- 1Fertility Preservation Lab, Reproductive Medicine Center, Guangdong Second Provincial General Hospital, Guangzhou, China
- 2State Key Laboratory of Stem Cell and Reproductive Biology, Institute of Zoology, Chinese Academy of Sciences, Beijing, China
- 3Department of Veterinary Pathobiology, University of Missouri, Columbia, MO, United States
Mitochondrial energy insufficiency is strongly associated with oocyte activation disorders. Ca2+, especially that in the mitochondrial matrix, plays a pivotal role in mitochondrial energy supplementation, but the underlying mechanisms are still only poorly understood. An encoded mitochondrial matrix Ca2+ probe (Mt-GCaMP6s) was introduced to observe mitochondrial Ca2+ ([Ca2+]m) dynamic changes during oocyte maturation and activation. We found that active mitochondria surrounding the nucleus showed a higher [Ca2+]m than those distributed in the cortex during oocyte maturation. During oocyte partheno-activation, the patterns of Ca2+ dynamic changes were synchronous in the cytoplasm and mitochondria. Such higher concentration of mitochondrial matrix Ca2+ was closely related to the distribution of mitochondrial calcium uptake (MICU) protein. We further showed that higher [Ca2+]m mitochondria around the chromosomes in oocytes might have a potential role in stimulating mitochondrial energy for calmodulin-responsive oocyte spindle formation, while synchronizing Ca2+ functions in the cytoplasm and nuclear area are important for oocyte activation.
Introduction
According to the WHO 2016 report, at least one of 10 couples in developed countries cannot have children within 5 years of marriage, half of which are due to female infertility (Chow et al., 2016). Oocyte activation inefficiency is a major problem causing female infertility (Schatten et al., 2014). Oocyte activation rates in obese, diabetic and aging women are low, affecting the development of preimplantation embryos and also pregnancy (Minge et al., 2008; Igosheva et al., 2010; Jungheim et al., 2010; Shankar et al., 2011; Luzzo et al., 2012; Wang et al., 2017). Such disorders are strongly related with mitochondrial energy supply insufficiency (Gesink Law et al., 2007; Rittenberg et al., 2011). Resolving the low quality of oocytes caused by metabolic abnormalities and improving the efficiency of assisted reproduction are key issues that researchers need to address.
Ca2+ is one of the major signaling molecules regulating several cell functions, such as celldivision, arrest, and apoptosis. At fertilization, oocyte activation begins with a series of crucial steps triggered by periodical repetitive increases and decreases in intracellular Ca2+ ([Ca2+]i) concentrations known as [Ca2+]i oscillations (Stricker, 1999; Wakai et al., 2013). Mitochondrial function and intracellular [Ca2+]i are two critical factors for the oocyte’s developmental potential (Wacquier et al., 2019). Although [Ca2+]i oscillations are required for oocyte activation (Yazawa et al., 2007), very little is known about how cytoplasmic and mitochondrial Ca2+ regulates the energy supply mode transition during oocyte activation.
Mitochondrial Ca2+ is a key factor initiating mitochondrial metabolism and regulating mitochondrial activity. Mitochondrial matrix Ca2+ homeostasis is strictly regulated by the transmission of Ca2+ between the cytoplasm, ER and mitochondria (Dumollard et al., 2006), which plays important roles in cellular physiology. Mitochondrial Ca2+ regulates membrane potential, stimulates rate-limiting enzymes in the Krebs cycle, and accelerates ATP synthesis (Chacko and Eliceiri, 2018). Ca2+ oscillations triggered by sperm-oocyte fusion also affect fertilized oocyte mitochondria and promote the transition of mitochondria from the resting state to the activation state (Dumollard et al., 2006). Our previous results showed that mitochondrial activity plays a critically important role in maintaining [Ca2+]i oscillations (Wang et al., 2018). Although there are some reports on the mechanisms of Ca2+ and mitochondria interactions during cell differentiation (Mallilankaraman et al., 2012a,b), the relationship between [Ca2+]m and mitochondrial metabolism is unknown in oocytes.
In this study, we observed the dynamic [Ca2+]m changes during in vitro maturation and activation of oocytes, and we further studied the functions of matrix Ca2+ on mitochondrial activity, with the ultimate goal to improve oocyte quality by modifying the metabolism of mitochondria within the oocyte.
Materials and Methods
Ethics Statement
Female ICR mice were purchased from the Beijing Vital River Laboratory Animal Technology Co., Ltd. All mice were handled in accordance with the institutional animal care policies of the Institute of Zoology, Chinese Academy of Sciences. Mice were maintained under a 12 h light and 12 h darkness cycle in a specific pathogen-free stage at the Central Animal Laboratory of the Institute of Zoology. The Laboratory Animal Care and Use Committee of the Institute of Zoology approved this study.
Oocyte Collection and Partheno-Activation
Female mice were injected with 10 IU pregnant mare serum gonadotropin (PMSG, Ningbo Hormone Product Co., Ltd., Cixi, China). After 44–48 h, GV oocytes were collected by ovarian mincing. Female mice were injected with 10 IU PMSG followed 44–48 h later by injection of 10 IU human chorionic gonadotropin (hCG, Ningbo Hormone Product Co., Ltd., Cixi, China). After 15 h of hCG, ovulated MII oocytes were collected and denuded in 1 mg/mL hyaluronidase. Matured oocyte [Ca2+]i/[Ca2+]m oscillations were induced by 10 mM strontium chloride (SrCl2, 10025-70-4, Sangon Biotech, Shanghai, China) in Ca2+-free CZB for 4 h. After partheno-activation, eggs were transferred into KSOM (MR-106, Merck Millipore, MA, United States).
Plasmid Construction of Vectors
An encoded Ca2+-sensitive probe was constructed based on the cpGFP (Circularly Permuted Green Fluorescent Protein) system and calmodulin (GCaMP6s) to detect Ca2+ concentrations (Akerboom et al., 2012; Zhong and Schleifenbaum, 2019). The mitochondrial location probe plasmid was constructed to express the Ca2+ detection protein probe with mitochondrial localization signal peptide cloned from Trmt10c gene (Metodiev et al., 2016; Oerum et al., 2018). For cloning of genes encoding MICU protein, voltage-dependent anion selective channel (VDAC) and CaMs, The coding sequences (CDS) were obtained by RT-PCR with template of ovary cDNA cloned into the pMD-18-T vector (Takara, Dalian, Liaoning, China). 18-T vector were sliced and linked CDS to upstream of mCherry. Two plasmids, p-UC57-Mt-GCaMG6s (Supplementary Figure S1A) and p-GEMHE-MICU-mCherry (Supplementary Figure S1B), were dissolved in water and stored at −20°C.
In vitro Transcription of cRNA
Templates of in vitro transcription from constructed plasmids were obtained by PCR with primers M13F and M13R (Supplementary Figures S1C–F). PCR products were diluted in RNAse-free water. cRNA transcripts were synthesized in vitro with T7 RNA polymerase mMESSAGE mMACHINE T7 kit (Ambion, Life Co., Calsbad, CA, United States). Poly(A) tail was added to the sequence end by polymerase tailing kit (PAP5104, Lucigen, Beijing, China). The RNA solutions were then stored at −80°C in a final concentration of 400 μg/mL until further use. Approximately 50 pl of RNA solution was injected into each GV oocyte.
GV Oocyte Microinjection
The M2 medium and M16 medium containing 2.5 μM milrinone were prepared and warmed to 37°C. Milrinone is a phosphodiesterase inhibitor that maintains meiotic arrest once oocytes are removed from the follicles (Stein and Schindler, 2011). Micro-drops each containing 20 μl M16 medium with milrinone were prepared in a dish and overlaid with mineral oil. Injection pipettes were made by pulling borosilicate-glass capillary with filament in a mechanical puller. We used a Flaming-Brown micropipette puller (Model P-97) with the following settings: P = 540, Heat = 300, Pull = 130, Vel = 100, Time = 150. Microinjection platform was prepared by placing a 10 μl micro-drop of M2 medium with milrinone on a chamber slide, and the drop was covered with mineral oil and placed on the microscope stage. Injection and holding pipettes were placed into the drop of M2 medium with milrinone. Microinjection of cRNA was performed with Narishige micromanipulators (Narishige Inc., Sea Cliff, NY) under a Nikon TE 200 (Nikon UK Ltd., Kingston upon Thames, Surrey, United Kingdom) and finished within 30 min. After cRNA injection, oocytes were arrested at the GV stage in M16 medium containing 2.5 μM milrinone for 4 h. Then the oocytes were transferred to M16 medium and cultured under mineral oil at 37°, in an atmosphere of 5% CO2 in air for 14 h in vitro maturation.
Real-Time Recording of [Ca2+]i and [Ca2+]m Changes
We set out to confirm [Ca2+]i oscillations regulation on mitochondrial activity during oocyte activation. [Ca2+]i oscillations were observed by staining with 2μM Fluo4 AM (488 nm excitation, 525 nm emission) in the partheno-activation system. Rhod2 is a classical dye for determining mitochondrial Ca2+ concentration ([Ca2+]m) with a higher Ca2+ affinity (Garcia-Perez et al., 2008; Eisner et al., 2010). We recorded [Ca2+]m dynamic changes with 2 μM Rhod2 AM (561 nm excitation, 590 nm emission) in a partheno-activation system. Mt-GCaMP6s was introduced to obtain the patterns of mitochondrial matrix Ca2+ dynamic changes. Real-time images were obtained using a time-lapse confocal laser microscope (UltraVIEW-VoX; PerkinElmer, MA, United States) and recorded at 2 frames per minute. [Ca2+]i and [Ca2+]m intensities were detected using an argon laser.
Real-Time Recording of Distribution of Activated Mitochondria
Activated mitochondria were imaged with 0.1 μM MitoRed (C2005, Beyotime, China), a cell permeable potential-sensitive fluorescent mitochondrial dye emitting in red (561 nm excitation, 590 nm emission) channel, which has a high affinity with higher potential mitochondria (Chazotte, 2011), by using a time-lapse confocal laser microscope (UltraVIEW-VoX; PerkinElmer, United States). The GV oocyte maturation was conducted by incubation in M16 (M7167, Sigma-Aldrich, United States) with 0.1 μM MitoRed. MII oocytes were activated in Ca2+-free-CZB with SrCl2, staining with 0.1 μM MitoRed. A software Volocity was used to analyze fluorescence intensity.
Statistical Analysis
All experiments were conducted at least three times. No fewer than 40 oocytes were collected and examined in each experiment and no fewer than three biological repeats for one group were conducted. We presented information of samples with Means and Standard Deviations (SD). Results were analyzed by SPSS 19.0. The significance of differences among groups was analyzed by the Chi-square test; p < 0.05 were considered statistically significant.
Results
Dynamic Changes in Mitochondrial Matrix Ca2+ During Partheno-Activation of Oocytes
In order to compare cytoplasmic and mitochondrial Ca2+ dynamic changes during oocyte partheno-activation, three dyes were employed to observe the Ca2+ concentration patterns in PMSG/HCG primed ovulated MII oocytes. Firstly, we detected Ca2+ dynamic changes in the cytoplasm ([Ca2+]i) and mitochondria ([Ca2+]m) by co-staining with Fluo4 AM (Figure 1A arrow) and Rhod2 AM (Figure 1A arrowhead). Oocyte [Ca2+]i oscillations were detected using Fluo4 AM in the partheno-activation system, while Rhod2 AM was reported as a classic dye for determining [Ca2+]m in somatic cells (Garcia-Perez et al., 2008; Eisner et al., 2010). Rhod2 signal showed the same pattern as [Ca2+]i oscillations (Figure 1B). Ca2+ concentrations in the cytoplasm and mitochondria changed synchronously during partheno-activation. We suggest that patterns of mitochondrial Ca2+ during oocyte activation may be the same as that of [Ca2+]i oscillations.
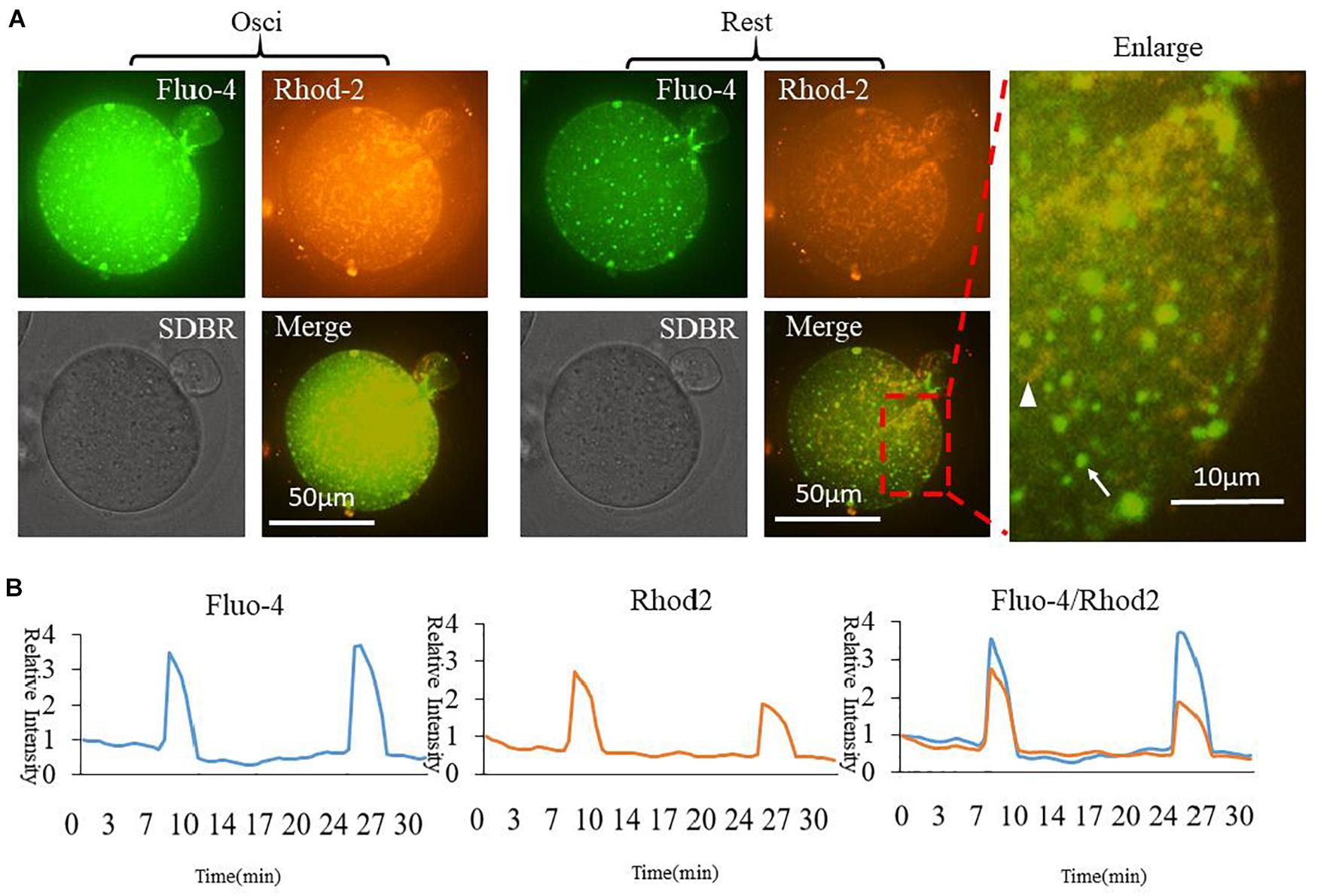
Figure 1. Fluo4 and Rhod2 dynamic changes during oocyte partheno-activation. (A) Fluo4AM and Rhod2AM staining of oscillating (Osci) and resting oocytes during activation. In the enlarged panel, arrow indicates Fluo4 staining of cytoplasmic Ca2+, and arrowhead indicates Rhod2 staining of mitochondrial Ca2+. The two dyes display clear different locations. (B) Relative fluorescence intensity of two continuous [Ca2+]i and [Ca2+]m oscillations. Ordinate is marked as Relative Intensity (relative to the fluorescence intensity of the start point).
In order to further confirm the changes of [Ca2+]m during oocyte activation, we applied in vitro transcription and microinjection (Stein and Schindler, 2011)of cRNA encoding a cpGFP (circularly permuted green fluorescent protein) based-Ca2+ probe with a mitochondrial signal peptide (Mt-GCaMP6s) (Supplementary Figure S1A; Akerboom et al., 2012) to determine [Ca2+]m patterns. Indeed, most of the mitochondria could be stained by Rhod2 while only some energized mitochondria were stained by GCaMP6s (Figure 2). In comparison, GCaMP6s is more suitable for detecting the dynamic changes of [Ca2+]m in oocytes. Finally regardless of which dye was used, [Ca2+]i and [Ca2+]m oscillations had the same pattern during oocyte activation. Ca2+ changes in the cytoplasm and mitochondria were synchronous.
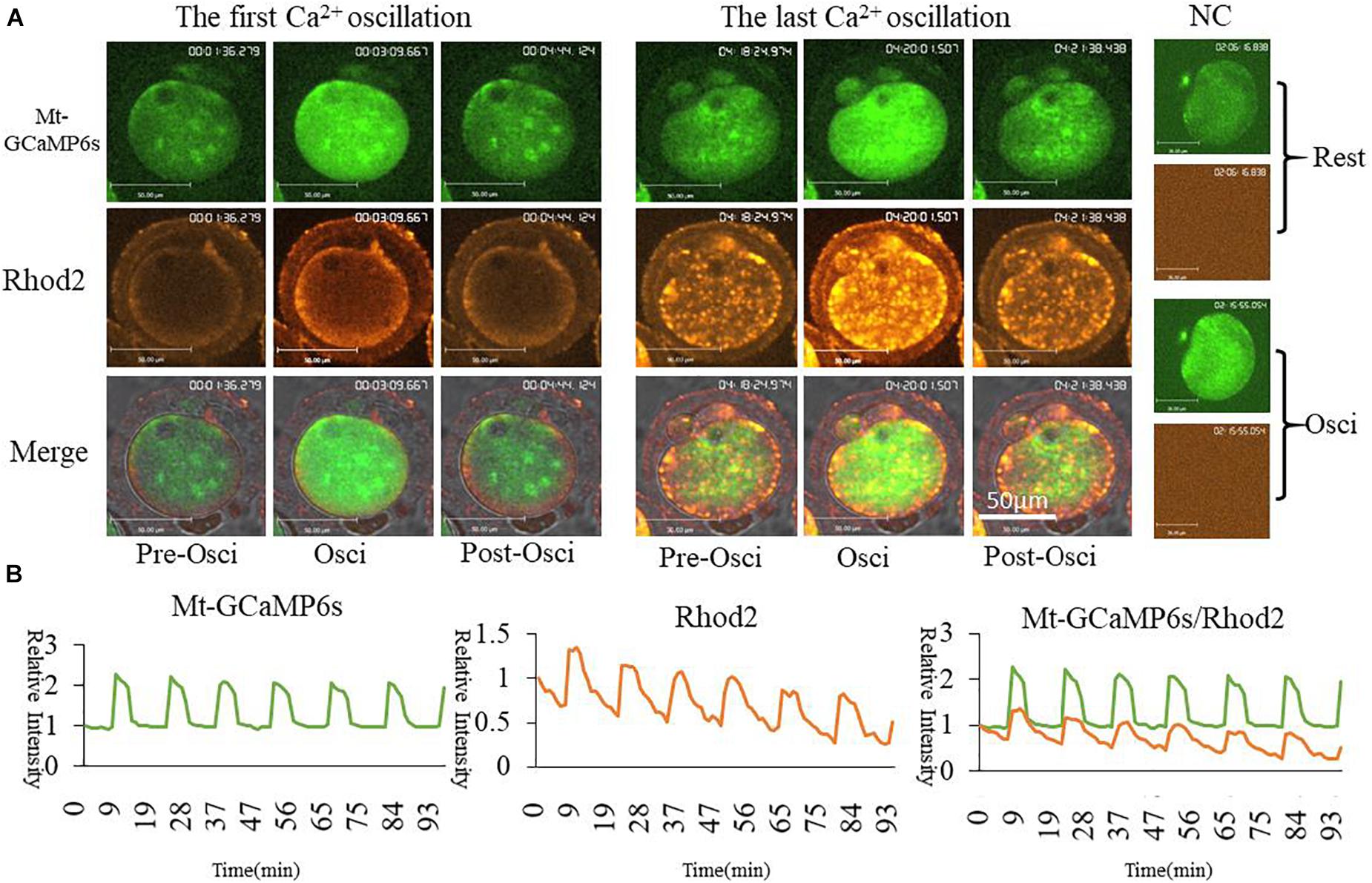
Figure 2. Mt-GCaMP6s and Rhod2 dynamic changes during oocyte partheno-activation. (A) First and last [Ca2+]m oscillations in partheno-activated oocytes. (B) Relative fluorescence intensity of [Ca2+]m oscillations as revealed by Mt-GCaMP6s and Rhod2. Patterns of Mt-GCaMP6s and Rhod2 are synchronous. Ordinate is marked as Relative Intensity (relative to the fluorescence intensity of the start point).
Dynamic Changes in [Ca2+]m During Oocyte Meiotic Maturation
We selected Mt-GCaMP6s to observe the dynamic pattern of [Ca2+]m changes in mitochondria during GV oocyte in vitro maturation (Figure 3 and Supplementary Video S1). Mitochondrial [Ca2+]m was located to the surrounding area of the germinal vesicle, which was clearly higher than that located at the oocyte cortex. After GVBD, higher [Ca2+]m mitochondria were observed to distribute around the spindle. Mitochondrial metabolism is much stronger in the energy-demanding part of the oocyte. Interestingly, there were two increasing [Ca2+]m spikes during oocyte maturation (Figure 3B). The first [Ca2+]m spike was observed at 9 h, before the first polar body extrusion, in about half of the GV oocytes examined (46.3 ± 8.3%). 1st polar body non-extruded oocytes did not show any Ca2+ spike. The second spike occurred 4–5 h after the first polar body emission, i.e., at about 14 h of culture in 33.2 ± 6.2% of oocytes which exhibited the first spike (Figure 3C). This coincided with the suitable window when the oocytes are ready for activation. These two time points were likely most critical for energy requirements.
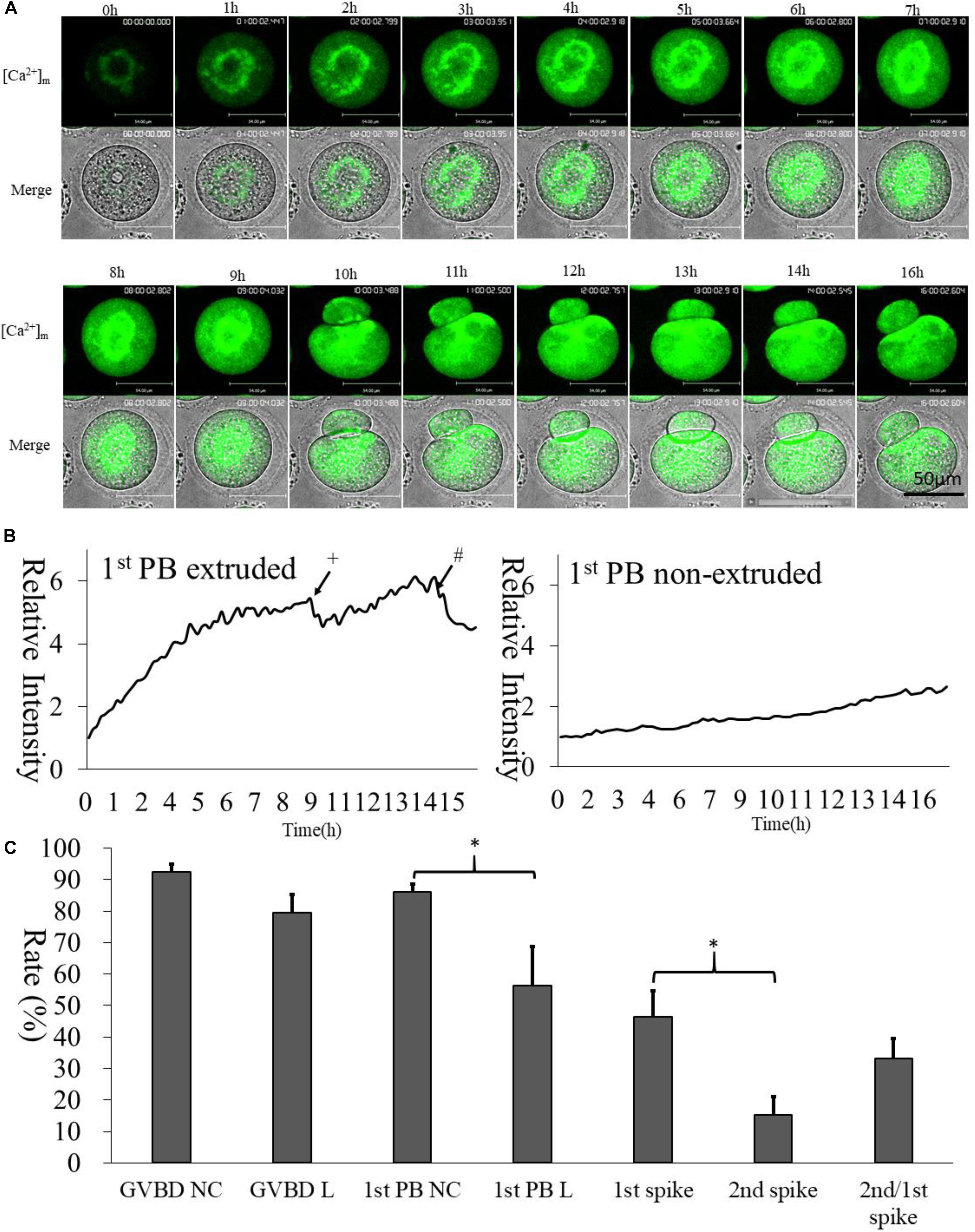
Figure 3. [Ca2+]m dynamic changes during oocyte maturation. (A) Mt-GCaMP6s staining of GV oocyte maturation. (B) Fluorescence intensity of [Ca2+]m changes in mitochondria during maturation. The left curve indicates 1st polar body-extruded oocytes, and the right curve indicates GVBD but 1st poly body- non-extruded oocytes, which lacks [Ca2+]m dynamic changes as in vitro matured MII oocytes. +The first polar body extrusion. #The second [Ca2+]m spike in MII oocyte. Ordinate is marked as Relative Intensity (relative to the fluorescence intensity of the start point). (C) Developmental rate of oocytes. “NC” indicates cRNA injected control oocytes cultured in 5% CO2 incubator. “L” indicated long-term laser irradiation in living cell station. *Indicates significance of differences (p < 0.05).
Higher [Ca2+]m and Activated Mitochondrial Distribution in Oocytes
Mt-GCaMP6s was further applied to observe the dynamic relationship between [Ca2+]m and activated mitochondrial distribution during oocyte maturation (Figure 4) and during a 4-h short term observation (Supplementary Figure S2). MitoRed-stained activated mitochondria surrounded the chromosomes and spindle with a higher [Ca2+]m. Next, we employed a partheno-activation system (Ca2+-free CZB with SrCl2) to observe the changes of [Ca2+]m and activated mitochondria distribution during oocyte activation (Figure 5). We found that there were two types of [Ca2+]m distributions of different mitochondria. In one part staining was maintained for a long time. The higher [Ca2+]m co-localized with the MitoRed positive mitochondria. [Ca2+]m in the other part of mitochondria changed as [Ca2+]i oscillation patterns rapidly increased and decreased. We found that during activation, [Ca2+]i oscillations in oocytes will drive Ca2+ changes in the mitochondria, but it does not affect these MitoRed positive mitochondria.
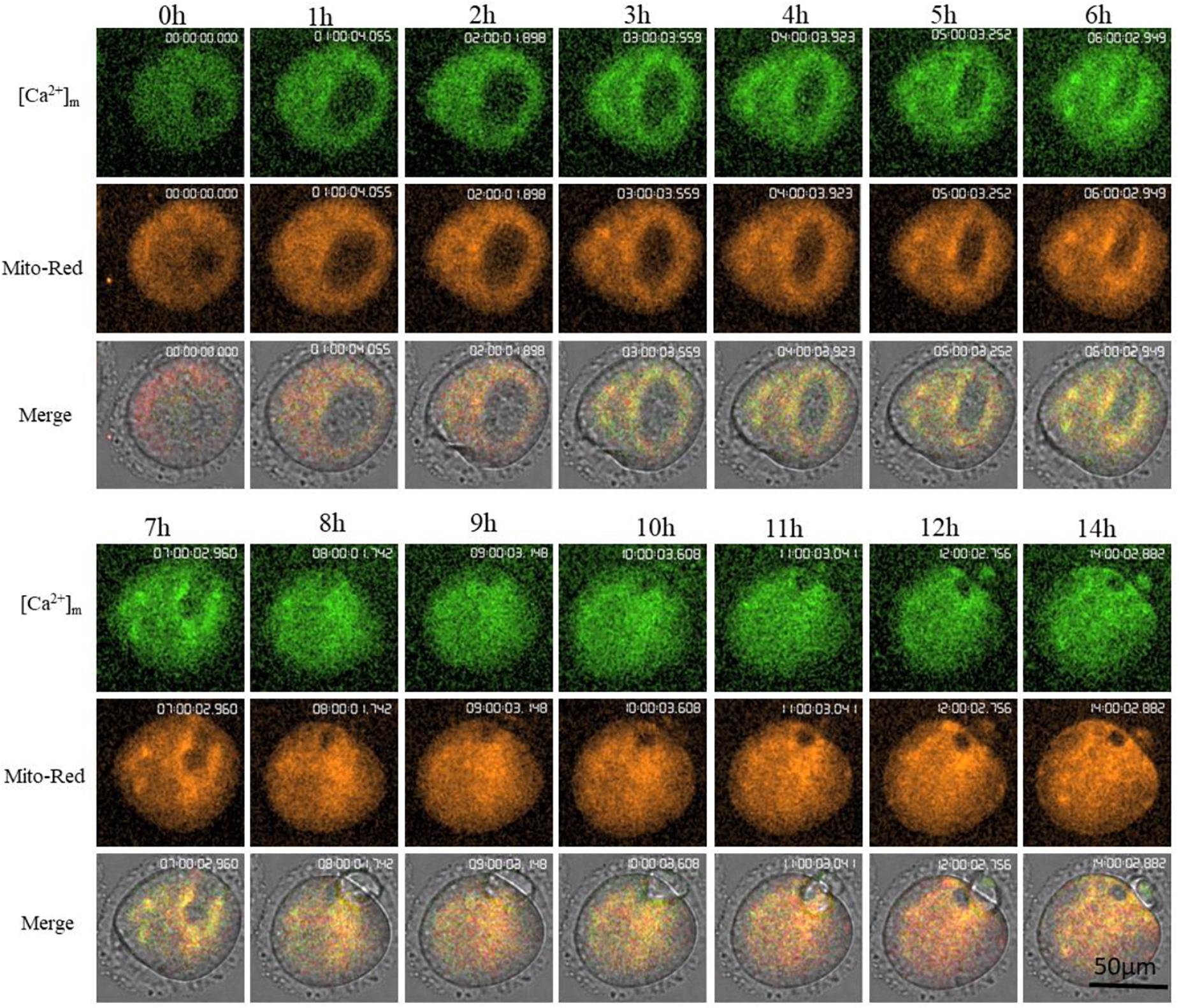
Figure 4. Higher [Ca2+]m and activated mitochondria distribution during oocyte maturation. [Ca2+]m indicates Mt-GCaMP6s staining of mitochondria. Mito-Red indicates activated mitochondria. [Ca2+]m and Mito-Red shows co-localization.
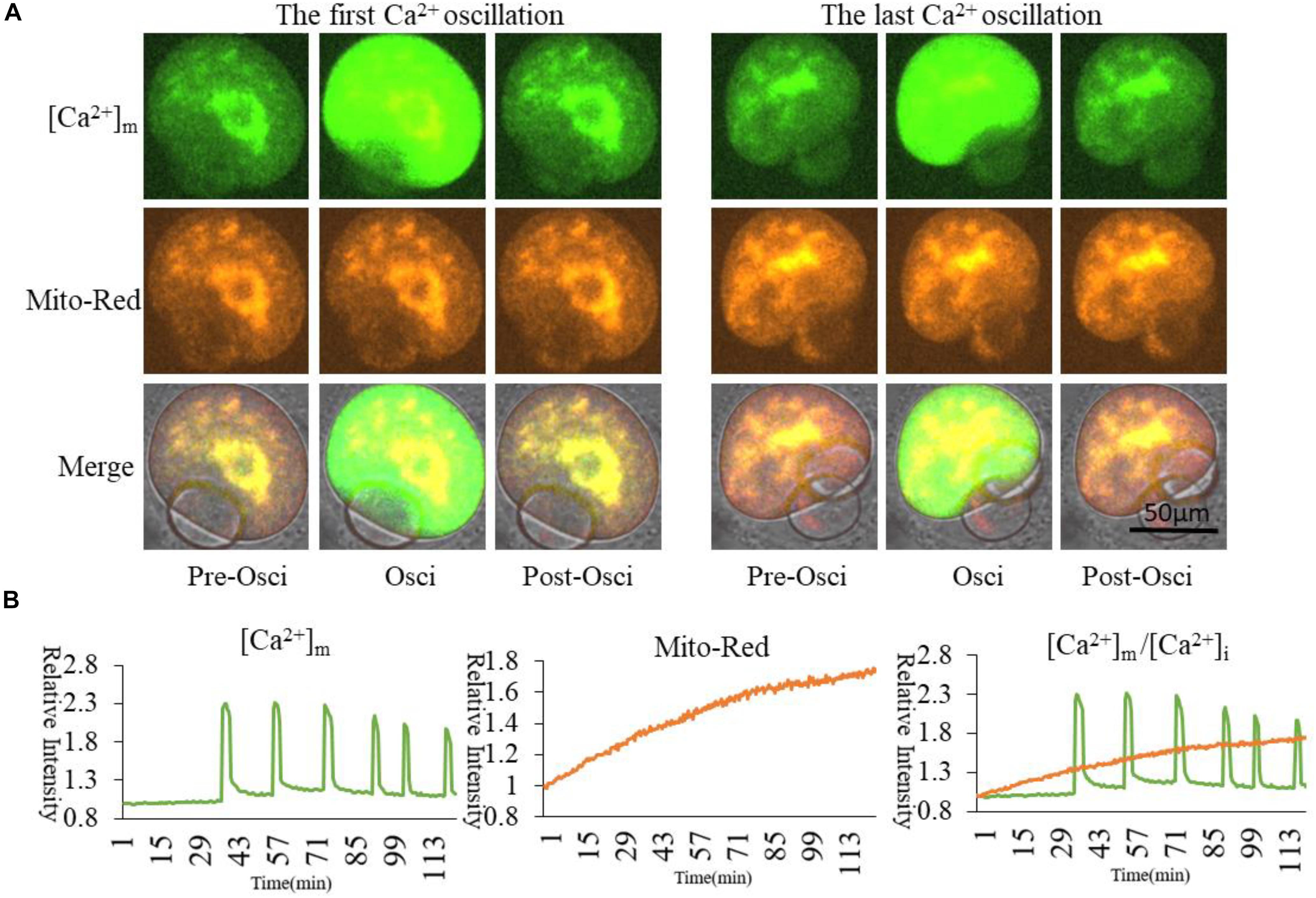
Figure 5. [Ca2+]m and activated mitochondria distribution during oocyte partheno-activation. [Ca2+]m indicates Mt-GCaMP6s staining of mitochondria. (A) [Ca2+]m and activated mitochondria distribution at first and last Ca2+ oscillations. Mito-Red indicates activated mitochondria. One part of higher [Ca2+]m and Mito-Red shows an evident co-localization that is maintained for a long time in mitochondria surrounding chromosomes. [Ca2+]m in most cortical mitochondria changed with [Ca2+]i oscillations. (B) Fluorescence intensity of [Ca2+]m and Mito-Red changes during oocyte maturation and activation. Ordinate is marked as Relative Fluorescence Intensity (relative to the fluorescence intensity of the start point).
Higher [Ca2+]m Related to the Distribution of MICU
Mitochondrial calcium uniporter (MCU) has multiple subunits, and its Ca2+ influx activity is controlled by regulatory subunits, including mitochondrial calcium uptakers (MICU) (Xing et al., 2019). In vitro transcription vector of MICU was constructed to confirm the relationship between [Ca2+]m and MICU. MICU was found to co-distribute with higher [Ca2+]m mitochondria in the GV oocytes (Figure 6). In PA oocytes, such distribution was similar as that of activated mitochondria and higher [Ca2+]m mitochondria. During activation, [Ca2+]i oscillations will drive Ca2+ changes in the mitochondria, but it did not affect these Mito-Red positive mitochondria. MICU distribution in oocytes was similar to Mito-Red positive activated mitochondria. This may indicate that MICU is related to mitochondrial matrix Ca2+ and mitochondrial activity.
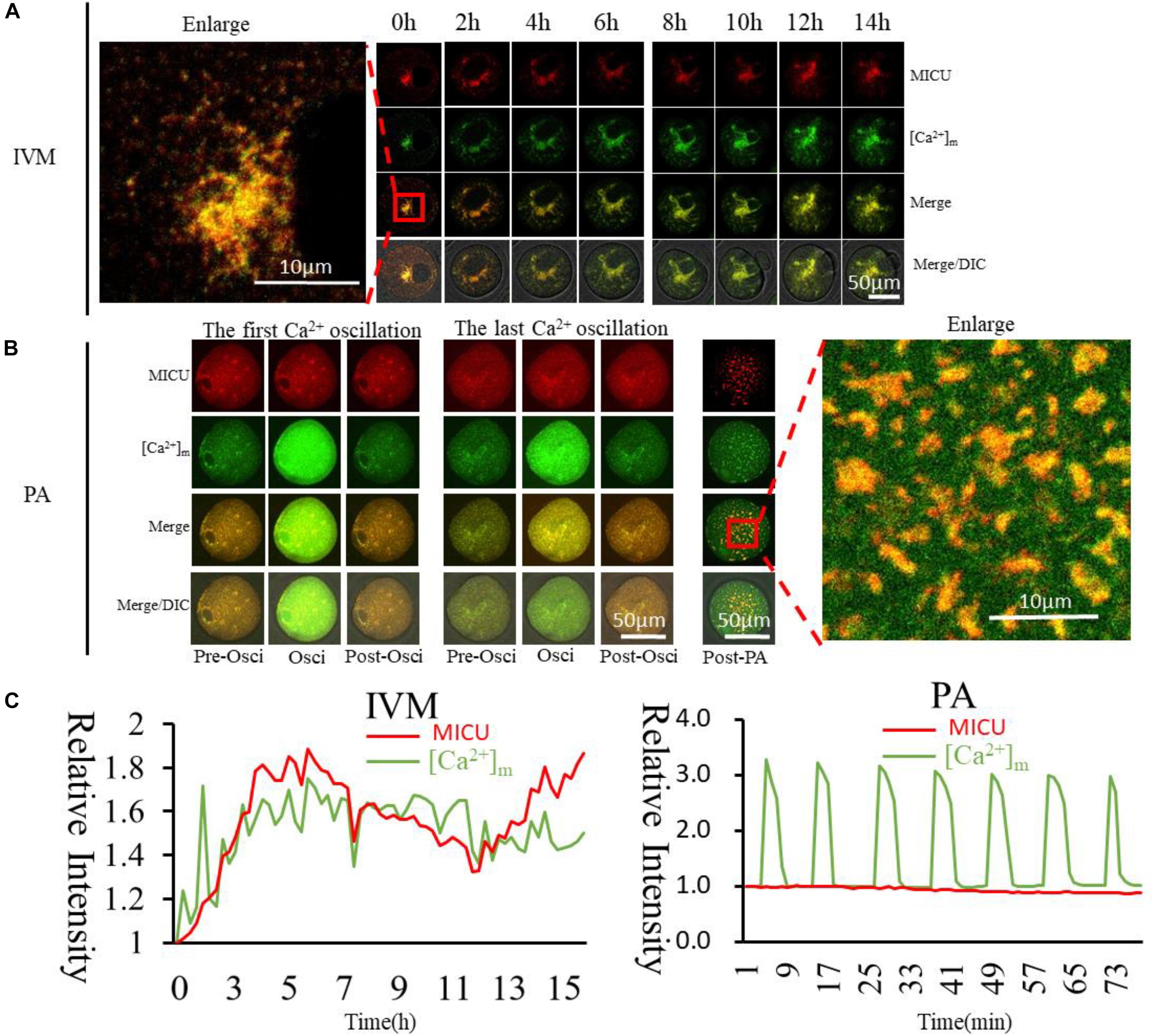
Figure 6. Dynamic distribution of MICU and higher [Ca2+]m mitochondria during oocyte maturation and activation. (A,B) MICU and higher [Ca2+]m mitochondria distribution during oocyte maturation and activation. IVM, In vitro Maturation; PA, Parthenogenetic Activation. (C) Fluorescence intensity of MICU (Red) and [Ca2+]m (Green)changes during maturation and activation. Ordinate is marked as Relative Fluorescence Intensity (relative to the fluorescence intensity of the start point).
VDACs (voltage-dependent anion channels) are a widespread on the outer mitochondrial membranes of most organisms (De Pinto et al., 2003). The OMM functions as a link between mitochondrial metabolism and the rest of the cell (Cassara et al., 2009). Some other reports also demonstrated the presence of VDACs in the plasma membrane and other organelles (Bathori et al., 2000; Shoshan-Barmatz et al., 2006). In vitro transcription of VDAC was constructed to observe the distribution of VDAC in mouse oocytes and to confirm the relationship between [Ca2+]m and VDAC. The distribution of [Ca2+]m and VDAC were obviously different. VDAC distributed in most parts of oocytes (Supplementary Figure S3).
Calmodulin and Higher [Ca2+]m Mitochondria Distribution in Oocytes
Calmodulins (CaMs), including two members termed CaM1 and CaM2, are thought to be the initial responding proteins in the cell to sense dynamic changes in Ca2+ concentration. CaMs are expected to distribute in oocytes around the most sensitive organelles for Ca2+. Firstly, we cloned the CaM1 and CaM2 cDNA sequences and linked them with mCherry as pGEMHE-CaM1-mCherry and pGEMHE-CaM2-mCherry plasmids (Supplementary Figure S1); then we observed the localization of CaMs during oocyte maturation. Both CaM1 and CaM2 are located at the spindle (Supplementary Figure S4), which indicates that CaM1 and CaM2 have similar locations and functions in spindle formation as previously reported (Xu et al., 1996; Mehta et al., 2014).
Since CaM1 and CaM2 have similar distributions, we wanted to confirm the relationship between [Ca2+]m and CaM2. Higher [Ca2+]m mitochondria surrounded the spindle, while CaM2 was localized on the spindle (Figure 7). We conclude that CaM2 is more directly involved in spindle formation, while [Ca2+]m regulates mitochondrial activity for energy supplementation. [Ca2+]i oscillations triggering cytoplasmic Ca2+ increase during oocyte activation will synchronize mitochondrial energy supplementation, spindle formation and maintenance as well as other cellular events during oocyte activation.
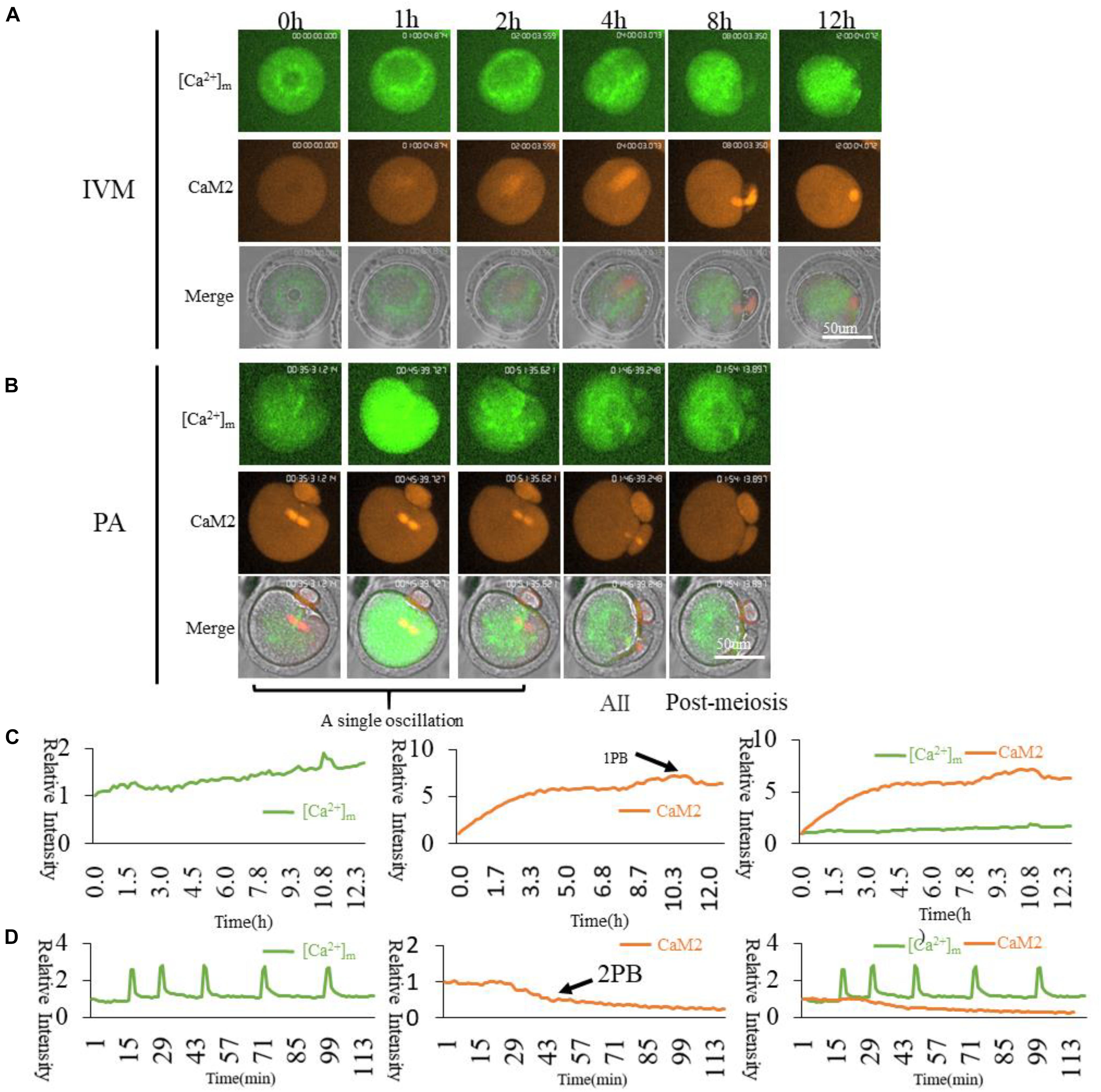
Figure 7. CaM2 and higher [Ca2+]m mitochondria dynamic distribution during oocyte maturation and activation. (A,B) CaM2 and higher [Ca2+]m mitochondria distribution during oocyte maturation and activation. IVM, In vitro Maturation; PA, Parthenogenetic Activation. (C,D) Fluorescence intensity of CaM2 (Red) and [Ca2+]m (Green) changes during maturation and activation. Ordinate is marked as Relative Fluorescence Intensity (relative to the fluorescence intensity of the start point).
Discussion
Abnormal metabolism such as hypertension, hyperglycemia and hyperlipidemia will cause long-term stress in the oocyte’s endoplasmic reticulum and mitochondria (Wang et al., 2019), which severely damages the quality of oocytes, thereby leading to lower pregnancy rates in women (Jungheim et al., 2013a). Increased Ca2+ signaling plays an important role in oocyte activation, which is a prerequisite for embryonic development (Dupont et al., 2010). Ca2+ signaling to specific organelles, such as the mitochondrial matrix for oxidative metabolism (Griffiths and Rutter, 2009), regulates organelle-specific cell functions (Marchant et al., 2002). Mitochondrial damage in oocytes reduces a woman’s reproductive capacity (Jungheim et al., 2013b), caused by inefficient oocyte activation (Ou and Sun, 2017).
Mitochondrial Ca2+ uptake has been studied for more than five decades (Rottenberg and Scarpa, 1974). Mitochondrial matrix calcium ([Ca2+]m) dynamics plays vital roles in regulating fundamental cellular and organelle functions including bioenergetics (Van Blerkom et al., 1995; Dumollard et al., 2007; Fink et al., 2017). The cytosolic Ca2+ influx to mitochondria (Viola et al., 2009) increases [Ca2+]m to stimulate important enzymes in the Krebs cycle (Rizzuto et al., 2012), which provides enough ATP to support the increased energy requirement for maintaining mammalian oocyte activation (Chacko and Eliceiri, 2018). A large amount of Ca2+ is accumulated in the mitochondrial matrix to promote the transition of mitochondria from the resting state to the activated state (Dumollard et al., 2006), and changes in [Ca2+]m will modify the mitochondrial metabolism to a certain extent. It is known that mitochondrial Ca2+ uptake is the basis for providing the necessary link between ATP supply and demand during cardiomyocyte contraction (Hajnoczky et al., 1995). Disturbances in Ca2+ fluxes lead to reduced bioenergetics of the cell or cellular death (Naon and Scorrano, 2014; Mishra, 2016; Samanta et al., 2018). In contrast, severe heart disease, such as reperfusion injury, is caused by excessive intake of mitochondria to induce Ca2+ overload and increase ROS production, even leading to cell apoptosis (Gordo et al., 2002; Ozil et al., 2005; Bonora et al., 2017). To prevent this, elevated [Ca2+]m rapidly migrates from the mitochondria into the cytoplasm and ER (Hajnoczky et al., 1995; Berridge et al., 2000; Bootman et al., 2001) which is essential for cells to perform accurate functions. Our previous study showed that mitochondrial activity plays an important role in the maintenance of [Ca2+]i oscillations during oocyte activation (Wang et al., 2018); moreover, [Ca2+]i oscillations also activate mitochondrial ATP production during fertilization (Campbell and Swann, 2006). We focused on the interplay between mitochondrial metabolism and Ca2+ signaling during oocyte maturation and activation in this study.
Our current understanding of [Ca2+]m regulation in oocytes is still limited. In order to determine the relationship between [Ca2+]m and [Ca2+]i dynamics, we focused on whether the Ca2+ changes in the mitochondria are independent or accompanied by changes in cytoplasmic [Ca2+]i oscillations. Rhod2 is a dye used to detect mitochondrial Ca2+ (Dubouskaya et al., 2018). The localizations of Fluo-4 and Rhod2 in oocytes were significantly different. It was clearly seen that Fluo-4 was distributed in the cytoplasm (arrow in Figure 1A), while Rhod2 distribution was similar to that of the mitochondria (arrowhead in Figure 1A). From the fluorescence analysis results (Figure 1B), it can be seen that Fluo-4 AM and Rhod2 AM have the same patterns as oscillations. We thus have confirmed that Ca2+ in the cytoplasm and in the mitochondria change simultaneously.
Although Rhod2 is used as a classic Ca2+ probe in mitochondria (Dubouskaya et al., 2018), it is not widely used in oocytes. In order to ensure an accurate pattern of the mitochondrial Ca2+ dynamic change, synchronized with the cytoplasmic [Ca2+]i oscillations, we applied an encoded Ca2+ probe with N-terminal mitochondrial localization signal peptide (Mt-GCaMP6s) to detect the patterns of [Ca2+]m (Supplementary Figure S1A). After injection of Mt-GCaMP6s cRNA, [Ca2+]m still exhibited oscillation patterns synchronized with Rhod2. Because Rhod2 displayed exactly the same pattern as [Ca2+]i oscillations (Figure 1), [Ca2+]m exhibited a same pattern in synchrony with [Ca2+]i. We also found that there are some differences in the position of Rhod2 and Mt-GCaMP6s; Rhod2-stained mitochondria are increased in quantity compared to Mt-GCaMP6s positive mitochondria. Indeed, most mitochondria can be stained by Rhod2, while only some of them displaying much higher [Ca2+]m mitochondria will be stained by Mt-GCaMP6s (Figure 2). Comparative consideration of such two mitochondrial Ca2+ dyes, Mt-GCaMP6s may be more suitable to distinguish the changes of Ca2+ concentration in mitochondria. Further studies are needed to better understand the difference between Rhod2 and Mt-GCaMP6s positive mitochondria.
Next, we wanted to detect the dynamic changes of [Ca2+]m during GV oocyte maturation. We found that higher [Ca2+]m mitochondria distributed around the germinal vesicle (Figure 3 and Supplementary Video S1). After GVBD, [Ca2+]m was higher in the area surrounding the spindle. During emission of the first polar body, [Ca2+]m in the equatorial plate area increased significantly. Mitochondrial metabolism is much stronger in the energy-demanding part of the oocyte. Interestingly, there were two spikes of [Ca2+]m around the time of first polar body extrusion (Figure 3B). Right curve indicates “GVBD but 1st poly body- non-extruded oocytes” displayed that maturation failed oocytes after GVBD, which lacks a [Ca2+]m dynamic changes pattern as that matured MII oocytes. The irradiation of the laser caused failure of the first polar body extrusion in some oocytes (Figure 3C). The 2nd spike only displayed in second meiotic oocytes which had extruded 1st polar body. The highest [Ca2+]m was found in MII oocytes in preparation for activation. These two time points are likely the most energy requiring critical periods for oocytes.
Is this dynamic change in mitochondrial [Ca2+]m related to activated mitochondria? To address this question the relationship between [Ca2+]m and the distribution of active mitochondria was studied. MitoRed is a dye localized to activated mitochondria (Chazotte, 2011). During GV oocyte in vitro maturation, we observed [Ca2+]m by Mt-GCaMP6s and activated mitochondria by MitoRed (Figure 4), respectively. Mito-Red may have cytotoxicity for long-term observations, so that we could not obtain a clear imaging as Mt-GCaMP6s single signal (488 nm excitation) during oocyte maturation (Figure 3A). In order to obtain a clear location information of [Ca2+]m and activated mitochondria, we performed a short 4-h observation with double concentration of MitoRed (Supplementary Figure S2). Double concentration of Mito-Red led to overexposure and cytotoxic effects after more than 4 h of observation. Higher [Ca2+]m and activated mitochondria showed a much clearer co-localization than during long term observation. If higher [Ca2+]m was co-localized with activated mitochondria, Ca2+ dynamic entry into mitochondria may participate in mitochondrial metabolism transfer from the resting to the activated state. There was a clear co-localization between higher [Ca2+]m and MitoRed-positive mitochondria surrounding the chromosomes (Figure 4 and Supplementary Figure S2). During GV oocyte maturation from prophase I to metaphase II, chromosomes and the spindle require energy in the entire oocyte. The co-localization of higher [Ca2+]m and MitoRed-positive mitochondria indicated that higher activated mitochondria required a higher [Ca2+]m. The localization relationship between [Ca2+]m and active mitochondria during oocyte partheno-activation was also observed next (Figure 5). [Ca2+]m and MitoRed still had clear co-localizations in the resting state. With [Ca2+]i oscillations, all of the mitochondria in the entire oocyte have a higher [Ca2+]m, increasing in the flashing state, which was consistent with the result of Figure 1. While oocytes were in the cytoplasmic Ca2+ flashing state, the [Ca2+]m increases, and the MitoRed positive mitochondria remain in a stable distribution pattern. One part of the mitochondria maintained a higher [Ca2+]m around the nucleus, and the other mitochondrial [Ca2+]m will increase instantaneously carried by [Ca2+]i, resulting in a pulse-like function enhancement. The role of [Ca2+]m oscillations on mitochondrial metabolism needs to be further studied.
The MCU, which is identified as a highly selective Ca2+ channel (Xing et al., 2019), uptakes Ca2+ across the inner mitochondrial membrane, playing critical roles in various mitochondrial functions (Kirichok et al., 2004; Mammucari et al., 2017). MCU consists of multiple subunits, and its Ca2+ influx activity is controlled by regulatory subunits (Yu et al., 2014), including two regulators MICU1 (Perocchi et al., 2010) and MICU2 (Patron et al., 2014). MICU1is localized to the inner mitochondrial membrane with conformational changes upon Ca2+ binding (Kamer and Mootha, 2014). In our study, MICU distributed with higher [Ca2+]m mitochondria in the GV oocytes (Figure 6), however in PAoocytes, distribution of MICU was similar as activated mitochondria with no change as [Ca2+]i oscillations. This may indicate that MICU is related to mitochondrial Ca2+ and mitochondrial activity. We also observed that VDAC (Supplementary Figure S4), which is widely believed to be distributed on the outer membrane, has a different distribution from the higher [Ca2+]m mitochondria, indicating that the process of regulating Ca2+ entry and exit from the mitochondrial matrix into and out of the mitochondria is not related to VDAC.
Dynamic Ca2+ responds to its corresponding functions, and this change is determined by a protein initiation signal pathway with [Ca2+]i sensing. Most studied Ca2+-responsive proteins are members of the calmodulin family. The calmodulin family contains CaM1 and CaM2. We examined the relationship between CaMs and higher [Ca2+]m of mitochondria. We cloned CaM1 and CaM2 linked with mCherry (Supplementary Figure S1). In GV oocytes, two types of cRNA were injected and time-lapse confocal laser microscopy was conducted. We found both CaM1 and CaM2 in the spindle area (Supplementary Figure S4). CaM2 and [Ca2+]m were examined to show the relationship between cytoplasmic Ca2+sensor CaM2 and active mitochondria. It can be seen in Figure 7 that higher [Ca2+]m mitochondria are distributed surrounding the spindle, however, CaM2 is associated with the spindle (Figure 7A). When activated, CaM2 associates with the spindle until the second polar body extrusion (Figure 7B). The main function of the CaMs family may be directly involved in microtubule organization and spindle formation as described before (Fan and Sun, 2004). The distribution of higher [Ca2+]m and activated mitochondria around the spindle also indicates that the change of calcium-regulated spindle dynamic changes requires a continuous supply of energy by higher [Ca2+]m stimulated mitochondria. Cortical region Mito-Red signals are independent of [Ca2+]m dynamic changes, which was not our expected result. Cortical [Ca2+]m oscillations may have some other function of mitochondria regulations. There may be another possibility that another probe but not Mito-Red is suitable for detecting the mitochondrial state during partheno-activation. An accurate distribution of higher [Ca2+]m mitochondria in oocytes is important for oocyte spindle reformation.
Deepening our understanding and basic knowledge about oocyte maturation/activation and energy metabolism is of great value for solving clinical problems such as insufficient oocyte cytoplasmic maturation and activation as well as insufficient energy supply caused by mitochondrial damage. Studying the regulatory mechanisms between [Ca2+]m and mitochondrial metabolism in oocytes will provide more possibilities for improving the efficiency of assisted reproduction.
Data Availability Statement
The raw data supporting the conclusions of this article will be made available by the authors, without undue reservation.
Ethics Statement
All mice were handled in accordance with the institutional animal care policies of the Institute of Zoology, Chinese Academy of Sciences. The Laboratory Animal Care and Use Committee of the Institute of Zoology approved this study.
Author Contributions
FW, Q-YS, and X-HO conceived and designed the experiments. FW and others conducted experiments. FW and Q-YS analyzed the data. FW, HS, X-HO, and Q-YS wrote the manuscript. All authors contributed to the article and approved the submitted version.
Funding
This work was sponsored by the National Natural Science Foundation of China (Grant Nos. 81971357 and 81901477) and the Science Foundation of Guangdong Second Provincial General Hospital (Grant No. YN- 2018-004).
Conflict of Interest
The authors declare that the research was conducted in the absence of any commercial or financial relationships that could be construed as a potential conflict of interest.
Supplementary Material
The Supplementary Material for this article can be found online at: https://www.frontiersin.org/articles/10.3389/fcell.2020.585932/full#supplementary-material
Supplementary Figure 1 | Constructed vectors for in vitro expression. (A,B) Complete cyclic plasmids maps of Mt-GCaMP6s and mCherry labeled protein. (C–F) Templates for cRNA in vitro expression by PCR with primers M13F and M13R.
Supplementary Figure 2 | Short term observation of higher [Ca2+]m and activated mitochondrial distribution during oocyte maturation. Double concentration of Mito-Red led to overexposure and cytotoxic effect after more than 4 h of observation. Higher [Ca2+]m and activated mitochondria show clear co-localization.
Supplementary Figure 3 | Dynamic distribution of VDAC and higher [Ca2+]m mitochondria during oocyte maturation and activation. (A,B) VDAC and higher [Ca2+]m mitochondria distribution during oocyte maturation and activation. C: Fluorescence intensity of VDAC (Red) and [Ca2+]m (Green) changes during maturation and activation. Ordinate is marked as Relative Fluorescence Intensity (relative to the fluorescence intensity of the start point).
Supplementary Figure 4 | CaM1 and CaM2 distribution in mature oocytes. Both CaM1 and CaM2 are located at the spindle.
Supplementary Video 1 | [Ca2+]m dynamic changes during oocyte maturation.
Abbreviations
[Ca2+] I, Intracellular Ca2+ Concentration; no[Ca2+]m, Mitochondrial Matrix Ca2+ Concentration; cpGFP, Circularly Permuted Green Fluorescent Protein; GV, Germinal Vesicle; GVBD, Germinal Vesicle Breakdown; MII, Metaphase II; Mt-GCaMP6s, Encoding Mitochondrial Ca2+ Probe Based cpGFP With A Mitochondrial Signal Peptide.
References
Akerboom, J., Chen, T. W., Wardill, T. J., Tian, L., Marvin, J. S., Mutlu, S., et al. (2012). Optimization of a GCaMP calcium indicator for neural activity imaging. J. Neurosci. 32, 13819–13840. doi: 10.1523/JNEUROSCI.2601-12.2012
Bathori, G., Parolini, I., Szabo, I., Tombola, F., Messina, A., Oliva, M., et al. (2000). Extramitochondrial porin: facts and hypotheses. J. Bioenerg. Biomembr. 32, 79–89. doi: 10.1023/a:1005516513313
Berridge, M. J., Lipp, P., and Bootman, M. D. (2000). The versatility and universality of calcium signalling. Nat. Rev. Mol. Cell. Biol. 1, 11–21. doi: 10.1038/35036035
Bonora, M., Morganti, C., Morciano, G., Pedriali, G., Lebiedzinska-Arciszewska, M., Aquila, G., et al. (2017). Mitochondrial permeability transition involves dissociation of F1FO ATP synthase dimers and C-ring conformation. EMBO Rep. 18, 1077–1089. doi: 10.15252/embr.201643602
Bootman, M. D., Lipp, P., and Berridge, M. J. (2001). The organisation and functions of local Ca(2+) signals. J. Cell. Sci. 114(Pt 12), 2213–2222.
Campbell, K., and Swann, K. (2006). Ca2+ oscillations stimulate an ATP increase during fertilization of mouse eggs. Dev. Biol. 298, 225–233. doi: 10.1016/j.ydbio.2006.06.032
Cassara, M. C., Menzel, V. A., Hinsch, K. D., Wrenzycki, C., and Hinsch, E. (2009). Voltage-dependent anion channels 1 and 2 are expressed in porcine oocytes. Biosci. Rep. 30, 193–200. doi: 10.1042/BSR20090088
Chacko, J. V., and Eliceiri, K. W. (2018). Autofluorescence lifetime imaging of cellular metabolism: sensitivity toward cell density, pH, intracellular, and intercellular heterogeneity. Cytometry A 95, 56–69. doi: 10.1002/cyto.a.23603
Chazotte, B. (2011). Labeling mitochondria with MitoTracker dyes. Cold Spring Harb. Protoc. 2011, 990–992. doi: 10.1101/pdb.prot5648
Chow, K. M., Cheung, M. C., and Cheung, I. K. (2016). Psychosocial interventions for infertile couples: a critical review. J. Clin. Nurs. 25, 2101–2113. doi: 10.1111/jocn.13361
De Pinto, V., Messina, A., Accardi, R., Aiello, R., Guarino, F., Tomasello, M. F., et al. (2003). New functions of an old protein: the eukaryotic porin or voltage dependent anion selective channel (VDAC). Ital. J. Biochem. 52, 17–24.
Dubouskaya, T. G., Hrynevich, S. V., Waseem, T. V., and Fedorovich, S. V. (2018). Calcium release from intracellular stores is involved in mitochondria depolarization after lowering extracellular pH in rat brain synaptosomes. Acta Neurobiol. Exp. 78, 343–351.
Dumollard, R., Duchen, M., and Carroll, J. (2007). The role of mitochondrial function in the oocyte and embryo. Curr. Top. Dev. Biol. 77, 21–49. doi: 10.1016/S0070-2153(06)77002-8
Dumollard, R., Duchen, M., and Sardet, C. (2006). Calcium signals and mitochondria at fertilisation. Semin. Cell. Dev. Biol. 17, 314–323. doi: 10.1016/j.semcdb.2006.02.009
Dupont, G., Heytens, E., and Leybaert, L. (2010). Oscillatory Ca2+ dynamics and cell cycle resumption at fertilization in mammals: a modelling approach. Int. J. Dev. Biol. 54, 655–665. doi: 10.1387/ijdb.082845gd
Eisner, V., Parra, V., Lavandero, S., Hidalgo, C., and Jaimovich, E. (2010). Mitochondria fine-tune the slow Ca(2+) transients induced by electrical stimulation of skeletal myotubes. Cell Calcium 48, 358–370. doi: 10.1016/j.ceca.2010.11.001
Fan, H. Y., and Sun, Q. Y. (2004). Involvement of mitogen-activated protein kinase cascade during oocyte maturation and fertilization in mammals. Biol. Reprod. 70, 535–547. doi: 10.1095/biolreprod.103.022830
Fink, B. D., Bai, F., Yu, L., and Sivitz, W. I. (2017). Regulation of ATP production: dependence on calcium concentration and respiratory state. Am. J. Physiol. Cell. Physiol. 313, C146–C153. doi: 10.1152/ajpcell.00086.2017
Garcia-Perez, C., Hajnoczky, G., and Csordas, G. (2008). Physical coupling supports the local Ca2+ transfer between sarcoplasmic reticulum subdomains and the mitochondria in heart muscle. J. Biol. Chem. 283, 32771–32780. doi: 10.1074/jbc.M803385200
Gesink Law, D. C., Maclehose, R. F., and Longnecker, M. P. (2007). Obesity and time to pregnancy. Hum. Reprod. 22, 414–420. doi: 10.1093/humrep/del400
Gordo, C., Rodrigues, P., Kurokawa, M., Jellerette, T., Exley, G. E., Warner, C., et al. (2002). Intracellular calcium oscillations signal apoptosis rather than activation in in vitro aged mouse eggs. Biol. Reprod. 66, 1828–1837.
Griffiths, E. J., and Rutter, G. A. (2009). Mitochondrial calcium as a key regulator of mitochondrial ATP production in mammalian cells. Biochim. Biophys. Acta 1787, 1324–1333. doi: 10.1016/j.bbabio.2009.01.019
Hajnoczky, G., Robb-Gaspers, L. D., Seitz, M. B., and Thomas, A. P. (1995). Decoding of cytosolic calcium oscillations in the mitochondria. Cell 82, 415–424.
Igosheva, N., Abramov, A. Y., Poston, L., Eckert, J. J., Fleming, T. P., Duchen, M. R., et al. (2010). Maternal diet-induced obesity alters mitochondrial activity and redox status in mouse oocytes and zygotes. PLoS One 5:e10074. doi: 10.1371/journal.pone.0010074
Jungheim, E. S., Schoeller, E. L., Marquard, K. L., Louden, E. D., Schaffer, J. E., and Moley, K. H. (2010). Diet-induced obesity model: abnormal oocytes and persistent growth abnormalities in the offspring. Endocrinology 151, 4039–4046. doi: 10.1210/en.2010-0098
Jungheim, E. S., Schon, S. B., Schulte, M. B., DeUgarte, D. A., Fowler, S. A., and Tuuli, M. G. (2013a). IVF outcomes in obese donor oocyte recipients: a systematic review and meta-analysis. Hum. Reprod. 28, 2720–2727. doi: 10.1093/humrep/det292
Jungheim, E. S., Travieso, J. L., and Hopeman, M. M. (2013b). Weighing the impact of obesity on female reproductive function and fertility. Nutr. Rev. 71(Suppl. 1), S3–S8. doi: 10.1111/nure.12056
Kamer, K. J., and Mootha, V. K. (2014). MICU1 and MICU2 play nonredundant roles in the regulation of the mitochondrial calcium uniporter. EMBO Rep. 15, 299–307. doi: 10.1002/embr.201337946
Kirichok, Y., Krapivinsky, G., and Clapham, D. E. (2004). The mitochondrial calcium uniporter is a highly selective ion channel. Nature 427, 360–364. doi: 10.1038/nature02246
Luzzo, K. M., Wang, Q., Purcell, S. H., Chi, M., Jimenez, P. T., Grindler, N., et al. (2012). High fat diet induced developmental defects in the mouse: oocyte meiotic aneuploidy and fetal growth retardation/brain defects. PLoS One 7:e49217. doi: 10.1371/journal.pone.0049217
Mallilankaraman, K., Cardenas, C., Doonan, P. J., Chandramoorthy, H. C., Irrinki, K. M., Golenar, T., et al. (2012a). MCUR1 is an essential component of mitochondrial Ca2+ uptake that regulates cellular metabolism. Nat. Cell. Biol. 14, 1336–1343. doi: 10.1038/ncb2622
Mallilankaraman, K., Doonan, P., Cardenas, C., Chandramoorthy, H. C., Muller, M., Miller, R., et al. (2012b). MICU1 is an essential gatekeeper for MCU-mediated mitochondrial Ca(2+) uptake that regulates cell survival. Cell 151, 630–644. doi: 10.1016/j.cell.2012.10.011
Mammucari, C., Gherardi, G., and Rizzuto, R. (2017). Structure, activity regulation, and role of the mitochondrial calcium uniporter in health and disease. Front. Oncol. 7:139. doi: 10.3389/fonc.2017.00139
Marchant, J. S., Ramos, V., and Parker, I. (2002). Structural and functional relationships between Ca2+ puffs and mitochondria in Xenopus oocytes. Am. J. Physiol. Cell. Physiol. 282, C1374–C1386. doi: 10.1152/ajpcell.00446.2001
Mehta, S., Aye-Han, N. N., Ganesan, A., Oldach, L., Gorshkov, K., and Zhang, J. (2014). Calmodulin-controlled spatial decoding of oscillatory Ca2+ signals by calcineurin. eLife 3:e03765. doi: 10.7554/eLife.03765
Metodiev, M. D., Thompson, K., Alston, C. L., Morris, A. A. M., He, L., Assouline, Z., et al. (2016). Recessive mutations in TRMT10C cause defects in mitochondrial RNA processing and multiple respiratory chain deficiencies. Am. J. Hum. Genet. 98, 993–1000. doi: 10.1016/j.ajhg.2016.03.010
Minge, C. E., Bennett, B. D., Norman, R. J., and Robker, R. L. (2008). Peroxisome proliferator-activated receptor-gamma agonist rosiglitazone reverses the adverse effects of diet-induced obesity on oocyte quality. Endocrinology 149, 2646–2656. doi: 10.1210/en.2007-1570
Mishra, P. (2016). Interfaces between mitochondrial dynamics and disease. Cell Calcium 60, 190–198. doi: 10.1016/j.ceca.2016.05.004
Naon, D., and Scorrano, L. (2014). At the right distance: ER-mitochondria juxtaposition in cell life and death. Biochim. Biophys. Acta 1843, 2184–2194. doi: 10.1016/j.bbamcr.2014.05.011
Oerum, S., Roovers, M., Rambo, R. P., Kopec, J., Bailey, H. J., Fitzpatrick, F., et al. (2018). Structural insight into the human mitochondrial tRNA purine N1-methyltransferase and ribonuclease P complexes. J. Biol. Chem. 293, 12862–12876. doi: 10.1074/jbc.RA117.001286
Ou, X. H., and Sun, Q. Y. (2017). Mitochondrial replacement techniques or therapies (MRTs) to improve embryo development and to prevent mitochondrial disease transmission. J. Genet. Genomics 44, 371–374. doi: 10.1016/j.jgg.2017.07.003
Ozil, J. P., Markoulaki, S., Toth, S., Matson, S., Banrezes, B., Knott, J. G., et al. (2005). Egg activation events are regulated by the duration of a sustained [Ca2+]cyt signal in the mouse. Dev. Biol. 282, 39–54. doi: 10.1016/j.ydbio.2005.02.035
Patron, M., Checchetto, V., Raffaello, A., Teardo, E., Vecellio Reane, D., Mantoan, M., et al. (2014). MICU1 and MICU2 finely tune the mitochondrial Ca2+ uniporter by exerting opposite effects on MCU activity. Mol. Cell. 53, 726–737. doi: 10.1016/j.molcel.2014.01.013
Perocchi, F., Gohil, V. M., Girgis, H. S., Bao, X. R., McCombs, J. E., Palmer, A. E., et al. (2010). MICU1 encodes a mitochondrial EF hand protein required for Ca(2+) uptake. Nature 467, 291–296. doi: 10.1038/nature09358
Rittenberg, V., Sobaleva, S., Ahmad, A., Oteng-Ntim, E., Bolton, V., Khalaf, Y., et al. (2011). Influence of BMI on risk of miscarriage after single blastocyst transfer. Hum. Reprod. 26, 2642–2650. doi: 10.1093/humrep/der254
Rizzuto, R., De Stefani, D., Raffaello, A., and Mammucari, C. (2012). Mitochondria as sensors and regulators of calcium signalling. Nat. Rev. Mol. Cell. Biol. 13, 566–578. doi: 10.1038/nrm3412
Rottenberg, H., and Scarpa, A. (1974). Calcium uptake and membrane potential in mitochondria. Biochemistry 13, 4811–4817. doi: 10.1021/bi00720a020
Samanta, K., Mirams, G. R., and Parekh, A. B. (2018). Sequential forward and reverse transport of the Na(+) Ca(2+) exchanger generates Ca(2+) oscillations within mitochondria. Nat. Commun. 9:156. doi: 10.1038/s41467-017-02638-2
Schatten, H., Sun, Q. Y., and Prather, R. (2014). The impact of mitochondrial function/dysfunction on IVF and new treatment possibilities for infertility. Reprod. Biol. Endocrinol. 12:111. doi: 10.1186/1477-7827-12-111
Shankar, K., Zhong, Y., Kang, P., Lau, F., Blackburn, M. L., Chen, J. R., et al. (2011). Maternal obesity promotes a proinflammatory signature in rat uterus and blastocyst. Endocrinology 152, 4158–4170. doi: 10.1210/en.2010-1078
Shoshan-Barmatz, V., Israelson, A., Brdiczka, D., and Sheu, S. S. (2006). The voltage-dependent anion channel (VDAC): function in intracellular signalling, cell life and cell death. Curr. Pharm. Des. 12, 2249–2270. doi: 10.2174/138161206777585111
Stein, P., and Schindler, K. (2011). Mouse oocyte microinjection, maturation and ploidy assessment. J. Vis. Exp. 53:2851. doi: 10.3791/2851
Stricker, S. A. (1999). Comparative biology of calcium signaling during fertilization and egg activation in animals. Dev. Biol. 211, 157–176. doi: 10.1006/dbio.1999.9340
Van Blerkom, J., Davis, P. W., and Lee, J. (1995). ATP content of human oocytes and developmental potential and outcome after in-vitro fertilization and embryo transfer. Hum. Reprod. 10, 415–424.
Viola, H. M., Arthur, P. G., and Hool, L. C. (2009). Evidence for regulation of mitochondrial function by the L-type Ca2+ channel in ventricular myocytes. J. Mol. Cell. Cardiol. 46, 1016–1026. doi: 10.1016/j.yjmcc.2008.12.015
Wacquier, B., Combettes, L., and Dupont, G. (2019). Cytoplasmic and mitochondrial calcium signaling: a two-way relationship. Cold Spring Harb. Perspect. Biol. 11:a035139. doi: 10.1101/cshperspect.a035139
Wakai, T., Zhang, N., Vangheluwe, P., and Fissore, R. A. (2013). Regulation of endoplasmic reticulum Ca(2+) oscillations in mammalian eggs. J. Cell. Sci. 126(Pt 24), 5714–5724. doi: 10.1242/jcs.136549
Wang, F., Chen, Z., Ren, X., Tian, Y., Liu, C., Jin, P., et al. (2017). Hormone-sensitive lipase deficiency alters gene expression and cholesterol content of mouse testis. Reproduction 153, 175–185. doi: 10.1530/REP-16-0484
Wang, F., Ren, X.-F., Chen, Z., Li, X.-L., Zhu, H.-J., Li, S., et al. (2019). The N-terminal His-tag affects the triglyceride lipase activity of hormone-sensitive lipase in testis. J. Cell. Biochem. 120, 13706–13716. doi: 10.1002/jcb.28643
Wang, F., Yuan, R. Y., Li, L., Meng, T. G., Fan, L. H., Jing, Y., et al. (2018). Mitochondrial regulation of [Ca(2+)]i oscillations during cell cycle resumption of the second meiosis of oocyte. Cell Cycle 17, 1471–1486. doi: 10.1080/15384101.2018.1489179
Xing, Y., Wang, M., Wang, J., Nie, Z., Wu, G., Yang, X., et al. (2019). Dimerization of MICU proteins controls Ca(2+) influx through the mitochondrial Ca(2+) uniporter. Cell. Rep. 26, 1203.e4–1212.e4. doi: 10.1016/j.celrep.2019.01.022
Xu, Z., Lefevre, L., Ducibella, T., Schultz, R. M., and Kopf, G. S. (1996). Effects of calcium-BAPTA buffers and the calmodulin antagonist W-7 on mouse egg activation. Dev. Biol. 180, 594–604. doi: 10.1006/dbio.1996.0331
Yazawa, H., Yanagida, K., and Sato, A. (2007). Human round spermatids from azoospermic men exhibit oocyte-activation and Ca2+ oscillation-inducing activities. Zygote 15, 337–346. doi: 10.1017/S0967199407004339
Yu, X. J., Yi, Z., Gao, Z., Qin, D., Zhai, Y., Chen, X., et al. (2014). The subcortical maternal complex controls symmetric division of mouse zygotes by regulating F-actin dynamics. Nat. Commun. 5:4887. doi: 10.1038/ncomms5887
Keywords: mitochondrial Ca2+, oocyte activation, oocyte maturation, oocyte metabolism, Ca2+ oscillations
Citation: Wang F, Meng T-G, Li J, Hou Y, Luo S-M, Schatten H, Sun Q-Y and Ou X-H (2020) Mitochondrial Ca2 + Is Related to Mitochondrial Activity and Dynamic Events in Mouse Oocytes. Front. Cell Dev. Biol. 8:585932. doi: 10.3389/fcell.2020.585932
Received: 22 July 2020; Accepted: 22 September 2020;
Published: 27 October 2020.
Edited by:
Xiang-Shun Cui, Chungbuk National University, South KoreaReviewed by:
Cheng-guang Liang, Inner Mongolia University, ChinaShen Yin, Qingdao Agricultural University, China
Copyright © 2020 Wang, Meng, Li, Hou, Luo, Schatten, Sun and Ou. This is an open-access article distributed under the terms of the Creative Commons Attribution License (CC BY). The use, distribution or reproduction in other forums is permitted, provided the original author(s) and the copyright owner(s) are credited and that the original publication in this journal is cited, in accordance with accepted academic practice. No use, distribution or reproduction is permitted which does not comply with these terms.
*Correspondence: Qing-Yuan Sun, c3VucXlAZ2QyaC5vcmcuY24=; Xiang-Hong Ou, b3V4aWFuZ2hvbmcyMDAzQDE2My5jb20=