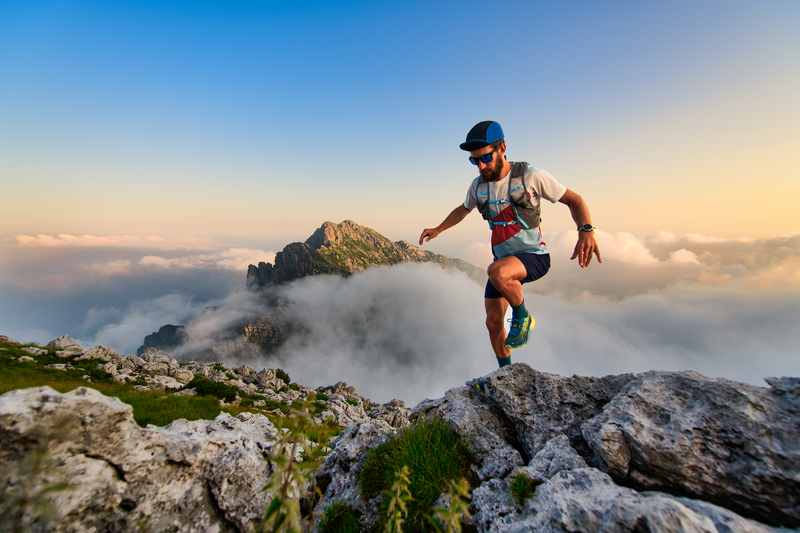
95% of researchers rate our articles as excellent or good
Learn more about the work of our research integrity team to safeguard the quality of each article we publish.
Find out more
REVIEW article
Front. Cell Dev. Biol. , 22 September 2020
Sec. Molecular and Cellular Pathology
Volume 8 - 2020 | https://doi.org/10.3389/fcell.2020.586189
This article is part of the Research Topic Bacteria and Bacterial Metabolites: Molecular Interplay with Gut Immunity View all 11 articles
There is an important task of current medicine to identify mechanisms and new markers of subclinical atherosclerosis in order to develop early targets for the diagnosis and treatment of this disease, since it causes such widespread diseases as myocardial infarction, stroke, sudden death, and other common reasons of disability and mortality in developed countries. In recent years, studies of the human microbiome in different fields of medicine have become increasingly popular; there is evidence from numerous studies of the significant contribution of microbiome in different steps of atherogenesis. This review attempted to determine the current status of the databases PubMed and Scopus (until May, 2020) to highlight current ideas on the potential role of microbiome and its metabolites in atherosclerosis development, its mechanisms of action in lipids metabolism, endothelial dysfunction, inflammatory pathways, and mitochondrial dysfunction. Results of clinical studies elucidating the relationship of microbiome with subclinical atherosclerosis and cardiovascular disease considered in this article demonstrate strong association of microbiome composition and its metabolites with atherosclerosis and cardiovascular disease. Data on microbiome impact in atherogenesis open a wide perspective to develop new diagnostic and therapeutic approaches, but further comprehensive studies are necessary.
Today atherosclerosis remains one of the most important problems of current medicine, since it causes such widespread diseases as myocardial infarction, stroke, sudden death, and other common causes of disability and mortality in developed countries. The development of atherosclerosis has a long asymptomatic phase; the first clinical manifestations appear with a significant vascular lesion. Despite the fact that modern science understands the basic mechanisms of atherogenesis, individual factors, which determining the variability of the atherosclerosis progression, are still not well studied. In this regard, it is an important task to identify mechanisms and new markers of subclinical atherosclerosis in order to develop early targets for the diagnosis and treatment of this disease. Therefore, the need to search for additional factors of the pathogenesis of this disease remains relevant (Orekhov and Ivanova, 2017).
In recent years, studies of the human microbiome in different fields of medicine have become increasingly popular (Huttenhower et al., 2012). The relationship of the gut microbiome is shown with autoimmune, inflammatory (Belkaid and Hand, 2014; Opazo et al., 2018), neurodegenerative (Endres and Schäfer, 2018), infectious diseases (Lazar et al., 2018), and cancer (Villéger et al., 2019). Studies of microbiome to understand its relationship with cardiovascular diseases, in particular, mechanisms of atherogenesis has great potential (Kazemian et al., 2020).
The gut microbiome is the first largest and most complex community of microorganisms in the human body, which consists of bacteria, viruses, archaea, fungi, protozoa, and bacteriophages. It is an ecological community of microorganisms inhabiting the gastrointestinal tract along its entire length consists of a trillion bacteria and is encoded by more than 100 times more genes than the human genome (Mills et al., 2019). The gut microbiome is an important component of homeostasis involved in physiology and metabolism in human body (Sommer and Bäckhed, 2013). It participates in the digestion processes, providing fermentation of undigested substances, the synthesis of certain vitamins, energy metabolism, and regulation of the intestinal barrier function (Ahmad et al., 2019; Derovs et al., 2019). Recent studies have shown the key role of the microbiome in the functioning of the human immune system (Belkaid and Harrison, 2017). The composition of the intestinal microbiota depends on a number of factors, such as nutrition, lifestyle, gender, age, and the use of antibiotics (Jandhyala et al., 2015; Kundu et al., 2017).
This review reflects current ideas about the role of the microbiome in the processes of atherogenesis. Using the electronic databases PubMed and Scopus, a search was conducted for the keywords “microbiome,” “atherosclerosis,” “metabolites of the microbiome,” “TMAO” until May 2020. References’ lists of the identified reviews and original research articles were hand-selected for papers those may have been missed during primary search. Only articles published in English were selected. We found about 500 articles and focused on the studies on the influence of the microbiome on the pathogenesis of atherosclerosis.
Numerous experimental and clinical studies investigate the role of microbiome and its metabolites in different steps of atherosclerosis development. Further in this article we will consider the main effects of the microbiome on lipid metabolism, inflammation, endothelial dysfunction, mitochondrial dysfunction during atherogenesis. Figure 1 represents a brief scheme of microbiome effects in basic mechanisms of atherogenesis.
Lipid metabolism disturbance is the initial stage and one of the main reasons of atherosclerosis development, which is manifested in lipid accumulation in vessel wall (Pieczynska et al., 2020). The gut microbiota may disturb lipid metabolism both in mice and humans. Microbiome composition may further correlate with atherosclerotic lesion progression (Schoeler and Caesar, 2019). Intestinal microorganisms can modulate human metabolism at the level of small molecules, including the conversion of food components into hormone-like signals and biologically active metabolites. About 10% of small molecules circulating in the bloodstream have the origin of intestinal microbiome (Herrema et al., 2020). One of such biologically active metabolites of the intestinal microbiota, which may be involved in the development of atherosclerosis, is trimethylamine N-oxide (TMAO).
The increasing level of TMAO in plasma is correlated with the lipid deposition in blood vessels and endothelial dysfunction (Hardin et al., 2019). TMAO also may impair cholesterol reverse transport, which is promoted by bile acid biosynthesis (Sun et al., 2016). Bile acids can be ligands for nuclear farnesoid X receptor (FXR), which mediates the expression of genes regulating bile acid biosynthesis and excretion (Lefebvre et al., 2009). Members of cytochrome P450 superfamily, cholesterol 7 alpha-hydroxylase (CYP7A1) and cholesterol 27 alpha-hydroxylase (CYP27A1), are important bile acid synthetic enzymes responsible for maintaining cholesterol metabolism homeostasis (Sayin et al., 2013; Lau et al., 2017). Defective metabolism of bile acids and cholesterol is associated with atherosclerosis. Recently, the link of TMAO-induced atherosclerosis with bile acid metabolism was studied in apoE−/− mice on TMAO-containing diet (Ding et al., 2018). Specifically, it was elucidated that TMAO can repress hepatic bile acid synthesis pathway by activation of small heterodimer partner (SHP) and FXR, which can down-regulate of Cyp7a1 and Cyp27a1 gene expression (Koeth et al., 2013; Ding et al., 2018). Moreover, it was demonstrated that the levels of triglyceride, total cholesterol, low-density lipoprotein cholesterol (LDL-c), as well as, total plaque areas in the aortas in TMAO administration mice were significantly increased (Ding et al., 2018).
Human studies revealed the association of altered microbiota, represented mostly by some taxa from phylum Actinobacteria, with increased triglyceride level, decreased high-density lipoprotein cholesterol (HDL-c), and slightly changed LDL-c level. HDL mediates efflux of cholesterol from cells and then transports it to liver to be excreted from an organism (Fisher et al., 2012). It was elucidated in two studies on obese and healthy European individuals, that elevated fasting triglycerides and diminished HDL-c are associated with the low abundance of the gut microbiota (Cotillard et al., 2013; Nielsen et al., 2013). Furthermore, as a result of the systemic analysis of blood lipid levels, body mass index, and gut microbiota, conducted in 893 participants from the LifeLines-DEEP cohort, it was suggested that the gut microbiota may influence only certain aspects of lipid metabolism and distinct classes of lipoproteins, such as HDL and very low-density lipoproteins (VLDL) (Fu et al., 2015). Enrichment in Bacteroides, Eubacterium, and Roseburia microbes, which are common microbiota in healthy people, can correlate with decrement of body mass index and plasma triglycerides and increment of plasma HDL (Nakaya and Ikewaki, 2018). In addition, the full suppression of gut microbiota may enhance macrophage-to-feces reverse cholesterol transport (Canyelles et al., 2018). However, there are a lot of blind spots in the TMA/FMO3/TMAO/bile acids axes in human. Thus, further studies that help to clarify the exact role of gut microbiome and TMAO in cholesterol and bile acid metabolism in human, are necessary.
Atherosclerosis is a chronic inflammatory disease, which is characterized by the accumulation of monocytes and lymphocytes within the arterial wall, the release of chemokines, interleukins, and activation of pro-inflammatory pathways within endothelial cells (Libby, 2012; Tuttolomondo et al., 2012; Nikiforov et al., 2019). Possible association of gut microbiome composition, diet, atherosclerosis, and inflammation is under thorough investigation (van den Munckhof et al., 2018). For example, saturated fat rich diet can dramatically change the gut microbiome composition, elevating the intestinal absorption of gut-derived microbial products that lead to an increased concentration of plasma lipopolysaccharide (LPS), which is called metabolic endotoxemia (Sonnenburg and Bäckhed, 2016). The activation of inflammatory signaling pathways in the intestine is one of the factors determining the influence of the gut microbiome on the development of atherosclerosis. LPS, the main component of the outer membrane of gram-negative bacteria, can penetrate the bloodstream as a result of the intestinal mucosa barrier function disturbance (Ahmad et al., 2019). Consequently, metabolic endotoxemia provokes macrophage activation via TLR4/MyD88/TRIF signaling (Caesar et al., 2015). TLR4/MyD88/TRIF is activated by the lipid A component of LPS and this causes inflammatory signaling pathways (Herrema et al., 2020; Caesar et al., 2015). Thus, microbiome alteration based on a diet type may influence on pro-inflammatory activation, which is correlated with atherosclerosis since pro-inflammatory macrophages are one of the key cells in atherogenesis. The role of microbiome composition in pro-inflammatory status was further demonstrated in mice on high-fat diet, which is positively correlated with metabolic endotoxemia and endothelial dysfunction (Toral et al., 2014). It was shown, that Lactobacillus coryniformis administration as a probiotic promoted the increase in the intestinal mucus glycoprotein mucin-3, which restores gut barrier function and subsequently decreases plasma LPS level. Moreover, L. coryniformis administration lowered IL-6 and IL-1β expression in aorta of these mice. In addition, it was elucidated in germ free mice that the absence of microbiota has beneficial effect on arterial lesion and helps to decrease inflammatory cytokines in macrophages (Kasahara et al., 2017).
Genetic predisposition to atherosclerosis development is widely studied nowadays. Mitochondrial dysfunction is one of the possible genetic factors that may lead to the progression of atherosclerosis (Sazonova et al., 2017; Orekhov et al., 2020). In previous studies the association of mitochondrial heteroplasmy and atherosclerosis was demonstrated. Several variants of mitochondrial heteroplasmy were found in atherosclerotic lesions of human aorta (Sazonova et al., 2015; Sobenin et al., 2019) as well as the correlation of these mitochondrial mutations in white blood cells with carotid atherosclerosis was demonstrated in some clinical trials (Kirichenko et al., 2018, 2020a). Inflammatory changes leading to the progression of atherosclerosis, the manifestation of CVD and the development of their complications, develop with age due to many factors, including oxidative stress caused by mitochondrial dysfunction as well as inflammatory processes caused by changes in the composition of the microbiota described above (Ostan et al., 2016; Ferrucci and Fabbri, 2018). At the same time, association of mitochondrial dysfunction and alterations of gut microbiota are studied in several trials (Ma et al., 2014; Hirose et al., 2017). It was demonstrated in subjects with Crohn’s disease that downregulation of mitochondrial proteins, which indicates the central role of mitochondria dysfunction in the pathogenesis of inflammatory bowel disease, is associated with a depletion of butyrate producers, suggesting a signaling role for butyrate in host mitochondrial genes expression (Mottawea et al., 2016). It is assumed that gut microbiota signaling to mitochondria leads to the activation of immune cells and inflammation, and changes the function of the epithelial barrier (Jackson and Theiss, 2019). It was proposed that production of toxins by dysbiotic gut microbiome activates neuronal innate immunity and inflammatory processes that lead to the development of neurodegenerative processes in patients with Parkinson’s disease (Cardoso and Empadinhas, 2018). As for the possible relationship of the microbiome and mitochondria in the pathogenesis of atherosclerosis, the mechanisms of their interaction are not well studied at present. However, we can assume the combined influence of these factors on the development of atherosclerosis, since mitochondria are bacterial in origin, and therefore microbiome products can affect their functions, stimulating processes that play an important role in atherogenesis (Clark and Mach, 2017; Bajpai et al., 2018). In particular, gut microbiome influences the production of reactive oxygen species (ROS) in mitochondria and regulates their inflammatory activity (Saint-Georges-Chaumet and Edeas, 2016; Clark and Mach, 2017). Overproduction of ROS stimulates inflammatory reactions which affect the development of atherosclerosis (Forrester et al., 2018). On the other hand, in mice, engineered for overproduction of ROS in mitochondria and in aged mice, the composition of the intestinal microbiota changed compared to the initial one, Shannon diversity significantly decreased, that demonstrates the reverse effect of mitochondrial dysfunction on microbiome. At the same study, in mice treated with N-acetylcysteine or engineered to produce more mitochondrial catalase, ROS production decreased significantly that led to significant increase in Shannon diversity (Yardeni et al., 2019). So, the microbiome-mitochondria interaction may be an important factor in mechanisms of atherogenesis, but further studies are required.
The prevailing phyla of bacteria compounded the gut microbiome are the Actinobacteria, Bacteroides, Firmicutes, Proteobacteria, and Verrucomicrobiota. The balance between Bacteroides and Firmicutes may determine the health of gastrointestinal tract (Jama et al., 2019). Shift to Firmicutes domination may lead to dysbiotic environments, which is beneficial to TMAO accumulation. TMAO is produced in liver by flavin-containing monooxygenase 3 (FMO3) from the precursor trimethylamine (TMA), which is generated in gut from metabolic intermediates incorporating the TMA-moiety, such as betaine, γ-butyrobetaine, choline, phosphatidylcholine, and L-carnitine (Chen K. et al., 2017). TMA lyases are gut microbe enzymes, which convert choline to TMA. An inhibition of these lyases may reduce atherosclerosis in mice (Canyelles et al., 2018).
Circulating plasma TMAO levels are determined by several factors, including the consumption of its metabolic precursors, medication, and liver flavin monooxygenase activity (Janeiro et al., 2018). The level of TMAO largely depends on the composition of the intestinal microbiome. The predominant bacterial types are Bacteroides and Firmicutes, and Actinobacteria, Proteobacteria, and Verrucomicrobia are less common. The splitting processes of the main source of TMAO, choline, are regulated by the intestinal microbiota, in particular, the phylum Firmicutes and Proteobacteria, and the genera Anaerococcus hydrogenalis, Clostridium asparagiforme, Clostridium hathewayi, Clostridium sporogenes, Escherichia fergusonii, Proteus penneri, Providencia rettgeri, and Edwardsiella tarda. The main microbial species responsible for the degradation of another TMAO source of L-carnitine are the phylum Proteobacteria and Bacteroides and the family Prevotellaceae (Zhu et al., 2020).
Recent clinical studies confirm the close relationship of atherosclerosis with the level of TMAO. Increased plasma TMAO levels in patients are associated with an increased risk of adverse cardiovascular events such as stroke, heart attack, and death (Tang et al., 2013). It was found in the study on patients with acute coronary syndrome (ACS) that elevated TMAO value is a high predictor of long-term mortality risk (Senthong et al., 2016b).
The early stage of atherosclerosis is the accumulation of foam cells in the intima of the arteries. Most foam cells come from macrophages that can regulate lipoprotein metabolism (Poznyak et al., 2020). The association of TMAO with stimulation of atherosclerosis progression was first demonstrated in animal models where TMAO led to the formation of foam cells in atherosclerotic lesions by increasing expression of the CD36 and SR-A1 scavenger receptors (Wang et al., 2011). It is known that the main reason for the formation of foam cells is the excessive influx of modified LDL and the accumulation of cholesterol esters in macrophages. Macrophages express various CD36 and SR-A1 scavenger receptors, as well as the lectin-like receptor oxLDL-1 (LOX-1), with affinity to oxidized low-density lipoproteins (oxLDL). This in turn induces the formation of foam cells (Chistiakov et al., 2017). It has been shown that macrophage foam cell formation was increased in apoE−/− mice on TMAO-, choline-, or L-carnitine-fed diets (Wang et al., 2011). Up-regulation of macrophage scavenger receptors CD36 and SR-A by TMAO is one the plausible mechanisms underlying this process (Koeth et al., 2013). TMAO is probably involved in the process of atherogenesis, contributing to the migration of macrophages, the accumulation of ox-LDL in them, and their transformation into foam cells (Mohammadi et al., 2016; Janeiro et al., 2018). In addition, studies revealed that TMAO positively regulates the expression of VCAM-1 in endothelial cells, leading to an increase in monocyte adhesion, which is an early sign of foamy cell formation (Ma et al., 2017).
Trimethylamine N-oxide is believed to enhance atherosclerosis by influencing inflammatory processes in vascular wall by activating the inflammatory pathway (Ahmad et al., 2019). The processes of inflammation are involved in all stages of the development of atherosclerosis, from the initial lesions to the terminal stage of thrombotic complications. Most often, the processes begin with inflammatory changes in the vascular endothelium, characterized by the expression of VCAM-1 and monocyte adhesion (Ma et al., 2017). Elevating plasma level of TMAO is associated with the high risk of atherosclerosis and cardiovascular disease due to chronic inflammation and the recruitment of leukocytes to endothelium (Al-Obaide et al., 2017). TMAO promotes macrophage migration and an increase in the expression of inflammatory cytokines such as TNF-α and IL-6 and a decrease of the expression of the anti-inflammatory cytokine IL-10 (Zhu et al., 2020). In response to inflammatory activation, macrophages can penetrate the vascular endothelium and accumulate in the intima, thereby causing the formation of plaques (Chistiakov et al., 2017). It was shown that an increase in TMAO levels led to the activation of a mitogen-activated protein kinase (MAPK), a signaling cascade of nuclear factor-κB (NF-κB), and also to over-expression of pro-inflammatory genes, inflammatory cytokines, adhesion molecules, and chemokines, so TMAO elevation stimulates the inflammatory response within endothelial and smooth muscle cells (Seldin et al., 2016). In addition, TMAO can trigger vascular inflammation by the NLRP3 mechanism – inflammasome is an IL-1β family cytokine-activating protein complex, consisting of the pattern recognition receptor NLRP3, adaptor protein apoptotic speck-like protein, and inactive pro-caspase-1, and involved in the regulation of inflammatory response (Chen M. L. et al., 2017). It was also shown, that TMAO can activate inflammation via NOD-like receptor family and may lead to NLRP3 inflammasome activation through the SIRT3-SOD2-mtROS signaling pathway in human umbilical vein endothelial cell and aortas from apoE−/− mice. It was demonstrated at the same study that TMAO may induce the expression of cyclooxygenase-2 (COX-2), IL-6, E-selectin, and intercellular adhesion molecule-1 (ICAM-1) in primary human aortic endothelium cells. It can also promote production of COX-2, IL-6, TNF-α, and ICAM-1 in vascular smooth muscle cells (Matsumoto et al., 2020).
In addition to the activation of NLRP3 – inflammasome, TMAO can affect the processes leading to endothelial dysfunction, which is also an important factor in the development of atherosclerosis. In experiments on cell cultures, it has been shown that TMAO can induce oxidative stress and activate NLRP3 – inflammasome, results in the release of inflammatory cytokines, and reduce the level of endothelial nitric oxide synthase (eNOS) and the production of nitric oxide (NO) (Sun et al., 2016). Intracellular inflammation and oxidative stress reactions lead to the formation of ROS and a decrease in NO. In addition, this leads to inhibition of the function of circulating endothelial progenitor cells (EPCs), which also has a negative effect on maintaining the function of the vascular wall. All these pathophysiological processes ultimately lead to endothelial dysfunction and the development of atherosclerosis (Chou et al., 2019).
The effect of TMAO on platelet activation processes and following thrombus formation, which play an important role in atherosclerotic events, has been shown in several studies (Kasahara and Rey, 2019). A dose-dependent effect was revealed between the increased content of TMAO in blood plasma and platelet hyperreactivity. Most likely, this is due to the influence of TMAO on the release of intracellular Ca2+ stores from platelets and their activation as a result of stimulation of ADP, thrombin, collagen, arachidonic acid (Zhu et al., 2016).
Besides TMAO, which makes a significant contribution to the development of atherosclerosis, there are other biologically active metabolites of the gut microbiota. One of them is short chain fatty acids (SCFA), such as acetate, butyrate and propionate, resulting from the fermentation of undigested dietary fiber. They play an important regulatory role, act as substrates in cholesterol and lipid metabolism (Chen et al., 2020; Zeng and Tan, 2020). SCFA have anti-inflammatory effects by inhibiting the migration and proliferation of cells of the immune system and the production of cytokines, which can weaken the progression of atherosclerosis (Ohira et al., 2017).
Other metabolites of the gut microbiome are aromatic amino acids. The metabolism of phenylalanine, tyrosine, tryptophan, and histidine leads to the formation of compounds that can also affect the development of cardiovascular diseases. For example, tryptophan metabolism results in indole ethanol (IE), indolepropionic acid (IPA) and indole acrylic acid (IA), which are absorbed through the intestines and have anti-inflammatory activity, which can be a beneficial for CVD development (Kasahara and Rey, 2019). Other uremic toxins, indoxyl sulfate and p-cresyl sulfate, are metabolites derived from tyrosine, phenylalanine, and tryptophan by gut microbiota (Nallu et al., 2017; Pereira-Fantini et al., 2017). Indoxyl sulfate is produced in liver from the Escherichia coli metabolite indole. Indoxyl sulfate can activate IL-6 expression in both endothelium and smooth muscle cells via organic anion transporters/aryl hydrocarbon receptor/NF-κB pathway that has been elucidated in mice studies (Matsumoto et al., 2020). It has also been studied that indoxyl sulfate can increase ICAM-1, TNFα, and monocyte chemoattractant protein-1 (MCP-1) expression (Tumur et al., 2010). Elevated plasma p-cresyl sulfate is a risk factor of cardiovascular disease in patients with chronic kidney disease, since it can up-regulate MCP-1, ICAM-1, TNF-α, and vascular cell adhesion molecule-1 expression in endothelium cells. It was also demonstrated that increased p-cresyl sulfate level may promote atherosclerotic lesion in apoE−/− mice with chronic kidney disease (Jing et al., 2016). Furthermore, it was shown in human study, that oat and barley beta-glucans, prebiotic fibers with proven cholesterol-lowering activity, significantly reduced LDL and total cholesterol, and serum p-cresyl sulfate levels, which may have positive effect on endothelial function (Cosola et al., 2017).
It is believed that circadian rhythms affect the development of atherosclerosis since immune cell activity depends on the circadian clock (Winter and Soehnlein, 2018). Conventional cardiovascular risk factors such as blood pressure, pulse rate, endothelial function are also subjected to diurnal variation (Kawano et al., 2002; Paschos and FitzGerald, 2010). The composition of gut microbiota, which is associated with atherogenesis, is also characterized by diurnal variations. However, it is still not entirely clear how the circadian clock and gut microbiota, in particular bacterial metabolites such as TMAO, are associated with the progression of atherosclerosis. Clock (Circadian Locomotor Output Cycles Kaput) and Bmal1 [Brain and Muscle ARNT (aryl hydrocarbon receptor nuclear translocator) -Like 1], two major circadian clock genes, play important regulatory role in atherogenesis, expression of Bmal1 and Clock decreased in patients with atherosclerosis (Winter and Soehnlein, 2018; Man et al., 2020). TMAO induces increased expression of lncRNA-NEAT1, Clock and Bmal1 and inhibits the MAPK pathways (Wu et al., 2019a). Some researchers suggest that pro-atherosclerotic changes in the composition of the microbiota are due to the influence of toxic products, in particular, the environmental pollutant acrolein. Acrolein exposure increased MMP9, decreased Clock and Bmal1, and activated MAPK-pathways in human umbilical vein endothelial cells in plasma of apolipoprotein-E deficient mice fed a high fat diet with acrolein. At the same, in this model feeding with acrolein changed the composition of the gut microbiota – increase of Firmicutes and decrease of Bacteroidetes and these changes correlated with atherosclerotic plaque, MMP9 and Bmal1 levels (Wu et al., 2019b). Some analogy in the change in daily fluctuations in the composition of the intestinal microbiota was found in the study on a mouse model of diabetes. Rhythmic oscillations of gut microbiota were observed in diabetic mice as well as analysis of circulating metabolites showed changes in the daily concentration of metabolites of the histidine pathway and the metabolism of betaine, methionine, cysteine, followed by an increase in TMAO production (Beli et al., 2019). Moreover, in the study of atherosclerosis, associated with infection of Porphyromonas gingivalis that causes periodontitis, circadian clock disruption enhanced atherosclerosis progression in Bmal1−/− ApoE−/− mice (Xie et al., 2020). Despite of a number of studies on the influence of circadian rhythms on atherogenesis and the composition of gut microbiome and its metabolites, the mechanism of the influence of diurnal variations in the microbiome on the development of atherosclerosis is still an actual topic for research in the near future.
It is known that one of the surrogate markers and an indicator of early atherosclerosis, widely used in epidemiological and interventional studies, is the carotid intima-media thickness (cIMT) measured in common carotid arteries by high-resolution ultrasonography (Baldassarre et al., 2010; Kirichenko et al., 2020b). On the other hand, there is increasing evidence showing the association of TMAO with an increased risk of cardiovascular disease development (Senthong et al., 2016a; Haghikia et al., 2018). Table 1 summarizes the clinical studies on the association of TMAO with atherosclerosis and CVD.
The positive correlation of cIMT values with circulating TMAO levels, regardless of gender, age, and visceral fat mass was demonstrated in 220 participants of Tübingen Lifestyle Intervention Program. In this study, lifestyle intervention led to improvement of cardiovascular risk factor, but TMAO level did not change significantly, at the same time, cIMT decreased significantly only in participants with large TMAO decrease (Randrianarisoa et al., 2016). It was shown in another study in 175 CVD-free HIV-infected patients, who have a higher cardiovascular risk than non-infected individuals due to HIV infection, that cIMT was higher toward higher TMAO concentration (Montrucchio et al., 2020). There are some contradictions regarding the role of TMAO in early atherosclerosis, probably caused by insufficient data. In some studies, patients with atherosclerosis did not have an elevated serum TMAO level compared with healthy participants, but an increase in the content of its precursor, L-carnitine, was found (Skagen et al., 2016). Another clinical study in 817 patients aged 33–45 years showed that the concentration of TMAO was associated neither with cIMT nor with coronary calcium measured by computed tomography, which may indicate a slight effect of TMAO in the development of early atherosclerosis in comparison with other studies in older people (Meyer et al., 2016). In addition to studies on the association of the TMAO level and cIMT in patients with subclinical atherosclerosis, this indicator is also used for cardiovascular risk prognosis in patients with clinical manifestations of atherosclerosis, namely, coronary artery disease (CAD). In particular, it was shown in over 4000 participants mean aged 63 years old at least single-vessel CAD, that elevated plasma concentration of TMAO was a strong predictor of increased risk of adverse cardiovascular events such as myocardial infarction, stroke and death after 3 years of follow-up. At baseline, the addition of TMAO to traditional risk factors as a covariate significantly improved cardiovascular risk estimation (Tang et al., 2013). These data may indicate the effect of TMAO at later stages of the atherosclerosis development, in high-risk subgroups, as well as among older people.
In addition to TMAO, the association of microbiome composition and other microbiome characteristics with atherosclerosis and CVD are also being investigated in epidemiological studies. For example, sequencing of gut microbiome in 218 individuals with atherosclerosis-based cardiovascular disease and 187 healthy controls demonstrates that among major genera of the gut microbiome, there is a relative reduction in Bacteroides and Prevotella, and enrichment in Streptococcus and Escherichia in atherosclerotic patients. At the same time, the abundance of Enterobacteriaceae including E. coli, Klebsiella spp., Enterobacter aerogenes, Eggerthella lenta, and Ruminococcus gnavus was higher in atherosclerotic patients than in control samples (q-value < 0.05 for all) (Jie et al., 2017). The study in patients, who undergone surgery to excise an atherosclerotic plaque, in comparison with CVD-free participants, shows, that genus Collinsella was enriched in patients with symptomatic atherosclerosis and Eubacterium and Roseburia were enriched in controls (p < 0.05) (Karlsson et al., 2012). In another study, the composition of gut microbiota was compared in 39 patients with CAD, 30 patients with coronary risk factors and 50 healthy individuals; the order of Lactobacillales was increased (p < 0.001) and the phylum Bacteroidetes (Bacteroides + Prevotella) was decreased (p < 0.001) in CAD group (Emoto et al., 2016).
Besides gut microbiome, oral cavity is also an important source of bacteria which affect atherosclerosis and CVD development. So, it was demonstrated in almost 12,000 study participants that poor dental hygiene increases risk of cardiovascular disease events and cardiovascular disease death (p = 0.001, for trend) (de Oliveira et al., 2010). INVEST study demonstrates the association of periodontal bacterial burden with cIMT in 657 patients over 55 years old without history of stroke, myocardial infarction, or chronic inflammatory diseases. cIMT increased across tertiles of etiologic bacterial burden which summing the standardized values for the bacteria causative of periodontal disease (P. gingivalis, Tannerella forsythensis, Aggregatibacter Actinomycetemcomitans, and Treponema denticola) in the subgingival plaque (p = 0.03) as well as across predominance of causative bacteria over other health-associated bacteria (p = 0.002) (Desvarieux et al., 2005). It was also demonstrated in the study of oral microbiota in atherosclerotic patients that abundance of Anaeroglobus was significantly higher in patients with symptomatic atherosclerosis than in control group (Fak et al., 2015). Additionally, it was detected in the study in 492 participants, that median salivary levels of A. actinomycetemcomitans were significantly higher in a group of patients with stable coronary artery disease (p = 0.014) and in a group with ACS (p = 0.044) than in control group (Hyvärinen et al., 2012).
This review highlighted currently published data on the impact of microbiome in basic steps of the pathogenesis of atherosclerosis. Studies investigating the association of human microbiome with subclinical atherosclerosis and cardiovascular disease demonstrate prognostic value of TMAO in cardiovascular risk assessment and revealed several types of bacteria affecting the development of atherosclerosis and its clinical complications. Data on microbiome impact in atherosclerosis development open a wide perspective to develop new diagnostic and therapeutic approaches which can be especially relevant in case of subclinical atherosclerosis. Further comprehensive study of the mechanisms of microbiome impact in atherosclerosis development is still necessary.
AO and W-KW: conceptualization and design the review, and review final version approval. TK, VS, YM, and VK: bibliographic research. TK, VS, and YM: writing – original draft preparation. TK, VS, and VK: table and figure design. W-KW and TK: supervision. AO: funding acquisition. All authors contributed to the article and approved the submitted version.
This work was supported by Russian Science Foundation (Grant # 20-15-00337).
The authors declare that the research was conducted in the absence of any commercial or financial relationships that could be construed as a potential conflict of interest.
Ahmad, A. F., Dwivedi, G., O’Gara, F., Caparros-Martin, J., and Ward, N. C. (2019). The gut microbiome and cardiovascular disease: current knowledge and clinical potential. Am. J. Physiol. Heart Circ. Physiol. 317, H923–H938. doi: 10.1152/ajpheart.00376.2019
Al-Obaide, M., Singh, R., Datta, P., Rewers-Felkins, K., Salguero, M., Al-Obaidi, I., et al. (2017). Gut microbiota-dependent trimethylamine-N-oxide and serum biomarkers in patients with T2DM and advanced CKD. J. Clin. Med. 6:86. doi: 10.3390/jcm6090086
Bajpai, P., Darra, A., and Agrawal, A. (2018). Microbe-mitochondrion crosstalk and health: an emerging paradigm. Mitochondrion 39, 20–25. doi: 10.1016/j.mito.2017.08.008
Baldassarre, D., Nyyssönen, K., Rauramaa, R., de Faire, U., Hamsten, A., Smit, A. J., et al. (2010). Cross-sectional analysis of baseline data to identify the major determinants of carotid intima-media thickness in a European population: the IMPROVE study. Eur. Heart J. 31, 614–622. doi: 10.1093/eurheartj/ehp496
Beli, E., Prabakaran, S., Krishnan, P., Evans-Molina, C., and Grant, M. B. (2019). Loss of diurnal oscillatory rhythms in gut microbiota correlates with changes in circulating metabolites in type 2 diabetic db/db mice. Nutrients 11:2310. doi: 10.3390/nu11102310
Belkaid, Y., and Hand, T. W. (2014). Role of the microbiota in immunity and inflammation. Cell 157, 121–141. doi: 10.1016/j.cell.2014.03.011
Belkaid, Y., and Harrison, O. J. (2017). Homeostatic immunity and the microbiota. Immunity 46, 562–576. doi: 10.1016/j.immuni.2017.04.008
Caesar, R., Tremaroli, V., Kovatcheva-Datchary, P., Cani, P. D., and Bäckhed, F. (2015). Crosstalk between gut microbiota and dietary lipids aggravates WAT inflammation through TLR signaling. Cell Metab. 22, 658–668. doi: 10.1016/j.cmet.2015.07.026
Canyelles, M., Tondo, M., Cedó, L., Farràs, M., Escolà-Gil, J., and Blanco-Vaca, F. (2018). Trimethylamine N-oxide: a link among diet, gut microbiota, gene regulation of liver and intestine cholesterol homeostasis and HDL function. Int. J. Mol. Sci. 19:3228. doi: 10.3390/ijms19103228
Cardoso, S. M., and Empadinhas, N. (2018). The microbiome-mitochondria dance in prodromal Parkinson’s disease. Front. Physiol. 9:471. doi: 10.3389/fphys.2018.00471
Chen, K., Zheng, X., Feng, M., Li, D., and Zhang, H. (2017). Gut microbiota-dependent metabolite trimethylamine N-oxide contributes to cardiac dysfunction in western diet-induced obese mice. Front. Physiol. 8:139. doi: 10.3389/fphys.2017.00139
Chen, M. L., Zhu, X. H., Ran, L., Lang, H. D., Yi, L., and Mi, M. T. (2017). Trimethylamine-N-oxide induces vascular inflammation by activating the NLRP3 inflammasome through the SIRT3-SOD2-mtROS signaling pathway. J. Am. Heart Assoc. 6:e006347. doi: 10.1161/JAHA.117.006347
Chen, X. F., Chen, X., and Tang, X. (2020). Short-chain fatty acid, acylation and cardiovascular diseases. Clin. Sci. 134, 657–676. doi: 10.1042/CS20200128
Chistiakov, D. A., Melnichenko, A. A., Myasoedova, V. A., Grechko, A. V., and Orekhov, A. N. (2017). Mechanisms of foam cell formation in atherosclerosis. J. Mol. Med. 95, 1153–1165.
Chou, R. H., Chen, C. Y., Chen, I. C., Huang, H. L., Lu, Y. W., Kuo, C. S., et al. (2019). Trimethylamine N-oxide, circulating endothelial progenitor cells, and endothelial function in patients with stable angina. Sci. Rep. 9:4249. doi: 10.1038/s41598-019-40638-y
Clark, A., and Mach, N. (2017). The crosstalk between the gut microbiota and mitochondria during exercise. Front. Physiol. 8:319. doi: 10.3389/fphys.2017.00319
Cosola, C., De Angelis, M., Rocchetti, M. T., Montemurno, E., Maranzano, V., Dalfino, G., et al. (2017). Beta-glucans supplementation associates with reduction in P-cresyl sulfate levels and improved endothelial vascular reactivity in healthy individuals. PLoS One 12:e0169635. doi: 10.1371/journal.pone.0169635
Cotillard, A., Kennedy, S. P., Kong, L. C., Prifti, E., Pons, N., Le Chatelier, E., et al. (2013). Dietary intervention impact on gut microbial gene richness. Nature 500, 585–588. doi: 10.1038/nature12480
de Oliveira, C., Watt, R., and Hamer, M. (2010). Toothbrushing, inflammation, and risk of cardiovascular disease: results from Scottish health survey. BMJ 340:c2451. doi: 10.1136/bmj.c2451
Derovs, A., Laivacuma, S., and Krumina, A. (2019). Targeting microbiota: what do we know about it at present? Medicina 55:459. doi: 10.3390/medicina55080459
Desvarieux, M., Demmer, R. T., Rundek, T., Boden-Albala, B., Jacobs, D. R. Jr., Sacco, R. L., et al. (2005). Periodontal microbiota and carotid intima-media thickness: the oral infections and vascular disease epidemiology study (INVEST). Circulation 111, 576–582. doi: 10.1161/01.CIR.0000154582.37101.15
Ding, L., Chang, M., Guo, Y., Zhang, L., Xue, C., Yanagita, T., et al. (2018). Trimethylamine-N-oxide (TMAO)-induced atherosclerosis is associated with bile acid metabolism. Lipids Health Dis. 17:286.
Emoto, T., Yamashita, T., Sasaki, N., Hirota, Y., Hayashi, T., So, A., et al. (2016). Analysis of gut microbiota in coronary artery disease patients: a possible link between gut microbiota and coronary artery disease. J. Atheroscler. Thromb. 23, 908–921. doi: 10.5551/jat.32672
Endres, K., and Schäfer, K. H. (2018). Influence of commensal microbiota on the enteric nervous system and its role in neurodegenerative diseases. J. Innate Immun. 10, 172–180. doi: 10.1159/000488629
Fak, F., Tremaroli, V., Bergström, G., and Bäckhed, F. (2015). Oral microbiota in patients with atherosclerosis. Atherosclerosis 243, 573–578. doi: 10.1016/j.atherosclerosis.2015.10.097
Ferrucci, L., and Fabbri, E. (2018). Inflammageing: chronic inflammation in ageing, cardiovascular disease, and frailty. Nat. Rev. Cardiol. 15, 505–522.
Fisher, E. A., Feig, J. E., Hewing, B., Hazen, S. L., and Smith, J. D. (2012). High-density lipoprotein function, dysfunction, and reverse cholesterol transport. Arterioscler. Thromb. Vasc. Biol. 32, 2813–2820. doi: 10.1161/ATVBAHA.112.300133
Forrester, S. J., Kikuchi, D. S., Hernandes, M. S., Xu, Q., and Griendling, K. K. (2018). Reactive oxygen species in metabolic and inflammatory signaling. Circ. Res. 122, 877–902. doi: 10.1161/CIRCRESAHA.117.311401
Fu, J., Bonder, M. J., Cenit, M. C., Tigchelaar, E. F., Maatman, A., Dekens, J. A. M., et al. (2015). The gut microbiome contributes to a substantial proportion of the variation in blood lipids. Circ. Res. 117, 817–824. doi: 10.1161/CIRCRESAHA.115.306807
Haghikia, A., Li, X. S., Liman, T. G., Bledau, N., Schmidt, D., Zimmermann, F., et al. (2018). Gut microbiota-dependent trimethylamine N-oxide predicts risk of cardiovascular events in patients with stroke and is related to proinflammatory monocytes. Arterioscler. Thromb. Vasc. Biol. 38, 2225–2235. doi: 10.1161/ATVBAHA.118.311023
Hardin, S. J., Singh, M., Eyob, W., Molnar, J. C., Homme, R. P., George, A. K., et al. (2019). Diet-induced chronic syndrome, metabolically transformed trimethylamine-N-oxide, and the cardiovascular functions. Rev. Cardiovasc. Med. 20, 121–128. doi: 10.31083/j.rcm.2019.03.518
Herrema, H., Nieuwdorp, M., and Groen, A. K. (2020). “Microbiome and cardiovascular disease,” in Handbook of Experimental Pharmacology, ed. J. E. Barrett (Berlin: Springer), 1–24. doi: 10.1007/164_2020_356
Hirose, M., Künstner, A., Schilf, P., Sünserhauf, A., Rupp, J., Jöhren, O., et al. (2017). Mitochondrial gene polymorphism is associated with gut microbial communities in mice. Sci. Rep. 7:15293. doi: 10.1038/s41598-017-15377-7
Huttenhower, C., Gevers, D., Knight, R., Abubucker, S., Badger, J. H., Chinwalla, A. T., et al. (2012). Structure, function and diversity of the healthy human microbiome. Nature 486, 207–214. doi: 10.1038/nature11234
Hyvärinen, K., Mäntylä, P., Buhlin, K., Paju, S., Nieminen, M. S., Sinisalo, J., et al. (2012). A common periodontal pathogen has an adverse association with both acute and stable coronary artery disease. Atherosclerosis 223, 478–484. doi: 10.1016/j.atherosclerosis.2012.05.021
Jackson, D. N., and Theiss, A. L. (2019). Gut bacteria signaling to mitochondria in intestinal inflammation and cancer. Gut Microbes 26, 1–20. doi: 10.1080/19490976.2019.1592421
Jama, H. A., Kaye, D. M., and Marques, F. Z. (2019). The gut microbiota and blood pressure in experimental models. Curr. Opin. Nephrol. Hypertens. 28, 97–104. doi: 10.1097/MNH.0000000000000476
Jandhyala, S. M., Talukdar, R., Subramanyam, C., Vuyyuru, H., Sasikala, M., and Reddy, D. N. (2015). Role of the normal gut microbiota. World J. Gastroenterol. 21, 8787–8803. doi: 10.3748/wjg.v21.i29.8787
Janeiro, M. H., Ramírez, M. J., Milagro, F. I., Martínez, J. A., and Solas, M. (2018). Implication of trimethylamine n-oxide (TMAO) in disease: potential biomarker or new therapeutic target. Nutrients 10:1398. doi: 10.3390/nu10101398
Jie, Z., Xia, H., Zhong, S. L., Feng, Q., Li, S., Liang, S., et al. (2017). The gut microbiome in atherosclerotic cardiovascular disease. Nat. Commun. 8:845.
Jing, Y. J., Ni, J. W., Ding, F. H., Fang, Y. H., Wang, X. Q., Wang, H. B., et al. (2016). P-Cresyl sulfate is associated with carotid arteriosclerosis in hemodialysis patients and promotes atherogenesis in apoE-/- mice. Kidney Int. 89, 439–449. doi: 10.1038/ki.2015.287
Karlsson, F. H., Fåk, F., Nookaew, I., Tremaroli, V., Fagerberg, B., Petranovic, D., et al. (2012). Symptomatic atherosclerosis is associated with an altered gut metagenome. Nat. Commun. 3:1245. doi: 10.1038/ncomms2266
Kasahara, K., and Rey, F. E. (2019). The emerging role of gut microbial metabolism on cardiovascular disease. Curr. Opin. Microbiol. 50, 64–70. doi: 10.1016/j.mib.2019.09.007
Kasahara, K., Tanoue, T., Yamashita, T., Yodoi, K., Matsumoto, T., Emoto, T., et al. (2017). Commensal bacteria at the crossroad between cholesterol homeostasis and chronic inflammation in atherosclerosis. J. Lipid Res. 58, 519–528. doi: 10.1194/jlr.M072165
Kawano, H., Motoyama, T., Yasue, H., Hirai, N., Waly, H. M., Kugiyama, K., et al. (2002). Endothelial function fluctuates with diurnal variation in the frequency of ischemic episodes in patients with variant angina. J. Am. Coll. Cardiol. 40, 266–270.
Kazemian, N., Mahmoudi, M., Halperin, F., Wu, J. C., and Pakpour, S. (2020). Gut microbiota and cardiovascular disease: opportunities and challenges. Microbiome 8:36.
Kirichenko, T. V., Ragino, Y. I., Voevoda, M. I., Urazalina, S. J., Khasanova, Z. B., Orekhova, V. A., et al. (2020a). Data on association of mitochondrial heteroplasmy with carotid intima-media thickness in subjects from Russian and Kazakh populations. Data Brief 29:105136. doi: 10.1016/j.dib.2020.105136
Kirichenko, T. V., Sukhorukov, V. N., Markin, A. M., Nikiforov, N. G., Liu, P.-Y., Sobenin, I. A., et al. (2020b). Medicinal plants as a potential and successful treatment option in the context of atherosclerosis. Front. Pharmacol. 11:403. doi: 10.3389/fphar.2020.00403
Kirichenko, T. V., Sobenin, I. A., Khasanova, Z. B., Orekhova, V. A., Melnichenko, A. A., Demakova, N. A., et al. (2018). Data on association of mitochondrial heteroplasmy and cardiovascular risk factors: comparison of samples from Russian and Mexican populations. Data Brief 18, 16–21. doi: 10.1016/j.dib.2018.02.068
Koeth, R. A., Wang, Z., Levison, B. S., Buffa, J. A., Org, E., Sheehy, B. T., et al. (2013). Intestinal microbiota metabolism of l-carnitine, a nutrient in red meat, promotes atherosclerosis. Nat. Med. 19, 576–585. doi: 10.1038/nm.3145
Kundu, P., Blacher, E., Elinav, E., and Pettersson, S. (2017). Our gut microbiome: the evolving inner self. Cell 171, 1481–1493. doi: 10.1016/j.cell.2017.11.024
Lau, K., Srivatsav, V., Rizwan, A., Nashed, A., Liu, R., Shen, R., et al. (2017). Bridging the gap between gut microbial dysbiosis and cardiovascular diseases. Nutrients 9:859. doi: 10.3390/nu9080859
Lazar, V., Ditu, L. M., Pircalabioru, G. G., Gheorghe, I., Curutiu, C., Holban, A. M., et al. (2018). Aspects of gut microbiota and immune system interactions in infectious diseases, immunopathology, and cancer. Front. Immunol. 9:1830. doi: 10.3389/fimmu.2018.01830
Lefebvre, P., Cariou, B., Lien, F., Kuipers, F., and Staels, B. (2009). Role of bile acids and bile acid receptors in metabolic regulation. Physiol. Rev. 89, 147–191. doi: 10.1152/physrev.00010.2008
Li, X. S., Obeid, S., Klingenberg, R., Gencer, B., Mach, F., Räber, L., et al. (2017). Gut microbiota-dependent trimethylamine N-oxide in acute coronary syndromes: a prognostic marker for incident cardiovascular events beyond traditional risk factors. Eur. Heart J. 38, 814–824. doi: 10.1093/eurheartj/ehw582
Libby, P. (2012). Inflammation in atherosclerosis. Arterioscler. Thromb. Vasc. Biol. 32, 2045–2051. doi: 10.1161/ATVBAHA.108.179705
Ma, G. H., Pan, B., Chen, Y., Guo, C. X., Zhao, M. M., Zheng, L. M., et al. (2017). Trimethylamine N-oxide in atherogenesis: impairing endothelial self-repair capacity and enhancing monocyte adhesion. Biosci. Rep. 37:BSR20160244. doi: 10.1042/BSR20160244
Ma, J., Coarfa, C., Qin, X., Bonnen, P. E., Milosavljevic, A., Versalovic, J., et al. (2014). mtDNA haplogroup and single nucleotide polymorphisms structure human microbiome communities. BMC Genomics 15:257. doi: 10.1186/1471-2164-15-257
Man, A. W. C., Xia, N., Daiber, A., and Li, H. (2020). The roles of gut microbiota and circadian rhythm in the cardiovascular protective effects of polyphenols. Br. J. Pharmacol. 177, 1278–1293. doi: 10.1111/bph.14850
Matsumoto, T., Kojima, M., Takayanagi, K., Taguchi, K., and Kobayashi, T. (2020). Role of S-equol, indoxyl sulfate, and trimethylamine N-oxide on vascular function. Am. J. Hypertens. 33, 793–803. doi: 10.1093/ajh/hpaa053
Meyer, K. A., Benton, T. Z., Bennett, B. J., Jacobs, D. R., Lloyd-Jones, D. M., Gross, M. D., et al. (2016). Microbiota-dependent metabolite trimethylamine n-oxide and coronary artery calcium in the coronary artery risk development in young adults study (CARDIA). J. Am. Heart Assoc. 5:e003970. doi: 10.1161/JAHA.116.003970
Mills, S., Stanton, C., Lane, J. A., Smith, G. J., and Ross, R. P. (2019). Precision nutrition and the microbiome, part I: current state of the science. Nutrients 11:923. doi: 10.3390/nu11040923
Mohammadi, A., Najar, A. G., Yaghoobi, M. M., Jahani, Y., and Vahabzadeh, Z. (2016). Trimethylamine-N-oxide treatment induces changes in the ATP-binding cassette transporter A1 and scavenger receptor A1 in murine macrophage J774A.1 cells. Inflammation 39, 393–404.
Montrucchio, C., De Nicolò, A., D’Ettorre, G., D’Ascenzo, F., Lazzaro, A., Tettoni, M., et al. (2020). Serum trimethylamine-N-oxide concentrations in people living with HIV and the effect of probiotic supplementation. Int. J. Antimicrob. Agents 55:105908. doi: 10.1016/j.ijantimicag.2020.105908
Mottawea, W., Chiang, C. K., Mühlbauer, M., Starr, A. E., Butcher, J., Abujamel, T., et al. (2016). Altered intestinal microbiota-host mitochondria crosstalk in new onset Crohn’s disease. Nat. Commun. 7:13419. doi: 10.1038/ncomms13419
Nakaya, K., and Ikewaki, K. (2018). Microbiota and HDL metabolism. Curr. Opin. Lipidol. 29, 18–23. doi: 10.1097/MOL.0000000000000472
Nallu, A., Sharma, S., Ramezani, A., Muralidharan, J., and Raj, D. (2017). Gut microbiome in chronic kidney disease: challenges and opportunities. Transl. Res. 179, 24–37. doi: 10.1016/j.trsl.2016.04.007
Nielsen, T., Qin, J., Prifti, E., Hildebrand, F., Falony, G., Almeida, M., et al. (2013). Richness of human gut microbiome correlates with metabolic markers. Nature 500, 541–546. doi: 10.1038/nature12506
Nikiforov, N. G., Wetzker, R., Kubekina, M. V., Petukhova, A. V., Kirichenko, T. V., and Orekhov, A. N. (2019). Trained circulating monocytes in atherosclerosis: ex vivo model approach. Front. Pharmacol. 10:725. doi: 10.3389/fphar.2019.00725
Ohira, H., Tsutsui, W., and Fujioka, Y. (2017). Are short chain fatty acids in gut microbiota defensive players for inflammation and atherosclerosis? J. Atheroscler. Thromb. 24, 660–672. doi: 10.5551/jat.RV17006
Opazo, M. C., Ortega-Rocha, E. M., Coronado-Arrázola, I., Bonifaz, L. C., Boudin, H., Neunlist, M., et al. (2018). Intestinal microbiota influences non-intestinal related autoimmune diseases. Front. Microbiol. 9:432. doi: 10.3389/fmicb.2018.00432
Orekhov, A. N., and Ivanova, E. A. (2017). Introduction of the special issue atherosclerosis and related diseases. Vessel Plus 1, 163–165. doi: 10.20517/2574-1209.2017.33
Orekhov, A. N., Nikiforov, N. N., Ivanova, E. A., and Sobenin, I. A. (2020). Possible role of mitochondrial DNA mutations in chronification of inflammation: focus on atherosclerosis. J. Clin. Med. 9:E978. doi: 10.3390/jcm9040978
Ostan, R., Monti, D., Gueresi, P., Bussolotto, M., Franceschi, C., and Baggio, G. (2016). Gender, aging and longevity in humans: an update of an intriguing/neglected scenario paving the way to a gender-specific medicine. Clin. Sci. 130, 1711–1725. doi: 10.1042/CS20160004
Paschos, G. K., and FitzGerald, G. A. (2010). Circadian clocks and vascular function. Circ. Res. 106, 833–841. doi: 10.1161/CIRCRESAHA.109.211706
Pereira-Fantini, P. M., Byars, S. G., Pitt, J., Lapthorne, S., Fouhy, F., Cotter, P. D., et al. (2017). Unravelling the metabolic impact of SBS-associated microbial dysbiosis: insights from the piglet short bowel syndrome model. Sci. Rep. 7:43326. doi: 10.1038/srep43326
Pieczynska, M. D., Yang, Y., Petrykowski, S., Horbanczuk, O. K., Atanasov, A. G., and Horbanczuk, J. O. (2020). Gut microbiota and its metabolites in atherosclerosis development. Molecules 25:594. doi: 10.3390/molecules25030594
Poznyak, A. V., Wu, W.-K., Melnichenko, A. A., Wetzker, R., Sukhorukov, V., Markin, A. M., et al. (2020). Signaling pathways and key genes involved in regulation of foam cell formation in atherosclerosis. Cells 9:584. doi: 10.3390/cells9030584
Randrianarisoa, E., Lehn-Stefan, A., Wang, X., Hoene, M., Peter, A., Heinzmann, S. S., et al. (2016). Relationship of serum trimethylamine N-oxide (TMAO) levels with early atherosclerosis in humans. Sci. Rep. 6:26745. doi: 10.1038/srep26745
Saint-Georges-Chaumet, Y., and Edeas, M. (2016). Microbiota-mitochondria inter-talk: consequence for microbiota-host interaction. Pathog. Dis. 74:ftv096. doi: 10.1093/femspd/ftv096
Sayin, S. I., Wahlström, A., Felin, J., Jäntti, S., Marschall, H. U., Bamberg, K., et al. (2013). Gut microbiota regulates bile acid metabolism by reducing the levels of tauro-beta-muricholic acid, a naturally occurring FXR antagonist. Cell Metab. 17, 225–235. doi: 10.1016/j.cmet.2013.01.003
Sazonova, M. A., Sinyov, V. V., Barinova, V. A., Ryzhkova, A. I., Zhelankin, A. V., Postnov, A. Y., et al. (2015). Mosaicism of mitochondrial genetic variation in atherosclerotic lesions of the human aorta. Biomed Res. Int. 2015:825468. doi: 10.1155/2015/825468
Sazonova, M. A., Sinyov, V. V., Ryzhkova, A. I., Galitsyna, E. V., Khasanova, Z. B., Postnov, A. Y., et al. (2017). Role of mitochondrial genome mutations in pathogenesis of carotid atherosclerosis. Oxid. Med. Cell. Longev. 2017:6934394. doi: 10.1155/2017/6934394
Schoeler, M., and Caesar, R. (2019). Dietary lipids, gut microbiota and lipid metabolism. Rev. Endocr. Metab. Disord. 20, 461–472.
Seldin, M. M., Meng, Y., Qi, H., Zhu, W. F., Wang, Z., Hazen, S. L., et al. (2016). Trimethylamine N-oxide promotes vascular inflammation through signaling of mitogen-activated protein kinase and nuclear factor-κb. J. Am. Heart Assoc. 5:e002767. doi: 10.1161/JAHA.115.002767
Senthong, V., Li, X. S., Hudec, T., Coughlin, J., Wu, Y., Levison, B., et al. (2016a). Plasma trimethylamine N-oxide, a gut microbe–generated phosphatidylcholine metabolite, is associated with atherosclerotic burden. J. Am. Coll. Cardiol. 67, 2620–2628. doi: 10.1016/j.jacc.2016.03.546
Senthong, V., Wang, Z., Li, X. S., Fan, Y., Wu, Y., Tang, W. H. W., et al. (2016b). Intestinal microbiota-generated metabolite Trimethylamine-N-oxide and 5-year mortality risk in stable coronary artery disease: the contributory role of intestinal microbiota in a COURAGE-like patient cohort. J. Am. Heart Assoc. 5:e002816. doi: 10.1161/JAHA.115.002816
Skagen, K., Trøseid, M., Ueland, T., Holm, S., Abbas, A., Gregersen, I., et al. (2016). The carnitine-butyrobetaine-trimethylamine-N-oxide pathway and its association with cardiovascular mortality in patients with carotid atherosclerosis. Atherosclerosis 247, 64–69. doi: 10.1016/j.atherosclerosis.2016.01.033
Sobenin, I. A., Zhelankin, A. V., Khasanova, Z. B., Sinyov, V. V., Medvedeva, L. V., Sagaidak, M. O., et al. (2019). Heteroplasmic variants of mitochondrial DNA in atherosclerotic lesions of human aortic intima. Biomolecules 9:455. doi: 10.3390/biom9090455
Sommer, F., and Bäckhed, F. (2013). The gut microbiota-masters of host development and physiology. Nat. Rev. Microbiol. 11, 227–238. doi: 10.1038/nrmicro2974
Sonnenburg, J. L., and Bäckhed, F. (2016). Diet–microbiota interactions as moderators of human metabolism. Nature 535, 56–64. doi: 10.1038/nature18846
Sun, X., Jiao, X., Ma, Y., Liu, Y., Zhang, L., He, Y., et al. (2016). Trimethylamine N-oxide induces inflammation and endothelial dysfunction in human umbilical vein endothelial cells via activating ROS-TXNIP-NLRP3 inflammasome. Biochem. Biophys. Res. Commun. 481, 63–70.
Tang, W. H. W., Wang, Z., Levison, B. S., Koeth, R. A., Britt, E. B., Fu, X., et al. (2013). Intestinal microbial metabolism of phosphatidylcholine and cardiovascular risk. N. Engl. J. Med. 368, 1575–1584. doi: 10.1056/NEJMoa1109400
Toral, M., Gómez-Guzmán, M., Jiménez, R., Romero, M., Sánchez, M., Utrilla, M. P., et al. (2014). The probiotic Lactobacillus coryniformis CECT5711 reduces the vascular pro-oxidant and pro-inflammatory status in obese mice. Clin. Sci. 127, 33–45. doi: 10.1042/CS20130339
Tumur, Z., Shimizu, H., Enomoto, A., Miyazaki, H., and Niwa, T. (2010). Indoxyl sulfate upregulates expression of ICAM-1 and MCP-1 by oxidative stress-induced NF-κB activation. Am. J. Nephrol. 31, 435–441. doi: 10.1159/000299798
Tuttolomondo, A., Di Raimondo, D., Pecoraro, R., Arnao, V., Pinto, A., and Licata, G. (2012). Atherosclerosis as an inflammatory disease. Curr. Pharm. Des. 18, 4266–4288. doi: 10.2174/138161212802481237
van den Munckhof, I. C. L., Kurilshikov, A., ter Horst, R., Riksen, N. P., Joosten, L. A. B., Zhernakova, A., et al. (2018). Role of gut microbiota in chronic low-grade inflammation as potential driver for atherosclerotic cardiovascular disease: a systematic review of human studies. Obes. Rev. 19, 1719–1734. doi: 10.1111/obr.12750
Villéger, R., Lopès, A., Carrier, G., Veziant, J., Billard, E., Barnich, N., et al. (2019). Intestinal microbiota: a novel target to improve anti-tumor treatment? Int. J. Mol. Sci. 20:4584. doi: 10.3390/ijms20184584
Wang, Z., Klipfell, E., Bennett, B. J., Koeth, R., Levison, B. S., Dugar, B., et al. (2011). Gut flora metabolism of phosphatidylcholine promotes cardiovascular disease. Nature 472, 57–65. doi: 10.1038/nature09922
Winter, C., and Soehnlein, O. (2018). The potential of chronopharmacology for treatment of atherosclerosis. Curr. Opin. Lipidol. 29, 368–374. doi: 10.1097/MOL.0000000000000545
Wu, X., Chen, L., Zeb, F., Huang, Y., An, J., Ren, J., et al. (2019a). Regulation of circadian rhythms by NEAT1 mediated TMAO-induced endothelial proliferation: a protective role of asparagus extract. Exp. Cell Res. 382:111451. doi: 10.1016/j.yexcr.2019.05.032
Wu, X., Chen, L., Zeb, F., Li, C., Jiang, P., Chen, A., et al. (2019b). Clock-Bmal1 mediates MMP9 induction in acrolein-promoted atherosclerosis associated with gut microbiota regulation. Environ. Pollut. 252, 1455–1463. doi: 10.1016/j.envpol.2019.06.042
Xie, M., Tang, Q., Nie, J., Zhang, C., Zhou, X., Yu, S., et al. (2020). BMAL1-downregulation aggravates porphyromonas gingivalis-induced atherosclerosis by encouraging oxidative stress. Circ. Res. 126, e15–e29. doi: 10.1161/CIRCRESAHA.119.315502
Yardeni, T., Tanes, C. E., Bittinger, K., Mattei, L. M., Schaefer, P. M., Singh, L. N., et al. (2019). Host mitochondria influence gut microbiome diversity: a role for ROS. Sci. Signal. 12:eaaw3159. doi: 10.1126/scisignal.aaw3159
Zeng, C., and Tan, H. (2020). Gut microbiota and heart, vascular injury. Adv. Exp. Med. Biol. 1238, 107–141. doi: 10.1007/978-981-15-2385-4_8
Zhu, W., Gregory, J. C., Org, E., Buffa, J. A., Gupta, N., Wang, Z., et al. (2016). Gut microbial metabolite TMAO enhances platelet hyperreactivity and thrombosis risk. Cell 165, 111–124. doi: 10.1016/j.cell.2016.02.011
Keywords: microbiome, atherosclerosis, inflammation, TMAO, mitochondria, lipoproteins
Citation: Kirichenko TV, Markina YV, Sukhorukov VN, Khotina VA, Wu W-K and Orekhov AN (2020) A Novel Insight at Atherogenesis: The Role of Microbiome. Front. Cell Dev. Biol. 8:586189. doi: 10.3389/fcell.2020.586189
Received: 22 July 2020; Accepted: 31 August 2020;
Published: 22 September 2020.
Edited by:
Yongguo Cao, Jilin University, ChinaReviewed by:
Soon Yew Tang, University of Pennsylvania, United StatesCopyright © 2020 Kirichenko, Markina, Sukhorukov, Khotina, Wu and Orekhov. This is an open-access article distributed under the terms of the Creative Commons Attribution License (CC BY). The use, distribution or reproduction in other forums is permitted, provided the original author(s) and the copyright owner(s) are credited and that the original publication in this journal is cited, in accordance with accepted academic practice. No use, distribution or reproduction is permitted which does not comply with these terms.
*Correspondence: Tatiana V. Kirichenko, dC1nb3JjaGFrb3ZhQG1haWwucnU=
Disclaimer: All claims expressed in this article are solely those of the authors and do not necessarily represent those of their affiliated organizations, or those of the publisher, the editors and the reviewers. Any product that may be evaluated in this article or claim that may be made by its manufacturer is not guaranteed or endorsed by the publisher.
Research integrity at Frontiers
Learn more about the work of our research integrity team to safeguard the quality of each article we publish.