- 1Department of Molecular Medicine, Faculty of Pharmacy and Medicine, Sapienza University of Rome, Rome, Italy
- 2Department of Medical and Surgical Sciences and Biotechnology, Faculty of Pharmacy and Medicine, Sapienza University of Rome, Rome, Italy
- 3Mediterranea Cardiocentro, Naples, Italy
- 4Department of Maternal, Infantile, and Urological Sciences, “Umberto I” Hospital, Rome, Italy
- 5Department of Bioengineering, Sanford Consortium for Regenerative Medicine, University of California, San Diego, San Diego, CA, United States
The increased knowledge in cell signals and stem cell differentiation, together with the development of new technologies, such as 3D bioprinting, has made the generation of artificial tissues more feasible for in vitro studies and in vivo applications. In the human body, cell fate, function, and survival are determined by the microenvironment, a rich and complex network composed of extracellular matrix (ECM), different cell types, and soluble factors. They all interconnect and communicate, receiving and sending signals, modulating and responding to cues. In the cardiovascular field, the culture of stem cells in vitro and their differentiation into cardiac phenotypes is well established, although differentiated cardiomyocytes often lack the functional maturation and structural organization typical of the adult myocardium. The recreation of an artificial microenvironment as similar as possible to the native tissue, though, has been shown to partly overcome these limitations, and can be obtained through the proper combination of ECM molecules, different cell types, bioavailability of growth factors (GFs), as well as appropriate mechanical and geometrical stimuli. This review will focus on the role of the ECM in the regulation of cardiac differentiation, will provide new insights on the role of supporting cells in the generation of 3D artificial tissues, and will also present a selection of the latest approaches to recreate a cardiac microenvironment in vitro through 3D bioprinting approaches.
Introduction
The regulation of cell proliferation, differentiation, and function is influenced by several factors, such as signaling molecules, cell-cell interaction, and the extracellular matrix (ECM), which are collectively defined as the microenvironment. Among their many functions in homeostasis, these factors play a fundamental role also in tissue development, turnover, and repair. The regulation of stem cell behavior is a particularly delicate task: in fact, embryonic stem cells (ESCs) are the main players during embryogenesis, and are tightly regulated and coordinated to maintain their stemness, as well as their activation and functional differentiation. Moreover, the increasing use of induced pluripotent stem cells (iPSCs) to obtain differentiated cell types requires particular attention also on the signals regulating their biology. Indeed, this very specific microenvironment is now commonly defined as the stem cell “niche,” and its understanding and modeling could boost advancements in the field of tissue engineering, and even subsequent in vivo therapeutic applications (Chimenti et al., 2017).
The most studied cell regulatory signals are mediated by growth factors (GFs) and soluble cues, widely used in ex vivo research to differentiate adult and ESCs into mature cells. The role of molecules such as insulin-like and fibroblast GFs (Bendall et al., 2007), Wnt proteins (Reya et al., 2003; Lie et al., 2005), and many other cytokines (Endele et al., 2014) have been extensively studied, while the role of the ECM in the regulation of the microenvironment and the niche still remains to be fully understood. The ECM is not an inert, structural scaffold, as once thought: it maintains indeed the structure and function of organs, participating in their development and remodeling. ECM allows cell-cell communication and it modulates cell motility, adhesion, survival, and proliferation (Miyamoto et al., 1996; Ross and Borg, 2001). The ECM is composed of structural proteins, such as collagens, and numerous non-structural regulatory proteins, such as proteoglycans (PGs). Within the niche, different ECM molecules can regulate stem cell behavior by direct interactions with integrin receptors expressed on the cellular membrane, by modulating tissue compliance and consequent cell response through the mechanosensing machinery, or through the binding of different GFs and their controlled spatiotemporal presentation to the neighboring cells.
In the heart, ECM proteins surround and protect all cell types: cardiomyocytes, fibroblasts, endothelial cells, pericytes, and smooth muscle cells. All together, proteins, cells, and their cross-talk, create an interconnected microenvironment, which promptly reacts to external changes and cues such as biochemical (GFs, cytokines, hormones), electrical and mechanical signals (such as changes in contraction, stretch, and pressure), necessary to maintain myocardial integrity and functional responsiveness, especially during development and physiological post-natal remodeling (Howard and Baudino, 2013). Cardiac ECM, in fact, is a dynamic structure, constantly undergoing synthesis and degradation, modifying its composition in space and time in response to contingencies, from early development to post-natal life, aging, and possibly diseased states (Schenke-Layland et al., 2012; Rienks et al., 2014).
In this review, we will focus on the role of the ECM in the regulation of the cardiac microenvironment, starting briefly from tissue development during embryogenesis, to the role of different ECM molecules in cardiac differentiation and proliferation, both in vitro and in vivo. Moreover, we will also address the role of supporting cells and describe the most promising approaches to recreate in vitro a physiologically relevant cardiac microenvironment through 3D bioprinting technology.
The Cardiac Extracellular Matrix
During embryogenesis, the heart is the first functional organ to develop, thanks to the proliferation, migration, and differentiation of cardiac precursor cells (CPCs). These cells migrate from the primitive streak in the embryo, organizing themselves into the mesoderm (Abu-issa and Kirby, 2007), from which the CPCs derive; then they differentiate into all the major cell types of the heart, including cardiomyocytes (CMs), smooth muscle cells, endothelial cells, and fibroblasts (Yustzey and Kirby, 2002; Kelly et al., 2014; Witman and Sahara, 2018). The primitive cardiac tube has an outer layer of myocardium and an inner one of endothelium, divided by an acellular space filled with a provisional ECM, also known as the cardiac jelly, which is essential for the maintenance of the tube structure during its transformation into the definite heart form (Huang et al., 1995). This matrix is enriched in hyaluronic acid (HA) and PGs, which render it highly hydrated and malleable (Bernanke and Markwald, 1984; Funderburg and Markwald, 1986). Cardiac jelly components sequester GFs and influence CPC movement and differentiation, and are extremely important during epithelial-to-mesenchymal transition (EMT), which is necessary for many ontological steps to generate adult tissues and organs (Butcher and Markwald, 2007; Thiery et al., 2009; Votteler et al., 2010). Cardiac jelly also contains fibronectin, laminin, different types of collagen, fibulin, and fibrillin, that are fundamental in orchestrating tissue growth, organization, and differentiation (Icardo and Manasek, 1983; Mjaatvedt et al., 1987; Little et al., 1989; Bouchey et al., 1996).
After birth, the heart undergoes remodeling to adapt to the mechanical, chemical, and electrical changes of the growing body. These alterations in organ geometry are accompanied by changes in ECM composition, and are necessary to maintain efficient cardiac function (Baudino et al., 2006). Many ECM components are involved. Collagen I is the most abundant in both developing and adult tissue. Still, in the latter, its density and crosslinking are higher, and a decrease in the ratio between collagen I and collagen III is observed (Thompson et al., 1979; Borg, 1982; Hanson et al., 2013; González et al., 2019). Fibronectin (FN), instead, decreases in the adult tissue (Kim et al., 1999; Lockhart et al., 2011) with a concomitant increase of elastin (Kim et al., 1999). These changes are generally associated with an increase in cardiac stiffness, with the heart tissue becoming less compliant during heart development and CM differentiation, as demonstrated by the threefold increase of left ventricle stiffness 5 days following birth, up to an elastic modulus of approximately 10–20 kPa (Jacot et al., 2008; Horn, 2015).
Extracellular matrix proteins not only act as anchored ligands: they are also reservoirs for GFs, cytokines, and proteases, in particular matrix metalloproteinases (MMPs) and tissue inhibitor of metalloproteinases (TIMPS) (Hynes, 2009; Brizzi et al., 2012). MMPs degrade collagen (Daley et al., 2008), while simultaneously releasing small bioactive peptides and GFs stored within the matrix, releasing soluble ligands that participate in the homeostasis of the microenvironment. Indeed, FN, vitronectin, collagens, and PGs, avidly bind many GFs, such as FGFs and VEGFs, regulating their presentation, distribution, and activation (Brizzi et al., 2012; Nakayama et al., 2014). Likewise, collagen IV binds BMP4 (Wang et al., 2008).
Role of the ECM in Cardiac Differentiation
As mentioned earlier, cells and ECM are directly connected, and the matrix can influence their growth, organization, and differentiation; similarly, the microenvironment regulates stem cell proliferation, hence the maintenance of potency or differentiation into a specific cell lineage (Figure 1).
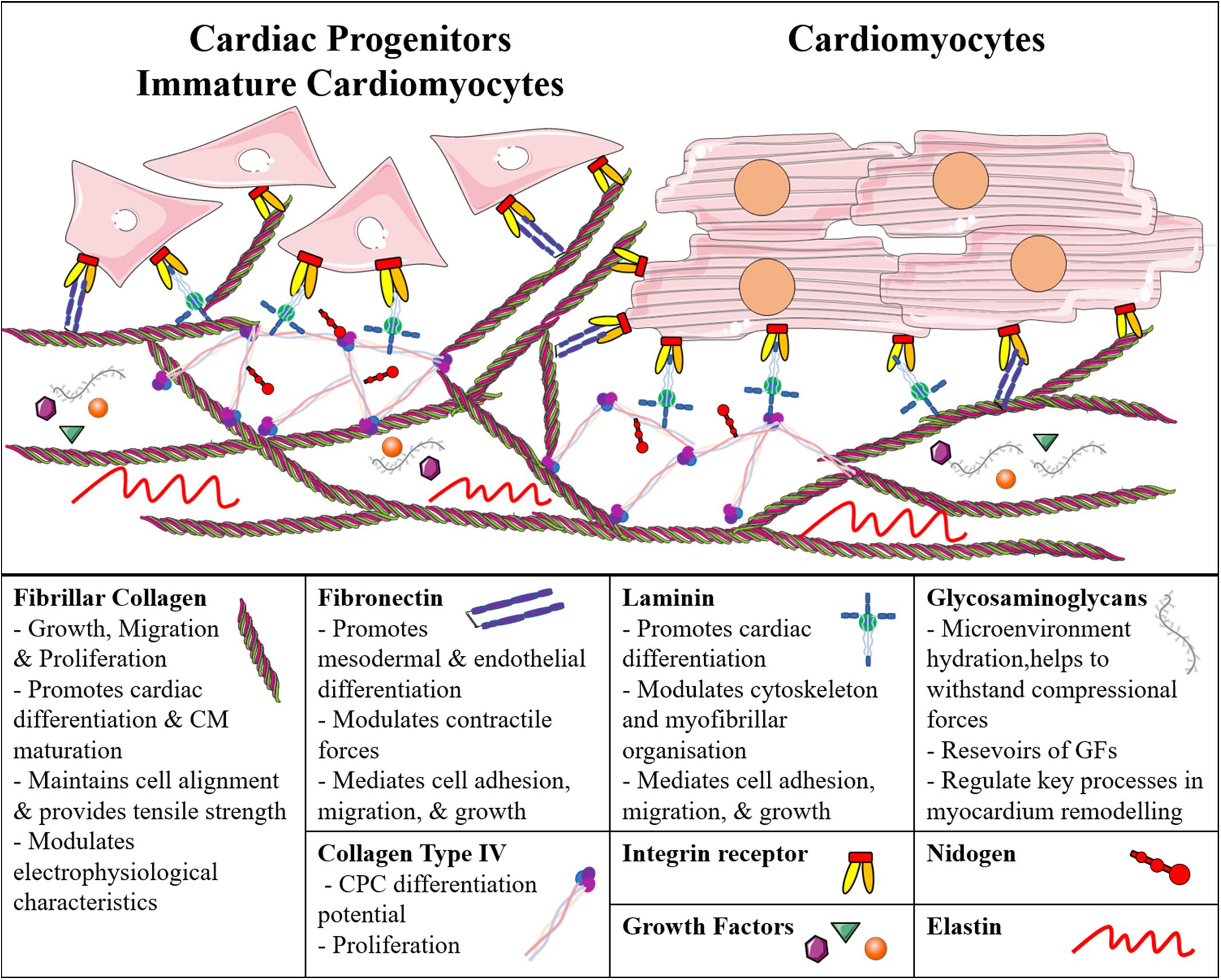
Figure 1. Schematic representation of the main cardiac extracellular matrix components and their direct interaction with immature and adult cardiomyocytes. CM, Cardiomyocyte; CPC, Cardiac Progenitor Cell; GF, Growth Factor.
Cells adhere to ECM proteins through integrin receptors, which activate intracellular signal transductions and are known to regulate many developmentally relevant processes (Wozniak et al., 2004; Saleem et al., 2009; Sa et al., 2014; Neiman et al., 2019). For example, the interaction between collagen type I and β1-integrin was studied in murine iPSC-derived embryoid bodies, and was shown to be important for cell growth and cardiac lineage commitment (Zeng et al., 2013). Collagen type I has been reported to support the maturation of murine (m) and human (h)iPSC-derived CMs, and to modulate intracellular calcium homeostasis and their electrophysiological properties (Lu et al., 2011; Edalat et al., 2019), while collagen type IV can increase the differentiation potential of m/hCPCs (Schenke-Layland et al., 2012). Both of them promote proliferation of CPCs and maintain the pluripotency of mESCs in vitro (Lu et al., 2017). It is worth mentioning that even with the same biochemical compositions (e.g., molecular collagen), different microstructures (e.g., fibrous versus porous) can nonetheless differentially affect the phenotype of cardiac cell types (Chimenti et al., 2011).
Similarly, Cheng et al. (2013) described that FN promotes mesodermal differentiation via β1-integrin through the activation of the Wnt/β-catenin pathway in mESCs. Laminin (LM), instead, has been described to support ESC-derived CPC differentiation, and modulate the cytoskeleton and myofibrillar organization (Battista et al., 2005; Sa et al., 2014). Similar to single ECM components, culturing ESC-derived CPCs on matrices with different combinations of these ECM proteins has shown efficient cell attachment, cardiac differentiation, and survival (Battista et al., 2005; Jung et al., 2015; Lu et al., 2017). Sa et al. (2014) have investigated the effects of various ratios of FN and LN, and found that human ESCs differentiating on 70:30 FN:LN yielded CMs with higher efficiency compared to simple gelatin coatings. Several commercial ECM-based products are available for in vitro studies on substrates with multiple components. MatrigelTM, a basement membrane-derived matrix, promotes EMT, generating precardiac mesoderm, and can promote CM differentiation (Zhang et al., 2012). Similarly, CardiogelTM, a mixture of collagen types I and III, LM, FN, and PGs derived from cardiac fibroblasts, leads to better adherence and maturation of CMs (Vanwinkle et al., 1996). Therefore, despite many studies have shown the importance of different ECM molecules in cardiac cell differentiation and functionality, in order to recreate the proper microenvironment in vitro, it is essential to provide the cells with all the ECM molecules present in the native tissue. For this reason, tissue-derived biomaterials are increasingly being used to culture both progenitor and differentiated CMs. Different decellularization and recellularization strategies of cardiac tissue or whole heart have been developed, using myocardium originating from rats (Ott et al., 2008; Carvalho et al., 2012; Wang et al., 2019), porcines (Singelyn and Christman, 2010; Ferng et al., 2017; Lee et al., 2017), bovines (Arslan et al., 2018), and even humans (Johnson et al., 2014; Sánchez et al., 2015; Guyette et al., 2016; Masumoto et al., 2016; Tang-Quan et al., 2018). Interestingly, cardiac progenitor stromal cells, isolated from newborn mice or human fetal heart, can increase their proliferative capacity and expression of key cardiac transcription factors when cultured in a cardiac-derived ECM hydrogel compared to collagen only, both in 2D (French et al., 2012) and 3D (Gaetani et al., 2016). Decellularized mouse hearts allow hiPSC-derived CPCs to migrate, proliferate, and differentiate into all cardiac cell types, exhibiting efficient spontaneous beating (Lu et al., 2013). Furthermore, human decellularized cardiac tissue has been shown to support miPSC and mESC viability and differentiation into CMs (Oberwallner et al., 2015). Other authors have exploited slices of decellularized human hearts; for example, Garreta et al. (2016) found that the electrical and mechanical functionality of hiPSC-derived CMs increased when grown on such slices, compared to Matrigel.
Besides biochemical signals, the ECM regulates cell behavior also by modulating the mechanical properties of the microenvironment. Cells respond to mechanical cues by altering their cytoskeletal organization, myofibril striation and alignment, by expressing specific combinations of cell adhesion molecules (CAMs), and activating signaling pathways and mechanosensitive ion channels (Ireland and Simmons, 2015; Tallawi et al., 2015). In fact, CMs differentiate better on substrates of stiffness similar to the native tissue (∼10 kPa) (Engler et al., 2008; Jacot et al., 2008; Bhana et al., 2010; Young and Engler, 2011; Tallawi et al., 2015). Indeed, when the substrate is too rigid or too soft, CMs overstrain themselves or do inefficient work, respectively; in both cases it results in an impaired contractile capacity.
New Approaches in Modeling the Artificial Microenvironment
As mentioned previously, the regulation of the microenvironment depends on multiple factors such as cell-cell and cell-ECM interaction, as well as GFs, cytokines, and small molecule signaling, that overall modulate cellular phenotypes. Under these premises, many groups have developed novel methods to grow cardiac progenitor and differentiated cells, creating new microenvironments through 3D culture systems, bioprinting, cardiac patches, and organoid chambers.
Among these approaches, 3D bioprinting can generate tissues using different cells or scaffolds simultaneously, thus having the potential to recreate in vitro, at least in part, the complex microenvironment of any given tissue through the accurate design of the biochemical properties and structure of biomaterials, together with the proper cellular composition. In the last years, many synthetic and biological materials have been used to make bioinks. Cardiac-derived primitive stromal cells, CPCs, and iPSC-derived CMs have been printed in many types of biomaterials, including alginate, HA, and gelatin, among others, and have been used for regenerative medicine and tissue modeling applications (Gaetani et al., 2012, 2015). As mentioned above, several studies have shown how using a single ECM component fails to recapitulate the complexity of the natural cardiac ECM, and thus cannot efficiently reproduce the innate cellular microenvironment (Gardin et al., 2020). Protocols for decellularized extracellular matrix (dECM) have been recently adapted to be used in conjunction with printing technology. They could represent a very promising scaffold for 3D cell culture, as they retain the complex biochemical composition of the native tissue. In this regard, Pati and Cho (2017) have designed a method to prepare dECM from porcine hearts as a bioink, and have used it to bioprint an MSC-laden construct by extrusion-based 3D printing technology. The resulting dECM had highly preserved levels of collagen and glycosaminoglycans, providing a native-like environment for the maintenance of a 3D structured tissue (Pati and Cho, 2017). The importance of cardiac ECM as a component of bioinks has been also highlighted by Jang et al. (2017), who used cardiac porcine dECM complexed with UV-treated vitamin B2 to prepare cardiac-specific hydrogels. These scaffolds were used in combination with human infant CPCs to fabricate 3D bioprinted cardiac constructs with a stiffness similar to the native myocardium, which enhanced viability, proliferation, and cardiac differentiation of CPCs (Jang et al., 2017). Similarly, Bejleri et al. (2018) have developed a 3D bioprinted patch containing heart dECM for the delivery of human pediatric CPCs. The authors demonstrated that these dECM patches improved cardiogenic differentiation and angiogenic potential when compared to cells grown in gelatin-methacryloyl patches, highlighting the importance of the proper ECM composition in the microenvironment for physiologic cell behavior (Bejleri et al., 2018). Similarly, Yu et al. (2019) described a method to make cardiac dECM bioinks to fabricate patient-specific tissues, with high control over micro-architecture and mechanical properties using a digital light processing (DLP)-based 3D bioprinter. The results showed that dECM bioinks provide a suitable microenvironment to support maturation of hiPSC-derived CMs (Yu et al., 2019).
As mentioned previously, the microenvironment is also modulated by cellular diversity, including supporting cells, such as stromal primitive cells, pericytes, or fibroblasts (Figure 2; Castaldo and Chimenti, 2018; Forte et al., 2018). Several studies in 3D culture systems have shown the beneficial effects of fibroblasts co-culture on ESC- or iPSC-derived CM differentiation (Liau et al., 2011; Ou et al., 2011; Valls-Margarit et al., 2019). These beneficial effects are probably due to the secretion of GFs and deposition of new ECM, which in turn may increase the compaction of the artificial tissues, resulting in better cell-to-cell contact. Furthermore, these systems most likely allow more complex tissue mechanical properties, thereby enabling adequate mechanosensing signaling, which is a fundamental player for tissue maturation (Pesce et al., 2017). Interestingly, Zhang et al. (2019) recently showed that co-culture of hiPSC-cardiac fibroblasts with hiPSC-CMs is beneficial for the electrophysiological properties of CMs compared to co-culture with dermal fibroblasts. The study highlighted the importance of tissue-specificity for boundary conditions in the microenvironment, and could be used not only to improve the maturation of cultured cells, but also to study potential specific mechanosensing signaling mediated by tissue specific cells (Zhang et al., 2019). Besides tissue-specificity, it is also important to consider phenotypic changes due to pathological conditions that may alter the behavior of resident fibroblasts and other stromal cells, and consequently the balance between cardiogenic and fibrotic signaling inside the microenvironment (Pagano et al., 2017). Similar to fibroblasts, also endothelial and smooth muscle cells have been shown to increase the structural or electrical maturation of hiPSC-derived CMs (Giacomelli et al., 2017; Pasquier et al., 2017; Vuorenpää et al., 2017). In fact, hiPSC-derived smooth muscle cells and pericytes could increase the structural maturation of iPSC-derived CMs in a 3D collagen I-matrigel scaffold (Masumoto et al., 2016). Recently, the group has reported a new personalized strategy to engineer immuno-compatible patient-specific cardiac patches. In detail, a biopsy of adipose tissue was isolated from patients, and the cellular and acellular components were separated. While the cells were reprogrammed to become pluripotent stem cells, the ECM was processed into a personalized hydrogel able to support hiPSC differentiation into cardiac cells in vitro (Edri et al., 2019). Afterward, the same groups made a more complex 3D model adding endothelial cells as a source of vascular structures, thus obtaining patient-specific vascularized cardiac patches (Noor et al., 2019).
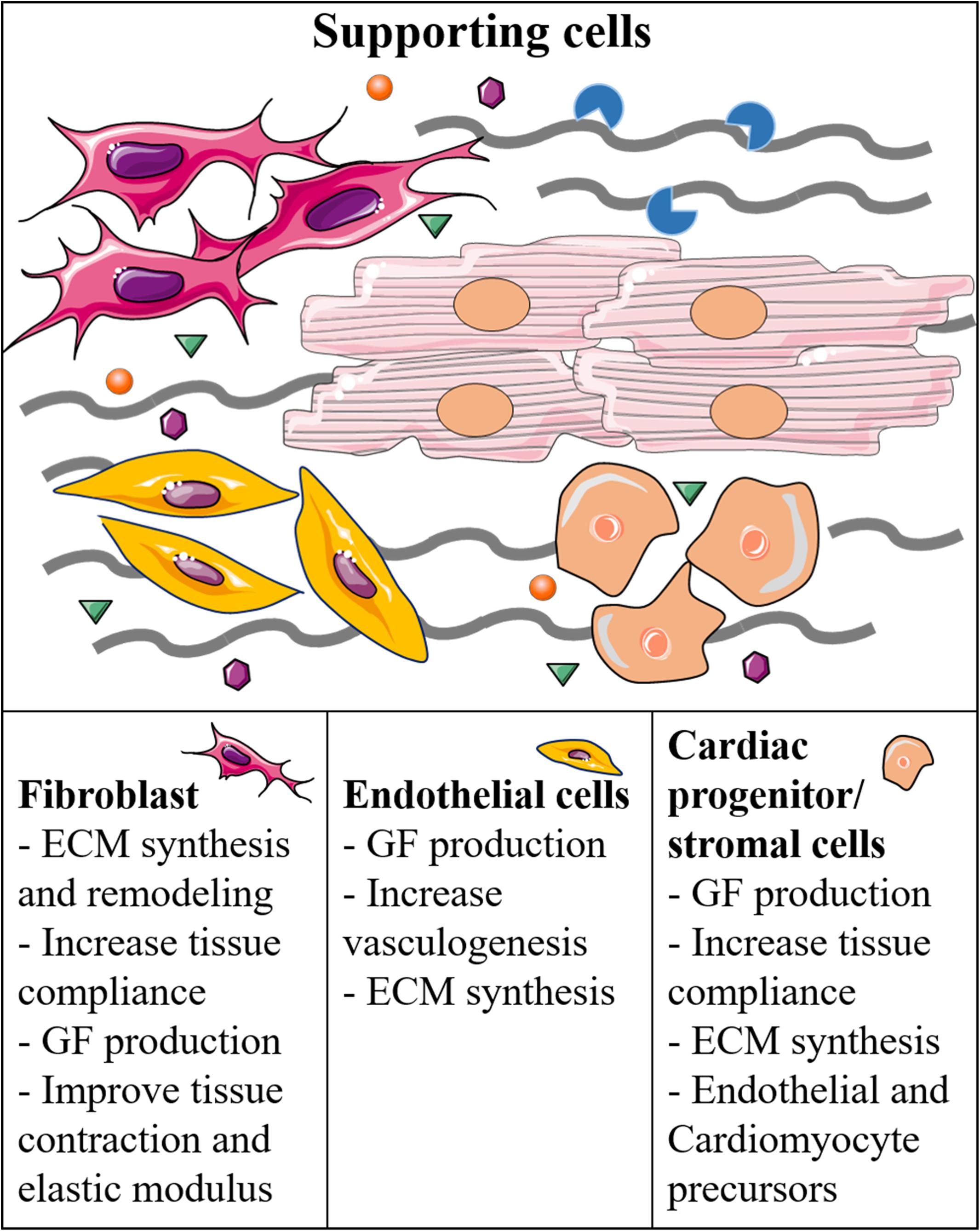
Figure 2. Supporting cells and their function in the regulation of the cardiac microenvironment and generation of cardiac-like tissues.
Other examples to generate highly complex structures include the work of Li et al. (2018) that have created for the first time a human ventricle-like cardiac organoid chamber (hvCOC) to generate advanced models resembling cardiac geometry. HiPSC-derived CMs and dermal fibroblasts have been embedded in a collagen-based ECM, and transferred to an agarose mold with a centrally placed inflatable balloon. This allowed to form the hollow 3D hvCOC that enhanced the maturation of ventricular CMs, providing a unique microenvironment that could mimic fluid pumping, similar to a mature heart chamber (Li et al., 2018). Similarly, Macqueen’s team created a tissue-engineered scale model of the human left ventricle with rat ventricle myocytes or hiPSC-derived CMs seeded on a FN-coated scaffold. The scaffold was made by pull spinning a mixture of PCL and gelatin onto a mandrel in the shape of an ellipsoidal ventricle to promote native-like anisotropic myocardial tissue genesis and chamber-level contractile function (Macqueen et al., 2018).
Conclusion
Reconstructing and mimicking the complexity of native tissues is a challenging objective of contemporary research. Biochemical composition, intercellular communication, co-culture of multiple cell types, and mechanosensing represent key features, among many others, that need to be finely tuned in order to create physiologically relevant microenvironments for cell integration and differentiation. All these features can significantly affect the desired yield and the expected phenotype of artificial tissues and constructs. Increasing knowledge of the basic mechanisms regulating tissue homeostasis, together with advancements in biomaterial preparation and bioprinting, are gradually allowing the design and development of artificial microenvironments with in creasing complexity. This progress will provide innovative tools for ex vivo research, as well as new strategies for tissue repair in regenerative medicine.
Author Contributions
RG, OP, and VP contributed to the text and figures. EM and IC contributed to the text and figure editing. All authors contributed to the article and approved the submitted version.
Funding
This project has received funding from the Euratom Research and Training Programme 2014–2018 under grant agreement no. 755523 and by grant #RG11916B85CDBF76 from Sapienza University of Rome to IC.
Conflict of Interest
The authors declare that the research was conducted in the absence of any commercial or financial relationships that could be construed as a potential conflict of interest.
References
Abu-issa, R., and Kirby, M. L. (2007). Heart field: from mesoderm to heart tube. Annu. Rev. Cell Dev. Biol. 23, 45–68. doi: 10.1146/annurev.cellbio.23.090506.123331
Arslan, Y. E., Galata, Y. F., Sezgin Arslan, T., and Derkus, B. (2018). Trans-differentiation of human adipose-derived mesenchymal stem cells into cardiomyocyte-like cells on decellularized bovine myocardial extracellular matrix-based films. J. Mater. Sci. Mater. Med. 29:127. doi: 10.1007/s10856-018-6135-4
Battista, S., Guarnieri, D., Borselli, C., Zeppetelli, S., Borzacchiello, A., Mayol, L., et al. (2005). The effect of matrix composition of 3D constructs on embryonic stem cell differentiation. Biomaterials 26, 6194–6207. doi: 10.1016/j.biomaterials.2005.04.003
Baudino, T. A., Carver, W., Giles, W., Borg, T. K., Troy, A., Carver, W., et al. (2006). Cardiac fibroblasts: friend or foe? Am. J. Physiol. Heart Circ. Physiol. 291, H1015–H1026. doi: 10.1152/ajpheart.00023.2006
Bejleri, D., Streeter, B. W., Nachlas, A. L. Y., Brown, M. E., Gaetani, R., Christman, K. L., et al. (2018). Bioprinted cardiac patch composed of cardiac-specific extracellular matrix and progenitor cells for heart repair. Adv. Healthc. Mater. 7:1800672. doi: 10.1002/adhm.201800672
Bendall, S. C., Stewart, M. H., Menendez, P., George, D., Vijayaragavan, K., Werbowetski-Ogilvie, T., et al. (2007). IGF and FGF cooperatively establish the regulatory stem cell niche of pluripotent human cells in vitro. Nature 448, 1015–1021. doi: 10.1038/nature06027
Bernanke, D. H., and Markwald, R. R. (1984). Effects of two glycosaminoglycans on seeding of cardiac cushion tissue cells into a collagen-lattice culture system. Anat. Rec. 210, 25–31. doi: 10.1002/ar.1092100105
Bhana, B., Iyer, R. K., Chen, W. L. K., Zhao, R., Sider, K. L., Likhitpanichkul, M., et al. (2010). Influence of substrate stiffness on the phenotype of heart cells. Biotechnol. Bioeng. 105, 1148–1160. doi: 10.1002/bit.22647
Borg, T. K. (1982). Development of the connective tissue network in the neonatal hamster heart. Am. J. Anat. 443, 435–443. doi: 10.1002/aja.1001650407
Bouchey, D., Argraves, W. S., and Little, C. D. (1996). Fibulin-1, vitronectin, and fibronectin expression during avian cardiac valve and septa development. Anat. Rec. 551, 540–551. doi: 10.1002/(sici)1097-0185(199604)244:4<540::aid-ar12>3.0.co;2-p
Brizzi, M. F., Tarone, G., and Defilippi, P. (2012). Extracellular matrix, integrins, and growth factors as tailors of the stem cell niche. Curr. Opin. Cell Biol. 24, 645–651. doi: 10.1016/j.ceb.2012.07.001
Butcher, J. T., and Markwald, R. R. (2007). Valvulogenesis: the moving target. Philos. Trans. R. Soc. Lond. B Biol. Sci. 362, 1489–1503. doi: 10.1098/rstb.2007.2130
Carvalho, J. L., de Carvalho, P. H., Gomes, D. A., and Goes, A. M. (2012). Characterization of decellularized heart matrices as biomaterials for regular and whole organ tissue engineering and initial in-vitro recellularization with ips cells. Physiol. Behav. 176, 139–148. doi: 10.1016/j.physbeh.2017.03.040
Castaldo, C., and Chimenti, I. (2018). Cardiac progenitor cells: the matrix has you. Stem Cells Transl. Med. 7, 506–510. doi: 10.1002/sctm.18-0023
Cheng, P., Andersen, P., Hassel, D., Kaynak, B. L., Limphong, P., Juergensen, L., et al. (2013). Fibronectin mediates mesendodermal cell fate decisions. Development 140, 2587–2596. doi: 10.1242/dev.089052
Chimenti, I., Massai, D., Morbiducci, U., Beltrami, A. P., Pesce, M., and Messina, E. (2017). Stem cell spheroids and ex vivo niche modeling: rationalization and scaling-up. J. Cardiovasc. Transl. Res. 10, 150–166. doi: 10.1007/s12265-017-9741-5
Chimenti, I., Rizzitelli, G., Gaetani, R., Angelini, F., Ionta, V., Forte, E., et al. (2011). Human cardiosphere-seeded gelatin and collagen scaffolds as cardiogenic engineered bioconstructs. Biomaterials 32, 9271–9281. doi: 10.1016/j.biomaterials.2011.08.049
Daley, W. P., Peters, S. B., and Larsen, M. (2008). Extracellular matrix dynamics in development and regenerative medicine. J. Cell Sci. 121, 255–264. doi: 10.1242/jcs.006064
Edalat, S. G., Jang, Y., Kim, J., and Park, Y. (2019). Collagen type I containing hybrid hydrogel enhances cardiomyocyte maturation in a 3D cardiac model. Polymers 11:687. doi: 10.3390/polym11040687
Edri, R., Gal, I., Noor, N., Harel, T., Fleischer, S., Adadi, N., et al. (2019). Personalized hydrogels for engineering diverse fully autologous tissue implants. Adv. Mater. 31:1803895. doi: 10.1002/adma.201803895
Endele, M., Etzrodt, M., and Schroeder, T. (2014). Instruction of hematopoietic lineage choice by cytokine signaling. Exp. Cell Res. 329, 207–213. doi: 10.1016/j.yexcr.2014.07.011
Engler, A. J., Carag-krieger, C., Johnson, C. P., Raab, M., Tang, H., Speicher, D. W., et al. (2008). Embryonic cardiomyocytes beat best on a matrix with heart-like elasticity : scar-like rigidity inhibits beating. J. Cell Sci. 121, 3794–3802. doi: 10.1242/jcs.029678
Ferng, A. S., Connell, A. M., Marsh, K. M., Qu, N., Medina, A. O., Bajaj, N., et al. (2017). Acellular porcine heart matrices: whole organ decellularization with 3D-bioscaffold & vascular preservation. J. Clin. Transl. Res. 3, 260–270. doi: 10.18053/jctres.03.201702.001
Forte, E., Furtado, M. B., and Rosenthal, N. (2018). The interstitium in cardiac repair: role of the immune–stromal cell interplay. Nat. Rev. Cardiol. 15, 601–616. doi: 10.1038/s41569-018-0077-x
French, K. M., Boopathy, A. V., Dequach, J. A., Chingozha, L., Lu, H., Christman, K. L., et al. (2012). A naturally derived cardiac extracellular matrix enhances cardiac progenitor cell behavior in vitro. Acta Biomater. 8, 4357–4364. doi: 10.1016/j.actbio.2012.07.033
Funderburg, F. M., and Markwald, R. R. (1986). Conditioning of native substrates by chondroitin sulfate proteoglycans during cardiac mesenchymal cell migration. J. Cell Biol. 103, 2475–2487. doi: 10.1083/jcb.103.6.2475
Gaetani, R., Doevendans, P. A., Metz, C. H. G., Alblas, J., Messina, E., Giacomello, A., et al. (2012). Cardiac tissue engineering using tissue printing technology and human cardiac progenitor cells. Biomaterials 33, 1782–1790. doi: 10.1016/j.biomaterials.2011.11.003
Gaetani, R., Feyen, D. A. M., Verhage, V., Slaats, R., Messina, E., Christman, K. L., et al. (2015). Epicardial application of cardiac progenitor cells in a 3D-printed gelatin/hyaluronic acid patch preserves cardiac function after myocardial infarction. Biomaterials 61, 339–348. doi: 10.1016/j.biomaterials.2015.05.005
Gaetani, R., Yin, C., Srikumar, N., Braden, R., Doevendans, P. A., Sluijter, J. P. G., et al. (2016). Cardiac-derived extracellular matrix enhances cardiogenic properties of human cardiac progenitor cells. Cell Transplant. 25, 1653–1663. doi: 10.3727/096368915X689794
Gardin, C., Ferroni, L., Latremouille, C., Chachques, J. C., Mitrečić, D., and Zavan, B. (2020). Recent applications of three dimensional printing in cardiovascular medicine. Cells 9:742. doi: 10.3390/cells9030742
Garreta, E., de Oñate, L., Fernández-Santos, M. E., Oria, R., Tarantino, C., Climent, A. M., et al. (2016). Myocardial commitment from human pluripotent stem cells: rapid production of human heart grafts. Biomaterials 98, 64–78. doi: 10.1016/j.biomaterials.2016.04.003
Giacomelli, E., Bellin, M., Sala, L., van Meer, B. J., Tertoolen, L. G. J., Orlova, V. V., et al. (2017). Three-dimensional cardiac microtissues composed of cardiomyocytes and endothelial cells co-differentiated from human pluripotent stem cells. Development 144, 1008–1017. doi: 10.1242/dev.143438
González, A., López, B., Ravassa, S., San José, G., and Díez, J. (2019). The complex dynamics of myocardial interstitial fibrosis in heart failure. Focus on collagen cross-linking. Biochim. Biophys. Acta Mol. Cell Res. 1866, 1421–1432. doi: 10.1016/j.bbamcr.2019.06.001
Guyette, J. P., Charest, J. M., Mills, R. W., Jank, B. J., Moser, P. T., Gilpin, S. E., et al. (2016). Bioengineering human myocardium on native extracellular matrix. Physiol. Behav. 176, 139–148. doi: 10.1016/j.physbeh.2017.03.040
Hanson, K. P., Jung, J. P., Tran, Q. A., Hsu, S. P. P., Iida, R., Ajeti, V., et al. (2013). Spatial and temporal analysis of extracellular matrix proteins in the developing murine heart: a blueprint for regeneration. Tissue Eng. Part A 19, 1132–1143. doi: 10.1089/ten.tea.2012.0316
Horn, M. A. (2015). Cardiac physiology of aging: extracellular considerations. Compr. Physiol. 5, 1069–1121. doi: 10.1002/cphy.c140063
Howard, C. M., and Baudino, T. A. (2013). Dynamic cell–cell and cell–ECM interactions in the heart. J. Mol. Cell. Cardiol. 70, 19–26. doi: 10.1016/j.yjmcc.2013.10.006
Huang, J., Potts, J. A. Y. D., Vincent, E. B., Weeks, D. L., and Runyanbf, R. B. (1995). Mechanisms of cell transformation in the embryonic heart. Ann. N. Y. Acad. Sci. 752, 317–330. doi: 10.1111/j.1749-6632.1995.tb17441.x
Hynes, R. O. (2009). Extracellular matrix: not just pretty fibrils. Science 326, 1216–1219. doi: 10.1126/science.1176009.Extracellular
Icardo, J. M., and Manasek, F. J. (1983). An indirect immunofluorescence study of the distribution of fibronectin during the formation of the cushion tissue mesenchyme in the embryonic heart. Dev. Biol. 101, 336–345. doi: 10.1016/0012-1606(84)90147-7
Ireland, R. G., and Simmons, C. A. (2015). Human pluripotent stem cell mechanobiology: manipulating the biophysical microenvironment for regenerative medicine and tissue engineering applications. Stem Cells 33, 3187–3196. doi: 10.1002/stem.2105
Jacot, J. G., McCulloch, A. D., and Omens, J. H. (2008). Substrate stiffness affects the functional maturation of neonatal rat ventricular myocytes. Biophys. J. 95, 3479–3487. doi: 10.1529/biophysj.107.124545
Jang, J., Park, H. J., Kim, S. W., Kim, H., Park, J. Y., Na, S. J., et al. (2017). 3D printed complex tissue construct using stem cell-laden decellularized extracellular matrix bioinks for cardiac repair. Biomaterials 112, 264–274. doi: 10.1016/j.biomaterials.2016.10.026
Johnson, T. D., Dequach, J. A., Gaetani, R., Ungerleider, J., Elhag, D., Nigam, V., et al. (2014). Human versus porcine tissue sourcing for an injectable myocardial matrix hydrogel. Biomater. Sci. 2, 735–744. doi: 10.1039/c3bm60283d
Jung, J. P., Hu, D., Domian, I. J., and Ogle, B. M. (2015). An integrated statistical model for enhanced murine cardiomyocyte differentiation via optimized engagement of 3D extracellular matrices. Sci. Rep. 5:18705. doi: 10.1038/srep18705
Kelly, R. G., Buckingham, M. E., and Moorman, A. F. (2014). Heart fields and cardiac morphogenesis. Cold Spring Harb. Perspect. Med. 4:a015750. doi: 10.1101/cshperspect.a015750
Kim, H. D., Yoon, C. S., Kim, H., and Rah, B. J. (1999). Expression of extracellular matrix components fibronectin and laminin in the human fetal heart. Cell Struct. Funct. 24, 19–26. doi: 10.1247/csf.24.19
Lee, P. F., Chau, E., Cabello, R., Yeh, A. T., Sampaio, L. C., Gobin, A. S., et al. (2017). Inverted orientation improves decellularization of whole porcine hearts. Acta Biomater. 49, 181–191. doi: 10.1016/j.actbio.2016.11.047
Li, R. A., Keung, W., Cashman, T. J., Backeris, P. C., Johnson, B. V., Bardot, E. S., et al. (2018). Bioengineering an electro-mechanically functional miniature ventricular heart chamber from human pluripotent stem cells HHS public access. Biomaterials 163, 116–127. doi: 10.1016/j.biomaterials.2018.02.024
Liau, B., Christoforou, N., Leong, K., and Bursac, N. (2011). Pluripotent stem cell-derived cardiac tissue patch with advanced structure and function. Biomaterials 32, 9180–9187. doi: 10.1038/jid.2014.371
Lie, D. C., Colamarino, S. A., Song, H. J., Désiré, L., Mira, H., Consiglio, A., et al. (2005). Wnt signalling regulates adult hippocampal neurogenesis. Nature 437, 1370–1375. doi: 10.1038/nature04108
Little, C. D., Piquet, D. M., Davis, L. A., Walters, L., and Drake, C. J. (1989). Distribution of laminin, collagen type IV, collagen type I, and fibronectin in chicken cardiac jelly/basement membrane. Anat. Rec. 224, 417–425. doi: 10.1002/ar.1092240310
Lockhart, M., Wirrig, E., Phelps, A., and Wessels, A. (2011). Extracellular matrix and heart development. Birth Defects Res. Part A Clin. Mol. Teratol. 91, 535–550. doi: 10.1002/bdra.20810
Lu, J., Kaestle, K., Huang, J., Liu, Q., Zhang, P., Gao, L., et al. (2017). Interactions of human embryonic stem cell-derived cardiovascular progenitor cells with immobilized extracellular matrix proteins. J. Biomed. Mater. Res. Part A 105, 1094–1104. doi: 10.1002/jbm.a.36005
Lu, T. Y., Lin, B., Kim, J., Sullivan, M., Tobita, K., Salama, G., et al. (2013). Repopulation of decellularized mouse heart with human induced pluripotent stem cell-derived cardiovascular progenitor cells. Nat. Commun. 4:2307. doi: 10.1038/ncomms3307
Lu, Y. Y., Chen, Y. C., Kao, Y. H., Wu, T. J., Chen, S. A., and Chen, Y. J. (2011). Extracellular matrix of collagen modulates intracellular calcium handling and electrophysiological characteristics of HL-1 cardiomyocytes with activation of angiotensin II type 1 receptor. J. Card. Fail. 17, 82–90. doi: 10.1016/j.cardfail.2010.10.002
Macqueen, L. A., Sheehy, S. P., Chantre, C. O., Zimmerman, J. F., Pasqualini, F. S., Liu, X., et al. (2018). A tissue-engineered scale model of the heart ventricle. Nat. Biomed. Eng. 2, 930–941. doi: 10.1038/s41551-018-0271-5
Masumoto, H., Nakane, T., Tinney, J. P., Yuan, F., Ye, F., Kowalski, W. J., et al. (2016). The myocardial regenerative potential of three-dimensional engineered cardiac tissues composed of multiple human iPS cell-derived cardiovascular cell lineages. Sci. Rep. 6:29933. doi: 10.1038/srep29933
Miyamoto, S., Teramoto, H., and Gutkind, J. S. (1996). Integrins can collaborate with growth factors for phosphorylation of receptor tyrosine kinases and MAP kinase activation: roles of integrin aggregation and occupancy of receptors. J. Cell Biol. 135, 1633–1642. doi: 10.1083/jcb.135.6.1633
Mjaatvedt, C. H., Lepera, R. C., and Markwald, R. R. (1987). Myocardial specificity for initiating endothelial-mesenchymal cell transition in embryonic chick heart correlates with a particulate distribution of fibronectin. Dev. Biol. 119, 59–67. doi: 10.1016/0012-1606(87)90206-5
Nakayama, K. H., Hou, L., and Huang, N. F. (2014). Role of extracellular matrix signaling cues in modulating cell fate commitment for cardiovascular tissue engineering. Adv. Healthc. Mater. 3, 628–641. doi: 10.1002/adhm.201300620
Neiman, G., Scarafía, M. A., La Greca, A., Velazque, N. L. S., Garate, X., Waisman, A., et al. (2019). Integrin alpha-5 subunit is critical for the early stages of human pluripotent stem cell cardiac differentiation. Sci. Rep. 9:18077.
Noor, N., Shapira, A., Edri, R., Gal, I., Wertheim, L., and Dvir, T. (2019). 3D printing of personalized thick and perfusable cardiac patches and hearts. Adv. Sci. 6:1900344. doi: 10.1002/advs.201900344
Oberwallner, B., Brodarac, A., Anić, P., Šarić, T., Wassilew, K., Neef, K., et al. (2015). Human cardiac extracellular matrix supports myocardial lineage commitment of pluripotent stem cells. Eur. J. Cardiothorac. Surg. 47, 416–425. doi: 10.1093/ejcts/ezu163
Ott, H. C., Matthiesen, T. S., Goh, S. K., Black, L. D., Kren, S. M., Netoff, T. I., et al. (2008). Perfusion-decellularized matrix: using nature’s platform to engineer a bioartificial heart. Nat. Med. 14, 213–221. doi: 10.1038/nm1684
Ou, D. B., He, Y., Chen, R., Teng, J. W., Wang, H. T., Zeng, D., et al. (2011). Three-dimensional co-culture facilitates the differentiation of embryonic stem cells into mature cardiomyocytes. J. Cell. Biochem. 112, 3555–3562. doi: 10.1002/jcb.23283
Pagano, F., Angelini, F., Castaldo, C., Picchio, V., Messina, E., Sciarretta, S., et al. (2017). Normal versus pathological cardiac fibroblast-derived extracellular matrix differentially modulates cardiosphere-derived cell paracrine properties and commitment. Stem Cells Int. 2017:7396462. doi: 10.1155/2017/7396462
Pasquier, J., Gupta, R., Rioult, D., Hoarau-Véchot, J., Courjaret, R., Machaca, K., et al. (2017). Coculturing with endothelial cells promotes in vitro maturation and electrical coupling of human embryonic stem cell–derived cardiomyocytes. J. Hear Lung Transplant. 36, 684–693. doi: 10.1016/j.healun.2017.01.001
Pati, F., and Cho, D. W. (2017). Bioprinting of 3D tissue models using decellularized extracellular matrix bioink. Methods Mol. Biol. 1612, 381–390. doi: 10.1007/978-1-4939-7021-6_27
Pesce, M., Messina, E., Chimenti, I., and Beltrami, A. P. (2017). Cardiac mechanoperception: a life-long story from early beats to aging and failure. Stem Cells Dev. 26, 77–90. doi: 10.1089/scd.2016.0206
Reya, T., Duncan, A. W., Ailles, L., Domen, J., Scherer, D. C., Willert, K., et al. (2003). A role for Wnt signalling in self-renewal of haematopoietic stem cells. Nature 423, 409–414. doi: 10.1038/nature01593
Rienks, M., Papageorgiou, A.-P., Frangogiannis, N. G., and Heymans, S. (2014). Myocardial extracellular matrix. Circ. Res. 114, 872–888. doi: 10.1161/CIRCRESAHA.114.302533
Ross, R. S., and Borg, T. K. (2001). Integrins and the myocardium. Circ. Res. 88, 1112–1119. doi: 10.1161/hh1101.091862
Sa, S., Wong, L., and McCloskey, K. E. (2014). Combinatorial fibronectin and laminin signaling promote highly efficient cardiac differentiation of human embryonic stem cells. Biores. Open Access 3, 150–161. doi: 10.1089/biores.2014.0018
Saleem, S., Li, J., Yee, S., Fellows, G. F., Goodyer, C. G., and Wang, R. (2009). β1 integrin/FAK/ERK signalling pathway is essential for human fetal islet cell differentiation and survival. J. Pathol. 219, 182–192. doi: 10.1002/path
Sánchez, P. L., Fernández-Santos, M. E., Costanza, S., Climent, A. M., Moscoso, I., Gonzalez-Nicolas, M. A., et al. (2015). Acellular human heart matrix: a critical step toward whole heart grafts. Biomaterials 61, 279–289. doi: 10.1016/j.biomaterials.2015.04.056
Schenke-Layland, K., Nsair, A., Van Handel, B., Angelis, E., Gluck, J. M., Votteler, M., et al. (2012). Recapitulation of the embryonic cardiovascular progenitor cell niche. Biomaterials 32, 2748–2756. doi: 10.1016/j.biomaterials.2010.12.046.Recapitulation
Singelyn, J. M., and Christman, K. L. (2010). Injectable materials for the treatment of myocardial infarction and heart failure: the promise of decellularized matrices. J. Cardiovasc. Transl. Res. 3, 478–486. doi: 10.1007/s12265-010-9202-x
Tallawi, M., Rai, R., Boccaccini, A. R., and Aifantis, K. E. (2015). Effect of substrate mechanics on cardiomyocyte maturation and growth. Tissue Eng. Part B Rev. 21, 157–165. doi: 10.1089/ten.teb.2014.0383
Tang-Quan, K. R., Mehta, N. A., Sampaio, L. C., and Taylor, D. A. (2018). Whole cardiac tissue bioscaffolds. Adv. Exp. Med. Biol. 1098, 59–83. doi: 10.1007/978-3-319-97421-7
Thiery, J. P., Acloque, H., Huang, R. Y. J., and Nieto, M. A. (2009). Epithelial-mesenchymal transitions in development and disease. Cell 139, 871–890. doi: 10.1016/j.cell.2009.11.007
Thompson, R. P., Fitzharris, T. P., Denslow, S., and LeRoy, E. C. (1979). Collagen synthesis in the developing chick heart. Tex. Rep. Biol. Med. 39, 305–319.
Valls-Margarit, M., Iglesias-García, O., Di Guglielmo, C., Sarlabous, L., Tadevosyan, K., Paoli, R., et al. (2019). Engineered macroscale cardiac constructs elicit human myocardial tissue-like functionality. Stem Cell Rep. 13, 207–220. doi: 10.1016/j.stemcr.2019.05.024
Vanwinkle, W. B., Snuggs, M. B., and Buja, L. M. (1996). Cardiogel: a biosynthetic extracellular matrix for cardiomyocyte culture. Vitr. Cell. Dev. Biol. Anim. 32, 478–485. doi: 10.1007/BF02723051
Votteler, M., Kluger, P. J., Walles, H., and Schenke-layland, K. (2010). Stem cell microenvironments - unveiling the secret of how stem cell fate is defined. Macromol. Biosci. 10, 1302–1315. doi: 10.1002/mabi.201000102
Vuorenpää, H., Penttinen, K., Heinonen, T., Pekkanen-Mattila, M., Sarkanen, J. R., Ylikomi, T., et al. (2017). Maturation of human pluripotent stem cell derived cardiomyocytes is improved in cardiovascular construct. Cytotechnology 69, 785–800. doi: 10.1007/s10616-017-0088-1
Wang, X., Harris, R. E., Bayston, L. J., and Ashe, H. L. (2008). Type IV collagens regulate BMP signalling in Drosophila. Nature 2, 313–315. doi: 10.1038/nature07214
Wang, Z., Long, D. W., Huang, Y., Chen, W. C. W., Kim, K., and Wang, Y. (2019). Decellularized neonatal cardiac extracellular matrix prevents widespread ventricular remodeling in adult mammals after myocardial infarction. Acta Biomater. 87, 140–151. doi: 10.1016/j.actbio.2019.01.062
Witman, N., and Sahara, M. (2018). Cardiac progenitor cells in basic biology and regenerative medicine. Stem Cells Int. 2018:8283648. doi: 10.1155/2018/8283648
Wozniak, M. A., Modzelewska, K., Kwong, L., and Keely, P. J. (2004). Focal adhesion regulation of cell behavior. Biochim. Biophys. Acta Mol. Cell Res. 1692, 103–119. doi: 10.1016/j.bbamcr.2004.04.007
Young, J. L., and Engler, A. J. (2011). Hydrogels with time-dependent material properties enhance cardiomyocyte differentiation in vitro. Bone 32, 1002–1009. doi: 10.1038/jid.2014.371
Yu, C., Ma, X., Zhu, W., Wang, P., Miller, K. L., Stupin, J., et al. (2019). Scanningless and continuous 3D bioprinting of human tissues with decellularized extracellular matrix. Biomaterials 194, 1–13. doi: 10.1016/j.biomaterials.2018.12.009
Yustzey, K. E., and Kirby, M. L. (2002). Wherefore heart thou? Embryonic origins of cardiogenic. Dev. Dyn. 320, 307–320. doi: 10.1002/dvdy.10068
Zeng, D., Ou, D. B., Wei, T., Ding, L., Liu, X. T., Hu, X. L., et al. (2013). Collagen/β1 integrin interaction is required for embryoid body formation during cardiogenesis from murine induced pluripotent stem cells. BMC Cell Biol. 14:5. doi: 10.1186/1471-2121-14-5
Zhang, J., Klos, M., Wilson, G. F., Herman, A. M., Lian, X., Raval, K. K., et al. (2012). Extracellular matrix promotes highly efficient cardiac differentiation of human pluripotent stem cells: the matrix sandwich method. Circ. Res. 111, 1125–1136. doi: 10.1161/CIRCRESAHA.112.273144
Keywords: cardiac microenvironment, extracellular matrix, cardiac tissue engineering, cardiac differentiation, 3D bioprinting
Citation: Pagliarosi O, Picchio V, Chimenti I, Messina E and Gaetani R (2020) Building an Artificial Cardiac Microenvironment: A Focus on the Extracellular Matrix. Front. Cell Dev. Biol. 8:559032. doi: 10.3389/fcell.2020.559032
Received: 04 May 2020; Accepted: 18 August 2020;
Published: 04 September 2020.
Edited by:
Pavel Makarevich, Lomonosov Moscow State University, RussiaReviewed by:
Natalia Kalinina, Lomonosov Moscow State University, RussiaJinah Jang, Pohang University of Science and Technology, South Korea
Copyright © 2020 Pagliarosi, Picchio, Chimenti, Messina and Gaetani. This is an open-access article distributed under the terms of the Creative Commons Attribution License (CC BY). The use, distribution or reproduction in other forums is permitted, provided the original author(s) and the copyright owner(s) are credited and that the original publication in this journal is cited, in accordance with accepted academic practice. No use, distribution or reproduction is permitted which does not comply with these terms.
*Correspondence: Roberto Gaetani, cm9iZXJ0by5nYWV0YW5pQHVuaXJvbWExLml0