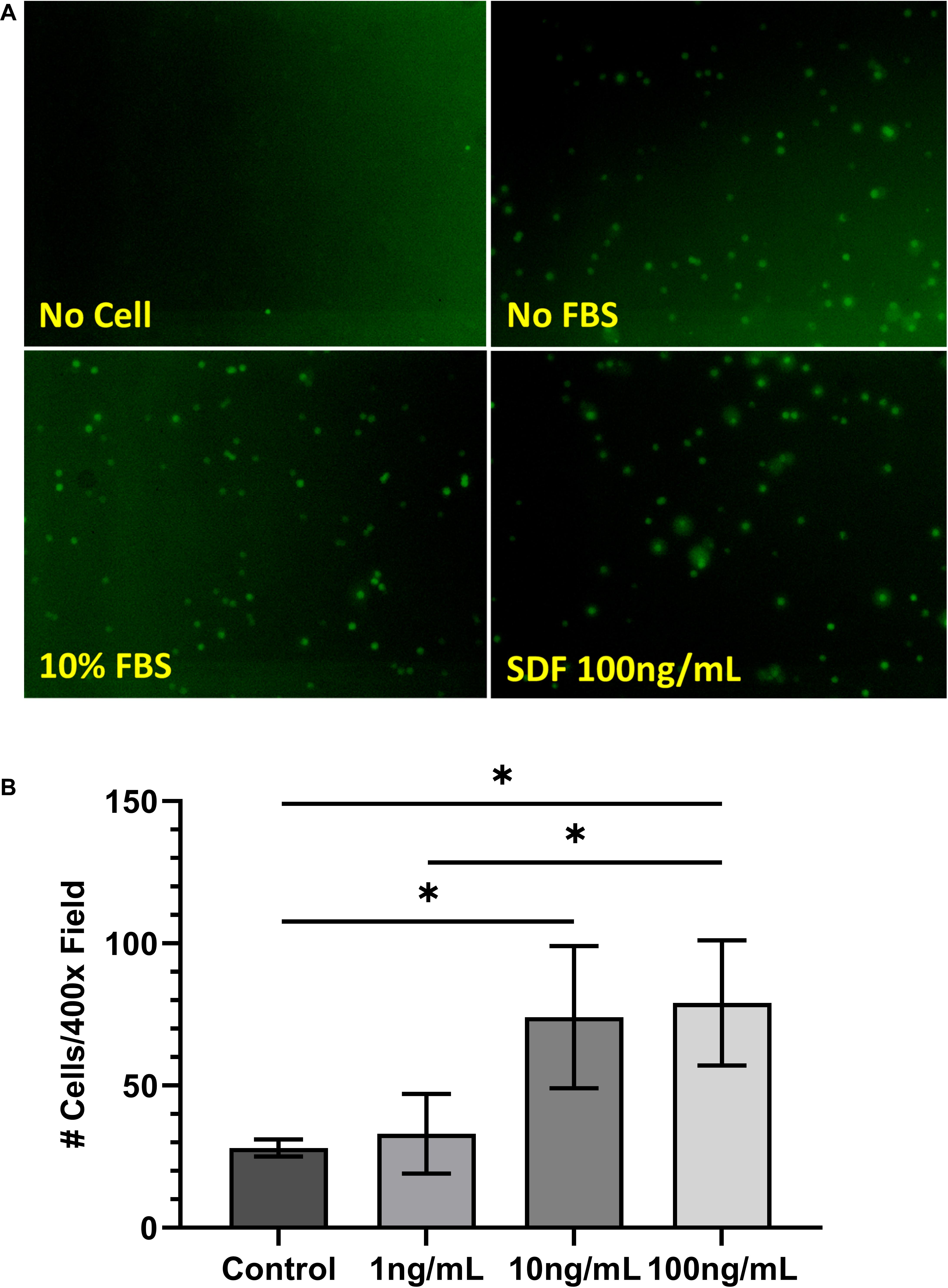
Figure 2. (A) Morphology of carboxyfluorescein diacetate–succinimidyl ester-stained bone marrow-derived mesenchymal stem cells (BMSCs) following cell migration assay. (B) Results of cell migration assay of BMSCs treated with stromal cell-derived factor-1 (1, 10, and 100 ng/ml). Values are represented as average number of cells counted per ×400 field. ∗P < 0.05.
To determine the optimal dosage of SDF-1 for chemoattraction of BMSCs, SDF-1 was added to the Transwell system at concentrations of 1, 10, and 100 ng/ml. Cell migration was increased significantly from the control group at both 10 ng/ml (28 ± 3 vs. 74 ± 25 cells/×400 field, respectively; P = 0.04) and 100 ng/ml (79 ± 22 cells/×400 field; P = 0.04) concentrations of SDF-1, which is consistent with previous literature (Figure 2B) (Ji et al., 2013). Cell migration did not differ significantly between the control group and the SDF-1 group containing 1 ng/ml (33 ± 14 cells/×400 field; P = 0.56).
In vivo Cartilage Repair
No post-operative complications were noted throughout the study period. SDF-1-treated defects showed complete filling of mature cartilage tissue with strong proteoglycan content, smooth borders, and substantial incorporation into surrounding cartilage (Figures 3A–C). Modified O’Driscoll scores at 4 weeks post-operatively showed a trend toward improvement in cartilage repair quality in the SDF-1 group relative to the empty control group (13 ± 8 vs. 3 ± 1; P = 0.07); however, the difference was not statistically significant. No significant difference in cartilage repair quality was found at 4 weeks post-operatively between the SDF-1 group and the gelatin-only group (8 ± 5; P = 0.26). Modified O’Driscoll scores at 8 weeks post-operatively showed a significant improvement in cartilage quality in the SDF-1 group relative to the empty control group (18 ± 6 vs. 5 ± 3; P = 0.009) and a trend toward improvement when compared with the gelatin-only-scaffold group (9 ± 4; P = 0.09); however, the difference was not statistically significant. No significant difference in O’Driscoll scores was found when comparing the empty control and gelatin-only-scaffold groups at 4 weeks (P = 0.14) or 8 weeks post-operatively (P = 0.17; Figure 3D).
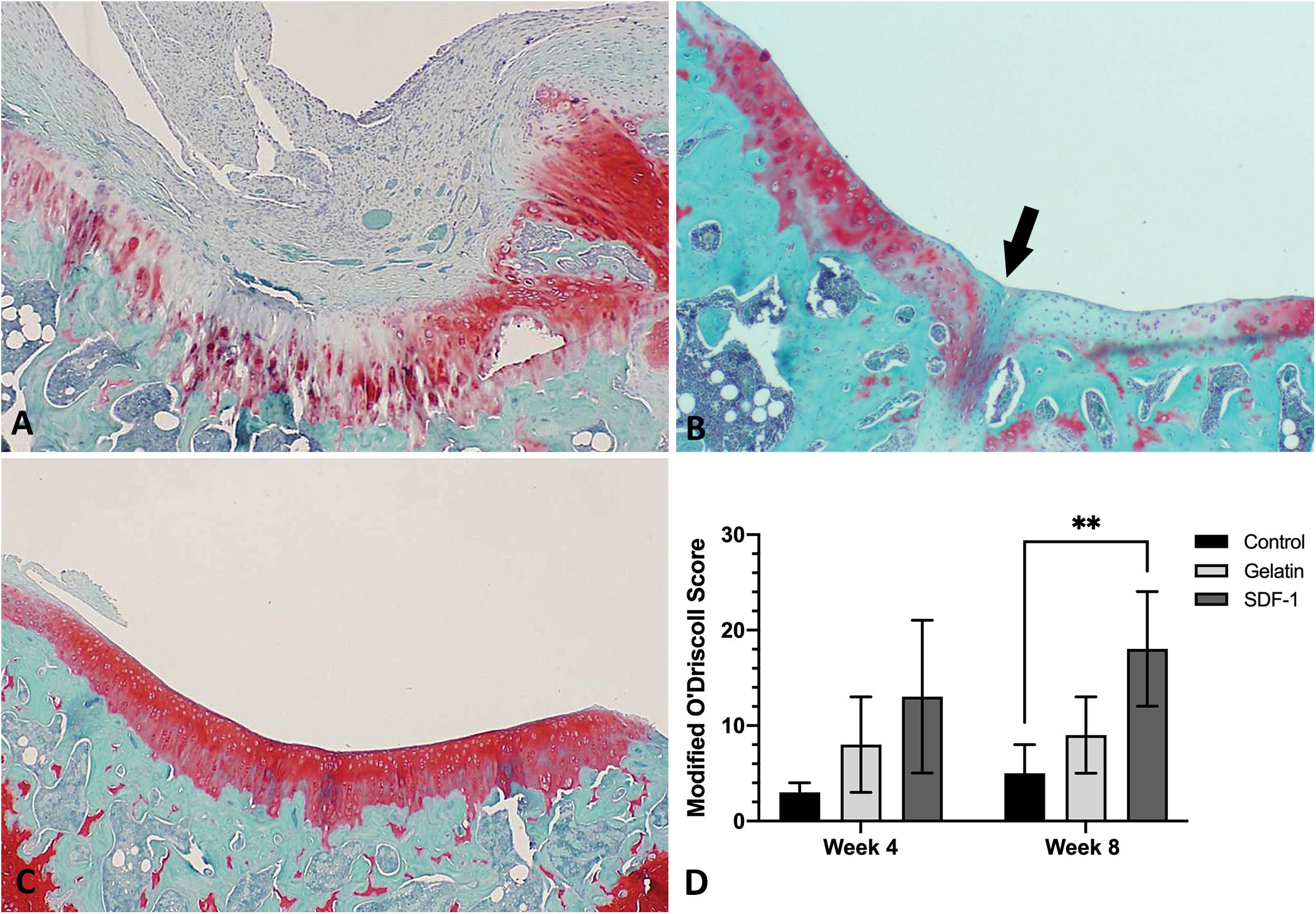
Figure 3. Histological evaluation with Safranin O/Fast Green to compare the repair of microfracture defects with implantation of gelatin–collagen I scaffolds carrying stromal cell-derived factor-1 (SDF-1) (50 ng/ml) and control groups in vivo (×40 magnification) at 8 weeks post-operatively. Representative images were selected based upon the best quality of cartilage repair within each group. (A) Defect only group. (B) Gelatin only group. (C) Gelatin and SDF-1 group. (D) Quantitative comparison of cartilage repair quality with Modified O’Driscoll scores at 4 and 8 weeks post-operatively. ∗∗P < 0.01.
Discussion
Microfracture is one of the most widely used techniques for the repair of articular cartilage (Oussedik et al., 2015; Wylie et al., 2015). However, microfracture results in filling of the cartilage defect with fibrocartilage, which is less durable than native hyaline cartilage and prone to re-injury (Krych et al., 2012; Xing et al., 2013; Oussedik et al., 2015). Implantable cell-free scaffolds coated in chemoattractive factors may improve the quality of cartilage repair following microfracture (Mishima and Lotz, 2008; Chanda et al., 2010; Fong et al., 2011; Eseonu and De Bari, 2015; Truong et al., 2017; Lu et al., 2018). In this study, we determined the optimal concentration of SDF-1 for MSC chemoattraction in vitro and found that incorporating SDF-1 into an implantable scaffold yielded a superior quality of cartilage repair when compared with microfracture alone.
SDF-1 promotes MSC migration and homing to the bone marrow through its receptor, CXCR4 (Shi et al., 2007; Baek et al., 2011). In addition to MSCs, CXCR4 is also expressed on hematopoietic progenitor cells, chondrocytes, and fibroblasts (Kucia et al., 2005; Villalvilla et al., 2014). As the SDF-1/CXCR4 axis is not exclusive to MSC homing, determining the optimal dosage of SDF-1 for the homing of MSCs to cartilage defects is necessary. Previously, a concentration of 50–200 ng/ml was reported to enhance the chemoattraction of rat BMSCs in vitro. However, when the concentration of SDF-1 was increased to 400 ng/ml and above, the chemoattractant effect began to significantly decrease (Ji et al., 2013). In our study, we confirmed the optimal concentration of SDF-1 to be between 10 and 100 ng/ml.
To apply SDF-1 in vivo, we used a gelatin carrier system developed for the application of growth factors (Dines et al., 2007, 2011; Uggen et al., 2010; Cummings et al., 2012). In vitro testing revealed that the release of growth factors from the carrier system could be sustained at a standard rate within the first 48 h (Uggen et al., 2010; Cummings et al., 2012). After implantation, the growth factors carried by the system maintained their functional effect in vivo (Dines et al., 2007; Uggen et al., 2010; Cummings et al., 2012). In our study, the gelatin–SDF-1 solution was loaded on a bovine collagen I scaffold for implantation in a rat microfracture model. When assessing the compatibility of MSCs with collagen scaffolds, type I collagen scaffolds exhibited superior attachment of BMSCs and cartilage-derived MSCs than type II collagen scaffolds. Moreover, the cells attached on the type I collagen scaffold maintained their spindle shape as a monolayer, while the cells on the type II collagen scaffold were round and tightly packed (Zhang et al., 2013). In clinical application, type I collagen scaffolds combined with hydroxyapatite showed improvement in the repair of cartilage defects (Filardo et al., 2013; Delcogliano et al., 2014).
In our study, implantation of an SDF-1-coated scaffold at the site of microfracture resulted in a significant improvement in cartilage repair, with results seen as early as 4 weeks post-operatively. Similarly, using a rabbit model, Chen et al. (2015) found that type I collagen scaffolds carrying SDF-1 (100 ng/ml) enhanced the repair of cartilage defects after 12 weeks. Zhang et al. (2013) utilized collagen I scaffolds carrying SDF-1 (120 ng/ml) on a partial-thickness cartilage defect using a rabbit model and found improved repair quality in the SDF-1 treatment group at 6 weeks post-operatively. In comparison to previous studies, our SDF-1 carrier system achieved nearly complete cartilage defect filling at 8 weeks post-operatively using an SDF-1 concentration of 50 ng/ml. A subsequent elution profile of our scaffold revealed an initial bolus release of SDF-1, followed by a continuous, steady release over the following 5 days, consistent with available literature (Sun et al., 2016). This may explain why we were able to achieve significant results at a lower concentration than those of other studies. While low SDF-1 levels are tied to healing, high SDF-1 levels have been paradoxically implicated in rheumatoid arthritis and osteoarthritis (Villalvilla et al., 2014; Kanbe et al., 2015). Thus, maintaining therapeutic levels of SDF-1 in the joint space by optimizing the biomechanics of the delivery system is vital.
The limitations of our study include the small sample size and relatively short post-operative time points. We found no significant difference in the quality of cartilage repair between groups at 4 weeks post-operatively as measured by Modified O’Driscoll scores. However, significant improvement in repair was noted at 8 weeks post-operatively in the SDF-1-treated group when compared with the empty defect group. When compared with the gelatin-dipped scaffold group, the SDF-1-treated group showed a tendency for improvement in the Modified O’Driscoll scores, but the difference did not meet statistical significance. In future studies, conducting analyses at longer time points, such as at 12- or 16-weeks post-operatively, may allow for a more appropriate length of time for mature tissue healing. Furthermore, increasing the sample size of the study would generate greater power to elucidate the difference between the gelatin- and SDF-1-treated scaffold groups. Immunohistochemical staining of healed tissue for collagen types I and II would provide definitive evidence of repair with hyaline vs. fibrocartilage; fibrocartilage contains a significant amount of type I collagen, while hyaline cartilage contains little to none. Based on our in vitro cell migration studies, a concentration of 50 ng/ml SDF-1 was chosen for the in vivo studies to optimize chemotaxis to the defect site with a minimal dose. However, we recognize that the kinetics of SDF-1 in vivo may differ significantly from the kinetics observed in in vitro studies. Future areas of study may include comparing a variety of SDF-1 concentrations in vivo to confirm the ideal concentration for cartilage repair and performing tests of durability and mechanical loading to provide evidence of clinically meaningful results. Other factors commonly implicated in cartilage repair, such as granulocyte-macrophage colony-stimulating factor transforming growth factor beta, platelet-rich plasma, and fibroblast growth factor, should be compared directly to and in combination with SDF-1 to formulate an optimal cocktail for cartilage repair (Kang et al., 2008; Huh et al., 2014; Harada et al., 2015; Truong et al., 2017).
In this study, we successfully demonstrated a cost-effective, one-step process for enhancing microfracture to improve the outcome of cartilage repair. We confirmed the chemotactic properties of SDF-1 on rat MSCs in vitro and found an optimized dosage range for chemotaxis between 10 and 100 ng/ml, consistent with previous literature. Furthermore, we successfully demonstrated a simple method of incorporating SDF-1 into a biocompatible scaffold at the site of osteochondral defect. SDF-1-treated defects displayed robust resurfacing with hyaline-like cartilage with minimal fibrous tissue compared with the control groups. Overall, the results suggest that scaffolds treated with SDF-1 can improve the quality of cartilage repair in an osteochondral defect.
Data Availability Statement
The raw data supporting the conclusions of this article will be made available by the authors, without undue reservation.
Ethics Statement
The animal study was reviewed and approved by the Institutional Animal Care and Use Committee of the Feinstein Institutes for Medical Research.
Author Contributions
HL, NS, and DG contributed to the concept, design, and supervision of the study. TM, JS, PP, and HL collected, analyzed, and interpreted the data. TM, HL, and DG were involved in the writing and critical review of the manuscript. TM and DG conducted the final editing and proofreading. All the authors read and approved the final manuscript.
Funding
This work was supported by a grant from the Department of Orthopaedic Surgery at Northwell Health.
Conflict of Interest
The authors declare that the research was conducted in the absence of any commercial or financial relationships that could be construed as a potential conflict of interest.
References
Anderson, D. D., Chubinskaya, S., Guilak, F., Martin, J. A., Oegema, T. R., Olson, S. A., et al. (2011). Post-traumatic osteoarthritis: improved understanding and opportunities for early intervention. J. Orthop. Res. 29, 802–809. doi: 10.1002/jor.21359
Baek, S. J., Kang, S. K., and Ra, J. C. (2011). In vitro migration capacity of human adipose tissue-derived mesenchymal stem cells reflects their expression of receptors for chemokines and growth factors. Exp. Mol. Med. 43, 596–603. doi: 10.3858/emm.2011.43.10.069
Chanda, D., Kumar, S., and Ponnazhagan, S. (2010). Therapeutic potential of adult bone marrow-derived mesenchymal stem cells in diseases of the skeleton. J. Cell. Biochem. 111, 249–257. doi: 10.1002/jcb.22701
Chen, P., Tao, J., Zhu, S., Cai, Y., Mao, Q., Yu, D., et al. (2015). Radially oriented collagen scaffold with SDF-1 promotes osteochondral repair by facilitating cell homing. Biomaterials 39, 114–123. doi: 10.1016/j.biomaterials.2014.10.049
Csaki, C., Schneider, P. R., and Shakibaei, M. (2008). Mesenchymal stem cells as a potential pool for cartilage tissue engineering. Ann. Anat. 190, 395–412. doi: 10.1016/j.aanat.2008.07.007
Cummings, S. H., Grande, D. A., Hee, C. K., Kestler, H. K., Roden, C. M., Shah, N. V., et al. (2012). Effect of recombinant human platelet-derived growth factor-BB-coated sutures on Achilles tendon healing in a rat model: a histological and biomechanical study. J. Tissue Eng. 3:2041731412453577. doi: 10.1177/2041731412453577
Daher, R. J., Chahine, N. O., Greenberg, A. S., Sgaglione, N. A., and Grande, D. A. (2009). New methods to diagnose and treat cartilage degeneration. Nat. Rev. Rheumatol. 5, 599–607. doi: 10.1038/nrrheum.2009.204
Dahlin, R. L., Kinard, L. A., Lam, J., Needham, C. J., Lu, S., Kasper, F. K., et al. (2014). Articular chondrocytes and mesenchymal stem cells seeded on biodegradable scaffolds for the repair of cartilage in a rat osteochondral defect model. Biomaterials 35, 7460–7469.
Delcogliano, M., de Caro, F., Scaravella, E., Ziveri, G., De Biase, C. F., Marotta, D., et al. (2014). Use of innovative biomimetic scaffold in the treatment for large osteochondral lesions of the knee. Knee Surg. Sports Traumatol. Arthrosc. 22, 1260–1269. doi: 10.1007/s00167-013-2717-3
Dines, J. S., Cross, M. B., Dines, D., Pantazopoulos, C., Kim, H. J., Razzano, P., et al. (2011). In vitro analysis of an rhGDF-5 suture coating process and the effects of rhGDF-5 on rat tendon fibroblasts. Growth Factors 29, 1–7. doi: 10.3109/08977194.2010.526605
Dines, J. S., Weber, L., Razzano, P., Prajapati, R., Timmer, M., Bowman, S., et al. (2007). The effect of growth differentiation factor-5-coated sutures on tendon repair in a rat model. J. Shoulder Elbow Surg. 16 (Suppl. 5), S215–S221. doi: 10.1016/j.jse.2007.03.001
Eseonu, O. I., and De Bari, C. (2015). Homing of mesenchymal stem cells: mechanistic or stochastic? Implications for targeted delivery in arthritis. Rheumatology (Oxford) 54, 210–218. doi: 10.1093/rheumatology/keu377
Filardo, G., Kon, E., Di Martino, A., Busacca, M., Altadonna, G., and Marcacci, M. (2013). Treatment of knee osteochondritis dissecans with a cell-free biomimetic osteochondral scaffold: clinical and imaging evaluation at 2-year follow-up. Am. J. Sports Med. 41, 1786–1793. doi: 10.1177/0363546513490658
Fong, E. L., Chan, C. K., and Goodman, S. B. (2011). Stem cell homing in musculoskeletal injury. Biomaterials 32, 395–409. doi: 10.1016/j.biomaterials.2010.08.101
Furman, B. D., Olson, S. A., and Guilak, F. (2006). The development of posttraumatic arthritis after articular fracture. J. Orthop. Trauma 20, 719–725. doi: 10.1097/01.bot.0000211160.05864.14
Gomoll, A. H., and Minas, T. (2014). The quality of healing: articular cartilage. Wound Repair Regen. 22, 30–38. doi: 10.1111/wrr.12166
Harada, Y., Nakasa, T., Mahmoud, E. E., Kamei, G., Adachi, N., Deie, M., et al. (2015). Combination therapy with intra-articular injection of mesenchymal stem cells and articulated joint distraction for repair of a chronic osteochondral defect in the rabbit. J. Orthop. Res. 33, 1466– 1473.
Hayashi, S., Nakasa, T., Ishikawa, M., Nakamae, A., Miyaki, S., and Adachi, N. (2018). Histological evaluation of early-phase changes in the osteochondral unit after microfracture in a full-thickness cartilage defect rat model. Am. J. Sports Med. 46, 3032–3039. doi: 10.1177/0363546518787287
Haynesworth, S. E., Goshima, J., Goldberg, V. M., and Caplan, A. I. (1992). Characterization of cells with osteogenic potential from human marrow. Bone 13, 81–88.
Huh, S. W., Shetty, A. A., Kim, S. J., Kim, Y. J., Choi, N. Y., Jun, Y. J., et al. (2014). The effect of platelet rich plasma combined with microfracture for the treatment of chondral defect in a rabbit knee. Tissue Eng. Regen. Med. 11, 178–185.
Hwang, H. D., Lee, J. T., Koh, J. T., Jung, H. M., Lee, H. J., and Kwon, T. G. (2015). Sequential treatment with SDF-1 and BMP-2 potentiates bone formation in calvarial defects. Tissue Eng. Part A 21, 2125–2135. doi: 10.1089/ten.TEA.2014.0571
Ji, W., Yang, F., Ma, J., Bouma, M. J., Boerman, O. C., Chen, Z., et al. (2013). Incorporation of stromal cell-derived factor-1alpha in PCL/gelatin electrospun membranes for guided bone regeneration. Biomaterials 34, 735–745. doi: 10.1016/j.biomaterials.2012.10.016
Kanbe, K., Chiba, J., Inoue, Y., Taguchi, M., and Yabuki, A. (2015). SDF-1 and CXCR4 in synovium are associated with disease activity and bone and joint destruction in patients with rheumatoid arthritis treated with golimumab. Mod. Rheumatol. 26, 46–50. doi: 10.3109/14397595.2015.1054088
Kang, S.-W., Bada, L. P., Kang, C.-S., Lee, J.-S., Kim, C.-H., Park, J.-H., et al. (2008). Articular cartilage regeneration with microfracture and hyaluronic acid. Biotechnol. Lett. 30, 435–439.
Kon, E., Delcogliano, M., Filardo, G., Fini, M., Giavaresi, G., Francioli, S., et al. (2010). Orderly osteochondral regeneration in a sheep model using a novel nano-composite multilayered biomaterial. J. Orthop. Res. 28, 116–124. doi: 10.1002/jor.20958
Krych, A. J., Harnly, H. W., Rodeo, S. A., and Williams, R. J. III (2012). Activity levels are higher after osteochondral autograft transfer mosaicplasty than after microfracture for articular cartilage defects of the knee: a retrospective comparative study. J. Bone Joint Surg. Am. 94, 971–978. doi: 10.2106/JBJS.K.00815
Kucia, M., Reca, R., Miekus, K., Wanzeck, J., Wojakowski, W., Janowska-Wieczorek, A., et al. (2005). Trafficking of normal stem cells and metastasis of cancer stem cells involve similar mechanisms: pivotal role of the SDF-1-CXCR4 axis. Stem Cells 23, 879–894. doi: 10.1634/stemcells.2004-0342
Lee, J.-M., and Im, G.-I. (2012). SOX trio-co-transduced adipose stem cells in fibrin gel to enhance cartilage repair and delay the progression of osteoarthritis in the rat. Biomaterials 33, 2016–2024.
Liu, H., Li, M., Du, L., Yang, P., and Ge, S. (2015). Local administration of stromal cell-derived factor-1 promotes stem cell recruitment and bone regeneration in a rat periodontal bone defect model. Mater. Sci. Eng. C Mater. Biol. Appl. 53, 83–94. doi: 10.1016/j.msec.2015.04.002
Lu, J., Shen, X., Sun, X., Yin, H., Yang, S., Lu, C., et al. (2018). Increased recruitment of endogenous stem cells and chondrogenic differentiation by a composite scaffold containing bone marrow homing peptide for cartilage regeneration. Theranostics 8, 5039–5058. doi: 10.7150/thno.26981
Mahmoud, E. E., Kamei, N., Shimizu, R., Wakao, S., Dezawa, M., Adachi, N., et al. (2017). Therapeutic potential of multilineage-differentiating stress-enduring cells for osteochondral repair in a rat model. Stem Cells Int. 2017, 8154569.
Mazzetti, I., Magagnoli, G., Paoletti, S., Uguccioni, M., Olivotto, E., Vitellozzi, R., et al. (2004). A role for chemokines in the induction of chondrocyte phenotype modulation. Arthritis Rheum. 50, 112–122. doi: 10.1002/art.11474
Mishima, Y., and Lotz, M. (2008). Chemotaxis of human articular chondrocytes and mesenchymal stem cells. J. Orthop. Res. 26, 1407–1412. doi: 10.1002/jor.20668
Mithoefer, K., McAdams, T., Williams, R. J., Kreuz, P. C., and Mandelbaum, B. R. (2009). Clinical efficacy of the microfracture technique for articular cartilage repair in the knee: an evidence-based systematic analysis. Am. J. Sports Med. 37, 2053–2063. doi: 10.1177/0363546508328414
Murata, K., Kitaori, T., Oishi, S., Watanabe, N., Yoshitomi, H., Tanida, S., et al. (2012). Stromal cell-derived factor 1 regulates the actin organization of chondrocytes and chondrocyte hypertrophy. PLoS One 7:e37163. doi: 10.1371/journal.pone.0037163
Oussedik, S., Tsitskaris, K., and Parker, D. (2015). Treatment of articular cartilage lesions of the knee by microfracture or autologous chondrocyte implantation: a systematic review. Arthroscopy 31, 732–744. doi: 10.1016/j.arthro.2014.11.023
Qi, Y., Du, Y., Li, W., Dai, X., Zhao, T., and Yan, W. (2014). Cartilage repair using mesenchymal stem cell (MSC) sheet and MSCs-loaded bilayer PLGA scaffold in a rabbit model. Knee Surg. Sports Traumatol. Arthrosc. 22, 1424–1433. doi: 10.1007/s00167-012-2256-3
Richter, D. L., Schenck, R. C. Jr., Wascher, D. C., and Treme, G. (2016). Knee articular cartilage repair and restoration techniques: a review of the literature. Sports Health 8, 153–160. doi: 10.1177/1941738115611350
Richter, W. (2009). Mesenchymal stem cells and cartilage in situ regeneration. J. Intern. Med. 266, 390–405. doi: 10.1111/j.1365-2796.2009.02153.x
Shi, M., Li, J., Liao, L., Chen, B., Li, B., Chen, L., et al. (2007). Regulation of CXCR4 expression in human mesenchymal stem cells by cytokine treatment: role in homing efficiency in NOD/SCID mice. Haematologica 92, 897–904.
Sun, H., Wang, J., Deng, F., Liu, Y., Zhuang, X., Xu, J., et al. (2016). Co-delivery and controlled release of stromal cell-derived factor-1α chemically conjugated on collagen scaffolds enhances bone morphogenetic protein-2-driven osteogenesis in rats. Mol. Med. Rep. 14, 737–745. doi: 10.3892/mmr.2016.5339
Tampieri, A., Sandri, M., Landi, E., Pressato, D., Francioli, S., Quarto, R., et al. (2008). Design of graded biomimetic osteochondral composite scaffolds. Biomaterials 29, 3539–3546. doi: 10.1016/j.biomaterials.2008.05.008
Toupadakis, C. A., Wong, A., Genetos, D. C., Chung, D. J., Murugesh, D., Anderson, M. J., et al. (2012). Long-term administration of AMD3100, an antagonist of SDF-1/CXCR4 signaling, alters fracture repair. J. Orthop. Res. 30, 1853–1859. doi: 10.1002/jor.22145
Truong, M. D., Choi, B. H., Kim, Y. J., Kim, M. S., and Min, B. H. (2017). Granulocyte macrophage – colony stimulating factor (GM-CSF) significantly enhances articular cartilage repair potential by microfracture. Osteoarthritis Cartilage 25, 1345–1352. doi: 10.1016/j.joca.2017.03.002
Uggen, C., Dines, J., McGarry, M., Grande, D., Lee, T., and Limpisvasti, O. (2010). The effect of recombinant human platelet-derived growth factor BB-coated sutures on rotator cuff healing in a sheep model. Arthroscopy 26, 1456–1462. doi: 10.1016/j.arthro.2010.02.025
Villalvilla, A., Gomez, R., Roman-Blas, J. A., Largo, R., and Herrero-Beaumont, G. (2014). SDF-1 signaling: a promising target in rheumatic diseases. Expert Opin. Ther. Targets 18, 1077–1087. doi: 10.1517/14728222.2014.930440
Wei, L., Kanbe, K., Lee, M., Wei, X., Pei, M., Sun, X., et al. (2010). Stimulation of chondrocyte hypertrophy by chemokine stromal cell-derived factor 1 in the chondro-osseous junction during endochondral bone formation. Dev. Biol. 341, 236–245. doi: 10.1016/j.ydbio.2010.02.033
Wylie, J. D., Hartley, M. K., Kapron, A. L., Aoki, S. K., and Maak, T. G. (2015). What is the effect of matrices on cartilage repair? A systematic review. Clin. Orthop. Relat. Res. 473, 1673–1682. doi: 10.1007/s11999-015-4141-0
Xing, L., Jiang, Y., Gui, J., Lu, Y., Gao, F., and Xu, Y. (2013). Microfracture combined with osteochondral paste implantation was more effective than microfracture alone for full-thickness cartilage repair. Knee Surg. Sports Traumatol. Arthrosc. 21, 1770–1776. doi: 10.1007/s00167-012-2031-5
Zhang, W., Chen, J., Tao, J., Jiang, Y., Hu, C., Huang, L., et al. (2013). The use of type 1 collagen scaffold containing stromal cell-derived factor-1 to create a matrix environment conducive to partial-thickness cartilage defects repair. Biomaterials 34, 713–723. doi: 10.1016/j.biomaterials.2012.10.027
Keywords: osteoarthritis-therapies < cartilage, subchondral arthroplasty, tissue scaffolds, mesenchymal stem cell, microfracture (MFX), stromal cell derived factor-1 (CXCL12), bone marrow stem cell-based therapy
Citation: Mustapich T, Schwartz J, Palacios P, Liang H, Sgaglione N and Grande DA (2021) A Novel Strategy to Enhance Microfracture Treatment With Stromal Cell-Derived Factor-1 in a Rat Model. Front. Cell Dev. Biol. 8:595932. doi: 10.3389/fcell.2020.595932
Received: 18 August 2020; Accepted: 31 December 2020;
Published: 04 February 2021.
Edited by:
Tiago Lazzaretti Fernandes, University of São Paulo, BrazilReviewed by:
David Arthur Hart, University of Calgary, CanadaPedro Debieux, Albert Einstein Israelite Hospital, Brazil
Copyright © 2021 Mustapich, Schwartz, Palacios, Liang, Sgaglione and Grande. This is an open-access article distributed under the terms of the Creative Commons Attribution License (CC BY). The use, distribution or reproduction in other forums is permitted, provided the original author(s) and the copyright owner(s) are credited and that the original publication in this journal is cited, in accordance with accepted academic practice. No use, distribution or reproduction is permitted which does not comply with these terms.
*Correspondence: Taylor Mustapich, dGF5bG9yLm11c3RhcGljaEBlaW5zdGVpbm1lZC5vcmc=