- Department of Pharmacology and Toxicology, National Institute of Pharmaceutical Education and Research (NIPER), Mohali, India
Parkinson’s disease (PD) is a neurodegenerative disorder characterized by the symptoms of motor deficits and cognitive decline. There are a number of therapeutics available for the treatment of PD, but most of them suffer from serious side effects such as bradykinesia, dyskinesia and on-off effect. Therefore, despite the availability of these pharmacological agents, PD patients continue to have an inferior quality of life. This has warranted a need to look for alternate strategies and molecular targets. Recent evidence suggests the Transient Receptor Potential (TRP) channels could be a potential target for the management of motor and non-motor symptoms of PD. Though still in the preclinical stages, agents targeting these channels have shown immense potential in the attenuation of behavioral deficits and signaling pathways. In addition, these channels are known to be involved in the regulation of ionic homeostasis, which is disrupted in PD. Moreover, activation or inhibition of many of the TRP channels by calcium and oxidative stress has also raised the possibility of their paramount involvement in affecting the other molecular mechanisms associated with PD pathology. However, due to the paucity of information available and lack of specificity, none of these agents have gone into clinical trials for PD treatment. Considering their interaction with oxidative stress, apoptosis and excitotoxicity, TRP channels could be considered as a potential future target for the treatment of PD.
Introduction
Parkinson’s disease (PD) is a neurodegenerative condition characterized mainly by the loss of dopaminergic neurons in regions of the basal ganglia. It is the second most prevalent form of neurodegenerative disorder and stands next to Alzheimer’s disease (AD) in terms of number of individuals affected across the globe (Parkinson’s News Today, 2020). There are a number of therapeutics (Levodopa, Dopamine (D2) receptor agonists, monoamine oxidase B inhibitors, catechol-o-methyl transferase inhibitors, Adenosine 2A antagonists) approved for the treatment of PD. However, most of them provide only symptomatic relief. Also, currently available drugs show severe side effects and require frequent dosing resulting in low patient compliance of these medications. This has warranted a search for new mechanisms as well as molecular targets which could be utilized for the development of drug candidates in PD (Kumar et al., 2009; Uppalapati et al., 2014; Das and Sharma, 2015; Zhen and Chu, 2020). There are a number of in vitro and in vivo models which have been used for the therapeutic screening of pharmacological agents targeting these new molecular targets in PD. PD models include administration of neurotoxins such as 1-methyl-4-phenyl-1,2,3,6-tetrahy- dropyridine (MPTP), 1-methyl-4-phenylpyridinium (MPP+), rotenone, 6-hydroxydopmaine (6-OHDA) and alpha-synuclein pro-fibrils to induce dopaminergic neuronal death in the substantia nigra and the striatum. In addition, transgenic mice (knock-in and knock-out) are available for induction of PD. Pathophysiology of PD is complex and multi-factorial. Studies done using these preclinical models as well as PD patients have pointed out at the involvement of Ca2+ overload and oxidative stress in PD pathology. Cumulative evidence in recent times has demonstrated a major contribution of Transient Receptor Potential (TRP) channels toward Ca2+ mediated excitotoxicity and oxidative stress-induced dopaminergic neuronal death in PD. In total, 28 members of the TRP family have been discovered in mammals which are activated in response to diverse physiological stimuli (Zheng, 2013; Samanta et al., 2018). However, most of these members are non-selective cation channels which are responsible for the influx of monovalent and divalent ions, including Ca2+ into the cell. Oxidative stress and reactive oxygen species (ROS) have also been postulated to play a role in mechanisms involving activation and inhibition of these TRP channels (Adhya and Sharma, 2019; Akyuva and Naziroglu, 2020). These properties make them an attractive target for the treatment of neurodegenerative conditions, including PD (Thapak et al., 2020c). As a result, last few decades have seen enormous progress in the field of TRP research, and many of the TRP channel modulators like AMG571, MK-2295 and AZD1386 (TRPV1 antagonists) have even managed to progress to the clinical trials for the treatment of pain disorders (Kaneko and Szallasi, 2014). However, further analysis of these TRP channel modulators has exposed their dark side with side effects raging form hyperthermia and to reduced noxious perception to heat (Gavva, 2009; Kaneko and Szallasi, 2014). Thus, most of these studies had to be terminated owing to the risk of scalding injuries to the patients. Keeping in mind the crucial role of these channels, it is now expected that detailed structure-activity relationships and pharmacophore studies would help to improve their safety and efficacy and ensure the translational success of TRP channel modulators in PD.
In this review, we have provided a detailed account of preclinical evidence suggesting the vital involvement of TRP channels in PD and how their knowledge may help researchers in this field gain in-depth insight about these channels. Additionally, a number of upcoming drug candidates targeting TRP channels have also been discussed.
TRP Family
TRP family of ion channels was first discovered in 1969 when some of the drosophila fly mutants turned blind in response to bright light (Cosens and Manning, 1969). This identification was followed by a series of biochemical, electrophysiological and molecular studies which led to the characterization of the TRP channel family. It is now well established that these channels are evolutionarily conserved across species and are found in most cells, tissues, and organisms. Subsequently, a total of 28 members belonging to 6 subfamilies were identified in mammals which include TRPV (Vanilloid), TRPM (Melastatin), TRPC (Canonical), TRPML (Mucolipin), TRPA (Ankyrin), and TRPP (Polycystic) (Li, 2017). Besides, other families, such as TRPY and TRPN were also identified in the non-mammalian species. Amongst these, TRPY is the most distant relative which is only present in yeast. It plays a role in the release of Ca2+ from the vacuoles when exposed to hyperosmotic conditions (Denis and Cyert, 2002; Venkatachalam and Montell, 2007). TRPN has also been reported but only in a limited number of vertebrates such as xenopus and zebrafish, where it serves as the mechanosensitive channel for performing the normal physiological function (Hardie, 2007). However, these channels bear little relevance to human disease and hence have not been discussed in greater detail. Structurally all the TRP channels form six transmembrane helixes with both the N and C terminal located within the cytoplasm. These channels are activated in response to a diverse range of physiological and sensory stimuli which include mechanical, thermal, electrical and chemical cues (Steinberg et al., 2014). Therefore, these TRP channels are involved in the sensation of touch, vision, olfaction, temperature and nociception (Voets et al., 2005). Moreover, these TRPs exhibit a widespread expression in the brain areas involved in pathophysiology of PD, making them a very relevant molecular target for the discovery of new drugs to treat PD (Table 1). The detailed role of TRP channels has been discussed in the further sections.
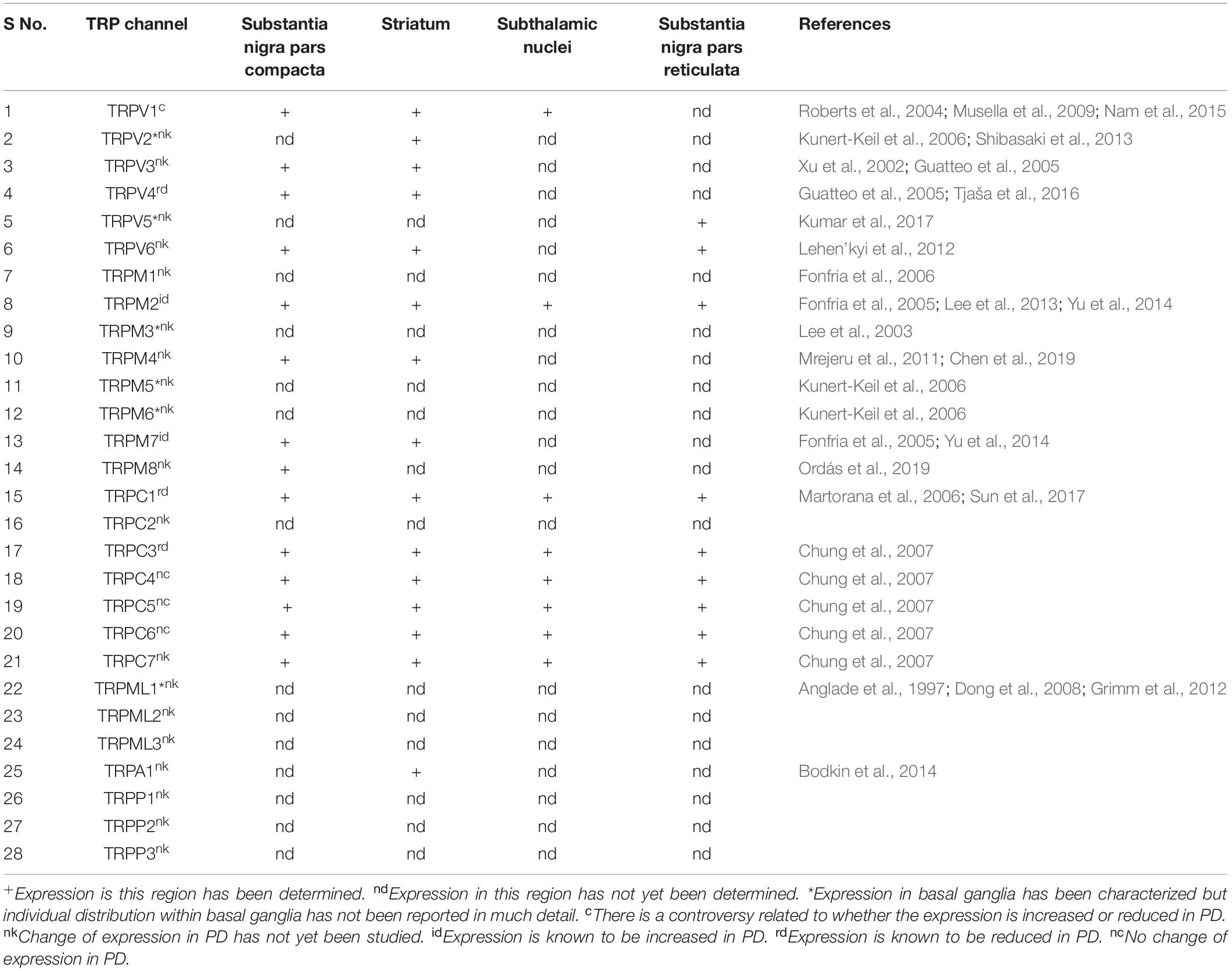
Table 1. Expression of the different TRP channels in the regions involved in the pathophysiology of PD.
Contribution of TRP Channels to PD
TRPV Involvement in PD
TRPV channels are abundantly expressed in several areas of the brain. Although different members of the TRPV subfamily perform a very diverse physiological role, all of these have modest permeability toward Ca2+. It makes them important for various central nervous system (CNS) functions, including learning and memory (Thapak et al., 2020a). It has therefore been reported that any change in the expression of TRPV channels contributes toward CNS disease pathologies such as AD, PD, cerebral ischemia, depression and anxiety (Bashiri et al., 2018; Thapak et al., 2020c).
Amongst the members of the TRPV family, TRPV1/4/5 have been studied for their involvement in PD pathology. TRPV1 is expressed in the regions, striatum and substantia nigra, which are known to be affected in PD (Musella et al., 2009; Nam et al., 2015). However, its role remains controversial pertaining to modulation of different downstream signaling pathways by it. Though an upregulation of TRPV1 has been reported by several authors in different PD models, both agonists and antagonists targeting these have been successful in alleviating symptoms of disease in the preclinical stages (Figure 1) (Nam et al., 2015; Li et al., 2019).
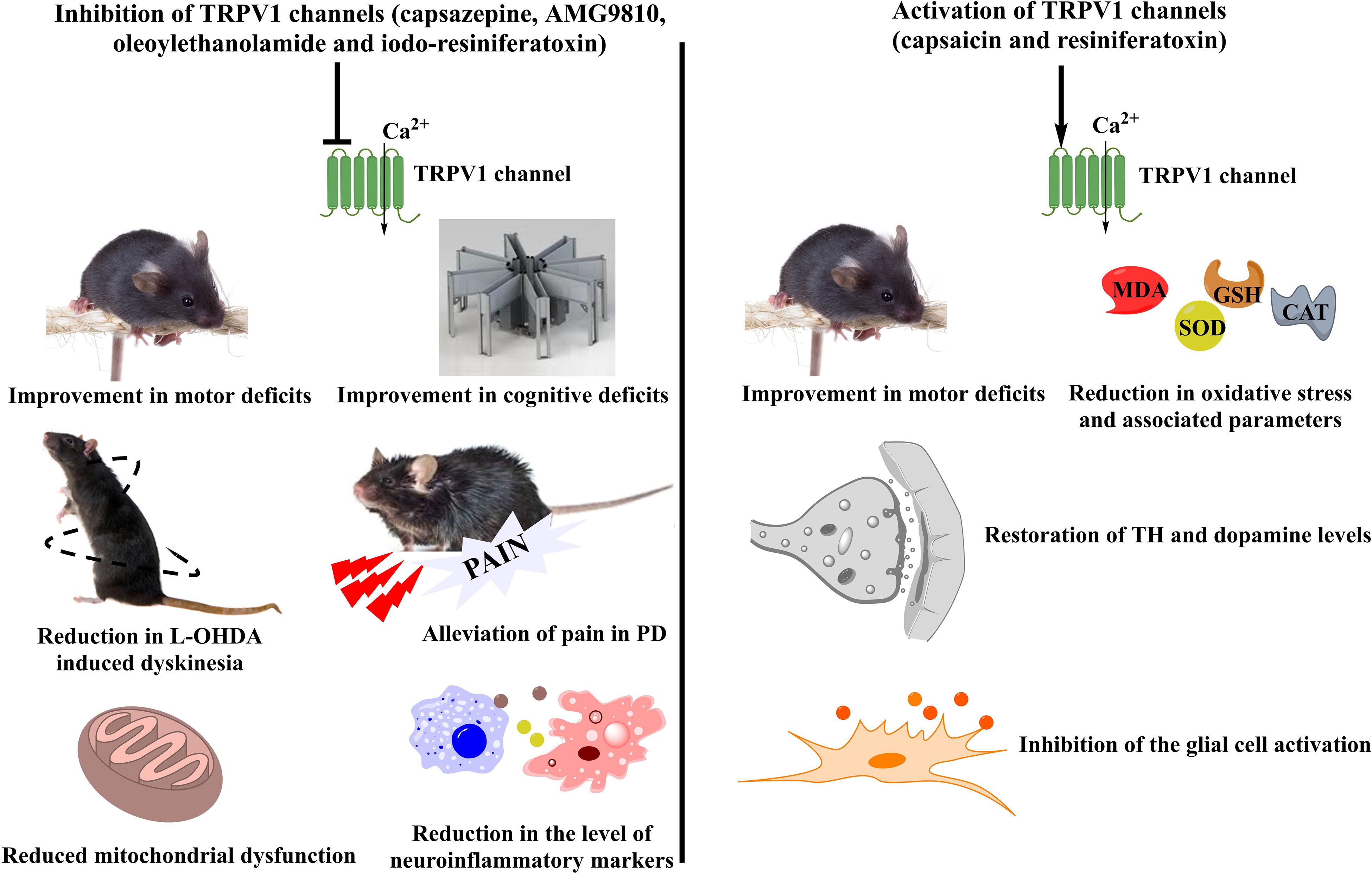
Figure 1. Controversial role of TRPV1 channels in PD. A number of TRPV1 modulators have been tested for their beneficial effects in PD. Different agents working either via TRPV1 inhibition or TRPV1 activation have been studied for the mitigation of PD symptoms in preclinical models. Inhibition of TRPV1 led to an improvement in the motor and cognitive deficits of PD accompanied by reduced mitochondrial dysfunction and decreased levels of inflammatory mediators. Moreover, TRPV1 inhibitors also showed a reduction in the 6-OHDA induced dyskinesias and provided relief from pain which is one of the major non-motor symptoms of PD. On the other hand, TRPV1 activation had similar effects on improvement of motor coordination. It also reduced the levels of oxidative stress and led to the restoration of the tyrosine hydroxylase and dopamine levels. Furthermore, a significant reduction in the microglial activation was also observed after the administration of TRPV1 activators. TRPV1, Transient Receptor Potential Vanilloid 1; PD, Parkinson’s disease; 6-OHDA, 6-hydroxydopamine; TH, Tyrosine hydroxylase; MDA, Malondialdehyde; SOD, superoxide dismutase; GSH, glutathione; CAT, catalase.
Studies related to other TRPV channels like TRPV4/5 have also been carried out but remain rather limited. Expression of TRPV4 was downregulated in SNpc and striatum of rats after 6-OHDA administration suggesting a possible correlation between TRPV4 expression and neuronal death (Tjaša et al., 2016). Similarly, there is not much data to support the involvement of TRPV5 channels in PD. However, a transcriptome analysis suggested its possible role in the regulation of the pathophysiological hallmarks of PD in 6-OHDA treated rats (Li et al., 2019). Nevertheless, the lack of enough data and the absence of any pharmacological study targeting these channels have denied them a concrete claim as a possible drug target for PD.
TRPM Involvement in PD
TRPM channel subfamily has been extensively explored for its potential involvement in pathophysiology of several neurodegenerative disorders including PD (Ostapchenko et al., 2015; Sun et al., 2018b; Thapak et al., 2020b). It has been reported that the members of the TRPM subfamily lead to an influx of monovalent and divalent cations inside the cell which might serve as a contributing factor for the excitotoxicity mediated neuronal death (Adhya and Sharma, 2019).
Amongst these, TRPM2 channels have gained importance for their involvement in several mechanisms related to neuronal death. These channels are activated in response to oxidative stress and adenosine diphosphate ribose (ADPR), both of which are increased in PD. Though the permeability ratio of TRPM2 for Ca2+ to Na+ (PCa:PNa) is nearly 0.7 indicating a higher permeability for Na+, a substantial Ca2+ influx also takes place because of the longer opening time of TRPM2 channels (Belrose and Jackson, 2018). The increased Ca2+ levels as a result of TRPM2 activation lead to excitotoxicity and contribute toward the aggravation of the underlying PD pathology.
Apart from TRPM2, TRPM7 has also been investigated for its involvement in PD. Genetic variants of TRPM7 have been linked to the ionic dyshomeostasis, mitochondrial dysfunction, inflammation and increased oxidative stress, all of which led to its identification as a candidate susceptibility gene for familial PD (Hermosura et al., 2005; Hermosura and Garruto, 2007). In addition, PC-12 cells exposed to 6-OHDA also showed an increased expression of TRPM7, which was reduced after overexpression of miR-22. Furthermore, miR-22 overexpression also protected from the effects of TRPM7 upregulation by inhibition of apoptosis, reduction of ROS and improved cell viability (Yang et al., 2016). Despite these findings, there are other controversial reports which have observed neuroprotective effects of the TRPM7 mediated Mg2+ influx in the MPP+ based in vitro model (Shindo et al., 2015). Hence, more studies with selective TRPM7 modulators are required to conclusively elucidate the role of these channels in PD.
TRPM8 is another candidate belonging to the TRPM subfamily which has been identified as a risk factor for pain in PD (Williams et al., 2020). Pain is a frequently occurring non-motor symptom, which has been reported in about 86% of the PD patients (Beiske et al., 2009; Skogar and Lokk, 2016). Therefore, strategies targeting TRPM8 channels could be utilized in future for the management of pain in PD patients.
In totality, these findings suggest that TRPM channels are critical molecular players for neuronal death in PD (Figure 2). However, the dearth of information related to their signaling pathways has largely limited the development of selective modulators for these channels in the PD models.
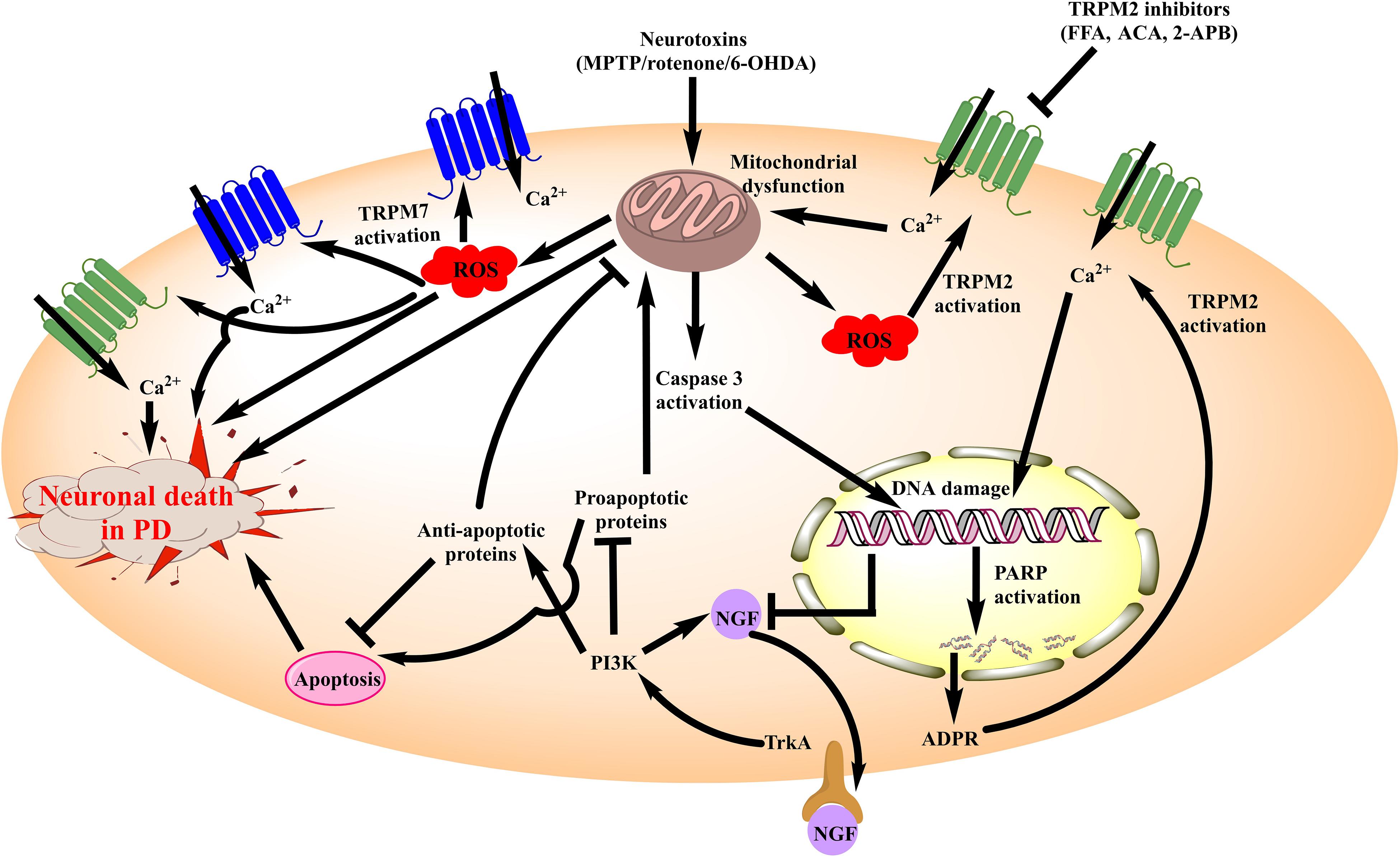
Figure 2. Schematic diagram illustrating the role of TRPM channels in neuronal death associated with PD. Amongst the TRPM channels, TRPM2 and TRPM7 have been elucidated for their involvement in PD. Neurotoxins such as MPTP, 6-OHDA and rotenone inhibit the mitochondrial complexes producing mitochondrial dysfunction. It generates ROS which acts as an activator of both TRPM2 and TRPM7 leading to the increased Ca2+ influx within neurons. These events in totality increase the neuronal death in PD besides aggravating mitochondrial dysfunction. Moreover, cytochrome c (Cyt c) is released from the mitochondria in this process which promotes pro-apoptotic signaling and damages DNA. Damage to the DNA leads to poly ADP ribose polymerase (PARP) mediated ADPR formation which is also an activator of TRPM2 channel. Therefore, the cascade of events exhibits a positive feedback loop which aggravates neuronal death. Besides, there is a reduced expression of NGF and associated TrkA/PI3K signaling, which intensifies the pro-apoptotic response and plays its part in the TRPM2 and TRPM7 mediated neuronal death in PD. MPTP, 1-methyl-4-phenyl-1,2,3,6-tetrahydropyridine; 6-OHDA, 6-hydroxy dopamine; ROS, reactive oxygen species; FFA, Flufenamic acid; ACA, N-(p-amylcinnamoyl) anthranilic acid; 2-APB, 2-Aminoethoxydiphenyl borate; TRPM, Transient receptor potential melastatin 2; NGF, Nerve growth factor; PARP, poly ADP ribose polymerase; PI3K, phosphoinositide 3-kinase; ADPR, Adenosine di-phosphate ribose; TrkA, Tropomyosin receptor kinase A.
TRPC Involvement in PD
TRPC subfamily has a widespread distribution in the CNS. It was also shown to exhibit high expression in the areas of SNpc and striatum, both of which are involved in the pathophysiology of PD (Sukumaran et al., 2017). As a result, different members of the TRPC subfamily have been investigated for their potential involvement in PD. TRPC1 was the first member of the TRPC subfamily to be identified in mammals. It is localized to the dendritic processes and co-expressed with tyrosine hydroxylase (TH) in the dopaminergic neurons of the SNpc (Martorana et al., 2006). This pointed out at the physiological relevance of TRPC1 channels in the dopaminergic system and raised the possibility that these channels may contribute toward the pathogenesis of PD. Studies carried out using the MPP+/MPTP model showed that the expression of TRPC1 was reduced in both the SNpc as well as PC12 neuronal cells in PD. Besides, TRPC1–/– mice also reported motor deficits and increased Terminal deoxynucleotidyl transferase dUTP nick end labeling (TUNEL)-positive cells in the basal ganglia suggesting the critical role of TRPC1 in regulating apoptotic signaling within the cell (He et al., 2016). Similar results were observed in other studies where TRPC1 overexpression protected from the effects of 6-OHDA, MPP+ and α-synuclein toxicity in the neuronal cells (Bollimuntha et al., 2005; Sukumaran et al., 2018).
Several groups then looked at the downstream signaling associated with the neuroprotection offered by TRPC1 channels in PD models (Selvaraj et al., 2012). One of the possible mechanisms for this was the reduced endoplasmic reticulum (ER) stress which is otherwise increased in PD (Deng and Bai, 2012). It was shown that overexpression of TRPC1 channels in the SH-SY5Y cells treated with MPP+ led to the restoration of phosphatidylinositol-3-kinase (PI3K)/mammalian target of rapamycin (Akt/mTOR) signaling and increased dopaminergic neuronal survival. Interestingly, similar findings were reported in the brains of the PD patients and TRPC1–/– mice which further showed an increased unfolded protein response (UPR) and reduced number of dopamine neurons in the brain (Selvaraj et al., 2012). On the other hand, TRPC1 deletion mediated ER stress was found to be associated with increased ROS, dysregulation of the glucose-regulated protein 78 (GRP78) and alteration in protein kinase RNA-like ER kinase (PERK) signaling pathways (Wang et al., 2018). In addition, potential crosstalk between the TRPC1-Stromal interaction molecule (STIM) and L-type calcium channels was also established using an electrophysiological study in the SNpc of the TRPC1–/– mice (Sun et al., 2017). As L-type calcium channel blockers like isradipine have already gone into clinical trials for PD, TRPC1 performing the same physiological function in dopaminergic neurons could be looked at as a molecular target in PD (Simuni et al., 2010; Sun et al., 2017). Consistent with these results are the findings of Sun et al. (2018a), where MPP+ was found to reduce TRPC1 expression and store-operated calcium entry in the mesenchymal stem cell-derived dopaminergic neurons.
Other pathways that were affected in PD due to reduced TRPC1 expression include attenuation of autophagy as well as impairment of nuclear factor kappa-light-chain-enhancer of activated B cells (NF-kB) and Tropomyosin receptor kinase A (TrkA) signaling (Yu et al., 2013; Sukumaran et al., 2018). In contrast to these findings, downregulation of STIM, a TRPC1 activator was shown to prevent oxidative stress and apoptosis mediated neuronal death in 6-OHDA treated PC-12 cells (Li et al., 2014; Sukumaran et al., 2017). However, despite this discrepancy, most of the other studies have suggested a beneficial role of TRPC1 channels in the pathophysiology of PD are shown in Figure 3.
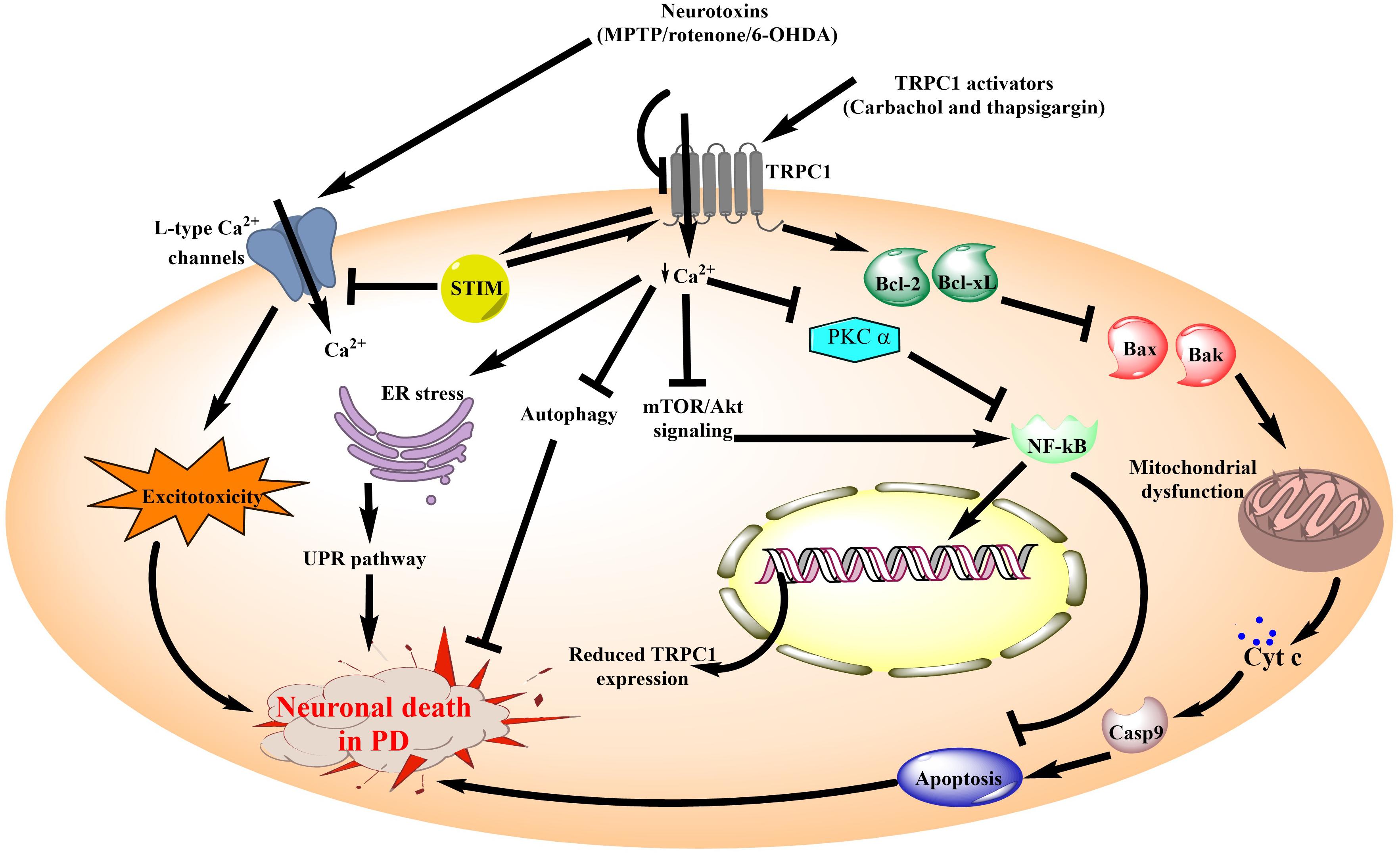
Figure 3. Schematic diagram illustrating the role of TRPC1 channels in neuronal death associated with PD. TRPC1 channel is the most widely studied member of the TRPC subfamily for its involvement in PD. Ca2+ influx through TRPC1 channels is known to be neuroprotective in the PD models. Additionally, review of literature suggests that inhibition of Ca2+ influx from TRPC1 by the administration of neurotoxins causes aggravation of the PD pathology. It leads to inhibition of mTOR/Akt signaling as well as reduced levels of PKC α. These events reduce NF-κB expression which in turn increases the DNA damage and pro-apoptotic signaling to compromise neuronal survival. On the other hand, TRPC1 activation directly promotes anti-apoptotic signaling to reduce mitochondrial dysfunction and associated cell death in PD. In addition, diminished Ca2+ influx from the TRPC1 channels results in impaired autophagy and ER stress. Moreover, in association with STIM, TRPC1 channels inhibit the Ca2+ influx via L-type calcium channels which are one of the major contributors toward excitotoxicity mediated neuronal death in PD. MPTP, 1-methyl-4-phenyl-1,2,3,6-tetrahydropyridine; 6-OHDA, 6-hydroxy dopamine; TRPC1, Transient Receptor Potential Canonical 1; ER, endoplasmic reticulum; STIM, Stromal interaction molecule; UPR, unfolded protein response; mTOR, mammalian target of rapamycin; Akt, Protein kinase B; PKC, Protein kinase C; NF-κB, nuclear factor kappa-light-chain-enhancer of activated B cells; Cyt c, cytochrome c; Casp9, caspase 9; Bcl2, B-cell lymphoma 2; Bcl-xl, B-cell lymphoma-extra large; Bax, Bcl2-associated X protein; Bak, Bcl-2 homologous antagonist/killer.
Other TRPC channels such as TRPC3 are also expressed in the SNpc and striatal regions of the brain. These are important for the regulation of firing intensity and neuronal depolarization in these brain regions (Zhou et al., 2008; Xie and Zhou, 2014). Additionally, these channels are also required for synaptic transmission and motor coordination, making them an important target for investigation in the context of PD (Hartmann et al., 2008). Initial observation though suggested little to no change in the TRPC3 expression in PD, studies carried out much later have suggested otherwise (Selvaraj et al., 2009, 2012; Yu et al., 2013; Streifel et al., 2014). In the 6-OHDA model, data from RT-PCR studies showed reduced expression of TRPC3 in the SNpc (Yu et al., 2013). Similar results were reported by other groups which revealed that the inhibitory effects of neurotoxins, MPP+ and 6-OHDA on TRPC3 was mediated through alteration in the purinergic calcium signaling (Lei et al., 2008; Streifel et al., 2014). However, drugs targeting TRPC3 have shown neuroprotective effects further challenging the role of molecular mechanisms related to TRPC3 in PD.
Besides the channels discussed in detail, most studies have invariably found no change in the expression of other TRPC channels including TRPC4/5/6 in different PD models as well as patients (Selvaraj et al., 2012; Lortet et al., 2013; Sukumaran et al., 2018). But, their ability to regulate the influx of calcium and other cations into the cell makes these channels an attractive candidate for studies in future.
TRPML Involvement in PD
TRPML channels are non-selective cation channels localized intracellularly on the endosomes and lysosomes. These are responsible for the intracellular calcium influx after activation by different cellular pathways (Grimm et al., 2012). Amongst the members of TRPML subfamily, mutations in TRPML1 have also been linked to the lysosomal storage disorder, mucolipidosis type IV (MLIV) which is also a form of neurodegeneration. It is known to produce motor and cognitive deficits, similar to those seen in PD patients (Dong et al., 2008). Moreover, the role of TRPML1 in the autophagy-related signaling has also been elucidated, making it an important channel for cell survival. Although there is no direct evidence as of now for its involvement, it has been hypothesized that TRPML1 activation could restore the impaired autophagy seen in PD patients (Anglade et al., 1997). Furthermore, mutations in GBA gene, which codes for lysosomal enzyme glucocerebrosidase is one of the potential risk factors for PD and has also been known to cause lysosomal dysfunction (DePaolo et al., 2009). Therefore, there are several studies currently underway to investigate the possible role of TRPML channels in PD (The Michael J. Fox Foundation for Parkinson’s Research, 20191; The Michael J. Fox Foundation for Parkinson’s Research, 20202). These studies are expected to provide deeper insights into the pathophysiology of PD and help in the identification of new molecules which could be useful for PD management.
TRPA Involvement in PD
TRPA channel subfamily remains relatively unexplored in the context of PD. Though there is no study pertaining to its direct involvement, agents acting on these channels have provided important clues related to the development of side effects associated with current PD therapy. These have been discussed ahead in much more detail.
Potential Pharmacological Interventions Targeting TRP Channels in PD
A number of pharmacological agents have shown protective effects across different in vitro and in vivo studies. Detailed investigations pertaining to the beneficial effect of these agents has been discussed ahead and in Table 2.
TRPV1
A number of TRPV1 modulators have been studied by different groups in PD models. These include TRPV1 agonists (capsaicin and resiniferatoxin) as well as TRPV1 antagonists (capsazepine, AMG9810, oleoylethanolamide and iodo-resiniferatoxin). These agents exerted beneficial effects in the PD models. Capsaicin is an active phytochemical present in several Capsicum spp. which is highly selective toward TRPV1 channels (EC50 = 0.71 μM). It has been studied extensively for its disease-modifying effects in PD (Caterina et al., 1997; Musfiroh et al., 2013). It accorded neuroprotection in both the in vitro and in vivo rodent systems as well as in the drosophila model of PD. Capsaicin was further shown to improve rotarod performance and led to the restoration of dopaminergic signaling in the MPTP treated mice. Additionally, it also prevented glial cell activation and reduced oxidative stress in the astrocytes of MPTP induced PD mice. These effects were reversed after treatment with TRPV1 antagonists (capsazepine and iodo-resiniferatoxin) which confirmed that the beneficial effects were indeed attributed to the activation of TRPV1 channels (Chung et al., 2017). Similar observations were made in a study by Nam et al., which demonstrated that neuroprotective effects of capsaicin on TRPV1 were mediated through endogenous ciliary neurotrophic factor (CNTF) levels and CNTF-α receptors (Nam et al., 2015). It has been shown that the symptoms of PD first appear after 60–70% of the dopaminergic neuronal death has already taken place (Cheng et al., 2010). In a study, capsaicin was found to be capable of restoring the dopaminergic function even after the complete injury to the substantia nigra pars compacta (SNpc) and striatum (Kim et al., 2019). These results suggest the possibility for capsaicin to be effective in the later stages of PD when traditional medications fail to work. Besides, capsaicin also exerted a positive modulatory effect against oxidative insults and reversed the behavioral deficits induced by MPTP and 6-OHDA in the rodent models (Fong et al., 2016; Zhao et al., 2017). Capsaicin treated animals showed an increase in distance traveled in the open field and a significant reduction in the amphetamine-induced rotations after induction of PD. It was accompanied by a significant reduction in the malondialdehyde (MDA) levels and restoration of the superoxide dismutase (SOD) and catalase activity in the SNpc (Nam et al., 2015; Zhao et al., 2017).
The neuroprotective effects of capsaicin were further replicated in the drosophila fly mutants, which expressed a gene for human α-synuclein. These PD flies displayed a progressive form of neurodegeneration characterized by loss of dopamine function, oxidative stress and impaired performance in the climbing assay. Reversal of the pathological changes occurred in a dose-dependent manner which confirmed the therapeutic potential of capsaicin through TRPV1 activation for PD treatment (Siddique et al., 2012, 2018).
In contrast to these studies, other groups have shown that blockade of TRPV1 channels is also helpful in relieving some of the PD symptoms. A study involving the use of AMG9810, a selective TRPV1 blocker demonstrated a positive attenuation of the motor deficits after 6-OHDA administration which was evident from the rotarod test. Additionally, cresyl violet staining also showed that there was reduced neuronal death in the SNpc of the AMG9810 treated animals (Razavinasab et al., 2013). In line with the same, another TRPV1 blocker, capsazepine also exhibited neuroprotective effects in a number of PD models. Moreover, it was also found to reduce the occurrence of levodopa (L-DOPA) induced dyskinesia which is a prominent side effect seen with L-DOPA therapy in PD (Morgese et al., 2007). The most probable explanation for these effects came from a study where it was observed that TRPV1 blockade positively regulates the crosstalk between endocannabinoid receptors and TRPV1 downstream signaling which helps prevent L-DOPA induced dyskinesias (Lee et al., 2006; Giuffrida and Morgese, 2007; Morgese et al., 2007; Dos-Santos-Pereira et al., 2016). It is also supported by the findings in which oleoylethanolamide, another TRPV1 blocker showed protection in the 6-OHDA induced hemiparkinsonian model (González-Aparicio and Moratalla, 2014). Furthermore, blockade of TRPV1 has also been elucidated to play a crucial role in the modulation of pain pathways in PD (Li et al., 2020). TRPV1 expression was upregulated in the trigeminal subnucleus caudalis (Vc) which plays a role in central terminal sensitization of primary nociceptive neurons. In addition, its co-expression with 5-HT3A receptors in the rat dorsal root ganglion (DRG) and ability of TRPV1 blockers to inhibit the pain induced by 5-HT3A receptor agonists makes it a useful target for pain modulation in PD (Zeitz et al., 2002; Li et al., 2020).
Despite these promising results, the therapeutic window for the beneficial effects of TRPV1 modulators is very narrow and higher doses of TRPV1 blockers such as AMG9810 have been associated with learning and memory impairments in the preclinical studies (Razavinasab et al., 2013). Moreover, its interaction with endocannabinoids raised concern over the possible addictive potential and development of tolerance for these agents. However, when investigated, no such association was observed in studies involving TRPV1 blockers which makes them a viable option for studies in future (González-Aparicio and Moratalla, 2014).
In totality, these shreds of evidence suggested that targeting TRPV1 channels could be of therapeutic relevance for combating the motor and non-motor symptoms of PD. Therefore, to provide more proof to the speculation, Capsaicin is being investigated in patients for the elucidation of mechanisms related to swallowing and cough dysfunction in PD3. Another TRPV1 agonist, resiniferatoxin was shown to be effective in controlling the motor symptoms in the AAV-A53T knock-in PD mouse model (Sorrento Therapeutics Inc, 2020). According to the recent reports, it is soon expected to enter clinical trials which could open up new avenues of research for the management of PD.
Together, these studies capture a basic insight into the pharmacological modulation of TRPV channels which could be targeted in future for the treatment of PD. But, as the doubts over the clear molecular mechanisms, clinical efficacy and specificity persist, more detailed investigations are still necessary for the development of TRPV channel modulators as drug candidates in future.
TRPM2
Studies conducted in preclinical models as well as PD patients, showed an increase in TRPM2 expression, suggesting its possible involvement in PD (Sun et al., 2018b; An et al., 2019). It was also demonstrated that MPP+ exposure leads to increased levels of TRPM2 accompanied by elevated oxidative stress and increased apoptosis in the SH-SY5Y neurons. It further resulted in reduced cell viability and caspase 3 activation in these neuronal cells (An et al., 2019; Ding et al., 2019). These effects were reversed by treatment with TRPM2 blockers, flufenamic acid (FFA) and N-(p-amylcinnamoyl) anthranilic acid (ACA). Moreover, similar results were obtained when knockdown of TRPM2 was carried out using a siRNA which resulted in reduced neuronal death and inhibition of apoptosis in the SH-SY5Y cells (Sun et al., 2018b). The detailed mechanism of this neuroprotection was delineated later in another study which attributed it to the working network of long non-coding RNA, p21 and microRNA, miR-625 present inside the neurons. It was also reported that long non-coding RNA, p21 is a positive modulator of TRPM2 expression and its knockdown could rescue from the toxicity induced by MPP+ treatment (Ding et al., 2019).
Other authors have also confirmed the involvement of TRPM2 in PD with the help of different toxin-based models in vitro and in vivo. In a study by Yu et al. (2014) it was shown that TRPM2 expression was significantly increased in the SNpc of 6-OHDA treated rats. This increased expression was attenuated by treatment with nerve growth factor, which accorded neuroprotection through phosphatidylinositol 3-kinase (PI3K) signaling pathway (Yu et al., 2014). Furthermore, it was demonstrated that TRPM2 mediated increased ROS production and caspase 3 activity was suppressed by increased expression of transcription factor GATA3 binding protein (GATA3) (Zhou and Han, 2017). This suggests the involvement of multiple downstream pathways affected by TRPM2 associated neuronal death in PD. In line with these shreds of evidence, rotenone also led to increased TRPM2 activation by ROS dependent mechanisms (Freestone, 2009). These effects were reversed after treatment with TRPM2 blockers FFA and 2-aminoethoxydiphenyl borate (2-APB), which confirmed the potential of TRPM2 blockade in the treatment of PD (Nazıroğlu et al., 2011). Furthermore, electrophysiological findings have revealed that increased spontaneous firing of the substantia nigra pars reticulata (SNr) GABAergic neurons seen in PD is modulated by increased activation of the TRPM2 channels (Lee et al., 2013). Conclusively, the close association of TRPM2 and intracellular ionic homeostasis has been established and any change in it makes the individual more susceptible toward PD (Hermosura and Garruto, 2007). Though there is ample evidence available for its involvement, there is no report which has investigated the effect of pharmacological interventions targeting TRPM2 in vivo. This has largely hindered the development of more potent analogs which could be tried for their translational potential of TRPM2 in PD. Further, the neuroprotective potential of TRPM2 antagonists in neurodegenerative disorders like AD strengthens the claim of TRPM2 antagonists as neuroprotective agents (Thapak et al., 2020b).
TRPC1
Silencing of TRPC1 as well as its functional blockade was also associated with mitochondrial dysfunction and decrease in the TH levels after sub-chronic administration of MPTP to the C57BL/6 mice. These pathological changes were reversed after treatment with TRPC1 activators carbachol and thapsigargin or after TRPC1 overexpression which led to an increase in anti-apoptotic signaling (Selvaraj et al., 2009). Similar results were observed in other studies involving transgenic or toxin model-based systems (Bollimuntha et al., 2005; Sukumaran et al., 2018).
TRPC3/6/7
Studies have either reported reduced or no change in the expression of TRPC3/6/7 channels in PD (Selvaraj et al., 2009, 2012; Yu et al., 2013; Streifel et al., 2014). However, the neuroprotective effects of SKF-96365, a non-selective TRPC3/6/7 blocker in the MPP+ model suggests a need to validate these findings (Chen et al., 2013). Therefore, challenging the reproducibility of these observations in other model systems could help resolve these concerns in the near future.
TRPA1
Expression of TRPA channels has not been directly correlated to the pathophysiology of PD. However, it was observed that apomorphine which is an approved medication for PD is a bimodal modulator of TRPA1 channels, the only known member of the TRPA subfamily (Schulze et al., 2013). Apomorphine is often used as an add-on therapy for the management of “off” episodes in PD but exhibits adverse effects like nausea and local reactions at the injection site (Carbone et al., 2019). Schulze et al. (2013) demonstrated with the help of cultured dorsal route ganglion (DRG) neurons and in the enterochromaffin model cell line QGP-1, that these adverse effects might be attributed to the activation of TRPA1 channels by apomorphine. Therefore, strategies targeting TRPA1 channels could be useful for the management of side-effects associated with apomorphine and other currently available medications for PD.
Challenges in the Development of TRP Channel Modulators as Drug Candidates
TRP channels are involved in the regulation of a number of physiological processes in different organs of the body. Though alteration of their functions in some of the brain areas may be involved in the pathophysiology of PD, targeting these alterations in a selective and specific manner poses a greater challenge. This was evident during the studies of TRPV1 modulators in other conditions like pain where a number of clinical trials were terminated because of the off-target effects (Krarup et al., 2011; Rowbotham et al., 2011). Encouraging results obtained in rodent studies with these agents couldn’t be replicated in patients due to a number of side effects like a diminished response to damaging heat, alteration in body temperature and reduced perception of taste were observed (Gavva et al., 2008). Therefore, management of safety and toxicity still remain the biggest hurdle for the translational success of TRP channel modulators. Another largely neglected area of research is the organellar distribution of TRP channels where they are coupled together to perform a plethora of unknown functions (Zhang et al., 2018). Besides, a report has also highlighted that the neuroprotection accorded by these agents is sex-specific which adds further complexity to the clinical development of these agents (Jia et al., 2011). Additionally, a number of agonists and antagonists of the same TRPs like TRPV1 and TRPM8 are together being investigated in the clinical trials which tell us that there is a lot more to the story than what we understand at present (Moran et al., 2011; De Caro et al., 2019; Garami et al., 2020). This further raises the possibility of these modulators to be working through other unknown mechanisms that have not been investigated till date. To develop the understanding, several transgenic mice (TRP knock in and knock out) were made, but high embryonic or postnatal lethality has largely limited their use. This has further pushed back the progress of this field and necessitated the use of alternative approaches (Park et al., 2011; Woudenberg-Vrenken et al., 2011). Another pitfall in TRP research is the lack of selective agents as activators or inhibitors for any of the ion channel. As the structure and function of TRP channels are conserved across species, preclinical and animal data using selective agents could be highly useful for the further development of these agents (Hof et al., 2019). Therefore, increased knowledge of the TRP structure is also required and needs to be studied with the help of new approaches.
Despite these limitations, there are a number of clinically approved agents like probenecid and tranilast (TRPV2), glibenclamide (TRPM4), flufenamic acid and clotrimazole (TRPM2) and menthol (TRPM8) which have also been reported to modulate TRP channel activity. There are proof of concept studies which have found them to be effective in different CNS disorders (Thapak et al., 2020a; Zubov et al., 2020). Hence, it is possible that TRP channels may be indistinctly important for brain functions, and these molecules may pave the way for a completely new class of drugs for brain-related disorders including PD.
Conclusion
TRP channels perform a plethora of physiological functions in the CNS. The increased understanding of their role has led several researchers to investigate their likely contribution in the pathophysiology of neurodegenerative disorders, including PD. Moreover, their regulatory function in ionic homeostasis has made TRP channels an attractive pharmacological target where Ca2+ dyshomeostasis, oxidative stress and excitotoxicity mediated neuronal death are reported. The current review has presented a brief overview of the reports which have studied the pathophysiological involvement of TRP channels in PD. Additionally, beneficial effects of many of the pharmacological agents targeting TRP channels have also been highlighted, which suggests the possibility of a novel class of therapeutics for PD treatment in future. Amongst the agents known so far, ones targeting TRPV1, TRPM2 and TRPC1 have been most promising for PD treatment. Design of novel and selective analogs targeting these TRPs is expected to open up new avenues of research in future. Though these findings pertaining to the involvement of TRP channels in PD are encouraging, more studies highlighting the molecular mechanisms at length are required to ensure the translational success of these compounds in the clinical trials.
Author Contributions
BV and SSS conceived the idea of the manuscript. BV wrote all the sections. SSS corrected the manuscript. Both authors approved the final proof for submission.
Conflict of Interest
The authors declare that the research was conducted in the absence of any commercial or financial relationships that could be construed as a potential conflict of interest.
Acknowledgments
We would like to acknowledge fellowship support provided by The Council of Scientific and Industrial Research to BV.
Footnotes
- ^ https://www.michaeljfox.org/grant/assessing-trpml1-agonists-gba-parkinsons-disease
- ^ https://www.michaeljfox.org/grant/development-novel-trpml1-activator-parkinsons-disease
- ^ https://clinicaltrials.gov/ct2/show/NCT03321019
References
Adhya, P., and Sharma, S. S. (2019). Redox TRPs in diabetes and diabetic complications: Mechanisms and pharmacological modulation. Pharmacol. Res. 146:104271. doi: 10.1016/j.phrs.2019.104271
Akyuva, Y., and Naziroglu, M. (2020). Resveratrol attenuates hypoxia-induced neuronal cell death, inflammation and mitochondrial oxidative stress by modulation of TRPM2 channel. Sci. Rep. 10:6449. doi: 10.1038/s41598-020-63577-5
An, X., Fu, Z., Mai, C., Wang, W., Wei, L., Li, D., et al. (2019). Increasing the TRPM2 Channel Expression in Human Neuroblastoma SH-SY5Y Cells Augments the Susceptibility to ROS-Induced Cell Death. Cells 8:28. doi: 10.3390/cells8010028
Anglade, P., Vyas, S., Javoy-Agid, F., Herrero, M. T., Michel, P. P., Marquez, J., et al. (1997). Apoptosis and autophagy in nigral neurons of patients with Parkinson’s disease. Histol Histopathol 12, 25–31.
Bashiri, H., Hosseini-Chegeni, H., Alsadat Sharifi, K., Sahebgharani, M., and Salari, A. A. (2018). Activation of TRPV1 receptors affects memory function and hippocampal TRPV1 and CREB mRNA expression in a rat model of biliary cirrhosis. Neurol. Res. 40, 938–947. doi: 10.1080/01616412.2018.1504158
Beiske, A. G., Loge, J. H., Rønningen, A., and Svensson, E. (2009). Pain in Parkinson’s disease: Prevalence and characteristics. Pain 141, 173–177. doi: 10.1016/j.pain.2008.12.004
Belrose, J. C., and Jackson, M. F. (2018). TRPM2: a candidate therapeutic target for treating neurological diseases. Acta Pharmacol. Sin. 39, 722–732. doi: 10.1038/aps.2018.31
Bodkin, J. V., Thakore, P., Aubdool, A. A., Liang, L., Fernandes, E. S., Nandi, M., et al. (2014). Investigating the potential role of TRPA1 in locomotion and cardiovascular control during hypertension. Pharmacol. Res. Perspect. 2:e00052. doi: 10.1002/prp2.52
Bollimuntha, S., Singh, B. B., Shavali, S., Sharma, S. K., and Ebadi, M. (2005). TRPC1-mediated inhibition of 1-methyl-4-phenylpyridinium ion neurotoxicity in human SH-SY5Y neuroblastoma cells. J. Biol. Chem. 280, 2132–2140. doi: 10.1074/jbc.M407384200
Carbone, F., Djamshidian, A., Seppi, K., and Poewe, W. (2019). Apomorphine for Parkinson’s Disease: Efficacy and Safety of Current and New Formulations. CNS Drugs 33, 905–918. doi: 10.1007/s40263-019-00661-z
Caterina, M. J., Schumacher, M. A., Tominaga, M., Rosen, T. A., Levine, J. D., and Julius, D. (1997). The capsaicin receptor: a heat-activated ion channel in the pain pathway. Nature 389, 816–824. doi: 10.1038/39807
Chen, B., Ng, G., Gao, Y., Low, S. W., Sandanaraj, E., Ramasamy, B., et al. (2019). Non-Invasive Multimodality Imaging Directly Shows TRPM4 Inhibition Ameliorates Stroke Reperfusion Injury. Transl. Stroke Res. 10, 91–103. doi: 10.1007/s12975-018-0621-3
Chen, T., Zhu, J., Zhang, C., Huo, K., Fei, Z., and Jiang, X. F. (2013). Protective effects of SKF-96365, a non-specific inhibitor of SOCE, against MPP+-induced cytotoxicity in PC12 cells: potential role of Homer1. PLoS One 8:e55601. doi: 10.1371/journal.pone.0055601
Cheng, H. C., Ulane, C. M., and Burke, R. E. (2010). Clinical progression in Parkinson disease and the neurobiology of axons. Ann. Neurol. 67, 715–725. doi: 10.1002/ana.21995
Chung, Y. C., Baek, J. Y., Kim, S. R., Ko, H. W., Bok, E., Shin, W. H., et al. (2017). Capsaicin prevents degeneration of dopamine neurons by inhibiting glial activation and oxidative stress in the MPTP model of Parkinson’s disease. Exp. Mol. Med. 49:e298. doi: 10.1038/emm.2016.159
Chung, Y. H., Kim, D., Moon, N. J., Oh, C. S., Lee, E., Shin, D. H., et al. (2007). Immunohistochemical study on the distribution of canonical transient receptor potential channels in rat basal ganglia. Neurosci. Lett. 422, 18–23. doi: 10.1016/j.neulet.2007.05.042
Cosens, D. J., and Manning, A. (1969). Abnormal electroretinogram from a Drosophila mutant. Nature 224, 285–287. doi: 10.1038/224285a0
Das, N. R., and Sharma, S. S. (2015). Peroxisome Proliferator Activated Receptor Gamma Coactivator 1 Alpha: An Emerging Target for Neuroprotection in Parkinson’s Disease. CNS Neurol. Disord. Drug Targets 14, 1024–1030. doi: 10.2174/1871527314666150326000018
De Caro, C., Cristiano, C., Avagliano, C., Bertamino, A., Ostacolo, C., Campiglia, P., et al. (2019). Characterization of New TRPM8 Modulators in Pain Perception. Int. J. Mol. Sci. 20:5544. doi: 10.3390/ijms20225544
Deng, J., and Bai, J. (2012). The role of TRPC1 and Ca∼(2+) in the endoplasmic reticulum stress of Parkinson’s disease. Chin. Bull. Life Sci. 2012:15.
Denis, V., and Cyert, M. S. (2002). Internal Ca(2+) release in yeast is triggered by hypertonic shock and mediated by a TRP channel homologue. J. Cell Biol. 156, 29–34. doi: 10.1083/jcb.200111004
DePaolo, J., Goker-Alpan, O., Samaddar, T., Lopez, G., and Sidransky, E. (2009). The association between mutations in the lysosomal protein glucocerebrosidase and parkinsonism. Mov. Disord. 24, 1571–1578. doi: 10.1002/mds.22538
Ding, X. M., Zhao, L. J., Qiao, H. Y., Wu, S. L., and Wang, X. H. (2019). Long non-coding RNA-p21 regulates MPP(+)-induced neuronal injury by targeting miR-625 and derepressing TRPM2 in SH-SY5Y cells. Chem. Biol. Interact 307, 73–81. doi: 10.1016/j.cbi.2019.04.017
Dong, X. P., Cheng, X., Mills, E., Delling, M., Wang, F., Kurz, T., et al. (2008). The type IV mucolipidosis-associated protein TRPML1 is an endolysosomal iron release channel. Nature 455, 992–996. doi: 10.1038/nature07311
Dos-Santos-Pereira, M., Da-Silva, C. A., Guimarães, F. S., and Del-Bel, E. (2016). Co-administration of cannabidiol and capsazepine reduces L-DOPA-induced dyskinesia in mice: Possible mechanism of action. Neurobiol. Dis. 94, 179–195. doi: 10.1016/j.nbd.2016.06.013
Fonfria, E., Marshall, I. C., Boyfield, I., Skaper, S. D., Hughes, J. P., Owen, D. E., et al. (2005). Amyloid beta-peptide(1-42) and hydrogen peroxide-induced toxicity are mediated by TRPM2 in rat primary striatal cultures. J. Neurochem. 95, 715–723. doi: 10.1111/j.1471-4159.2005.03396.x
Fonfria, E., Murdock, P. R., Cusdin, F. S., Benham, C. D., Kelsell, R. E., and Mcnulty, S. (2006). Tissue distribution profiles of the human TRPM cation channel family. J. Recept. Signal. Transduct. Res. 26, 159–178. doi: 10.1080/10799890600637506
Fong, G. M., Johnstone, D., Antao, S., Stone, J., and Witting, P. (2016). Mechanism of Neuroprotection by Capsaicin in a Model of Parkinson’s Disease. Free Radical. Bio. Med. 100:S54. doi: 10.1016/j.freeradbiomed.2016.10.141
Freestone, P. S. (2009). Effects of rotenone and 6-OHDA on dopaminergic neurons of the Substantia nigra studied in vitro, Doctor of Philosophy. Auckland: University of Auckland.
Garami, A., Shimansky, Y. P., Rumbus, Z., Vizin, R. C. L., Farkas, N., Hegyi, J., et al. (2020). Hyperthermia induced by transient receptor potential vanilloid-1 (TRPV1) antagonists in human clinical trials: Insights from mathematical modeling and meta-analysis. Pharmacol. Ther. 208:107474. doi: 10.1016/j.pharmthera.2020.107474
Gavva, N. R. (2009). Setbacks in the clinical development of TRPV1 antagonists: what next? Open Drug Discover. J. 1, 1–35. doi: 10.2174/1877381800901010001
Gavva, N. R., Treanor, J. J., Garami, A., Fang, L., Surapaneni, S., Akrami, A., et al. (2008). Pharmacological blockade of the vanilloid receptor TRPV1 elicits marked hyperthermia in humans. Pain 136, 202–210. doi: 10.1016/j.pain.2008.01.024
Giuffrida, A., and Morgese, M. G. (2007). Direct and indirect cannabinoid agonists reduce L-dopa-induced-dyskinesias via CB1-and TRPV1-mediated mechanisms. Feder. Am. Soc. Exp. Biol. 21, A787–A787.
González-Aparicio, R., and Moratalla, R. (2014). Oleoylethanolamide reduces L-DOPA-induced dyskinesia via TRPV1 receptor in a mouse model of Parkinson’s disease. Neurobiol. Dis. 62, 416–425. doi: 10.1016/j.nbd.2013.10.008
Grimm, C., Hassan, S., Wahl-Schott, C., and Biel, M. (2012). Role of TRPML and two-pore channels in endolysosomal cation homeostasis. J. Pharmacol. Exp. Ther. 342, 236–244. doi: 10.1124/jpet.112.192880
Guatteo, E., Chung, K. K., Bowala, T. K., Bernardi, G., Mercuri, N. B., and Lipski, J. (2005). Temperature sensitivity of dopaminergic neurons of the substantia nigra pars compacta: involvement of transient receptor potential channels. J. Neurophysiol. 94, 3069–3080. doi: 10.1152/jn.00066.2005
Hardie, R. C. (2007). TRP channels and lipids: from Drosophila to mammalian physiology. J. Physiol. 578, 9–24. doi: 10.1113/jphysiol.2006.118372
Hartmann, J., Dragicevic, E., Adelsberger, H., Henning, H. A., Sumser, M., Abramowitz, J., et al. (2008). TRPC3 channels are required for synaptic transmission and motor coordination. Neuron 59, 392–398. doi: 10.1016/j.neuron.2008.06.009
He, K., Qi, F., Guo, C., Zhan, S., Xu, H., Liu, J., et al. (2016). Movement deficits and neuronal loss in basal ganglia in TRPC1 deficient mice. Oncotarget 7, 69337–69346. doi: 10.18632/oncotarget.12567
Hermosura, M. C., and Garruto, R. M. (2007). TRPM7 and TRPM2-Candidate susceptibility genes for Western Pacific ALS and PD? Biochim. Biophys. Acta 1772, 822–835. doi: 10.1016/j.bbadis.2007.02.008
Hermosura, M. C., Nayakanti, H., Dorovkov, M. V., Calderon, F. R., Ryazanov, A. G., Haymer, D. S., et al. (2005). A TRPM7 variant shows altered sensitivity to magnesium that may contribute to the pathogenesis of two Guamanian neurodegenerative disorders. Proc. Natl. Acad. Sci. U S A. 102, 11510–11515. doi: 10.1073/pnas.0505149102
Hof, T., Chaigne, S., Récalde, A., Sallé, L., Brette, F., and Guinamard, R. (2019). Transient receptor potential channels in cardiac health and disease. Nat. Rev. Cardiol. 16, 344–360. doi: 10.1038/s41569-018-0145-2
Jia, J., Verma, S., Nakayama, S., Quillinan, N., Grafe, M. R., Hurn, P. D., et al. (2011). Sex differences in neuroprotection provided by inhibition of TRPM2 channels following experimental stroke. J. Cereb. Blood Flow Metab. 31, 2160–2168. doi: 10.1038/jcbfm.2011.77
Kaneko, Y., and Szallasi, A. (2014). Transient receptor potential (TRP) channels: a clinical perspective. Br. J. Pharmacol. 171, 2474–2507. doi: 10.1111/bph.12414
Kim, K. I., Baek, J. Y., Jeong, J. Y., Nam, J. H., Park, E. S., Bok, E., et al. (2019). Delayed Treatment of Capsaicin Produces Partial Motor Recovery by Enhancing Dopamine Function in MPP(+)-lesioned Rats via Ciliary Neurotrophic Factor. Exp. Neurobiol. 28, 289–299. doi: 10.5607/en.2019.28.2.289
Krarup, A. L., Ny, L., Astrand, M., Bajor, A., Hvid-Jensen, F., Hansen, M. B., et al. (2011). Randomised clinical trial: the efficacy of a transient receptor potential vanilloid 1 antagonist AZD1386 in human oesophageal pain. Aliment Pharmacol. Ther. 33, 1113–1122. doi: 10.1111/j.1365-2036.2011.04629.x
Kumar, P., Kaundal, R. K., More, S., and Sharma, S. S. (2009). Beneficial effects of pioglitazone on cognitive impairment in MPTP model of Parkinson’s disease. Behav. Brain Res. 197, 398–403. doi: 10.1016/j.bbr.2008.10.010
Kumar, S., Singh, U., Goswami, C., and Singru, P. S. (2017). Transient receptor potential vanilloid 5 (TRPV5), a highly Ca(2+)-selective TRP channel in the rat brain: relevance to neuroendocrine regulation. J. Neuroendocrinol. 29:28235149. doi: 10.1111/jne.12466
Kunert-Keil, C., Bisping, F., Krüger, J., and Brinkmeier, H. (2006). Tissue-specific expression of TRP channel genes in the mouse and its variation in three different mouse strains. BMC Genomics 7:159. doi: 10.1186/1471-2164-7-159
Lee, C. R., Machold, R. P., Witkovsky, P., and Rice, M. E. (2013). TRPM2 channels are required for NMDA-induced burst firing and contribute to H(2)O(2)-dependent modulation in substantia nigra pars reticulata GABAergic neurons. J. Neurosci. 33, 1157–1168. doi: 10.1523/jneurosci.2832-12.2013
Lee, J., Di Marzo, V., and Brotchie, J. M. (2006). A role for vanilloid receptor 1 (TRPV1) and endocannabinnoid signalling in the regulation of spontaneous and L-DOPA induced locomotion in normal and reserpine-treated rats. Neuropharmacology 51, 557–565. doi: 10.1016/j.neuropharm.2006.04.016
Lee, N., Chen, J., Sun, L., Wu, S., Gray, K. R., Rich, A., et al. (2003). Expression and characterization of human transient receptor potential melastatin 3 (hTRPM3). J. Biol. Chem. 278, 20890–20897. doi: 10.1074/jbc.M211232200
Lehen’kyi, V., Raphaël, M., and Prevarskaya, N. (2012). The role of the TRPV6 channel in cancer. J. Physiol. 590, 1369–1376. doi: 10.1113/jphysiol.2011.225862
Lei, Z., Junfeng, W., Ruiliang, G., and Xiaomin, W. (2008). TRPC3-mediated Inhibition of MPP∼+ Neurotoxicity in MN9D Cells. J. Cap. Med. Univ. 2008:15.
Li, B., Xiao, L., Wang, Z. Y., and Zheng, P. S. (2014). Knockdown of STIM1 inhibits 6-hydroxydopamine-induced oxidative stress through attenuating calcium-dependent ER stress and mitochondrial dysfunction in undifferentiated PC12 cells. Free Radic. Res. 48, 758–768. doi: 10.3109/10715762.2014.905687
Li, H. (2017). TRP Channel Classification. Adv. Exp. Med. Biol. 976, 1–8. doi: 10.1007/978-94-024-1088-4_1
Li, J., Sun, Y., and Chen, J. (2019). Transcriptome sequencing in a 6-hydroxydopamine rat model of Parkinson’s disease. Genes Genet. Syst. 94, 61–69. doi: 10.1266/ggs.18-00036
Li, M., Zhu, M., Xu, Q., Ding, F., Tian, Y., and Zhang, M. (2020). Sensation of TRPV1 via 5-hydroxytryptamine signaling modulates pain hypersensitivity in a 6-hydroxydopamine induced mice model of Parkinson’s disease. Biochem. Biophys. Res. Commun. 521, 868–873. doi: 10.1016/j.bbrc.2019.10.204
Lortet, S., Lacombe, E., Boulanger, N., Rihet, P., Nguyen, C., Kerkerian-Le Goff, L., et al. (2013). Striatal molecular signature of subchronic subthalamic nucleus high frequency stimulation in parkinsonian rat. PLoS One 8:e60447. doi: 10.1371/journal.pone.0060447
Martorana, A., Giampà, C., Demarch, Z., Viscomi, M. T., Patassini, S., Sancesario, G., et al. (2006). Distribution of TRPC1 receptors in dendrites of rat substantia nigra: a confocal and electron microscopy study. Eur. J. Neurosci. 24, 732–738. doi: 10.1111/j.1460-9568.2006.04932.x
Moran, M. M., Mcalexander, M. A., Bíró, T., and Szallasi, A. (2011). Transient receptor potential channels as therapeutic targets. Nat. Rev. Drug. Discov. 10, 601–620. doi: 10.1038/nrd3456
Morgese, M. G., Cassano, T., Cuomo, V., and Giuffrida, A. (2007). Anti-dyskinetic effects of cannabinoids in a rat model of Parkinson’s disease: role of CB(1) and TRPV1 receptors. Exp. Neurol. 208, 110–119. doi: 10.1016/j.expneurol.2007.07.021
Mrejeru, A., Wei, A., and Ramirez, J. M. (2011). Calcium-activated non-selective cation currents are involved in generation of tonic and bursting activity in dopamine neurons of the substantia nigra pars compacta. J. Physiol. 589, 2497–2514. doi: 10.1113/jphysiol.2011.206631
Musella, A., De Chiara, V., Rossi, S., Prosperetti, C., Bernardi, G., Maccarrone, M., et al. (2009). TRPV1 channels facilitate glutamate transmission in the striatum. Mol. Cell Neurosci. 40, 89–97. doi: 10.1016/j.mcn.2008.09.001
Musfiroh, I., Mutakin, M., Angelina, T., and Muchtaridi, M. (2013). Capsaicin level of various capsicum fruits. Int. J. Pharm. Pharm. Sci. 5, 248–251.
Nam, J. H., Park, E. S., Won, S. Y., Lee, Y. A., Kim, K. I., Jeong, J. Y., et al. (2015). TRPV1 on astrocytes rescues nigral dopamine neurons in Parkinson’s disease via CNTF. Brain 138, 3610–3622. doi: 10.1093/brain/awv297
Nazıroğlu, M., Özgül, C., Çelik, Ö, Çiğ, B., and Sözbir, E. (2011). Aminoethoxydiphenyl borate and flufenamic acid inhibit Ca2+ influx through TRPM2 channels in rat dorsal root ganglion neurons activated by ADP-ribose and rotenone. J. Membr. Biol. 241, 69–75. doi: 10.1007/s00232-011-9363-9
Ordás, P., Hernández-Ortego, P., Vara, H., Fernández-Peña, C., Reimúndez, A., Morenilla-Palao, C., et al. (2019). Expression of the cold thermoreceptor TRPM8 in rodent brain thermoregulatory circuits. J. Comp. Neurol. 2019:30942489. doi: 10.1002/cne.24694
Ostapchenko, V. G., Chen, M., Guzman, M. S., Xie, Y. F., Lavine, N., Fan, J., et al. (2015). The Transient Receptor Potential Melastatin 2 (TRPM2) Channel Contributes to β-Amyloid Oligomer-Related Neurotoxicity and Memory Impairment. J. Neurosci. 35, 15157–15169. doi: 10.1523/jneurosci.4081-14.2015
Park, U., Vastani, N., Guan, Y., Raja, S. N., Koltzenburg, M., and Caterina, M. J. (2011). TRP vanilloid 2 knock-out mice are susceptible to perinatal lethality but display normal thermal and mechanical nociception. J. Neurosci. 31, 11425–11436. doi: 10.1523/jneurosci.1384-09.2011
Parkinson’s News Today. (2020). Parkinsons disease statistics. Available online at: https://parkinsonsnewstoday.com/parkinsons-disease-statistics/ (accessed on July 13, 2020).
Razavinasab, M., Shamsizadeh, A., Shabani, M., Nazeri, M., Allahtavakoli, M., Asadi-Shekaari, M., et al. (2013). Pharmacological blockade of TRPV1 receptors modulates the effects of 6-OHDA on motor and cognitive functions in a rat model of Parkinson’s disease. Fundam Clin. Pharmacol. 27, 632–640. doi: 10.1111/fcp.12015
Roberts, J. C., Davis, J. B., and Benham, C. D. (2004). [3H]Resiniferatoxin autoradiography in the CNS of wild-type and TRPV1 null mice defines TRPV1 (VR-1) protein distribution. Brain Res. 995, 176–183. doi: 10.1016/j.brainres.2003.10.001
Rowbotham, M. C., Nothaft, W., Duan, W. R., Wang, Y., Faltynek, C., Mcgaraughty, S., et al. (2011). Oral and cutaneous thermosensory profile of selective TRPV1 inhibition by ABT-102 in a randomized healthy volunteer trial. Pain 152, 1192–1200. doi: 10.1016/j.pain.2011.01.051
Samanta, A., Hughes, T. E. T., and Moiseenkova-Bell, V. Y. (2018). Transient Receptor Potential (TRP) Channels. Subcell Biochem. 87, 141–165. doi: 10.1007/978-981-10-7757-9_6
Schulze, A., Oehler, B., Urban, N., Schaefer, M., and Hill, K. (2013). Apomorphine is a bimodal modulator of TRPA1 channels. Mol. Pharmacol. 83, 542–551. doi: 10.1124/mol.112.081976
Selvaraj, S., Sun, Y., Watt, J. A., Wang, S., Lei, S., Birnbaumer, L., et al. (2012). Neurotoxin-induced ER stress in mouse dopaminergic neurons involves downregulation of TRPC1 and inhibition of AKT/mTOR signaling. J. Clin. Invest. 122, 1354–1367. doi: 10.1172/jci61332
Selvaraj, S., Watt, J. A., and Singh, B. B. (2009). TRPC1 inhibits apoptotic cell degeneration induced by dopaminergic neurotoxin MPTP/MPP(+). Cell Calcium. 46, 209–218. doi: 10.1016/j.ceca.2009.07.008
Shibasaki, K., Ishizaki, Y., and Mandadi, S. (2013). Astrocytes express functional TRPV2 ion channels. Biochem. Biophys. Res. Commun. 441, 327–332. doi: 10.1016/j.bbrc.2013.10.046
Shindo, Y., Yamanaka, R., Suzuki, K., Hotta, K., and Oka, K. (2015). Intracellular magnesium level determines cell viability in the MPP(+) model of Parkinson’s disease. Biochim. Biophys. Acta 1853, 3182–3191. doi: 10.1016/j.bbamcr.2015.08.013
Siddique, Y. H., Naz, F., and Jyoti, S. (2018). Effect of capsaicin on the oxidative stress and dopamine content in the transgenic Drosophila model of Parkinson’s disease. Acta Biol. Hung 69, 115–124. doi: 10.1556/018.69.2018.2.1
Siddique, Y., Ara, G., Jyoti, S., and Afzal, M. (2012). Effect of capsaicin on the climbing ability in Drosophila model of Parkinson’s disease. Am. J. Drug Discov. Dev. 2, 50–54. doi: 10.3923/ajdd.2012.50.54
Simuni, T., Borushko, E., Avram, M. J., Miskevics, S., Martel, A., Zadikoff, C., et al. (2010). Tolerability of isradipine in early Parkinson’s disease: a pilot dose escalation study. Mov. Disord. 25, 2863–2866. doi: 10.1002/mds.23308
Skogar, O., and Lokk, J. (2016). Pain management in patients with Parkinson’s disease: challenges and solutions. J. Multidiscip. Healthc. 9, 469–479. doi: 10.2147/jmdh.s105857
Sorrento Therapeutics Inc. (2020). Sorrento Therapeutics Announces Discovery of a Potential Non-Dopaminergic Approach to Controlling Parkinson’s Motor Symptoms With Resiniferatoxin (RTX) Intrathecal Administration. California: Sorrento Therapeutics, Inc. Available online at: https://investors.sorrentotherapeutics.com/node/10481/pdf (accessed on May 6, 2020).
Steinberg, X., Lespay-Rebolledo, C., and Brauchi, S. (2014). A structural view of ligand-dependent activation in thermoTRP channels. Front. Physiol. 5:171. doi: 10.3389/fphys.2014.00171
Streifel, K. M., Gonzales, A. L., De Miranda, B., Mouneimne, R., Earley, S., and Tjalkens, R. (2014). Dopaminergic neurotoxicants cause biphasic inhibition of purinergic calcium signaling in astrocytes. PLoS One 9:e110996. doi: 10.1371/journal.pone.0110996
Sukumaran, P., Sun, Y., Antonson, N., and Singh, B. B. (2018). Dopaminergic neurotoxins induce cell death by attenuating NF-κB-mediated regulation of TRPC1 expression and autophagy. FASEB J. 32, 1640–1652. doi: 10.1096/fj.201700662RR
Sukumaran, P., Sun, Y., Schaar, A., Selvaraj, S., and Singh, B. B. (2017). TRPC Channels and Parkinson’s Disease. Adv. Exp. Med. Biol. 976, 85–94. doi: 10.1007/978-94-024-1088-4_8
Sun, Y., Selvaraj, S., Pandey, S., Humphrey, K. M., Foster, J. D., Wu, M., et al. (2018a). MPP(+) decreases store-operated calcium entry and TRPC1 expression in Mesenchymal Stem Cell derived dopaminergic neurons. Sci. Rep. 8:11715. doi: 10.1038/s41598-018-29528-x
Sun, Y., Sukumaran, P., Selvaraj, S., Cilz, N. I., Schaar, A., Lei, S., et al. (2018b). TRPM2 Promotes Neurotoxin MPP(+)/MPTP-Induced Cell Death. Mol. Neurobiol. 55, 409–420. doi: 10.1007/s12035-016-0338-9
Sun, Y., Zhang, H., Selvaraj, S., Sukumaran, P., Lei, S., Birnbaumer, L., et al. (2017). Inhibition of L-Type Ca(2+) Channels by TRPC1-STIM1 Complex Is Essential for the Protection of Dopaminergic Neurons. J. Neurosci. 37, 3364–3377. doi: 10.1523/jneurosci.3010-16.2017
Thapak, P., Bishnoi, M., and Sharma, S. S. (2020a). Amelioration of diabetes-induced cognitive impairment by Transient Receptor Potential Vanilloid 2 (TRPV2) channel inhibitor: Behavioral and mechanistic study. Neurochem. Int. 139:104783. doi: 10.1016/j.neuint.2020.104783
Thapak, P., Bishnoi, M., and Sharma, S. S. (2020b). Pharmacological inhibition of Transient Receptor Potential Melastatin 2 (TRPM2) Channels Attenuates Diabetes-induced Cognitive Deficits in Rats: A Mechanistic Study. Curr. Neurovasc. Res. 17, 249–258. doi: 10.2174/1567202617666200415142211
Thapak, P., Vaidya, B., Joshi, H. C., Singh, J. N., and Sharma, S. S. (2020c). Therapeutic potential of pharmacological agents targeting TRP channels in CNS disorders. Pharmacol. Res. 159:105026. doi: 10.1016/j.phrs.2020.105026
Tjaša, O., Kolmančič, K., Tratnjek, L., Živin, A., and Živin, M. (2016). “Immunohistochemical Staining of Trpv4 Channels in Rat Parkinson Disease Model,” in Proceedings of the MEi: CogSci Conference 2016, (Bratislava: Comenius University).
Uppalapati, D., Das, N. R., Gangwal, R. P., Damre, M. V., Sangamwar, A. T., and Sharma, S. S. (2014). Neuroprotective Potential of Peroxisome Proliferator Activated Receptor- α Agonist in Cognitive Impairment in Parkinson’s Disease: Behavioral, Biochemical, and PBPK Profile. PPAR Res. 2014:753587. doi: 10.1155/2014/753587
Venkatachalam, K., and Montell, C. (2007). TRP channels. Annu. Rev. Biochem. 76, 387–417. doi: 10.1146/annurev.biochem.75.103004.142819
Voets, T., Talavera, K., Owsianik, G., and Nilius, B. (2005). Sensing with TRP channels. Nat. Chem. Biol. 1, 85–92. doi: 10.1038/nchembio0705-85
Wang, D., Yu, H., Xu, B., Xu, H., Zhang, Z., Ren, X., et al. (2018). TRPC1 Deletion Causes Striatal Neuronal Cell Apoptosis and Proteomic Alterations in Mice. Front. Aging. Neurosci. 10:72. doi: 10.3389/fnagi.2018.00072
Williams, N. M., Hubbard, L., Sandor, C., Webber, C., Hendry, H., Lawton, M., et al. (2020). Genome-Wide Association Study of Pain in Parkinson’s Disease Implicates TRPM8 as a Risk Factor. Mov. Disord. 35, 705–707. doi: 10.1002/mds.28001
Woudenberg-Vrenken, T. E., Sukinta, A., Van Der Kemp, A. W., Bindels, R. J., and Hoenderop, J. G. (2011). Transient receptor potential melastatin 6 knockout mice are lethal whereas heterozygous deletion results in mild hypomagnesemia. Nephron. Physiol. 117, 11–19. doi: 10.1159/000320580
Xie, Y. F., and Zhou, F. (2014). TRPC3 channel mediates excitation of striatal cholinergic interneurons. Neurol. Sci. 35, 1757–1761. doi: 10.1007/s10072-014-1827-0
Xu, H., Ramsey, I. S., Kotecha, S. A., Moran, M. M., Chong, J. A., Lawson, D., et al. (2002). TRPV3 is a calcium-permeable temperature-sensitive cation channel. Nature 418, 181–186. doi: 10.1038/nature00882
Yang, C. P., Zhang, Z. H., Zhang, L. H., and Rui, H. C. (2016). Neuroprotective Role of MicroRNA-22 in a 6-Hydroxydopamine-Induced Cell Model of Parkinson’s Disease via Regulation of Its Target Gene TRPM7. J. Mol. Neurosci. 60, 445–452. doi: 10.1007/s12031-016-0828-2
Yu, Y.-P., Xie, A.-M., and Chi, X.-L. (2013). The effect of TrkA signaling pathway on the expressions of TRPC1, TRPC3 in 6-hydroxydopamine lesioned rat treated by electroacupuncture. Glob. J. Card. Cereb. Dis. 1:1. doi: 10.14725/gjccd.v1n1a203
Yu, Y.-P., Xie, A.-M., and Yang, X.-H. (2014). TrkA Pathway (s) are Involves in the Regulation of TRPM2 and TRPM7 Expression in the Substantia Nigra of the Parkinson’s Disease Rat Model Induced by 6-Hydroxydopamine. Adv. Res. 2, 782–796. doi: 10.9734/AIR/2014/10543
Zeitz, K. P., Guy, N., Malmberg, A. B., Dirajlal, S., Martin, W. J., Sun, L., et al. (2002). The 5-HT3 subtype of serotonin receptor contributes to nociceptive processing via a novel subset of myelinated and unmyelinated nociceptors. J. Neurosci. 22, 1010–1019. doi: 10.1523/jneurosci.22-03-01010.2002
Zhang, X., Hu, M., Yang, Y., and Xu, H. (2018). Organellar TRP channels. Nat. Struct. Mol. Biol. 25, 1009–1018. doi: 10.1038/s41594-018-0148-z
Zhao, Z., Wang, J., Wang, L., Yao, X., Liu, Y., Li, Y., et al. (2017). Capsaicin Protects Against Oxidative Insults and Alleviates Behavioral Deficits in Rats with 6-OHDA-Induced Parkinson’s Disease via Activation of TRPV1. Neurochem. Res. 42, 3431–3438. doi: 10.1007/s11064-017-2388-4
Zhen, X. C., and Chu, H. Y. (2020). Emerging novel approaches to drug research and diagnosis of Parkinson’s disease. Acta Pharmacol. Sin. 41, 439–441. doi: 10.1038/s41401-020-0369-7
Zheng, J. (2013). Molecular mechanism of TRP channels. Compr. Physiol. 3, 221–242. doi: 10.1002/cphy.c120001
Zhou, F. W., Matta, S. G., and Zhou, F. M. (2008). Constitutively active TRPC3 channels regulate basal ganglia output neurons. J. Neurosci. 28, 473–482. doi: 10.1523/jneurosci.3978-07.2008
Zhou, Y., and Han, D. (2017). GATA3 modulates neuronal survival through regulating TRPM2 in Parkinson’s disease. Int. J. Clin. Exp. Med. 10, 15178–15186.
Keywords: Parkinson’s disease, TRP channels, TRPM2, TRPV1, TRPC1
Citation: Vaidya B and Sharma SS (2020) Transient Receptor Potential Channels as an Emerging Target for the Treatment of Parkinson’s Disease: An Insight Into Role of Pharmacological Interventions. Front. Cell Dev. Biol. 8:584513. doi: 10.3389/fcell.2020.584513
Received: 17 July 2020; Accepted: 30 October 2020;
Published: 20 November 2020.
Edited by:
Sandra Derouiche, National Institute for Physiological Sciences (NIPS), JapanReviewed by:
Sonia A. L. Corrêa, Manchester Metropolitan University, United KingdomAjay Prakash, Post Graduate Institute of Medical Education and Research (PGIMER), India
Copyright © 2020 Vaidya and Sharma. This is an open-access article distributed under the terms of the Creative Commons Attribution License (CC BY). The use, distribution or reproduction in other forums is permitted, provided the original author(s) and the copyright owner(s) are credited and that the original publication in this journal is cited, in accordance with accepted academic practice. No use, distribution or reproduction is permitted which does not comply with these terms.
*Correspondence: Shyam Sunder Sharma, c3NzaGFybWFAbmlwZXIuYWMuaW4=