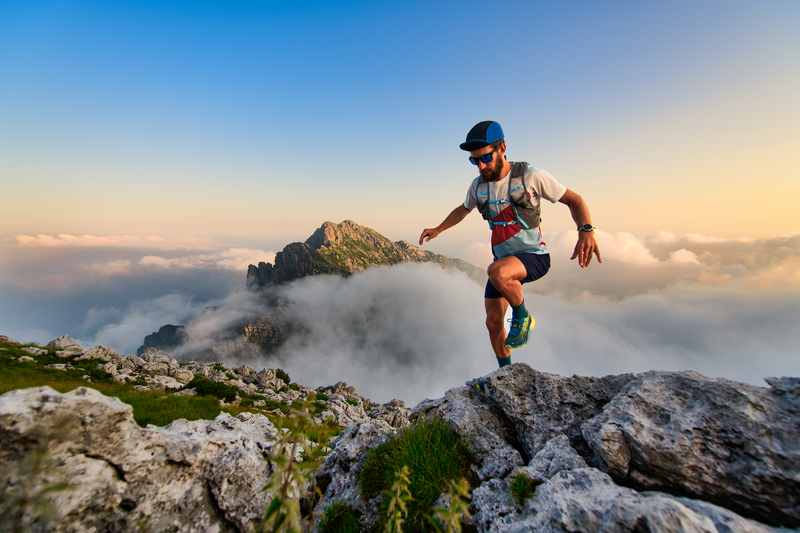
95% of researchers rate our articles as excellent or good
Learn more about the work of our research integrity team to safeguard the quality of each article we publish.
Find out more
REVIEW article
Front. Cell Dev. Biol. , 30 November 2020
Sec. Signaling
Volume 8 - 2020 | https://doi.org/10.3389/fcell.2020.569377
This article is part of the Research Topic Proteoglycans as Mediators of Cell Behavior View all 13 articles
Proteoglycans are a diverse group of molecules which are characterized by a central protein backbone that is decorated with a variety of linear sulfated glycosaminoglycan side chains. Proteoglycans contribute significantly to the biochemical and mechanical properties of the interstitial extracellular matrix where they modulate cellular behavior by engaging transmembrane receptors. Proteoglycans also comprise a major component of the cellular glycocalyx to influence transmembrane receptor structure/function and mechanosignaling. Through their ability to initiate biochemical and mechanosignaling in cells, proteoglycans elicit profound effects on proliferation, adhesion and migration. Pathologies including cancer and cardiovascular disease are characterized by perturbed expression of proteoglycans where they compromise cell and tissue behavior by stiffening the extracellular matrix and increasing the bulkiness of the glycocalyx. Increasing evidence indicates that a bulky glycocalyx and proteoglycan-enriched extracellular matrix promote malignant transformation, increase cancer aggression and alter anti-tumor therapy response. In this review, we focus on the contribution of proteoglycans to mechanobiology in the context of normal and transformed tissues. We discuss the significance of proteoglycans for therapy response, and the current experimental strategies that target proteoglycans to sensitize cancer cells to treatment.
Proteoglycans are proteins with covalently attached GAG chains, which regulate tissue development and have been implicated in pathologies such as cancer. Proteoglycans engage cell surface receptors to induce biochemical signaling, and help modulate the mechanical properties of the ECM including its stiffness. Alongside glycoproteins and glycolipids, proteoglycans act as structural components of the cellular glycocalyx, where they influence cell signaling by regulating the structure function of transmembrane receptors. Here we focus on the role of proteoglycans in tumor mechanics including their impact on extracellular matrix stiffness and the tumor glycocalyx.
In cancer development, oncogenic mutations and the loss of tumor suppressors have long been viewed as the critical initiating events. Nevertheless, there is a growing consensus that even the cells carrying the most powerful oncogenic mutations require an intricate set of microenvironmental conditions to foster their malignant transformation and cancer progression. Among these microenvironmental factors, tissue stiffening which accompanies the fibrotic response that characterizes all solid tumors, has emerged as a key factor that can foster malignancy and collaborate with oncogenes to disrupt tissue organization and promote the growth, survival, invasion and ultimately the metastatic dissemination of the cancer cells (Northey et al., 2017). Maintaining tissue organization requires retention of a state of tensional homeostasis that is mediated by a balance between the stiffness of the tissue stroma and the actomyosin contractility of the cells within the tissue. An abnormal and sustained increase in the stiffness of the stroma such as occurs with fibrosis will increase the contractility of the cells in the tissue to destabilize cell-cell junctions, compromise polarity and destroy tissue architecture. Similarly, an increase in cellular contractility, that is induced following oncogene activation (e.g., Ras or Her2), disrupts tissue architecture as well. It destabilizes cell-cell adhesions, and stimulates the remodeling and stiffening of the ECM until such time as the cell reaches a state of tensional equilibrium with its mechanical microenvironment (Paszek et al., 2005). Thus, chronically elevated tension will compromise tissue architecture and this in turn can foster malignant transformation and promote tumor progression (DuFort et al., 2011; Samuel et al., 2011).
A stiffened tissue is frequently accompanied by chronic inflammation which, in turn, increases stiffness even further (Maller et al., 2020). Consistently, pathologies such as kidney, lung and liver fibrosis are characterized by inflammation as well as increased levels of ECM proteins including interstitial collagen, that stiffen the stroma of the tissue. Tissue fibrosis is accompanied by increased risk of cancer development (Adler et al., 2016; Noguchi et al., 2018). Moreover, tissue stiffening correlates clinically with tumor progression and aggression in malignant gliomas (Miroshnikova et al., 2016; Barnes et al., 2018), as well as adenocarcinoma of the breast (Acerbi et al., 2015), and pancreas (Laklai et al., 2016).
There are three types of mechanical stress that tumor cells experience: tensile, compressive and shear pressure, reviewed in (Butcher et al., 2009; Northey et al., 2017) (Figure 1).
Figure 1. Within a growing tumor, cells are subjected to mechanical forces, in response to which the cells exert tensile, compressive or shear stress. Tensile pressure (tissue tension), stems from ECM stiffening and remodeling; compressive pressure applied occurs when tumor cells proliferate in a confined space leading to vascular compression and damage; and shear force that is experienced by the cancer cells in the circulation.
Tensile stress, or tension, arises from an increase in the local tissue stiffness caused by a rigid ECM and its ability to stimulate actomyosin contractility in the cells within the tissue. This is associated with ECM remodeling, accumulation of proteins such as fibronectin and increased abundance and cross-linking of collagen fibers (Levental et al., 2009; Schedin and Keely, 2011). While type I collagen is critical for increasing the tensile strength of most peripheral tissues, hyaluronic acid, tenascin and small proteoglycans including biglycan and lumican, contribute substantially to the stiffness of the brain parenchyma (Kim and Kumar, 2014; Miroshnikova et al., 2016).
Cellular tension can also arise through the increased activity of GTPases such as Rho that are stimulated by elevated cytokine activation of G protein coupled receptors or by activated oncogenes such as Ras (Samuel et al., 2011; Sanz-Moreno et al., 2011; Laklai et al., 2016). High mechanical tension is sensed by cellular transmembrane receptors such as integrins. Integrins are hetero-dimeric transmembrane proteins that serve as primary receptors of ECM components. Integrins consist of dimeric α and β chains that each have multiple isoforms, creating 24 known unique combinations that are expressed in a tissue-specific way and selectively bind to the ECM proteins (Humphries et al., 2006). Integrin activation can be induced either outside – in via ligand binding or inside - out via intracellular signaling. In either instance, integrin activation induces a conformational change in the heterodimer that unfolds the integrin to potentiate its ligand binding and facilitate recruitment of cytoplasmic proteins that initiate integrin clustering to form nascent adhesions.
Focal adhesion formation, however, requires cellular actomyosin contractility to reach a sufficient level that is proportional to the stiffness of the ECM. At a critical threshold talin-vinculin binding is favored as is the recruitment and activation of focal adhesion kinase (FAK), ROCKI and ROCKII, Src, as well as several adaptor proteins, including paxillin that foster the assembly of focal adhesions. Within these adhesion complexes vinculin and talin function as “molecular clutches” linking the integrin directly to the network of actin filaments underneath the cell membrane. When cellular tension is sufficiently high and prolonged, these molecular clutches will promote the formation of stress fibers which will then be further strengthened and stabilized with participation of the final components of the focal adhesion complex – zyxin and VASP (Kanchanawong et al., 2010) (Figure 2).
Figure 2. Mechano-transduction in cells is mediated through integrin activation upon binding to the ECM components, such as fibronectin. Syndecans (S) facilitate this interaction by binding the ECM with HS chains. Intracellular syndecan domains activate signaling with PKCα (P) and via binding to PDZ-motif proteins. Integrin activation recruits a host of focal adhesion components, including integrin-binding adaptor proteins – talin and paxillin (PX); actin binding cytoskeletal proteins VASP and vinculin (V); and focal-adhesion associated kinases Src, FAK and ROCK. VASP and zyxin (Z) facilitate actin polymerization and connect it to the focal adhesion complex. Phosphorylation of p130Cas by Src allows docking and activation of several signaling pathways, such as the MAPK, the c-Jun N-terminal kinase (JNK) pathway and others.
In response to focal adhesion assembly, FAK and Src kinases, phosphorylate a large scaffolding protein p130Cas. p130Cas acts as a docking block for multiple signaling proteins that can activate each other in close proximity, therefore amplifying their down-stream signaling cascades. These include ILK – CDC42 and ILK – Rac pathways, which regulate filopodia and invadopodia formation and cell polarization and contractility (Alexander et al., 2008). These functions allow the cells to initiate movement in response to mechano-stimulation, or, in the case of cancer cells, to move toward and invade the neighboring tissues after being exposed to a stiff environment.
In addition, cell survival and proliferative pathways are also induced via interaction with p130Cas. Erk and PI3K signaling is up-regulated upon integrin activation in a p130Cas-dependent manner (Cabodi et al., 2010; Janoštiak et al., 2014). Further evidence of p130Cas involvement in mechanosignaling comes from a study which determined that physical stretching of the cells on elastic substrates induced p130Cas to assume an unfolded conformation, open to phosphorylation by Src. This, in turn, promoted down-stream signaling. Notably, p130Cas unfolding and phosphorylation is significantly higher among the cells on the edges of the 3D in vitro colonies, compared to the bulk of the cells inside. This suggests that mechanical stretching directly activates pro-survival signaling in invading cells enabling them to migrate and disseminate (Sawada et al., 2006).
Elevated cellular contractility is also characteristic of the cells that carry a bulky glycocalyx. Glycocalyx is a dense cell surface coating composed of glycoproteins and proteoglycans, which reinforce the external barrier of a cell, and actively regulate mechano-transduction and growth factor signaling. In aggressive metastatic cancer cells, glycocalyx is frequently enhanced (Paszek et al., 2014; Barnes et al., 2018). Bulky glycocalyx was shown to facilitate adhesion assembly and augment integrin-mediated signaling (Paszek et al., 2014).
Finally, stiff microenvironment activates a critical mechanosignaling pathway –YAP and transcriptional coactivator with a PDZ-binding motif (TAZ) (Dupont et al., 2011). YAP/TAZ are transcriptional co-activators that shuttle between cytoplasm and the nucleus, where they bind to DNA transcription factor TEAD and activate expression of its target genes, supporting cell survival and decreasing apoptosis. This signaling pathway is mechano-responsive, and several mechanisms for mechano-regulation of YAP/TAZ have been proposed. First, inhibition of ROCK kinase prevents YAP/TAZ nuclear localization (Dupont et al., 2011), which suggests that focal adhesion assembly is required for YAP/TAZ signaling. In addition, inhibitors of actomyosin and actin polymerization, as well as integrins, also inhibit YAP/TAZ (Dupont, 2016). Finally, it has also been shown that in cells stretched out on a stiff substrate, the nucleus is compressed. This causes YAP/TAZ to translocate directly inside via nuclear import channels, bypassing up-stream regulation, and to induce expression of its target genes (Elosegui-Artola et al., 2017). These results point to YAP/TAZ activation as a sensor of mechanosignaling.
Several approaches have been developed to explore the causal relationship between tissue tension and disease development including malignant transformation and tumor progression. Cellular mechanosignaling and actomyosin actomyosin tension reduction can be achieved by inhibiting integrin focal adhesion signaling or integrin focal adhesion assembly via knockdown or inhibition of key adhesion components including talin, vinculin, focal adhesion kinase and ROCK. Mechanosignaling and actomyosin tension can be enhanced through expression of a β1-integrin engineered to promote inter-molecular associations that foster clustering of the molecules by introducing a mutant β1-integrin with a single amino acid substitution: V737N. This single substitution of hydrophobic valine residue with a hydrophilic asparagine promotes integrin clustering by reducing repulsive forces in the transmembrane domain of the integrin. Expression of the V737N β1-integrin enhances the assembly of focal adhesions accompanied by elevated p397FAK and increased ROCK activity that translate into higher actomyosin contractility and potentiate growth factor receptor dependent activation of MAPK, PI3K and Stat3 signaling (Paszek et al., 2005; Levental et al., 2009; Laklai et al., 2016). Conversely, reducing tenascin C expression in aggressive glioblastoma cells significantly decreases the stiffness of the brain tumor ECM leading to significantly lower tumor aggression (Miroshnikova et al., 2016).
In matrix collagen-rich tissues, higher stiffness coupled with the reorganization of the collagen into thickened, oriented fibers facilitates the directed invasion of the cancer cells to promote their migration through the interstitial stroma that ultimately favors their dissemination and metastasis (Ahmadzadeh et al., 2017). Cells exposed to a chronically stiffened ECM with sustained myc, catenin, YAP/TAZ and TGFβ activity often undergo an EMT that promotes their phenotypic switch to a motile state that is highly resistant to anti-cancer treatments (Barnes et al., 2018). Epithelial tumor cells that have undergone an EMT down-regulate cell-cell adhesion receptors such as E-cadherin that compromise their potential to maintain polarized tissue structures. In addition to the loss of E-cadherin, polarization is also accompanied by a decrease in syndecan-1 proteoglycan on the cell surface (Sun et al., 1998). Syndecan-1 loss was later found to independently induce the EMT in several cancers, leading to increased migration and invasion (Wang et al., 2018). Cells that have undergone EMT are also more contractile and exert higher forces at their integrin adhesions (Mekhdjian et al., 2017), decrease cytokeratin expression and upregulate the intermediate filament protein vimentin and exhibit a softening of their nuclei (Liu et al., 2015; Northey et al., 2017). Intriguingly, EMT substantially increases expression of glycoproteins that contribute to the cellular glycocalyx. Cells with a bulky glycocalyx can relax the bulk of their cortical tension and this allows them to squeeze through the confined spaces often encountered within a rigid, dense tumor microenvironment (Shurer et al., 2019). Consistently, tumors that express a bulkier glycocalyx are often more aggressive and metastatic and circulating tumor cells frequently express high levels of glycoproteins that contribute to a bulky glycocalyx (Paszek et al., 2014).
Not surprisingly, the stiffened, high-tension tumor environment stimulates growth factor and cytokine dependent cancer cell growth and survival, and promotes invasion, migration and dissemination of the tumor cells. Consistently, breast and pancreatic tumor cells and glioblastoma cells in which integrin-dependent mechanosignaling was potentiated through expression of the V737N mutant β1-integrin, undergo an EMT that fosters their motility and invasion in culture and in vivo, and reduces the survival of experimentally manipulated mice (Laklai et al., 2016; Mekhdjian et al., 2017; Barnes et al., 2018).
The rapid proliferation of tumor cells within a confined area can increase compression stress. Compression stress in tumors can also arise through elevated water retention mediated by increased concentration of proteoglycans and hyaluronic acid (DuFort et al., 2016). Compression stress within a confined tumor can create a mechanical stress gradient with the forces declining at the tumor periphery. High compression stress within the tumor can occlude blood and lymphatic vessel integrity that induces tumor hypoxia and increases interstitial fluid pressure (Sarntinoranont et al., 2003). High tumor compression stress contributes to cancer aggression either by directly activating signaling pathways or by indirectly inducing hypoxia to stimulate HIF1a-dependent gene expression that each can increase tumor cell growth, survival and invasion, and promote EMT to drive cancer aggression and metastasis (Tse et al., 2012).
Shear stress is yet another type of mechanical stress cancer cells experience in the tumor tissue. The shear stress is created by the blood and interstitial fluid flow the cancer cells encounter once they extravasate into the vasculature or lymphatics during their metastatic dissemination. These shear stresses can stimulate signaling pathways that enhance tumor cell aggression and influence vessel patency. For instance, Polacheck et al. (2017) show how vascular flow can induce cleavage of the transmembrane Notch 1 receptor that permits the resulting Notch transmembrane domain to form a complex with adherens junctions that enhanced cell-cell adhesion stability. In cancer cells, continuous exposure to fluid flow promotes migration and invasion capacity. Some reports suggest that proteoglycan-rich glycocalyx acts as a mechano-sensor of the interstitial flow and enables mechanosignaling activation, while enzymatic reduction of glycocalyx bulk inhibits flow-induced signaling (Shi et al., 2011; Qazi et al., 2013).
In summary, as structural components of the glycocalyx and the ECM, proteoglycans function as integral regulators of bio-mechanics in both normal and malignant tissues. The following sections provide an overview of the proteoglycan diversity, describe their known function in cancer development, and summarize the reports of proteoglycans’ involvement in the regulation of mechanosignaling.
Proteoglycans are a class of glycosylated proteins widely expressed in various tissues, which play an important role in a variety of cellular interactions and signaling events (Couchman and Pataki, 2012). Proteoglycans are one of the principal components of the mammalian glycocalyx, which along with glycoproteins and glycolipids form the sponge-like mix of proteins and lipids which acts as a barrier between the cell surface and the extracellular matrix (ECM), and regulates the inter actions between the two (Monne et al., 2013; Buffone and Weaver, 2020). In addition, proteoglycans are a major component of the ECM and are also present intracellularly and pericellularly. Proteoglycans are characterized by at least one covalently bound GAG chain, although they can also contain other N-, and O-linked glycans found in glycoproteins or glycolipids (Mulloy and Rider, 2006; Nikitovic et al., 2018). These GAG chains have very distinct glycosylation patterns which consist of repeating disaccharide units, are long (∼80 monosaccharides for GAGs while ∼10–15 for N-glycans), are sulfated at various different points of the GAG chain and of each monosaccharide, and have a much more linear structure as compared to N- or O-glycans (Iozzo and Schaefer, 2015; Lindahl et al., 2015). Furthermore, GAG chains are characterized by the Galactose–Galactose–Xylose motif (Gal–Gal–Xyl), which initiates the chains and can contain either IdoA or GlcA, monosaccharides unique to GAG (Gandhi and Mancera, 2008; Pomin and Mulloy, 2018). Furthermore, GAGs differ from glycoproteins and glycolipids in that the GlcA/IdoA and hexosamine sugars form repeating disaccharides which compose the long GAG chains.
There are five main GAG chains: heparin, CS, DS, KS, and HS, all of which are sulfated (Soares da Costa et al., 2017). Each GAG consists of unique monosaccharide building blocks as: heparin contains GlcNAc, GalNAc, IdoA, and GlcA (Shriver et al., 2012), CS contains GalNAc and GlcA, DS contains IdoA and GalNAc, KS contains galactose (Gal) and GlcNAc (Funderburgh, 2002), and HS contains GlcNAc, GalNAc, IdoA, and GlcA (Lindahl et al., 2015). The differences in composition of each of the GAGs, which have been determined by sequencing for most of the GAGs except for KS, expressed on proteoglycans have profound effects on their function both inside and outside of the cell.
An important distinction between proteoglycans and other glycosylated molecules such as mucins, glycoproteins, and glycolipids, which are primarily present on the cell surface (Buffone et al., 2013, 2017; Mondal et al., 2016), is that proteoglycans can be localized either intracellularly, pericellularly, on the cell surface, or be shed into the extracellular matrix (Figure 3) (Vogel and Peterson, 1981; Yanagishita, 1993; Lin, 2004; Iozzo et al., 2009). The different members of the proteoglycan classes and their functions have been beautifully reviewed by others (Iozzo and Schaefer, 2015), but we will briefly cover the pertinent proteoglycans in terms of the topic of this review.
Figure 3. Proteoglycans’ localization. Serglycin (SG) is the only intracellular proteoglycan, found in secretory vesicles (SV). It carries both HS (blue) and CS (red) GAG chains. Transmembrane proteoglycans include, but are not limited to phosphacan (P), betaglycan (B), syndecans (S) and glypicans (G). In the ECM, SLRPs are short proteoglycans that carry either a CS or a DS (green) GAG chain. Other proteoglycans include testican (T) and hyalectins that aggregate with hyaluronic acid (HA). Basement membrane (BM) contains agrin (A), perlecan (PR) and collagens XV (XV) and XVIII (XVIII).
There is only one proteoglycan in the mammalian genome which resides in the intracellular space and that is serglycin. Serglycin is interesting as it is located in the secretory granules of cells and holds together all of the proteases and other components of the granule (Kolset and Tveit, 2008). Serglycin can carry a variable number of heparin or CS side chains (Nugent, 2000).
There are two major families of cell-surface proteoglycans: syndecans 1–4 (Cheng et al., 2016) which have a transmembrane domain and glypicans 1–6 (Li N. et al., 2018) which are anchored to the cell membrane through a GPI lipid. Syndecans have primarily HS GAGs but can also have CS chains which are implicated in a variety of biological processes, most critically in binding growth factors in tissues (Forsten-Williams et al., 2008). Enzymatic processing of syndecans represents an important regulator of their function. Syndecans can be secreted into the pericellular environment by MMPs (Rossi et al., 2014) and into the extracellular matrix through partial degradation of their domains by proteases to perform other functions (Teng et al., 2012).
Heparanase is an endoglycosidase that can cleave heparan sulfate side chains. This can result in the release of growth factors or chemokines bound by syndecans. In many malignancies, high expression of heparanase has been associated with an aggressive tumor phenotype (Ramani et al., 2013). Furthermore, post-assembly processing by plasma membrane-bound endo-6-O-sulfatases (SULF1 and SULF2) changes the sulfation pattern of HS chains, which in turn leads to an altered ligand/receptor interaction (Bishop et al., 2007). In liver cancer, the activation of SULF1/SULF2 promotes the tumor growth (Graham et al., 2016).
Glypicans are HSPGs, that are linked to the cell surface by GPI anchor. Glypicans can modulate a variety of cell signaling pathways including Wnt, frizzled, BMP, hedgehog (Hh), and bFGF (Cassiman et al., 1992; Capurro et al., 2005, 2008; Filmus and Capurro, 2014). Increased expression of glypicans has been found in pediatric cancers and liver cancer (Li N. et al., 2018). Other proteoglycans which reside at the cell surface include betaglycan (Bilandzic and Stenvers, 2012) and phosphacan (Maurel et al., 1994). They are discussed further in other reviews (Iozzo and Schaefer, 2015).
There are four pericellular proteoglycans that reside predominantly within the basement membrane: perlecan, agrin, and collagens XV and XVIII (Iozzo et al., 2009). Perlecan is a massive proteoglycan (>500 KD) which contains HS GAG chains that cross-link cell surface molecules to ECM components in the basement membrane (Gubbiotti et al., 2017). Perlecan is secreted by smooth muscle and vascular cells and plays a role in a variety of cellular processes including cell adhesion, inflammation, wound healing, endocytosis, and cardiovascular development (Whitelock et al., 1999; Sasse et al., 2008; Zoeller et al., 2008; Jung et al., 2013). Agrin is a similar proteoglycan in structure to perlecan that also cross-links the cell surface to the ECM that differs from perlecan by having both HS and CS GAGs and is expressed primarily in the brain where it is known to interact with NCAMs (Tsen et al., 1995; Winzen et al., 2003). Finally, collagen XV, which carries CS GAG chains, and collagen XVIII, with HS chains, are proteoglycans which are present in all human and mouse basement membranes that play distinct roles in the structural support and angiogenesis (Heinämäki et al., 1994; Eklund et al., 2001).
The ECM proteoglycans are the largest class of proteoglycans. This group of molecules consists of the calcium binding testican family, the SLRPs and lectin (hyalectin). Hyaluronic acid is also frequently present in the extracellular space. Testican proteoglycans carry HS and CS GAGs (Bonnet et al., 1992), are modular in structure like perlecan, and are found in both the testis and CNS where they play a strong role in neuronal development (Schnepp et al., 2005). Conversely, SLRPs contain over 18 distinct members and are much smaller than other proteoglycans (30–40 kDa). They are defined by a core that consists of leucine-rich repeats (Iozzo et al., 2011). SLRPs are widely present in almost all of the ECM in the meninges, pericardium and peritoneum and are involved in regulating organ shape (Iozzo, 1997, 1999).
The hyalectin class of proteoglycans has four members: aggrecan, versican, neurocan, and brevican, which are characterized by tri-domain structure in which the N-terminus binds hyaluronan, the C-terminus binds lectins, and the central domain contains the GAG (predominantly CS) side chains (Iozzo, 1998). The hyalectins play important roles in maintaining the structure of the brain, cartilage, and vasculature. Aggrecan is known to form large complexes (∼200 MDa) with hyaluronan, collagen, and other proteoglycans and glycoproteins and this hydrated gel-like ECM structure forms the load bearing component of cartilage (Heinegård, 2009; Wight et al., 2011). Versican has four isoforms (V0, V1, V2, and V3) which are differentially expressed in tissues such as the brain, heart, and vascular smooth muscle (Zimmermann and Ruoslahti, 1989; Naso et al., 1994; Zimmermann and Dours-Zimmermann, 2008). Versican’s function is to facilitate cell migration and resolution of inflammation as it interacts with cell surface receptors on the leukocyte surface to enhance interactions with the vasculature (Wight et al., 2014). Neurocan is a proteoglycan with CS GAGs expressed in the brain that binds to NCAM, tenascin, and hyaluronan and inhibits neurite outgrowth at the sites of injury (Davies et al., 2004; Liu et al., 2006). Finally, brevican is a critical proteoglycan in brain function as its expression has been linked to glioblastoma, brain tissue injury, and Alzheimer’s disease (Yamada et al., 1994; Frischknecht and Seidenbecher, 2012).
Several distinct functions of proteoglycans define their complex role in biological systems. To begin with, proteoglycans promote cell-to-cell and cell-to-matrix interactions. Proteoglycans also trap and release various growth factors and cytokines. For example, HS GAG chains of transmembrane proteoglycans can bind bFGF (Faham et al., 1996; Li Y. et al., 2016). As bFGF and other growth factors trapped by the GAG chains of proteoglycans are gradually dissolved, they prompt proliferation of the neighboring cells, and induce vascular growth and tissue regeneration (Hao et al., 2018; Li R. et al., 2018). In addition, enzymatic processing of proteoglycans by proteases and heparanases represents another important factor that modifies their biological activity (Cheng et al., 2016).
Proteoglycans, most prominently HSPGs, are often dysregulated during tumor development (Fjeldstad and Kolset, 2005; Varki et al., 2016). However, their role in cancer is highly context dependent. The following section describes the known contribution of intracellular, pericellular and ECM proteoglycans in cancer development and progression.
Heparin- and CS-decorated serglycin is the sole known intracellular proteoglycan, expressed most prominently by the endothelial, hematopoietic and myeloid cells. It can be detected both intracellularly, where it localizes to the secretory vesicles, and is secreted into the extracellular space (Schick et al., 2001; Kolseth et al., 2015). Serglycin secreted by both cancer-associated stroma cells, as well as the cancer cells themselves, binds to the transmembrane glycoprotein CD44 (Guo et al., 2017).
Activation of CD44 in cancer cells promotes stemness, drives sphere formation, and increases invasion and migration (Skandalis et al., 2019). These effects are mediated through a transcription factor, Nanog, which sustains the non-committed state of the cells (Guo et al., 2017). Completing a positive-feedback loop, serglycin itself appears to promote CD44 expression, since a knock-down of serglycin leads to a drop in the cells with high CD44 levels (Zhang et al., 2020). In lung and colorectal cancer, high expression and secretion of serglycin is associated with a poor clinical outlook and treatment resistance (Xu et al., 2018), while the knock-down of serglycin decreases tumor burden in vivo (Guo et al., 2017). In line with these findings, serglycin was also found to support stemness in glioblastoma cells, where it prevents astrocytic differentiation and promotes cell proliferation and tumor growth (Manou et al., 2020).
The clinical consequence of high expression of transmembrane proteoglycans is variable among cancer patients. The current consensus emphasizes a highly context- and cancer type-dependent manner in which certain proteoglycans regulate the tumor development and progression.
Sdc-1 is found in several normal tissues, as well as in the malignant tumors (Kind et al., 2019). In some cases, high expression of Sdc-1 has been linked to poor prognosis. Notably, high levels of Sdc-1 in breast cancer cells correlated with higher incidence of brain metastasis (Sayyad et al., 2019). Sdc-1 is also more abundant in the ER-negative tumors, suggesting that its expression marks this more aggressive breast cancer phenotype (Barbareschi et al., 2003; Baba et al., 2006; Qiao et al., 2019). This has been corroborated by reports showing that Sdc-1 expression is directly repressed by ER (Fleurot et al., 2019). In agreement with this, recent work described Sdc-1 as an important marker of CSC phenotype in inflammatory breast cancer – a subtype with a notoriously poor prognosis (Ibrahim et al., 2017). In that study, Stat3, NFκB, Notch-1, Notch-2, and EGFR signaling was significantly reduced upon Sdc-1 knock-down. These data suggest that Sdc-1 could serve as a modulator of CSC phenotype and may be a promising therapeutic target. Notch pathway activation may specifically rely on the HS GAG chains of the Sdc-1, as a recent study showed that overexpression of HS-sulfotransferase enzymes regulates Notch signaling in breast cancer cells. However, this effect is specific to the triple-negative breast cancer (TNBC) (Teixeira et al., 2020).
These findings are in contrast with other work, where Sdc-1 depletion led to the increased breast cancer cell motility and invasion facilitated by stronger integrin adhesion to ECM components, such as fibronectin (Ibrahim et al., 2012; Hassan et al., 2013). Some of this discrepancy in the role of Sdc-1 in breast cancer development may also be due to its differential expression between cell types. For example, one study of the normal breast tissue found that Sdc-1 expression was higher in the stromal component compared to lobular epithelium (Lundström et al., 2006). The authors found that in women with high mammographic density (MD), Sdc-1 expression shifted from epithelial to stromal cells. As high MD has been linked to increased breast cancer incidence (Vachon et al., 2007), this suggests that stromal Sdc-1 expression is important for breast cancer development. In support of this, orthotopic injection of aggressive murine mammary tumor cell lines into Sdc-1–/– mice prevented lung metastasis compared to wild-type animals. These findings identify Sdc-1 as a key component of a lung metastatic niche (Chute et al., 2018).
Pro- and anti-tumorigenic role of Sdc-1 may also be context-dependent. In colorectal cancer cells, high expression of Sdc-1 reduced tumor growth and invasion through down-regulation of MAPK and Stat3 signaling (Wang et al., 2019). Additionally, low expression of Sdc-1 corresponded with poor prognosis in colorectal cancer patients (Li K. et al., 2019). In this cancer type, Sdc-1 silencing promoted EMT and a stem-like phenotype and stimulated integrin signaling through FAK kinase phosphorylation. This resulted in accelerated tumor growth and possible resistance to radiation therapy (Katakam et al., 2020). Similarly, in gastric carcinomas, high Sdc-1 expression correlated with a less aggressive tumor phenotype (Charchanti et al., 2019). Notably, normal tissues of the gastro-intestinal tract have high levels of Sdc-1 (Schick et al., 2001), which suggests that Sdc-1 expression is lost at some point during cancer development and progression in some of the patients, conferring a survival advantage.
This is supported by several studies, which show that Sdc-1 expression is lost during EMT in both cancer (Yang et al., 2011; Farfán et al., 2018; Kalscheuer et al., 2019) and normal tissues (Sun et al., 1998). EMT is an important developmental program of embryogenesis, which can be hijacked by the cancer cells during tumor growth to promote invasion and metastasis. As previously mentioned, increased tissue stiffness promotes EMT and up-regulates mechanosignaling in cancer (Wei et al., 2015; Zhang et al., 2016; Matte et al., 2019). This raises a possibility that high tumor stiffness promotes a mesenchymal-like phenotype in cancer cells, which in turn leads to the loss of Sdc-1, among other consequences.
Several other transmembrane proteoglycans have also been linked to an aggressive cancer phenotype. Syndecan 2 (Sdc-2) expression is increased in a range of cancers including lung adenocarcinoma and colon cancer, where it promotes expression of matrix metalo-proteinase 9 and 7 (MMP9 and MMP7) respectively (Jang et al., 2016; Tsoyi et al., 2019). MMPs regulate cancer cell invasion by digesting the ECM immediately surrounding the cancer cells, and by inducing shedding of E-cadherin adhesion proteins. Sdc-2 is therefore linked to the EMT and cancer invasion (Mytilinaiou et al., 2017). Similarly, Syndecan 4 (Sdc-4) has been shown to promote EMT in the papillary thyroid carcinoma cells (Chen L.L. et al., 2018). NG2 is another transmembrane proteoglycan, which is often over-expressed in cancer and has been linked to poor prognosis (reviewed in Nicolosi et al., 2015).
In contrast to the transmembrane proteoglycans discussed above, betaglycan, also known as TGFβ-receptor 3 (TGFβR3), has been associated with positive outcome in cancer patients (Nicolosi et al., 2015). Nicolosi et al. found that abundant betaglycan inhibits NF-κB and MAPK signaling and reduces tumor growth and invasion. In line with this, the loss of betaglycan in renal cell carcinoma lifts this inhibition and induces TGF-β signaling. This results in rapid metastasis in vivo (Nishida et al., 2018).
A recent study described activation of Wnt/β-catenin/c-Myc signaling axis by one of the transmembrane GPI-anchored proteoglycan glypican-4 (GPC4), and the resulting up-regulation of glycolysis in colorectal cancer. Moreover, proteasomal degradation of GPC4 resulted in Wnt/β-catenin/c-Myc signaling attenuation and metabolic reprogramming, which was favorable for the survival of the tumor-bearing mice (Fang et al., 2019). By contrast, glypican-3 (GPC3) has been reported to act as a tumor suppressor. GPC3 silencing promoted breast cancer growth, while ectopic re-expression of GPC3 inhibited growth in vitro (Xiang et al., 2001).
The transmembrane proteoglycan Neuropilin-1 (NRP1) is one of the proteoglycans which serves as a co-receptor in several growth-factor signaling pathways, including EGFR-Akt (Rizzolio et al., 2012), fibroblast growth factor 2 (FGF2) (West et al., 2005), PDGF – TGFβ (Cao et al., 2010) and VEGF signaling. Notably, NRP1-mediated activation of the VEGF signaling is dependent on the HS or CS GAG chain attachment to its core protein. In the absence of full glycosylation, stimulation with VEGF no longer promotes growth, invasion and formation of blood vessels (Shintani et al., 2006; Hendricks et al., 2016).
Perlecan is a proteoglycan highly expressed in basement membranes, peri-vascular regions and tumor-adjacent stroma regions in most solid cancer types (Figure 3) (Cruz et al., 2020). Multiple HS chains attached to perlecan protein core account for its ability to bind a variety of growth factors, cytokines and cell surface receptors (Whitelock et al., 2008). Controlled gradual release of these bound growth factors maintains structured tissue homeostasis, which becomes impaired in malignant tumors (Cruz et al., 2020).
Perlecan has been identified in a recent study, as a mediator of the intricate relationship between cancer cells and CAFs in PDAC (Vennin et al., 2019). The authors found that PDAC cells carrying a p53-gain of function (GOF) mutation, induced a transcriptional change in CAFs, prompting them to support invasion and metastasis. In these tumors, CAFs stimulated cross-linking of collagen fibers, which resulted in higher mechanical stiffness levels compared to the less aggressive counterparts with the loss of p53. Notably, conditioned media, collected from CAFs of p-53-GOF tumors was sufficient to induce collagen remodeling in vitro, suggesting that some of the soluble secreted factors are responsible for this change. A mass spectrometry analysis of the CAF-derived secretome revealed a high abundance of perlecan. Further experiments demonstrated that perlecan was necessary and sufficient for induction of collagen remodeling, increase in cancer cell actomyosin contractility and promotion of invasion of the PDAC cells into the matrix (Vennin et al., 2019). Moreover, immune response against the tumor cells was significantly increased following perlecan depletion. In line with this, antibody targeting perlecan inhibited tumor growth in a PDX mouse model of TNBC. Interestingly, a stronger anti-tumor effect was observed in nude mice than in the Nod/scid (NSG) mice, suggesting that perlecan helps create an immunosuppressive tumor microenvironment (Kalscheuer et al., 2019).
It has also been reported that during bone metastasis in prostate cancer, accumulation of perlecan promotes cell-cell adhesion instead of cell-ECM, preventing spreading of the cancer cells. Perlecan cleavage by matrix metalloproteinase-7 (MMP-7) facilitates cancer cell-ECM adhesion, dispersion and eventually leads to bone metastasis via induction of FAK-dependent invasion (Grindel et al., 2018). These examples underscore the highly context-dependent manner in which various proteoglycans impact tumor progression.
In addition to perlecan, other pericellular proteoglycans have also been shown to have pro-metastatic functions. Agrin, another HS-bearing proteoglycan, has a limited expression in the normal tissue, but is progressively up-regulated during malignant transformation in oral cancer (Rivera et al., 2018). A knock-down of agrin reduced cancer cell proliferation, invasion and sphere formation. Similarly to perlecan, agrin from conditioned media was sufficient to rescue proliferation and invasion in the agrin-silenced cells, suggesting that soluble agrin mediates its pro-metastatic function. In addition, it has been shown that agrin facilitates angiogenesis. Agrin produced by cancer cells, binds to and stabilizes its receptors β1-integrin and Lrp4 on the surface of the vascular endothelial cells. Coupled with an increasing stiffness inside a tumor, this interaction promotes formation of focal adhesions and stabilization of VEGFR2. Activation of VEGFR2, in turn, leads to branching and proliferation of the endothelial cells, and formation of new blood vessels (Njah et al., 2019).
Other pericellular and basal membrane proteoglycans, such as collagen XVIII and collagen XV have also been implicated in cancer progression support, however, limited evidence has been reported on this subject. In metastatic gastric cancer, as well as in non-small cell lung cancer, high expression of collagen XVIII correlated with a poor clinical outcome (Iizasa et al., 2004; Lee et al., 2010). However, both collagen XVIII and XV are integral components of the basal membrane. While their expression in the tumor may be up-regulated, both collagens are often depleted from the basement membrane. This makes it more permeable and thus enables epithelial tumor cells to invade (Amenta et al., 2003).
Abundance of extracellular proteoglycans in the ECM has been linked to a more aggressive phenotype of the tumors and stiffer microenvironment (Varga et al., 2012). Accumulation of ECM proteoglycans is also known to result in higher tissue stiffness. In a normal breast, higher tissue stiffness corresponds to higher mammographic density (MD), a well-established risk-factor for breast cancer development, as mentioned above (Vachon et al., 2007). High MD is characteristic for breast tissue rich in ECM proteoglycans, including hyalectans (aggrecan, versican, neurocan, and brevican), basement membrane proteoglycans (ex. perlecan), and SLRPs such as lumican and decorin (Alowami et al., 2003; Shawky et al., 2015).
It is important to note, however, that while proteoglycan expression level is often associated with cancer, the extent of their modification with GAG chains may be even more important. A recent study examined a pattern of extracellular proteoglycans in the ECM of malignant brain tumors. The authors discovered that CS-modified proteoglycans, such as aggrecan, versican, neurocan, and brevican, concentrated on the edge of the low-grade gliomas. The CS GAGs promoted adhesion between tumor cells and attracted reactive astrocytes, thus helping limit the invasion of the tumor cells into the brain tissue. The more aggressive high-grade tumors, however, had a low abundance of CS GAGs, which the authors connected to the invasive nature of those lesions (Silver et al., 2013). This proposed barrier function is supported by evidence from previous reports, where extracellular proteoglycans were found to direct brain tissue development by imposing spatial limits between distinct regions of the nervous tissue and the outer borders of the brain and spinal cord (Golding et al., 1999).
Testican 1, encoded by SPOCK1 gene, has been shown to promote cancer growth. SPOCK1 knock-down strongly reduces tumor growth and metastasis in an in vivo model of breast cancer (Perurena et al., 2017). Similarly, in colorectal cancer, SPOCK1 was found to be over-expressed and contributed to the activation of PI3K-Akt signaling (Zhao et al., 2016). This is in agreement with a recent report that demonstrated activation of the same signaling cascade in glioma cells (Yang et al., 2016). In all cases, tumor-supporting role of testican 1 was linked to induction of EMT and activation of Wnt signaling (Miao et al., 2013). A link between testican 1 expression and EMT is further supported by evidence that resistance to a broad range of targeted inhibitors, associated with a mesenchymal-like phenotype, is reversed upon testican 1 knock-down (Kim et al., 2014).
The role of other members of the testican family is less clear, as there are only a handful of published reports on this subject. It appears that testicans have a role in the inhibition of MMPs, a group of enzymes that catalyze matrix digestion and facilitate tumor cell invasion. Testican 3 was found to inhibit certain MMPs, potentially preventing cancer spread. Conversely, testican 2 was found to have an opposite effect, counteracting the inhibition imposed by testican 3 (Kim et al., 2014). In summary, contribution of the broader testican family to cancer progression requires further investigation.
The role of SLRP family of proteoglycans, which includes decorin, biglycan and lumican, in cancer is different between its members (reviewed in Appunni et al., 2019). SLRPs are abundant in the ECM of most tissues, where they bind to various cell receptors, regulating signaling cascades. For example, one of the SLRPs, decorin, has been shown to bind multiple receptor-tyrosine kinases, inhibiting their activation (Neill et al., 2016). Decorin was shown to have a particularly high affinity for the EGFR. Binding of decorin to EGFR led to ERK-mediated expression of p21 and subsequent cell cycle arrest (Moscatello et al., 1998). In addition, decorin can engage toll-like receptors 2 and 4, inducing inflammatory response. These functions explain the tumor suppressive and anti-metastatic role of decorin, which has been reported across several cancer types (reviewed in Neill et al., 2016).
Some of the members of the hyalectin family of proteoglycans have been shown to play a role in cancer. For instance, elevated levels of versican have been reported in gastric (Shen et al., 2015), lung (Asano et al., 2017), and endometrial cancer (Kodama et al., 2007), where its expression correlated with poor clinical prognosis. In breast cancer, expression of versican was only enriched in malignant tumors, but not in the non-malignant lesions (Vachon et al., 2007).
Brevican, has been found to promote motility of astrocytoma cells, supporting progression of this cancer from low- to high-grade glioma (Lu et al., 2012). Neurocan, another hyalectan that is found in the CNS, is increased in neuroblastomas. Several studies found that high neurocan expression has a strong correlation with shortened patient survival (Su et al., 2017; Zhong et al., 2018).
Hyaluronan, also referred to as hyaluronic acid, is a unique GAG which is composed entirely of the non-sulfated repeating disaccharides made of N-acetyl glucosamine (GlcNAc) and D-GlcA residues. As it does not have a protein core, it cannot be considered a proteoglycan. However, hyaluronan binds to multiple proteins and proteoglycans within the ECM and contributes greatly to structural integrity of different tissues, as well as to cancer development and progression. Its role in cancer is diverse and has been a subject of several comprehensive reviews (Rankin and Frankel, 2016; Liu et al., 2019).
Stiffer tumors are characterized by two important changes involving proteoglycans: a bulkier glycocalyx of the cancer cells, and a stiffer, denser ECM. Both of these changes require abundant proteoglycans with long, bulky GAG chain modifications (Table 1). Proteoglycans and hyaluronan, in either unbound or bound form, make up a significant part of cancer ECM and are actively involved in the regulation of its stiffness. One of the starkest pieces of evidence to support this comes from an unusual source – a study of the human tooth. The authors describe the proteoglycans present at the interfaces between different regions of the tooth that come under high levels of mechanical stress. These KS- and CS-decorated SLRPs are essential for the structural integrity of the tooth, as their enzymatic digestion reduces the stiffness of the tissue, making it less resistant to mechanical loads (Kurylo et al., 2016). The implication of this finding is that proteoglycans can markedly contribute to the regulation of tissue mechanics.
In cancer, perhaps the most notable example of this are the tumors of the CNS. Brain ECM contains large quantities of hyaluronan (Wolf et al., 2019), CS-modified proteoglycans, predominantly lecticans, as well as small glycoproteins and adhesive molecules – laminin, tenascin C, and fibronectin. Proteoglycans interact with the adhesive molecules, creating a network of varying density. For example, hyaluronan binding to tenascin C results in a more rigid ECM with higher stiffness. This is coupled with the fact that tenascin C is over-expressed in malignant tumors and is distributed in a gradient manner, with the highest abundance in the central regions, decreasing toward the periphery. This was shown to be controlled by HIF1α (Miroshnikova et al., 2016; Chen J.E. et al., 2018), which is highly expressed in the poorly vascularized, hypoxic regions in the core parts of the tumors. Both the presence of hypoxic areas and high stiffness have a strong association with poor prognosis in glioblastomas, suggesting that hypoxia-driven production of tenascin C, and its binding to hyaluronan underlie the mechanism of stiffness increase, which fuels glioblastoma progression (Miroshnikova et al., 2016). Other studies have shown that ECM enrichment with hyaluronan independently induces a stretched, contractile phenotype in glioma cells, promoting their invasion (Wang et al., 2014; Pogoda et al., 2017). Notably, hyaluronan abundance does not induce tumor cell proliferation, but instead reduces tumor volume. Despite this, it is associated with poor prognosis, as abundant hyaluronan promotes cancer cell invasion and dissemination, rather than the growth of a single parental tumor.
Outside of the CNS, proteoglycans are involved in mechano-transduction by modulating ECM composition and organization. Two SLRPs – decorin and biglycan are known to be essential for the regulation of collagen fiber assembly in tendons. A knock-down of these proteoglycans results in larger collagen fibers that can tolerate smaller mechanical loads. The overall tendon stiffness is also reduced upon decorin and biglycan knock-down (Zhang et al., 2006; Robinson et al., 2017). In a follow-up study, it was determined that the DS GAG chains attached to decorin were required for binding and remodeling of collagen (Lewis et al., 2010). These and other studies link decorin to mechano-regulation and indicate that the presence of SLRPs is important for the maintenance of soft ECM environments. In support of this, decorin acts as a tumor suppressor in many cancer types, while its loss precipitates cancer progression (Iozzo et al., 1999). Another SLRP, biglycan also contributes to the ECM remodeling. In melanoma models, the loss of biglycan expression in mouse embryonic fibroblasts results in softer ECM with less collagen crosslinking and reduced collagen fiber density. Among patients with melanoma, low expression of biglycan, either by the tumor or the stroma cells, is strongly linked to better clinical prognosis (Andrlová et al., 2017). In summary, the evidence of SLRPs’ involvement in regulation of mechanosignaling in cancers is still limited and merits further investigation.
Mounting evidence points to another secreted proteoglycan – agrin as a modulator of mechanosignaling in cancer. Agrin was first described as a ligand of Lrp4, which binds to a scaffolding protein MUSK. This complex serves as a platform for multiple signaling pathways, supporting cancer growth and preventing apoptosis (Zong and Jin, 2013). One of the down-stream signaling cascades that is activated by agrin is YAP/TAZ (Chakraborty et al., 2017).
In the same study, the authors uncovered the mechanism of mechano-sensing by agrin. Cells cultured on stiff substrates produce and secrete higher amounts of agrin. In turn, abundant agrin in the ECM potently increases stiffness of the tumors in vivo, which is dependent on collagen accumulation. Through its receptors, agrin stimulates YAP signaling, inducing YAP nuclear localization. Conversely, a knock-down of agrin results in YAP translocation back into the cytosol and a decreased expression of YAP target genes. Notably, this effect is exclusively mediated through agrin, while stiffness alone in absence of agrin expression is not sufficient to activate YAP and its target genes (Chakraborty et al., 2017). Corroborating the role of agrin in mechanosignaling, in the subsequent study of hepato-carcinomas, it was determined that agrin stabilizes focal adhesion complexes and facilitates FAK signaling. As a consequence, agrin depletion impedes cell adhesion and invasion and has a dramatic effect on tumor growth, practically eliminating tumors completely (Chakraborty et al., 2015).
In support of these findings, it was shown that agrin, produced both by the cancer cells, as well as the vascular endothelial cells, is required for activation of angiogenesis, blood vessel sprouting and cancer cell adhesion to the endothelial cells (Njah et al., 2019). The authors found that agrin binding to its Lrp4 receptor, induced β1 integrin – FAK signaling, formation of focal adhesion complexes and stabilized protein levels of VEGFR2, which is critical for endothelial cell migration and proliferation. Importantly, these agrin-mediated effects were stiffness-dependent, suggesting that stiff environments in tumors promote angiogenesis via agrin-mediated mechanosignaling (Njah et al., 2019).
Aggrecan is another proteoglycan of the ECM, whose expression is primarily limited to cartilage. Its abundance and modifications determine cartilage stiffness and elasticity. It has been shown that the length of CS and KS GAG chains attached to aggrecan decreases with age, along with an overall depletion of aggrecan. This results in progressively stiffer tissue (Nia et al., 2015). While this change occurs in normal development, it may become exacerbated with age and lead to development of osteoarthritis – degradation of cartilage (Alberton et al., 2019). It is feasible that aggrecan expression and the length of its GAG chains contribute to the regulation of osteosarcomas, or metastasis of other cancers to the bone. However, to the best of our knowledge, this has not yet been investigated.
The role of ECM proteoglycans in mechanosignaling has not been fully investigated. However, more is known about the transmembrane and peri-cellular proteoglycans in the context of mechano-regulation. It has been reported that tumors with up-regulated tissue mechanics have bulkier glycocalyx (Barnes et al., 2018). In addition, bulky glycocalyx further amplifies mechanosignaling through focal adhesions, creating a positive-feedback loop which propagates malignant phenotype (Figure 4) (Woods et al., 2017). As key components of glycocalyx, proteoglycans at the interface of the cell-to-matrix interaction, contribute to the regulation of this response.
Figure 4. Stiff matrix, rich in tenascin (T) and proteoglycans promote bulkier glycocalyx and up-regulate mechanosignaling through focal adhesions (FA) and down-stream pathways. Activation of mechanosignaling results in production of more proteoglycans that contribute to bulkier glycocalyx and stiffer ECM, thus concluding a positive-feedback loop in tumor tissue.
Some proteoglycans can serve as co-receptors of integrins. One of the best-studied examples of that is syndecans, which facilitate recognition and binding of external ligands, including growth factors and components of the ECM, through their HS chains within the range of (≈300 nm (Sarrazin et al., 2011). Syndecans interact with different integrins on the cell surface, forming diverse combinations. Their binding can occur both within the cytoplasmic and the extracellular domains, and facilitates formation and maturation of the adhesion complexes. Examples of this include interaction of Sdc-4 and Sdc-1 with α2β1 integrins, which promotes invasion of KRAS-mutant cells into collagen gels (Vuoriluoto et al., 2011). In addition, it was found that Sdc-1 mediates coupling of αvβ3 integrin with IGFR1 (Beauvais and Rapraeger, 2010). Sdc-1 directly binds to the integrins, which prompts formation of an integrin-IGFR1 complex and IGFR1 auto-phosphorylation. Integrin interaction with IGFR1 was found to be necessary for the activation of integrin signaling and cell spreading on substrates (Beauvais and Rapraeger, 2010).
Sdc-1 binding to disintegrin and MMP domain-containing protein 12 (ADAM 12) induces a conformational change that promotes binding of β1 integrins and subsequent cell spreading (Iba et al., 2000). In line with this, Sdc-1 expression in stromal fibroblasts induces rearrangement of the collagen fibers, allowing breast cancer cells to adhere and extravasate more efficiently (Yang et al., 2011). In summary, these studies provide evidence that Sdc-1 is involved in focal adhesion formation and supports activation of the down-stream signaling.
In addition, cell surface proteoglycans have been shown to act as mechano-sensors of shear force in vascular endothelial cells. In normal conditions, the endothelial cells align themselves with the direction of the flow, creating a uniform layer. One study has found that debulking of the endothelial glycocalyx with HS degrading heparinase III prevents such re-alignment of the cells. The authors found that this effect is dependent on Sdc-1, as the Sdc-1 knock-down also abolished cell realignment as well as remodeling of the fibrillar actin network, while a knock-down of glypican-1 did not (Ebong et al., 2014). The distinct roles of these proteoglycans in shear force sensing may be explained by the difference in their membrane tethering. Sdc-1 transverses the plasma membrane with a transmembrane domain that serves as a stable anchor connecting it to the cytoskeleton. In contrast, glypican-1 is more loosely attached with a GPI anchor and is often found within lipid rafts in the membrane. A recent study found that shear stress conditions induce re-distribution and clustering of glypican-1 on the cell surface, while Sdc-1 remains in place (Zeng et al., 2013).
Sdc-4 has also been described as essential for the formation and stabilization of focal adhesions (Woods and Couchman, 2001). Evidence in support of this includes a study, where mechanical force applied directly to the Sdc-4 through magnetic pulling of the conjugated beads, resulted in activation of β1-integrins, activation of down-stream signaling and an increase in cortical stiffness (Chronopoulos et al., 2020). Conversely, cells with a knock-down of Sdc-4 lose FAK and β1 integrins expression at the leading edge and fail to establish stable adhesions. Sdc-4 silencing also results in un-coupling of vinculin from filamentous actin network and its dispersion in the cytosol. As a result of these events, loss of Sdc-4 leads to reduced adhesion and increased proliferation (Cavalheiro et al., 2017). Furthermore, Sdc-4 has been shown to bind to the ECM proteins, such as fibronectin, with the HS chains, forming a stable deformation-resistant bond, adding to the strength of integrin-fibronectin binding (Kennelly et al., 2019).
Sdc-4, has been shown to directly interact with an α-actinin. α-actinin is an actin fiber-remodeling protein, which cooperates with myosin II to facilitate cell contractility and locomotion (Figure 2). Sdc-4 therefore serves as an important sensor of mechano-stimulation and regulator of cellular traction force (Okina et al., 2012).
All syndecans have also been shown to interact with a diverse group of PDZ-domain carrying proteins, which are important for tethering receptor proteins to the plasma membrane and serve as scaffolding proteins in signaling cascades (Cheng et al., 2016). Syndecans interact with PDZ-domain proteins through a conserved tetra-peptide region of their intracellular domain (Grootjans et al., 1997; Hsueh et al., 1998). These syndecan-PDZ protein complexes have been shown to be important for the cytoskeletal rearrangement, cell spreading and migration, with implications for cancer dissemination and metastasis (Tkachenko et al., 2006; Kashyap et al., 2015). Since there are four different syndecans and over 250 PDZ-domain proteins, specificity of their interactions is regulated through post-translational modifications of both the PDZ-domain and the cytoplasmic domain of syndecans, within or next to the PDZ-binding region. For example, phosphorylation of the cytoplasmic region of Sdc-1 prevents its interaction with syntenin-1 (Sulka et al., 2009), but it strengthens its binding with T-lymphoma invasion and metastasis-inducing protein 1 (Tiam1) (Keller-Pinter et al., 2017) – both PDZ-domain proteins, which regulate cytoskeletal organization and cell migration.
Finally, serglycin, as the only known intracellular proteoglycan has not been thoroughly investigated in terms of its contribution to mechanosignaling. One report has linked high abundance of serglycin with up-regulation of both mRNA expression and nuclear localization of YAP/TAZ (Zhang et al., 2020). YAP/TAZ activation is known to occur in response to multiple different inputs, although the mechanism of serglycin-mediated activation of YAP/TAZ is not completely understood. Stimulation of cells with either serglycin over-expression, or treatment with conditioned media collected from serglycin over-expressing cells, induced FAK signaling. This suggests that secreted serglycin promotes focal adhesion signaling, which in turn activates YAP/TAZ. In line with this, serglycin knock-down prevented activation of YAP/TAZ and the down-stream genes in a breast cancer model. This resulted in improved sensitivity to chemo-therapeutic treatment (Zhang et al., 2020).
Considering this intriguing observation, further studies are warranted to better understand the regulation of mechanosignaling by serglycin in cancer cells.
As outlined in chapters above, proteoglycans contribute to cancer progression, which is at least partially mediated through their role in mechanosignaling. From this perspective, proteoglycans represent attractive potential therapeutic targets. The search for clinical applications began in 1983 with the first report of the anti-angiogenic and anti-tumor properties of unfractionated heparin (Folkman et al., 1983). Since then, cancer type- and tumor stage-specific expression of certain proteoglycans have been described, positioning them as useful diagnostic and prognostic markers. For example, high expression of pericellular agrin on the interface between the cancer cells and the surrounding ECM, serves as a strong negative prognostic marker in PDAC. Knock-down of agrin, on the contrary, reduces both the primary and the metastatic tumor load in vivo (Tian et al., 2020). The study points to agrin’s role in the modulation of mechanosignaling, and support of adhesion and migration as the likely explanation for this effect. Changes in proteoglycan abundance and modifications also contribute to the ECM remodeling during tumor growth and the development of therapeutic resistance (Theocharis and Karamanos, 2019). For example, in melanoma, resistance against BRAF-inhibitor was accompanied by increased ECM levels of versican and biglycan (Girard et al., 2020). There is therefore evidence that inhibition of mechanosignaling, regulated by certain proteoglycans holds substantial therapeutic promise, although it mostly remains to be explored.
Another avenue of modifying proteoglycans for therapeutic gain is through modulation of their enzymatic processing (Shen et al., 2015). GAG chains attached to the protein cores of proteoglycans can be modified by sulfatases, which remove a sulfo group on HS chains, reducing the binding of growth factors. GAG chains can also be shortened or cleaved with a similar effect by appropriate enzymes, depending on the composition of the chain. For instance, in pancreatic cancer, increased levels of heparanase – the enzyme that cleaves HS GAGs, have been implicated in angiogenesis and metastasis, and correlated with poor prognosis (Pirinen et al., 2005).
This prompted investigation of heparanase inhibitors as potential therapeutics. For example, a significant tumor cell growth reduction in vitro and prolonged survival in vivo were reported upon treatment with a HS-mimetic heparanase inhibitor PG545. In addition to the survival advantage, PGP545 treatment also changed tumor cell phenotype, with the remaining tumor showing reduced levels of VEGF, vimentin, and collagen I. Histological analysis of these tumors showed high level of differentiation, suggesting that PGP545 may reverse tumor EMT (Ostapoff et al., 2013). In agreement with these findings, other studies found that PG545 treatment inhibited angiogenesis and reduced tumor burden in mouse models of breast, lung and prostate cancer (Kodama et al., 2007). Despite these promising preclinical results, as of 2019, no heparanase inhibitor has been tested in patients, as selective targeting of heparanase remains challenging (Shen et al., 2015).
Additionally, expression of specific proteoglycans by the tumor tissues can be exploited in various targeted drug delivery systems (Fuster and Esko, 2005; Misra et al., 2015). Nanocarrier systems such as liposomes or antibody-drug conjugates can improve drug delivery and anti-tumor activity of classical chemotherapeutics, while reducing their toxicity (Peer et al., 2007). Lee et al. developed cisplatin-loaded CS-binding liposomes, that effectively inhibited tumor growth in liver metastasis murine model (Lee et al., 2002). Similarly, antibody-drug conjugate targeting CSPG4 triggered melanoma regression in vivo (Hoffmann et al., 2020). Several similar approaches that utilize HSPGs have been proposed for treatment of breast (Khatun et al., 2013) and liver cancers (Longmuir et al., 2009).
Several groups identified cell surface proteoglycan glypican-1 as a specific marker of circulating cancer-cell-derived exosomes in pancreas and breast tumors. Glypican-1-positive exosomes may therefore serve as a tool for early detection of cancer (Melo et al., 2015). Similarly, HS proteoglycan Sdc-1 has been reported as an exosome constituent specific for glioblastoma, but not low-grade glioma (Indira Chandran et al., 2019).
Recently, chimeric antigen receptor (CAR) T-cells have been developed as a therapeutic tool that showed an impressive efficacy in B-cell lymphomas (Jackson et al., 2016). Subsequently, several cell surface proteoglycans have been suggested as CAR T-cell targets in solid tumors. Pellegatta et al. reported CART treatment targeted at CS proteoglycan 4 (CSPG4) was effective in glioblastoma mouse models (Pellegatta et al., 2018). Similarly, Glypican-3 was suggested as CAR-T-cell target for melanoma, lung and hepatocellular carcinoma, respectively (Shimizu et al., 2019). Despite groundbreaking discovery of CAR T-cell therapy in hematopoietic malignancies, their application in treatment of solid tumors remains challenging, likely due to the characteristic microenvironment of solid tumors (Newick et al., 2017). In summary, application of proteoglycans in cancer therapy holds potential, but requires further studies.
The role of tissue mechanics and mechanosignaling in tumor development and progression is slowly becoming appreciated. Considering that proteoglycans make up a significant part of the ECM and serve as an interface between the cancer cells and their environment, it is safe to assume their involvement in the regulation of mechano-sensing and mechanosignaling. In this review, we summarize published reports that support this assumption. We conclude that the role of proteoglycans in mechanosignaling deserves thorough investigation, as it may uncover novel therapeutic approaches that could potentially curb cancer progression in patients.
AB and VMW both conceived and wrote the review. ABJr and MŽ contributed sections of the review. All authors contributed to the article and approved the submitted version.
This work was supported by NIGMS 1R21GM133060-01 to ABJr, NCI 2R01CA227942-18A1, Cancer Research UK brain tumor award and NCI RO1CA227942, NINDS RO1NS109911-01 NCIRO1CA222508, NINDS 1R01NS109911-01 to VMW. AB and MŽ were supported by a grant from Cancer Research UK - R01CA174929; and an NIH NCI R01CA227942 grant.
The authors declare that the research was conducted in the absence of any commercial or financial relationships that could be construed as a potential conflict of interest.
bFGF, basic fibroblast growth factor; CSC, cancer stem cell; CAFs, cancer-associated fibroblasts; CNS, central nervous system; CS, chondroitin sulfate; JNK, c-JunN-terminal kinase; DS, dermatan sulfate; EGFR, epidermal growth factor receptor; EMT, epithelial to mesenchymal transition; ER, estrogen receptor; ECM, extracellular matrix; FGF, fibroblast growth factor; FAK, focal adhesion kinase; FA, focal adhesion; GlcA, glucuronic acid; GPI, glycosyl-phosphatidyl-inositol; GPC3, GPC4, glypican-3, 4; GAG, glycosaminoglycan; HS, heparan sulfate; HSPG, heparan sulphate proteoglycans; HIF1 α, hypoxia-inducible factor 1 α; IdoA, iduronic acid; IGFR1, insulin-like growth factor receptor 1; KS, keratan sulfate; Lrp4, lipoprotein-related receptor-4; MAPK, MAP kinase; MMPs, matrix metalloproteinases; MUSK, MMP domain-containing muscle-specific kinase; GalNAc, N-acetylgalactosamine; GlcNAc, N-acetylglucosamine; NCAMs, neural cell adhesion molecules; NG2, Neural/glial antigen 2; NRP1, Neuropilin-1; PDAC, pancreatic ductal adenocarcinoma; TAZ, PDZ-binding motif; ROCKI and ROCKII, Rho-associated protein kinase 1 and 2; SLRPs, small leucine-rich proteoglycans; Sdc-1, 2, Syndecan 1 and 2; TGF β R3, transforming growth factor β-receptor 3; VEGFR, vascular endothelial growth factor receptor; VASP, vasodilator-stimulated phosphoprotein; YAP, yes-associated protein; ADAM 12, protein 12.
Acerbi, I., Cassereau, L., Dean, I., Shi, Q., Au, A., Park, C., et al. (2015). Human breast cancer invasion and aggression correlates with ECM stiffening and immune cell infiltration. Integr. Biol. 7, 1120–1134. doi: 10.1039/c5ib00040h
Adler, M., Larocca, L., Trovato, F. M., Marcinkowski, H., Pasha, Y., and Taylor-Robinson, S. D. (2016). Evaluating the risk of hepatocellular carcinoma in patients with prominently elevated liver stiffness measurements by FibroScan: a multicentre study. HPB 18, 678–683. doi: 10.1016/j.hpb.2016.05.005
Ahmadzadeh, H., Webster, M. R., Behera, R., Jimenez Valencia, A. M., Wirtz, D., Weeraratna, A. T., et al. (2017). Modeling the two-way feedback between contractility and matrix realignment reveals a nonlinear mode of cancer cell invasion. Proc. Natl. Acad. Sci. U. S. A. 114, E1617–E1626. doi: 10.1073/pnas.1617037114
Alberton, P., Dugonitsch, H. C., Hartmann, B., Li, P., Farkas, Z., Saller, M. M., et al. (2019). Aggrecan hypomorphism compromises articular cartilage biomechanical properties and is associated with increased incidence of spontaneous osteoarthritis. Int. J. Mol. Sci. 20:1008. doi: 10.3390/ijms20051008
Alexander, N. R., Branch, K. M., Parekh, A., Clark, E. S., Iwueke, I. C., Guelcher, S. A., et al. (2008). Extracellular matrix rigidity promotes invadopodia activity. Curr. Biol. 18, 1295–1299. doi: 10.1016/j.cub.2008.07.090
Alowami, S., Troup, S., Al-Haddad, S., Kirkpatrick, I., and Watson, P. H. (2003). Mammographic density is related to stroma and stromal proteoglycan expression. Breast Cancer Res. 5, R129–R135. doi: 10.1186/bcr622
Amenta, P. S., Hadad, S., Lee, M. T., Barnard, N., Li, D., and Myers, J. C. (2003). Loss of types XV and XIX collagen precedes basement membrane invasion in ductal carcinoma of the female breast. J. Pathol. 199, 298–308. doi: 10.1002/path.1303
Andrlová, H., Mastroianni, J., Madl, J., Kern, J. S., Melchinger, W., Dierbach, H., et al. (2017). Biglycan expression in the melanoma microenvironment promotes invasiveness via increased tissue stiffness inducing integrin-β1 expression. Oncotarget 8, 42901–42916. doi: 10.18632/oncotarget.17160
Appunni, S., Anand, V., Khandelwal, M., Gupta, N., Rubens, M., and Sharma, A. (2019). Small leucine rich proteoglycans (decorin, biglycan and lumican) in cancer. Clin. Chim. Acta 491, 1–7. doi: 10.1016/j.cca.2019.01.003
Asano, K., Nelson, C. M., Nandadasa, S., Aramaki-Hattori, N., Lindner, D. J., Alban, T., et al. (2017). Stromal versican regulates tumor growth by promoting angiogenesis. Sci. Rep. 7:17225. doi: 10.1038/s41598-017-17613-6
Baba, F., Swartz, K., van Buren, R., Eickhoff, J., Zhang, Y., Wolberg, W., et al. (2006). Syndecan-1 and syndecan-4 are overexpressed in an estrogen receptor-negative, highly proliferative breast carcinoma subtype. Breast Cancer Res. Treat. 98, 91–98. doi: 10.1007/s10549-005-9135-2
Barbareschi, M., Maisonneuve, P., Aldovini, D., Cangi, M. G., Pecciarini, L., Mauri, F. A., et al. (2003). High syndecan-1 expression in breast carcinoma is related to an aggressive phenotype and to poorer prognosis. Cancer 98, 474–483. doi: 10.1002/cncr.11515
Barnes, J. M., Kaushik, S., Bainer, R. O., Sa, J. K., Woods, E. C., Kai, F., et al. (2018). A tension-mediated glycocalyx-integrin feedback loop promotes mesenchymal-like glioblastoma. Nat. Cell Biol. 20, 1203–1214. doi: 10.1038/s41556-018-0183-3
Beauvais, D. M., and Rapraeger, A. C. (2010). Syndecan-1 couples the insulin-like growth factor-1 receptor to inside-out integrin activation. J. Cell Sci. 123, 3796–3807. doi: 10.1242/jcs.067645
Bilandzic, M., and Stenvers, K. L. (2012). Reprint of: betaglycan: a multifunctional accessory. Mol. Cell. Endocrinol. 359, 13–22. doi: 10.1016/j.mce.2012.03.020
Bishop, J. R., Schuksz, M., and Esko, J. D. (2007). Heparan sulphate proteoglycans fine-tune mammalian physiology. Nature 446, 1030–1037. doi: 10.1038/nature05817
Bonnet, F., Perin, J. P., Maillet, P., Jolles, P., and Alliel, P. M. (1992). Characterization of a human seminal plasma glycosaminoglycan-bearing polypeptide. Biochem. J. 288, 565–569. doi: 10.1042/bj2880565
Buffone, A., Mondal, N., Gupta, R., McHugh, K. P., Lau, J. T. Y., and Neelamegham, S. (2013). Silencing α1,3-fucosyltransferases in human leukocytes reveals a role for FUT9 enzyme during E-selectin-mediated cell adhesion. J. Biol. Chem. 288, 1620–1633. doi: 10.1074/jbc.m112.400929
Buffone, A., Nasirikenari, M., Manhardt, C. T., Lugade, A., Bogner, P. N., Sackstein, R., et al. (2017). Leukocyte-borne α(1,3)-fucose is a negative regulator of β 2 -integrin-dependent recruitment in lung inflammation. J. Leukoc. Biol. 101, 459–470. doi: 10.1189/jlb.3a0516-215rr
Buffone, A., and Weaver, V. M. (2020). Don’t sugarcoat it: how glycocalyx composition influences cancer progression. J. Cell Biol. 219:e201910070. doi: 10.1083/jcb.201910070
Butcher, D. T., Alliston, T., and Weaver, V. M. (2009). A tense situation: forcing tumour progression. Nat. Rev. Cancer 9, 108–122. doi: 10.1038/nrc2544
Cabodi, S., del Pilar Camacho-Leal, M., Di Stefano, P., and Defilippi, P. (2010). Integrin signalling adaptors: not only figurants in the cancer story. Nat. Rev. Cancer 10, 858–870. doi: 10.1038/nrc2967
Cao, S., Yaqoob, U., Das, A., Shergill, U., Jagavelu, K., Huebert, R. C., et al. (2010). Neuropilin-1 promotes cirrhosis of the rodent and human liver by enhancing PDGF/TGF-beta signaling in hepatic stellate cells. J. Clin. Invest. 120, 2379–2394. doi: 10.1172/JCI41203
Capurro, M. I., Xiang, Y.-Y., Lobe, C., and Filmus, J. (2005). Glypican-3 promotes the growth of hepatocellular carcinoma by stimulating canonical Wnt signaling. Cancer Res. 65, 6245–6254. doi: 10.1158/0008-5472.CAN-04-4244
Capurro, M. I., Xu, P., Shi, W., Li, F., Jia, A., and Filmus, J. (2008). Glypican-3 inhibits hedgehog signaling during development by competing with patched for Hedgehog binding. Dev. Cell 14, 700–711. doi: 10.1016/j.devcel.2008.03.006
Cassiman, J. J., Van den Berghe, H., and David, G. (1992). Differential expression of cell surface heparan sulfate proteoglycans in human mammary epithelial cells and lung fibroblasts. J. Biol. 267, 1116–1122.
Cavalheiro, R. P., Lima, M. A., Jarrouge-Bouças, T. R., Viana, G. M., Lopes, C. C., Coulson-Thomas, V. J., et al. (2017). Coupling of vinculin to F-actin demands Syndecan-4 proteoglycan. Matrix Biol. 63, 23–37. doi: 10.1016/j.matbio.2016.12.006
Chakraborty, S., Lakshmanan, M., Swa, H. L. F., Chen, J., Zhang, X., Ong, Y. S., et al. (2015). An oncogenic role of Agrin in regulating focal adhesion integrity in hepatocellular carcinoma. Nat. Commun. 6:6184. doi: 10.1038/ncomms7184
Chakraborty, S., Njah, K., Pobbati, A. V., Lim, Y. B., Raju, A., Lakshmanan, M., et al. (2017). Agrin as a mechanotransduction signal regulating YAP through the hippo pathway. Cell Rep. 18, 2464–2479. doi: 10.1016/j.celrep.2017.02.041
Charchanti, A., Papoudou Bai, A., Samantas, E., Papakostas, P., Skarlos, P., Kanavaros, P., et al. (2019). Association of low Syndecan-1 expression with adverse histopathological parameters in gastric carcinomas. J. BUON 24, 1106–1112.
Chen, J. E., Lumibao, J., Blazek, A., Gaskins, H. R., and Harley, B. (2018). Hypoxia activates enhanced invasive potential and endogenous hyaluronic acid production by glioblastoma cells. Biomater Sci. 6, 854–862. doi: 10.1039/c7bm01195d
Chen, L. L., Gao, G.-X., Shen, F.-X., Chen, X., Gong, X.-H., and Wu, W.-J. (2018). SDC4 gene silencing favors human papillary thyroid carcinoma cell apoptosis and inhibits epithelial mesenchymal transition via Wnt/β-catenin pathway. Mol. Cells 41, 853–867. doi: 10.14348/molcells.2018.0103
Cheng, B., Montmasson, M., Terradot, L., and Rousselle, P. (2016). Syndecans as cell surface receptors in cancer biology. a focus on their interaction with PDZ domain proteins. Front. Pharmacol. 7:10. doi: 10.3389/fphar.2016.00010
Chronopoulos, A., Thorpe, S. D., Cortes, E., Lachowski, D., Rice, A. J., Mykuliak, V. V., et al. (2020). Syndecan-4 tunes cell mechanics by activating the kindlin-integrin-RhoA pathway. Nat. Mater. 19, 669–678. doi: 10.1038/s41563-019-0567-1
Chute, C., Yang, X., Meyer, K., Yang, N., O’Neil, K., Kasza, I., et al. (2018). Syndecan-1 induction in lung microenvironment supports the establishment of breast tumor metastases. Breast Cancer Res. 20:66. doi: 10.1186/s13058-018-0995-x
Couchman, J. R., and Pataki, C. A. (2012). An introduction to proteoglycans and their localization. J. Histochem. Cytochem. 60, 885–897. doi: 10.1369/0022155412464638
Cruz, L. A., Tellman, T. V., and Farach-Carson, M. C. (2020). Flipping the molecular switch: influence of perlecan and its modifiers in the tumor microenvironment. Adv. Exp. Med. Biol. 1245, 133–146. doi: 10.1007/978-3-030-40146-7_6
Davies, J. E., Tang, X., Denning, J. W., Archibald, S. J., and Davies, S. J. A. (2004). Decorin suppresses neurocan, brevican, phosphacan and NG2 expression and promotes axon growth across adult rat spinal cord injuries. Eur. J. Neurosci. 19, 1226–1242. doi: 10.1111/j.1460-9568.2004.03184.x
DuFort, C. C., DelGiorno, K. E., Carlson, M. A., Osgood, R. J., Zhao, C., Huang, Z., et al. (2016). Interstitial pressure in pancreatic ductal adenocarcinoma is dominated by a gel-fluid phase. Biophys. J. 110, 2106–2119. doi: 10.1016/j.bpj.2016.03.040
DuFort, C. C., Paszek, M. J., and Weaver, V. M. (2011). Balancing forces: architectural control of mechanotransduction. Nat. Rev. Mol. Cell Biol. 12, 308–319. doi: 10.1038/nrm3112
Dupont, S. (2016). Role of YAP/TAZ in cell-matrix adhesion-mediated signalling and mechanotransduction. Exp. Cell Res. 343, 42–53. doi: 10.1016/j.yexcr.2015.10.034
Dupont, S., Morsut, L., Aragona, M., Enzo, E., Giulitti, S., Cordenonsi, M., et al. (2011). Role of YAP/TAZ in mechanotransduction. Nature 474, 179–183. doi: 10.1038/nature10137
Ebong, E. E., Lopez-Quintero, S. V., Rizzo, V., Spray, D. C., and Tarbell, J. M. (2014). Shear-induced endothelial NOS activation and remodeling via heparan sulfate, glypican-1, and syndecan-1. Integr. Biol. 6, 338–347. doi: 10.1039/c3ib40199e
Eklund, L., Piuhola, J., Komulainen, J., Sormunen, R., Ongvarrasopone, C., Fassler, R., et al. (2001). Lack of type XV collagen causes a skeletal myopathy and cardiovascular defects in mice. Proc. Natl. Acad. Sci. U.S.A. 98, 1194–1199. doi: 10.1073/pnas.98.3.1194
Elosegui-Artola, A., Andreu, I., Beedle, A. E. M., Lezamiz, A., Uroz, M., Kosmalska, A. J., et al. (2017). Force triggers YAP nuclear entry by regulating transport across nuclear pores. Cell 171, 1397.e14–1410.e14. doi: 10.1016/j.cell.2017.10.008
Faham, S., Hileman, R. E., Fromm, J. R., Linhardt, R. J., and Rees, D. C. (1996). Heparin structure and interactions with basic fibroblast growth factor. Science 271, 1116–1120. doi: 10.1126/science.271.5252.1116
Fang, Y., Shen, Z.-Y., Zhan, Y.-Z., Feng, X.-C., Chen, K.-L., Li, Y.-S., et al. (2019). CD36 inhibits β-catenin/c-myc-mediated glycolysis through ubiquitination of GPC4 to repress colorectal tumorigenesis. Nat Commun. 10:3981. doi: 10.1038/s41467-019-11662-3
Farfán, N., Ocarez, N., Castellón, E. A., Mejía, N., de Herreros, A. G., and Contreras, H. R. (2018). The transcriptional factor ZEB1 represses Syndecan 1 expression in prostate cancer. Sci. Rep. 8:11467. doi: 10.1038/s41598-018-29829-1
Filmus, J., and Capurro, M. (2014). The role of glypicans in hedgehog signaling. Matrix Biol. 35, 248–252. doi: 10.1016/j.matbio.2013.12.007
Fjeldstad, K., and Kolset, S. O. (2005). Decreasing the metastatic potential in cancers–targeting the heparan sulfate proteoglycans. Curr. Drug Targets 6, 665–682. doi: 10.2174/1389450054863662
Fleurot, E., Goudin, C., Hanoux, V., Bonnamy, P.-J., and Levallet, J. (2019). Estrogen receptor α regulates the expression of syndecan-1 in human breast carcinoma cells. Endocr. Relat. Cancer 26, 615–628. doi: 10.1530/erc-18-0285
Folkman, J., Langer, R., Linhardt, R. J., Haudenschild, C., and Taylor, S. (1983). Angiogenesis inhibition and tumor regression caused by heparin or a heparin fragment in the presence of cortisone. Science 221, 719–725. doi: 10.1126/science.6192498
Forsten-Williams, K., Chu, C. L., Fannon, M., Buczek-Thomas, J. A., and Nugent, M. A. (2008). Control of growth factor networks by heparan sulfate proteoglycans. Ann. Biomed. Eng. 36, 2134–2148. doi: 10.1007/s10439-008-9575-z
Frischknecht, R., and Seidenbecher, C. I. (2012). Brevican: a key proteoglycan in the perisynaptic extracellular matrix of the brain. Int. J. Biochem. Cell Biol. 44, 1051–1054. doi: 10.1016/j.biocel.2012.03.022
Funderburgh, J. L. (2002). Keratan sulfate biosynthesis. IUBMB Life 54, 187–194. doi: 10.1080/15216540214932
Fuster, M. M., and Esko, J. D. (2005). The sweet and sour of cancer: glycans as novel therapeutic targets. Nat. Rev. Cancer 5, 526–542. doi: 10.1038/nrc1649
Gandhi, N. S., and Mancera, R. L. (2008). The structure of glycosaminoglycans and their interactions with proteins. Chem. Biol. Drug Des. 72, 455–482. doi: 10.1111/j.1747-0285.2008.00741.x
Girard, C. A., Lecacheur, M., Ben Jouira, R., Berestjuk, I., Diazzi, S., Prod’homme, V., et al. (2020). A feed-forward mechanosignaling loop confers resistance to therapies targeting the MAPK pathway in BRAF-mutant melanoma. Cancer Res. 80, 1927–1941. doi: 10.1158/0008-5472.CAN-19-2914
Golding, J. P., Tidcombe, H., Tsoni, S., and Gassmann, M. (1999). Chondroitin sulphate-binding molecules may pattern central projections of sensory axons within the cranial mesenchyme of the developing mouse. Dev. Biol. 216, 85–97. doi: 10.1006/dbio.1999.9497
Graham, K., Murphy, J. I., and Dhoot, G. K. (2016). SULF1/SULF2 reactivation during liver damage and tumour growth. Histochem. Cell Biol. 146, 85–97. doi: 10.1007/s00418-016-1425-8
Grindel, B. J., Martinez, J. R., Tellman, T. V., Harrington, D. A., Zafar, H., Nakhleh, L., et al. (2018). Matrilysin/MMP-7 cleavage of perlecan/HSPG2 complexed with semaphorin 3A supports FAK-mediated stromal invasion by prostate cancer cells. Sci. Rep. 8:7262. doi: 10.1038/s41598-018-25435-3
Grootjans, J. J., Zimmermann, P., Reekmans, G., Smets, A., Degeest, G., Dürr, J., et al. (1997). Syntenin, a PDZ protein that binds syndecan cytoplasmic domains. Proc. Natl. Acad. Sci. U. S. A. 94, 13683–13688. doi: 10.1073/pnas.94.25.13683
Gubbiotti, M. A., Neill, T., and Iozzo, R. V. (2017). A current view of perlecan in physiology and pathology: a mosaic of functions. Matrix Biol. 5, 285–298. doi: 10.1016/j.matbio.2016.09.003
Guo, J.-Y., Hsu, H.-S., Tyan, S.-W., Li, F.-Y., Shew, J.-Y., Lee, W.-H., et al. (2017). Serglycin in tumor microenvironment promotes non-small cell lung cancer aggressiveness in a CD44-dependent manner. Oncogene 36, 2457–2471. doi: 10.1038/onc.2016.404
Hao, W., Han, J., Chu, Y., Huang, L., Zhuang, Y., Sun, J., et al. (2018). Collagen/Heparin Bi-affinity multilayer modified collagen scaffolds for controlled bFGF release to improve angiogenesis in vivo. Macromol. Biosci. 18:1870028. doi: 10.1002/mabi.201870028
Hassan, H., Greve, B., Pavao, M. S. G., Kiesel, L., Ibrahim, S. A., and Götte, M. (2013). Syndecan-1 modulates β-integrin-dependent and interleukin-6-dependent functions in breast cancer cell adhesion, migration, and resistance to irradiation. FEBS J. 280, 2216–2227. doi: 10.1111/febs.12111
Heinämäki, P., Rehn, M., Kivirikko, S., Muona, A., Hintikka, E., and Pihlajaniemi, T. (1994). Exon-intron organization of the human type VI collagen gene and comparison of type XV collagen with its homologue tyep XVIII. Matrix Biol. 14, 351–352. doi: 10.1016/0945-053x(94)90035-3
Heinegård, D. (2009). Fell-muir lecture: proteoglycans and more - from molecules to biology. International J. Exp. Pathol. 90, 575–586. doi: 10.1111/j.1365-2613.2009.00695.x
Hendricks, C., Dubail, J., Brohee, L., Delforge, Y., Colige, A., and Deroanne, C. (2016). A novel physiological glycosaminoglycan-deficient splice variant of neuropilin-1 is anti-tumorigenic in vitro and in vivo. PLoS One 11:e0165153. doi: 10.1371/journal.pone.0165153
Hoffmann, R. M., Crescioli, S., Mele, S., Sachouli, E., Cheung, A., Chui, C. K., et al. (2020). A novel antibody-drug conjugate (ADC) delivering a DNA mono-alkylating payload to chondroitin sulfate proteoglycan (CSPG4)-Expressing Melanoma. Cancers 12:1029. doi: 10.3390/cancers12041029
Hsueh, Y. P., Yang, F. C., Kharazia, V., Naisbitt, S., Cohen, A. R., Weinberg, R. J., et al. (1998). Direct interaction of CASK/LIN-2 and syndecan heparan sulfate proteoglycan and their overlapping distribution in neuronal synapses. J. Cell Biol. 142, 139–151. doi: 10.1083/jcb.142.1.139
Humphries, J. D., Byron, A., and Humphries, M. J. (2006). Integrin ligands at a glance. J. Cell Sci. 119, 3901–3903. doi: 10.1242/jcs.03098
Iba, K., Albrechtsen, R., Gilpin, B., Frohlich, C., Loechel, F., Zolkiewska, A., et al. (2000). The cysteine-rich domain of human ADAM 12 supports cell adhesion through syndecans and triggers signaling events that lead to beta1 integrin-dependent cell spreading. J. Cell Biol. 149, 1143–1156. doi: 10.1083/jcb.149.5.1143
Ibrahim, S. A., Gadalla, R., El-Ghonaimy, E. A., Samir, O., Mohamed, H. T., Hassan, H., et al. (2017). Syndecan-1 is a novel molecular marker for triple negative inflammatory breast cancer and modulates the cancer stem cell phenotype via the IL-6/STAT3, Notch and EGFR signaling pathways. Mol. Cancer 16:57. doi: 10.1186/s12943-017-0621-z
Ibrahim, S. A., Yip, G. W., Stock, C., Pan, J.-W., Neubauer, C., Poeter, M., et al. (2012). Targeting of syndecan-1 by microRNA miR-10b promotes breast cancer cell motility and invasiveness via a Rho-GTPase- and E-cadherin-dependent mechanism. Int. J. Cancer 131, E884–E896. doi: 10.1002/ijc.27629
Iizasa, T., Chang, H., Suzuki, M., Otsuji, M., Yokoi, S., Chiyo, M., et al. (2004). Overexpression of collagen XVIII is associated with poor outcome and elevated levels of circulating serum endostatin in non-small cell lung cancer. Clin. Cancer Res. 10, 5361–5366. doi: 10.1158/1078-0432.CCR-04-43
Indira Chandran, V., Welinder, C., Mansson, A.-S., Offer, S., Freyhult, E., Pernemalm, M., et al. (2019). Ultrasensitive immunoprofiling of plasma extracellular vesicles identifies syndecan-1 as a potential tool for minimally invasive diagnosis of glioma. Clin. Cancer Res. 25, 3115–3127. doi: 10.1158/1078-0432.CCR-18-2946
Iozzo, R. V. (1997). The family of the small leucine-rich proteoglycans: key regulators of matrix assembly and cellular growth. Crit. Rev. Biochem. Mol. Biol. 32, 141–174. doi: 10.3109/10409239709108551
Iozzo, R. V. (1998). Matrix proteoglycans: from molecular design to cellular function. Annu. Rev. Biochem. 67, 609–652. doi: 10.1146/annurev.biochem.67.1.609
Iozzo, R. V. (1999). The biology of the small leucine-rich proteoglycans. J. Biol. Chem. 274, 18843–18846. doi: 10.1074/jbc.274.27.18843
Iozzo, R. V., Chakrani, F., Perrotti, D., McQuillan, D. J., Skorski, T., Calabretta, B., et al. (1999). Cooperative action of germ-line mutations in decorin and p53 accelerates lymphoma tumorigenesis. Proc. Natl. Acad. Sci. U. S. A. 96, 3092–3097. doi: 10.1073/pnas.96.6.3092
Iozzo, R. V., Goldoni, S., Berendsen, A. D., and Young, M. F. (2011). Small Leucine-Rich Proteoglycans. Berlin: Springer, doi: 10.1007/978-3-642-16555-9_6
Iozzo, R. V., and Schaefer, L. (2015). Proteoglycan form and function: a comprehensive nomenclature of proteoglycans. Matrix Biol. 42, 11–55. doi: 10.1016/j.matbio.2015.02.003
Iozzo, R. V., Zoeller, J. J., and Nyström, A. (2009). Basement membrane proteoglycans: modulators Par Excellence of cancer growth and angiogenesis. Mol. Cells 27, 503–513. doi: 10.1007/s10059-009-0069-0
Jackson, H. J., Rafiq, S., and Brentjens, R. J. (2016). Driving CAR T-cells forward. Nat. Rev. Clin. Oncol. 13, 370–383. doi: 10.1038/nrclinonc.2016.36
Jang, B., Jung, H., Chung, H., Moon, B.-I., and Oh, E.-S. (2016). Syndecan-2 enhances E-cadherin shedding and fibroblast-like morphological changes by inducing MMP-7 expression in colon cancer cells. Biochem. Biophys. Res. Commun. 477, 47–53. doi: 10.1016/j.bbrc.2016.06.019
Janoštiak, R., Pataki, A. C., Brábek, J., and Rösel, D. (2014). Mechanosensors in integrin signaling: the emerging role of p130Cas. Eur. J. Cell Biol. 93, 445–454. doi: 10.1016/j.ejcb.2014.07.002
Jung, M., Lord, M. S., Cheng, B., Guy Lyons, J., Alkhouri, H., Margaret Hughes, J., et al. (2013). Mast cells produce novel shorter forms of perlecan that contain functional endorepellin. J. Biol. Chem. 288, 3289–3304. doi: 10.1074/jbc.m112.387811
Kalscheuer, S., Khanna, V., Kim, H., Li, S., Sachdev, D., DeCarlo, A., et al. (2019). Discovery of HSPG2 (Perlecan) as a therapeutic target in triple negative breast cancer. Sci. Rep. 9:12492. doi: 10.1038/s41598-019-48993-6
Kanchanawong, P., Shtengel, G., Pasapera, A. M., Ramko, E. B., Davidson, M. W., Hess, H. F., et al. (2010). Nanoscale architecture of integrin-based cell adhesions. Nature 468, 580–584. doi: 10.1038/nature09621
Kashyap, R., Roucourt, B., Lembo, F., Fares, J., Carcavilla, A. M., Restouin, A., et al. (2015). Syntenin controls migration, growth, proliferation, and cell cycle progression in cancer cells. Front. Pharmacol. 6:241. doi: 10.3389/fphar.2015.00241
Katakam, S. K., Tria, V., Sim, W., Yip, G. W., Molgora, S., Karnavas, T., et al. (2020). The heparan sulfate proteoglycan syndecan-1 regulates colon cancer stem cell function via a focal adhesion kinase—Wnt signaling axis. FEBS J. Online ahead of print doi: 10.1111/febs.15356
Keller-Pinter, A., Ughy, B., Domoki, M., Pettko-Szandtner, A., Letoha, T., Tovari, J., et al. (2017). The phosphomimetic mutation of syndecan-4 binds and inhibits Tiam1 modulating Rac1 activity in PDZ interaction–dependent manner. PLoS One 12:e0187094. doi: 10.1371/journal.pone.0187094
Kennelly, T. M., Li, Y., Cao, Y., Qwarnstrom, E. E., and Geoghegan, M. (2019). Distinct binding interactions of α5β1-integrin and proteoglycans with fibronectin. Biophys. J. 117, 688–695. doi: 10.1016/j.bpj.2019.07.002
Khatun, Z., Nurunnabi, M., Reeck, G. R., Cho, K. J., and Lee, Y.-K. (2013). Oral delivery of taurocholic acid linked heparin-docetaxel conjugates for cancer therapy. J. Control. Release 170, 74–82. doi: 10.1016/j.jconrel.2013.04.024
Kim, H.-P., Han, S.-W., Song, S.-H., Jeong, E.-G., Lee, M.-Y., Hwang, D., et al. (2014). Testican-1-mediated epithelial-mesenchymal transition signaling confers acquired resistance to lapatinib in HER2-positive gastric cancer. Oncogene 33, 3334–3341. doi: 10.1038/onc.2013.285
Kim, Y., and Kumar, S. (2014). CD44-mediated adhesion to hyaluronic acid contributes to mechanosensing and invasive motility. Mol. Cancer Res. 12, 1416–1429. doi: 10.1158/1541-7786.MCR-13-0629
Kind, S., Merenkow, C., Büscheck, F., Möller, K., Dum, D., Chirico, V., et al. (2019). Prevalence of syndecan-1 (CD138) expression in different kinds of human tumors and normal tissues. Disease Markers 2019:4928315. doi: 10.1155/2019/4928315
Kodama, J., Hasengaowa, Kusumoto, T., Seki, N., Matsuo, T., Ojima, Y., et al. (2007). Prognostic significance of stromal versican expression in human endometrial cancer. Ann. Oncol. 18, 269–274. doi: 10.1093/annonc/mdl370
Kolset, S. O., and Tveit, H. (2008). Serglycin–structure and biology. Cell. Mol. Life Sci. 65, 1073–1085.
Kolseth, I. B. M., Reine, T. M., Vuong, T. T., Meen, A. J., Fan, Q., Jenssen, T. G., et al. (2015). Serglycin is part of the secretory repertoire of LPS-activated monocytes. Immun. Inflamm. Dis. 3, 23–31. doi: 10.1002/iid3.47
Kurylo, M. P., Grandfield, K., Marshall, G. W., Altoe, V., Aloni, S., and Ho, S. P. (2016). Effect of proteoglycans at interfaces as related to location, architecture, and mechanical cues. Arch. Oral Biol. 63, 82–92. doi: 10.1016/j.archoralbio.2015.11.021
Laklai, H., Miroshnikova, Y. A., Pickup, M. W., Collisson, E. A., Kim, G. E., Barrett, A. S., et al. (2016). Genotype tunes pancreatic ductal adenocarcinoma tissue tension to induce matricellular fibrosis and tumor progression. Nat. Med. 22, 497–505. doi: 10.1038/nm.4082
Lee, C. M., Tanaka, T., Murai, T., Kondo, M., Kimura, J., Su, W., et al. (2002). Novel chondroitin sulfate-binding cationic liposomes loaded with cisplatin efficiently suppress the local growth and liver metastasis of tumor cells in vivo. Cancer Res. 62, 4282–4288.
Lee, K. S., Park, G. S., Hong, S. H., Byun, J. H., Woo, I. S., Jeon, H.-M., et al. (2010). Prognostic relevance of collagen XVIII expression in metastatic gastric carcinoma. Tumour Biol. 31, 165–170. doi: 10.1007/s13277-010-0022-z
Levental, K. R., Yu, H., Kass, L., Lakins, J. N., Egeblad, M., Erler, J. T., et al. (2009). Matrix crosslinking forces tumor progression by enhancing integrin signaling. Cell 139, 891–906. doi: 10.1016/j.cell.2009.10.027
Lewis, J. L., Krawczak, D. A., Oegema, T. R. Jr., and Westendorf, J. J. (2010). Effect of decorin and dermatan sulfate on the mechanical properties of a neocartilage. Connect. Tissue Res. 51, 159–170. doi: 10.3109/03008200903174342
Li, K., Li, L., Wu, X., Yu, J., Ma, H., Zhang, R., et al. (2019). Loss of SDC1 expression is associated with poor prognosis of colorectal cancer patients in Northern China. Dis. Markers 2019:3768708. doi: 10.1155/2019/3768708
Li, N., Gao, W., Zhang, Y.-F., and Ho, M. (2018). Glypicans as cancer therapeutic targets. Trends Cancer Res. 4, 741–754. doi: 10.1016/j.trecan.2018.09.004
Li, R., Li, Y., Wu, Y., Zhao, Y., Chen, H., Yuan, Y., et al. (2018). Heparin-Poloxamer thermosensitive hydrogel loaded with bFGF and NGF enhances peripheral nerve regeneration in diabetic rats. Biomaterials 168, 24–37. doi: 10.1016/j.biomaterials.2018.03.044
Li, Y., Sun, C., Yates, E. A., Jiang, C., Wilkinson, M. C., and Fernig, D. G. (2016). Heparin binding preference and structures in the fibroblast growth factor family parallel their evolutionary diversification. Open Biol. 6:150275. doi: 10.1098/rsob.150275
Lin, X. (2004). Functions of heparan sulfate proteoglycans in cell signaling during development. Development 131, 6009–6021. doi: 10.1242/dev.01522
Lindahl, U., Couchman, J., Kimata, K., and Esko, J. D. (2015). Proteoglycans and Sulfated Glycosaminoglycans. Available online at: https://europepmc.org/books/n/glyco3/ch17/?extid=28876832&src=med (accessed April 25, 2020).
Liu, B. P., Cafferty, W. B. J., Budel, S. O., and Strittmatter, S. M. (2006). Extracellular regulators of axonal growth in the adult central nervous system. Philos. Trans. R. Soc. B Biol. Sci. 361, 1593–1610. doi: 10.1098/rstb.2006.1891
Liu, C.-Y., Lin, H.-H., Tang, M.-J., and Wang, Y.-K. (2015). Vimentin contributes to epithelial-mesenchymal transition cancer cell mechanics by mediating cytoskeletal organization and focal adhesion maturation. Oncotarget 6, 15966–15983. doi: 10.18632/oncotarget.3862
Liu, M., Tolg, C., and Turley, E. (2019). Dissecting the dual nature of hyaluronan in the tumor microenvironment. Front. Immunol. 10:947. doi: 10.3389/fimmu.2019.00947
Longmuir, K. J., Haynes, S. M., Baratta, J. L., Kasabwalla, N., and Robertson, R. T. (2009). Liposomal delivery of doxorubicin to hepatocytes in vivo by targeting heparan sulfate. Int. J. Pharm. 382, 222–233. doi: 10.1016/j.ijpharm.2009.07.030
Lu, R., Wu, C., Guo, L., Liu, Y., Mo, W., Wang, H., et al. (2012). The role of brevican in glioma: promoting tumor cell motility in vitro and in vivo. BMC Cancer 12:607. doi: 10.1186/1471-2407-12-607
Lundström, E., Sahlin, L., Skoog, L., Hägerström, T., Svane, G., Azavedo, E., et al. (2006). Expression of syndecan-1 in histologically normal breast tissue from postmenopausal women with breast cancer according to mammographic density. Climacteric 9, 277–282. doi: 10.1080/13697130600865741
Maller, O., Drain, A. P., Barrett, A. S., Borgquist, S., Ruffell, B., Thanh, P. T., et al. (2020). Inflammation promotes tumor aggression by stimulating stromal cell-dependent collagen crosslinking and stromal stiffening. bioRxiv [Preprint] doi: 10.1101/2020.02.13.948141
Manou, D., Bouris, P., Kletsas, D., Götte, M., Greve, B., Moustakas, A., et al. (2020). Serglycin activates pro-tumorigenic signaling and controls glioblastoma cell stemness, differentiation and invasive potential. Matrix Biol. Plus 6:100033. doi: 10.1016/j.mbplus.2020.100033
Matte, B. F., Kumar, A., Placone, J. K., Zanella, V. G., Martins, M. D., Engler, A. J., et al. (2019). Matrix stiffness mechanically conditions EMT and migratory behavior of oral squamous cell carcinoma. J. Cell Sci. 132:jcs224360. doi: 10.1242/jcs.224360
Maurel, P., Rauch, U., Flad, M., Margolis, R. K., and Margolis, R. U. (1994). Phosphacan, a chondroitin sulfate proteoglycan of brain that interacts with neurons and neural cell-adhesion molecules, is an extracellular variant of a receptor-type protein tyrosine phosphatase. Proc. Natl. Acad. Sci. U.S.A. 91, 2512–2516. doi: 10.1073/pnas.91.7.2512
Mekhdjian, A. H., Kai, F., Rubashkin, M. G., Prahl, L. S., Przybyla, L. M., McGregor, A. L., et al. (2017). Integrin-mediated traction force enhances paxillin molecular associations and adhesion dynamics that increase the invasiveness of tumor cells into a three-dimensional extracellular matrix. Mol. Biol. Cell 28, 1467–1488. doi: 10.1091/mbc.e16-09-0654
Melo, S. A., Luecke, L. B., Kahlert, C., Fernandez, A. F., Gammon, S. T., Kaye, J., et al. (2015). Glypican-1 identifies cancer exosomes and detects early pancreatic cancer. Nature 523, 177–182. doi: 10.1038/nature14581
Miao, L., Wang, Y., Xia, H., Yao, C., Cai, H., and Song, Y. (2013). SPOCK1 is a novel transforming growth factor-β target gene that regulates lung cancer cell epithelial-mesenchymal transition. Biochem. Biophys. Res. Commun. 440, 792–797. doi: 10.1016/j.bbrc.2013.10.024
Miroshnikova, Y. A., Mouw, J. K., Barnes, J. M., Pickup, M. W., Lakins, J. N., Kim, Y., et al. (2016). Tissue mechanics promote IDH1-dependent HIF1α-tenascin C feedback to regulate glioblastoma aggression. Nat. Cell Biol. 18, 1336–1345. doi: 10.1038/ncb3429
Misra, S., Hascall, V. C., Atanelishvili, I., Moreno Rodriguez, R., Markwald, R. R., and Ghatak, S. (2015). Utilization of glycosaminoglycans/proteoglycans as carriers for targeted therapy delivery. Int. J. Cell Biol. 2015:537560. doi: 10.1155/2015/537560
Mondal, N., Stolfa, G., Antonopoulos, A., Zhu, Y., Wang, S.-S., Buffone, A., et al. (2016). Glycosphingolipids on human myeloid cells stabilize E-selectin–dependent rolling in the multistep leukocyte adhesion cascadesignificance. Arterioscler. Thromb. Vasc. Biol. 36, 718–727. doi: 10.1161/atvbaha.115.306748
Monne, H. M.-S., Danne, R., Róg, T., Ilpo, V., and Gurtovenko, A. (2013). Structure of glycocalyx. Biophys. J. 104, 251a.
Moscatello, D. K., Santra, M., Mann, D. M., McQuillan, D. J., Wong, A. J., and Iozzo, R. V. (1998). Decorin suppresses tumor cell growth by activating the epidermal growth factor receptor. J. Clin. Invest. 101, 406–412. doi: 10.1172/JCI846
Mulloy, B., and Rider, C. C. (2006). Cytokines and proteoglycans: an introductory overview. Biochem. Soc. Trans. 34, 409–413. doi: 10.1042/BST0340409
Mytilinaiou, M., Nikitovic, D., Berdiaki, A., Kostouras, A., Papoutsidakis, A., Tsatsakis, A. M., et al. (2017). Emerging roles of syndecan 2 in epithelial and mesenchymal cancer progression. IUBMB Life 69, 824–833. doi: 10.1002/iub.1678
Naso, M. F., Zimmermann, D. R., and Iozzo, R. V. (1994). Characterization of the complete genomic structure of the human versican gene and functional analysis of its promoter. J. Biol. Chem. 269, 32999–33008.
Neill, T., Schaefer, L., and Iozzo, R. V. (2016). Decorin as a multivalent therapeutic agent against cancer. Adv. Drug Deliv. Rev. 97, 174–185. doi: 10.1016/j.addr.2015.10.016
Newick, K., O’Brien, S., Moon, E., and Albelda, S. M. (2017). CAR T cell therapy for solid tumors. Annu. Rev. Med. 68, 139–152. doi: 10.1146/annurev-med-062315-120245
Nia, H. T., Han, L., Bozchalooi, I. S., Roughley, P., Youcef-Toumi, K., Grodzinsky, A. J., et al. (2015). Aggrecan nanoscale solid-fluid interactions are a primary determinant of cartilage dynamic mechanical properties. ACS Nano 9, 2614–2625. doi: 10.1021/nn5062707
Nicolosi, P. A., Dallatomasina, A., and Perris, R. (2015). Theranostic impact of NG2/CSPG4 proteoglycan in cancer. Theranostics 5, 530–544. doi: 10.7150/thno.10824
Nikitovic, D., Berdiaki, A., Spyridaki, I., Krasanakis, T., Tsatsakis, A., and Tzanakakis, G. N. (2018). Proteoglycans—biomarkers and targets in cancer therapy. Front. Endocrinol. 9:69. doi: 10.3389/fendo.2018.00069
Nishida, J., Miyazono, K., and Ehata, S. (2018). Decreased TGFBR3/betaglycan expression enhances the metastatic abilities of renal cell carcinoma cells through TGF-β-dependent and -independent mechanisms. Oncogene 37, 2197–2212. doi: 10.1038/s41388-017-0084-0
Njah, K., Chakraborty, S., Qiu, B., Arumugam, S., Raju, A., Pobbati, A. V., et al. (2019). A role of agrin in maintaining the stability of vascular endothelial growth factor receptor-2 during tumor angiogenesis. Cell Rep. 28, 949.e7–965.e7. doi: 10.1016/j.celrep.2019.06.036
Noguchi, S., Saito, A., and Nagase, T. (2018). YAP/TAZ signaling as a molecular link between fibrosis and cancer. Int. J. Mol. Sci. 19:3674. doi: 10.3390/ijms19113674
Northey, J. J., Przybyla, L., and Weaver, V. M. (2017). Tissue Force programs cell fate and tumor aggression. Cancer Discov. 7, 1224–1237. doi: 10.1158/2159-8290.CD-16-0733
Nugent, M. A. (2000). Heparin sequencing brings structure to the function of complex oligosaccharides. Proc. Natl. Acad. Sci. U. S. A. 97, 10301–10303. doi: 10.1073/pnas.97.19.10301
Okina, E., Grossi, A., Gopal, S., Multhaupt, H. A. B., and Couchman, J. R. (2012). Alpha-actinin interactions with syndecan-4 are integral to fibroblast-matrix adhesion and regulate cytoskeletal architecture. Int. J. Biochem. Cell Biol. 44, 2161–2174. doi: 10.1016/j.biocel.2012.08.017
Ostapoff, K. T., Awasthi, N., Cenik, B. K., Hinz, S., Dredge, K., Schwarz, R. E., et al. (2013). PG545, an angiogenesis and heparanase inhibitor, reduces primary tumor growth and metastasis in experimental pancreatic cancer. Mol. Cancer Ther. 12, 1190–1201. doi: 10.1158/1535-7163.MCT-12-1123
Paszek, M. J., DuFort, C. C., Rossier, O., Bainer, R., Mouw, J. K., Godula, K., et al. (2014). The cancer glycocalyx mechanically primes integrin-mediated growth and survival. Nature 511, 319–325. doi: 10.1038/nature13535
Paszek, M. J., Zahir, N., Johnson, K. R., Lakins, J. N., Rozenberg, G. I., Gefen, A., et al. (2005). Tensional homeostasis and the malignant phenotype. Cancer Cell 8, 241–254. doi: 10.1016/j.ccr.2005.08.010
Peer, D., Karp, J. M., Hong, S., Farokhzad, O. C., Margalit, R., and Langer, R. (2007). Nanocarriers as an emerging platform for cancer therapy. Nat. Nanotechnol. 2, 751–760. doi: 10.1038/nnano.2007.387
Pellegatta, S., Savoldo, B., Di Ianni, N., Corbetta, C., Chen, Y., Patané, M., et al. (2018). Constitutive and TNFα-inducible expression of chondroitin sulfate proteoglycan 4 in glioblastoma and neurospheres: implications for CAR-T cell therapy. Sci. Transl. Med. 10:eaao2731. doi: 10.1126/scitranslmed.aao2731
Perurena, N., Zandueta, C., Martínez-Canarias, S., Moreno, H., Vicent, S., Almeida, A. S., et al. (2017). EPCR promotes breast cancer progression by altering SPOCK1/testican 1-mediated 3D growth. J. Hematol. Oncol. 10:23. doi: 10.1186/s13045-017-0399-x
Pirinen, R., Leinonen, T., Böhm, J., Johansson, R., Ropponen, K., Kumpulainen, E., et al. (2005). Versican in nonsmall cell lung cancer: relation to hyaluronan, clinicopathologic factors, and prognosis. Hum. Pathol. 36, 44–50. doi: 10.1016/j.humpath.2004.10.010
Pogoda, K., Bucki, R., Byfield, F. J., Cruz, K., Lee, T., Marcinkiewicz, C., et al. (2017). Soft substrates containing hyaluronan mimic the effects of increased stiffness on morphology, motility, and proliferation of glioma cells. Biomacromolecules 18, 3040–3051. doi: 10.1021/acs.biomac.7b00324
Polacheck, W. J., Kutys, M. L., Yang, J., Eyckmans, J., Wu, Y., Vasavada, H., et al. (2017). A non-canonical Notch complex regulates adherens junctions and vascular barrier function. Nature 552, 258–262. doi: 10.1038/nature24998
Pomin, V. H., and Mulloy, B. (2018). Glycosaminoglycans and proteoglycans. Pharmaceuticals 11:27. doi: 10.3390/ph11010027
Qazi, H., Palomino, R., Shi, Z.-D., Munn, L. L., and Tarbell, J. M. (2013). Cancer cell glycocalyx mediates mechanotransduction and flow-regulated invasion. Integr. Biol. 5, 1334–1343. doi: 10.1039/c3ib40057c
Qiao, W., Liu, H., Guo, W., Li, P., and Deng, M. (2019). Prognostic and clinical significance of syndecan-1 expression in breast cancer: a systematic review and meta-analysis. Eur. J. Surg. Oncol. 45, 1132–1137. doi: 10.1016/j.ejso.2018.12.019
Ramani, V. C., Purushothaman, A., Stewart, M. D., Thompson, C. A., Vlodavsky, I., Au, J. L.-S., et al. (2013). The heparanase/syndecan-1 axis in cancer: mechanisms and therapies. FEBS J. 280, 2294–2306.
Rankin, K. S., and Frankel, D. (2016). Hyaluronan in cancer - from the naked mole rat to nanoparticle therapy. Soft Matter 12, 3841–3848. doi: 10.1039/c6sm00513f
Rivera, C., Zandonadi, F. S., Sánchez-Romero, C., Soares, C. D., Granato, D. C., González-Arriagada, W. A., et al. (2018). Agrin has a pathological role in the progression of oral cancer. Br. J. Cancer 118, 1628–1638. doi: 10.1038/s41416-018-0135-5
Rizzolio, S., Rabinowicz, N., Rainero, E., Lanzetti, L., Serini, G., Norman, J., et al. (2012). Neuropilin-1-dependent regulation of EGF-receptor signaling. Cancer Res. 72, 5801–5811. doi: 10.1158/0008-5472.can-12-0995
Robinson, K. A., Sun, M., Barnum, C. E., Weiss, S. N., Huegel, J., Shetye, S. S., et al. (2017). Decorin and biglycan are necessary for maintaining collagen fibril structure, fiber realignment, and mechanical properties of mature tendons. Matrix Biol. 64, 81–93. doi: 10.1016/j.matbio.2017.08.004
Rossi, G. D., De Rossi, G., Evans, A. R., Kay, E., Woodfin, A., McKay, T. R., et al. (2014). Shed syndecan-2 inhibits angiogenesis. J. Cell Sci. 127, 4788–4799. doi: 10.1242/jcs.153015
Samuel, M. S., Lopez, J. I., McGhee, E. J., Croft, D. R., Strachan, D., Timpson, P., et al. (2011). Actomyosin-mediated cellular tension drives increased tissue stiffness and β-catenin activation to induce epidermal hyperplasia and tumor growth. Cancer Cell 19, 776–791. doi: 10.1016/j.ccr.2011.05.008
Sanz-Moreno, V., Gaggioli, C., Yeo, M., Albrengues, J., Wallberg, F., Viros, A., et al. (2011). ROCK and JAK1 signaling cooperate to control actomyosin contractility in tumor cells and stroma. Cancer Cell 20, 229–245. doi: 10.1016/j.ccr.2011.06.018
Sarntinoranont, M., Rooney, F., and Ferrari, M. (2003). Interstitial stress and fluid pressure within a growing tumor. Ann. Biomed. Eng. 31, 327–335. doi: 10.1114/1.1554923
Sarrazin, S., Lamanna, W. C., and Esko, J. D. (2011). Heparan sulfate proteoglycans. Cold Spring Harb. Perspect. Biol. 3:a004952.
Sasse, P., Malan, D., Fleischmann, M., Roell, W., Gustafsson, E., Bostani, T., et al. (2008). Perlecan is critical for heart stability. Cardiovasc. Res. 80, 435–444. doi: 10.1093/cvr/cvn225
Sawada, Y., Tamada, M., Dubin-Thaler, B. J., Cherniavskaya, O., Sakai, R., Tanaka, S., et al. (2006). Force sensing by mechanical extension of the Src family kinase substrate p130Cas. Cell 127, 1015–1026. doi: 10.1016/j.cell.2006.09.044
Sayyad, M. R., Puchalapalli, M., Vergara, N. G., Wangensteen, S. M., Moore, M., Mu, L., et al. (2019). Syndecan-1 facilitates breast cancer metastasis to the brain. Breast Cancer Res. Treat. 178, 35–49. doi: 10.1007/s10549-019-05347-0
Schedin, P., and Keely, P. J. (2011). Mammary gland ECM remodeling, stiffness, and mechanosignaling in normal development and tumor progression. Cold Spring Harb. Perspect. Biol. 3:a003228. doi: 10.1101/cshperspect.a003228
Schick, B. P., Gradowski, J. F., and San Antonio, J. D. (2001). Synthesis, secretion, and subcellular localization of serglycin proteoglycan in human endothelial cells. Blood 97, 449–458. doi: 10.1182/blood.v97.2.449
Schnepp, A., Lindgren, P. K., Hulsmann, H., Kroger, S., Paulsson, M., and Hartmann, U. (2005). Mouse testican-2: expression, glycosylation, and effects on neurite outgrowth. J. Biol. Chem. 280, 11274–11280. doi: 10.1074/jbc.m414276200
Shawky, M. S., Ricciardelli, C., Lord, M., Whitelock, J., Ferro, V., Britt, K., et al. (2015). Proteoglycans: potential agents in mammographic density and the associated breast cancer risk. J. Mammary Gland Biol. Neoplasia 20, 121–131. doi: 10.1007/s10911-015-9346-z
Shen, X.-H., Lin, W.-R., Xu, M.-D., Qi, P., Dong, L., Zhang, Q.-Y., et al. (2015). Prognostic significance of versican expression in gastric adenocarcinoma. Oncogenesis 4:e178. doi: 10.1038/oncsis.2015.36
Shi, Z.-D., Wang, H., and Tarbell, J. M. (2011). Heparan sulfate proteoglycans mediate interstitial flow mechanotransduction regulating MMP-13 expression and cell motility via FAK-ERK in 3D collagen. PLoS One 6:e15956. doi: 10.1371/journal.pone.0015956
Shimizu, Y., Suzuki, T., Yoshikawa, T., Endo, I., and Nakatsura, T. (2019). Next-generation cancer immunotherapy targeting glypican-3. Front. Oncol. 9:248. doi: 10.3389/fonc.2019.00248
Shintani, Y., Takashima, S., Asano, Y., Kato, H., Liao, Y., Yamazaki, S., et al. (2006). Glycosaminoglycan modification of neuropilin-1 modulates VEGFR2 signaling. EMBO J. 25, 3045–3055. doi: 10.1038/sj.emboj.7601188
Shriver, Z., Capila, I., Venkataraman, G., and Sasisekharan, R. (2012). Heparin and heparan sulfate: analyzing structure and microheterogeneity. Handb. Exp. Pharmacol. 207, 159–176. doi: 10.1007/978-3-642-23056-1_8
Shurer, C. R., Kuo, J. C.-H., Roberts, L. M., Gandhi, J. G., Colville, M. J., Enoki, T. A., et al. (2019). Physical principles of membrane shape regulation by the glycocalyx. Cell 177, 1757.e27–1770.e27. doi: 10.1016/j.cell.2019.04.017
Silver, D. J., Siebzehnrubl, F. A., Schildts, M. J., Yachnis, A. T., Smith, G. M., Smith, A. A., et al. (2013). Chondroitin sulfate proteoglycans potently inhibit invasion and serve as a central organizer of the brain tumor microenvironment. J. Neurosci. 33, 15603–15617. doi: 10.1523/JNEUROSCI.3004-12.2013
Skandalis, S. S., Karalis, T. T., Chatzopoulos, A., and Karamanos, N. K. (2019). Hyaluronan-CD44 axis orchestrates cancer stem cell functions. Cell. Signal. 63:109377. doi: 10.1016/j.cellsig.2019.109377
Soares da Costa, D., Reis, R. L., and Pashkuleva, I. (2017). Sulfation of glycosaminoglycans and its implications in human health and disorders. Annu. Rev. Biomed. Eng. 19, 1–26. doi: 10.1146/annurev-bioeng-071516-044610
Su, Z., Kishida, S., Tsubota, S., Sakamoto, K., Cao, D., Kiyonari, S., et al. (2017). Neurocan, an extracellular chondroitin sulfate proteoglycan, stimulates neuroblastoma cells to promote malignant phenotypes. Oncotarget 8, 106296–106310. doi: 10.18632/oncotarget.22435
Sulka, B., Lortat-Jacob, H., Terreux, R., Letourneur, F., and Rousselle, P. (2009). Tyrosine dephosphorylation of the syndecan-1 PDZ binding domain regulates syntenin-1 recruitment. J. Biol. Chem. 284, 10659–10671. doi: 10.1074/jbc.M807643200
Sun, D., Mcalmon, K. R., Davies, J. A., Bernfield, M., and Hay, E. D. (1998). Simultaneous loss of expression of syndecan-1 and E-cadherin in the embryonic palate during epithelial-mesenchymal transformation. Int. J. Dev. Biol. 42, 733–736.
Teixeira, F. C. O. B., Kumar, A. V., Katakam, S. K., Cocola, C., Pelucchi, P., Graf, M., et al. (2020). The heparan sulfate sulfotransferases HS2ST1 and HS3ST2 are novel regulators of breast cancer stem-cell properties. Front. Cell Dev. Biol. 8:559554. doi: 10.3389/fcell.2020.559554
Teng, Y. H.-F., Aquino, R. S., and Park, P. W. (2012). Molecular functions of syndecan-1 in disease. Matrix Biol. 31, 3–16. doi: 10.1016/j.matbio.2011.10.001
Theocharis, A. D., and Karamanos, N. K. (2019). Proteoglycans remodeling in cancer: underlying molecular mechanisms. Matrix Biol. 7, 220–259. doi: 10.1016/j.matbio.2017.10.008
Tian, C., Öhlund, D., Rickelt, S., Lidström, T., and Huang, Y. (2020). Cancer cell–derived matrisome proteins promote metastasis in pancreatic ductal adenocarcinoma. Cancer Res. 80, 1461–1474.
Tkachenko, E., Elfenbein, A., Tirziu, D., and Simons, M. (2006). Syndecan-4 clustering induces cell migration in a PDZ-dependent manner. Circ. Res. 98, 1398–1404. doi: 10.1161/01.RES.0000225283.71490.5a
Tse, J. M., Cheng, G., Tyrrell, J. A., Wilcox-Adelman, S. A., Boucher, Y., Jain, R. K., et al. (2012). Mechanical compression drives cancer cells toward invasive phenotype. Proc. Natl. Acad. Sci. U. S. A. 109, 911–916. doi: 10.1073/pnas.1118910109
Tsen, G., Halfter, W., Kröger, S., and Cole, G. J. (1995). Agrin is a heparan sulfate proteoglycan. J. Biol. Chem. 270, 3392–3399. doi: 10.1074/jbc.270.7.3392
Tsoyi, K., Osorio, J. C., Chu, S. G., Fernandez, I. E., De Frias, S. P., Sholl, L., et al. (2019). Lung Adenocarcinoma syndecan-2 potentiates cell invasiveness. Am. J. Respir. Cell Mol. Biol. 60, 659–666. doi: 10.1165/rcmb.2018-0118OC
Vachon, C. M., van Gils, C. H., Sellers, T. A., Ghosh, K., Pruthi, S., Brandt, K. R., et al. (2007). Mammographic density, breast cancer risk and risk prediction. Breast Cancer Res. 9:217. doi: 10.1186/bcr1829
Varga, I., Hutóczki, G., Szemcsák, C. D., Zahuczky, G., Tóth, J., Adamecz, Z., et al. (2012). Brevican, neurocan, tenascin-C and versican are mainly responsible for the invasiveness of low-grade astrocytoma. Pathol. Oncol. Res. 18, 413–420. doi: 10.1007/s12253-011-9461-0
Varki, A., Cummings, R. D., Esko, J. D., Stanley, P., Hart, G. W., and Aebi, M., et al. (eds) (2016). Essentials of Glycobiology. Cold Spring Harbor, NY: Cold Spring Harbor Laboratory.
Vennin, C., Mélénec, P., Rouet, R., Nobis, M., Cazet, A. S., Murphy, K. J., et al. (2019). CAF hierarchy driven by pancreatic cancer cell p53-status creates a pro-metastatic and chemoresistant environment via perlecan. Nat. Commun. 10:3637. doi: 10.1038/s41467-019-10968-6
Vogel, K. G., and Peterson, D. W. (1981). Extracellular, surface, and intracellular proteoglycans produced by human embryo lung fibroblasts in culture (IMR-90). J. Biol. Chem. 256, 13235–13242.
Vuoriluoto, K., Högnäs, G., Meller, P., Lehti, K., and Ivaska, J. (2011). Syndecan-1 and -4 differentially regulate oncogenic K-ras dependent cell invasion into collagen through α2β1 integrin and MT1-MMP. Matrix Biol. 30, 207–217. doi: 10.1016/j.matbio.2011.03.003
Wang, C., Tong, X., and Yang, F. (2014). Bioengineered 3D brain tumor model to elucidate the effects of matrix stiffness on glioblastoma cell behavior using PEG-based hydrogels. Mol. Pharm. 11, 2115–2125. doi: 10.1021/mp5000828
Wang, S., Zhang, X., Wang, G., Cao, B., Yang, H., Jin, L., et al. (2019). Syndecan-1 suppresses cell growth and migration via blocking JAK1/STAT3 and Ras/Raf/MEK/ERK pathways in human colorectal carcinoma cells. BMC Cancer 19:1160. doi: 10.1186/s12885-019-6381-y
Wang, X., He, J., Zhao, X., Qi, T., Zhang, T., and Kong, C. (2018). Syndecan-1 suppresses epithelial-mesenchymal transition and migration in human oral cancer cells. Oncol. Rep. 39, 1835–1842. doi: 10.3892/or.2018.6271
Wei, S. C., Fattet, L., Tsai, J. H., Guo, Y., Pai, V. H., Majeski, H. E., et al. (2015). Matrix stiffness drives epithelial–mesenchymal transition and tumour metastasis through a TWIST1–G3BP2 mechanotransduction pathway. Nat. Cell Biol. 17, 678–688. doi: 10.1038/ncb3157
West, D. C., Rees, C. G., Duchesne, L., Patey, S. J., Terry, C. J., Turnbull, J. E., et al. (2005). Interactions of multiple heparin binding growth factors with neuropilin-1 and potentiation of the activity of fibroblast growth factor-2. J. Biol. Chem. 280, 13457–13464. doi: 10.1074/jbc.m410924200
Whitelock, J. M., Graham, L. D., Melrose, J., Murdoch, A. D., Iozzo, R. V., and Underwood, P. A. (1999). Human perlecan immunopurified from different endothelial cell sources has different adhesive properties for vascular cells. Matrix Biol. 18, 163–178. doi: 10.1016/s0945-053x(99)00014-1
Whitelock, J. M., Melrose, J., and Iozzo, R. V. (2008). Diverse cell signaling events modulated by perlecan. Biochemistry 47, 11174–11183. doi: 10.1021/bi8013938
Wight, T. N., Kang, I., and Merrilees, M. J. (2014). Versican and the control of inflammation. Matrix Biol. 35, 152–161. doi: 10.1016/j.matbio.2014.01.015
Wight, T. N., Toole, B. P., and Hascall, V. C. (2011). “Hyaluronan and the aggregating proteoglycans,” in The Extracellular Matrix: an Overview. Biology of Extracellular Matrix, ed. R. Mecham (Berlin: Springer), 147–195. doi: 10.1007/978-3-642-16555-9_5
Winzen, U., Cole, G. J., and Halfter, W. (2003). Agrin is a chimeric proteoglycan with the attachment sites for heparan sulfate/chondroitin sulfate located in two multiple serine-glycine clusters. J. Biol. Chem. 278, 30106–30114. doi: 10.1074/jbc.m212676200
Wolf, K. J., Chen, J., Coombes, J. D., Aghi, M. K., and Kumar, S. (2019). Dissecting and rebuilding the glioblastoma microenvironment with engineered materials. Nat. Rev. Mater. 4, 651–668. doi: 10.1038/s41578-019-0135-y
Woods, A., and Couchman, J. R. (2001). Syndecan-4 and focal adhesion function. Curr. Opin. Cell Biol. 13, 578–583. doi: 10.1016/s0955-0674(00)00254-4
Woods, E. C., Kai, F., Barnes, J. M., Pedram, K., Pickup, M. W., Hollander, M. J., et al. (2017). A bulky glycocalyx fosters metastasis formation by promoting G1 cell cycle progression. Elife 6:e25752. doi: 10.7554/eLife.25752
Xiang, Y. Y., Ladeda, V., and Filmus, J. (2001). Glypican-3 expression is silenced in human breast cancer. Oncogene 20, 7408–7412. doi: 10.1038/sj.onc.1204925
Xu, Y., Xu, J., Yang, Y., Zhu, L., Li, X., and Zhao, W. (2018). SRGN promotes colorectal cancer metastasis as a critical downstream target of HIF-1α. Cell. Physiol. Biochem. 48, 2429–2440. doi: 10.1159/000492657
Yamada, H., Watanabe, K., Shimonaka, M., and Yamaguchi, Y. (1994). Molecular cloning of brevican, a novel brain proteoglycan of the aggrecan/versican family. J. Biol. Chem. 269, 10119–10126.
Yanagishita, M. (1993). Function of proteoglycans in the extracellular matrix. Pathol. Int. 43, 283–293. doi: 10.1111/j.1440-1827.1993.tb02569.x
Yang, J., Yang, Q., Yu, J., Li, X., Yu, S., and Zhang, X. (2016). SPOCK1 promotes the proliferation, migration and invasion of glioma cells through PI3K/AKT and Wnt/β-catenin signaling pathways. Oncol. Rep. 35, 3566–3576. doi: 10.3892/or.2016.4757
Yang, N., Mosher, R., Seo, S., Beebe, D., and Friedl, A. (2011). Syndecan-1 in breast cancer stroma fibroblasts regulates extracellular matrix fiber organization and carcinoma cell motility. Am. J. Pathol. 178, 325–335. doi: 10.1016/j.ajpath.2010.11.039
Zeng, Y., Waters, M., Andrews, A., Honarmandi, P., Ebong, E. E., Rizzo, V., et al. (2013). Fluid shear stress induces the clustering of heparan sulfate via mobility of glypican-1 in lipid rafts. Am. J. Physiol. Heart Circ. Physiol. 305, H811–H820. doi: 10.1152/ajpheart.00764.2012
Zhang, G., Ezura, Y., Chervoneva, I., Robinson, P. S., Beason, D. P., Carine, E. T., et al. (2006). Decorin regulates assembly of collagen fibrils and acquisition of biomechanical properties during tendon development. J. Cell. Biochem. 98, 1436–1449. doi: 10.1002/jcb.20776
Zhang, K., Grither, W. R., Van Hove, S., Biswas, H., Ponik, S. M., Eliceiri, K. W., et al. (2016). Mechanical signals regulate and activate SNAIL1 protein to control the fibrogenic response of cancer-associated fibroblasts. J. Cell Sci. 129, 1989–2002. doi: 10.1242/jcs.180539
Zhang, Z., Qiu, N., Yin, J., Zhang, J., Liu, H., Guo, W., et al. (2020). SRGN crosstalks with YAP to maintain chemoresistance and stemness in breast cancer cells by modulating HDAC2 expression. Theranostics 10, 4290–4307. doi: 10.7150/thno.41008
Zhao, P., Guan, H.-T., Dai, Z.-J., Ma, Y.-G., Liu, X.-X., and Wang, X.-J. (2016). Knockdown of SPOCK1 inhibits the proliferation and invasion in colorectal cancer cells by suppressing the PI3K/Akt pathway. Oncol. Res. 24, 437–445. doi: 10.3727/096504016X14685034103554
Zhong, X., Liu, Y., Liu, H., Zhang, Y., Wang, L., and Zhang, H. (2018). Identification of potential prognostic genes for neuroblastoma. Front. Genet. 9:589. doi: 10.3389/fgene.2018.00589
Zimmermann, D. R., and Dours-Zimmermann, M. T. (2008). Extracellular matrix of the central nervous system: from neglect to challenge. Histochem. Cell Biol. 130, 635–653. doi: 10.1007/s00418-008-0485-9
Zimmermann, D. R., and Ruoslahti, E. (1989). Multiple domains of the large fibroblast proteoglycan, versican. EMBO J. 8, 2975–2981. doi: 10.1002/j.1460-2075.1989.tb08447.x
Zoeller, J. J., McQuillan, A., Whitelock, J., Ho, S.-Y., and Iozzo, R. V. (2008). A central function for perlecan in skeletal muscle and cardiovascular development. J. Cell Biol. 181, 381–394. doi: 10.1083/jcb.200708022
Keywords: proteoglycans, GAG, cancer, mechanosignaling, glycocalyx
Citation: Barkovskaya A, Buffone A Jr, Žídek M and Weaver VM (2020) Proteoglycans as Mediators of Cancer Tissue Mechanics. Front. Cell Dev. Biol. 8:569377. doi: 10.3389/fcell.2020.569377
Received: 03 June 2020; Accepted: 04 November 2020;
Published: 30 November 2020.
Edited by:
Rachel K. Okolicsanyi, Queensland University of Technology, AustraliaReviewed by:
Rizaldy Paz Scott, Northwestern University, United StatesCopyright © 2020 Barkovskaya, Buffone, Žídek and Weaver. This is an open-access article distributed under the terms of the Creative Commons Attribution License (CC BY). The use, distribution or reproduction in other forums is permitted, provided the original author(s) and the copyright owner(s) are credited and that the original publication in this journal is cited, in accordance with accepted academic practice. No use, distribution or reproduction is permitted which does not comply with these terms.
*Correspondence: Valerie M. Weaver, dmFsZXJpZS53ZWF2ZXJAdWNzZi5lZHU=
Disclaimer: All claims expressed in this article are solely those of the authors and do not necessarily represent those of their affiliated organizations, or those of the publisher, the editors and the reviewers. Any product that may be evaluated in this article or claim that may be made by its manufacturer is not guaranteed or endorsed by the publisher.
Research integrity at Frontiers
Learn more about the work of our research integrity team to safeguard the quality of each article we publish.