- Institute of Reproductive Health, Tongji Medical College, Huazhong University of Science and Technology, Wuhan, China
Reproduction is an energy demanding function and only take place in case of sufficient available energy status in mammals. Metabolic diseases such as anorexia nervosa are clinically associated with reduced fertility. AMP-activated protein kinase (AMPK), as a major regulator of cellular energy homeostasis, is activated in limited energy reserves to ensure the orderly progress of various physiological activities. In recent years, mounting evidence shows that AMPK is involved in the regulation of reproductive function through multiple mechanisms. AMPK is likely to be a metabolic sensor integrating central and peripheral signals. In this review, we aim to explore the preclinical studies published in the last decade that investigate the role of AMP-activated protein kinase in the reproductive field, and its role as a target for drug therapy of reproductive system-related diseases. We also emphasized the emerging roles of AMPK in transcriptional regulation of reproduction processes and metabolisms, which are tightly related to the energy state and fertility of an organism.
Introduction
AMP-activated protein kinase (AMPK) is a heterotrimeric complex composed of a catalytic α-subunit and two regulatory subunits: β and γ. The most well-known physiological function of AMPK is to act as an energy sensor to maintain metabolic homeostasis (Hardie, 2007). In response to decreased ATP/AMP ratio or glucose starvation, the liver kinase B1 (LKB1) phosphorylates the threonine-172 residue of AMPK and mediates its activation through canonical and non-canonical mechanisms. These two activation mechanisms occur in the cytoplasm and lysosome, respectively (Gonzalez et al., 2020). Furthermore, hormones and DNA damage can also trigger AMPK activation by the Ca2+/calmodulin-dependent kinase, CaMKK2 (Gonzalez et al., 2020). After activated, AMPK switches off ATP-consuming pathways such as protein synthesis, glycogenolysis, and lipogenesis, and turns on ATP-generating pathways such as fatty acid oxidation, glycolysis, and autophagy by regulating downstream factors, thereby ensuring nutrient supply (Hardie, 2007).
It is known that mammalian reproduction is an energy-consuming process that occurs when there is adequate nutrition (Dupont et al., 2014). Metabolic disorders are clinically associated with fertility decline. For instance, polycystic ovary syndrome (PCOS) is a common reproductive disorder that causes infertility in women of childbearing age often accompanied by insulin resistance and hyperandrogenemia (Diamanti-Kandarakis, 2008). Besides, anorexia nervosa or obesity may cause impaired ovarian function and spermatogenesis (Group, 2006; Pasquali, 2006; Mah and Wittert, 2010). Therefore, the relationship between reproduction and energy metabolism has attracted extensive attention. The properties of AMPK as an energy sensor allow it to couple the energy status of the body to reproductive function (Duval, 2011). Hypothalamic AMPK is central to energy homeostasis (Huynh et al., 2016). Both anorectic signals (leptin, insulin) and orexigenic signals (ghrelin, neuropeptide Y) affect energy intake and energy expenditure through the regulation of hypothalamic AMPK activity (Minokoshi et al., 2004), thereby ensuring the orderly progress of various physiological activities. During nutrient starvation, AMPK, as a catabolic enzyme, promotes cellular catabolism and augments energy production through the inhibition of mTOR (mammalian target of rapamycin) by directly phosphorylating the TSC2 (tumor suppressor Tuberous Sclerosis Complex 2) and RAPTOR. Under nutrient-rich conditions, the inhibition of mTOR is diminished and the whole-body energy balance is positive, it is conducive to reproduction (Gonzalez et al., 2020). In addition to the above indirect effects on reproduction, AMPK in the hypothalamus and pituitary is able to directly regulate gonadal steroid hormones production and affect fertility in response to peripheral metabolic signals (Tulipano, 2020). However, the global physiological actions of AMPK in reproduction include not only the acute response of AMPK activation through directly phosphorylating downstream metabolic enzymes or regulating the hypothalamic pituitary gonadal (HPG) axis, but also AMPK-mediated transcriptional events via affecting the interaction between transcription regulators (TFs) and their DNA recognition sites.
In this review, we aim to explore the preclinical studies published in the last decade that investigate functions of AMP-activated protein kinase in the reproductive field, and its role as a target for drug therapy of reproductive system related diseases. We also discussed the emerging roles of AMPK in transcriptional regulation of reproduction processes and metabolisms, which are tightly related to the energy state and fertility of an organism.
AMPK Is Involved in the Modulation of the Hypothalamic Pituitary Gonadal (HPG) Axis
The HPG axis is of vital importance in mammalian reproductive system (Schneider, 2004). The secretion and gene transcription of the pituitary gonadotropins luteinizing hormone (LH) and follicle-stimulating hormone (FSH) are driven by pulsatile release of gonadotropin-releasing hormone (GnRH) from neurons in the hypothalamus. Gonadotropins control gonadal steroid hormones production and in turn sex hormones exert feedback regulation on GnRH, LH and FSH synthesis and secretion (Burger et al., 2004). In response to energy insufficiency, the bodies usually choose to reduce all the dispensable energy expenditure in mammals. Therefore, GnRH pulsatility was suppressed, and fertility decreased to allow energy accumulation for individual survival. That is to say, there must be some signaling pathways in the center that serve as a bridge to link the diminished energy reserves with the reproductive neuroendocrine axis. In fact, hypothalamic AMPK activity is tightly related to feeding and thermogenesis, thus controlling the energy homeostasis of the organism (Lim et al., 2010; Wang and Cheng, 2018). It is worth noting that AMPK is highly expressed in those nuclei associated with reproductive control in the hypothalamus, such as the paraventricular and arcuate nuclei (Torsoni et al., 2016). Hence, AMPK pathways in the hypothalamus are likely to mediate the effects of peripheral signals on reproductive function. Kisspeptin, a protein encoded by the Kiss1 gene, can promote GnRH secretion and affect puberty after binding with its receptor G-protein-coupled receptor-54 (GPR54) (Seminara et al., 2003; Pinilla et al., 2012; Herbison, 2016). Evidence is accumulating that AMPK activation inhibits GnRH release in GT1-7 cell, a mouse immortalized hypothalamic GnRH neuron (Coyral-Castel et al., 2008; Wen et al., 2008, 2012). On the one hand, AMPK activation induced by adiponectin represses the promoter activity of the Kiss1 gene via inhibition of the translocation of specificity protein-1 (SP1) from the cytoplasm to the nucleus and subsequently influences GnRH secretion (Wen et al., 2012). On the other hand, the trafficking and exocytosis of secretory vesicles is ATP-dependent (Nagiec et al., 1995). As a consequence, it is plausible that AMPK may be activated to reduce GnRH secretion to increase ATP levels in limited fuel availability. However, these experiments were carried out in immortalized cells while GnRH neurons themselves do not express Kiss1 (Pinilla et al., 2012), so the reliability of these results needs to be further confirmed. In previous years, two clinical studies have observed that metformin treatment at early stage could delay menarche and ameliorate metabolic disorder in girls with precocious pubarche (Ibanez et al., 2011a,b). A paper published in 2018 documented that the regulatory effect of AMPK activation on adolescent metabolic control under negative energy balance was achieved by reducing kisspeptin expression in hypothalamic KISS1 neurons (Roa et al., 2018). Conditional deletion of the AMPKα1 subunit in Kiss1 cells largely prevented the delay in puberty onset caused by malnutrition (Roa et al., 2018). Other AMPK subunits may be involved in the partial compensation of the lack of AMPKα1 as the AMPKα2 catalytic subunit in Kiss1 neurons has been reported to be responsible for the reproductive adaptations to acute metabolic distress (Torsoni et al., 2016).
Notably, the different subunits of AMPK are also expressed in the pituitary (Tosca et al., 2011) and the role of pituitary gonadotroph cells as a metabolic sensor to integrate energy status with fertility has been known (Duval, 2011). Clinically, PCOS is characterized by elevated LH to FSH ratios, but metformin treatment can reduce serum LH concentration in PCOS women (Velazquez et al., 1994; Genazzani et al., 2004; Oride et al., 2010). It is likely that AMPK activation mediates the effect of metformin on pituitary gonadotropin-secreting cells (Duval, 2011). In mouse LβT2 cells (mouse pituitary cell), inhibition of AMPK activity or reduction of AMPK levels prevented GnRH-stimulated LH gene transcription (Andrade et al., 2013). Interestingly, Andrade et al. reported that AMPK effects are mediated at least in part by diminishing the EGR-1 protein level (EGR-1 is a transcription factor required for LH expression and induced to synthesize in response to GnRH) (Andrade et al., 2013). However, this outcome is contrary to that of Lu et al. (2008) who found that AMPK activation by adiponectin reduces GnRH-stimulated LH secretion, and this repression can be mimicked by AICAR (5-aminoimidazole-4-carboxamide riboside), an activator of AMPK. In rat pituitary cells, metformin-induced AMPK activation strongly decreases LH and FSH in response to GnRH and FSH release induced by activin via inhibiting the MAPK3/1 and SMAD2 signaling pathways, respectively (Tosca et al., 2011). Subsequently, these alterations in gonadotropin secretion would likely lead to changes in steroid synthesis in the gonad. Unlike previous studies using rodent cells, metformin can only inhibit basal, but not GnRH-stimulated FSH secretion, without altering LH release in non-human primate pituitary cells in vitro (Vazquez-Borrego et al., 2018; Figure 1). These inconsistent conclusions may be due to differences in species or experimental methods, indicating that further work is required to establish the viability of exploring the effect of AMPK on the HPG axis in vivo and in vitro.
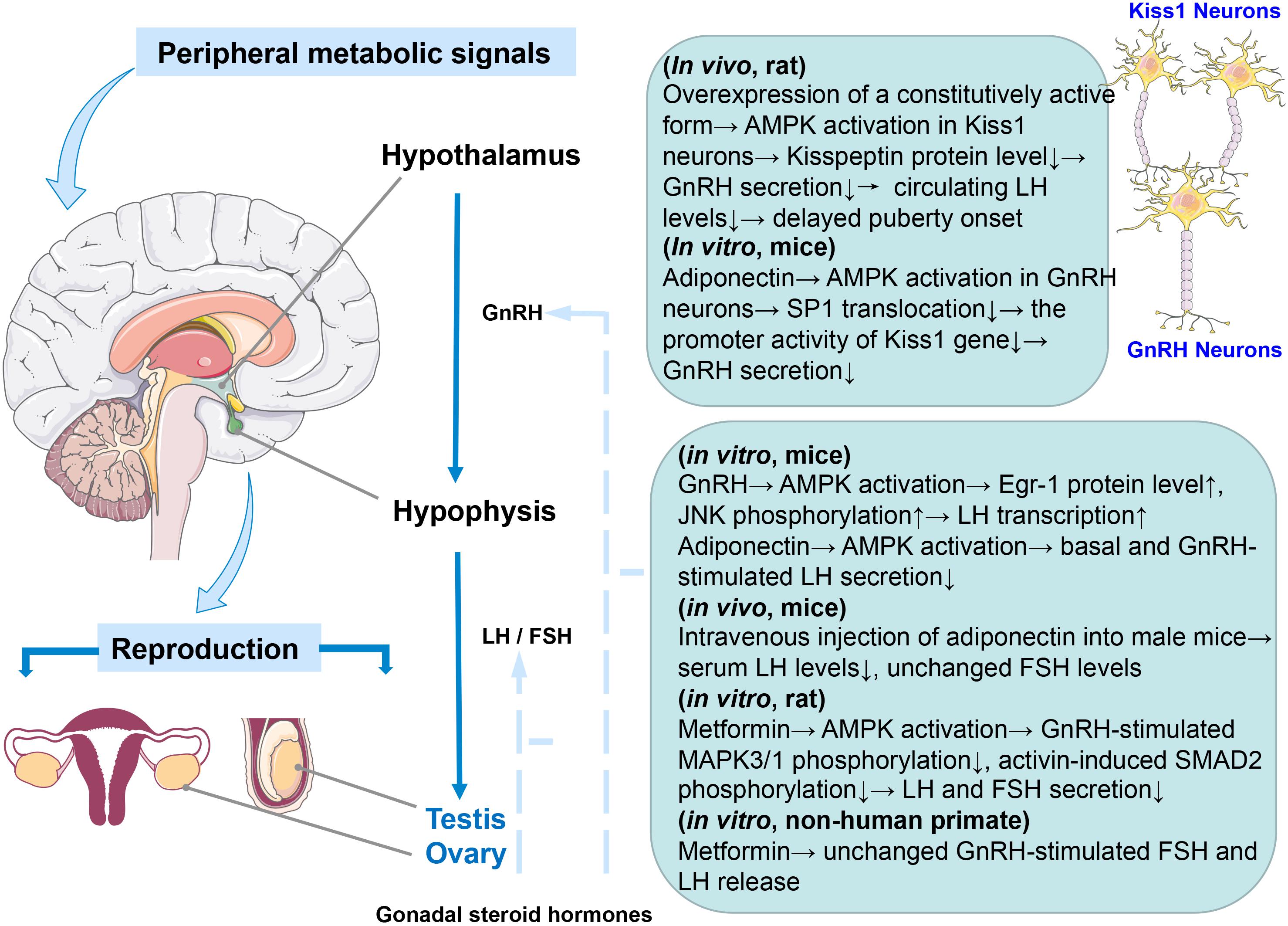
Figure 1. Graphical summary of the effect of AMPK activation on the HPG axis. Species and in vivo or in vitro experiments are notified.
AMPK in Male Reproduction
It is well known that the Liver Kinase B1 (LKB1), located upstream of AMPK, regulates AMPK activity (Lizcano et al., 2004). In humans, LKB1 gene mutation usually results in Peutz-Jeghers syndrome (PJS) and male infertility (Ulbright et al., 2007). Likewise, loss of LKB1 signaling in mice affects the release of mature spermatids into the seminiferous tubules (Denison et al., 2011). Additionally, genetically deleted testis-specific serine kinase (TSSK) family genes, which also belong to the AMPK branch, leading to male infertility due to haploinsufficiency (Xu et al., 2008). Thus, the fact that AMPK-related genes’ defects induce a reduction in male fertility and the widespread expression of AMPK in various testicular cells suggests its essential role in male reproduction. We will focus on the functions of AMPK in male gonadal somatic cells and spermatogenesis to summarize as following sections.
The Effect of AMPK on Male Gonadal Somatic Cells
Male gonadal somatic cells contain two types of cells, Sertoli and Leydig cells. Sertoli cells protect and nourish developing germ cells, and the cell junction between Sertoli cell formed near the basement membrane of seminiferous epithelium constitutes the blood-testis barrier (BTB), providing a suitable microenvironment for spermatogenesis and its maturation. Additionally, Leydig cells can synthesize and secrete androgen to promote spermatogenesis (Gerber et al., 2016). Taken together, spermatogenesis is inseparable from the normal function of Sertoli and Leydig cells. Tanwar et al. (2012) reported that conditional deletion of LKB1 in Sertoli cells of mice resulted in germ cell loss and Sertoli cell-only seminiferous tubular phenotype, which is similar to that of human PJS patients (Ulbright et al., 2007). Some seminal researches have previously shown that LKB1 (Baas et al., 2004) and its downstream effector AMPK are responsible for establishing apicobasal cell polarity (Forcet et al., 2005; Lee et al., 2007) and AMPK activation reinforces tight junction function through directly phosphorylating its substrates in apical junctional complexes (Lee et al., 2007; Zhang et al., 2011; Shiomi et al., 2015; Aznar et al., 2016) or driving key epithelial transcription factors (Sun et al., 2017). Thus, it is no surprise that the LKB1 knockout mice also show defects in Sertoli cell polarity and testicular junctional complexes, which indicates the involvement of AMPK-mediated mTOR inhibition in this biological process (Tanwar et al., 2012). It is well known that the maintenance of Sertoli cell polarity and their tight junction, an integral part of the BTB, are essential for male germ cell development and spermatogenesis. The barrier functions as a “fence” to prevent the diffusion of harmful substances and the escape of sperm antigen. Autoimmune orchitis is one of the diseases caused by barrier dysfunction (Perez et al., 2012). However, the AMPK-mediated epithelial barrier repair in a pulmonary microvascular endothelial cell wound healing model makes it a possible drug target (Creighton et al., 2011). To date, the protective effect of metformin-induced AMPK activation on epithelial barrier dysfunction in different tissues has been discovered in succession (Seo-Mayer et al., 2011; Spruss et al., 2012; Liu et al., 2014; Xue et al., 2016; Chen et al., 2018). Sertoli cells, which are closely linked to germ cells, engulf the extra cytoplasm of elongated spermatids before their release and play a key role in shaping the head of the spermatozoon (Sakai and Yamashina, 1989). Loss-function of AMPKα1 in mouse Sertoli cells causes a decrease in male fertility with abnormal sperm head morphology due to destroyed tight junction between Sertoli cells and germ cells (Bertoldo et al., 2016). In addition, AMPK activity can influence the intracellular metabolism and cell proliferation of Sertoli cells. Galardo et al. (2007) found that stimulation of rat Sertoli cells with AICAR treatment increase lactate production through several biochemical mechanisms, including promoting glucose transport and the conversion of pyruvate to lactate as well as improving lactate export to germ cells, suggesting a role for AMPK modulating the nutritional function of Sertoli cells. Specific deletion of AMPKα1 in Sertoli cells showed an increase in lipid droplets (Bertoldo et al., 2016), which is consistent with cytoplasmic vacuolization of Sertoli cells in human PJS patients (Ulbright et al., 2007). Paradoxically, there was no change in the number of Sertoli cells when AMPKα1 inactivated in vivo, but inactivation of AMPKα1 in vitro promoted Sertoli cell proliferation (Bertoldo et al., 2016). This is supported by a series of other in vitro experiments. For instance, administration of AMPK activator metformin to human and murine organotypic testes cultured in vitro led to a decrease in the number of Sertoli cells (Tartarin et al., 2012b). When pregnant mice or sexually immature chicken were exposed to metformin, loss of testis weight, and decreased proliferative activity of Sertoli cells can be observed (Tartarin et al., 2012b; Faure et al., 2016). These studies suggested that AMPK activation could inhibit cell division during Sertoli cell development. Another intriguing observation was that despite the number of aforementioned Sertoli cells of chicken was reduced, the population of spermatogonia was unchanged (Faure et al., 2016). Besides, the AMPK α1 subunit is expressed in somatic cells (Sertoli and Leydig cells) (Tartarin et al., 2012a). AMPK α1 KO male mice show a decrease in fertility, with an increased mean number of Leydig cells (Tartarin et al., 2012a). The smooth endoplasmic reticulum activity was enhanced, and testosterone synthesis increased in AMPK α1 KO testes. Owing to the negative feedback, the serum LH and FSH concentrations became lower (Tartarin et al., 2012a). In rat Leydig cells, the AMPK activator resveratrol reduces the secretion of testosterone by affecting the transport of cholesterol into mitochondria and conversion of progesterone into androstenedione (Svechnikov et al., 2009). This is in line with androgen reduction in organotypic testis cultured in vitro with metformin stimulation (Tartarin et al., 2012b). Therefore, the above-mentioned data strongly suggest that AMPK inhibits the production of androgen in Leydig cells and thus affects spermatogenesis.
The Function of AMPK in Spermatogenesis
Spermatogonia undergo two rounds of meiosis and subsequent morphological change to develop into a spermatozoon during mammalian spermatogenesis (Mruk and Cheng, 2015). Ejaculated spermatozoa do not yet have the ability to complete egg fertilization in mammals. They need to go through some cellular processes in the female genital tract, including capacitation and acrosome reaction, and eventually fertilize the oocytes (Castillo et al., 2019). At this time, spermatozoa have already lost corresponding organelles to allow transcription and translation of genes, so the acquisition of these physiological abilities must rely on the modification of existing proteins (Castillo et al., 2019). AMPK and its phosphorylated form are expressed in a variety of mammalian sperm and mainly localized at the acrosome and at the mid-piece of flagellum (Martin-Hidalgo et al., 2018). Spermatozoa motility depends on flagellum beating, and mitochondria exist in the middle of the flagellum to provide energy for the movement (Gu et al., 2019). AMPK activity has been reported to be essential for spermatozoa motility. For example, AMPK inhibitor compound C (CC) treatment reduces a potent decrease in the number of mobile spermatozoa, and those remnant active spermatozoa move with significantly lower speed, but the use of CC itself does not impair sperm viability (Hurtado de Llera et al., 2012). Interestingly, AMPK activator A769662 does not improve spermatozoa motility (Hurtado de Llera et al., 2015), indicating that AMPK activity needs to fluctuate within a specific physiological range, and excessive inhibition or activation is not conducive to maintaining optimal sperm motility. In addition, the AMPKα1 knockout mice presented a reduced number of pups per litter due to decreased mitochondria number and their abnormal arrangement along microtubules in KO spermatozoa (Tartarin et al., 2012a). Similarly, specific deletion of Tssk2 in mice can also affect spermatozoa motility since it phosphorylates a protein of the axoneme central apparatus in the flagellum (Zhang et al., 2008). These studies raise the possibility that AMPK phosphorylates specific proteins in the flagellum, thereby influencing sperm motility.
Mitochondrial function is a prerequisite for sperm to achieve flagellum swing (Gu et al., 2019). The mitochondrial membrane potential (ΔΨm) is generally used to reflect mitochondrial function (Sakamuru et al., 2016). Inhibition of AMPK activity in boar spermatozoa by CC treatment resulted in instability of ΔΨm and plasma membrane lipid disorganization (Hurtado de Llera et al., 2013). Plasma membrane disorder is most likely to occur at the apical part of the acrosome, where a majority of phosphorylated AMPK protein accumulates, leading to impaired acrosomal integrity (Hurtado de Llera et al., 2013). Male germ cells will encounter various metabolic stresses in the process of transit through the female genital tract or semen cryopreservation (Huang et al., 2015). In this case, the integrity of sperm structure and intracellular adaptive physiological responses, such as AMPK regulating cell metabolism when ATP is limited, are required for successful fertilization. A study has reported that intracellular messengers Ca2+and cAMP, as well as PKA, PKC, and CaMKKα/β signaling pathways are involved in the activation of AMPK in boar spermatozoa in response to a variety of physiological or pharmacological stimuli (Hurtado de Llera et al., 2014).
Data shows a decline in sperm quality among men of different races (Levine et al., 2017; Mishra et al., 2018), and in order to improve fertility, semen cryopreservation has become a fundamental part of assisted reproductive techniques (ARTs) during recent years. However, the freeze-thaw process has detrimental effects on the biological functions of spermatozoa. It can decrease the percentage of mobile spermatozoa and dramatically increase reactive oxygen species (ROS) generation and the percentage of apoptotic-like sperm cells (Shabani Nashtaei et al., 2017). Since sperm membranes are rich in polyunsaturated fatty acids (Dandekar et al., 2002), they are very susceptible to lipid peroxidation. Although there are different kinds of antioxidant enzymes in semen to protect sperm from lipid peroxidation (Aitken et al., 1996; Gadea et al., 2004), the freeze-thaw process has detrimental effects on enzyme activity and decreases the scavenging capacity of seminal plasma (Lasso et al., 1994; Bilodeau et al., 2000). AMPK activation of chicken sperm before cryopreservation enables sperm to store more ATP, which helps to restore antioxidant enzyme activity to remove ROS and limit lipid peroxidation, thus improving sperm motility and acrosome reaction, and ensuring a better quality of cryopreserved sperm (Nguyen et al., 2015). Moreover, considering that cryopreservation-induced ROS can generate a significant DNA damage in some genome regions (Thomson et al., 2009; Aitken and De Iuliis, 2010) and successful fertilization requires the sperm normal DNA integrity (Zini et al., 2008), it is important to use antioxidants to reduce oxidative DNA damage (Agarwal et al., 2004). In human frozen-thawed sperm, AMPK activator resveratrol (RSV), a known antioxidant, alleviated oxidative stress induced by cryopreservation and at least partially restored some functional properties of sperm, while CC supplementation showed an opposite effect (Shabani Nashtaei et al., 2017). Subsequent published paper showed that the addition of RSV could protect sperm DNA integrity and key paternal transcripts from the adverse effects of cryopreservation by enhancing the activity of AMPK (Shabani Nashtaei et al., 2018). The protective mechanism of AMPK activation on cryopreservation-induced DNA damage in human spermatozoa still remains obscure, which may be associated with the role of AMPK in autophagy (Li et al., 2017) or DNA damage repair (Hashimoto et al., 2018; Chen et al., 2020). These results indicates that AMPK is a drug target for optimizing sperm cryopreservation protocol and AMPK activity is crucial to protect sperm from lipid peroxidation and ROS (Figure 2).
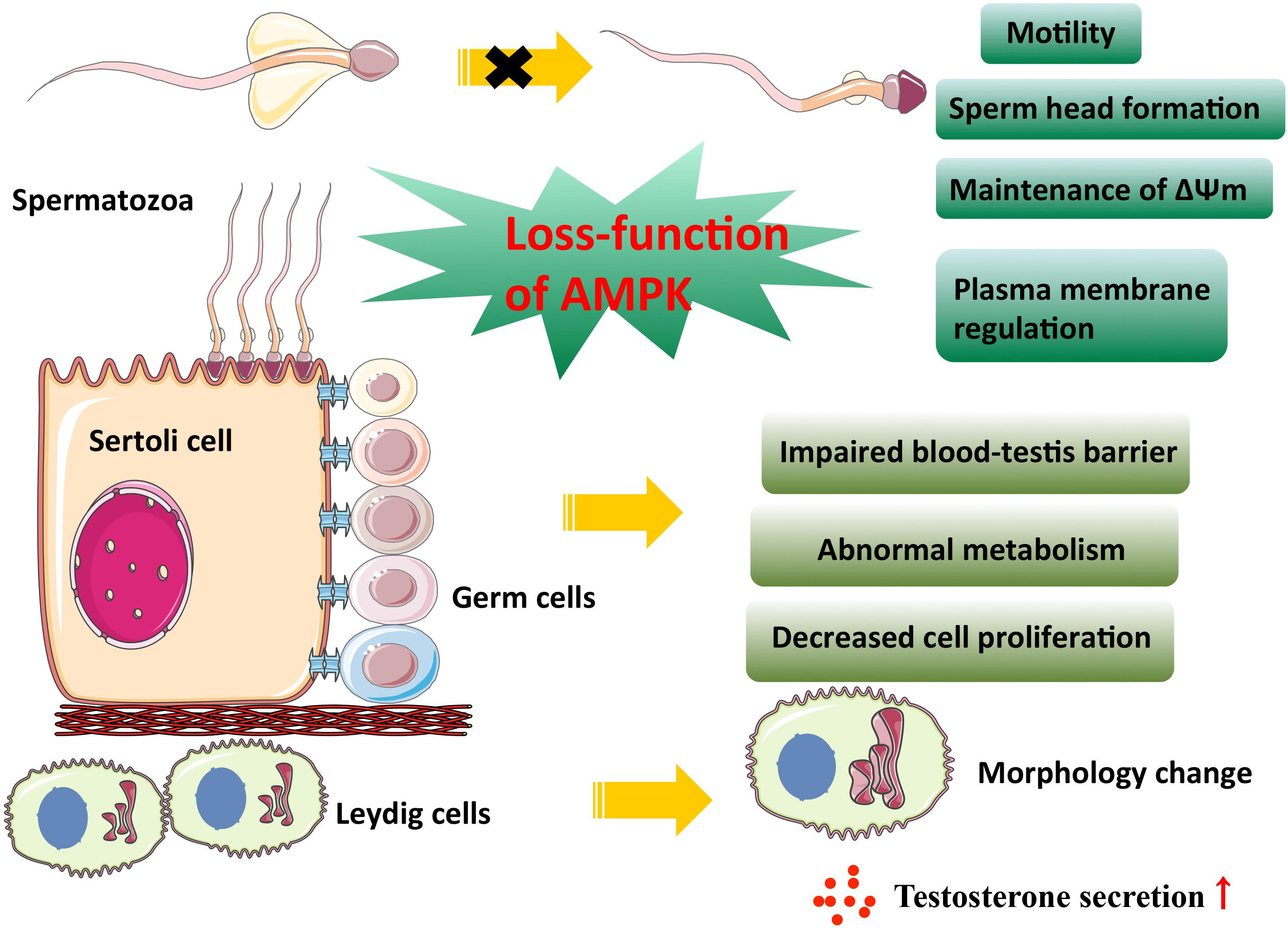
Figure 2. AMPK activity in male reproduction. This figure summarizes the effect of AMPK on male gonadal somatic cells and spermatogenesis. As is shown above, the loss-function of AMPK leads to the impaired physiological function of somatic cells and spermatozoa.
AMPK in Female Reproduction
Mammalian oogenesis begins in the fetus. The primordial germ cells proliferate and differentiate into oogonia, and undergo the first meiosis, reaching diplotene at birth known as a germinal vesicle (GV). When the estrus cycle arrives, the oocytes continue the first meiotic division, including germinal vesicle breakdown (GVBD), emitting the first polar body, and stopping at the middle stage of the second meiosis which won’t be completed until successful fertilization (Sathananthan et al., 2006). Both folliculogenesis and embryo development require energy. As a central regulator of energy homeostasis, AMPK is closely associated with female reproduction, including follicular development, granulosa cell proliferation, and pregnant regulation.
Role of AMPK in Follicular Development
The mammalian ovary is a heterogeneous organ containing follicles at various developmental stages, and its principal function is the release of the mature oocyte for fertilization (McGee and Hsueh, 2000). Although many follicles exist in the ovary, most of them are primordial follicles, which consist of an immature oocyte and several surrounding flattened somatic cells called primordial follicle granulosa cells (McGee and Hsueh, 2000). In mammals, the original pool of primordial follicles is the only source of all developing follicles for the entire reproductive life. The prerequisite for successful fertilization is the activation of primordial follicles. AMPK protein is reported to be expressed in mammalian ovaries, including oocytes, granulosa cells, theca cells, and corpus luteum (Tosca et al., 2005, 2007a; Lu et al., 2017). A large number of researches show that AMPK is involved in the regulation of primordial follicle activation (Lu et al., 2017). In situ ovarian intramural administration of AMPK inhibitor CC in mice, primordial follicle activation and stimulation of follicle growth were observed, and thus more healthy pups were delivered (Lu et al., 2017). AMPK inhibition by CC promotes the growth of cultured ovary in vitro by activating mTOR and increasing the expression of Ctgf in the YAP signaling pathway (Lu et al., 2017). Importantly, conditional knockout of LKB1 or mTOR inhibitor gene TSC1 in mouse oocytes caused severe subfertility and the pool of primordial follicles exhausted due to early excessive activation (Jiang et al., 2016). In comparison, specific deletion of TSC1 in mouse granulosa cells does not compromise mouse fertility, and the conditional knockout mice can produce more ovulating oocytes (Huang et al., 2013). This phenotype could be explained by increased mTORC1 activity, while rapamycin as a specific mTORC1 inhibitor effectively reversed the condition (Huang et al., 2013; Cheng et al., 2015; Jiang et al., 2016). In addition to using the knockout mouse models in vivo, researchers utilized mammalian oocytes by in vitro maturation (IVM) technique to investigate the effect of AMPK activation on follicular development. AMPK activation using AICAR or other activators in vitro can delay oocyte maturation in swine cumulus-oocyte complexes (COCs), but only in the presence of cumulus cells (Santiquet et al., 2014). Cumulus cells are necessary for promoting the inhibitory effect of AMPK activation on the recovery of swine and bovine oocyte meiosis (Santiquet et al., 2014). During IVM, the level of AMPK phosphorylation gradually decreased in immature bovine oocytes and cumulus cells. The AMPK activator metformin blocks most oocytes in the GV stage by inhibiting the activation of key factors involved in protein synthesis, while CC reduced AMPK phosphorylation in COCs and accelerated the resumption of oocyte meiosis (Tosca et al., 2007b). However, metformin did not inhibit the resumption of denuded oocytes meiosis, suggesting that the inhibition of oocyte maturation by metformin requires the presence of cumulus cells (Tosca et al., 2007b). Communication between the oocyte and cumulus cells is supported by gap junctions, which are important for controlling oocyte maturation. In bovine COCs, AMPK also exists in the gap junctions (Bilodeau-Goeseels et al., 2007). Therefore, the occurrence of oocyte maturation is highly likely to need signals induced by AMPK inhibition from cumulus cells that transmit through gap junctions. Intriguingly, phospho-AMPK provides a meiotic maturation signal and induces oocyte maturation in mice (Downs et al., 2002; Chen et al., 2006). The activation of AMPK not only promotes GVBD in mouse oocytes but also participates in the regulation of the progression of meiosis to MII (Downs et al., 2010; Table 1). More studies will be needed to determine the role of AMPK in follicular development as it appears there are significant differences between species.
AMPK in Granulosa Cell Secretion and Proliferation
It is well known that FSH and IGF-I regulate the growth and differentiation of granular cells in mice (Baker et al., 1996; Zhou et al., 1997; Abel et al., 2000). Mice lacking IGF-I had stunted follicular development and were unable to produce Graafian follicles successfully (Baker et al., 1996; Zhou et al., 1997). Adashi et al. (1986) demonstrated that FSH and IGF-I could synergistically act on the MAPK ERK1/2 pathway in granular cells to increase progesterone secretion. In rat and bovine, when primary granulosa cells treated with AICAR or metformin, the activation of AMPK inhibited phosphorylation of MAPK ERK1/2 and affected the expression of 3β-hydroxysteroid dehydrogenase (3β-HSD) and the steroidogenic acute regulatory (StAR) protein, resulting in decreased progesterone secretion (Tosca et al., 2005, 2007a). Besides, metformin can inhibit the proliferation and protein synthesis of bovine granulosa cells through an AMPK-dependent mechanism (Tosca et al., 2006). Similarly, metformin treatment could block the expansion of bovine cumulus cells in vitro (Tosca et al., 2007b).
AMPK in Mammalian Pregnancy Regulation
A healthy fetus delivery demands uterine receptivity increase and normal placenta in the maternal uterus to allow embryo implantation. AMPK is commonly expressed in the all-female reproductive system (McCallum et al., 2018), suggesting that AMPK is essential for normal pregnancy in females. AMPK activation has been shown to reduce fibrosis or scar mature failure after injuring the liver, heart, kidney, intestine, and peritoneum (Noppe et al., 2014; Chen et al., 2017; Cieslik et al., 2017; Shin et al., 2017). Similarly, the conditional deletion of AMPKα in mouse uteri failed scar-free regeneration of the endometrium after delivery and severe endometrial fibrosis, thereby causing embryo implantation failure and lifetime fecundity reduction (McCallum et al., 2018). Another latest report supports the role of AMPK in establishing uterine receptivity and demonstrates that AMPK activity plays a prominent part in epithelial cell proliferation and decidualization (Griffiths et al., 2020). In addition, pharmacological AMPK activation can promote uterine artery vasodilation, which indicates that AMPK activation may help to maintain uteroplacental blood flow, thereby ensuring a normal pregnancy process (Skeffington et al., 2016). AMPK is also required for the growth and differentiation of mammalian embryos. Double knockout of PRKAA1 and PRKAA2 in mice resulted in embryonic lethality around 10.5 days of gestation (Viollet et al., 2009). However, AMPK activation beyond the normal physiological range is detrimental to optimal preimplantation embryo development. Applying AICAR to mouse 2-cell embryos decreases blastocyst formation and inhibits trophectoderm differentiation. AICAR treated embryos displayed altered blastocyst formation gene expression and increased tight junction permeability, which is irreversible. This effect is confirmed with other AMPK activators, metformin and phenformin (Calder et al., 2017). The result suggested that AMPK activity must be tightly controlled to facilitate normal preimplantation development and blastocyst formation. Given that there is no evidence to ensure the harmlessness of metformin for fetal development, the use of metformin during pregnancy should be carefully considered. A study reported that the inhibition of AMPK activity in trophoblast stem cells blocked their normal differentiation under cellular stress (Zhong et al., 2010). After knocking down AMPKα1 and α2 in the mouse trophoblast progenitor cell line SM10, the researchers observed an impaired proliferation and differentiation of progenitor cells (Carey et al., 2014). Glucose is a primary energy source for the growing baby, but after knockdown AMPKα, the glucose transportability in progenitor and differentiated labyrinthine cells decreased. This finding is consistent with a study showing that AMPK regulates Glut3 transporters’ movement toward the plasma membrane and thus increases glucose transport in neurons (Weisova et al., 2009). In summary, AMPK impacts various aspects of normal pregnancy in females, not only as an energy sensor but also participates in the precise regulation of tissue growth and differentiation (Figure 3).
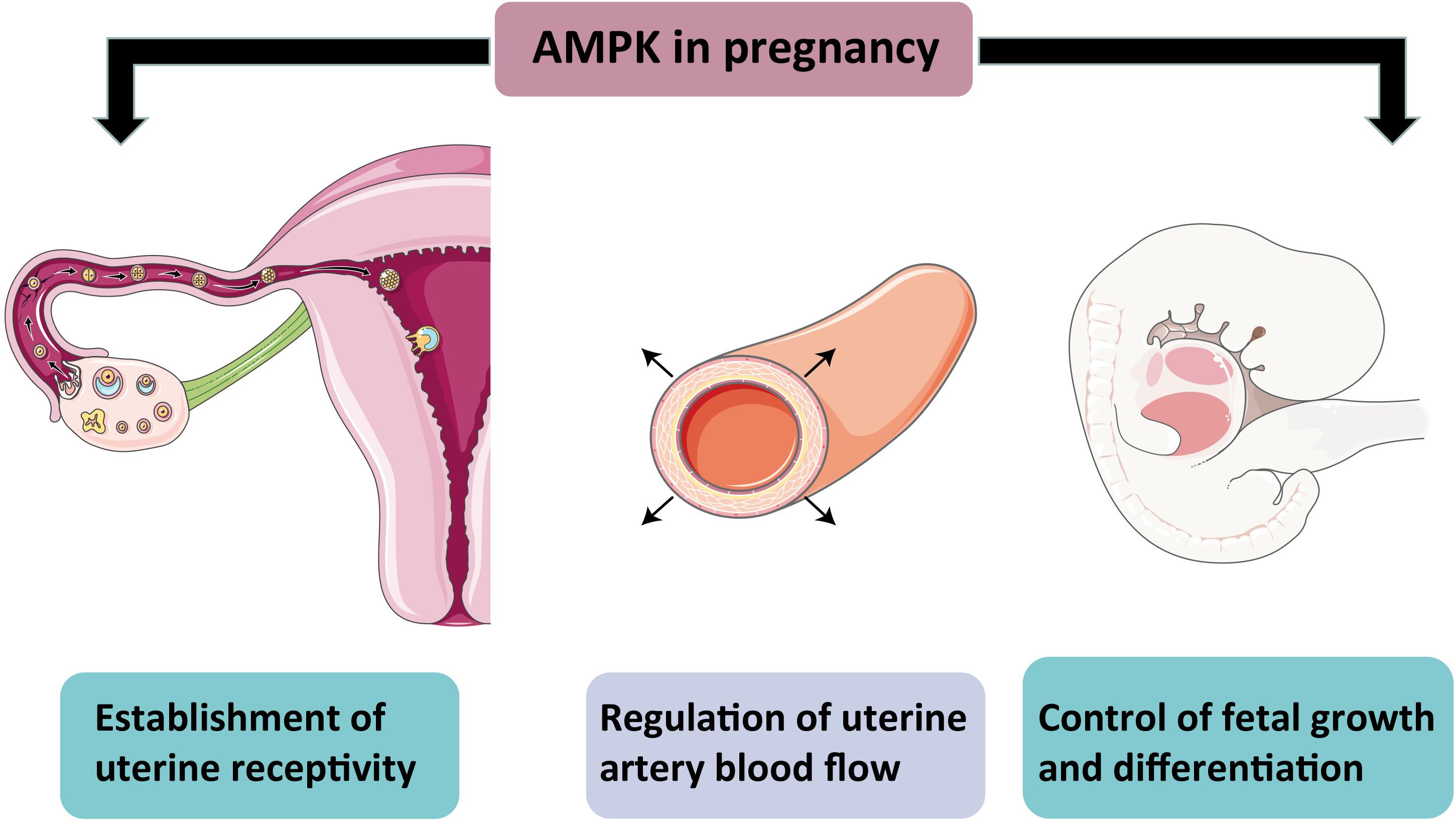
Figure 3. Schematic representation of AMPK action in pregnancy. The diagram shows the importance of AMPK for a normal pregnancy. AMPK activity helps establish uterine receptivity and regulates arterial blood flow and controls normal fetal growth and development.
AMPK in Genital Diseases
Genital Cancers
The American Cancer Society reported that there were 317, 260 genital cancers cases diagnosed with a mortality rate of 21.3% in 2019 in the United States alone, which seriously endangers human reproductive health (Siegel et al., 2020). Currently, there are many therapeutic regimes for genital malignancy, but recurrence always tends to occur, and the overall prognosis for patients remains still poor. Hence, developing novel molecular targets for cancers is imperatively needed. LKB1 is the product of the tumor suppressor gene STK11. Mutation of LKB1/STK11 leads to PJS, and such patients have an increased risk of several cancers (Hearle et al., 2006). As a classical AMPK upstream kinase, blocking LKB1 activation in rat liver cells significantly abolished AMPK activation (Woods et al., 2003). The close correlation between LKB1 and AMPK reminds us that AMPK may regulate the tumor-suppressive effect of LKB1. Clinically, metformin is often used in combination with other anticancer drugs to reduce cancer incidence and achieve better chemotherapy outcomes (Gadducci et al., 2016), suggesting that AMPK may be involved in carcinogenesis. Several studies have reported that AMPK protein functions in genital system cancers. For example, it is reported that 20% of cervical cancer have LKB1 gene mutation (Wingo et al., 2009). Only those cervical cancer cells harboring intact LKB1-AMPK-mTOR signaling are sensitive to the administration of metformin (Xiao et al., 2012). Higher expression of AMPK correlated with a smaller-size cervical tumor (Choi et al., 2015). Therefore, the above studies showed that the activation of AMPK is critical for retarding cervical cancer cell growth. In addition, AMPK activation could induce apoptosis of ovarian cancer cells and delay endometrial cancer progression by inhibiting the mTOR and/or AKT pathways (Erdemoglu et al., 2009; Xie et al., 2011; Yung et al., 2016), which suggesting AMPK appears to serve as a tumor suppressor and exhibits an anti-cancer effect on genital system cancers. Although the principle how of AMPK plays an anti-cancer effect is far from understood, researches on the role of AMPK in cell-cell junction and cell cycle shed light on its other possible mechanisms. AMPK-mediated regulation of apicobasal polarity establishment and tight junction assembly (Forcet et al., 2005; Zhang et al., 2006; Lee et al., 2007) are important to maintain cell normalization and defect in this process increases tumor cell growth (Aznar et al., 2016). It’s now widely accepted that cell cycle arrest is associated with the anti-tumorigenic effect. AMPK can promote a cell cycle arrest at the level of G1 and G2 by directly phosphorylating and thus stabilizing p53 (Imamura et al., 2001; Jones et al., 2005). P53 is a key to understand how AMPK affects proliferation and its regulation by AMPK also reveals the link between metabolism and cell cycle. However, during tumor development processes, cancer cells are often in a state of metabolic stress (hypoxia, hormone deficiency, or radiochemotherapy, etc.), which activates a stress-response molecule AMPK, inhibiting anabolism and promoting catabolism to provide ATP, helping cancer cell escape the crisis (Park et al., 2009). When androgen-dependent prostate cancer cells are subjected to androgen deprivation or hypoxia, AMPK activation induces autophagy, degrading intracellular organelles, and providing sufficient nutrients for the survival of cancer cells (Chhipa et al., 2010). Thus, AMPK activation-induced autophagy is a protective survival mechanism for androgen-dependent prostate cancer cells in a harsh living environment, promoting prostate cancer cells’ transformation into an androgen-independent phenotype (Chhipa et al., 2011). Although AMPK activity has been repeatedly reported to be related to prostate cancer cell growth and poor prognosis (Park et al., 2009), paradoxically, AMPK activation also induces apoptosis of DU-145 prostate cancer cells (Sauer et al., 2012). Based on the current literature reports, AMPK may have a duplex implication in genital system cancer development. AMPK can either counteract growth-stimulating signaling mediated by mTOR activation or act as a metabolic survival factor in cancer cells, depending on different cancer cell types and other compensations within the cell. Further investigation is needed to answer the question and determine whether AMPK can be as a target for drug therapy of reproductive system related diseases.
PCOS
Polycystic ovary syndrome (PCOS) is a common gynecological endocrine disease that affects about 6–21% of women in reproductive age and is an important cause of female infertility (Joham et al., 2015). Although the exact pathogenesis remains unclear, PCOS patients are often present with ovarian cortical thickening and multiple immature follicles, accompanied by hyperandrogenic and insulin resistance. Metformin is commonly used clinically to treat PCOS patients, which can reduce the serum progesterone and estrogen concentrations, increase patients’ ovulation, fertilization, and pregnancy rates (Vandermolen et al., 2001). Previously, it was widely believed that metformin, as an insulin sensitizer, exerted its effects by improving insulin resistance in PCOS patients (Morley et al., 2017). However, in recent years, studies have found that metformin is likely to rely on AMPK activation mechanism to participate in the treatment of PCOS. AMPK activators resveratrol and metformin have been shown to treat PCOS in rats, reduce the body and ovary weights, testosterone levels were observed, ameliorated the elevated number of secondary and atretic follicles (Furat Rencber et al., 2018). In a study mentioned above, metformin reduces granulosa cells basal and FSH-stimulated steroid hormone secretion by activating AMPK, which improves the formation of excessive follicular cysts in the ovaries of patients with PCOS and promotes the development of dominant follicles (Tosca et al., 2007a). It is important to note that the endometrium of PCOS patients is also in a state of insulin resistance. Compared to healthy fertile women, the expression of AMPKα and insulin-dependent glucose transporter (GLUT4) decrease in the endometrium of PCOS patients, which are associated with adverse reproductive outcomes. The downstream target of AMPK, myocyte enhancer factor 2A (MEF2A), is involved in the regulation of GLUT4 expression. When AMPK activation increases MEF2A expression, MEF2A is transported to the nucleus and binds to the GLUT4 promoter. Therefore, the application of metformin can increase the expression of GLUT4 in the endometrium of PCOS patients, thereby improving endometrial metabolic function and increasing pregnancy rates (Carvajal et al., 2013).
Transcription Regulation Roles of AMPK in Reproduction
Transcription regulation is a multistep and complicated process that heavily relies on the interaction between TFs and their DNA recognition sites to modulate the activity of genes (Chen and Rajewsky, 2007). In recent years, the emerging role of AMPK signaling in the control of gene expression through TF regulation has been documented. Indeed, when energy shortage triggers AMPK activation due to exercise/contraction in skeletal muscle, peroxisome proliferator-activated receptor-γ co-activator 1α (PGC1α) and transcription factor EB (TFEB)/transcription factor E3 (TFE3) translocate to the nucleus where they activate mitochondrial genes and metabolic genes such as pathways linked to the expression of glucose transporters, glycolytic enzymes (Mansueto et al., 2017; Markby and Sakamoto, 2020). An excellent review published in 2020 has summarized the transcriptional regulation by AMPK signaling (Sukumaran et al., 2020). And more notably, while significant effort has been given to explore the mechanism of how AMPK impacts gene expression, its involvement in the field of reproduction are less appreciated.
In fact, AMPK can influence reproduction activity not only through acute effects of AMPK activation such as direct phosphorylation of key enzymes but also through controlling slower transcriptional events via modulation of TFs. Both the aforementioned semen cryopreservation and environmental exposure to toxic substances will put spermatozoa under the threat of ROS (Li et al., 2017; Shabani Nashtaei et al., 2017). However, the spermatozoa still retain their capacity of fertilization, indicating spermatozoa themselves have a series of intracellular biochemical reactions to achieve survival in the face of oxidative stress. Increasing evidence has demonstrated that exposure to cadmium (Cd) can result in reproductive toxicity in humans (Pant et al., 2003) and rodents (Li et al., 2016), which is due to Cd-induced ROS accumulation and then DNA damage (Filipic, 2012; Li et al., 2016). Sperm DNA integrity is essential for the accurate transmission of genetic information, and its damage is one of the causes of abnormal sperm and male infertility (Gunes et al., 2015). It has been reported that ATM was activated by Cd-induced DNA damage and then positively regulates autophagy through repression of mTOR signaling via the AMPK pathway in mouse spermatocytes (Li et al., 2017). Subsequently, autophagy provided energy for DNA damage repair via selective degradation of damaged molecules and organelles (Towers and Thorburn, 2016) and thus had a protective role in DNA stability. In vivo, AMPK and mTOR always drive autophagy in opposite directions. AMPK facilitates autophagy directly by phosphorylating autophagy-related ULK1 and PIK3C3/VPS34 complexes or regulating the nuclear translocation of autophagy-related transcription factors such as ACSS2 and FOXO3, TFEB, while mTOR1 has an opposite, inhibitory role in these processes (Li and Chen, 2019), thereby maintaining the dynamic balance of autophagy. Actually, autophagy is a catabolic process mediated by lysosomes (Towers and Thorburn, 2016). A paper published by Young et al. (2016) linked AMPK and lysosomal biogenesis to germ cell specification, revealing the possible mechanism by which AMPK determines cell fates. Embryonic stem cells (ESCs) possess the capability to differentiate into multiple cell types (Young et al., 2016). The spatial and temporal integration of numerous signaling cascades govern the induction of lineage-specific transcription factors and determine cell differentiation (Gadue et al., 2006). The transcription factors, TFEB and TFE3, are known as important regulators of lysosomal biogenesis and autophagic processes, whose activation induces Coordinated Lysosomal Expression and Regulation (CLEAR) target genes and up-regulates endolysosomes, thus providing a suitable microenvironment for the canonical Wnt pathway to free β-catenin and controlling the endodermal differentiation program (Young et al., 2016). Mouse ESCs lacking AMPK displayed severe endodermal defects because increased mTOR signaling inhibits TFEB activity, indicating the important role of AMPK in cell fate determination during differentiation (Young et al., 2016). Interestingly, two teams recently reported that AMPK could dephosphorylate TFEB/TFE3, induce their nuclear localization independently of mTOR activity (Collodet et al., 2019; El-Houjeiri et al., 2019), and activate TFEB/TFE3 boost the expression of lysosomal and inflammatory genes in macrophages in response to infection (Hipolito et al., 2018; El-Houjeiri et al., 2019). Additionally, stimulation of AMPK could disturb the expression of specific developmental control genes such as the pax3 gene in the embryo and cause durable changes in phenotype under oxidative stress (Wu et al., 2012). Hence, it is hardly difficult to see that AMPK is involved in a wide variety of biological processes, including the biosynthesis regulation of sex hormones. AMPK activation, whether the use of activators or overexpression of the constitutively active form, inhibits cyclic AMP (cAMP) -mediated steroidogenic enzyme promoter activities and gene expression, ultimately reducing testosterone production in Leydig cells (Ahn et al., 2012). The importance of testosterone in maintaining male fertility is indisputable, while the role of estradiol should not be ignored. The aromatase enzyme in testis irreversibly converts androgens into estrogens, and the infertile aromatase KO mice support the direct actions of estrogen on spermatogenesis (Robertson et al., 2001). A study using human testicular tissues has demonstrated that the expression pattern of pAMPK was inversely associated with aromatase expression and AMPK phosphorylation inhibits the nuclear translocation of the cAMP response element-binding protein- regulated transcription co-activator (CRCT), resulting in failed aromatase expression (Ham et al., 2016), indicating the important role of AMPK in the estrogen-mediated development of germ cells. Taken together, these observations lead to the conclusion that AMPK influences the binding between TFs and their target DNA sequences, thus affecting gene expression in the field of reproduction (Figure 4).
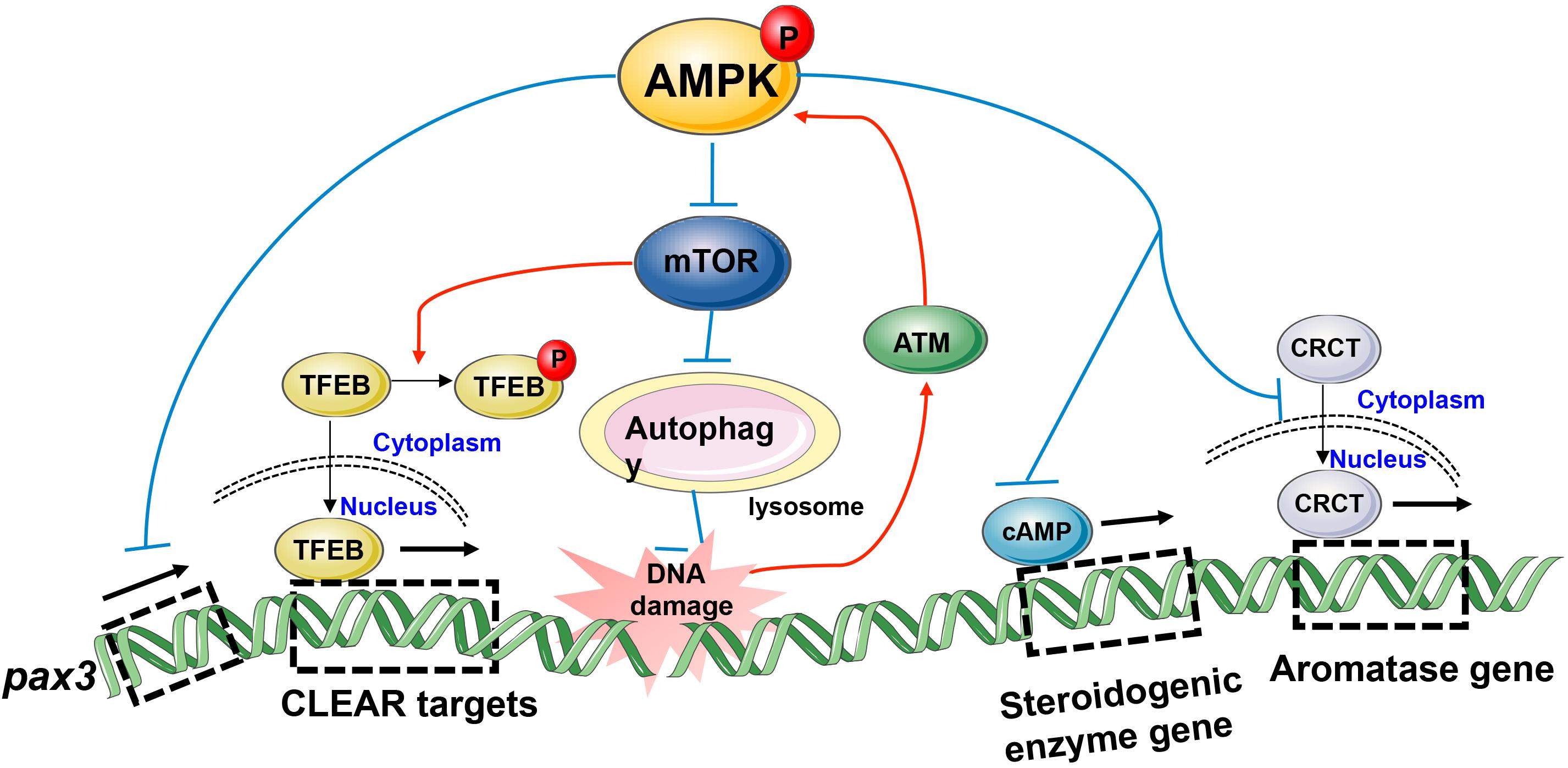
Figure 4. Model depicting transcriptional regulation by phospho-AMPK in the field of reproduction. AMPK activation impacts gene expression involved in biological processes such as embryonic development, spermatocyte DNA damage repair, and gonadal steroid hormones production.
Conclusion
A large body of research has shown that AMPK is not limited to serve as a major energy sensor to monitor the supply of nutrients, but also involved in the regulation of reproduction through several different strategies. AMPK affects the secretion of GnRH and gonadotropins and integrates energy status with fertility at the level of the hypothalamus and pituitary. Furthermore, AMPK itself is also expressed in the gonad, and is involved in regulating spermatogenesis and follicular development. Of particular concern is the emerging role of AMPK in transcriptional regulation, in which AMPK alters the transcription efficiency of reproduction-related genes by affecting the binding of transcription factors to their specific target sequences. Here, we briefly summarized the effects of AMPK activation on gene expression involving biological processes such as embryonic development, spermatocyte DNA damage repair and gonadal steroid hormone production, hoping to engender interest in the field to fully understand how reproduction and metabolism are tightly connected in the body through transcriptional regulation by AMPK activation.
Author Contributions
WY and LW drafted the manuscript. FW and SY revised the manuscript. All authors approved the final version of the manuscript.
Funding
This work was supported by grants from the National Natural Science Foundation of China (81971444 to SY and 31900511 to FW), the Science, Technology and Innovation Commission of Shenzhen Municipality (JCYJ20170244 to SY), and the Fundamental Research Funds for the Central Universities, Huazhong University of Science and Technology (No. 2019kfyXJJS089 to FW).
Conflict of Interest
The authors declare that the research was conducted in the absence of any commercial or financial relationships that could be construed as a potential conflict of interest.
References
Abel, M. H., Wootton, A. N., Wilkins, V., Huhtaniemi, I., Knight, P. G., and Charlton, H. M. (2000). The effect of a null mutation in the follicle-stimulating hormone receptor gene on mouse reproduction. Endocrinology 141, 1795–1803. doi: 10.1210/endo.141.5.7456
Adashi, E. Y., Resnick, C. E., Svoboda, M. E., and Van Wyk, J. J. (1986). Somatomedin-C as an amplifier of follicle-stimulating hormone action: enhanced accumulation of adenosine 3’,5’-monophosphate. Endocrinology 118, 149–155. doi: 10.1210/endo-118-1-149
Agarwal, A., Nallella, K. P., Allamaneni, S. S., and Said, T. M. (2004). Role of antioxidants in treatment of male infertility: an overview of the literature. Reprod. Biomed. Online 8, 616–627. doi: 10.1016/s1472-6483(10)61641-0
Ahn, S. W., Gang, G. T., Tadi, S., Nedumaran, B., Kim, Y. D., Park, J. H., et al. (2012). Phosphoenolpyruvate carboxykinase and glucose-6-phosphatase are required for steroidogenesis in testicular Leydig cells. J. Biol. Chem. 287, 41875–41887. doi: 10.1074/jbc.m112.421552
Aitken, R. J., Buckingham, D. W., Carreras, A., and Irvine, D. S. (1996). Superoxide dismutase in human sperm suspensions: relationship with cellular composition, oxidative stress, and sperm function. Free Radic. Biol. Med. 21, 495–504. doi: 10.1016/0891-5849(96)00119-0
Aitken, R. J., and De Iuliis, G. N. (2010). On the possible origins of DNA damage in human spermatozoa. Mol. Hum. Reprod. 16, 3–13. doi: 10.1093/molehr/gap059
Andrade, J., Quinn, J., Becker, R. Z., and Shupnik, M. A. (2013). AMP-activated protein kinase is a key intermediary in GnRH-stimulated LHbeta gene transcription. Mol. Endocrinol. 27, 828–839. doi: 10.1210/me.2012-1323
Aznar, N., Patel, A., Rohena, C. C., Dunkel, Y., Joosen, L. P., Taupin, V., et al. (2016). AMP-activated protein kinase fortifies epithelial tight junctions during energetic stress via its effector GIV/Girdin. eLife 5:e20795.
Baas, A. F., Kuipers, J., van der Wel, N. N., Batlle, E., Koerten, H. K., Peters, P. J., et al. (2004). Complete polarization of single intestinal epithelial cells upon activation of LKB1 by STRAD. Cell 116, 457–466. doi: 10.1016/s0092-8674(04)00114-x
Baker, J., Hardy, M. P., Zhou, J., Bondy, C., Lupu, F., Bellve, A. R., et al. (1996). Effects of an Igf1 gene null mutation on mouse reproduction. Mol. Endocrinol. 10, 903–918. doi: 10.1210/me.10.7.903
Bertoldo, M. J., Guibert, E., Faure, M., Guillou, F., Rame, C., Nadal-Desbarats, L., et al. (2016). Specific deletion of AMP-activated protein kinase (alpha1AMPK) in mouse Sertoli cells modifies germ cell quality. Mol. Cell Endocrinol. 423, 96–112. doi: 10.1016/j.mce.2016.01.001
Bilodeau, J. F., Chatterjee, S., Sirard, M. A., and Gagnon, C. (2000). Levels of antioxidant defenses are decreased in bovine spermatozoa after a cycle of freezing and thawing. Mol. Reprod. Dev. 55, 282–288. doi: 10.1002/(sici)1098-2795(200003)55:3<282::aid-mrd6>3.0.co;2-7
Bilodeau-Goeseels, S., Sasseville, M., Guillemette, C., and Richard, F. J. (2007). Effects of adenosine monophosphate-activated kinase activators on bovine oocyte nuclear maturation in vitro. Mol. Reprod. Dev. 74, 1021–1034. doi: 10.1002/mrd.20574
Burger, L. L., Haisenleder, D. J., Dalkin, A. C., and Marshall, J. C. (2004). Regulation of gonadotropin subunit gene transcription. J. Mol. Endocrinol. 33, 559–584. doi: 10.1677/jme.1.01600
Calder, M. D., Edwards, N. A., Betts, D. H., and Watson, A. J. (2017). Treatment with AICAR inhibits blastocyst development, trophectoderm differentiation and tight junction formation and function in mice. Mol. Hum. Reprod. 23, 771–785. doi: 10.1093/molehr/gax050
Carey, E. A., Albers, R. E., Doliboa, S. R., Hughes, M., Wyatt, C. N., Natale, D. R., et al. (2014). AMPK knockdown in placental trophoblast cells results in altered morphology and function. Stem Cells Dev. 23, 2921–2930. doi: 10.1089/scd.2014.0092
Carvajal, R., Rosas, C., Kohan, K., Gabler, F., Vantman, D., Romero, C., et al. (2013). Metformin augments the levels of molecules that regulate the expression of the insulin-dependent glucose transporter GLUT4 in the endometria of hyperinsulinemic PCOS patients. Hum. Reprod. 28, 2235–2244. doi: 10.1093/humrep/det116
Castillo, J., Bogle, O. A., Jodar, M., Torabi, F., Delgado-Duenas, D., Estanyol, J. M., et al. (2019). Proteomic changes in human sperm during sequential in vitro capacitation and acrosome reaction. Front. Cell Dev. Biol. 7:295. doi: 10.3389/fcell.2019.00295
Chen, J., Hudson, E., Chi, M. M., Chang, A. S., Moley, K. H., Hardie, D. G., et al. (2006). AMPK regulation of mouse oocyte meiotic resumption in vitro. Dev. Biol. 291, 227–238. doi: 10.1016/j.ydbio.2005.11.039
Chen, K., and Rajewsky, N. (2007). The evolution of gene regulation by transcription factors and microRNAs. Nat. Rev. Genet. 8, 93–103. doi: 10.1038/nrg1990
Chen, L., Wang, J., You, Q., He, S., Meng, Q., Gao, J., et al. (2018). Activating AMPK to restore tight junction assembly in intestinal epithelium and to attenuate experimental colitis by metformin. Front. Pharmacol. 9:761. doi: 10.3389/fphar.2018.00761
Chen, M., Liu, J., Yang, L., and Ling, W. (2017). AMP-activated protein kinase regulates lipid metabolism and the fibrotic phenotype of hepatic stellate cells through inhibition of autophagy. FEBS Open Biol. 7, 811–820. doi: 10.1002/2211-5463.12221
Chen, Z., Wang, C., Jain, A., Srivastava, M., Tang, M., Zhang, H., et al. (2020). AMPK interactome reveals new function in non-homologous end joining DNA repair. Mol. Cell Proteom. 19, 467–477. doi: 10.1074/mcp.ra119.001794
Cheng, Y., Kim, J., Li, X. X., and Hsueh, A. J. (2015). Promotion of ovarian follicle growth following mTOR activation: synergistic effects of AKT stimulators. PLoS One 10:e0117769. doi: 10.1371/journal.pone.0117769
Chhipa, R. R., Wu, Y., and Ip, C. (2011). AMPK-mediated autophagy is a survival mechanism in androgen-dependent prostate cancer cells subjected to androgen deprivation and hypoxia. Cell Signal. 23, 1466–1472. doi: 10.1016/j.cellsig.2011.04.008
Chhipa, R. R., Wu, Y., Mohler, J. L., and Ip, C. (2010). Survival advantage of AMPK activation to androgen-independent prostate cancer cells during energy stress. Cell Signal. 22, 1554–1561. doi: 10.1016/j.cellsig.2010.05.024
Choi, C. H., Chung, J. Y., Cho, H., Kitano, H., Chang, E., Ylaya, K., et al. (2015). Prognostic significance of AMP-dependent kinase alpha expression in cervical cancer. Pathobiology 82, 203–211. doi: 10.1159/000434726
Cieslik, K. A., Trial, J., and Entman, M. L. (2017). Aicar treatment reduces interstitial fibrosis in aging mice: suppression of the inflammatory fibroblast. J. Mol. Cell Cardiol. 111, 81–85. doi: 10.1016/j.yjmcc.2017.08.003
Collodet, C., Foretz, M., Deak, M., Bultot, L., Metairon, S., Viollet, B., et al. (2019). AMPK promotes induction of the tumor suppressor FLCN through activation of TFEB independently of mTOR. FASEB J. 33, 12374–12391. doi: 10.1096/fj.201900841r
Coyral-Castel, S., Tosca, L., Ferreira, G., Jeanpierre, E., Rame, C., Lomet, D., et al. (2008). The effect of AMP-activated kinase activation on gonadotrophin-releasing hormone secretion in GT1-7 cells and its potential role in hypothalamic regulation of the oestrous cyclicity in rats. J. Neuroendocrinol. 20, 335–346. doi: 10.1111/j.1365-2826.2007.01643.x
Creighton, J., Jian, M., Sayner, S., Alexeyev, M., and Insel, P. A. (2011). Adenosine monophosphate-activated kinase alpha1 promotes endothelial barrier repair. FASEB J. 25, 3356–3365. doi: 10.1096/fj.10-179218
Dandekar, S. P., Nadkarni, G. D., Kulkarni, V. S., and Punekar, S. (2002). Lipid peroxidation and antioxidant enzymes in male infertility. J. Postgrad. Med. 48, 186–189; discussion189–190.
Denison, F. C., Smith, L. B., Muckett, P. J., O’Hara, L., Carling, D., and Woods, A. (2011). LKB1 is an essential regulator of spermatozoa release during spermiation in the mammalian testis. PLoS One 6:e28306. doi: 10.1371/journal.pone.0028306
Diamanti-Kandarakis, E. (2008). Polycystic ovarian syndrome: pathophysiology, molecular aspects and clinical implications. Expert Rev. Mol. Med. 10:e3.
Downs, S. M., Hudson, E. R., and Hardie, D. G. (2002). A potential role for AMP-activated protein kinase in meiotic induction in mouse oocytes. Dev. Biol. 245, 200–212. doi: 10.1006/dbio.2002.0613
Downs, S. M., Ya, R., and Davis, C. C. (2010). Role of AMPK throughout meiotic maturation in the mouse oocyte: evidence for promotion of polar body formation and suppression of premature activation. Mol. Reprod. Dev. 77, 888–899. doi: 10.1002/mrd.21229
Dupont, J., Reverchon, M., Bertoldo, M. J., and Froment, P. (2014). Nutritional signals and reproduction. Mol. Cell Endocrinol. 382, 527–537. doi: 10.1016/j.mce.2013.09.028
Duval, D. L. (2011). PRKA/AMPK: integrating energy status with fertility in pituitary gonadotrophs. Biol. Reprod. 84, 205–206. doi: 10.1095/biolreprod.110.089722
El-Houjeiri, L., Possik, E., Vijayaraghavan, T., Paquette, M., Martina, J. A., Kazan, J. M., et al. (2019). The transcription factors TFEB and TFE3 Link the FLCN-AMPK signaling axis to innate immune response and pathogen resistance. Cell Rep. 26, 3613–3628.e6.
Erdemoglu, E., Guney, M., Giray, S. G., Take, G., and Mungan, T. (2009). Effects of metformin on mammalian target of rapamycin in a mouse model of endometrial hyperplasia. Eur. J. Obstet. Gynecol. Reprod. Biol. 145, 195–199. doi: 10.1016/j.ejogrb.2009.04.034
Faure, M., Guibert, E., Alves, S., Pain, B., Rame, C., Dupont, J., et al. (2016). The insulin sensitiser metformin regulates chicken Sertoli and germ cell populations. Reproduction 151, 527–538. doi: 10.1530/rep-15-0565
Filipic, M. (2012). Mechanisms of cadmium induced genomic instability. Mutat. Res. 733, 69–77. doi: 10.1016/j.mrfmmm.2011.09.002
Forcet, C., Etienne-Manneville, S., Gaude, H., Fournier, L., Debilly, S., Salmi, M., et al. (2005). Functional analysis of Peutz-Jeghers mutations reveals that the LKB1 C-terminal region exerts a crucial role in regulating both the AMPK pathway and the cell polarity. Hum. Mol. Genet. 14, 1283–1292. doi: 10.1093/hmg/ddi139
Furat Rencber, S., Kurnaz Ozbek, S., Eraldemir, C., Sezer, Z., Kum, T., Ceylan, S., et al. (2018). Effect of resveratrol and metformin on ovarian reserve and ultrastructure in PCOS: an experimental study. J. Ovarian Res. 11:55.
Gadducci, A., Biglia, N., Tana, R., Cosio, S., and Gallo, M. (2016). Metformin use and gynecological cancers: a novel treatment option emerging from drug repositioning. Crit. Rev. Oncol. Hematol. 105, 73–83. doi: 10.1016/j.critrevonc.2016.06.006
Gadea, J., Selles, E., Marco, M. A., Coy, P., Matas, C., Romar, R., et al. (2004). Decrease in glutathione content in boar sperm after cryopreservation. Effect of the addition of reduced glutathione to the freezing and thawing extenders. Theriogenology 62, 690–701. doi: 10.1016/j.theriogenology.2003.11.013
Gadue, P., Huber, T. L., Paddison, P. J., and Keller, G. M. (2006). Wnt and TGF-beta signaling are required for the induction of an in vitro model of primitive streak formation using embryonic stem cells. Proc. Natl. Acad. Sci. U.S.A. 103, 16806–16811. doi: 10.1073/pnas.0603916103
Galardo, M. N., Riera, M. F., Pellizzari, E. H., Cigorraga, S. B., and Meroni, S. B. (2007). The AMP-activated protein kinase activator, 5-aminoimidazole-4-carboxamide-1-b-D-ribonucleoside, regulates lactate production in rat Sertoli cells. J. Mol. Endocrinol. 39, 279–288. doi: 10.1677/jme-07-0054
Genazzani, A. D., Battaglia, C., Malavasi, B., Strucchi, C., Tortolani, F., and Gamba, O. (2004). Metformin administration modulates and restores luteinizing hormone spontaneous episodic secretion and ovarian function in nonobese patients with polycystic ovary syndrome. Fertil. Steril. 81, 114–119. doi: 10.1016/j.fertnstert.2003.05.020
Gerber, J., Heinrich, J., and Brehm, R. (2016). Blood-testis barrier and Sertoli cell function: lessons from SCCx43KO mice. Reproduction 151, R15–R27.
Gonzalez, A., Hall, M. N., Lin, S. C., and Hardie, D. G. (2020). AMPK and TOR: the yin and yang of cellular nutrient sensing and growth control. Cell Metab. 31, 472–492. doi: 10.1016/j.cmet.2020.01.015
Griffiths, R. M., Pru, C. A., Behura, S. K., Cronrath, A. R., McCallum, M. L., Kelp, N. C., et al. (2020). AMPK is required for uterine receptivity and normal responses to steroid hormones. Reproduction 159, 707–717. doi: 10.1530/rep-19-0402
Group, E. C. W. (2006). Nutrition and reproduction in women. Hum. Reprod. Update 12, 193–207. doi: 10.1093/humupd/dmk003
Gu, N. H., Zhao, W. L., Wang, G. S., and Sun, F. (2019). Comparative analysis of mammalian sperm ultrastructure reveals relationships between sperm morphology, mitochondrial functions and motility. Reprod. Biol. Endocrinol. 17:66.
Gunes, S., Al-Sadaan, M., and Agarwal, A. (2015). Spermatogenesis, DNA damage and DNA repair mechanisms in male infertility. Reprod. Biomed. Online 31, 309–319. doi: 10.1016/j.rbmo.2015.06.010
Ham, S., Brown, K. A., Simpson, E. R., and Meachem, S. J. (2016). Immunolocalisation of aromatase regulators liver kinase B1, phosphorylated AMP-activated protein kinase and cAMP response element-binding protein-regulated transcription co-activators in the human testis. Reprod. Fertil. Dev. doi: 10.1071/RD15390
Hardie, D. G. (2007). AMP-activated/SNF1 protein kinases: conserved guardians of cellular energy. Nat. Rev. Mol. Cell Biol. 8, 774–785. doi: 10.1038/nrm2249
Hashimoto, T., Murata, Y., Urushihara, Y., Shiga, S., Takeda, K., and Hosoi, Y. (2018). Severe hypoxia increases expression of ATM and DNA-PKcs and it increases their activities through Src and AMPK signaling pathways. Biochem. Biophys. Res. Commun. 505, 13–19. doi: 10.1016/j.bbrc.2018.09.068
Hearle, N., Schumacher, V., Menko, F. H., Olschwang, S., Boardman, L. A., Gille, J. J., et al. (2006). Frequency and spectrum of cancers in the Peutz-Jeghers syndrome. Clin. Cancer Res. 12, 3209–3215. doi: 10.1158/1078-0432.ccr-06-0083
Herbison, A. E. (2016). Control of puberty onset and fertility by gonadotropin-releasing hormone neurons. Nat. Rev. Endocrinol. 12, 452–466. doi: 10.1038/nrendo.2016.70
Hipolito, V. E. B., Ospina-Escobar, E., and Botelho, R. J. (2018). Lysosome remodelling and adaptation during phagocyte activation. Cell Microbiol. 20:e12824. doi: 10.1111/cmi.12824
Huang, L., Wang, Z. B., Jiang, Z. Z., Hu, M. W., Lin, F., Zhang, Q. H., et al. (2013). Specific disruption of Tsc1 in ovarian granulosa cells promotes ovulation and causes progressive accumulation of corpora lutea. PLoS One 8:e54052. doi: 10.1371/journal.pone.0054052
Huang, V. W., Lee, C. L., Lee, Y. L., Lam, K. K., Ko, J. K., Yeung, W. S., et al. (2015). Sperm fucosyltransferase-5 mediates spermatozoa-oviductal epithelial cell interaction to protect human spermatozoa from oxidative damage. Mol. Hum. Reprod. 21, 516–526. doi: 10.1093/molehr/gav015
Hurtado de Llera, A., Martin-Hidalgo, D., Gil, M. C., Garcia-Marin, L. J., and Bragado, M. J. (2012). AMP-activated kinase AMPK is expressed in boar spermatozoa and regulates motility. PLoS One 7:e38840. doi: 10.1371/journal.pone.0038840
Hurtado de Llera, A., Martin-Hidalgo, D., Gil, M. C., Garcia-Marin, L. J., and Bragado, M. J. (2014). The calcium/CaMKKalpha/beta and the cAMP/PKA pathways are essential upstream regulators of AMPK activity in boar spermatozoa. Biol. Reprod. 90:29.
Hurtado de Llera, A., Martin-Hidalgo, D., Gil, M. C., Garcia-Marin, L. J., and Bragado, M. J. (2015). AMPK up-activation reduces motility and regulates other functions of boar spermatozoa. Mol. Hum. Reprod. 21, 31–45. doi: 10.1093/molehr/gau091
Hurtado de Llera, A., Martin-Hidalgo, D., Rodriguez-Gil, J. E., Gil, M. C., Garcia-Marin, L. J., and Bragado, M. J. (2013). AMP-activated kinase, AMPK, is involved in the maintenance of plasma membrane organization in boar spermatozoa. Biochim. Biophys. Acta 1828, 2143–2151. doi: 10.1016/j.bbamem.2013.05.026
Huynh, M. K., Kinyua, A. W., Yang, D. J., and Kim, K. W. (2016). Hypothalamic AMPK as a regulator of energy homeostasis. Neural Plast. 2016:2754078.
Ibanez, L., Lopez-Bermejo, A., Diaz, M., Marcos, M. V., and de Zegher, F. (2011a). Early metformin therapy to delay menarche and augment height in girls with precocious pubarche. Fertil. Steril. 95, 727–730. doi: 10.1016/j.fertnstert.2010.08.052
Ibanez, L., Lopez-Bermejo, A., Diaz, M., Marcos, M. V., and de Zegher, F. (2011b). Early metformin therapy (age 8-12 years) in girls with precocious pubarche to reduce hirsutism, androgen excess, and oligomenorrhea in adolescence. J. Clin. Endocrinol. Metab. 96, E1262–E1267.
Imamura, K., Ogura, T., Kishimoto, A., Kaminishi, M., and Esumi, H. (2001). Cell cycle regulation via p53 phosphorylation by a 5’-AMP activated protein kinase activator, 5-aminoimidazole- 4-carboxamide-1-beta-D-ribofuranoside, in a human hepatocellular carcinoma cell line. Biochem. Biophys. Res. Commun. 287, 562–567. doi: 10.1006/bbrc.2001.5627
Jiang, Z. Z., Hu, M. W., Ma, X. S., Schatten, H., Fan, H. Y., Wang, Z. B., et al. (2016). LKB1 acts as a critical gatekeeper of ovarian primordial follicle pool. Oncotarget 7, 5738–5753. doi: 10.18632/oncotarget.6792
Joham, A. E., Teede, H. J., Ranasinha, S., Zoungas, S., and Boyle, J. (2015). Prevalence of infertility and use of fertility treatment in women with polycystic ovary syndrome: data from a large community-based cohort study. J. Womens Health 24, 299–307. doi: 10.1089/jwh.2014.5000
Jones, R. G., Plas, D. R., Kubek, S., Buzzai, M., Mu, J., Xu, Y., et al. (2005). AMP-activated protein kinase induces a p53-dependent metabolic checkpoint. Mol. Cell 18, 283–293. doi: 10.1016/j.molcel.2005.03.027
Lasso, J. L., Noiles, E. E., Alvarez, J. G., and Storey, B. T. (1994). Mechanism of superoxide dismutase loss from human sperm cells during cryopreservation. J. Androl. 15, 255–265.
Lee, J. H., Koh, H., Kim, M., Kim, Y., Lee, S. Y., Karess, R. E., et al. (2007). Energy-dependent regulation of cell structure by AMP-activated protein kinase. Nature 447, 1017–1020.
Levine, H., Jorgensen, N., Martino-Andrade, A., Mendiola, J., Weksler-Derri, D., Mindlis, I., et al. (2017). Temporal trends in sperm count: a systematic review and meta-regression analysis. Hum. Reprod. Update 23, 646–659. doi: 10.1093/humupd/dmx022
Li, R., Luo, X., Li, L., Peng, Q., Yang, Y., Zhao, L., et al. (2016). The protective effects of melatonin against oxidative stress and inflammation induced by acute cadmium exposure in mice testis. Biol. Trace Elem. Res. 170, 152–164. doi: 10.1007/s12011-015-0449-6
Li, R., Luo, X., Zhu, Y., Zhao, L., Li, L., Peng, Q., et al. (2017). ATM signals to AMPK to promote autophagy and positively regulate DNA damage in response to cadmium-induced ROS in mouse spermatocytes. Environ. Pollut. 231, 1560–1568. doi: 10.1016/j.envpol.2017.09.044
Lim, C. T., Kola, B., and Korbonits, M. (2010). AMPK as a mediator of hormonal signalling. J. Mol. Endocrinol. 44, 87–97. doi: 10.1677/jme-09-0063
Liu, Y., Tang, G., Li, Y., Wang, Y., Chen, X., Gu, X., et al. (2014). Metformin attenuates blood-brain barrier disruption in mice following middle cerebral artery occlusion. J. Neuroinflam. 11:177.
Lizcano, J. M., Goransson, O., Toth, R., Deak, M., Morrice, N. A., Boudeau, J., et al. (2004). LKB1 is a master kinase that activates 13 kinases of the AMPK subfamily, including MARK/PAR-1. EMBO J. 23, 833–843. doi: 10.1038/sj.emboj.7600110
Lu, M., Tang, Q., Olefsky, J. M., Mellon, P. L., and Webster, N. J. (2008). Adiponectin activates adenosine monophosphate-activated protein kinase and decreases luteinizing hormone secretion in LbetaT2 gonadotropes. Mol. Endocrinol. 22, 760–771. doi: 10.1210/me.2007-0330
Lu, X., Guo, S., Cheng, Y., Kim, J. H., Feng, Y., and Feng, Y. (2017). Stimulation of ovarian follicle growth after AMPK inhibition. Reproduction 153, 683–694. doi: 10.1530/rep-16-0577
Mah, P. M., and Wittert, G. A. (2010). Obesity and testicular function. Mol. Cell Endocrinol. 316, 180–186. doi: 10.1016/j.mce.2009.06.007
Mansueto, G., Armani, A., Viscomi, C., D’Orsi, L., De Cegli, R., Polishchuk, E. V., et al. (2017). Transcription factor EB controls metabolic flexibility during exercise. Cell Metab. 25, 182–196. doi: 10.1016/j.cmet.2016.11.003
Markby, G. R., and Sakamoto, K. (2020). Transcription factor EB and TFE3: new metabolic coordinators mediating adaptive responses to exercise in skeletal muscle? Am. J. Physiol. Endocrinol. Metab. 319, E763–E768.
Martin-Hidalgo, D., Hurtado de Llera, A., Calle-Guisado, V., Gonzalez-Fernandez, L., Garcia-Marin, L., and Bragado, M. J. (2018). AMPK function in mammalian spermatozoa. Int. J. Mol. Sci. 19:3293. doi: 10.3390/ijms19113293
McCallum, M. L., Pru, C. A., Smith, A. R., Kelp, N. C., Foretz, M., Viollet, B., et al. (2018). A functional role for AMPK in female fertility and endometrial regeneration. Reproduction 156, 501–513. doi: 10.1530/rep-18-0372
McGee, E. A., and Hsueh, A. J. (2000). Initial and cyclic recruitment of ovarian follicles. Endocr. Rev. 21, 200–214. doi: 10.1210/edrv.21.2.0394
Minokoshi, Y., Alquier, T., Furukawa, N., Kim, Y. B., Lee, A., Xue, B., et al. (2004). AMP-kinase regulates food intake by responding to hormonal and nutrient signals in the hypothalamus. Nature 428, 569–574. doi: 10.1038/nature02440
Mishra, P., Negi, M. P. S., Srivastava, M., Singh, K., and Rajender, S. (2018). Decline in seminal quality in Indian men over the last 37 years. Reprod. Biol. Endocrinol. 16:103.
Morley, L. C., Tang, T., Yasmin, E., Norman, R. J., and Balen, A. H. (2017). Insulin-sensitising drugs (metformin, rosiglitazone, pioglitazone, D-chiro-inositol) for women with polycystic ovary syndrome, oligo amenorrhoea and subfertility. Cochrane Database Syst. Rev. 11:CD003053.
Mruk, D. D., and Cheng, C. Y. (2015). The mammalian blood-testis barrier: its biology and regulation. Endocr. Rev. 36, 564–591. doi: 10.1210/er.2014-1101
Nagiec, E. E., Bernstein, A., and Whiteheart, S. W. (1995). Each domain of the N-ethylmaleimide-sensitive fusion protein contributes to its transport activity. J. Biol. Chem. 270, 29182–29188. doi: 10.1074/jbc.270.49.29182
Nguyen, T. M., Seigneurin, F., Froment, P., Combarnous, Y., and Blesbois, E. (2015). The 5’-AMP-Activated Protein Kinase (AMPK) is involved in the augmentation of antioxidant defenses in cryopreserved chicken sperm. PLoS One 10:e0134420. doi: 10.1371/journal.pone.0134420
Noppe, G., Dufeys, C., Buchlin, P., Marquet, N., Castanares-Zapatero, D., Balteau, M., et al. (2014). Reduced scar maturation and contractility lead to exaggerated left ventricular dilation after myocardial infarction in mice lacking AMPKalpha1. J. Mol. Cell Cardiol. 74, 32–43. doi: 10.1016/j.yjmcc.2014.04.018
Oride, A., Kanasaki, H., Purwana, I. N., and Miyazaki, K. (2010). Effects of metformin administration on plasma gonadotropin levels in women with infertility, with an in vitro study of the direct effects on the pituitary gonadotrophs. Pituitary 13, 236–241. doi: 10.1007/s11102-010-0223-x
Pant, N., Upadhyay, G., Pandey, S., Mathur, N., Saxena, D. K., and Srivastava, S. P. (2003). Lead and cadmium concentration in the seminal plasma of men in the general population: correlation with sperm quality. Reprod. Toxicol. 17, 447–450. doi: 10.1016/s0890-6238(03)00036-4
Park, H. U., Suy, S., Danner, M., Dailey, V., Zhang, Y., Li, H., et al. (2009). AMP-activated protein kinase promotes human prostate cancer cell growth and survival. Mol. Cancer Ther. 8, 733–741. doi: 10.1158/1535-7163.mct-08-0631
Pasquali, R. (2006). Obesity, fat distribution and infertility. Maturitas 54, 363–371. doi: 10.1016/j.maturitas.2006.04.018
Perez, C. V., Sobarzo, C. M., Jacobo, P. V., Pellizzari, E. H., Cigorraga, S. B., Denduchis, B., et al. (2012). Loss of occludin expression and impairment of blood-testis barrier permeability in rats with autoimmune orchitis: effect of interleukin 6 on Sertoli cell tight junctions. Biol. Reprod. 87:122.
Pinilla, L., Aguilar, E., Dieguez, C., Millar, R. P., and Tena-Sempere, M. (2012). Kisspeptins and reproduction: physiological roles and regulatory mechanisms. Physiol. Rev. 92, 1235–1316. doi: 10.1152/physrev.00037.2010
Roa, J., Barroso, A., Ruiz-Pino, F., Vazquez, M. J., Seoane-Collazo, P., Martinez-Sanchez, N., et al. (2018). Metabolic regulation of female puberty via hypothalamic AMPK-kisspeptin signaling. Proc. Natl. Acad. Sci. U.S.A. 115, E10758–E10767.
Robertson, K. M., Simpson, E. R., Lacham-Kaplan, O., and Jones, M. E. (2001). Characterization of the fertility of male aromatase knockout mice. J. Androl. 22, 825–830.
Sakai, Y., and Yamashina, S. (1989). Mechanism for the removal of residual cytoplasm from spermatids during mouse spermiogenesis. Anat. Rec. 223, 43–48. doi: 10.1002/ar.1092230107
Sakamuru, S., Attene-Ramos, M. S., and Xia, M. (2016). Mitochondrial membrane potential assay. Methods Mol. Biol. 1473, 17–22.
Santiquet, N., Sasseville, M., Laforest, M., Guillemette, C., Gilchrist, R. B., and Richard, F. J. (2014). Activation of 5’ adenosine monophosphate-activated protein kinase blocks cumulus cell expansion through inhibition of protein synthesis during in vitro maturation in Swine. Biol. Reprod. 91:51.
Sathananthan, A. H., Selvaraj, K., Girijashankar, M. L., Ganesh, V., Selvaraj, P., and Trounson, A. O. (2006). From oogonia to mature oocytes: inactivation of the maternal centrosome in humans. Microsc. Res. Tech. 69, 396–407. doi: 10.1002/jemt.20299
Sauer, H., Engel, S., Milosevic, N., Sharifpanah, F., and Wartenberg, M. (2012). Activation of AMP-kinase by AICAR induces apoptosis of DU-145 prostate cancer cells through generation of reactive oxygen species and activation of c-Jun N-terminal kinase. Int. J. Oncol. 40, 501–508.
Schneider, J. E. (2004). Energy balance and reproduction. Physiol Behav 81, 289–317. doi: 10.1016/j.physbeh.2004.02.007
Seminara, S. B., Messager, S., Chatzidaki, E. E., Thresher, R. R., Acierno, J. S. Jr., Shagoury, J. K., et al. (2003). The GPR54 gene as a regulator of puberty. N. Engl. J. Med. 349, 1614–1627.
Seo-Mayer, P. W., Thulin, G., Zhang, L., Alves, D. S., Ardito, T., Kashgarian, M., et al. (2011). Preactivation of AMPK by metformin may ameliorate the epithelial cell damage caused by renal ischemia. Am. J. Physiol. Renal Physiol. 301, F1346–F1357.
Shabani Nashtaei, M., Amidi, F., Sedighi Gilani, M. A., Aleyasin, A., Bakhshalizadeh, S., Naji, M., et al. (2017). Protective features of resveratrol on human spermatozoa cryopreservation may be mediated through 5’ AMP-activated protein kinase activation. Andrology 5, 313–326. doi: 10.1111/andr.12306
Shabani Nashtaei, M., Nekoonam, S., Naji, M., Bakhshalizadeh, S., and Amidi, F. (2018). Cryoprotective effect of resveratrol on DNA damage and crucial human sperm messenger RNAs, possibly through 5’ AMP-activated protein kinase activation. Cell Tissue Bank 19, 87–95. doi: 10.1007/s10561-017-9642-5
Shin, H. S., Ko, J., Kim, D. A., Ryu, E. S., Ryu, H. M., Park, S. H., et al. (2017). Metformin ameliorates the phenotype transition of peritoneal mesothelial cells and peritoneal fibrosis via a modulation of oxidative stress. Sci. Rep. 7:5690.
Shiomi, R., Shigetomi, K., Inai, T., Sakai, M., and Ikenouchi, J. (2015). CaMKII regulates the strength of the epithelial barrier. Sci. Rep. 5:13262.
Siegel, R. L., Miller, K. D., and Jemal, A. (2020). Cancer statistics, 2020. CA Cancer J. Clin. 70, 7–30.
Skeffington, K. L., Higgins, J. S., Mahmoud, A. D., Evans, A. M., Sferruzzi-Perri, A. N., Fowden, A. L., et al. (2016). Hypoxia, AMPK activation and uterine artery vasoreactivity. J. Physiol. 594, 1357–1369. doi: 10.1113/jp270995
Spruss, A., Kanuri, G., Stahl, C., Bischoff, S. C., and Bergheim, I. (2012). Metformin protects against the development of fructose-induced steatosis in mice: role of the intestinal barrier function. Lab. Invest. 92, 1020–1032. doi: 10.1038/labinvest.2012.75
Sukumaran, A., Choi, K., and Dasgupta, B. (2020). Insight on transcriptional regulation of the energy sensing AMPK and biosynthetic mTOR pathway genes. Front. Cell Dev. Biol. 8:671. doi: 10.3389/fcell.2020.00671
Sun, X., Yang, Q., Rogers, C. J., Du, M., and Zhu, M. J. (2017). AMPK improves gut epithelial differentiation and barrier function via regulating Cdx2 expression. Cell Death Differ. 24, 819–831. doi: 10.1038/cdd.2017.14
Svechnikov, K., Spatafora, C., Svechnikova, I., Tringali, C., and Soder, O. (2009). Effects of resveratrol analogs on steroidogenesis and mitochondrial function in rat Leydig cells in vitro. J. Appl. Toxicol. 29, 673–680. doi: 10.1002/jat.1456
Tanwar, P. S., Kaneko-Tarui, T., Zhang, L., and Teixeira, J. M. (2012). Altered LKB1/AMPK/TSC1/TSC2/mTOR signaling causes disruption of Sertoli cell polarity and spermatogenesis. Hum. Mol. Genet. 21, 4394–4405. doi: 10.1093/hmg/dds272
Tartarin, P., Guibert, E., Toure, A., Ouiste, C., Leclerc, J., Sanz, N., et al. (2012a). Inactivation of AMPKalpha1 induces asthenozoospermia and alters spermatozoa morphology. Endocrinology 153, 3468–3481. doi: 10.1210/en.2011-1911
Tartarin, P., Moison, D., Guibert, E., Dupont, J., Habert, R., Rouiller-Fabre, V., et al. (2012b). Metformin exposure affects human and mouse fetal testicular cells. Hum. Reprod. 27, 3304–3314. doi: 10.1093/humrep/des264
Thomson, L. K., Fleming, S. D., Aitken, R. J., De Iuliis, G. N., Zieschang, J. A., and Clark, A. M. (2009). Cryopreservation-induced human sperm DNA damage is predominantly mediated by oxidative stress rather than apoptosis. Hum. Reprod. 24, 2061–2070. doi: 10.1093/humrep/dep214
Torsoni, M. A., Borges, B. C., Cote, J. L., Allen, S. J., Mahany, E., Garcia-Galiano, D., et al. (2016). AMPKalpha2 in Kiss1 neurons is required for reproductive adaptations to acute metabolic challenges in adult female mice. Endocrinology 157, 4803–4816. doi: 10.1210/en.2016-1367
Tosca, L., Chabrolle, C., Uzbekova, S., and Dupont, J. (2007a). Effects of metformin on bovine granulosa cells steroidogenesis: possible involvement of adenosine 5’ monophosphate-activated protein kinase (AMPK). Biol. Reprod. 76, 368–378. doi: 10.1095/biolreprod.106.055749
Tosca, L., Froment, P., Rame, C., McNeilly, J. R., McNeilly, A. S., Maillard, V., et al. (2011). Metformin decreases GnRH- and activin-induced gonadotropin secretion in rat pituitary cells: potential involvement of adenosine 5’ monophosphate-activated protein kinase (PRKA). Biol. Reprod. 84, 351–362. doi: 10.1095/biolreprod.110.087023
Tosca, L., Froment, P., Solnais, P., Ferre, P., Foufelle, F., and Dupont, J. (2005). Adenosine 5’-monophosphate-activated protein kinase regulates progesterone secretion in rat granulosa cells. Endocrinology 146, 4500–4513. doi: 10.1210/en.2005-0301
Tosca, L., Solnais, P., Ferre, P., Foufelle, F., and Dupont, J. (2006). Metformin-induced stimulation of adenosine 5’ monophosphate-activated protein kinase (PRKA) impairs progesterone secretion in rat granulosa cells. Biol. Reprod. 75, 342–351. doi: 10.1095/biolreprod.106.050831
Tosca, L., Uzbekova, S., Chabrolle, C., and Dupont, J. (2007b). Possible role of 5’AMP-activated protein kinase in the metformin-mediated arrest of bovine oocytes at the germinal vesicle stage during in vitro maturation. Biol. Reprod. 77, 452–465. doi: 10.1095/biolreprod.107.060848
Towers, C. G., and Thorburn, A. (2016). Therapeutic targeting of autophagy. EBioMedicine 14, 15–23. doi: 10.1016/j.ebiom.2016.10.034
Tulipano, G. (2020). How treatments with endocrine and metabolic drugs influence pituitary cell function. Endocr. Connect. 9, R14–R27.
Ulbright, T. M., Amin, M. B., and Young, R. H. (2007). Intratubular large cell hyalinizing sertoli cell neoplasia of the testis: a report of 8 cases of a distinctive lesion of the Peutz-Jeghers syndrome. Am. J. Surg. Pathol. 31, 827–835. doi: 10.1097/pas.0b013e3180309e33
Vandermolen, D. T., Ratts, V. S., Evans, W. S., Stovall, D. W., Kauma, S. W., and Nestler, J. E. (2001). Metformin increases the ovulatory rate and pregnancy rate from clomiphene citrate in patients with polycystic ovary syndrome who are resistant to clomiphene citrate alone. Fertil. Steril 75, 310–315. doi: 10.1016/s0015-0282(00)01675-7
Vazquez-Borrego, M. C., Fuentes-Fayos, A. C., Gahete, M. D., Castano, J. P., Kineman, R. D., and Luque, R. M. (2018). The pituitary gland is a novel major site of action of metformin in non-human primates: a potential path to expand and integrate its metabolic actions. Cell Physiol. Biochem. 49, 1444–1459. doi: 10.1159/000493448
Velazquez, E. M., Mendoza, S., Hamer, T., Sosa, F., and Glueck, C. J. (1994). Metformin therapy in polycystic ovary syndrome reduces hyperinsulinemia, insulin resistance, hyperandrogenemia, and systolic blood pressure, while facilitating normal menses and pregnancy. Metabolism 43, 647–654. doi: 10.1016/0026-0495(94)90209-7
Viollet, B., Athea, Y., Mounier, R., Guigas, B., Zarrinpashneh, E., Horman, S., et al. (2009). AMPK: lessons from transgenic and knockout animals. Front. Biosci. 14:19–44. doi: 10.2741/3229
Wang, B., and Cheng, K. K. (2018). Hypothalamic AMPK as a mediator of hormonal regulation of energy balance. Int. J. Mol. Sci. 19:3552. doi: 10.3390/ijms19113552
Weisova, P., Concannon, C. G., Devocelle, M., Prehn, J. H., and Ward, M. W. (2009). Regulation of glucose transporter 3 surface expression by the AMP-activated protein kinase mediates tolerance to glutamate excitation in neurons. J. Neurosci. 29, 2997–3008. doi: 10.1523/jneurosci.0354-09.2009
Wen, J. P., Liu, C., Bi, W. K., Hu, Y. T., Chen, Q., Huang, H., et al. (2012). Adiponectin inhibits KISS1 gene transcription through AMPK and specificity protein-1 in the hypothalamic GT1-7 neurons. J Endocrinol 214, 177–189. doi: 10.1530/joe-12-0054
Wen, J. P., Lv, W. S., Yang, J., Nie, A. F., Cheng, X. B., Yang, Y., et al. (2008). Globular adiponectin inhibits GnRH secretion from GT1-7 hypothalamic GnRH neurons by induction of hyperpolarization of membrane potential. Biochem. Biophys. Res. Commun. 371, 756–761. doi: 10.1016/j.bbrc.2008.04.146
Wingo, S. N., Gallardo, T. D., Akbay, E. A., Liang, M. C., Contreras, C. M., Boren, T., et al. (2009). Somatic LKB1 mutations promote cervical cancer progression. PLoS One 4:e5137. doi: 10.1371/journal.pone.0005137
Woods, A., Johnstone, S. R., Dickerson, K., Leiper, F. C., Fryer, L. G., Neumann, D., et al. (2003). LKB1 is the upstream kinase in the AMP-activated protein kinase cascade. Curr. Biol. 13, 2004–2008. doi: 10.1016/j.cub.2003.10.031
Wu, Y., Viana, M., Thirumangalathu, S., and Loeken, M. R. (2012). AMP-activated protein kinase mediates effects of oxidative stress on embryo gene expression in a mouse model of diabetic embryopathy. Diabetologia 55, 245–254. doi: 10.1007/s00125-011-2326-y
Xiao, X., He, Q., Lu, C., Werle, K. D., Zhao, R. X., Chen, J., et al. (2012). Metformin impairs the growth of liver kinase B1-intact cervical cancer cells. Gynecol. Oncol. 127, 249–255. doi: 10.1016/j.ygyno.2012.06.032
Xie, Y., Wang, Y. L., Yu, L., Hu, Q., Ji, L., Zhang, Y., et al. (2011). Metformin promotes progesterone receptor expression via inhibition of mammalian target of rapamycin (mTOR) in endometrial cancer cells. J. Steroid. Biochem. Mol. Biol. 126, 113–120. doi: 10.1016/j.jsbmb.2010.12.006
Xu, B., Hao, Z., Jha, K. N., Zhang, Z., Urekar, C., Digilio, L., et al. (2008). Targeted deletion of Tssk1 and 2 causes male infertility due to haploinsufficiency. Dev. Biol. 319, 211–222. doi: 10.1016/j.ydbio.2008.03.047
Xue, Y., Zhang, H., Sun, X., and Zhu, M. J. (2016). Metformin improves ileal epithelial barrier function in interleukin-10 deficient mice. PLoS One 11:e0168670. doi: 10.1371/journal.pone.0168670
Young, N. P., Kamireddy, A., Van Nostrand, J. L., Eichner, L. J., Shokhirev, M. N., Dayn, Y., et al. (2016). AMPK governs lineage specification through Tfeb-dependent regulation of lysosomes. Genes Dev. 30, 535–552. doi: 10.1101/gad.274142.115
Yung, M. M., Ross, F. A., Hardie, D. G., Leung, T. H., Zhan, J., Ngan, H. Y., et al. (2016). Bitter Melon (Momordica charantia) extract inhibits tumorigenicity and overcomes cisplatin-resistance in ovarian cancer cells through targeting AMPK signaling cascade. Integr. Cancer Ther. 15, 376–389. doi: 10.1177/1534735415611747
Zhang, L., Jouret, F., Rinehart, J., Sfakianos, J., Mellman, I., Lifton, R. P., et al. (2011). AMP-activated protein kinase (AMPK) activation and glycogen synthase kinase-3beta (GSK-3beta) inhibition induce Ca2+-independent deposition of tight junction components at the plasma membrane. J. Biol. Chem. 286, 16879–16890. doi: 10.1074/jbc.m110.186932
Zhang, L., Li, J., Young, L. H., and Caplan, M. J. (2006). AMP-activated protein kinase regulates the assembly of epithelial tight junctions. Proc. Natl. Acad. Sci. U.S.A. 103, 17272–17277. doi: 10.1073/pnas.0608531103
Zhang, Z., Shen, X., Jones, B. H., Xu, B., Herr, J. C., and Strauss, J. F. III (2008). Phosphorylation of mouse sperm axoneme central apparatus protein SPAG16L by a testis-specific kinase, TSSK2. Biol. Reprod. 79, 75–83. doi: 10.1095/biolreprod.107.066308
Zhong, W., Xie, Y., Abdallah, M., Awonuga, A. O., Slater, J. A., Sipahi, L., et al. (2010). Cellular stress causes reversible, PRKAA1/2-, and proteasome-dependent ID2 protein loss in trophoblast stem cells. Reproduction 140, 921–930. doi: 10.1530/rep-10-0268
Zhou, J., Kumar, T. R., Matzuk, M. M., and Bondy, C. (1997). Insulin-like growth factor I regulates gonadotropin responsiveness in the murine ovary. Mol. Endocrinol. 11, 1924–1933. doi: 10.1210/mend.11.13.0032
Keywords: AMPK, GnRH, reproduction, spermatogenesis, transcription
Citation: Yang W, Wang L, Wang F and Yuan S (2020) Roles of AMP-Activated Protein Kinase (AMPK) in Mammalian Reproduction. Front. Cell Dev. Biol. 8:593005. doi: 10.3389/fcell.2020.593005
Received: 09 August 2020; Accepted: 23 October 2020;
Published: 19 November 2020.
Edited by:
Benoit Viollet, Institut National de la Santé et de la Recherche Médicale (INSERM), FranceReviewed by:
Joelle Dupont, INRAE, Centre Val de Loire, FrancePedro F. Oliveira, Health Sciences Research Centre, Portugal
Copyright © 2020 Yang, Wang, Wang and Yuan. This is an open-access article distributed under the terms of the Creative Commons Attribution License (CC BY). The use, distribution or reproduction in other forums is permitted, provided the original author(s) and the copyright owner(s) are credited and that the original publication in this journal is cited, in accordance with accepted academic practice. No use, distribution or reproduction is permitted which does not comply with these terms.
*Correspondence: Fengli Wang, d2FuZ2ZlbmdsaUBodXN0LmVkdS5jbg==; Shuiqiao Yuan, c2h1aXFpYW95dWFuQGh1c3QuZWR1LmNu