- 1Department of Histology, Faculty of Medicine, Universitas Indonesia, Jakarta, Indonesia
- 2Stem Cell Medical Technology Integrated Service Unit, Dr. Cipto Mangunkusumo General Hospital, Faculty of Medicine, Universitas Indonesia, Jakarta, Indonesia
- 3Stem Cell and Tissue Engineering Research Center, Indonesia Medical Education and Research Institute, Faculty of Medicine, Universitas Indonesia, Jakarta, Indonesia
- 4Division of Cardiovascular Sciences, Faculty of Biology, Medicine and Health, Manchester Academic Health Science Centre, The University of Manchester, Manchester, United Kingdom
- 5Division of Chemical Engineering, Department of Materials Engineering Science, Graduate School of Engineering Science, Osaka University, Toyonaka, Japan
- 6Department of Biochemistry and Molecular Biology, Faculty of Medicine, Universitas Indonesia, Jakarta, Indonesia
- 7Department of Orthopaedic and Traumatology, Dr. Cipto Mangunkusumo General Hospital, Faculty of Medicine, Universitas Indonesia, Jakarta, Indonesia
- 8Department of Biomedical Science, Faculty of Medicine, Universitas Airlangga, Surabaya, Indonesia
Background: The therapeutic capacity of mesenchymal stem cells (also known as mesenchymal stromal cells/MSCs) depends on their ability to respond to the need of the damaged tissue by secreting beneficial paracrine factors. MSCs can be genetically engineered to express certain beneficial factors. The aim of this systematic review is to compile and analyze published scientific literatures that report the use of engineered MSCs for the treatment of various diseases/conditions, to discuss the mechanisms of action, and to assess the efficacy of engineered MSC treatment.
Methods: We retrieved all published studies in PubMed/MEDLINE and Cochrane Library on July 27, 2019, without time restriction using the following keywords: “engineered MSC” and “therapy” or “manipulated MSC” and “therapy.” In addition, relevant articles that were found during full text search were added. We identified 85 articles that were reviewed in this paper.
Results: Of the 85 articles reviewed, 51 studies reported the use of engineered MSCs to treat tumor/cancer/malignancy/metastasis, whereas the other 34 studies tested engineered MSCs in treating non-tumor conditions. Most of the studies reported the use of MSCs in animal models, with only one study reporting a trial in human subjects. Thirty nine studies showed that the expression of beneficial paracrine factors would significantly enhance the therapeutic effects of the MSCs, whereas thirty three studies showed moderate effects, and one study in humans reported no effect. The mechanisms of action for MSC-based cancer treatment include the expression of “suicide genes,” induction of tumor cell apoptosis, and delivery of cytokines to induce an immune response against cancer cells. In the context of the treatment of non-cancerous diseases, the mechanism described in the reviewed papers included the expression of angiogenic, osteogenic, and growth factors.
Conclusion: The therapeutic capacity of MSCs can be enhanced by inducing the expression of certain paracrine factors by genetic modification. Genetically engineered MSCs have been used successfully in various animal models of diseases. However, the results should be interpreted cautiously because animal models might not perfectly represent real human diseases. Therefore, further studies are needed to explore the translational potential of genetically engineered MSCs.
Introduction
Mesenchymal stem cells (MSCs) or mesenchymal stromal cells are adult multipotent stem cells that can be differentiated to other cell types such as bones, cartilage, skeletal muscles, and connective tissues. MSCs can be isolated from various sources, including bone marrow, adipose tissue, and the mucoid tissue within the umbilical cord (Wharton’s jelly) (Pawitan et al., 2013, 2014; Budiyanti et al., 2015). A large number of studies, including several double-blind randomized clinical trials, have reported the use of MSCs in treating various conditions (Mohamadnejad et al., 2013; Pawitan et al., 2018; Freitag et al., 2019). Most of the studies showed that MSCs exert their beneficial effects mainly through the secretion of paracrine factors (Lee et al., 2011; Mirotsou et al., 2011; Mohamadnejad et al., 2013; Freitag et al., 2019). Moreover, MSC-based therapy has obtained approval for clinical use in some countries. For example, MSC-based therapies have been approved for the treatment of acute graft-versus-host disease, critical limb ischemia (Buerger’s disease), and complex perianal fistulas in adults due to Crohn’s disease in Japan, India, and the European Union, respectively (Robb et al., 2019). It is thought that the therapeutic capacity of MSCs largely depends on their ability to secrete beneficial factors to repair the damaged tissues or organs. Therefore, genetically engineered MSCs that can specifically express paracrine factors, which are needed in a particular pathological condition, may be more effective in treating the disease compared to native MSCs. The aim of this article is to systematically review the literatures on the use of genetically engineered MSCs in various diseases and to assess their safety and efficacy. This review will provide an update on the mechanisms of action of engineered MSCs in the treatment of various conditions.
Methods
This systematic review complies with the Preferred Reporting Items for Systematic Review (PRISMA) guidelines. The protocol was submitted to the PROSPERO database of systematic review protocols (registration number: CRD 42020163707). We have checked the Cochrane Library to ensure that there was no systematic review on a similar topic.
We performed a PubMed/MEDLINE and Cochrane database search for relevant published studies on July 27, 2019, without time restriction using the following search terms: “engineered MSC” AND “therapy” or “manipulated MSC” AND “therapy.” In addition, relevant articles found during full text search were reviewed.
All original articles on the therapeutic use of engineered MSC or manipulated MSC were included. Studies on engineered MSC or manipulated MSC therapy that did not provide data on the treated conditions or disease models, type of MSC manipulation, detail information on expressed protein, administration route, and outcome of the treatment were excluded from this review. Article selection was conducted by examination of the titles, abstracts, and full texts.
The data collected include the following: the treated disease or disease models, the species used, the number of animals/participants, the source of the MSC, MSC passage, the type of gene insertion and expressed protein, the outcomes of the treatment, adverse events, and mechanism of action.
Data analysis: the studies were grouped and tabulated according to the treated conditions/disease models, species, number of animals, MSC source and passage, vector used, types of gene insertion/expressed proteins, outcome, adverse event, and mechanism of action.
Data were analyzed descriptively to determine the safety and efficacy of a certain engineered MSC on a certain disease and the possible mechanism.
Results and Discussion
Using the keywords “engineered MSC” AND “therapy,” we identified 26 articles from the PubMed database, of which 17 articles were included for review. Six articles from the Cochrane Library database were identified using the same keywords (of which one was selected for review). Using the keywords “manipulated MSC” AND “therapy,” we identified 50 articles from the PubMed database, of which six papers were selected for review. The same keywords were used in the Cochrane database, and we have identified five articles, but none of them met the inclusion criteria for review. During full text search, we found 61 relevant articles that met the inclusion criteria, and thus, the total number of articles included in the review was 85 (Figure 1).
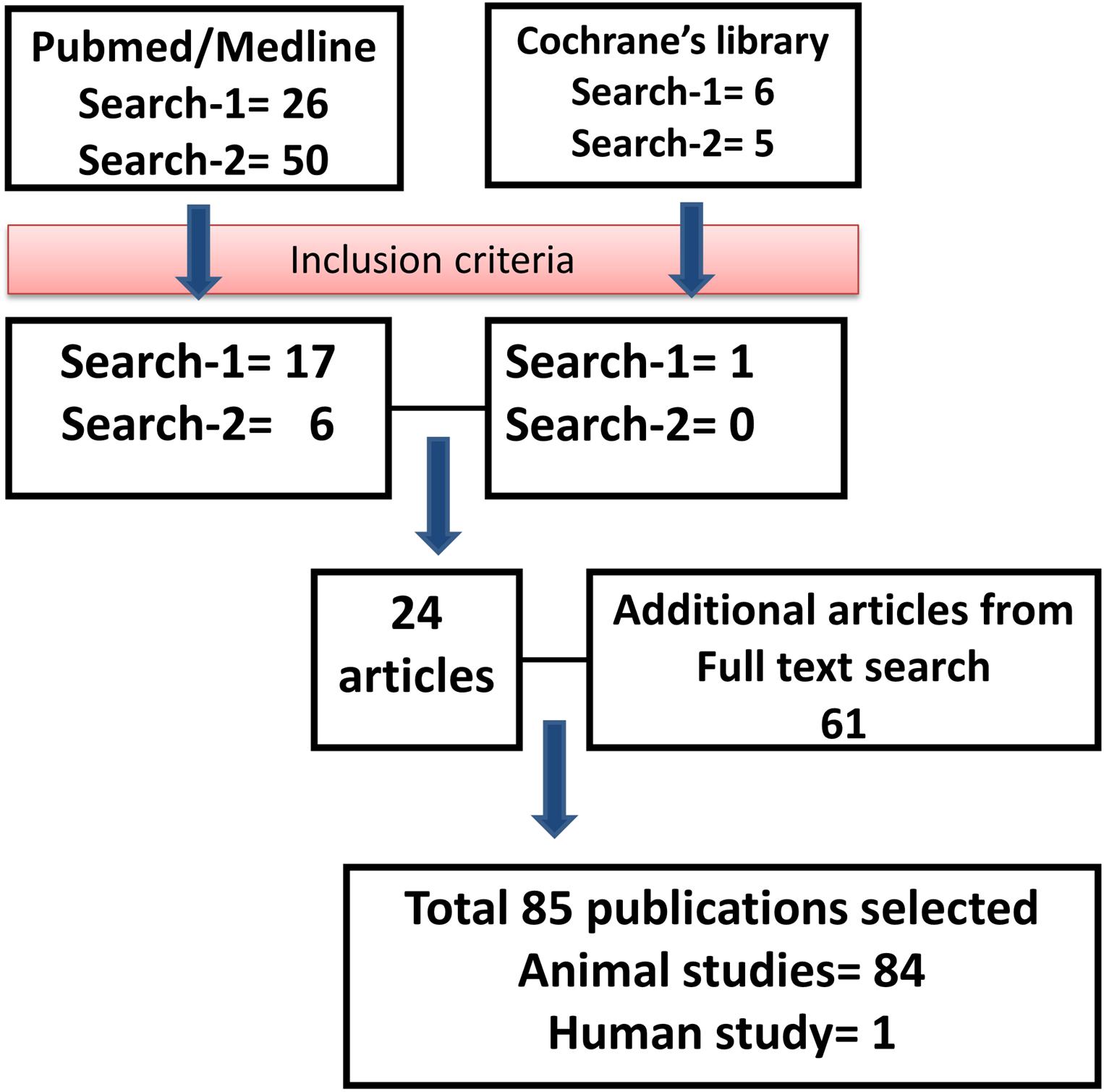
Figure 1. Outline of the literature search. Flowchart describing the protocol and literature search used in this systematic review.
The conditions/disease models treated by engineered MSC were grouped into two main groups: (i) tumor, cancer, malignancy, and metastasis (Supplementary Table 1) and (ii) non-tumor conditions (Supplementary Table 2).
Engineered MSCs were mostly used to treat tumor/cancer/malignancy/metastasis (51 studies, Supplementary Table 1), and the rest of the studies reported the use of engineered MSCs to treat various non-tumor conditions (34 studies, Supplementary Table 2). MSCs from several different sources and species were used in the reviewed papers, i.e., human bone marrow (BM) MSCs (Sasportas et al., 2009; You et al., 2009; Chang et al., 2010; Gao et al., 2010; Wang et al., 2012; Altaner et al., 2014; Harati et al., 2015; NguyenThai et al., 2015; Nouri et al., 2015; Beegle et al., 2016; Chung et al., 2016; Schug et al., 2018; Segaliny et al., 2019), human adipose tissue (AT) MSCs (Kucerova et al., 2007, 2008, 2014; Cavarretta et al., 2010; Altanerova et al., 2012; Zolochevska et al., 2012; Altaner et al., 2014; Grisendi et al., 2015; Matuskova et al., 2015; Tyciakova et al., 2015; Toro et al., 2016; Roudkenar et al., 2018), rat BM-MSCs (Tsuchida et al., 2003; Li et al., 2007; Jiang et al., 2009; Huang et al., 2010; Zhang et al., 2010, 2011; Zhao et al., 2010; Fei et al., 2012; Kosaka et al., 2012; Kim et al., 2013; Nakamura et al., 2013; Hu et al., 2014; Niu et al., 2014; Qi et al., 2015; Yao et al., 2017; Roudkenar et al., 2018), human embryonic stem cell (ESC) derived MSC (Bak et al., 2011), murine BM-MSCs (Kumar and Ponnazhagan, 2007; Chen et al., 2008; Ren et al., 2008; Xin et al., 2009; Conrad et al., 2011; Zhu et al., 2012; Zou et al., 2012; Fransson et al., 2014; Amara et al., 2016), mouse AT-MSC (Abrate et al., 2014; Krasikova et al., 2015, Krassikova et al., 2016), canine AT-MSCs (Seo et al., 2011; Ahn et al., 2013), human umbilical cord (UC) MSCs (Yan et al., 2013), rabbit BM-MSCs (Chang et al., 2004; Lin et al., 2010, 2012a; Fu et al., 2015; Wang et al., 2015b), pig BM-MSCs (autologous) (Chang et al., 2003; Chang et al., 2010), pig AT-MSCs (Lin et al., 2015), and rabbit AT-MSCs (Lin et al., 2011, 2012b; Lin et al., 2013). Only 2 out of 85 studies used autologous cells (Chang et al., 2003; Chang et al., 2010), and most of the reported studies used allogeneic cells. Some studies reported the use of human-derived MSCs in animal models, which could be regarded as xenogeneic cells, but no adverse immune reaction was reported. This might be due to the immune privilege and immune modulation properties of MSCs (Andreeva et al., 2017).
The genetic modification of the MSCs was achieved using different approaches, including viral and non-viral approaches. The types of viral vectors used for MSC genetic manipulation include the following: adenovirus (Chang et al., 2003, 2004; Chang et al., 2010; Tsuchida et al., 2003; Chen et al., 2008; Fritz et al., 2008; Jiang et al., 2009; Xin et al., 2009; Gao et al., 2010; Huang et al., 2010; Zhang et al., 2010; Zhao et al., 2010; Kosaka et al., 2012; Wang et al., 2012; Kim et al., 2013), lentivirus (Sasportas et al., 2009; Luetzkendorf et al., 2010; Bak et al., 2011; Seo et al., 2011; Zhang et al., 2011; Fei et al., 2012; Zolochevska et al., 2012; Ahn et al., 2013; Lee et al., 2013; Martinez-Quintanilla et al., 2013; Yan et al., 2013, 2017; Fransson et al., 2014; Niu et al., 2014; Qi et al., 2015; Wang et al., 2015b; Amara et al., 2016; Beegle et al., 2016), retrovirus (Kucerova et al., 2007, 2008, 2014; Cavarretta et al., 2010; Chang et al., 2010; Altanerova et al., 2012; Zou et al., 2012; Martinez-Quintanilla et al., 2013; Abrate et al., 2014; Grisendi et al., 2015; Lakota et al., 2015; Matuskova et al., 2015; Tyciakova et al., 2015; Chung et al., 2016; Toro et al., 2016), baculovirus (Lin et al., 2010, 2011, 2012a,b; Lin et al., 2015; Bak et al., 2011; Fu et al., 2015), adeno associated virus (Kumar and Ponnazhagan, 2007; Ren et al., 2008), and cytomegalovirus (Conrad et al., 2011). The non-viral approaches reported include the following: mRNA–lipofectamine-mediated transfection (Segaliny et al., 2019), PEGylated DNA-templated nano-composite system (Suryaprakash et al., 2019), plasmid DNA transfection (You et al., 2009; Harati et al., 2015; Krasikova et al., 2015, Krassikova et al., 2016; NguyenThai et al., 2015; Nouri et al., 2015; Mirzaei et al., 2018; Roudkenar et al., 2018; Schug et al., 2018), biotinylated MSCs (avidin link protein) (Yao et al., 2017), spermin pullulan (Nakamura et al., 2013; Hu et al., 2014), hyper-branched poly-amido-amine (hPAMAM) (Zhu et al., 2012), and jetPEI mediated transfection (Li et al., 2007). Most of the approaches were successful in transferring various genes into the MSCs and in inducing secretion of the protein of interest.
It is important to note that in gene therapy experiments, viral vectors – particularly adenovirus – often cause a strong immune reaction against the vector (Xin et al., 2009). However, when used to transfect MSCs in vitro, the transfected MSCs did not induce an adverse immune reaction following transplantation to host tissues (Xin et al., 2009).
The Use of Engineered MSCs to Treat Tumor/Cancer/Malignancy/Metastasis
Despite recent improvements in prognosis and treatment modalities, cancer remains one of the major causes of death, particularly when metastasized tumors become resistant to conventional surgical and chemoradiotherapeutic treatment strategies (Chulpanova et al., 2018). However, recent studies on stem cell-based therapies have provided promising results in the development of new strategies for cancer treatment (Zhang et al., 2017). MSCs appear to be one potential candidate for stem cell-based anti-cancer therapies. MSCs are a group of adult stem cells that exert tumor tropism behavior and play various roles in regulating cancer cell biology. Therefore, these cells have been used as vehicles to deliver substances to the primary tumor or metastatic tumor sites.
Mesenchymal stromal cells have attracted considerable attention in the field of tumor therapy because of their unique biological properties. In most circumstances, tumor cells reside in a complex microenvironment, which also contains other cell types such as endothelial cells, fibroblasts, and other bone marrow-derived cells such as macrophages (Lin et al., 2019). Within this microenvironment complex, tumor cells secret a spectrum of chemokines, cytokines, and growth factors to attract MSCs to the tumor sites. Meanwhile, MSCs could secrete cytokines or chemokines, which orchestrate the fate and development of tumor cells. MSCs are also safe to be used in allogeneic transplantation because they do not express co-stimulatory molecules that trigger graft rejection and immune responses (Chulpanova et al., 2018).
Some pre-clinical studies have shown that MSCs could be genetically manipulated to express cytotoxic agents to inhibit tumor growth (Lin et al., 2019). There are various kinds of studies using different strategies and animal models to test the efficacy of engineered MSCs for the treatment of cancer/metastasis. In some studies, engineered MSCs were delivered after the tumors or metastatic lesions were established in animal models (Chen et al., 2008; Ren et al., 2008; Xin et al., 2009; Gao et al., 2010; Bak et al., 2011; Conrad et al., 2011; Zhang et al., 2011; Wang et al., 2012; Kim et al., 2013; Lee et al., 2013; Martinez-Quintanilla et al., 2013; Yan et al., 2013; Abrate et al., 2014; Altaner et al., 2014; Hu et al., 2014; Harati et al., 2015; Krasikova et al., 2015, Krassikova et al., 2016; Lakota et al., 2015; Matuskova et al., 2015; Nouri et al., 2015; Chung et al., 2016; Yan et al., 2017; Yao et al., 2017; Mirzaei et al., 2018; Schug et al., 2018; Segaliny et al., 2019; Suryaprakash et al., 2019). In other studies, tumor cells and engineered MSCs were injected simultaneously in animal models (Fritz et al., 2008; Sasportas et al., 2009; You et al., 2009; Cavarretta et al., 2010; Chang et al., 2010; Seo et al., 2011; Altanerova et al., 2012; Fei et al., 2012; Zolochevska et al., 2012; Ahn et al., 2013; Kucerova et al., 2014; Grisendi et al., 2015; NguyenThai et al., 2015; Tyciakova et al., 2015), whereas other studies used the combination of both approaches (Kucerova et al., 2007, 2008; Luetzkendorf et al., 2010; Kosaka et al., 2012; Zou et al., 2012; Amara et al., 2016; Toro et al., 2016). Co-injection of a mixture of tumor cells and engineered MSCs was intended to know whether engineered MSCs could prevent tumor development. However, this approach is not suitable in the clinical setting because patients are treated when the tumors are already established. The use of engineered MSCs for cancer prevention may not be feasible for clinical application because we do not know which patient will develop a certain type of tumor.
The genetically engineered MSCs are often used in conjunction with treatment with chemotherapeutic drugs. Thus, it is crucial to understand the effects of the chemotherapeutic agents on the MSCs themselves. MSCs’ responses to chemotherapeutic drugs vary depending on the mechanisms of action of the anti-cancer drugs used. However, in general, MSCs can be considered as more resistant cells against chemotherapeutic agents because of their highly efficient DNA repair, higher level of anti-apoptotic protein, and higher anti-oxidant activity as reviewed in previous publications (Ruhle et al., 2018, 2019).
There are three different strategies to generate anti-cancer MSCs through genetic modification: (i) by inserting suicide gene carriers that will activate a non-toxic pro-drug to become a cytotoxic substance that can kill the tumor cells, (ii) by using MSCs as vehicles to deliver cytokines for cancer immunotherapy; and (iii) by using MSCs as agents to induce tumor cell apoptosis (Figure 2).
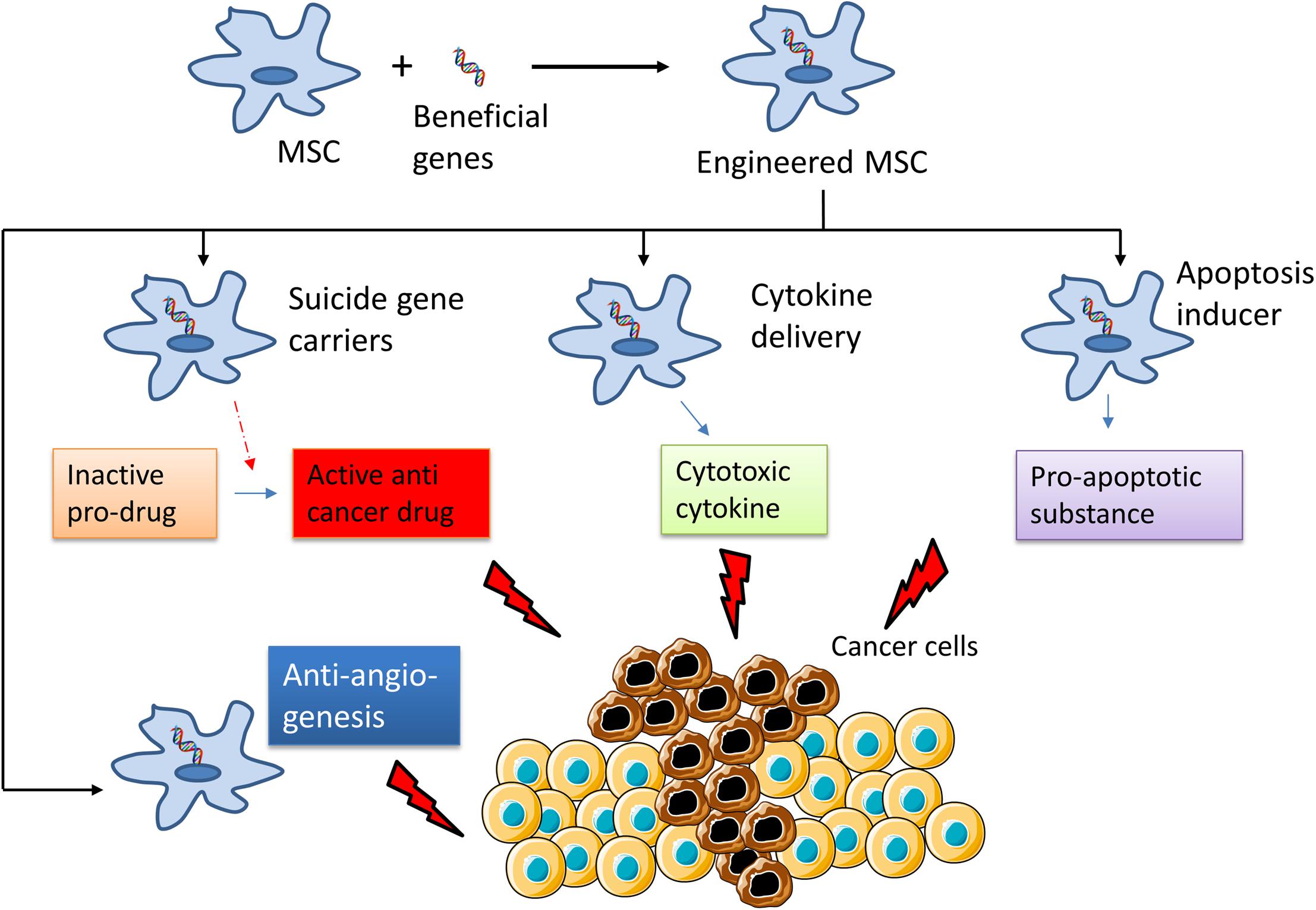
Figure 2. Summary of the mechanisms of the use of engineered Mesenchymal Stem Cells for cancer treatment. MSCs are genetically modified to express “suicide genes,” which can activate an inactive pro-drug to active anti-cancer drug; cytotoxic cytokines or apoptosis inducer that can kill cancer cells. Some part of this figure was created using Servier Medical Art templates, which are licensed under a Creative Commons Attribution 3.0 Unported License; https://smart.servier.com.
MSCs as Suicide Gene Carriers That Activate Non-toxic Pro-drugs Into Toxic Substances
One of the main challenges of the current first-line cancer therapies, especially chemotherapy, is systemic cytotoxicity, and limited delivery to the tumor sites. To tackle these problems, genetically modified MSCs carrying suicide genes have been employed to convert non-toxic pro-drugs into active agents for selective elimination of cancer cells. Various genes encoding these “suicide proteins” were used: cytosine deaminase (CD) or cytosine deaminase-uracil phosphoribosyltransferase (CD-UPRT), which converts the pro-drug 5-fluorocytosine (5-FC) into an active agent 5-fluorouracil (5-FU) (Kucerova et al., 2007, 2014; You et al., 2009; Cavarretta et al., 2010; Chang et al., 2010; Conrad et al., 2011; Altanerova et al., 2012; Fei et al., 2012; Kosaka et al., 2012; Abrate et al., 2014; Krasikova et al., 2015, Krassikova et al., 2016; Lakota et al., 2015; Matuskova et al., 2015; NguyenThai et al., 2015; Chung et al., 2016; Toro et al., 2016; Segaliny et al., 2019); herpes simplex virus thymidilate kinase (HSV-TK) (Bak et al., 2011; Martinez-Quintanilla et al., 2013; Nouri et al., 2015) or SV40-TK (Lee et al., 2013), which phosphorylates gancyclovir (GCV) into a toxic substance; and cytochrome P450 reductase (CYP), which converts cyclophosphamide (CPA) into cytotoxic metabolites (Amara et al., 2016).
A study showed that human AT-MSCs expressing cytosine deaminase (CD) could trigger dose-dependent apoptosis of human melanoma A375 cells in both in vitro and in vivo models (Kucerova et al., 2008). Subcutaneous injection of 20% AT-MSC-CD in a mixture with A375 cells resulted in a complete regression of 89% of the tumor-bearing animals within 14 days (Kucerova et al., 2008). Moreover, AT-MSC-CD administered systemically exhibited tumor tropism and suppressed tumor growth in the presence of 5-FC.
Other pieces of evidence showing the promising therapeutic potential of MSCs in suppressing melanoma tumors have been provided by a study using a more aggressive variant of melanoma cells, that is, EGFP-A375/Rel3, which exhibited altered cell adhesion and tumorigenic and metastatic properties (Kucerova et al., 2014). The combination of AT-MSC-CD/5-FC treatment with SU11274, an inhibitor of the c-Met/hepatocyte growth factor signaling pathway, could provide a complete cure in 9 out of 10 animals at 60 days after EGFP-A375/Rel3 cell injection (Kucerova et al., 2014). Augmentation of CD with herpes virus 1 (HSV-1) tegument protein VP22 in a CD-UPRT fusion construct could also enhance the therapeutic effects of the MSC-CD-UPRT combination (Krasikova et al., 2015). Consistent with studies using animal models, human BM-MSC-CD could migrate to the subcutaneous human gastric cancer MKN45 cells and induce tumor regression in the presence of 5-FC (You et al., 2009). Importantly, these studies have suggested the importance of identifying optimal timing and augmentation of the treatment to maximize the anti-tumor effects of the MSCs.
As mentioned previously, one major issue on the use of MSCs in combination with chemotherapeutic substances is the toxicity of the chemotherapeutic drugs on the MSCs. MSCs have been shown to have some degree of resistance against alkylating agents such as cyclophosphamide, melphalan, and busulfan (Nifontova et al., 2008; Kemp et al., 2011). It has also been reported that MSCs displayed high resistance against cisplatin, a platinum-based anti-cancer drug (Bellagamba et al., 2016; Nicolay et al., 2016). In addition, treatment with methotrexate did not affect the survival and proliferative capacity of MSCs (Mancheno-Corvo et al., 2013; Beane et al., 2014). However, studies on the effects of nucleoside analog 5-FU on MSCs are rather limited. One study suggested that low doses of 5-FU reduced MSC viability. However, others have demonstrated a lower level of senescence in MSCs treated with 5-FU compared to those treated with other chemotherapeutics agents such as doxorubycin, methotrexate, or busulfan (Qi et al., 2012). The origin of the MSCs may also determine that the response to 5-FU with adipose-derived MSCs appears to have lower sensitivity against 5-FU compared to bone marrow-derived MSCs (Ruhle et al., 2018).
MSCs as Vehicles for Cytokine Delivery in Cancer Immunotherapy
In addition to the application in delivering “suicide genes,” engineered MSCs have been used as tools to deliver anti-cancer cytokines to the tumor local environment. Thus, treatment using engineered MSCs has been regarded as one important approach in the field of cancer immunotherapy In a study on 786-0 renal cancer cell xenografts, MSCs expressing interleukin-12 could migrate to the tumor site and inhibit tumor growth through the activation of the natural killer cells and secretion of interferon γ at 14 days after MSC administration (Gao et al., 2010). In a mouse model of subcutaneous B16F10 melanoma xenograft, a combined treatment of canine AT-MSCs expressing interferon β (IFN-β) along with systemic administration of the platinum-containing anti-cancer drug cisplatin could significantly inhibit tumor growth at 14 days after tumor cell injection compared with a cisplatin monotherapy group. This method could also increase mouse survival up to 51 days after tumor cell injection compared to 20 days of survival in the control group (Seo et al., 2011). Consistently, subcutaneous injection of AT-MSC-IFN-β along with a low dose of systemic cisplatin could induce cell cycle arrest and apoptosis of LMeC melanoma xenografts (Ahn et al., 2013).
Another study reported that engineered MSCs expressing inflammatory cytokine TNFα could trigger caspase 3/7-dependent apoptosis of human A375 melanoma cells in vitro (Tyciakova et al., 2015). This study also showed that the subcutaneous injection of MSC-hTNFα and A375 melanoma cells in a 1:4 ratio could induce tumor regression up to 97.5% in the recipient mice.
In addition to the anti-tumor effects at the primary tumor site, the engineered MSCs might be able to control metastasized tumor growth as demonstrated in several studies. For example, systemic administration of hAT-MSC-CXCL10 could inhibit Treg cell-driven lung metastasized tumors and elevate the number of anti-tumor activated T-cells in the lungs in animals receiving B16F10-induced melanoma cells (Mirzaei et al., 2018). On the other hand, systemic administration of MSC–lipocalin 2 could inhibit liver metastasis through the downregulation of vascular endothelial growth factors in the liver in a mouse model of SW48 colon cancer intrasplenic xenografts (Harati et al., 2015). Together, these studies suggested that cytokine-based targeted therapy using MSCs might be effective in tackling a range of tumor cell types and possibly cancer metastasis.
Engineered MSCs That Target Tumor Cell Apoptosis
Another strategy in using MSCs for cancer therapy is to use these cells to deliver pro-apoptotic proteins to tumor cells (Sasportas et al., 2009; Luetzkendorf et al., 2010; Kim et al., 2013; Yan et al., 2013; Grisendi et al., 2015; Suryaprakash et al., 2019). Tumor necrosis factor (TNF)-related apoptosis-inducing ligand (TRAIL) is a transmembrane protein that binds to death domain-containing receptors and selectively triggers the extrinsic apoptotic pathway within cancer cells while sparing other cell types (Luetzkendorf et al., 2010). Because most current chemotherapy agents mainly act as DNA damage sensors and activate the intrinsic apoptotic pathway of cancer cells, MSC-TRAIL could be used in conjunction with first-line clinical therapies to provide synergistic treatment effects.
The therapeutic potential of MSC-TRAIL has been demonstrated in several pre-clinical models of sarcoma, lung cancer metastasis, renal cancer, colorectal cancer, and lymphomas. For example, co-injection of mixtures of human MSC-TRAIL with mouse colorectal cancer cells could effectively inhibit tumor growth via apoptosis activation. However, this therapeutic effect was abolished when TRAIL-MSCs were delivered through intravenous injection (Luetzkendorf et al., 2010). MSCs expressing human TRAIL could induce caspase 8 activation and apoptosis of RD-ES sarcoma cells while retaining anti-angiogenic effects (Grisendi et al., 2015). Engineered MSCs expressing TRAIL and herpes simplex virus thymidine kinase (MSC-TRAIL-TK) have shown efficacy in inducing apoptosis of renal carcinoma (RENCA) cells and inhibiting lung metastasis. Triple intravenous injections of MSC-TRAIL-TK could offer 100% survival rate and induce a complete cure for metastatic tumor-bearing mice (Kim et al., 2013).
mesenchymal stromal cell-TRAIL can be modified further to enhance their binding specificity to tumor cells. In a study using a mouse model of non-Hodgkin’s lymphoma, engineered MSCs expressing both CD20-specific single-chain Fv antibody fragment and TRAIL could migrate to established CD20-positive B-cell lymphoma line (BJAB) subcutaneous tumor in NOD/SCID mice and induce 65% tumor regression without causing liver toxicity compared to 42.7% of tumor regression in groups receiving MSC-TRAIL only (Yan et al., 2013).
So far, most studies using pre-clinical cancer models have shown consistent results, i.e., the engineered MSCs could inhibit tumor growth and enhance the survival rate of the tumor-bearing animals. However, a recent clinical report of a patient treated with engineered MSC-CD-UPRT and 5-FC for metastatic head and neck cancer has shown that there was no sign of tumor regression at 6 days after intravenous injection of the therapeutic stem cells. Moreover, tumor metastasis further progressed after 40 days of the treatment (Lakota et al., 2015). Therefore, success in animal studies should be interpreted with caution. Further studies to understand the biology, fate, and safety of grafted MSCs would be needed for successful clinical translation of MSC-based cancer therapies.
Other Genetic Strategies to Enhance the Anti-tumor Effects of MSCs
Formation of new blood vessels (angiogenesis) has been regarded as one of the key factors in the progression of cancers. Treatment with anti-angiogenetic agents appears to become one promising approach in cancer therapy. MSCs have been considered as effective vehicles to deliver such factors. Several lines of evidence have shown the use of genetic modification to generate MSCs that are capable of sending anti-angiogenetic factors to the tumor sites. For example, MSCs overexpressing endostatin have been reported to be effective in controlling the growth of ovarian cancer. It was shown that the beneficial effect was likely due to the inhibition of neovascularization, enhanced apoptosis, and inhibition of cell proliferation (Zheng et al., 2012). Equally important, the fact that MSCs can be engineered to express intact or single-chain antibody fragments (Frank et al., 2010) has opened the possibility to generate MSCs that are capable of secreting anti-angiogenetic antibodies, such as an anti-VEGF antibody.
Another strategy in the development of anti-cancer MSCs is by using these cells to deliver oncolytic adenovirus. One example of this strategy is the use of MSCs to carry and deliver the Delta-24-RGD adenovirus, which has a potent anti-glioma property (Yong et al., 2009). Experimental evidence in mice has demonstrated the efficacy of this approach in eradicating brain glioma (Yong et al., 2009).
Genetic Engineering and the Pro-tumorigenic Effect of MSCs
One important aspect of MSC-based therapy that may hamper the clinical translation is the capability of MSCs to promote cancer progression. Co-injection of MSCs with cancer cells in vivo can induce the growth of the tumor cells. This has been observed in models of ovarian (Spaeth et al., 2009), prostate (Jung et al., 2013), colorectal (De Boeck et al., 2013), and breast cancer (Karnoub et al., 2007). It is thought that the pro-tumorigenic effects of MSCs might be due to the cell-to-cell contact and/or secretion of paracrine factors (Babajani et al., 2020). In vitro studies showed that co-culturing of primary cancer cells with bone marrow-derived MSCs significantly reduced the apoptosis of the cancer cells. Direct cell-to-cell contact was proposed as the anti-apoptotic mechanism, although the ligands and/or the receptors that are responsible for the interaction are not known yet (Lee et al., 2019).
Equally important, several lines of evidence have suggested that MSCs that are recruited to the tumor microenvironment might secrete paracrine factors and cytokines that can facilitate tumor growth, angiogenesis, and survival against anti-cancer drugs. For example, injected MSCs to cancer sites produce pro-growth factors such as insulin-like growth factor, platelet-derived growth factor, and epidermal growth factor receptor (Akimoto et al., 2013), as well as cytokines and factors like IL-6 (Lin et al., 2013) and TGF-b (Ye et al., 2012). Interestingly, the secretory profiles of MSCs recruited to the tumor sites are different from those of primary MSCs outside the tumor microenvironment, suggesting that the pro-tumor phenotype might be due to the local niche produced by the tumor cells (Babajani et al., 2020).
It is important to note that in the majority of observations showing the pro-tumorigenic effects of MSCs, the researchers used native MSCs in their models. By contrast, most of the studies using genetically manipulated MSCs as outlined above have reported significant anti-tumor effects as opposed to the pro-tumorigenic effect. This suggests that genetic modification might be a promising strategy to control the pro-tumorigenic effects of MSCs. In addition, drug-loading has been demonstrated to be an effective approach to control the pro-tumorigenic phenotype. For example, drug priming with paclitaxel has been shown to improve the anti-tumor activity of MSCs and induce the anti-angiogenic effect by inhibition of ICAM1, VCAM1, and VEGF expressions (Pessina et al., 2011).
The Use of Engineered MSC to Treat Non-cancer Conditions
Engineered MSCs were used to treat various non-cancer conditions, including allogeneic liver transplantation (Niu et al., 2014; Qi et al., 2015), autoimmune encephalomyelitis (Fransson et al., 2014), nerve injury (Wang et al., 2015b), wound healing (Nakamura et al., 2013), critical limb ischemia (Beegle et al., 2016), acute kidney injury (Roudkenar et al., 2018), myocardial infarction (Li et al., 2007; Huang et al., 2010; Zhang et al., 2010; Zhu et al., 2012), various bone defects (Chang et al., 2003, 2004; Chang et al., 2010; Tsuchida et al., 2003; Jiang et al., 2009; Lin et al., 2010, 2011, 2012a,b; Lin et al., 2015; Zhao et al., 2010; Fu et al., 2015), lung fibrosis (Min et al., 2015), radiation-induced toxicity (Abdel-Mageed et al., 2009; Xue et al., 2013; Zhang et al., 2014; Wang et al., 2015a; Zhang et al., 2019), Huntington’s disease (Dey et al., 2010), and rheumatoid arthritis (Liu et al., 2013; Dong et al., 2020; Figure 3 and Supplementary Table 2).
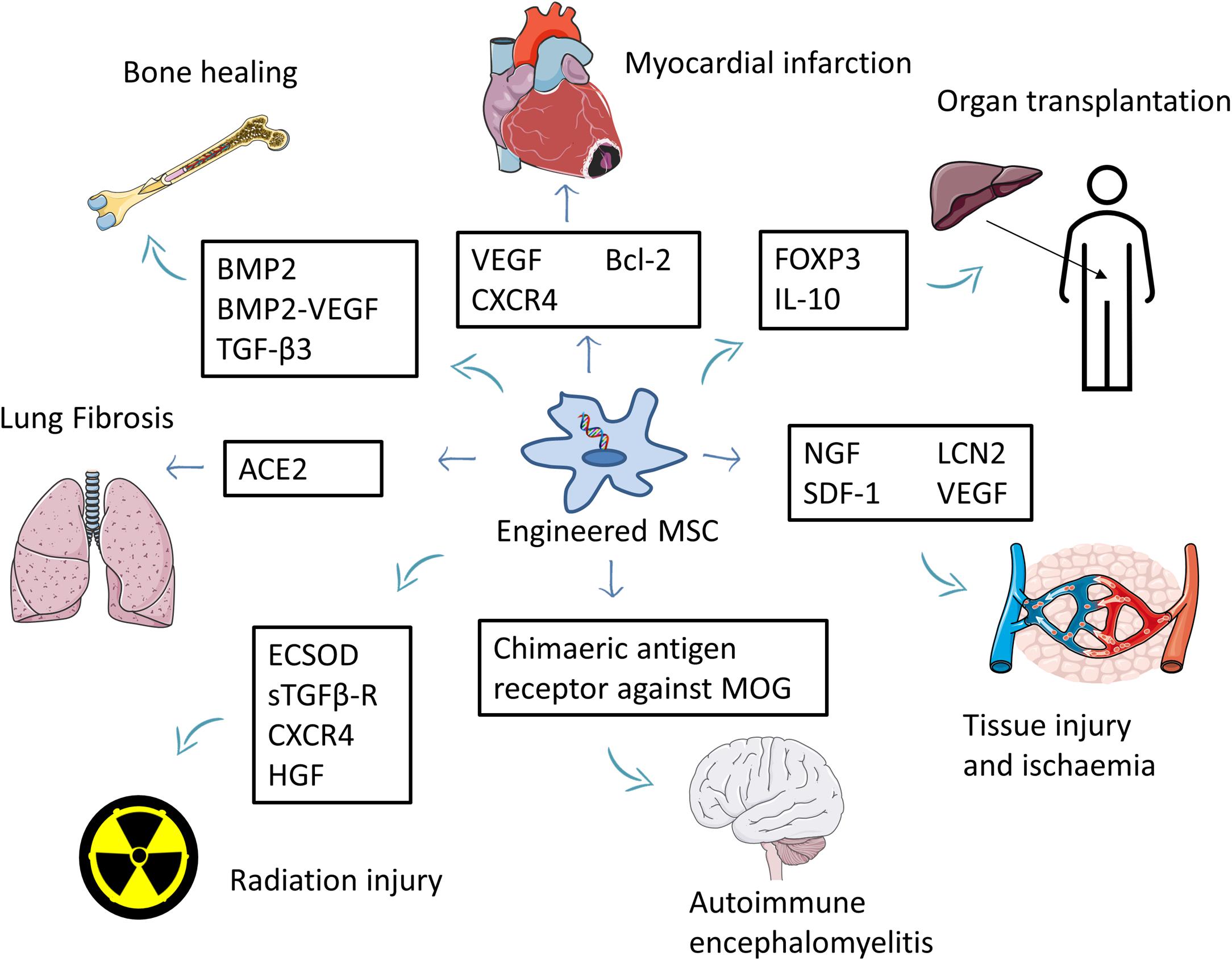
Figure 3. Summary of the use of engineered MSCs for the treatment of non-cancer diseases. MSCs overexpressing beneficial factors are used to treat several conditions such as myocardial infarction, tissue injury, ischemia, and autoimmune encephalomyelitis. Engineered MSCs were also used to improve bone healing and reduce the side effects of organ transplantation. Part of this figure was created using images from Servier Medical Art Commons Attribution 3.0 Unported License. (http://smart.servier.com). Servier Medical Art by Servier is licensed under a Creative Commons Attribution 3.0 Unported License.
Engineered MSCs to Prevent Rejection in Organ Transplantation
A study using a mouse model of allogeneic liver transplantation used engineered MSC expressing transcription factor fork head box P3 (Foxp3) to increase the median survival time of the animals receiving organ transplantation. Foxp3 caused an increase in Treg level, which has a strong immunomodulatory effect. The increase was T cell contact dependent and was associated with the upregulation of programmed death ligand 1 (PD-L1) expression in MSC (Qi et al., 2015). Another study used interleukin-10 secreting MSCs (MSC-IL10) to prevent rejection in an allogeneic liver transplantation model. It showed an increase in median survival time of transplanted animals compared to those injected with non-engineered MSCs, though non-engineered MSCs also demonstrated increased median survival time compared to control (non-MSC-injected animals) (Niu et al., 2014). This might be due to the native immunomodulation effect of MSC (Andreeva et al., 2017). Secretion of IL10 might increase the levels of Treg-associated anti-inflammatory cytokines (IL-10 and TGF-β1) and decrease Th17-associated pro-inflammatory cytokines (IL-17, IL-6, IFN-γ, TNF-α, and IL-23) (Niu et al., 2014).
Engineered MSCs for the Treatment of Autoimmune Encephalomyelitis
Engineered MSCs have been used in a study using a mouse model of experimental autoimmune encephalomyelitis (EAE). Fransson et al. (2014) examined the use of genetically engineered MSCs expressing a myelin oligodendrocyte glycoprotein (MOG) specific receptor (MSC-CARα-MOG). Anti-rat MOG antibodies cloned from a single-chain variable fragment (scFv) were introduced into a chimeric antigen receptor (CAR), producing a CARαMOG construct. This was then inserted into a lentiviral vector. They discovered that the engineered MSCs exerted beneficial effects in controlling the disease and showed that the intranasal MSC application route was better than intraperitoneal injection. Intranasal route of MSCs treatment caused symptom free at days 27–30. Non-engineered MSCs also caused reduced experimental autoimmune encephalomyelitis as indicated by a better EAE score compared to control when given via an intranasal route, but the improvement was not as good as those treated with MSC-CARα-MOG. When given via an intraperitoneal route, non-engineered MSCs showed a similar EAE score to that of control. The beneficial effect of MSC-CARαMOG might be due to the modulation of the inflammatory cytokines released by T-lymphocytes in the brain. In EAE, activated T-cells migrate across the blood–brain barrier to the brain parenchyma, resulting in brain inflammation, myelin loss, and axon damage. T-cells recovered from the brain of EAE mice produced a lower level of interferon-ɤand higher level of interleukin-17 when treated with MSC-CARαMOG compared to those treated with control MSC. Interestingly, T-cells isolated from the spleen did not show this phenotype, indicating a tissue-specific effect (Fransson et al., 2014).
Engineered MSCs for Treating Tissue Injuries and Ischemia
Wang and co-workers generated engineered MSCs that secrete nerve growth factor (NGF) and used them to treat nerve injury in a rabbit model. They showed that treatment with these cells (MSC-NGF) increased the formation of nerve fibers and the density of myelinated nerve fibers. It also decreased the deposition of myelin debris compared to control animals. The study demonstrated that the MSC-NGF cells enhanced the nerve regenerative capacity (Wang et al., 2015b).
Another study used stromal cell-derived factor-1 (SDF-1) secreting MSCs (MSC-SDF-1) to treat full thickness wound in a rat model. Wound closure and blood vessel formation were significantly improved in the MSC-SDF-1-treated group. Animals treated with non-engineered MSCs have also shown improvement compared to the control group, although not as significant as in the MSC-SDF-1-treated group. SDF-1 increased vascular endothelial growth factor (VEGF) expression, which induced angiogenesis. Furthermore, there was an increase in dermal fibroblast migration in treated animals (Nakamura et al., 2013).
The study by Beegle et al. (2016) used engineered MSCs expressing VEGF in a mouse model of critical limb ischemia. The results showed that mice treated with these MSCs displayed better blood flow at week 10 after treatment compared to the control group. This finding is expected as VEGF is known as a strong angiogenesis factor. It is important to note that allogeneic MSCs for critical limb ischemia have been approved for clinical use in India (Robb et al., 2019).
Using a mouse model with acute kidney injury, a study used lipocalin-2-secreting MSCs (MSC-Lcn2). The results showed that the number of cast and tubular necrosis was significantly decreased compared to mice treated with non-engineered MSCs and with the control groups. At day 21 following treatment, the serum creatinine level in the MSC-Lcn2-treated group decreased significantly to a level comparable to those of normal mice. The blood urea nitrogen level was also decreased, although the level was still higher than normal. Lipocalin-2 has been shown to upregulate the expression of various growth factors, such as hepatocyte growth factor (HGF), insulin-like growth factor, fibroblast growth factor, and VEGF. All of them have the capability to trigger kidney regeneration. This was indicated by the increase in the expression of kidney regenerative markers (i.e., the proximal tubular epithelium markers such as AQP-1 and CK18) and the decreased expression of kidney injury markers such as KIM-1 and cystatin C (Roudkenar et al., 2018).
Engineered MSCs for the Treatment of Myocardial Infarction
Our systematic literature search has indicated four studies on the use of engineered MSCs to treat myocardial infarction. One study was conducted using a mouse model, and the others used a rat model. The first study used VEGF-secreting MSCs and showed that treatment with these cells improved heart function compared to the control group. It was reported that the improvement was likely due to the increased in capillary density because VEGF is known as a potent angiogenic factor (Zhu et al., 2012).
Two studies used engineered MSCs that produce and secrete CXCR4 (MSC-CXCR4) (Huang et al., 2010; Zhang et al., 2010). In a study reported by Zhang et al. (2010) MSC-CXCR4 cells were implanted as cell sheets, and Huang et al. (2010) grew MSC-CXCR4 on the animals’ peritoneum and then applied them onto the scarred myocardium. Both approaches resulted in the improvement of heart function compared to the control group. CXCR4 increased cell engraftment and angiogenesis as well as reduced myocardial remodeling, hence improving heart function (Huang et al., 2010; Zhang et al., 2010). MSC-CXCR4 was also used in combination with diprotin A, a molecule that can inhibit the enzymatic degradation of the SDF-1α-CXCR4 complex by the enzyme CD26/dipeptidyl peptidase IV (DPP-IV). This would result in the prolongation of the CXCR4 activity. Treatment with the combination of MSC-CXCR4 and diprotin A produced better therapeutic effects compared to treatment with MSC-CXCR4 alone (Zhang et al., 2010).
The fourth study in the field of myocardial infarction indicated by our literature search involved the use of engineered MSCs overexpressing Bcl2 (MSC-Bcl2). Bcl2 is known as a potent anti-apoptotic agent. In this study, Li and co-authors demonstrated that treatment with MSC-Bcl2 resulted in the improvement of heart function following myocardial infarction compared to the control group. Bcl2 was shown to induce cell engraftment and VEGF expression level, which, in turn, increased angiogenesis and eventually improved heart function (Li et al., 2007).
The Use of Engineered MSCs to Induce Bone Healing
We found 13 studies on the use of genetically engineered MSCs for the treatment of various types of bone defects (Chang et al., 2003, 2004; Chang et al., 2010; Tsuchida et al., 2003; Jiang et al., 2009; Lin et al., 2010, 2011, 2012a,b, 2015; Lin et al., 2013; Zhao et al., 2010; Fu et al., 2015). In most studies, bone morphogenetic protein 2 (BMP-2) was overexpressed in the MSCs, either alone (Chang et al., 2003, 2004; Chang et al., 2010; Tsuchida et al., 2003; Jiang et al., 2009; Zhao et al., 2010) or in combination with VEGF (Lin et al., 2010, 2011, 2012a,b, 2015; Fu et al., 2015). Whilst in one study BMP2 overexpression was compared with TGF-β3 overexpression (Lin et al., 2013). All studies reported improvement in bone healing following implantation of engineered MSCs. This is not unsurprising as BMP2 is known as a strong inducer of osteogenesis (Chang et al., 2003, 2004; Chang et al., 2010; Tsuchida et al., 2003; Jiang et al., 2009; Lin et al., 2010, 2011, 2012a,b, 2015; Lin et al., 2013; Zhao et al., 2010; Fu et al., 2015). On the other hand, VEGF helped the process by promoting vascular network formation and hence enhanced the BMP2 osteogenesis effect (Lin et al., 2010, 2011, 2012a,b, 2015; Fu et al., 2015).
A study examined a combination treatment of MSC-BMP2 with the addition of various tissue scaffolds, such as ultrapure alginate, alginate, arginine–glycine–aspartic acid (RGD)–alginate, and collagen-1 (Chang et al., 2010). The authors reported that the best scaffold was the type 1 collagen as it degraded faster and improved bone regeneration. By contrast, RGD alginate was considered inferior because it inhibited initial chondrogenesis (Chang et al., 2010).
Tsuchida et al. (2003) compared the use of syngeneic MSCs with allogeneic MSCs in a rat model of bone defect. They showed that syngeneic MSCs exhibited better therapeutic effects than allogeneic MSCs. However, when the allogeneic MSCs were transfected with BMP2 and then implanted in combination with an immunosuppressant drug FK506, they found improvement of the therapeutic effects of the allogeneic MSCs to the level similar to that of syngeneic MSCs. This effect could be attributable to the BMP2 expression of the engineered allogeneic MSCs.
Several other studies examined the differential effects of the transient and constitutive expression of the BMP2 and VEGF combination in the MSCs. The data indicated that the constitutive expression of the osteogenic factor showed better therapeutic effects (Lin et al., 2011, 2012a).
BMP2-engineered MSCs seemed to produce better therapeutic effects compared to TGF-β3-engineered MSCs in a rabbit model of calvarial bone defect. A study reported that implantation of BMP2-engineered MSCs promoted bone formation and significantly improved bone volume and bone density. The better therapeutic effect of these cells was likely due to the ability of BMP2 to promote osteogenesis and chondrogenesis as well as to stimulate the formation of the dura mater, which is important in calvarial bone healing. The study also compared the use of two types of tissue scaffolds, i.e., poly(L-lactide-co-glycolide) (PLGA) and gelatin. It was shown that the gelatin sponge produced better chondroinductive ability than PLGA and subsequently improved bone healing (Lin et al., 2013).
A recent review from Freitas et al. summarized the diverse approaches in using genetically modified MSCs for the treatment of critical-size, non-union, and delayed bone defects. Typically, the MSCs were engineered with genes responsible for osteogenesis (e.g., TGF-β, BMPs, core binding factor α1/Cbfa1, and Osteorix/Osx), angiogenesis (e.g., VEGF), anti-apoptosis (e.g., human telomerase/hTERT), or non-coding RNA (microRNAs). The MSCs can be implanted in the lesions, or alternatively, the secretomes can be given either locally or systemically. The critical questions in utilizing engineered MSCs are how to ensure the safety of the genetic modification and how to standardize the end products of the therapy (Freitas et al., 2019).
The Use of Engineered MSCs to Improve Lung Fibrosis
A study showed that MSCs overexpressing angiotensin-converting enzyme 2 (ACE 2) produced a better therapeutic effect in a bleomycin-induced lung fibrosis model in mice (Min et al., 2015). Mice treated with the MSCs-ACE2 cells showed a significantly better lung phenotype compared to mice treated with ACE2 only or with non-engineered MSCs (Min et al., 2015; Supplementary Table 2). This finding indicated the synergistic actions of MSCs and ACE2. MSCs are known to have fibrinolytic properties (Srour and Thebaud, 2015), whereas ACE2 has an anti-fibrotic activity via the degradation of angiotensin 2 (Ang 2), which is known as a pro-fibrotic agent (Min et al., 2015).
Engineered MSCs to Alleviate Radiation-Induced Toxicity
Another condition that has been successfully treated by genetically engineered MSC is radiation-induced toxicity. A study using a mouse model receiving total body irradiation showed that genetically modified MSCs overexpressing extracellular superoxide dismutase (ECSOD) could alleviate the toxic effects of the radiation, resulting in an increase in the 35-day survival rate to 52%, which was significantly better compared to control (10%). The mechanism of action was likely due to the improved scavenging process of the superoxide anion by ECSOD, which might prevent hematologic toxicity (Abdel-Mageed et al., 2009).
Similarly, a study using a radiation-induced lung injury model in mice demonstrated that treatment with MSCs expressing soluble transforming growth factor-ß type II receptor (sTGF-ß-R) resulted in significantly higher survival (80%) compared to mice treated with native MSCs (40% survival rate), and none of the control animals (no MSC treatment) survived. It is believed that the fibrinolytic effect of MSC in combination with the anti-inflammatory effect of sTGF-ß-R is responsible for preventing inflammatory cell infiltration, pro-inflammatory cytokine secretion, and collagen overproduction in mice treated with MSC-sTGF-ß-R (Xue et al., 2013).
In keeping with those results, another study using a mouse model of radiation-induced lung injury analyzed the use of MSCs overexpressing CXCR4. The genetically engineered MSCs displayed better performance compared to native MSCs as CXCR4 expression facilitated MSC migration and homing to the injury sites. CXCR4 is a receptor for stem cell stromal cell-derived factor-1 (SDF-1), and the activation of CXCR4 by SDF-1 modulates the signaling pathway important for stem cell migration and homing (Zhang et al., 2014).
Two other studies related to radiation-induced toxicity involved the use of HGF. Wang et al. showed that MSCs expressing HGF showed a significantly better therapeutic capacity in alleviating radiation-induced intestinal injury compared to native MSCs. It is thought that the regenerative and anti-apoptotic effects of HGF might be the responsible factors in enhancing intestinal epithelial cell regeneration (Wang et al., 2015a). MSCs overexpressing HGF were also used in radiation-induced liver damage. These cells have been shown to produce better regenerative effects in the liver (Zhang et al., 2019).
The Use of Genetically Engineered MSC for the Treatment of Huntington’s Disease and Rheumatoid Arthritis
Huntington’s disease is caused by mutation in the huntingtin (HTT) gene resulting in the production of mutant huntingtin (mutHTT) protein. mutHTT may induce the death of the medium spiny neurons in the brain striatum. The mechanism of neuronal cell loss involves the reduction of neurotrophic factors, such as brain-derived neurotrophic factor (BDNF). A study using a mouse model of Huntington’s disease demonstrated that treatment with MSCs overexpressing BDNF and/or NGF produced better therapeutic effects compared with treatment with naive MSCs (Dey et al., 2010).
A study on rat and mouse models of arthritis showed that genetically modified MSCs expressing human soluble tumor necrosis factor receptor type 2 (hsTNFR) could partially prevent arthritis symptoms and produced better therapeutic effects than naive MSCs. However, MSC-based treatment was still less effective than treatment with dexamethasone. In addition to the intrinsic anti-inflammatory properties of the MSCs, the expression of hsTNFR might inhibit the pro-inflammatory effects of TNF-α. hsTNFR could bind and decoy TNFα and thus block the TNF-α-mediated inflammatory effect (Liu et al., 2013).
More recently, MSCs expressing HGF were reported for the treatment of rheumatoid arthritis in mice. The study showed that MSC-HGF could alleviate arthritis symptoms, but at the later phase of the disease, the effect was not better than treatment with naive MSCs. HGF at the early phase of the disease displayed an immunosuppression effect, but at a later phase, HGF induced the activation of fibroblast-like synoviocytes. These cells had the capacity to produce Il-6, which induced inflammation, cell proliferation, and decreased apoptosis (Dong et al., 2020).
Conclusion
Engineered MSCs have been used successfully to treat various diseases and conditions in animal models. However, it is important to note that animal models might not be similar to human diseases/conditions. Therefore, the results should be interpreted cautiously as a translational study in human so far did not show any positive result. Nevertheless, MSC-based therapy is still a promising new therapeutic modality for various conditions, and it is clear that enhancement of its therapeutic effects can be achieved by inducting the expression of beneficial paracrine factors. Further studies are still needed to accelerate the pre-clinical findings into clinical application.
Author Contributions
JP conceived the idea, performed the literature search and data entry on tumor/malignancy treatment, analyzed and compiled the data, and wrote the manuscript. TB performed a review on cancer treatment. WM performed a review on engineered MSCs to prevent rejection in organ transplantation, treatment of autoimmune encephalomyelitis tissue injuries, ischemia, and myocardial infarction. RA performed a review on a metastatic cancer model. RN performed the data entry and a review on engineered MSCs for bone regeneration. ID conceived the main idea and performed a review on clinical trials. DO conceived the idea, compiled the data, and wrote and edited the manuscript. All authors contributed to the article and approved the submitted version.
Funding
This study was supported by the International Research Collaboration (Hibah Kolaborasi Internasional) Universitas Indonesia (NKB-1927/UN2.R3.1/HKP.05.00/2019) to ID and British Heart Foundation Project Grants (PG/17/78/33304 and PG/18/40/33767) to DO.
Conflict of Interest
The authors declare that the research was conducted in the absence of any commercial or financial relationships that could be construed as a potential conflict of interest.
Supplementary Material
The Supplementary Material for this article can be found online at: https://www.frontiersin.org/articles/10.3389/fcell.2020.587776/full#supplementary-material
References
Abdel-Mageed, A. S., Senagore, A. J., Pietryga, D. W., Connors, R. H., Giambernardi, T. A., Hay, R. V., et al. (2009). Intravenous administration of mesenchymal stem cells genetically modified with extracellular superoxide dismutase improves survival in irradiated mice. Blood 113, 1201–1203. doi: 10.1182/blood-2008-07-170936
Abrate, A., Buono, R., Canu, T., Esposito, A., Del Maschio, A., Luciano, R., et al. (2014). Mesenchymal stem cells expressing therapeutic genes induce autochthonous prostate tumour regression. Eur. J. Cancer 50, 2478–2488. doi: 10.1016/j.ejca.2014.06.014
Ahn, J., Lee, H., Seo, K., Kang, S., Ra, J., and Youn, H. (2013). Anti-tumor effect of adipose tissue derived-mesenchymal stem cells expressing interferon-beta and treatment with cisplatin in a xenograft mouse model for canine melanoma. PLoS One 8:e74897. doi: 10.1371/journal.pone.0074897
Akimoto, K., Kimura, K., Nagano, M., Takano, S., To’a Salazar, G., Yamashita, T., et al. (2013). Umbilical cord blood-derived mesenchymal stem cells inhibit, but adipose tissue-derived mesenchymal stem cells promote, glioblastoma multiforme proliferation. Stem Cells Dev. 22, 1370–1386. doi: 10.1089/scd.2012.0486
Altaner, C., Altanerova, V., Cihova, M., Ondicova, K., Rychly, B., Baciak, L., et al. (2014). Complete regression of glioblastoma by mesenchymal stem cells mediated prodrug gene therapy simulating clinical therapeutic scenario. Int. J. Cancer 134, 1458–1465. doi: 10.1002/ijc.28455
Altanerova, V., Cihova, M., Babic, M., Rychly, B., Ondicova, K., Mravec, B., et al. (2012). Human adipose tissue-derived mesenchymal stem cells expressing yeast cytosinedeaminase::uracil phosphoribosyltransferase inhibit intracerebral rat glioblastoma. Int. J. Cancer 130, 2455–2463. doi: 10.1002/ijc.26278
Amara, I., Pramil, E., Senamaud-Beaufort, C., Devillers, A., Macedo, R., Lescaille, G., et al. (2016). Engineered mesenchymal stem cells as vectors in a suicide gene therapy against preclinical murine models for solid tumors. J. Control Release 239, 82–91. doi: 10.1016/j.jconrel.2016.08.019
Andreeva, E., Bobyleva, P., Gornostaeva, A., and Buravkova, L. (2017). Interaction of multipotent mesenchymal stromal and immune cells: bidirectional effects. Cytotherapy 19, 1152–1166. doi: 10.1016/j.jcyt.2017.07.001
Babajani, A., Soltani, P., Jamshidi, E., Farjoo, M. H., and Niknejad, H. (2020). Recent advances on drug-loaded mesenchymal stem cells with anti-neoplastic agents for targeted treatment of cancer. Front. Bioeng. Biotechnol. 8:748. doi: 10.3389/fbioe.2020.00748
Bak, X. Y., Lam, D. H., Yang, J., Ye, K., Wei, E. L., Lim, S. K., et al. (2011). Human embryonic stem cell-derived mesenchymal stem cells as cellular delivery vehicles for prodrug gene therapy of glioblastoma. Hum. Gene Ther. 22, 1365–1377. doi: 10.1089/hum.2010.212
Beane, O. S., Fonseca, V. C., and Darling, E. M. (2014). Adipose-derived stem cells retain their regenerative potential after methotrexate treatment. Exp. Cell Res. 327, 222–233. doi: 10.1016/j.yexcr.2014.06.015
Beegle, J. R., Magner, N. L., Kalomoiris, S., Harding, A., Zhou, P., Nacey, C., et al. (2016). Preclinical evaluation of mesenchymal stem cells overexpressing VEGF to treat critical limb ischemia. Mol. Ther. Methods Clin. Dev. 3:16053. doi: 10.1038/mtm.2016.53
Bellagamba, B. C., Abreu, B. R., Grivicich, I., Markarian, C. F., Chem, E., Camassola, M., et al. (2016). Human mesenchymal stem cells are resistant to cytotoxic and genotoxic effects of cisplatin in vitro. Genet. Mol. Biol. 39, 129–134. doi: 10.1590/1678-4685-gmb-2015-0057
Budiyanti, E., Liem, I., Pawitan, J., Wulandari, D., Jamaan, T., and Sumapradja, K. (2015). Umbilical cord derived mesenchymal stem cell proliferation in various platelet rich plasma and xeno-material containing medium. Int. J. Res. Pharm. Sci. 6, 7–13.
Cavarretta, I. T., Altanerova, V., Matuskova, M., Kucerova, L., Culig, Z., and Altaner, C. (2010). Adipose tissue-derived mesenchymal stem cells expressing prodrug-converting enzyme inhibit human prostate tumor growth. Mol. Ther. 18, 223–231. doi: 10.1038/mt.2009.237
Chang, D. Y., Yoo, S. W., Hong, Y., Kim, S., Kim, S. J., Yoon, S. H., et al. (2010). The growth of brain tumors can be suppressed by multiple transplantation of mesenchymal stem cells expressing cytosine deaminase. Int. J. Cancer 127, 1975–1983. doi: 10.1002/ijc.25383
Chang, S. C., Chung, H. Y., Tai, C. L., Chen, P. K., Lin, T. M., and Jeng, L. B. (2010). Repair of large cranial defects by hBMP-2 expressing bone marrow stromal cells: comparison between alginate and collagen type I systems. J. Biomed. Mater. Res. A 94, 433–441.
Chang, S. C., Chuang, H., Chen, Y. R., Yang, L. C., Chen, J. K., Mardini, S., et al. (2004). Cranial repair using BMP-2 gene engineered bone marrow stromal cells. J. Surg. Res. 119, 85–91. doi: 10.1016/j.jss.2003.08.003
Chang, S. C., Chuang, H. L., Chen, Y. R., Chen, J. K., Chung, H. Y., Lu, Y. L., et al. (2003). Ex vivo gene therapy in autologous bone marrow stromal stem cells for tissue-engineered maxillofacial bone regeneration. Gene Ther. 10, 2013–2019. doi: 10.1038/sj.gt.3302106
Chen, X., Lin, X., Zhao, J., Shi, W., Zhang, H., Wang, Y., et al. (2008). A tumor-selective biotherapy with prolonged impact on established metastases based on cytokine gene-engineered MSCs. Mol. Ther. 16, 749–756. doi: 10.1038/mt.2008.3
Chulpanova, D. S., Kitaeva, K. V., Tazetdinova, L. G., James, V., Rizvanov, A. A., and Solovyeva, V. V. (2018). Application of mesenchymal stem cells for therapeutic agent delivery in anti-tumor treatment. Front. Pharmacol. 9:259. doi: 10.3389/fphar.2018.00259
Chung, T., Na, J., Kim, Y. I., Chang, D. Y., Kim, Y. I., Kim, H., et al. (2016). Dihydropyrimidine dehydrogenase is a prognostic marker for mesenchymal stem cell-mediated cytosine deaminase gene and 5-fluorocytosine prodrug therapy for the treatment of recurrent gliomas. Theranostics 6, 1477–1490. doi: 10.7150/thno.14158
Conrad, C., Husemann, Y., Niess, H., Von Luettichau, I., Huss, R., Bauer, C., et al. (2011). Linking transgene expression of engineered mesenchymal stem cells and angiopoietin-1-induced differentiation to target cancer angiogenesis. Ann. Surg. 253, 566–571. doi: 10.1097/sla.0b013e3181fcb5d8
De Boeck, A., Pauwels, P., Hensen, K., Rummens, J. L., Westbroek, W., Hendrix, A., et al. (2013). Bone marrow-derived mesenchymal stem cells promote colorectal cancer progression through paracrine neuregulin 1/HER3 signalling. Gut 62, 550–560. doi: 10.1136/gutjnl-2011-301393
Dey, N. D., Bombard, M. C., Roland, B. P., Davidson, S., Lu, M., Rossignol, J., et al. (2010). Genetically engineered mesenchymal stem cells reduce behavioral deficits in the YAC 128 mouse model of Huntington’s disease. Behav. Brain Res. 214, 193–200. doi: 10.1016/j.bbr.2010.05.023
Dong, X., Kong, F., Liu, C., Dai, S., Zhang, Y., Xiao, F., et al. (2020). Pulp stem cells with hepatocyte growth factor overexpression exhibit dual effects in rheumatoid arthritis. Stem Cell Res. Ther. 11:229.
Fei, S., Qi, X., Kedong, S., Guangchun, J., Jian, L., and Wei, Q. (2012). The antitumor effect of mesenchymal stem cells transduced with a lentiviral vector expressing cytosine deaminase in a rat glioma model. J. Cancer Res. Clin. Oncol. 138, 347–357. doi: 10.1007/s00432-011-1104-z
Frank, R. T., Najbauer, J., and Aboody, K. S. (2010). Concise review: stem cells as an emerging platform for antibody therapy of cancer. Stem Cells 28, 2084–2087. doi: 10.1002/stem.513
Fransson, M., Piras, E., Wang, H., Burman, J., Duprez, I., Harris, R. A., et al. (2014). Intranasal delivery of central nervous system-retargeted human mesenchymal stromal cells prolongs treatment efficacy of experimental autoimmune encephalomyelitis. Immunology 142, 431–441. doi: 10.1111/imm.12275
Freitag, J., Bates, D., Wickham, J., Shah, K., Huguenin, L., Tenen, A., et al. (2019). Adipose-derived mesenchymal stem cell therapy in the treatment of knee osteoarthritis: a randomized controlled trial. Regen. Med. 14, 213–230. doi: 10.2217/rme-2018-0161
Freitas, J., Santos, S. G., Goncalves, R. M., Teixeira, J. H., Barbosa, M. A., and Almeida, M. I. (2019). Genetically engineered-MSC therapies for non-unions, delayed unions and critical-size bone defects. Int. J. Mol. Sci. 20:3430. doi: 10.3390/ijms20143430
Fritz, V., Noel, D., Bouquet, C., Opolon, P., Voide, R., Apparailly, F., et al. (2008). Antitumoral activity and osteogenic potential of mesenchymal stem cells expressing the urokinase-type plasminogen antagonist amino-terminal fragment in a murine model of osteolytic tumor. Stem Cells 26, 2981–2990. doi: 10.1634/stemcells.2008-0139
Fu, T. S., Chang, Y. H., Wong, C. B., Wang, I. C., Tsai, T. T., Lai, P. L., et al. (2015). Mesenchymal stem cells expressing baculovirus-engineered BMP-2 and VEGF enhance posterolateral spine fusion in a rabbit model. Spine J. 15, 2036–2044. doi: 10.1016/j.spinee.2014.11.002
Gao, P., Ding, Q., Wu, Z., Jiang, H., and Fang, Z. (2010). Therapeutic potential of human mesenchymal stem cells producing IL-12 in a mouse xenograft model of renal cell carcinoma. Cancer Lett. 290, 157–166. doi: 10.1016/j.canlet.2009.08.031
Grisendi, G., Spano, C., D’souza, N., Rasini, V., Veronesi, E., Prapa, M., et al. (2015). Mesenchymal progenitors expressing TRAIL induce apoptosis in sarcomas. Stem Cells 33, 859–869. doi: 10.1002/stem.1903
Harati, M. D., Amiri, F., Jaleh, F., Mehdipour, A., Harati, M. D., Molaee, S., et al. (2015). Targeting delivery of lipocalin 2-engineered mesenchymal stem cells to colon cancer in order to inhibit liver metastasis in nude mice. Tumour. Biol. 36, 6011–6018. doi: 10.1007/s13277-015-3277-6
Hu, Y. L., Miao, P. H., Huang, B., Zhang, T. Y., Hu, Z. J., Tabata, Y., et al. (2014). Reversal of tumor growth by gene modification of mesenchymal stem cells using spermine-pullulan/DNA nanoparticles. J. Biomed. Nanotechnol. 10, 299–308. doi: 10.1166/jbn.2014.1712
Huang, W., Zhang, D., Millard, R. W., Wang, T., Zhao, T., Fan, G. C., et al. (2010). Gene manipulated peritoneal cell patch repairs infarcted myocardium. J. Mol. Cell Cardiol. 48, 702–712. doi: 10.1016/j.yjmcc.2009.10.032
Jiang, X., Zhao, J., Wang, S., Sun, X., Zhang, X., Chen, J., et al. (2009). Mandibular repair in rats with premineralized silk scaffolds and BMP-2-modified bMSCs. Biomaterials 30, 4522–4532. doi: 10.1016/j.biomaterials.2009.05.021
Jung, Y., Kim, J. K., Shiozawa, Y., Wang, J., Mishra, A., Joseph, J., et al. (2013). Recruitment of mesenchymal stem cells into prostate tumours promotes metastasis. Nat. Commun. 4:1795.
Karnoub, A. E., Dash, A. B., Vo, A. P., Sullivan, A., Brooks, M. W., Bell, G. W., et al. (2007). Mesenchymal stem cells within tumour stroma promote breast cancer metastasis. Nature 449, 557–563. doi: 10.1038/nature06188
Kemp, K., Morse, R., Sanders, K., Hows, J., and Donaldson, C. (2011). Alkylating chemotherapeutic agents cyclophosphamide and melphalan cause functional injury to human bone marrow-derived mesenchymal stem cells. Ann. Hematol. 90, 777–789. doi: 10.1007/s00277-010-1141-8
Kim, S. W., Kim, S. J., Park, S. H., Yang, H. G., Kang, M. C., Choi, Y. W., et al. (2013). Complete regression of metastatic renal cell carcinoma by multiple injections of engineered mesenchymal stem cells expressing dodecameric TRAIL and HSV-TK. Clin. Cancer Res. 19, 415–427. doi: 10.1158/1078-0432.ccr-12-1568
Kosaka, H., Ichikawa, T., Kurozumi, K., Kambara, H., Inoue, S., Maruo, T., et al. (2012). Therapeutic effect of suicide gene-transferred mesenchymal stem cells in a rat model of glioma. Cancer Gene Ther. 19, 572–578. doi: 10.1038/cgt.2012.35
Krasikova, L. S., Karshieva, S. S., Cheglakov, I. B., and Belyavsky, A. V. (2015). [Mesenchymal stem cells expressing cytosine deaminase inhibit growth of murine melanoma B16F10 in vivo]. Mol. Bio. 49, 1007–1015.
Krassikova, L. S., Karshieva, S. S., Cheglakov, I. B., and Belyavsky, A. V. (2016). Combined treatment, based on lysomustine administration with mesenchymal stem cells expressing cytosine deaminase therapy, leads to pronounced murine Lewis lung carcinoma growth inhibition. J. Gene Med. 18, 220–233. doi: 10.1002/jgm.2894
Kucerova, L., Altanerova, V., Matuskova, M., Tyciakova, S., and Altaner, C. (2007). Adipose tissue-derived human mesenchymal stem cells mediated prodrug cancer gene therapy. Cancer Res. 67, 6304–6313. doi: 10.1158/0008-5472.can-06-4024
Kucerova, L., Matuskova, M., Pastorakova, A., Tyciakova, S., Jakubikova, J., Bohovic, R., et al. (2008). Cytosine deaminase expressing human mesenchymal stem cells mediated tumour regression in melanoma bearing mice. J. Gene Med. 10, 1071–1082. doi: 10.1002/jgm.1239
Kucerova, L., Skolekova, S., Demkova, L., Bohovic, R., and Matuskova, M. (2014). Long-term efficiency of mesenchymal stromal cell-mediated CD-MSC/5FC therapy in human melanoma xenograft model. Gene Ther. 21, 874–887. doi: 10.1038/gt.2014.66
Kumar, S., and Ponnazhagan, S. (2007). Bone homing of mesenchymal stem cells by ectopic alpha 4 integrin expression. FASEB J. 21, 3917–3927. doi: 10.1096/fj.07-8275com
Lakota, J., Gocarova, K., and Spanik, S. (2015). Treatment of metastatic head and neck cancer with mesenchymal stem cells combined with prodrug gene therapy. Exp. Oncol. 37:298. doi: 10.31768/2312-8852.2015.37(4):298
Lee, J. W., Fang, X., Krasnodembskaya, A., Howard, J. P., and Matthay, M. A. (2011). Concise review: mesenchymal stem cells for acute lung injury: role of paracrine soluble factors. Stem Cells 29, 913–919. doi: 10.1002/stem.643
Lee, M. W., Ryu, S., Kim, D. S., Lee, J. W., Sung, K. W., Koo, H. H., et al. (2019). Mesenchymal stem cells in suppression or progression of hematologic malignancy: current status and challenges. Leukemia 33, 597–611. doi: 10.1038/s41375-018-0373-9
Lee, W. Y., Zhang, T., Lau, C. P., Wang, C. C., Chan, K. M., and Li, G. (2013). Immortalized human fetal bone marrow-derived mesenchymal stromal cell expressing suicide gene for anti-tumor therapy in vitro and in vivo. Cytotherapy 15, 1484–1497. doi: 10.1016/j.jcyt.2013.06.010
Li, W., Ma, N., Ong, L. L., Nesselmann, C., Klopsch, C., Ladilov, Y., et al. (2007). Bcl-2 engineered MSCs inhibited apoptosis and improved heart function. Stem Cells 25, 2118–2127. doi: 10.1634/stemcells.2006-0771
Lin, C. Y., Chang, Y. H., Kao, C. Y., Lu, C. H., Sung, L. Y., Yen, T. C., et al. (2012a). Augmented healing of critical-size calvarial defects by baculovirus-engineered MSCs that persistently express growth factors. Biomaterials 33, 3682–3692. doi: 10.1016/j.biomaterials.2012.02.007
Lin, C. Y., Lin, K. J., Li, K. C., Sung, L. Y., Hsueh, S., Lu, C. H., et al. (2012b). Immune responses during healing of massive segmental femoral bone defects mediated by hybrid baculovirus-engineered ASCs. Biomaterials 33, 7422–7434. doi: 10.1016/j.biomaterials.2012.06.083
Lin, C. Y., Chang, Y. H., Li, K. C., Lu, C. H., Sung, L. Y., Yeh, C. L., et al. (2013). The use of ASCs engineered to express BMP2 or TGF-beta3 within scaffold constructs to promote calvarial bone repair. Biomaterials 34, 9401–9412. doi: 10.1016/j.biomaterials.2013.08.051
Lin, J. T., Wang, J. Y., Chen, M. K., Chen, H. C., Chang, T. H., Su, B. W., et al. (2013). Colon cancer mesenchymal stem cells modulate the tumorigenicity of colon cancer through interleukin 6. Exp. Cell Res. 319, 2216–2229. doi: 10.1016/j.yexcr.2013.06.003
Lin, C. Y., Chang, Y. H., Lin, K. J., Yen, T. C., Tai, C. L., Chen, C. Y., et al. (2010). The healing of critical-sized femoral segmental bone defects in rabbits using baculovirus-engineered mesenchymal stem cells. Biomaterials 31, 3222–3230. doi: 10.1016/j.biomaterials.2010.01.030
Lin, C. Y., Lin, K. J., Kao, C. Y., Chen, M. C., Lo, W. H., Yen, T. C., et al. (2011). The role of adipose-derived stem cells engineered with the persistently expressing hybrid baculovirus in the healing of massive bone defects. Biomaterials 32, 6505–6514. doi: 10.1016/j.biomaterials.2011.05.059
Lin, C. Y., Wang, Y. H., Li, K. C., Sung, L. Y., Yeh, C. L., Lin, K. J., et al. (2015). Healing of massive segmental femoral bone defects in minipigs by allogenic ASCs engineered with FLPo/Frt-based baculovirus vectors. Biomaterials 50, 98–106. doi: 10.1016/j.biomaterials.2015.01.052
Lin, W., Huang, L., Li, Y., Fang, B., Li, G., Chen, L., et al. (2019). Mesenchymal stem cells and cancer: clinical challenges and opportunities. Biomed. Res. Int. 2019:2820853.
Liu, L. N., Wang, G., Hendricks, K., Lee, K., Bohnlein, E., Junker, U., et al. (2013). Comparison of drug and cell-based delivery: engineered adult mesenchymal stem cells expressing soluble tumor necrosis factor receptor II prevent arthritis in mouse and rat animal models. Stem Cells Transl. Med. 2, 362–375. doi: 10.5966/sctm.2012-0135
Luetzkendorf, J., Mueller, L. P., Mueller, T., Caysa, H., Nerger, K., and Schmoll, H. J. (2010). Growth inhibition of colorectal carcinoma by lentiviral TRAIL-transgenic human mesenchymal stem cells requires their substantial intratumoral presence. J. Cell Mol. Med. 14, 2292–2304. doi: 10.1111/j.1582-4934.2009.00794.x
Mancheno-Corvo, P., Franquesa, M., De La Rosa, O., Ramirez, C., Garcia-Benzaquen, L., Fernandez, V., et al. (2013). Adipose mesenchymal stromal cell function is not affected by methotrexate and azathioprine. Biores Open Access 2, 431–439. doi: 10.1089/biores.2013.0040
Martinez-Quintanilla, J., Bhere, D., Heidari, P., He, D., Mahmood, U., and Shah, K. (2013). Therapeutic efficacy and fate of bimodal engineered stem cells in malignant brain tumors. Stem Cells 31, 1706–1714. doi: 10.1002/stem.1355
Matuskova, M., Kozovska, Z., Toro, L., Durinikova, E., Tyciakova, S., Cierna, Z., et al. (2015). Combined enzyme/prodrug treatment by genetically engineered AT-MSC exerts synergy and inhibits growth of MDA-MB-231 induced lung metastases. J. Exp. Clin. Cancer Res. 34:33.
Min, F., Gao, F., Li, Q., and Liu, Z. (2015). Therapeutic effect of human umbilical cord mesenchymal stem cells modified by angiotensin-converting enzyme 2 gene on bleomycin-induced lung fibrosis injury. Mol. Med. Rep. 11, 2387–2396. doi: 10.3892/mmr.2014.3025
Mirotsou, M., Jayawardena, T. M., Schmeckpeper, J., Gnecchi, M., and Dzau, V. J. (2011). Paracrine mechanisms of stem cell reparative and regenerative actions in the heart. J. Mol. Cell Cardiol. 50, 280–289. doi: 10.1016/j.yjmcc.2010.08.005
Mirzaei, H., Salehi, H., Oskuee, R. K., Mohammadpour, A., Mirzaei, H. R., Sharifi, M. R., et al. (2018). The therapeutic potential of human adipose-derived mesenchymal stem cells producing CXCL10 in a mouse melanoma lung metastasis model. Cancer Lett. 419, 30–39. doi: 10.1016/j.canlet.2018.01.029
Mohamadnejad, M., Alimoghaddam, K., Bagheri, M., Ashrafi, M., Abdollahzadeh, L., Akhlaghpoor, S., et al. (2013). Randomized placebo-controlled trial of mesenchymal stem cell transplantation in decompensated cirrhosis. Liver Int. 33, 1490–1496. doi: 10.1111/liv.12228
Nakamura, Y., Ishikawa, H., Kawai, K., Tabata, Y., and Suzuki, S. (2013). Enhanced wound healing by topical administration of mesenchymal stem cells transfected with stromal cell-derived factor-1. Biomaterials 34, 9393–9400. doi: 10.1016/j.biomaterials.2013.08.053
NguyenThai, Q. A., Sharma, N., Luong Do, H., Sodhi, S. S., Kim, J. H., Kim, N., et al. (2015). Targeted inhibition of osteosarcoma tumor growth by bone marrow-derived mesenchymal stem cells expressing cytosine deaminase/5-fluorocytosine in tumor-bearing mice. J. Gene Med. 17, 87–99. doi: 10.1002/jgm.2826
Nicolay, N. H., Lopez Perez, R., Ruhle, A., Trinh, T., Sisombath, S., Weber, K. J., et al. (2016). Mesenchymal stem cells maintain their defining stem cell characteristics after treatment with cisplatin. Sci. Rep. 6:20035.
Nifontova, I., Svinareva, D., Petrova, T., and Drize, N. (2008). Sensitivity of mesenchymal stem cells and their progeny to medicines used for the treatment of hematoproliferative diseases. Acta Haematol. 119, 98–103. doi: 10.1159/000120440
Niu, J., Yue, W., Song, Y., Zhang, Y., Qi, X., Wang, Z., et al. (2014). Prevention of acute liver allograft rejection by IL-10-engineered mesenchymal stem cells. Clin. Exp. Immunol. 176, 473–484. doi: 10.1111/cei.12283
Nouri, F. S., Wang, X., and Hatefi, A. (2015). Genetically engineered theranostic mesenchymal stem cells for the evaluation of the anticancer efficacy of enzyme/prodrug systems. J. Control Release 200, 179–187. doi: 10.1016/j.jconrel.2015.01.003
Pawitan, J., Feroniasanti, L., Kispa, T., Dilogo, I., Fasha, I., Kurniawati, T., et al. (2014). Simple method to isolate mesenchymal stem cells from bone marrow using xeno-free material: a preliminary study. Int. J. Pharm. Tech. Res. 7, 354–359.
Pawitan, J., Liem, I., Suryani, D., Bustami, A., and Purwoko, R. (2013). Simple lipoaspirate washing using a coffee filter. Asian Biomed. 7, 333–338.
Pawitan, J. A., Yang, Z., Wu, Y. N., and Lee, E. H. (2018). Towards standardized stem cell therapy in type 2 diabetes mellitus: a systematic review. Curr. Stem Cell Res. Ther. 13, 476–488. doi: 10.2174/1574888x13666180502143657
Pessina, A., Bonomi, A., Cocce, V., Invernici, G., Navone, S., Cavicchini, L., et al. (2011). Mesenchymal stromal cells primed with paclitaxel provide a new approach for cancer therapy. PLoS One 6:e28321. doi: 10.1371/journal.pone.0028321
Qi, H., Chen, G., Huang, Y., Si, Z., and Li, J. (2015). Foxp3-modified bone marrow mesenchymal stem cells promotes liver allograft tolerance through the generation of regulatory T cells in rats. J. Transl. Med. 13:274.
Qi, Z., Zhang, Y., Liu, L., Guo, X., Qin, J., and Cui, G. (2012). Mesenchymal stem cells derived from different origins have unique sensitivities to different chemotherapeutic agents. Cell Biol. Int. 36, 857–862. doi: 10.1042/cbi20110637
Ren, C., Kumar, S., Chanda, D., Kallman, L., Chen, J., Mountz, J. D., et al. (2008). Cancer gene therapy using mesenchymal stem cells expressing interferon-beta in a mouse prostate cancer lung metastasis model. Gene Ther. 15, 1446–1453. doi: 10.1038/gt.2008.101
Robb, K. P., Fitzgerald, J. C., Barry, F., and Viswanathan, S. (2019). Mesenchymal stromal cell therapy: progress in manufacturing and assessments of potency. Cytotherapy 21, 289–306. doi: 10.1016/j.jcyt.2018.10.014
Roudkenar, M. H., Halabian, R., Tehrani, H. A., Amiri, F., Jahanian-Najafabadi, A., Roushandeh, A. M., et al. (2018). Lipocalin 2 enhances mesenchymal stem cell-based cell therapy in acute kidney injury rat model. Cytotechnology 70, 103–117. doi: 10.1007/s10616-017-0107-2
Ruhle, A., Huber, P. E., Saffrich, R., Lopez Perez, R., and Nicolay, N. H. (2018). The current understanding of mesenchymal stem cells as potential attenuators of chemotherapy-induced toxicity. Int. J. Cancer 143, 2628–2639. doi: 10.1002/ijc.31619
Ruhle, A., Lopez Perez, R., Zou, B., Grosu, A. L., Huber, P. E., and Nicolay, N. H. (2019). The therapeutic potential of mesenchymal stromal cells in the treatment of chemotherapy-induced tissue damage. Stem Cell Rev. Rep. 15, 356–373. doi: 10.1007/s12015-019-09886-3
Sasportas, L. S., Kasmieh, R., Wakimoto, H., Hingtgen, S., Van De Water, J. A., Mohapatra, G., et al. (2009). Assessment of therapeutic efficacy and fate of engineered human mesenchymal stem cells for cancer therapy. Proc. Natl. Acad. Sci. U.S.A. 106, 4822–4827. doi: 10.1073/pnas.0806647106
Schug, C., Sievert, W., Urnauer, S., Muller, A. M., Schmohl, K. A., Wechselberger, A., et al. (2018). External beam radiation therapy enhances mesenchymal stem cell-mediated sodium-iodide symporter gene delivery. Hum. Gene Ther. 29, 1287–1300. doi: 10.1089/hum.2018.025
Segaliny, A. I., Cheng, J. L., Farhoodi, H. P., Toledano, M., Yu, C. C., Tierra, B., et al. (2019). Combinatorial targeting of cancer bone metastasis using mRNA engineered stem cells. EBioMedicine 45, 39–57. doi: 10.1016/j.ebiom.2019.06.047
Seo, K. W., Lee, H. W., Oh, Y. I., Ahn, J. O., Koh, Y. R., Oh, S. H., et al. (2011). Anti-tumor effects of canine adipose tissue-derived mesenchymal stromal cell-based interferon-beta gene therapy and cisplatin in a mouse melanoma model. Cytotherapy 13, 944–955. doi: 10.3109/14653249.2011.584864
Spaeth, E. L., Dembinski, J. L., Sasser, A. K., Watson, K., Klopp, A., Hall, B., et al. (2009). Mesenchymal stem cell transition to tumor-associated fibroblasts contributes to fibrovascular network expansion and tumor progression. PLoS One 4:e4992. doi: 10.1371/journal.pone.0004992
Srour, N., and Thebaud, B. (2015). Mesenchymal stromal cells in animal bleomycin pulmonary fibrosis models: a systematic review. Stem Cells Transl. Med. 4, 1500–1510. doi: 10.5966/sctm.2015-0121
Suryaprakash, S., Lao, Y. H., Cho, H. Y., Li, M., Ji, H. Y., Shao, D., et al. (2019). Engineered mesenchymal stem Cell/Nanomedicine spheroid as an active drug delivery platform for combinational glioblastoma therapy. Nano Lett. 19, 1701–1705. doi: 10.1021/acs.nanolett.8b04697
Toro, L., Bohovic, R., Matuskova, M., Smolkova, B., and Kucerova, L. (2016). Metastatic ovarian cancer can be efficiently treated by genetically modified mesenchymal stromal cells. Stem Cells Dev. 25, 1640–1651. doi: 10.1089/scd.2016.0064
Tsuchida, H., Hashimoto, J., Crawford, E., Manske, P., and Lou, J. (2003). Engineered allogeneic mesenchymal stem cells repair femoral segmental defect in rats. J. Orthop. Res. 21, 44–53. doi: 10.1016/s0736-0266(02)00108-0
Tyciakova, S., Matuskova, M., Bohovic, R., Polakova, K., Toro, L., Skolekova, S., et al. (2015). Genetically engineered mesenchymal stromal cells producing TNFalpha have tumour suppressing effect on human melanoma xenograft. J. Gene Med. 17, 54–67. doi: 10.1002/jgm.2823
Wang, G. X., Zhan, Y. A., Hu, H. L., Wang, Y., and Fu, B. (2012). Mesenchymal stem cells modified to express interferon-beta inhibit the growth of prostate cancer in a mouse model. J. Int. Med. Res. 40, 317–327. doi: 10.1177/147323001204000132
Wang, H., Sun, R. T., Li, Y., Yang, Y. F., Xiao, F. J., Zhang, Y. K., et al. (2015a). HGF gene modification in mesenchymal stem cells reduces radiation-induced intestinal injury by modulating immunity. PLoS One 10:e0124420. doi: 10.1371/journal.pone.0124420
Wang, L., Zhao, Y., Cao, J., Yang, X., and Lei, D. (2015b). Mesenchymal stem cells modified with nerve growth factor improve recovery of the inferior alveolar nerve after mandibular distraction osteogenesis in rabbits. Br. J. Oral. Maxillofac Surg. 53, 279–284. doi: 10.1016/j.bjoms.2014.12.014
Xin, H., Sun, R., Kanehira, M., Takahata, T., Itoh, J., Mizuguchi, H., et al. (2009). Intratracheal delivery of CX3CL1-expressing mesenchymal stem cells to multiple lung tumors. Mol. Med. 15, 321–327. doi: 10.2119/molmed.2009.00059
Xue, J., Li, X., Lu, Y., Gan, L., Zhou, L., Wang, Y., et al. (2013). Gene-modified mesenchymal stem cells protect against radiation-induced lung injury. Mol. Ther. 21, 456–465. doi: 10.1038/mt.2012.183
Yan, C., Li, S., Li, Z., Peng, H., Yuan, X., Jiang, L., et al. (2013). Human umbilical cord mesenchymal stem cells as vehicles of CD20-specific TRAIL fusion protein delivery: a double-target therapy against non-Hodgkin’s lymphoma. Mol. Pharm. 10, 142–151. doi: 10.1021/mp300261e
Yan, F., Li, X., Li, N., Zhang, R., Wang, Q., Ru, Y., et al. (2017). Immunoproapoptotic molecule scFv-Fdt-tBid modified mesenchymal stem cells for prostate cancer dual-targeted therapy. Cancer Lett. 402, 32–42. doi: 10.1016/j.canlet.2017.05.003
Yao, S., Li, X., Liu, J., Sun, Y., Wang, Z., and Jiang, Y. (2017). Maximized nanodrug-loaded mesenchymal stem cells by a dual drug-loaded mode for the systemic treatment of metastatic lung cancer. Drug. Deliv. 24, 1372–1383. doi: 10.1080/10717544.2017.1375580
Ye, H., Cheng, J., Tang, Y., Liu, Z., Xu, C., Liu, Y., et al. (2012). Human bone marrow-derived mesenchymal stem cells produced TGFbeta contributes to progression and metastasis of prostate cancer. Cancer Invest. 30, 513–518. doi: 10.3109/07357907.2012.692171
Yong, R. L., Shinojima, N., Fueyo, J., Gumin, J., Vecil, G. G., Marini, F. C., et al. (2009). Human bone marrow-derived mesenchymal stem cells for intravascular delivery of oncolytic adenovirus Delta24-RGD to human gliomas. Cancer Res. 69, 8932–8940. doi: 10.1158/0008-5472.can-08-3873
You, M. H., Kim, W. J., Shim, W., Lee, S. R., Lee, G., Choi, S., et al. (2009). Cytosine deaminase-producing human mesenchymal stem cells mediate an antitumor effect in a mouse xenograft model. J. Gastroenterol. Hepatol. 24, 1393–1400. doi: 10.1111/j.1440-1746.2009.05862.x
Zhang, C., Zhu, Y., Wang, J., Hou, L., Li, W., and An, H. (2019). CXCR4-overexpressing umbilical cord mesenchymal stem cells enhance protection against radiation-induced lung injury. Stem Cells Int. 2019:2457082.
Zhang, C. L., Huang, T., Wu, B. L., He, W. X., and Liu, D. (2017). Stem cells in cancer therapy: opportunities and challenges. Oncotarget 8, 75756–75766. doi: 10.18632/oncotarget.20798
Zhang, D., Huang, W., Dai, B., Zhao, T., Ashraf, A., Millard, R. W., et al. (2010). Genetically manipulated progenitor cell sheet with diprotin A improves myocardial function and repair of infarcted hearts. Am. J. Physiol. Heart Circ. Physiol. 299, H1339–H1347.
Zhang, J., Zhou, S., Zhou, Y., Feng, F., Wang, Q., Zhu, X., et al. (2014). Hepatocyte growth factor gene-modified adipose-derived mesenchymal stem cells ameliorate radiation induced liver damage in a rat model. PLoS One 9:e114670. doi: 10.1371/journal.pone.0114670
Zhang, X., Xu, W., Qian, H., Zhu, W., and Zhang, R. (2011). Mesenchymal stem cells modified to express lentivirus TNF-alpha Tumstatin(45-132) inhibit the growth of prostate cancer. J. Cell Mol. Med. 15, 433–444. doi: 10.1111/j.1582-4934.2009.00920.x
Zhao, J., Hu, J., Wang, S., Sun, X., Xia, L., Zhang, X., et al. (2010). Combination of beta-TCP and BMP-2 gene-modified bMSCs to heal critical size mandibular defects in rats. Oral. Dis. 16, 46–54. doi: 10.1111/j.1601-0825.2009.01602.x
Zheng, L., Zhang, D., Chen, X., Yang, L., Wei, Y., and Zhao, X. (2012). Antitumor activities of human placenta-derived mesenchymal stem cells expressing endostatin on ovarian cancer. PLoS One 7:e39119. doi: 10.1371/journal.pone.0039119
Zhu, K., Lai, H., Guo, C., Xu, D., and Wang, C. (2012). Novel vascular endothelial growth factor gene delivery system-manipulated mesenchymal stem cells repair infarcted myocardium. Exp. Biol. Med. 237, 678–687. doi: 10.1258/ebm.2012.011430
Zolochevska, O., Yu, G., Gimble, J. M., and Figueiredo, M. L. (2012). Pigment epithelial-derived factor and melanoma differentiation associated gene-7 cytokine gene therapies delivered by adipose-derived stromal/mesenchymal stem cells are effective in reducing prostate cancer cell growth. Stem Cells Dev. 21, 1112–1123. doi: 10.1089/scd.2011.0247
Keywords: engineered MSCs, tumor, cancer, metastasis, bone defect, animal models
Citation: Pawitan JA, Bui TA, Mubarok W, Antarianto RD, Nurhayati RW, Dilogo IH and Oceandy D (2020) Enhancement of the Therapeutic Capacity of Mesenchymal Stem Cells by Genetic Modification: A Systematic Review. Front. Cell Dev. Biol. 8:587776. doi: 10.3389/fcell.2020.587776
Received: 27 July 2020; Accepted: 01 October 2020;
Published: 30 October 2020.
Edited by:
Vivian Capilla-González, Andalusian Center for Molecular Biology and Regenerative Medicine (CABIMER), SpainReviewed by:
Alexander Rühle, Freiburg University Medical Center, GermanyDavid García-Bernal, University of Murcia, Spain
Copyright © 2020 Pawitan, Bui, Mubarok, Antarianto, Nurhayati, Dilogo and Oceandy. This is an open-access article distributed under the terms of the Creative Commons Attribution License (CC BY). The use, distribution or reproduction in other forums is permitted, provided the original author(s) and the copyright owner(s) are credited and that the original publication in this journal is cited, in accordance with accepted academic practice. No use, distribution or reproduction is permitted which does not comply with these terms.
*Correspondence: Delvac Oceandy, RGVsdmFjLk9jZWFuZHlAbWFuY2hlc3Rlci5hYy51aw==