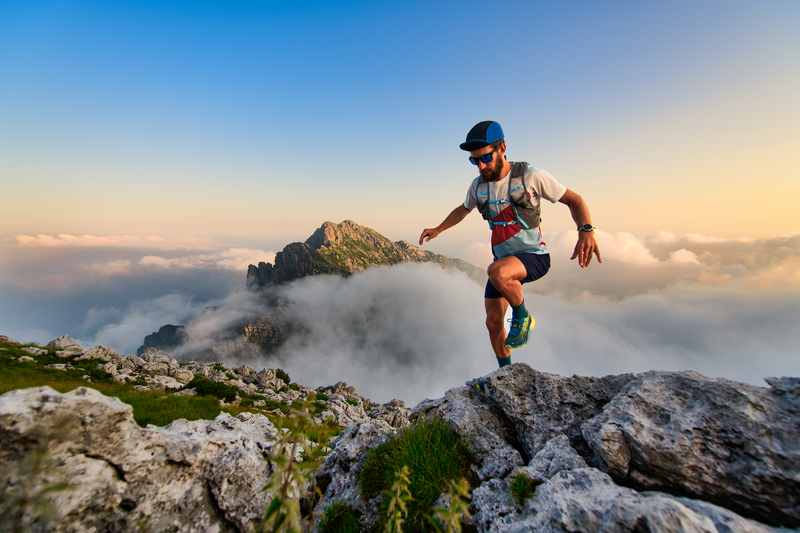
95% of researchers rate our articles as excellent or good
Learn more about the work of our research integrity team to safeguard the quality of each article we publish.
Find out more
REVIEW article
Front. Cell Dev. Biol. , 28 October 2020
Sec. Stem Cell Research
Volume 8 - 2020 | https://doi.org/10.3389/fcell.2020.550504
This article is part of the Research Topic Induced Pluripotent Stem Cell-Based Disease Modeling and Drug Discovery: Can We Recapitulate Cardiovascular Disease on a Culture Dish? View all 13 articles
Thoracic aortic diseases, whether sporadic or due to a genetic disorder such as Marfan syndrome, lack effective medical therapies, with limited translation of treatments that are highly successful in mouse models into the clinic. Patient-derived induced pluripotent stem cells (iPSCs) offer the opportunity to establish new human models of aortic diseases. Here we review the power and potential of these systems to identify cellular and molecular mechanisms underlying disease and discuss recent advances, such as gene editing, and smooth muscle cell embryonic lineage. In particular, we discuss the practical aspects of vascular smooth muscle cell derivation and characterization, and provide our personal insights into the challenges and limitations of this approach. Future applications, such as genotype-phenotype association, drug screening, and precision medicine are discussed. We propose that iPSC-derived aortic disease models could guide future clinical trials via “clinical-trials-in-a-dish”, thus paving the way for new and improved therapies for patients.
Thoracic aortic disease usually proceeds silently until presenting suddenly with dissection or rupture (Pinard et al., 2019). Despite the frequently catastrophic and life-threatening consequences, there are no proven medical treatments for thoracic aortic disease beyond blood pressure control. Surgical replacement of the diseased section of aorta, either emergent or prophylactically, can be associated with significant morbidity and does not prevent disease progression or re-presentation in the non-replaced parts of the vessel. The lack of effective medical therapies has highlighted the critical need to define the mechanisms underlying aortic dilatation and dissection to inform the development of new treatments (Milewicz et al., 2005).
In contrast to abdominal aortic aneurysms, which have been shown to have links to inflammation and atherosclerosis, thoracic aortic aneurysms and disorders are frequently due to genetic factors (Humphrey et al., 2015; Pinard et al., 2019). A key question is to what extent the different genetic syndromes and disorders have common disease-causing pathways. The underlying mechanisms leading to aortic disease are still unclear despite the use of several mouse models; indeed, therapeutic discoveries made using the mouse models have not yet been shown to be effective in patients. Consequently, there is a pressing need for further studies and a wider range of model systems that may more fully predict a clinical response.
Through their seminal discovery of induced pluripotent stem cells (iPSCs), Takahashi and Yamanaka have bestowed the tools to now establish patient-derived complex models of human genetic diseases (Takahashi and Yamanaka, 2006). The power of this approach lies in the fact that these cells contain the patient’s DNA, so exhibit both the causal genetic defects as well as the permissive genetic background that allows florid disease presentation. Furthermore, these cells represent a versatile and almost unlimited resource for the study of early disease processes and for drug discovery. Such is their potential utility for understanding and treating diseases that they have been referred to as “disease-in-a-dish” models (Tiscornia et al., 2011).
In this review we will critically discuss recent studies where iPSCs have been used to model thoracic aortic aneurysm and dissection (TAAD) disorders. Since these related disorders have already been reviewed in detail by others (Goldfinger et al., 2014; Michel et al., 2018), we will only briefly cover the diseases themselves and highlight the controversies and major questions that have emerged in this field. We will then devote the majority of this review to providing insights into the practical aspects, applications, strengths, and limitations of using iPSCs to model these conditions. Finally, we will explore potential future directions for this approach including precision medicine and “clinical-trials-in-a-dish.”
Thoracic aortic aneurysm and dissections commonly occur sporadically or in association with bicuspid aortic valves (BAV). Single gene disorders also cause thoracic aortopathies, notably in genes encoding extracellular matrix (ECM) components, transforming growth factor (TGF)-β signaling, or vascular smooth muscle cell (VSMC) contractile machinery (Brownstein et al., 2018). Marfan syndrome (MFS), caused by mutations in FBN1, is the commonest and best studied genetic disease resulting in TAAD. Other syndromic disorders include Loeys-Dietz syndrome (LDS) and vascular Ehlers-Danlos syndrome (vEDS) which are caused by mutations in the TGF-β signaling cascade (Lindsay et al., 2012) and in COL3A1 (Pepin et al., 2000), respectively. Mechanistically, it is likely that TAADs share common disease mechanisms. Improving our understanding of Mendelian genetic disorders is also likely to lead to effective treatments for sporadic and bicuspid valve-associated aortopathies.
Many TAAD disorders show considerable overlap in pathology with elevated matrix metalloproteinases (MMPs), elastin fiber breaks, proteoglycan, and glycosaminoglycan deposition and medial aortic VSMC loss, suggesting common final pathways for aneurysm development despite varying genetic causes. An intimal tear then leads to an influx of blood and medial dissection; a condition with a cumulative 1% mortality per hour if the dissection involves the ascending aorta – a type A dissection (Anagnostopoulos et al., 1972). This dramatic surgical emergency is due to the propensity of a type A dissection to progress retrogradely and involve the coronaries, leading to myocardial infarction, or the pericardium, leading to tamponade. The risk of dissection is in part a function of aneurysm size, although the correlation varies widely depending on the precise disease as well as other familial factors and co-morbidities such as the presence of hypertension. Notably, some disorders such as LDS or vEDS, can present with arterial dissection or rupture at relatively normal vessel dimensions (Pepin et al., 2000; Williams et al., 2007), emphasizing the need for additional prognostic markers to supplement cross-sectional imaging.
In this review, we use MFS as the exemplar for genetically mediated TAADs. We will discuss the biological controversies and clinical issues raised by MFS to illustrate the challenges in the management of patients with TAAD and areas where novel approaches may be helpful. MFS is an autosomal dominant, multi-system disease affecting approximately 1 in 5000 people, caused by mutations in the gene encoding fibrillin-1, a key connective tissue ECM protein (Dietz et al., 1991). Fibrillin-1 glycoproteins assemble into microfibrils, which have both structural and functional roles. These microfibrils provide elasticity and provide a template for elastin fiber formation, but can also regulate the bioavailability of growth factors, such as TGF-β (Chaudhry et al., 2007), and provide attachment motifs for cell-matrix interactions (Kielty et al., 1992; Bax et al., 2003).
The cardiovascular complications are potentially fatal, and affect men more strongly than women (Murdoch et al., 1972; Pyeritz and KcKusick, 1979). Patients can develop mitral valve prolapse and aortic regurgitation, with the significant complication being aortic dilatation. These aortic aneurysms typically form in the aortic root and arch, and predispose to rupture or dissection (Milewicz et al., 2005). As with other TAADs, VSMCs from MFS patients typically have high expression and activity of MMPs, elastic fiber fragmentation and VSMC death, which all lead to weakening of the aortic wall (Segura et al., 1998; Ikonomidis et al., 2006; Grewal and Gittenberger-de Groot, 2018). In addition, there is increased deposition of collagen and proteoglycans, which contributes to increased vessel stiffness (Andreotti et al., 1985; Cattell et al., 1994). Indeed, patients with MFS tend to have stiffer aortas compared to the general population (Jeremy et al., 1994; De Wit et al., 2013; Hannuksela et al., 2018).
Mouse models of MFS have been very useful to understand a variety of disease aspects. Two models are commonly reported in the literature – the FBN1C1039G/+ (Judge et al., 2004) and FBN1MgR/MgR (Pereira et al., 1999) models which represent moderate and severe disease, respectively. In addition to powerful tools to dissect the genetics, mouse models allow for deep phenotypic and histological characterization. The different stages of disease progression can also be investigated, making murine models essential for understanding disease mechanisms. The findings from these disease models, in addition to their drawbacks, will be discussed further below.
Groups, including ours, have used iPSCs to investigate the pathology underlying MFS. Longaker and colleagues used a MFS embryonic stem cell (ESC) line as well as patient-derived iPSCs to show how antagonism of BMP signaling by TGF-β signaling impaired osteogenesis, leading to abnormal skeletogenesis (Quarto et al., 2012a, b). More recently, we used patient-derived iPSCs differentiated into VSMCs to recapitulate many aspects of vascular disease found in patients (Granata et al., 2017). This included increased MMP expression and cell death, fragmentation of ECM microfibrils, and reduced proliferation (Figure 1). Interestingly, when cells were exposed to cyclic mechanical stretch, the disease phenotype was further exaggerated, suggesting that there are abnormalities in mechanosensing/transduction, in line with current thinking about the mechanisms leading to disease progression. These disease features were rescued by using CRISPR-Cas9 mediated single nucleotide correction resulting in an isogenic normal control. iPSC-based models of other aortic diseases have also been developed, and are summarized in Tables 1, 2. These models have successfully recapitulated key aspects of aortic diseases, and have enabled identification of potential disease mechanisms for further investigation.
Figure 1. Summary of aortic disease phenotype recapitulated in MFS iPSC model (Granata et al., 2017).
The TGF-β signaling pathway is crucial for normal VSMC function and it is a potent cytokine regulating proliferation, differentiation, extracellular matrix remodeling, and apoptosis (Guo, 2012). Activation of TGF-β receptors leads to canonical signaling through Smads but also non-canonical signaling through MAPKs (Zhang, 2017). Analysis of the lung of a severe mouse model of MFS, FBN1mgΔ, found increased activation of TGF-β (Neptune et al., 2003). Since treatment with a TGF-β neutralizing antibody rescued the lung phenotype, the Dietz lab hypothesized that the loss of microfibrils decreased the sequestration of TGF-β and in turn led to an increase in local TGF-β signaling. This line of thinking was supported by findings in the moderate FBN1C1039G/+ murine model (Judge et al., 2004), where increased canonical TGF-β signaling was detected in the dilated aorta. Treatment with a TGF-β neutralizing antibody once again rescued the disease phenotype (Habashi et al., 2006), as did blockade of the angiotensin II receptor type 1 (AT1R) with losartan, which reduced TGF-β expression and non-canonical signaling (Lavoie et al., 2005; Rodríguez-Vita et al., 2005; Holm et al., 2011).
Given the dramatic results with losartan in mouse models of MFS, a series of clinical trials in patients commenced. An initial retrospective analysis of a pediatric cohort of MFS patients suggested promising results in slowing aortic dilatation (Brooke et al., 2008). Several randomized trials have now been carried out comparing losartan either to β-blocker or to placebo (Groenink et al., 2013; Lacro et al., 2014; Milleron et al., 2015; Teixido-Tura et al., 2018). Surprisingly, despite some early promise in small trials, the largest single randomized study has shown that losartan had no statistically significant improvement in children and young adult patients when compared to β-blockers (Lacro et al., 2014). Related to the findings in the initial retrospective analysis, this larger study found that the younger subjects were more responsive to treatment with losartan compared to the older cohort, suggesting that there may be an early therapeutic window for targeting angiotensin II signaling.
Subsequent evidence from mouse studies has indicated that the nature of TGF-β signaling in TAAD progression is complex, and may also confer a protective effect. Post-natal VSMC-specific deletion of TGF-β receptor II (TβRII; Hu et al., 2015) or treatment with a TGF-β neutralizing antibody (Wang et al., 2010) led to severe aortopathy. Indeed, crossing FBN1C1039G/+ mice with a conditional knock-out for Tgfbr2 exacerbated the aortic phenotype, indicating that TGF-β may have a protective effect (Li et al., 2014; Wei et al., 2017). FBN1MgR/MgR is a severe model for MFS (Pereira et al., 1999) in which treatment with losartan slightly improved lifespan, but did not have the same impact as in the moderate FBN1C1039G/+ model (Xiong et al., 2012; Cook et al., 2015). In addition, treatment with a TGF-β neutralizing antibody was detrimental at P16, but beneficial at P45, indicative of a temporally dependent role for TGF-β in aneurysm formation (Cook et al., 2015). Other studies did not find any benefit of TGF-β or angiotensin II signaling inhibition in VSMCs (Angelov et al., 2017; Galatioto et al., 2018). Together, these lines of evidence indicate that the pathophysiology of MFS is more complex than just dysfunction of TGF-β signaling in VSMCs. The upregulation of TGF-β signaling in MFS may in part be a compensatory mechanism, rationalizing the increase observed in patients with severe aneurysm (Franken et al., 2013).
The losartan and TGF-β controversy indicates that further mechanistic validation is required when transitioning between mouse studies and patient treatment, particularly in the context of the human genome. While losartan was highly-effective and promising in a mouse model, its effectiveness was not matched in patients. This was potentially due to fundamental differences in the anatomy between murine and human aortas, but also due to the disparity between the dose required to elicit a response, and the dose deemed safe for human patients. Recently, another AT1R antagonist, irbesartan, was found to be effective in reducing aortic dilatation in children and young adults (Mullen et al., 2020). Although losartan and irbesartan both inhibit AT1R, irbesartan has greater bioavailability and a longer half-life, implying that the difference in outcome may be in part due to insufficient duration of action of losartan. In addition, while the mice used for the losartan studies were genetically homogeneous and treated at the same age, the human patients introduced variability via their disease-causing mutations, genetic backgrounds and ages at treatment. Although animal models allow us to study various stages of disease and are still needed to assess potential therapeutic targets, this case has highlighted the need for an additional platform to assess the viability of mechanisms and treatments in a variety of patient lines before applying them in the clinic.
If excess TGF-β signaling is not causal in MFS, then what is? The contractile machinery of VSMCs is composed of thin and thick filaments that contain α-smooth muscle actin (α-SMA; ACTA2) and smooth muscle myosin heavy chain (SM-MHC; MYH11), respectively. In healthy conditions, once stress has been sensed via integrins (Martinez-Lemus et al., 2003), VSMCs can secrete various factors such as MMPs, TGF-β and angiotensin II to adapt the ECM and modulate VSMC phenotype to maintain blood pressure homeostasis (O’Callaghan and Williams, 2000). TAAD-causing mutations in ACTA2 and MYH11 disrupt their function (Zhu et al., 2006; Guo et al., 2007), suggesting that reduced VSMC contractility may be an underlying disease mechanism. In an iPSC model of LDS, where a mutation in SMAD3 was created, the resulting VSMCs had decreased expression of contractile markers (Gong et al., 2020). Similarly, ECM mutations may disrupt the VSMC linkage to the matrix and ability to accurately sense wall stress. This is supported by electron microscopy images from MFS mice showing abnormally smooth elastic fibers due to reduced VSMC attachment (Bunton et al., 2001). It has therefore been proposed that abnormalities in mechanosensing, erroneous ECM remodeling and cellular response lead to aneurysm formation (Humphrey et al., 2015; Pinard et al., 2019). Another way in which mechanical forces may act could be by reduced vascular tone resulting in increased interstitial fluid leading to the formation of intramural edema and dissection (Mallat et al., 2016). This is supported by a study in rat abdominal aortic rings, where noradrenaline-stimulated VSMC contraction decreased hydraulic conductance (Chooi et al., 2017).
The mechanosensing hypothesis is supported by evidence from mouse models. Endothelial nitric oxide (NO)-mediated vasodilation exacerbated aortic aneurysm (Oller et al., 2017). In addition, treatment with calcium channel blockers as an alternative to current anti-hypertensive drugs also accelerated aneurysm formation in a model of MFS (Doyle et al., 2015). Post-natal Tgfbr2 knock-out in mice led to decreased contractile gene expression and compaction in a collagen gel assay (Li et al., 2014). Further assessment of these mice found compromised aortic mechanical properties compared to controls, and treatment of these animals with rapamycin restored some of these mechanical properties and prevented pressure-induced delamination in vitro (Ferruzzi et al., 2016). Rapamycin has been shown to improve VSMC contractility (Martin et al., 2004), and has been used to rescue VSMC de-differentiation phenotypes, including in vitro disease models of supravalvular aortic stenosis (SVAS) and BAV/TAA (Kinnear et al., 2013; Jiao et al., 2016).
The relationship between inappropriate mechanosensing and TAAD formation is not yet fully understood. In addition to using animal models, iPSC-derived VSMCs could be used to investigate this hypothesis, as they can be genetically modified and stretched using various cell-stretching apparatus. We observed worsening of the disease phenotype upon cyclic stretch in our in vitro model of MFS (Granata et al., 2017), indicating that current protocols result in VSMCs sufficiently mature to be capable of mechanotransduction. Substrate stiffness is also something which can be explored – as mentioned above, the aortas of patients with TAADs tend to be stiffer. Combining iPSC-based models with hydrogels of varying stiffnesses could provide insights into the role of vessel wall stiffness in aortic disease.
Samples of diseased aortas can only be obtained from late-stage disease at the time of surgery, thus providing markers and mechanistic insight corresponding to severe TAADs only. From a therapeutic stand-point, investigating late-stage tissue provides limited information for developing novel therapies to prevent progression or identifying biomarkers for various stages of disease. Another challenge of using tissue from patients is the lack of appropriate controls. It is highly unlikely that researchers can obtain clinical samples of a healthy individual’s aorta, but surgeons repairing a diseased aorta may collect biopsies from non-diseased sections, or at least from regions displaying no visible defects. However, such samples likely do not truly represent a healthy aorta, especially in the case of genetic disorders. Also, a region adjacent to the aneurysm could still exhibit defects in the ECM, signaling and response to mechanical stimuli. In addition, cytokines and growth factors in the circulation as well as local environmental cues may also contribute to the disease phenotype.
Early events in disease progression need to be better understood and characterized. As will be discussed further, there is significant variation in the disease presentation of MFS, even among individuals with the same causative mutation in FBN1. It is therefore difficult to predict from initial diagnosis whether disease progression will be mild or severe and this is a particular problem for sporadic cases with no family history. In addition, in disorders such as vEDS, patients do not tolerate surgery, with high post-operative mortality (Bergqvist et al., 2013). Consequently, treating patients at an early stage to prevent presentation or slow aneurysm growth would be ideal, and therefore understanding the early events in disease progression is critical.
These limitations may be circumvented by the use of iPSC-derived VSMCs. A virtually unlimited supply of cells can be generated from patient-derived iPSC lines along with genetically corrected isogenic controls. In our experience, both early and late events can be captured to some extent in vitro. For example, accumulation of disease phenotype with age is observed in the iPSC model of MFS. After differentiating MFS iPSCs to neural crest (NC)-derived VSMCs, we allow the cells to mature in serum for 30 days – during this time, the cells accrue a more severe phenotype in the dish, including increased proteolytic activity and apoptosis (Granata et al., 2017). We observed that NC-VSMCs at an earlier stage did not show the same intensity of disease characteristics, suggesting that, to an extent, we can mimic disease progression in vitro. We therefore suggest that iPSC-based models of VSMCs enable us to generate appropriate control cells and uncover events at various stages of disease progression.
Thoracic aortic aneurysm and dissections are a group of disorders with life-threatening circumstances, and although surgical intervention has increased the mean life expectancy from 45 to 70 years in MFS (Milewicz et al., 2005), new medical treatments need to be urgently identified. Confounding results between mouse and clinical studies have emphasized the need for an additional assessment platform. iPSC-based modeling of aortic disease can be employed, where mechanistic and patient-specific information is used to direct future clinical trials and precision medicine. In the next section, we will discuss practical considerations for constructing a “disease-in-a-dish.”
In vitro differentiation protocols are generally founded on developmental principles (Keller, 2005; Ayoubi et al., 2017). For VSMC development, a huge body of work exists and as a detailed discussion is beyond the scope of this review, we refer the reader to excellent reviews written by others (Owens et al., 2004; Owens, 2007). Briefly, after endothelial cells (ECs) form a lumen mural cells are recruited and invested to stabilize the nascent vessel through various signaling axes, such as TGF-β, PDGF-BB, Notch and angiopoietin/Tie2 (Drake, 2003; Liu et al., 2009; Stenzel et al., 2009; Patel-Hett and D’Amore, 2011). This leads to the establishment of transcriptional modules, including SRF, GATA factors, and myocardin (Croissant et al., 1996; Manabe and Owens, 2001a; Chen et al., 2002; Nishida et al., 2002; Du et al., 2003). In addition, post-transcriptional processes, such as miR-143/145, have also been shown to contribute to this VSMC identity (Boucher et al., 2011). Finally, changes in the epigenome have been shown to allow binding of key transcription factors to their promoters, and lead to stabilization of this VSMC-specific gene expression, while still allowing for phenotypic plasticity depending on the integration of various inputs by the cells (Manabe and Owens, 2001b). Together, these processes lead to the stable expression of VSMC-specific gene expression. These markers of VSMCs can be used in stem cell-derived products to assess their identity and serve as a point for quality control.
An iPSC model is only as good as the differentiation protocol used. A variety of VSMC differentiation protocols exist and we have summarized those protocols that have been used in aortic disease modeling in Table 2; general VSMC differentiation protocols have been reviewed thoroughly by others (Ayoubi et al., 2017). When choosing a protocol to model aortic disease, there are a few parameters to consider. First, the length and nature of the protocol – older methods describe embryoid body (EB) differentiations, where aggregated stem cells spontaneously differentiate into the three germ layers, recapitulating events during development (Itskovitz-Eldor et al., 2000). From this point, VSMC fate can be induced. Differentiation through EBs requires precise control of cell aggregates, in respect of both size and homogeneity, as these can influence differentiation and yield (Messana et al., 2008), potentially due to cytokines and small molecules exerting their effects mainly on the surface layers (Sachlos and Auguste, 2008). Cell sorting by FACS could circumvent this issue, however, there are considerations for time and cell viability following sorting. Although methods have been developed to reduce variation in EB size and density, including the use of microwells, and micropatterned scaffolds (Bauwens et al., 2008; Mohr et al., 2010), the field has largely moved away from EBs to monolayer methods (Cheung et al., 2012; Mummery et al., 2012; Patsch et al., 2015; Palakkan et al., 2017). Generally, pluripotent stem cells grown as monolayer colonies are first directed toward a specific embryonic pathway, and then differentiated into VSMCs. This allows for more uniform delivery of factors guiding differentiation, as there are fewer considerations for factor diffusion and availability (Suchorska et al., 2017). Monolayer methods are also more amenable to large-scale production, due to their relative homogeneity compared to EBs, and do not necessarily require any cell sorting.
Another important consideration would be the presence of appropriate VSMC markers, indicative of maturation and contractility. With the possible exception of SM-MHC and smoothelin (SMTN), most VSMC markers can be expressed in other cell types under certain conditions (Alexander and Owens, 2012). Therefore, if the aim is to obtain relatively mature and contractile VSMCs, staining or flow cytometry of SM-MHC and/or smoothelin would be more appropriate ways of monitoring differentiation quality, rather than a less selective marker such as α-SMA. If opting for SM-MHC antibody staining, however, we caution readers to carefully assess the data – cross-reactivity of smooth muscle and non-muscle myosin heavy chains by polyclonal antibodies can confound interpretation of results and can lead to over-estimation of SM-MHC content (Rovner et al., 1986). It should be noted that in vitro differentiated cells can easily lose SM-MHC and smoothelin expression when exposed to serum (Alexander and Owens, 2012), so quality control to identify these VSMC markers should be performed prior to culture in serum. Furthermore, some patient-derived lines of familial TAADs may have mutations in VSMC contractile genes such as MYH11 or ACTA2, so appropriate control lines, such as CRISPR-corrected isogenic lines, should be used in parallel in order to assess the quality of differentiations. In addition to marker expression, functional assays should also be performed. Identifying a protocol where the cells show VSMC-like responses, with rapid contraction, to vasoactive agonists such as carbachol would also be important to ensure that the correct cell type, or good differentiation, has been achieved. VSMC contraction should be noticeable on the scale of a few minutes, rather than hours (Table 2).
We appreciate that certain mutations will alter the expression of markers and function of resulting VSMCs. Care should be taken when establishing new disease models or lines to distinguish poor quality differentiations from genuine in vitro disease phenotypes. This may be particularly relevant in diseases or patient lines with mutations in genes affecting VSMC function, including the aforementioned MYH11 or ACTA2 mutations. In these cases, in addition to using gold standard isogenic controls, we strongly recommend careful and stringent quality control of the VSMC progenitor. This will reduce variability in the resulting VSMCs, and result in more consistent assessment of the disease phenotype.
Generating and analyzing contractile VSMCs is of crucial importance in recapitulating disease phenotypes. The importance of iPSC-derived maturity can be inferred firstly from the fact that TAADs are generally post-natal diseases rather than developmental. Moreover, with diseases related to VSMC de-differentiation, such as SVAS, restoration of full VSMC function and maturity in vitro would be an essential parameter of success for any new therapeutic. The inability of a differentiation protocol to yield mature VSMCs in control lines is likely to fatally compromise drug screening or testing with that protocol. In our experience, in addition to the specific protocol used, the contractile ability can be affected by user-dependent factors such as the seeding density during or after differentiation; these are important considerations as they contribute significantly to variation between differentiations, as will be highlighted below.
Vascular smooth muscle cell differentiation protocols can be further refined to improve the yield of contractile cells. In addition to reduction or replacement of serum in the maturation steps, small molecules can be introduced to improve yield of contractile VSMCs. Recently, a novel screening method was reported, where an MYH11 reporter ESC line was used to screen over 4,000 compounds that may improve SM-MHC expression (Zhang et al., 2019). This screen identified RepSox, a modulator of Notch signaling, as improving VSMC contractility in differentiations using PDGF-BB and TGF-β. In addition to improvement in initial levels of SM-MHC, cells treated with RepSox also maintained high levels of SM-MHC for at least 8 weeks after derivation, suggesting that this may be a new and interesting direction for VSMC differentiation protocols.
The VSMCs comprising the aorta are derived from distinct embryonic lineages: the descending aorta is derived from paraxial mesoderm (PM), the ascending aorta and aortic arch from NC and the aortic root from lateral plate mesoderm (LM; Jiang et al., 2000; Wasteson et al., 2008; Harmon and Nakano, 2013; Figure 2). These different aortic regions seem to have distinct susceptibility to aortic diseases, including genetically-triggered aortopathies, suggesting that in addition to haemodynamics and wall structure, the embryonic lineage of the VSMC may be an important determinant for disease development and progression, [reviewed by (Majesky, 2007)]. In addition, the nature of the border between VSMCs of different lineages could be an important consideration; while there is a distinct boundary at the aortic isthmus between the PM- and NC-derived VSMCs (Nakamura et al., 2006), the transition between LM- and NC-VSMCs in the aortic root is not as well defined. Lineage-tracing experiments in mice have shown that there is a significant area of overlap between these lineages at the base of the aorta (Harmon and Nakano, 2013; Sawada et al., 2017). Indeed, it has been suggested that the differential response to cytokines and/or ECM composition between these overlapping or adjacent VSMC populations underpins the origins of aortic aneurysm and dissection (Topouzis and Majesky, 1996; Cheung et al., 2012), an hypothesis supported by recent work in mice (Angelov et al., 2017; MacFarlane et al., 2019). Tgfbr2 deletion in VSMCs led to the development of thoracic aortic aneurysms, whereas treatment with a TGF-β neutralizing antibody resulted in abdominal aortic aneurysms (Angelov et al., 2017). Lineage tracking and sorting in a Loeys-Dietz mouse model showed a differential response of LM- and NC-derived VSMCs to TGF-β (MacFarlane et al., 2019).
Figure 2. The different regions of the thoracic aorta and their disease susceptibilities. The descending aorta comprises VSMCs from paraxial mesoderm, the aortic arch from neural crest, and the aortic root from lateral plate mesoderm. The boundary between the arch and descending aorta is clearly defined, whereas there is overlap between the VSMCs from NC and LM at the aortic root, as denoted by the dotted lines.
As a result, protocols describing the derivation of VSMCs corresponding to the different regions of the aorta may be important to consider in order to accurately reflect the disease (Cheung et al., 2012; Patsch et al., 2015; Jiao et al., 2016; Gong et al., 2020). Our in vitro model of MFS showed differences in fibrillin-1 deposition and disease severity in VSMCs depending on embryonic origin, highlighting the importance of studying specific cohorts of VSMCs when modeling a “disease-in-a-dish” (Granata et al., 2017). A model of BAV has also demonstrated that NC-VSMCs, but not PM-VSMCs, from patients with BAV and TAA have defects in differentiation and contractile function (Jiao et al., 2016). Currently published iPSC models for SVAS, however, did not use lineage-specific protocols in their investigation (Ge et al., 2012; Kinnear et al., 2013, 2020). In a 3D model of SVAS, a lineage-specific protocol also was not used, although the investigators inferred lineage based on responsiveness to cytokines (Dash et al., 2016). Finally, in a recent iPSC-based model of LDS, NC-, and LM-VSMCs exhibited distinct defects relating to contractile marker expression and response to TGF-β depending on lineage (Gong et al., 2020), mirroring the in vivo findings (MacFarlane et al., 2019). These studies collectively echo the importance of using lineage-specific protocols wherever possible when modeling aortic disease.
VSMC contractility in response to agonists is an important indicator of maturity and this can be assayed in 2D and 3D systems. Contraction of VSMCs can be examined upon exposure to either ionophore compounds such as potassium chloride, ionomycin, or carbachol or peptide hormones such as angiotensin II. Angiotensin II activates AT1R, stimulating a cascade of G-coupled protein signaling or tyrosine phosphorylation triggering MAPK signaling followed by intracellular calcium release, which leads to contraction (Griendling et al., 1997; Touyz and Schiffrin, 1997). The extent of contraction can be investigated by comparing cell surface area before and after agonist stimulation, or more sophisticated methods involving live-imaging and/or force measurements can be employed (Gaio et al., 2016; Halaidych et al., 2019; van Meer et al., 2019). Importantly, routine examination of contractile response should be assayed in iPSC-derived VSMCs to ensure the consistency of differentiations.
In addition to being a functional benchmark, contraction has the ability to drive maturation. VSMCs contract to counterbalance hemodynamic forces as well as circumferential strain in blood vessels and, in response to these, maintain blood flow and pressure (Zulliger et al., 2004; Alexander and Owens, 2012; Ahmadzadeh et al., 2019). Pulsatile stretch is interpreted by cells through intracellular signaling pathways leading to changes in proliferation, contraction, apoptosis, migration, and ECM remodeling (Haga et al., 2007). VSMC contraction does not only define the maturity of these cells, but the application of uniaxial mechanical forces using stretching platforms can itself induce functional differentiation of the nascent iPSC-derived VSMCs. Cyclic stretch is applied to VSMCs seeded on ECM-coated elastomer-bottomed culture plates and, over 6 to 48 h, the VSMCs align themselves based on the strain cues (Mantella et al., 2015). Stretched VSMCs have synchronized contraction and increased myocardin expression, indicative of enhanced contractility (Zhu et al., 2011; Raphel et al., 2012; Chiu et al., 2013; Qiu et al., 2013). It should be noted that uniaxial stretch promotes VSMC differentiation whereas equiaxial stretch has the opposite effect (Park et al., 2004) therefore the choice of method needs careful consideration.
Another mode of enhancing contractility is by the use of pulsatile flow, which has proved to be effective in improving both VSMC alignment and contractility in 2D as well as 3D culture systems (Shi and Tarbell, 2011). Cyclic stretch aided alignment of VSMCs and deposition of elastin as well as other ECM components such as collagen, which in turn enhanced tensile strength and elasticity of scaffolds, vascular rings and tissue engineered blood vessels (TEBVs) made of VSMCs and ECs (Solan et al., 2009; Cooper et al., 2014). Here, the stretched constructs demonstrated higher burst strength and elasticity compared to non-stretched counterparts, making them both more amenable for in vivo transplant and a more accurate disease model in vitro.
These simple 3D models are amenable to contractility assays and can supplement standard 2D in vitro systems. In addition, they offer the possibility to test VSMC interactions with other cell types such as ECs and fibroblasts (Jung et al., 2015; Ding-Yang et al., 2019). VSMCs embedded in collagen or Matrigel have been shown to reorganize and remodel their environment to more closely mimic in vivo ECM architecture (Song et al., 2001; van den Akker et al., 2012). Contraction can also be assayed in these 3D systems, which more closely resemble native blood vessels than 2D cultures. The collagen gel contraction assay is a typical one to assess functionality of VSMCs by measuring the reduction in gel area and has been applied to both primary and stem cell-derived VSMCs (Oishi et al., 2000; Sinha et al., 2006; van den Akker et al., 2012; Lee et al., 2019). Newer models employ the use of bioreactors for scale-up, and 3D hydrogel disks are prepared by mixing multiple cell types like VSMCs and ECs with collagen, and contraction assayed over 30 min to 1 h (Lin et al., 2019). Vascular rings, a 3D structure comprising VSMCs, can be created relatively quickly and changes in circumference or force generation can be assayed in response to contractile agonists (Bi et al., 2005; Dash et al., 2016). Dash and colleagues have successfully created rings using iPSC-derived VSMCs to create a preliminary 3D model of SVAS. Here, the vascular rings created from patient VSMCs exhibited reduced contractility, which was a similar finding to previously published 2D models of SVAS with the strength of analyzing collective force generation and contraction versus single cells in a monolayer (Ge et al., 2012; Kinnear et al., 2013).
An important consideration for aortic disease modeling is that the full of extent of VSMC dysfunction may not be evident in unstretched or unstimulated circumstances. For example, there may be defects in contraction or contractile responses which are critical for the disease phenotype which are not otherwise apparent. Subjecting cells to mechanical forces would emulate the in vivo strain, as well as triggering associated signaling pathways, such as the generation of physiological reactive oxygen species (Clempus and Griendling, 2006). This was recently highlighted by a study in vEDS mouse models, where the differences in collagen organization were only apparent after stretching (Dubacher et al., 2020). When developing new therapies for aortic disease, it is essential to ensure that the disease effect on VSMC contraction and mechanotransduction are sufficiently evaluated.
With the advances in tools for gene editing, the use of isogenic controls is now the gold-standard in iPSC modeling. Many stem cell banks have catalogs of extensively-characterized healthy iPSC lines and these can be used as controls compared to patient lines. However, diseases such as MFS have high inter- and intra-familial variability – the same mutation in FBN1 can result in varied disease presentations (Dietz et al., 1992). Consequently, gene editing, to provide a “corrected” wild-type version of the disease line, offers the significant advantage of an isogenic control line that has the same genetic background as the disease model but differs only by the few nucleotides that constitute the mutation. Although this approach is widely-used in many fields (Bassett, 2017), current aortic disease models mainly rely on healthy iPSC lines as controls (Table 1).
Of course, the gene editing tools used to correct a mutation can easily be used to create a mutation in an otherwise healthy control iPSC line. Several groups have used this approach to generate disease models without needing patient involvement (Paquet et al., 2016; Tidball et al., 2017; Frederiksen et al., 2019), including a recent model for LDS (Gong et al., 2020). Despite the obvious practical advantages of this strategy, we should sound a note of caution. If there is any variable expressivity of the mutation, then a permissive genetic background may be required for full disease manifestation in vitro, which unlike lines from patients with disease, is uncertain in healthy control iPSC lines. We predict that creating a patient mutation in a healthy line will not necessarily yield the same extent of cellular defects as the patient line. This will be particularly important for multi-variant disorders, but also when modeling disease from patients with milder clinical manifestations. In the case of the monogenic aortic diseases discussed here, genetic background likely plays an important role in influencing disease severity and presentation, as will be discussed later. In practical terms, in order to construct an accurate “disease-in-a-dish,” we recommend the use of patient lines and genetically-engineered isogenic controls as the gold standard. Alternatively, wherever possible, iPSCs from unaffected family members could also be used as controls, which partly mitigates the differences in genetic backgrounds. In a model of Hutchison-Gilford progeria (HGP), researchers obtained unaffected parental fibroblasts in addition to patient lines from the Coriell Institute cell bank (Zhang et al., 2011); unfortunately, in this case, parents and patients were from different families.
Many differentiation protocols exist for producing VSMCs and the choice of protocol can have important effects on the quality of disease modeling. This may be particularly pertinent in modeling aortic disease, as three lineages of VSMC are present in the aorta and may be involved with disease susceptibility, but also because aortopathies may result from improper VSMC function, such as abnormal proliferation and contractility. Once differentiated, iPSC-derived VSMCs provide a flexible system to address aspects of a disease – simple cell-based assays such as the assessment of proteolytic activity, proliferation, contractility, and response to mechanical stimuli can provide mechanistic insight. Lastly, gene editing tools allow researchers to create virtually any genetic modification in their patient-derived or healthy lines, creating opportunities to untangle issues such as the genotype-phenotype correlation in TAADs. Despite these advantages, there are a number of issues to be aware of which we will discuss next.
Cell maturity is a major consideration with iPSC-based modeling of aortic disease. Current iPSC differentiation protocols almost invariably result in cells which are closer to fetal VSMCs than to adult cells, as has been demonstrated in other fields (Mummery et al., 2012; Lundy et al., 2013; Hrvatin et al., 2014; Baxter et al., 2015). While this immaturity has been best characterized in cardiomyocyte and hepatic differentiation, a similar problem is likely to exist in VSMC differentiation; although the exact developmental stage, perhaps due to intrinsic VSMC plasticity (Alexander and Owens, 2012), is poorly characterized in most VSMC studies. Nevertheless, low levels of SM-MHC and smoothelin expression confirm that these iPSC-VSMCs are most likely to represent a fetal-like state. While this may be advantageous for developmental studies and disorders, caution is warranted for adult disease modeling and the potential drawbacks have been discussed earlier. It is possible to improve the maturity of the in vitro derived VSMCs using a range of strategies including EC co-culture (Collado et al., 2017), application of mechanical force (Park et al., 2004; Ghazanfari et al., 2009), small molecules or other growth factors such as TGF-β and retinoic acid (Martin et al., 2004; Yu et al., 2011; Wanjare et al., 2013; Zhang et al., 2019). Differentiation protocols continue to be refined, and protocols describing the derivation or indeed forward programming of adult-like VSMCs are eagerly awaited.
Vascular smooth muscle cells grown in 2D monoculture provide a reductive snapshot of the disease. VSMCs in the aorta are normally in contact with adventitial fibroblasts, other VSMCs in the medial lamellae and ECs lining the lumen. ECs are also closely-associated with microfibrils via integrins, and like VSMCs can also secrete fibrillin-1, although the extent and functional significance of this has not been extensively characterized (Weber et al., 2002; Rossi et al., 2010). Intimal ECs experience direct shear stress and can modulate the function of VSMCs by releasing vasoconstrictors or relaxants (Lilly, 2014). Paracrine signaling and physical interactions between ECs and VSMCs are essential for vessel development and homeostasis of mature vessels, regulating tone, blood pressure, and response to injury (Lilly, 2014). For example, endothelial signaling of TGF-β and Notch regulates VSMC phenotype and differentiation (Domenga et al., 2004; Jakobsson and van Meeteren, 2013). VSMC monoculture therefore neglects these potentially important cellular interactions, limiting the information available from such systems.
While the majority of studies investigating aortopathies focus on VSMCs, abnormalities in EC function have also been reported. NO is produced from ECs and regulates vascular tone by inhibiting VSMC contraction. MFS thoracic aortas showed differential relaxation curves in response to endothelial NO compared to wild-type controls, whereas the response in the abdominal aorta was similar for MFS or control (Chung et al., 2007). A mouse model of TAAs found that NO is implicated in TAA disease progression, where various models of TAA, including MFS, had improved aortic phenotypes when treated with NO synthase inhibitor L-NAME (Oller et al., 2017). Recently, cell-specific deletion of the AGTR1 was investigated in a severe model of MFS (Galatioto et al., 2018). The authors found that while there was no effect with VSMC-specific deletion of AGTR1 on disease end-points, specific ablation in ECs improved survival and decreased aortic diameter. This study highlighted that there are differential responses of ECs and VSMCs to cytokines and growth factors. This characteristic could be an important consideration for in vitro drug screens and discovery; once an interesting target has been identified, the response of ECs should also be studied prior to validation in vivo, as ECs clearly impact the disease mechanism in MFS, and likely other TAADs. This can be done in a variety of ways – ECs and VSMCs can be assessed independently or in 2D co-culture, which provides a simple way of studying both cell types together (Fillinger et al., 1997; Hastings et al., 2007). After co-culture, ECs can be purified using magnetic beads coated with anti-CD31 allowing separate downstream analysis of ECs and VSMCs (Wallace et al., 2007).
Hemodynamic forces within the blood vessel influence VSMC phenotype and function. VSMCs are not normally exposed to luminal blood flow, but instead experience low transmural interstitial flow, with cells closer to the intima experiencing greater force (Shi and Tarbell, 2011). In vitro, flow was found to increase VSMC contraction (Civelek et al., 2002), and induces alignment of cells perpendicular to the direction of flow (Lee et al., 2002). Studies using VSMCs alone have conflicting reports on the effect of flow on VSMC phenotype (Papadaki et al., 1996; Ueba et al., 1997; Haga et al., 2007; Shi et al., 2010), possibly due to varied forces and culture conditions. However, when VSMCs and ECs are co-cultured with shear stress, VSMC phenotype was found to be more contractile and with gene expression signatures closer to that of primary cells (Tsai et al., 2009; Collado et al., 2017).
The power of a 3D approach in HGP has been illustrated by the use of TEBVs generated from patient-derived iPSCs (Atchison et al., 2017; Abutaleb and Truskey, 2020). These TEBVs recapitulated the disease phenotypes and helped to elucidate the role of both VSMCs and ECs in disease progression. Both vasoconstriction and dilation were affected and increased medial wall thickness, calcification and apoptosis were observed. Furthermore, this 3D model was used for drug testing, where they demonstrated that the rapamycin analog everolimus increased vasoreactivity and improved VSMC differentiation. Further refinement of this model using both iPSC-derived ECs and VSMCs demonstrated that ECs are likely responsible for the abnormal response to shear stress (Atchison et al., 2020). Together, these studies highlight the importance of contributions of ECs and shear stress to VSMC biology.
When investigating aortopathies, co-culture and/or 3D approaches could be considered. While these methods provide the possibility of analyzing cells in a more native-like state, they are also more complicated, time-consuming to set up and require careful construction. A blood vessel wall contains multiple cell types, with distinct interactions being critical for their proper function. Hence, consideration of the relative ratios of VSMC, ECs and fibroblasts is required, as these can impact a number of properties including ECM deposition and modulation of VSMC phenotype (Lilly, 2014; Kuwabara and Tallquist, 2017). The arrangement and orientation of these cell types should also be considered, such that the natural hierarchy of cells forming the vasculature is respected. Inappropriate integration of these cell types could be detrimental for building an accurate disease model, obscuring critical differences between control and disease models. Finally, as we’ll discuss below, generating large amounts of iPSC-derived VSMCs can by itself be a laborious and time-consuming task; additional differentiations to ECs or set-up to create 3D systems could be difficult to accommodate in large scale.
Despite efforts to improve fidelity of iPSC-based models, the same pitfalls for any in vitro model remain. They lack key features provided by in vivo models, including involvement of the immune system and integration of complex physiological networks. We would like to emphasize that these iPSC models do not replace in vivo studies; instead, they complement and can accelerate the study of disease by providing a flexible platform for testing and screening. We therefore propose that with the current limitations, simple VSMC-based assays and screens in 2D could identify interesting mechanisms and targets, which can then be tested in a more complex, in vitro system before transitioning to in vivo models.
Hurdles facing iPSC-based disease modeling include difficulties in scaling up production of cells and variability between differentiations. There are physical limitations to manually culturing multiple lines of iPSCs and producing large amounts of cells. Currently, aortic disease modeling is done with a handful of patient lines and controls, with assays which don’t typically require large amounts of cells (Table 1). However, for modeling diseases using 3D methods, such as TEBVs or vascular rings, many millions of cells will be required. While we discussed the ability to create virtually any mutation in the lines, the sheer number of hours and hands required to culture many different cell lines could be inhibitory, let alone deriving large quantities from each line. VSMC-derivation protocols are currently multi-step procedures, which go through an intermediate or a VSMC precursor. In addition, protocols can also include a maturation step, where cells are cultured for up to a month to accrue their phenotype. As a result, when employing such protocols, a single line will yield four distinct cell-types to monitor and manage: iPSC, intermediate/precursor, immature VSMC and mature VSMC. In our experience, given the tiered nature of the VMSC differentiation protocols, creating good intermediates is essential to producing reliable and mature VSMCs, and their maintenance should not be neglected. The length of these protocols also means that there is more opportunity for variability in differentiations. Another complication is that different iPSC lines can also behave very differently, even among control or healthy iPSC lines; skill and experience are needed to ensure that all lines are appropriately handled during differentiation in order to reduce noise from interline variability. For example, a disease model line could have abnormal proliferation and the researcher must take this into account when deciding when to passage them.
How consistently can iPSCs be differentiated by the investigator, their colleagues or even other labs using the same protocol? Considerable variation in differentiations has been reported in various fields; for example, a multi-site analysis found substantial heterogeneity in neuronal differentiations between sites using the same lines and protocol (Volpato et al., 2018). Even within research groups, variation between lines and differentiations were observed for both EB and monolayer differentiations (Osafune et al., 2008; Hu et al., 2010). When studying the 9p21 vascular risk variant, multiple iPSC lines from the same patient or even the same line differentiated multiple times exhibited considerable transcriptional variability at both iPSC and VSMC stages (Lo Sardo et al., 2018). These findings underline the concern with regard to reproducibility of data. We certainly observe differences in VSMC differentiation between individuals in our group, stressing the influence the investigator has on the final outcome. Other researchers have also observed different levels of SM-MHC+ cells using the same protocol or have had to modify the protocol to obtain sufficient maturity in their hands (Cheung et al., 2012; He et al., 2018; Trillhaase et al., 2018; Zhang et al., 2019). These differences could be due to the use of different iPSC lines, but are likely also impacted by variation imparted by the user. Current iPSC models of aortic disease are focused on severe models of disease. However, when modeling the effects of a milder mutation or variant, the effect of genotype may not be observed if the differentiations themselves are highly variable.
A common issue we’d like to highlight for many differentiation protocols is the use of non-chemically-defined media and coatings, such as serum or Matrigel, and the reliance on cytokines where different batches of these reagents may have varying effects on differentiated cells. Currently in disease modeling, serum is used to stimulate growth of VSMCs in various protocols after differentiation (Table 2), and high levels of serum are known to result in loss of contractile phenotype (Alexander and Owens, 2012). Aside from the use of Matrigel, a near chemically-defined protocol to generate VSMCs has been developed (Patsch et al., 2015) and modified protocols have recently been used to model HGP (Atchison et al., 2020) and LDS (Gong et al., 2020). In addition, many VSMC protocols rely on growth factors, such as TGF-β and PDGF-BB for differentiation. While these protocols do work, investigators should be wary of the numerous factors which may influence the efficacy of these cytokines, such as storage method and batch-to-batch variation. In the cardiac field, a protocol using entirely chemically-defined media to produce cardiomyocytes was developed by systematically assessing the necessity of individual factors (Burridge et al., 2014). Interestingly, they found that only three components were crucial for cardiomyocyte differentiation. This protocol resulted in improved consistency of differentiations in the 11 iPSC lines that were tested. In addition to ease and consistency, this approach could also enable researchers to scale-up production more than is possible using cytokine and xeno-containing formulations. Similar advances have been made in other fields (Erceg et al., 2008; Touboul et al., 2010) and would be beneficial in advancing aortic disease modeling.
It goes without saying that new protocols have to be carefully assessed and compared with tissue or primary cells to ensure that that the stem cell-derived product has the correct identity. With advances in the past decade, decreasing price and availability of large-scale experiments (Hasin et al., 2017), detailed comparisons can be performed to assess the quality and consistency of differentiation protocols. This was an approach demonstrated by Patsch et al. (2015), where they showed high correlation between their differentiated and primary VSMCs using both transcriptomics and metabolomics. In addition, high-throughput “omics” can be used to assess the consistency of differentiations (Paull et al., 2015), and single-cell RNA sequencing has been used to identify pivotal steps in differentiation protocols (Chu et al., 2016; Han et al., 2018). We predict that future iterations of protocols will utilize these tools to help direct and objectively assess the quality of differentiation protocols.
Alternative approaches, such as direct reprogramming and forward reprogramming, may circumvent the imperfect approximations of developmental pathways used for typical differentiation protocols, and reduce the number of intermediates required (Figure 3A). Work on direct reprogramming has been shown in various fields (Kelaini et al., 2014), including the derivation of cardiomyocytes from fibroblasts (Ieda et al., 2010). Forward reprogramming has been demonstrated to rapidly convert hESCs into neurons, skeletal myocytes, and oligodendrocytes by overexpressing key lineage-specific transcription factors (Pawlowski et al., 2017). These approaches in VSMCs have only recently been reported, and warrant further investigation (Yeung et al., 2017; Hirai et al., 2018). In addition, it may be challenging to produce the significant region-specific VSMCs using these strategies with our current limited understanding of the fundamental differences between VSMC from varying embryonic origins.
Figure 3. Approaches to improving homogeneity of VSMC differentiations by using (A) direct or forward reprogramming methods, (B) automation, or (C) improved quality control and simplification of media components.
Until differentiation methods are refined, steps can be taken to improve reliability of current protocols with clearly-defined parameters for quality control at various stages. For example, stringent quality control should be performed after the derivation of an intermediate state before inducing cells toward a VSMC fate (Cheung et al., 2014); if the cells fail to meet the set criteria, they should not be used for further differentiation (Figure 3C). In addition, when VSMCs are produced, analysis of markers and/or function should be routinely assessed. These criteria should ideally be shared with collaborators in order to reduce the site-specific variability as described by Volpato et al. (2018). Furthermore, identification of novel surface markers exclusively expressed on contractile and mature VSMCs could be used for cell sorting and/or quality control. Lastly, wherever possible, the use of multiple iPSC clones from the same patient could also improve the signal-noise ratio, as different clones can themselves be highly variable (Lo Sardo et al., 2018; Popp et al., 2018).
Automated systems and machine learning could significantly reduce the input needed from the researcher when culturing multiple lines, improving consistency and enabling increased production. Automated iPSC culture systems have been developed and would present a solution to the workload and variability problems (Conway et al., 2015; Paull et al., 2015; Figure 3B). The method developed by Paull and colleagues describes the capacity to reprogram, expand and characterize hundreds of lines per month with significant reductions in reagent cost. In addition, transcriptomics analysis indicated that there was a significant reduction in variability in EB assays when compared to manual processing. This system was put to the test when iPSCs were differentiated into dopaminergic neurons using a 30-day protocol and the resulting cells maintained expected marker expression. This automated system was utilized by another group for cardiomyocyte differentiation, and found success with producing a maximum of 3 × 109 cardiomyocytes per batch (Denning et al., 2016). A recent method describing high-yield derivation of VSMCs based on an existing protocol (Patsch et al., 2015) was described, where VSMCs were derived in alginate hydrogel tubes (Lin et al., 2019). This method yielded 5 × 108 cells/ml in 10 days; as a result, bioengineering methods could rely on such advances for producing high numbers of cells.
The behavior of some patient lines with certain mutations can be tremendously divergent compared to control lines, requiring careful assessment from an experienced researcher to consider not only cell density, but also morphology, heterogeneity and survival. In our experience working with MFS patient iPSC lines, when deriving NC-VSMCs, the cells steadily exhibit more of the disease phenotype throughout the course of differentiation. They require much closer monitoring and the resulting differentiations can be more heterogeneous compared to controls, due to varied cell density caused by increased apoptosis and slower proliferation. Innovations in robotics and machine learning could overcome these bottlenecks. For example, machine learning has been developed to identify cells in phase contrast based on morphology alone without the need for molecular labeling (Kusumoto and Yuasa, 2019). This technology, in conjunction with modular automated systems, could be powerful for processing large numbers of iPSC lines, including cells derived from severely affected lines, as it could potentially remove the need for an experienced “eye” when culturing cells. However, at the moment, the protocols and technologies are not yet compatible with one another for robust, automated systems; the labor-intensive manual culture and differentiation of iPSC lines into VSMCs are current limitations for large-scale studies.
Induced pluripotent stem cell-based modeling of aortic disease is still relatively new, with only a handful of papers describing disease models (Table 1). Despite the practical advantages of using this system, there are limitations. Most notably, the cells obtained from differentiation are not as mature as VSMCs in tissue due in part to absent mechanical cues, lack of contact with ECs and other physiological signals. In addition, without appropriate quality control, variability between differentiations can result in noisy and inconsistent data. Large-scale experiments involving multiple lines are difficult to perform as manual passaging and differentiation is required as a result of the complexity of certain protocols. In spite of this, we are certain that continued refinement of differentiation protocols and technological advances will be able to overcome these limitations to create valuable tools for understanding, preventing and treating aortopathies.
The first engineered blood vessel was a relatively simple construct made from collagen and primary bovine VSMCs, which was then lined or coated with primary ECs and adventitial fibroblasts, respectively (Weinberg and Bell, 1986). Since then, efforts have been made to produce clinically-relevant TEBVs with the required mechanical specifications, as reviewed by (Kumar et al., 2011). Recently, tissue engineered vascular grafts (TEVGs; Carrabba and Madeddu, 2018; Song et al., 2018) and vasculature-on-a-chip (Kim et al., 2017) models have been developed to accommodate the gold standard properties of a transplantable graft using either self-assembling bioprinting technology or using natural or synthetic scaffolding (Konig et al., 2009; Wise et al., 2011). These models have the properties of a successful graft, such as an autologous endothelium, anti-thrombogenic properties and minimum integrity span of 21 months, with appropriate permeability, compliance, elastic modulus, and a minimum burst pressure of 1700 mmHg (Konig et al., 2009).
The use of TEVGs in regenerative medicine is still under development, with many groups innovating with novel ways to tackle the problems facing engineered grafts. For example, grafts comprising decellularized ECM on biodegradable scaffolds have been suggested to serve as readily available TEVGs; these have been tested in a variety of animals models (Dahl et al., 2011) and can exploit recent advances in 3D tissue printing to provide patient-specific grafts (Fukunishi et al., 2017; Best et al., 2018). Cell-free vessel grafts have been generated by allowing cells to secrete ECM for longer periods to more closely mimic the in vivo environment and are then decellularized (Lawson et al., 2016; Row et al., 2017). Furthermore, functionalization of TEVGs with biological signals such as the angiogenic cytokine VEGF have been shown to trigger in situ tissue endothelial regeneration (Koobatian et al., 2016). Although advances in traditional translational approaches for cardiac anomalies have paved the way for regenerative medicine, these TEVGs still suffer from a number of common issues including insufficient patency, integration, hemodynamics, immune-compatibility with the graft cell source and mechanical strength, as outlined by others (Pashneh-Tala et al., 2016; Matsuzaki et al., 2019; Skovrind et al., 2019).
Currently, if a TAAD patient’s aorta dilatates sufficiently, prophylactic surgical intervention is required. iPSC-based systems raise the possibility of developing regenerative cell therapies for patients with aortic disease, where TEVGs can be produced from patient iPSCs. In addition, the availability of gene editing tools means that the TAAD-causing mutation(s) can be corrected in a patient’s iPSCs. These, in turn, could be differentiated into VSMCs and developed into a healthy TEBV, to be used as an autologous bio-compatible graft. Furthermore, patient-derived iPSCs would provide immune-compatible grafts. These would be particularly useful in pediatric patients where cardiovascular grafts would ideally grow in line with the patient’s normal growth and development (Sugiura et al., 2018). To our knowledge, there have been limited applications of iPSC-based TEVGs, let alone in the context of aortic disease. In one case at least, TEVGs demonstrated mechanical strength comparable to that of native veins; when implanted in rats, they showed sustained mechanical function and patency (Sundaram et al., 2014; Luo et al., 2020). While the application of iPSC-derived VSMCs in regenerative medicine for the treatment of aortic disease is attractive, we would like to caution that this represents a very labor-intensive task. We discussed earlier the current difficulties in obtaining large numbers of consistently-differentiated VSMCs. In addition, the approaches highlighted above would need to be tailored to each individual patient. In our experience, establishing and characterizing a new iPSC line can take weeks before differentiations can be started, which can themselves take up to a month before TEBV construction can begin. The timeline grows even longer if gene editing also has to be involved. As an alternative, haplotype matched/allogenic iPSCs, MSCs or ESCs could be used providing the advantage of well-defined VSMC differentiation protocols but without needing to develop individual lines and grafts specifically for each patient (Sundaram et al., 2014; Gui et al., 2016; Elliott et al., 2019; Luo et al., 2020). These can be prepared in a variety of formats, including printed, electrospun, or decellularized scaffold grafts. This approach could be developed even further by the use of lineage-specific protocols to create the closest approximation possible of on-demand TEVGs, catering to different matrix compositions.
Aortopathies have profound effects on the life quality of affected patients; not being able to know what the severity of the disease is can be an enormous burden. This is complicated by the lack of understanding of genotype-phenotype correlation in many TAADs – even within families, disease severity can vary significantly. This is even more difficult in sporadic cases, where there is no family history to infer prognosis from. The best solution at the moment is to monitor the patient’s aorta by cross-sectional imaging, administer anti-hypertensives and intervene with surgery if the dilatation exceeds a threshold. However, what if we were able to predict the patient’s disease severity and likely progression?
In MFS, there is high inter- and intra-familial variation in patients. FBN1 is a large gene, encoded by 65 exons, with over 3,000 mutations identified to date (Collod-Béroud et al., 2003). Aside from neonatal MFS, there may be some broad genotype-phenotype correlation with FBN1 mutations; in MFS, mutations in exons 24–32 or premature terminations are associated with a more severe disease outcome with cardiovascular complications (Faivre et al., 2007). Disease-causing mutations of FBN1 can be categorized as dominant-negative or haploinsufficient. In dominant-negative forms, the mutant product interferes with normal microfibril formation or is mis-incorporated. Various studies in patient fibroblasts have found abnormalities with reduced synthesis, delayed intracellular processing, and secretion (Aoyama et al., 1994; Schrijver et al., 1999; Whiteman and Handford, 2003). Haploinsufficiency is typically caused by mis-sense or frameshift mutations; analysis of patient fibroblasts found a reduction in the mRNA levels of mutant fibrillin-1, and a disproportionately low amount of fibrillin-1 deposition (Schrijver et al., 2002). Large studies have concluded that mutations causing haploinsufficiency of fibrillin-1 resulted in a 2.5-fold increase in the risk of cardiovascular death compared to dominant-negative mutations (Franken et al., 2016), and that mutations involving cysteines tend to also result in more severe clinical presentations (Aubart et al., 2018).
Although these broad associations may explain in part some of the variation in disease severity observed between patients with different mutations, it is unclear what factors contribute to variation within families or between patients with the same mutation in different families. Variation in genetic background clearly plays a key role in the different expression of disease. However, identifying clear associations between genotype and phenotype can be challenging for rare diseases due to the statistical power needed to identify gene modifiers in population genomics. MFS is the most common TAAD, with an incidence for 1 in 5000, whereas diseases such as LDS and vEDS are even rarer. A small study in patients with TAAs identified that variants in ADCK4 and COL15A1 were associated with mild disease (Landis et al., 2017). Recent studies have shown that integrating multiple methods can overcome limitations of studying rare disorders (Aubart et al., 2018). Whole-exome sequencing and association studies in a large cohort of 1070 patient fibroblasts has identified interesting mutations and variants accompanying a more severe presentation of MFS (Aubart et al., 2018). Severe cases of MFS were associated with co-occurrence of another TAAD-causing mutation, including additional variants of FBN1 or SMAD3. Interestingly, severe disease was also associated with mutations in COL4A1; variants of COL4A1 have been reported in stroke and cerebral aneurysms (Lanfranconi and Markus, 2010). Three major modifier regions were identified, corresponding to loci encoding ECE1, PRKG1 and MMPs.
iPSC-based modeling could help with severity prediction in two ways – first, by deepening our understanding of the genetic variants interacting with disease-causing mutations, and second, by potentially providing a platform with which to assess patient-specific disease severity. Whole-exome sequencing of a patient’s genome could give clinicians an initial idea of the expected disease severity, based on the risk variants present. These identified variants could then be introduced into various iPSC lines to further underpin their role in modulating disease. This can be done in a variety of patient lines, isogenic controls and also in healthy iPSC lines. This approach was used in an investigation of metabolic disorders, where variants previously discovered using genome-wide association studies were investigated using patient iPSCs (Warren et al., 2017). From patient iPSCs, simple cell-based assays can be employed to construct a prediction of clinical severity in the patient. In the case of TAADs, this could be looking at proteolytic activity, abnormal ECM deposition or cell death. Guidelines for determining in vitro disease severity can be developed through iterative empirical testing until these in vitro benchmarks are sufficiently refined and can be robustly linked to clinical severity. This predictive tool could then be used in conjunction with clinical benchmarks to provide a more informed prognosis. Together, these methods could be used to predict the course of the disease and guide treatment for patients.
Patient-derived VSMCs can be subjected to drug testing to identify compounds which ameliorate function. The ease of assays in 2D culture systems makes it feasible to use multi-well formats, test their response to various drugs and analyze a range of readouts, including VSMC contraction, proliferation, and secretome. For example, multiple iPSC lines from a hypertensive pharmacogenomics cohort were differentiated to functional VSMCs and their responses to contractile agonists and inflammatory cytokine TNF-α were analyzed (Biel et al., 2015). This work established robust high throughput assays for pharmacogenomics studies, paving the way for future studies which may incorporate the use of isogenic controls. A recent report of a model for SVAS has used an iPSC model to test the effect of different classes and combinations of drugs, finding that mTOR inhibitor everolimus was the most effective at rescuing the disease phenotype (Kinnear et al., 2020). Interestingly, they found that combination therapy using everolimus and additional classes of drugs was not beneficial. As emphasized earlier, interesting drug targets identified from large-scale screening can then be tested in a more complex and physiological set-up, possibly incorporating shear stress and co-culture systems to better mimic the aorta (Collado et al., 2017), preferably using lineage-specific cells where possible. Indeed, Atchison and colleagues have developed a 3D model of HGP from iPSC-derived VSMCs to test drug toxicology efficacy and dose response for various drugs (Atchison et al., 2017).
Thoracic aortic aneurysm and dissections are chronic and life-long conditions. Although establishing, characterizing, creating isogenic controls and finally differentiating new patient lines is a laborious task, drug testing and personalized medicine for diseases such as TAADs would be a worthwhile investment for the patient. With advances in automation, machine learning and refinement of existing protocols, we predict that this entire process of patient-specific drug screens and personalized medicine will be streamlined and simplified. Furthermore, developments in vascular 3D modeling to reduce costs, variability and intricacy may eventually allow for high-throughput drug screening in 3D. In addition to therapies and precision medicine, another way in which iPSC modeling could be beneficial would be to test for vascular toxicology. These sorts of studies have been performed in the cardiac field (Zhang et al., 2012; Florido et al., 2017; Sharma et al., 2017). Given that the cardiovascular complications of diseases such as MFS can be fatal, it may be worthwhile to undertake toxicology studies on additional drugs that could be detrimental to aortic health. For example, based on research focused on tendon rupture, the commonly used quinolone antibiotics are thought to cause connective tissue defects by upregulating MMP expression (Sendzik et al., 2010; Tsai et al., 2010). Their use in an animal model of TAAD (LeMaire et al., 2018) and susceptible patients (Daneman et al., 2015; Lee et al., 2015; Pasternak et al., 2018; Noman et al., 2019) is associated with a higher risk of complications and they are no longer recommended for patients with aortic disease. Both established and new drugs could be screened in iPSC models to identify those that pose risks to patients with aortopathies.
Induced pluripotent stem cell models can provide guidance for future clinical trials (Figure 4). In the case of the various losartan clinical trials, while some patients may have responded well to treatment with losartan, noise from non-responders would render such data non-significant despite the success in mice (Figure 4A). This may be due to the nature of mutation in FBN1, disease severity, genetic background, age of treatment or contribution from all of the above. Prior to a clinical trial, pre-screening patient-derived VSMCs to identify the pathways that are likely deregulated in the cohort, or conducting a preliminary trial in vitro before the full trial involving patients could be valuable (Figure 4B).
Figure 4. Currently, successful use of a drug in animal models is the prerequisite for use in clinical trials (A); this may lead to an amelioration in disease phenotype in some individuals, but not all. “Clinical-trials-in-a-dish” can be performed, where the effects of a combination of drugs at low doses is tested on patient-derived VSMCs, allowing us to target multiple de-regulated pathways (B). This combination therapy could then be validated in rodent models prior to use in clinical trials, and may have an effect in more patients (C).
A multiplicity of signaling abnormalities has been found in MFS. We and others have identified that other non-canonical TGF-β signaling pathways are altered in MFS, including ERK and p38 (Carta et al., 2009; Habashi et al., 2011; Granata et al., 2017; Sato et al., 2018), and it is well-established that patient disease severity ranges widely. Other groups have identified a role for NO signaling contributing to the disease (Chung et al., 2007; Oller et al., 2017). How do we reconcile the multiple signaling abnormalities seen in this condition with disease pathophysiology? We propose that multiple pathways may be deregulated downstream of a single FBN1 mutation and that these may also be deregulated to different extents. Using iPSC-derived VSMCs, “clinical-trials-in-a-dish” involving multiple drugs at tolerable, clinically-relevant concentrations can be employed before introducing the best combination in clinical trials (Figure 4C).
There is no doubt that iPSCs and the ability to generate human disease models offer a powerful new weapon in our armamentarium against thoracic aortic diseases. In this review we have presented the current state-of-the-art and highlighted how this technology is being used to tackle critical questions in the field. A key strength of iPSC-based disease modeling is its link to individual patients, which encapsulates genetic variants or mutations in the context of a disease-susceptible genetic background. Rapid developments in differentiation protocols, including the ability to generate lineage specific VSMCs, have facilitated robust in vitro models. Together with ease of genetic modification, these models allow us to increasingly clearly delineate pathological mechanisms and carry out drug screening to develop much-needed new therapies for aortic disease.
We have tried in this review to offer our personal insights into the details and nuances of establishing iPSC-based in vitro disease models of aortopathies. We have also highlighted the challenges and limitations of such an approach, such as limited cell types and lack of 3D structure and blood flow, where appropriate. Despite the challenges, we are excited by the scientific and therapeutic opportunities presented by these model systems and particularly for future developments such as deeper genotype–phenotype analyses, vascular toxicology studies, “clinical trials-in-a-dish,” and precision medicine – potentially enabling better tailoring of therapy to individuals.
HD, DS, and SS: writing, reviewing, and editing of manuscript. All authors contributed to the article and approved the submitted version.
This work was supported by BHF Program Grant RG/17/5/32936 (HD and DS) and BHF Senior Fellowship FS/18/46/33663 (SS).
The authors declare that the research was conducted in the absence of any commercial or financial relationships that could be construed as a potential conflict of interest.
We thank the Wellcome-MRC Cambridge Stem Cell Institute for core support. We thank Dr. Peter Holt for helpful comments. Figures were created using BioRender.com.
α-SMA, α-smooth muscle actin; AT1R, Angiotensin II receptor, type 1; BAV, Bicuspid aortic valves; EB, Embryoid body; EC, Endothelial cell; ECM, Extracellular matrix; ESC, Embryonic stem cell; FC, Flow cytometry; HGP, Hutchison-Gilford progeria; IF, Immunofluorescence; iPSC, Induced pluripotent stem cell; KSR, Knock-out serum replacement; LDS, Loeys-Dietz syndrome; LM, Lateral plate mesoderm; MFS, Marfan syndrome; MMP, Matrix metalloproteinase; NC, Neural crest; NO, Nitric oxide; NR, Not reported; PM, Paraxial mesoderm; SMGM, Smooth Muscle Growth Medium; SM-MHC, Smooth muscle myosin heavy chain; SPC, Sphingosylphosphorylcholine; SVAS, Supravalvular aortic stenosis; TAAD, Thoracic aortic aneurysm and dissection; TEBV, Tissue-engineered blood vessel; TEVG, Tissue-engineered vascular graft; TGF, Transforming growth factor; T β RII, TGF- β receptor II; vEDS, Vascular Ehlers-Danlos syndrome; VSMC, Vascular smooth muscle cell.
Abutaleb, N. O., and Truskey, G. A. (2020). Human iPSCs stretch to improve tissue-engineered vascular grafts. Cell Stem Cell 26, 136–137. doi: 10.1016/j.stem.2020.01.011
Ahmadzadeh, H., Rausch, M. K., and Humphrey, J. D. (2019). Modeling lamellar disruption within the aortic wall using a particle-based approach. Sci. Rep. 9:15320.
Alexander, M. R., and Owens, G. K. (2012). Epigenetic control of smooth muscle cell differentiation and phenotypic switching in vascular development and disease. Annu. Rev. Physiol. 74, 13–40. doi: 10.1146/annurev-physiol-012110-142315
Anagnostopoulos, C. E., Prabhakar, M. J. S., and Kittle, C. F. (1972). Aortic dissections and dissecting aneurysms. Am. J. Cardiol. 30, 263–273. doi: 10.1016/0002-9149(72)90070-7
Andreotti, L., Bussotti, A., Cammelli, D., di Giovine, F., Sampognaro, S., Sterrantino, G., et al. (1985). Aortic connective tissue in ageing—a biochemical study. Angiology 36, 872–879. doi: 10.1177/000331978503601206
Angelov, S. N., Hu, J. H., Wei, H., Airhart, N., Shi, M., and Dichek, D. A. (2017). TGF-β (transforming growth factor-β) signaling protects the thoracic and abdominal aorta from angiotensin II-induced pathology by distinct mechanisms. Arterioscler. Thromb. Vasc. Biol. 37, 2102–2113. doi: 10.1161/ATVBAHA.117.309401
Aoyama, T., Francke, U., Dietz, H. C., and Furthmayr, H. (1994). Quantitative differences in biosynthesis and extracellular deposition of fibrillin in cultured fibroblasts distinguish five groups of Marfan syndrome patients and suggest distinct pathogenetic mechanisms. J. Clin. Invest. 94, 130–137. doi: 10.1172/JCI117298
Atchison, L., Abutaleb, N. O., Snyder-Mounts, E., Gete, Y., Ladha, A., Ribar, T., et al. (2020). iPSC-Derived endothelial cells affect vascular function in a tissue-engineered blood vessel model of hutchinson-gilford progeria syndrome. Stem Cell Rep. 14, 325–337. doi: 10.1016/j.stemcr.2020.01.005
Atchison, L., Zhang, H., Cao, K., and Truskey, G. A. (2017). A tissue engineered blood vessel model of hutchinson-gilford progeria syndrome using human iPSC-derived smooth muscle cells. Sci. Rep. 7:8168.
Aubart, M., Gazal, S., Arnaud, P., Benarroch, L., Gross, M.-S., Buratti, J., et al. (2018). Association of modifiers and other genetic factors explain Marfan syndrome clinical variability. Eur. J. Hum. Genet. 26, 1759–1772. doi: 10.1038/s41431-018-0164-9
Ayoubi, S., Sheikh, S. P., and Eskildsen, T. V. (2017). Human induced pluripotent stemcell-derived vascular smooth muscle cells: differentiation and therapeutic potential. Cardiovasc. Res. 113, 1282–1293. doi: 10.1093/cvr/cvx125
Bassett, A. R. (2017). Editing the genome of hiPSC with CRISPR/Cas9: disease models. Mamm. Genome 28, 348–364. doi: 10.1007/s00335-017-9684-9
Bauwens, C. L., Peerani, R., Niebruegge, S., Woodhouse, K. A., Kumacheva, E., Husain, M., et al. (2008). Control of human embryonic stem cell colony and aggregate size heterogeneity influences differentiation trajectories. Stem Cells 26, 2300–2310. doi: 10.1634/stemcells.2008-0183
Bax, D. V., Bernard, S. E., Lomas, A., Morgan, A., Humphries, J., Shuttleworth, C. A., et al. (2003). Cell adhesion to fibrillin-1 molecules and microfibrils is mediated by α5β1 and αvβ3 integrins. J. Biol. Chem. 278, 34605–34616. doi: 10.1074/jbc.M303159200
Baxter, M., Withey, S., Harrison, S., Segeritz, C. P., Zhang, F., Atkinson-Dell, R., et al. (2015). Phenotypic and functional analyses show stem cell-derived hepatocyte-like cells better mimic fetal rather than adult hepatocytes. J. Hepatol. 62, 581–589. doi: 10.1016/j.jhep.2014.10.016
Bergqvist, D., Björck, M., and Wanhainen, A. (2013). Treatment of vascular Ehlers-Danlos syndrome: a systematic review. Ann. Surg. 258, 257–261. doi: 10.1097/SLA.0b013e31829c7a59
Best, C., Strouse, R., Hor, K., Pepper, V., Tipton, A., Kelly, J., et al. (2018). Toward a patient-specific tissue engineered vascular graft. J. Tissue Eng. 9:2041731418764709. doi: 10.1177/2041731418764709
Bi, D., Nishimura, J., Niiro, N., Hirano, K., and Kanaide, H. (2005). Contractile properties of the cultured vascular smooth muscle cells. Circ. Res. 96, 890–897. doi: 10.1161/01.res.0000163018.66460.85
Biel, N. M., Santostefano, K. E., DiVita, B. B., El Rouby, N., Carrasquilla, S. D., Simmons, C., et al. (2015). Vascular smooth muscle cells from hypertensive patient-derived induced pluripotent stem cells to advance hypertension pharmacogenomics. Stem Cells Transl. Med. 4, 1380–1390. doi: 10.5966/sctm.2015-0126
Boucher, J. M., Peterson, S. M., Urs, S., Zhang, C., and Liaw, L. (2011). The miR-143/145 cluster is a novel transcriptional target of Jagged-1/Notch signaling in vascular smooth muscle cells. J. Biol. Chem. 286, 28312–28321. doi: 10.1074/jbc.M111.221945
Brooke, B. S., Habashi, J. P., Judge, D. P., Patel, N., Loeys, B., and Dietz, H. C. (2008). Angiotensin II blockade and aortic-root dilation in marfan’s syndrome. N. Engl. J. Med. 358, 2787–2795. doi: 10.1056/NEJMoa0706585
Brownstein, A. J., Ziganshin, B. A., Kuivaniemi, H., Body, S. C., Bale, A. E., and Elefteriades, J. A. (2018). Genes associated with thoracic aortic aneurysm and dissection: an update and clinical implications. Aorta 6, 13–20. doi: 10.12945/j.aorta.2017.17.003
Bunton, T. E., Jensen Biery, N., Myers, L., Gayraud, B., Ramirez, F., and Dietz, H. C. (2001). Phenotypic alteration of vascular smooth muscle cells precedes elastolysis in a mouse model of Marfan syndrome. Circ. Res. 88, 37–43. doi: 10.1161/01.RES.88.1.37
Burridge, P. W., Matsa, E., Shukla, P., Lin, Z. C., Churko, J. M., Ebert, A. D., et al. (2014). Chemically defned generation of human cardiomyocytes. Nat. Methods 11, 855–860. doi: 10.1038/nMeth.2999
Carrabba, M., and Madeddu, P. (2018). Current strategies for the manufacture of small size tissue engineering vascular grafts. Front. Bioeng. Biotechnol. 6:41. doi: 10.3389/fbioe.2018.00041
Carta, L., Smaldone, S., Zilberberg, L., Loch, D., Dietz, H. C., Rifkin, D. B., et al. (2009). p38 MAPK is an early determinant of promiscuous Smad2/3 signaling in the aortas of fibrillin-1 (Fbn1)-null mice. J. Biol. Chem. 284, 5630–5636. doi: 10.1074/jbc.M806962200
Cattell, M. A., Hasleton, P. S., and Anderson, J. C. (1994). Glycosaminoglycan content is increased in dissecting aneurysms of human thoracic aorta. Clin. Chim. Acta 226, 29–46. doi: 10.1016/0009-8981(94)90100-7
Chaudhry, S. S., Cain, S. A., Morgan, A., Dallas, S. L., Shuttleworth, C. A., and Kielty, C. M. (2007). Fibrillin-1 regulates the bioavailability of TGFβ1. J. Cell Biol. 176, 355–367. doi: 10.1083/jcb.200608167
Chen, J., Kitchen, C. M., Streb, J. W., and Miano, J. M. (2002). Myocardin: a component of a molecular switch for smooth muscle differentiation. J. Mol. Cell. Cardiol. 34, 1345–1356. doi: 10.1006/jmcc.2002.2086
Cheung, C., Bernardo, A. S., Pedersen, R. A., and Sinha, S. (2014). Directed differentiation of embryonic origin-specific vascular smooth muscle subtypes from human pluripotent stem cells. Nat. Protoc. 9, 929–938. doi: 10.1038/nprot.2014.059
Cheung, C., Bernardo, A. S., Trotter, M. W. B., Pedersen, R. A., and Sinha, S. (2012). Generation of human vascular smooth muscle subtypes provides insight into embryological origing-dependent disease susceptibility. Nat. Biotechnol. 30, 165–173. doi: 10.1038/nbt.2107
Chiu, C.-Z., Wang, B.-W., and Shyu, K.-G. (2013). Effects of cyclic stretch on the molecular regulation of myocardin in rat aortic vascular smooth muscle cells. J. Biomed. Sci. 20:50. doi: 10.1186/1423-0127-20-50
Chooi, K. Y., Comerford, A., Sherwin, S. J., and Weinberg, P. D. (2017). Noradrenaline has opposing effects on the hydraulic conductance of arterial intima and media. J. Biomech. 54, 4–10. doi: 10.1016/j.jbiomech.2017.01.027
Chu, L. F., Leng, N., Zhang, J., Hou, Z., Mamott, D., Vereide, D. T., et al. (2016). Single-cell RNA-seq reveals novel regulators of human embryonic stem cell differentiation to definitive endoderm. Genome Biol. 17:173. doi: 10.1186/s13059-016-1033-x
Chung, A. W. Y., Au Yeung, K., Cortes, S. F., Sandor, G. G. S., Judge, D. P., Dietz, H. C., et al. (2007). Endothelial dysfunction and compromised eNOS/Akt signaling in the thoracic aorta during the progression of Marfan syndrome. Br. J. Pharmacol. 150, 1075–1083. doi: 10.1038/sj.bjp.0707181
Civelek, M., Ainslie, K., Garanich, J. S., and Tarbell, J. M. (2002). Smooth muscle cells contract in response to fluid flow via a Ca2+-independent signaling mechanism. J. Appl. Physiol. 93, 1907–1917. doi: 10.1152/japplphysiol.00988.2001
Clempus, R. E., and Griendling, K. K. (2006). Reactive oxygen species signaling in vascular smooth muscle cells. Cardiovasc. Res. 71, 216–225. doi: 10.1016/j.cardiores.2006.02.033
Collado, M. S., Cole, B. K., Figler, R. A., Lawson, M., Manka, D., Simmers, M. B., et al. (2017). Exposure of induced pluripotent stem cell-derived vascular endothelial and smooth muscle cells in coculture to hemodynamics induces primary vascular cell-like phenotypes. Stem Cells Transl. Med. 6, 1673–1683. doi: 10.1002/sctm.17-0004
Collod-Béroud, G., Le Bourdelles, S., Ades, L., Ala-Kokko, L., Booms, P., Boxer, M., et al. (2003). Update of the UMD-FBN1 mutation database and creation of an FBN1 polymorphism database. Hum. Mutat. 22, 199–208. doi: 10.1002/humu.10249
Conway, M. K., Gerger, M. J., Balay, E. E., O’Connell, R., Hanson, S., Daily, N. J., et al. (2015). Scalable 96-well plate based iPSC culture and production using a robotic liquid handling system. J. Vis. Exp. 99:e52755. doi: 10.3791/52755
Cook, J. R., Clayton, N. P., Carta, L., Galatioto, J., Chiu, E., Smaldone, S., et al. (2015). Dimorphic effects of transforming growth factor-β signaling during aortic aneurysm progression in mice suggest a combinatorial therapy for Marfan syndrome. Arterioscler. Thromb. Vasc. Biol. 35, 911–917. doi: 10.1161/ATVBAHA.114.305150
Cooper, J. L., Favreau, J. T., Gaudette, G. R., and Rolle, M. W. (2014). “Effects of cyclic stretch on three-dimensional vascular smooth muscle cell rings,” in Proceedings of the 2014 40th Annual Northeast Bioengineering Conference (NEBEC), Boston, MA, 1–2. doi: 10.1109/nebec.2014.6972762
Croissant, J. D., Kim, J. H., Eichele, G., Goering, L., Lough, J., Prywes, R., et al. (1996). Avian serum response factor expression restricted primarily to muscle cell lineages is required for α-actin gene transcription. Dev. Biol. 177, 250–264. doi: 10.1006/dbio.1996.0160
Dahl, S. L. M., Kypson, A. P., Lawson, J. H., Blum, J. L., Strader, J. T., Li, Y., et al. (2011). Readily available tissue-engineered vascular grafts. Sci. Transl. Med. 3:68ra9. doi: 10.1126/scitranslmed.3001426
Daneman, N., Lu, H., and Redelmeier, D. A. (2015). Fluoroquinolones and collagen associated severe adverse events: a longitudinal cohort study. BMJ Open 5:e010077. doi: 10.1136/bmjopen-2015-010077
Dash, B. C., Levi, K., Schwan, J., Luo, J., Bartulos, O., Wu, H., et al. (2016). Tissue-engineered vascular rings from human iPSC-derived smooth muscle cells. Stem Cell Rep. 7, 19–28. doi: 10.1016/j.stemcr.2016.05.004
De Wit, A., Vis, K., and Jeremy, R. W. (2013). Aortic stiffness in heritable aortopathies: relationship to aneurysm growth rate. Hear. Lung Circ. 22, 3–11. doi: 10.1016/j.hlc.2012.08.049
Denning, C., Borgdorff, V., Crutchley, J., Firth, K. S. A., George, V., Kalra, S., et al. (2016). Cardiomyocytes from human pluripotent stem cells: from laboratory curiosity to industrial biomedical platform. Biochim. Biophys. Acta 1863, 1728–1748. doi: 10.1016/j.bbamcr.2015.10.014
Dietz, H. C., Cutting, G. R., Pyeritz, R. E., Maslen, C. L., Sakai, L. Y., Corson, G. M., et al. (1991). Marfan syndrome caused by a recurrent de novo missense mutation in the fibrillin gene. Nature 352, 337–339. doi: 10.1038/352337a0
Dietz, H. C., Pyeritz, R. E., Puffenberger, E. G., Kendzior, R. J., Corson, G. M., Maslen, C. L., et al. (1992). Marfan phenotype variability in a family segregating a missense mutation in the epidermal growth factor-like motif of the Fibrillin Gene. J. Clin. Invest. 89, 1674–1680. doi: 10.1172/jci115766
Ding-Yang, T., Kuan-Lun, H., Jyong-Huei, L., Wei-Tien, C., and Shih-Kang, F. (2019). Abstract 595: constructing a tissue engineered blood vessel using a self-folding biodegradable hydrogel bilayer. Arterioscler. Thromb. Vasc. Biol. 39, A595–A595. doi: 10.1161/atvb.39.suppl_1.595
Domenga, V., Fardoux, P., Lacombe, P., Monet, M., Maciazek, J., Krebs, L. T., et al. (2004). Notch3 is required for arterial identity and maturation of vascular smooth muscle cells. Genes Dev. 18, 2730–2735. doi: 10.1101/gad.308904
Doyle, J. J., Doyle, A. J., Wilson, N. K., Habashi, J. P., Bedja, D., Whitworth, R. E., et al. (2015). A deleterious gene-by-environment interaction imposed by calcium channel blockers in Marfan syndrome. eLife 4:e08648. doi: 10.7554/eLife.08648
Drake, C. J. (2003). Embryonic and adult vasculogenesis. Birth Defects Res. Part C Embryo Today 69, 73–82. doi: 10.1002/bdrc.10003
Du, K. L., Ip, H. S., Li, J., Chen, M., Dandre, F., Yu, W., et al. (2003). Myocardin is a critical serum response factor cofactor in the transcriptional program regulating smooth muscle cell differentiation. Mol. Cell. Biol. 23, 2425–2437. doi: 10.1128/mcb.23.7.2425-2437.2003
Dubacher, N., Münger, J., Gorosabel, M. C., Crabb, J., Ksiazek, A. A., Caspar, S. M., et al. (2020). Celiprolol but not losartan improves the biomechanical integrity of the aorta in a mouse model of vascular Ehlers–Danlos syndrome. Cardiovasc. Res. 116, 457–465. doi: 10.1093/cvr/cvz095
Elliott, M. B., Ginn, B., Fukunishi, T., Bedja, D., Suresh, A., Chen, T., et al. (2019). Regenerative and durable small-diameter graft as an arterial conduit. Proc. Natl. Acad. Sci. U.S.A. 116, 12710–12719. doi: 10.1073/pnas.1905966116
Erceg, S., Laínez, S., Ronaghi, M., Stojkovic, P., Pérez-Aragó, M. A., Moreno-Manzano, V., et al. (2008). Differentiation of human embryonic stem cells to regional specific neural precursors in chemically defined medium conditions. PLoS One 3:e2122. doi: 10.1371/journal.pone.0002122
Faivre, L., Collod-Beroud, G., Loeys, B. L., Child, A., Binquet, C., Gautier, E., et al. (2007). Effect of mutation type and location on clinical outcome in 1,013 probands with Marfan syndrome or related phenotypes and FBN1 mutations: an international study. Am. J. Hum. Genet. 81, 454–466. doi: 10.1086/520125
Ferruzzi, J., Murtada, S. I., Li, G., Jiao, Y., Uman, S., Ting, M. Y. L., et al. (2016). Pharmacologically improved contractility protects against aortic dissection in mice with disrupted transforming growth factor-β signaling despite compromised extracellular matrix properties. Arterioscler. Thromb. Vasc. Biol. 36, 919–927. doi: 10.1161/ATVBAHA.116.307436
Fillinger, M. F., Sampson, L. N., Cronenwett, J. L., Powell, R. J., and Wagner, R. J. (1997). Coculture of endothelial cells and smooth muscle cells in bilayer and conditioned media models. J. Surg. Res. 67, 169–178. doi: 10.1006/jsre.1996.4978
Florido, R., Smith Karen, L., Cuomo Kimberly, K., and Russell Stuart, D. (2017). Cardiotoxicity from human epidermal growth factor receptor-2 (HER2) targeted therapies. J. Am. Heart Assoc. 6:e006915. doi: 10.1161/jaha.117.006915
Franken, R., Den Hartog, A. W., De Waard, V., Engele, L., Radonic, T., Lutter, R., et al. (2013). Circulating transforming growth factor-β as a prognostic biomarker in Marfan syndrome. Int. J. Cardiol. 168, 2441–2446. doi: 10.1016/j.ijcard.2013.03.033
Franken, R., Groenink, M., De Waard, V., Feenstra, H. M. A., Scholte, A. J., Van Den Berg, M. P., et al. (2016). Genotype impacts survival in Marfan syndrome. Eur. Heart J. 37, 3285–3290. doi: 10.1093/eurheartj/ehv739
Frederiksen, H. R., Holst, B., Mau-Holzmann, U. A., Freude, K., and Schmid, B. (2019). Generation of two isogenic iPSC lines with either a heterozygous or a homozygous E280A mutation in the PSEN1 gene. Stem Cell Res. 35:101403. doi: 10.1016/j.scr.2019.101403
Fukunishi, T., Best, C. A., Sugiura, T., Opfermann, J., Ong, C. S., Shinoka, T., et al. (2017). Preclinical study of patient-specific cell-free nanofiber tissue-engineered vascular grafts using 3-dimensional printing in a sheep model. J. Thorac. Cardiovasc. Surg. 153, 924–932. doi: 10.1016/j.jtcvs.2016.10.066
Gaio, N., Van Meer, B., Quirós Solano, W., Bergers, L., Van de Stolpe, A., Mummery, C., et al. (2016). Cytostretch, an organ-on-chip platform. Micromachines 7:120. doi: 10.3390/mi7070120
Galatioto, J., Caescu, C. I., Hansen, J., Cook, J. R., Miramontes, I., Iyengar, R., et al. (2018). Cell type-specific contributions of the angiotensin II type 1a receptor to aorta homeostasis and aneurysmal disease-brief report. Arterioscler. Thromb. Vasc. Biol. 38, 588–591. doi: 10.1161/ATVBAHA.117.310609
Ge, X., Ren, Y., Bartulos, O., Lee, M. Y., Yue, Z., Kim, K. Y., et al. (2012). Modeling supravalvular aortic stenosis syndrome with human induced pluripotent stem cells. Circulation 126, 1695–1704. doi: 10.1161/CIRCULATIONAHA.112.116996
Ghazanfari, S., Tafazzoli-Shadpour, M., and Shokrgozar, M. A. (2009). Effects of cyclic stretch on proliferation of mesenchymal stem cells and their differentiation to smooth muscle cells. Biochem. Biophys. Res. Commun. 388, 601–605. doi: 10.1016/j.bbrc.2009.08.072
Goldfinger, J. Z., Halperin, J. L., Marin, M. L., Stewart, A. S., Eagle, K. A., and Fuster, V. (2014). Thoracic aortic aneurysm and dissection. J. Am. Coll. Cardiol. 64, 1725–1739. doi: 10.1016/j.jacc.2014.08.025
Gong, J., Zhou, D., Jiang, L., Qiu, P., Milewicz, D. M., Eugene Chen, Y., et al. (2020). In vitro lineage-specific differentiation of vascular smooth muscle cells in response to SMAD3 deficiency. Arterioscler. Thromb. Vasc. Biol. 40, 1651–1663. doi: 10.1161/atvbaha.120.313033
Granata, A., Serrano, F., Bernard, W. G., McNamara, M., Low, L., Sastry, P., et al. (2017). An iPSC-derived vascular model of Marfan syndrome identifies key mediators of smooth muscle cell death. Nat. Genet. 49, 97–109. doi: 10.1038/ng.3723
Grewal, N., and Gittenberger-de Groot, A. C. (2018). Pathogenesis of aortic wall complications in Marfan syndrome. Cardiovasc. Pathol. 33, 62–69. doi: 10.1016/j.carpath.2018.01.005
Griendling, K. K., Ushio-Fukai, M., Lassègue, B., and Alexander, R. W. (1997). Angiotensin II signaling in vascular smooth muscle. Hypertension 29, 366–370. doi: 10.1161/01.hyp.29.1.366
Groenink, M., Den Hartog, A. W., Franken, R., Radonic, T., De Waard, V., Timmermans, J., et al. (2013). Losartan reduces aortic dilatation rate in adults with Marfan syndrome: a randomized controlled trial. Eur. Heart J. 34, 3491–3500. doi: 10.1093/eurheartj/eht334
Gui, L., Dash, B. C., Luo, J., Qin, L., Zhao, L., Yamamoto, K., et al. (2016). Implantable tissue-engineered blood vessels from human induced pluripotent stem cells. Biomaterials 102, 120–129. doi: 10.1016/j.biomaterials.2016.06.010
Guo, D. C., Pannu, H., Tran-Fadulu, V., Papke, C. L., Yu, R. K., Avidan, N., et al. (2007). Mutations in smooth muscle α-actin (ACTA2) lead to thoracic aortic aneurysms and dissections. Nat. Genet. 39, 1488–1493. doi: 10.1038/ng.2007.6
Guo, X. (2012). Transforming growth factor-β and smooth muscle differentiation. World J. Biol. Chem. 3, 41–52. doi: 10.4331/wjbc.v3.i3.41
Habashi, J. P., Doyle, J. J., Holm, T. M., Aziz, H., Schoenhoff, F., Bedja, D., et al. (2011). Angiotensin II type 2 receptor signaling attenuates aortic aneurysm in mice through ERK antagonism. Science 332, 361–365. doi: 10.1126/science.1192152
Habashi, J. P., Judge, D. P., Holm, T. M., Cohn, R. D., Loeys, B. L., Cooper, T. K., et al. (2006). Losartan, an AT1 antagonist, prevents aortic aneurysm in a mouse model of Marfan syndrome. Scienc 312, 117–121. doi: 10.1126/science.1124287
Haga, J. H., Li, Y.-S. J., and Chien, S. (2007). Molecular basis of the effects of mechanical stretch on vascular smooth muscle cells. J. Biomech. 40, 947–960. doi: 10.1016/j.jbiomech.2006.04.011
Halaidych, O. V., Cochrane, A., van den Hil, F. E., Mummery, C. L., and Orlova, V. V. (2019). Quantitative analysis of intracellular Ca 2+ release and contraction in hiPSC-derived vascular smooth muscle cells. Stem Cell Rep. 12, 647–656. doi: 10.1016/j.stemcr.2019.02.003
Han, X., Chen, H., Huang, D., Chen, H., Fei, L., Cheng, C., et al. (2018). Mapping human pluripotent stem cell differentiation pathways using high throughput single-cell RNA-sequencing. Genome Biol. 19:47.
Hannuksela, M., Johansson, B., and Carlberg, B. (2018). Aortic stiffness in families with inherited non-syndromic thoracic aortic disease. Scand. Cardiovasc. J. 52, 301–307. doi: 10.1080/14017431.2018.1546895
Harmon, A. W., and Nakano, A. (2013). Nkx2-5 lineage tracing visualizes the distribution of second heart field-derived aortic smooth muscle. Genesis 51, 862–869. doi: 10.1002/dvg.22721
Hastings, N. E., Simmers, M. B., McDonald, O. G., Wamhoff, B. R., and Blackman, B. R. (2007). Atherosclerosis-prone hemodynamics differentially regulates endothelial and smooth muscle cell phenotypes and promotes pro-inflammatory priming. Am. J. Physiol. Cell Physiol. 293, 1824–1833. doi: 10.1152/ajpcell.00385.2007
He, J., Weng, Z., Wu, S. C. M., and Boheler, K. R. (2018). “Generation of induced pluripotent stem cells from patients with COL3A1 mutations and differentiation to smooth muscle cells for ECM-Surfaceome Analyses BT - The Surfaceome: methods and protocols,” in Methods in Molecular Biology, Vol. 1722, eds K. R. Boheler and R. L. Gundry (New York, NY: Springer), 261–302. doi: 10.1007/978-1-4939-7553-2_17
Hirai, H., Yang, B., Garcia-Barrio, M. T., Rom, O., Ma, P. X., Zhang, J., et al. (2018). Direct reprogramming of fibroblasts into smooth muscle-like cells with defined transcription factors-brief report. Arterioscler. Thromb. Vasc. Biol. 38, 2191–2197. doi: 10.1161/ATVBAHA.118.310870
Holm, T. M., Habashi, J. P., Doyle, J. J., Bedja, D., Chen, Y., van Erp, C., et al. (2011). Noncanonical TGF-beta signaling contributes to aortic aneurysm progression in Marfan syndrome mice. Science 332, 332–361. doi: 10.1126/science.1192149
Hrvatin, S., O’Donnell, C. W., Deng, F., Millman, J. R., Pagliuca, F. W., DiIorio, P., et al. (2014). Differentiated human stem cells resemble fetal not adult, β cells. Proc. Natl. Acad. Sci. U.S.A. 111, 3038–3043. doi: 10.1073/pnas.1400709111
Hu, B. Y., Weick, J. P., Yu, J., Ma, L. X., Zhang, X. Q., Thomson, J. A., et al. (2010). Neural differentiation of human induced pluripotent stem cells follows developmental principles but with variable potency. Proc. Natl. Acad. Sci. U.S.A. 107, 4335–4340. doi: 10.1073/pnas.0910012107
Hu, J. H., Wei, H., Jaffe, M., Airhart, N., Du, L., Angelov, S. N., et al. (2015). Postnatal deletion of the type II transforming growth factor-β receptor in smooth muscle cells causes severe aortopathy in mice. Arterioscler. Thromb. Vasc. Biol. 35, 2647–2656. doi: 10.1161/ATVBAHA.115.306573
Humphrey, J. D., Schwartz, M. A., Tellides, G., and Milewicz, D. M. (2015). Role of mechanotransduction in vascular biology: focus on thoracic aortic aneurysms and dissections. Circ. Res. 116, 1448–1461. doi: 10.1161/CIRCRESAHA.114.304936
Ieda, M., Fu, J. D., Delgado-Olguin, P., Vedantham, V., Hayashi, Y., Bruneau, B. G., et al. (2010). Direct reprogramming of fibroblasts into functional cardiomyocytes by defined factors. Cell 142, 375–386. doi: 10.1016/j.cell.2010.07.002
Ikonomidis, J. S., Jones, J. A., Barbour, J. R., Stroud, R. E., Clark, L. L., Kaplan, B. S., et al. (2006). Expression of matrix metalloproteinases and endogenous inhibitors within ascending aortic aneurysms of patients with Marfan syndrome. Circulation 114, I–365–I–370. doi: 10.1161/CIRCULATIONAHA.105.000810
Itskovitz-Eldor, J., Schuldiner, M., Karsenti, D., Eden, A., Yanuka, O., Amit, M., et al. (2000). Differentiation of human embryonic stem cells into embryoid bodies compromising the three embryonic germ layers. Mol. Med. 6, 88–95. doi: 10.1007/bf03401776
Jakobsson, L., and van Meeteren, L. A. (2013). Transforming growth factor β family members in regulation of vascular function: in the light of vascular conditional knockouts. Exp. Cell Res. 319, 1264–1270. doi: 10.1016/j.yexcr.2013.02.015
Jeon, E. S., Moon, H. J., Lee, M. J., Song, H. Y., Kim, Y. M., Bae, Y. C., et al. (2006). Sphigosylphosphorylcholine induces differentiation of human mesenchymal stem cells into smooth-muscle-like through a TGF-β-dependent mechanism. J. Cell Sci. 119, 4994–5005. doi: 10.1242/jcs.03281
Jeremy, R. W., Huang, H., Hwa, J., McCarron, H., Hughes, C. F., and Richards, J. G. (1994). Relation between age, arterial distensibility, and aortic dilatation in the Marfan syndrome. Am. J. Cardiol. 74, 369–373. doi: 10.1016/0002-9149(94)90405-7
Jiang, X., Rowitch, D. H., Soriano, P., McMahon, A. P., and Sucov, H. M. (2000). Fate of the mammalian cardiac neural crest. Development 127, 1607–1616.
Jiao, J., Xiong, W., Wang, L., Yang, J., Qiu, P., Hirai, H., et al. (2016). Differentiation defect in neural crest-derived smooth muscle cells in patients with aortopathy associated with bicuspid aortic valves. EBioMedicine 10, 282–290. doi: 10.1016/j.ebiom.2016.06.045
Judge, D. P., Biery, N. J., Keene, D. R., Geubtner, J., Myers, L., Huso, D. L., et al. (2004). Evidence for a critical contribution of haploinsufficiency in the complex pathogenesis of Marfan syndrome. J. Clin. Invest. 114, 172–181. doi: 10.1172/jci20641
Jung, Y., Ji, H., Chen, Z., Fai Chan, H., Atchison, L., Klitzman, B., et al. (2015). Scaffold-free, human mesenchymal stem cell-based tissue engineered blood vessels. Sci. Rep. 5:15116. doi: 10.1038/srep15116
Kelaini, S., Cochrane, A., and Margariti, A. (2014). Direct reprogramming of adult cells: avoiding the pluripotent state. Stem Cells Cloning 7, 19–29. doi: 10.2147/SCCAA.S38006
Keller, G. (2005). Embryonic stem cell differentiation: emergence of a new era in biology and medicine. Genes Dev. 19, 1129–1155. doi: 10.1101/gad.1303605
Kielty, C. M., Whittaker, S. P., Grant, M. E., and Shuttleworth, C. A. (1992). Attachment of human vascular smooth muscle cells to intact microfibrillar assemblies of collagen VI and fibrillin. J. Cell Sci. 103, 445–451.
Kim, S., Kim, W., Lim, S., and Jeon, J. S. (2017). Vasculature-on-a-chip for in vitro disease models. Bioengineering 4:E8. doi: 10.3390/bioengineering4010008
Kinnear, C., Agrawal, R., Loo, C., Pahnke, A., Rodrigues, D. C., Thompson, T., et al. (2020). Everolimus rescues the phenotype of Elastin insufficiency in patient induced pluripotent stem cell-derived vascular smooth muscle cells. Arterioscler. Thromb. Vasc. Biol. 1325–1339. doi: 10.1161/ATVBAHA.119.313936
Kinnear, C., Chang, W. Y., Khattak, S., Hinek, A., Thompson, T., De Carvalho Rodrigues, D., et al. (2013). Modelling and rescue of the vascular phenotype of williams-beuren syndrome in patient induced pluripotent stem cells. Stem Cells Transl. Med. 2, 2–15. doi: 10.5966/sctm.2012-0054
Konig, G., McAllister, T. N., Dusserre, N., Garrido, S. A., Iyican, C., Marini, A., et al. (2009). Mechanical properties of completely autologous human tissue engineered blood vessels compared to human saphenous vein and mammary artery. Biomaterials 30, 1542–1550. doi: 10.1016/j.biomaterials.2008.11.011
Koobatian, M. T., Row, S., Smith, R. J. Jr., Koenigsknecht, C., Andreadis, S. T., and Swartz, D. D. (2016). Successful endothelialization and remodeling of a cell-free small-diameter arterial graft in a large animal model. Biomaterials 76, 344–358. doi: 10.1016/j.biomaterials.2015.10.020
Kumar, V. A., Brewster, L. P., Caves, J. M., and Chaikof, E. L. (2011). Tissue engineering of blood vessels: functional requirements, progress, and future challenges. Cardiovasc. Eng. Technol. 2, 137–148. doi: 10.1007/s13239-011-0049-3
Kusumoto, D., and Yuasa, S. (2019). The application of convolutional neural network to stem cell biology. Inflamm. Regen. 39, 1–7.
Kuwabara, J. T., and Tallquist, M. D. (2017). Tracking adventitial fibroblast contribution to disease: a review of current methods to identify resident fibroblasts. Arterioscler. Thromb. Vasc. Biol. 37, 1598–1607. doi: 10.1161/ATVBAHA.117.308199
Lacro, R. V., Dietz, H. C., Sleeper, L. A., Yetman, A. T., Bradley, T. J., Colan, S. D., et al. (2014). Atenolol versus losartan in children and young adults with Marfan’s syndrome. N. Engl. J. Med. 371, 2061–2071. doi: 10.1056/NEJMoa1404731
Landis, B. J., Schubert, J. A., Lai, D., Jegga, A. G., Shikany, A. R., Foroud, T., et al. (2017). Exome sequencing identifies candidate genetic modifiers of syndromic and familial thoracic aortic aneurysm severity. J. Cardiovasc. Transl. Res. 10, 423–432. doi: 10.1007/s12265-017-9753-1
Lanfranconi, S., and Markus, H. S. (2010). COL4A1 mutations as a monogenic cause of cerebral small vessel disease: a systematic review. Stroke 41, 513–518. doi: 10.1161/STROKEAHA.110.581918
Lavoie, P., Robitaille, G., Agharazii, M., Ledbetter, S., Lebel, M., and Larivière, R. (2005). Neutralization of transforming growth factor-β attenuates hypertension and prevents renal injury in uremic rats. J. Hypertens. 23, 1895–1903. doi: 10.1097/01.hjh.0000182521.44440.c5
Lawson, J. H., Glickman, M. H., Ilzecki, M., Jakimowicz, T., Jaroszynski, A., Peden, E. K., et al. (2016). Bioengineered human acellular vessels for dialysis access in patients with end-stage renal disease: two phase 2 single-arm trials. Lancet 387, 2026–2034. doi: 10.1016/s0140-6736(16)00557-2
Lee, A. A., Graham, D. A., Dela Cruz, S., Ratcliffe, A., and Karlon, W. J. (2002). Fluid shear stress-induced alignment of cultured vascular smooth muscle cells. J. Biomech. Eng. 124, 37–43. doi: 10.1115/1.1427697
Lee, C.-C., Lee, M. G., Chen, Y.-S., Lee, S.-H., Chen, Y.-S., Chen, S.-C., et al. (2015). Risk of aortic dissection and aortic aneurysm in patients taking oral fluoroquinolone. JAMA Intern. Med. 175, 1839–1847. doi: 10.1001/jamainternmed.2015.5389
Lee, H. S., Yun, S. J., Ha, J. M., Jin, S. Y., Ha, H. K., Song, S. H., et al. (2019). Prostaglandin D(2) stimulates phenotypic changes in vascular smooth muscle cells. Exp. Mol. Med. 51:137. doi: 10.1038/s12276-019-0330-3
LeMaire, S. A., Zhang, L., Luo, W., Ren, P., Azares, A. R., Wang, Y., et al. (2018). Effect of ciprofloxacin on susceptibility to aortic dissection and rupture in mice. JAMA Surg. 153:e181804. doi: 10.1001/jamasurg.2018.1804
Li, W., Li, Q., Jiao, Y., Qin, L., Ali, R., Zhou, J., et al. (2014). Tgfbr2 disruption in postnatal smooth muscle impairs aortic wall homeostasis. J. Clin. Invest. 124, 755–767. doi: 10.1172/JCI69942
Lilly, B. (2014). We have contact: endothelial cell-smooth muscle cell interactions. Physiology 29, 234–241. doi: 10.1152/physiol.00047.2013
Lin, H., Qiu, X., Du, Q., Li, Q., Wang, O., Akert, L., et al. (2019). Engineered microenvironment for manufacturing human pluripotent stem cell-derived vascular smooth muscle cells. Stem Cell Rep. 12, 84–97. doi: 10.1016/j.stemcr.2018.11.009
Lindsay, M. E., Schepers, D., Bolar, N. A., Doyle, J. J., Gallo, E., Fert-Bober, J., et al. (2012). Loss-of-function mutations in TGFB2 cause a syndromic presentation of thoracic aortic aneurysm. Nat. Genet. 44, 922–927. doi: 10.1038/ng.2349
Liu, G. H., Barkho, B. Z., Ruiz, S., Diep, D., Qu, J., Yang, S. L., et al. (2011). Recapitulation of premature ageing with iPSCs from Hutchinson-Gilford progeria syndrome. Nature 472, 221–227. doi: 10.1038/nature09879
Liu, H., Kennard, S., and Lilly, B. (2009). NOTCH3 expression is induced in mural cells through an autoregulatory loop that requires Endothelial-expressed JAGGED1. Circ. Res. 104, 466–475. doi: 10.1161/CIRCRESAHA.108.184846
Lo Sardo, V., Chubukov, P., Ferguson, W., Kumar, A., Teng, E. L., Duran, M., et al. (2018). Unveiling the role of the most impactful cardiovascular risk locus through haplotype editing. Cell 175, P1796–1810.E20. doi: 10.1016/j.cell.2018.11.014
Lundy, S. D., Zhu, W. Z., Regnier, M., and Laflamme, M. A. (2013). Structural and functional maturation of cardiomyocytes derived from human pluripotent stem cells. Stem Cells Dev. 22, 1991–2002. doi: 10.1089/scd.2012.0490
Luo, J., Qin, L., Zhao, L., Gui, L., Ellis, M. W., Huang, Y., et al. (2020). Tissue-engineered vascular grafts with advanced mechanical strength from human iPSCs. Cell Stem Cell 26, 251–261.e8. doi: 10.1016/j.stem.2019.12.012
MacFarlane, E. G., Parker, S. J., Shin, J. Y., Ziegler, S. G., Creamer, T. J., Bagirzadeh, R., et al. (2019). Lineage-specific events underlie aortic root aneurysm pathogenesis in Loeys-Dietz syndrome. J. Clin. Invest. 129, 659–675. doi: 10.1172/JCI123547
Majesky, M. W. (2007). Developmental basis of vascular smooth muscle diversity. Arterioscler. Thromb. Vasc. Biol. 27, 1248–1258. doi: 10.1161/ATVBAHA.107.141069
Mallat, Z., Tedgui, A., and Henrion, D. (2016). Role of microvascular tone and extracellular matrix contraction in the regulation of interstitial fluid: implications for aortic dissection. Arterioscler. Thromb. Vasc. Biol. 36, 1742–1747. doi: 10.1161/ATVBAHA.116.307909
Manabe, I., and Owens, G. K. (2001a). CArG elements control smooth muscle subtype-specific expression of smooth muscle myosin in vivo. J. Clin. Invest. 107, 823–834. doi: 10.1172/JCI11385
Manabe, I., and Owens, G. K. (2001b). Recruitment of serum response factor and hyperacetylation of histones at smooth muscle-specific regulatory regions during differentiation of a novel P19-derived in vitro smooth muscle differentiation system. Circ. Res. 88, 1127–1134. doi: 10.1161/hh1101.091339
Mantella, L.-E. E., Quan, A., and Verma, S. (2015). Variability in vascular smooth muscle cell stretch-induced responses in 2D culture. Vasc. Cell 7:7.
Martin, K. A., Rzucidlo, E. M., Merenick, B. L., Fingar, D. C., Brown, D. J., Wagner, R. J., et al. (2004). The mTOR/p70 S6K1 pathway regulates vascular smooth muscle cell differentiation. Am. J. Physiol. Cell Physiol. 286, 507–517. doi: 10.1152/ajpcell.00201.2003
Martinez-Lemus, L. A., Wu, X., Wilson, E., Hill, M. A., Davis, G. E., Davis, M. J., et al. (2003). Integrins as unique receptors for vascular control. J. Vasc. Res. 40, 211–233. doi: 10.1159/000071886
Matsuzaki, Y., John, K., Shoji, T., and Shinoka, T. (2019). The evolution of tissue engineered vascular graft technologies: from preclinical trials to advancing patient care. Appl. Sci. 9:1274. doi: 10.3390/app9071274
Messana, J. M., Hwang, N. S., Coburn, J., Elisseeff, J. H., and Zhang, Z. (2008). Size of the embryoid body influences chondrogenesis of mouse embryonic stem cells. J. Tissue Eng. Regen. Med. 2, 499–506. doi: 10.1002/term
Mica, Y., Lee, G., Chambers, S. M., Tomishima, M. J., and Studer, L. (2013). Modeling neural crest induction, melanocyte specification, and disease-related pigmentation defects in hescs and patient-specific iPSCs. Cell Rep. 3, 1140–1152. doi: 10.1016/j.celrep.2013.03.025
Michel, J.-B., Jondeau, G., and Milewicz, D. M. (2018). From genetics to response to injury: vascular smooth muscle cells in aneurysms and dissections of the ascending aorta. Cardiovasc. Res. 114, 578–589. doi: 10.1093/cvr/cvy006
Milewicz, D. M., Dietz, H. C., and Miller, D. C. (2005). Treatment of aortic disease in patients with Marfan syndrome. Circulation 111, 150–157. doi: 10.1161/01.cir.0000155243.70456.f4
Milleron, O., Arnoult, F., Ropers, J., Aegerter, P., Detaint, D., Delorme, G., et al. (2015). Marfan Sartan: a randomized, double-blind, placebo-controlled trial. Eur. Heart J. 36, 2160–2166. doi: 10.1093/eurheartj/ehv151
Mohr, J. C., Zhang, J., Azarin, S. M., Soerens, A. G., de Pablo, J. J., Thomson, J. A., et al. (2010). The microwell control of embryoid body size in order to regulate cardiac differentiation of human embryonic stem cells. Biomaterials 31, 1885–1893. doi: 10.1016/j.biomaterials.2009.11.033
Mullen, M., Jin, X. Y., Child, A., Stuart, A. G., Dodd, M., Aragon-Martin, J. A., et al. (2020). Irbesartan in Marfan syndrome (AIMS): a double-blind, placebo-controlled randomised trial. Lancet 394, 2263–2270.
Mummery, C. L., Zhang, J., Ng, E. S., Elliott, D. A., Elefanty, A. G., and Kamp, T. J. (2012). Differentiation of human embryonic stem cells and induced pluripotent stem cells to cardiomyocytes: a methods overview. Circ. Res. 111, 344–358. doi: 10.1161/CIRCRESAHA.110.227512
Murdoch, J. L., Walker, B. A., Halpern, B. L., Kuzma, J. W., and McKusick, V. A. (1972). Life expectancy and causes of death in the Marfan syndrome. N. Engl. J. Med. 286, 804–808. doi: 10.1056/nejm197204132861502
Nakamura, T., Colbert, M. C., and Robbins, J. (2006). Neural crest cells retain multipotential characteristics in the developing valves and label the cardiac conduction system. Circ. Res. 98, 1547–1554. doi: 10.1161/01.RES.0000227505.19472.69
Neptune, E. R., Frischmeyer, P. A., Arking, D. E., Myers, L., Bunton, T. E., Gayraud, B., et al. (2003). Dysregulation of TGF-β activation contributes to pathogenesis in Marfan syndrome. Nat. Genet. 33, 407–411. doi: 10.1038/ng1116
Nishida, W., Nakamura, M., Mori, S., Takahashi, M., Ohkawa, Y., Tadokoro, S., et al. (2002). A triad of serum response factor and the GATA and NK families governs the transcription of smooth and cardiac muscle genes. J. Biol. Chem. 277, 7308–7317. doi: 10.1074/jbc.M111824200
Noman, A. T., Qazi, A. H., Alqasrawi, M., Ayinde, H., Tleyjeh, I. M., Lindower, P., et al. (2019). Fluoroquinolones and the risk of aortopathy: a systematic review and meta-analysis. Int. J. Cardiol. 274, 299–302. doi: 10.1016/j.ijcard.2018.09.067
O’Callaghan, C. J., and Williams, B. (2000). Mechanical strain-induced extracellular matrix production by human vascular smooth muscle cells: role of TGF-β1. Hypertension 36, 319–324. doi: 10.1161/01.HYP.36.3.319
Oishi, K., Itoh, Y., Isshiki, Y., Kai, C., Takeda, Y., Yamaura, K., et al. (2000). Agonist-induced isometric contraction of smooth muscle cell-populated collagen gel fiber. Am. J. Physiol. Physiol. 279, C1432–C1442. doi: 10.1152/ajpcell.2000.279.5.C1432
Oller, J., Méndez-Barbero, N., Ruiz, E. J., Villahoz, S., Renard, M., Canelas, L. I., et al. (2017). Nitric oxide mediates aortic disease in mice deficient in the metalloprotease Adamts1 and in a mouse model of Marfan syndrome. Nat. Med. 23, 200–212. doi: 10.1038/nm.4266
Osafune, K., Caron, L., Borowiak, M., Martinez, R. J., Fitz-Gerald, C. S., Sato, Y., et al. (2008). Marked differences in differentiation propensity among human embryonic stem cell lines. Nat. Biotechnol. 26, 313–315. doi: 10.1038/nbt1383
Owens, G. K. (2007). Molecular control of vascular smooth muscle cell differentiation and phenotypic plasticity. Novartis Found. Symp. 283, 174–191. doi: 10.1002/9780470319413.ch14
Owens, G. K., Kumar, M. S., and Wamhoff, B. R. (2004). Molecular regulation of vascular smooth muscle cell differentiation in development and disease. Physiol. Rev. 84, 767–801. doi: 10.1152/physrev.00041.2003
Palakkan, A. A., Nanda, J., and Ross, J. A. (2017). Pluripotent stem cells to hepatocytes, the journey so far. Biomed. Rep. 6, 367–373. doi: 10.3892/br.2017.867
Papadaki, M., McIntire, L. V., and Eskin, S. G. (1996). Effects of shear stress on the growth kinetics of human aortic smooth muscle cells in vitro. Biotechnol. Bioeng. 50, 555–561. doi: 10.1002/(sici)1097-0290(19960605)50:5<555::aid-bit10>3.0.co;2-i
Paquet, D., Kwart, D., Chen, A., Sproul, A., Jacob, S., Teo, S., et al. (2016). Efficient introduction of specific homozygous and heterozygous mutations using CRISPR/Cas9. Nature 533, 125–129. doi: 10.1038/nature17664
Park, J. S., Chu, J. S. F., Cheng, C., Chen, F., Chen, D., and Li, S. (2004). Differential effects of equiaxial and uniaxial strain on mesenchymal stem cells. Biotechnol. Bioeng. 88, 359–368. doi: 10.1002/bit.20250
Pashneh-Tala, S., MacNeil, S., and Claeyssens, F. (2016). The tissue-engineered vascular graft-past, present, and future. Tissue Eng. Part B. Rev. 22, 68–100. doi: 10.1089/ten.teb.2015.0100
Pasternak, B., Inghammar, M., and Svanström, H. (2018). Fluoroquinolone use and risk of aortic aneurysm and dissection: nationwide cohort study. BMJ 360:k678. doi: 10.1136/bmj.k678
Patel-Hett, S., and D’Amore, P. A. (2011). Signal transduction in vasculogenesis and developmental angiogenesis. Int. J. Dev. Biol. 55, 353–363. doi: 10.1387/ijdb.103213sp
Patsch, C., Challet-Meylan, L., Thoma, E. C., Urich, E., Heckel, T., O’Sullivan, J. F., et al. (2015). Generation of vascular endothelial and smooth muscle cells from human pluripotent stem cells. Nat. Cell Biol. 17, 994–1003. doi: 10.1038/ncb3205
Paull, D., Sevilla, A., Zhou, H., Hahn, A. K., Kim, H., Napolitano, C., et al. (2015). Automated, high-throughput derivation, characterization and differentiation of induced pluripotent stem cells. Nat. Methods 12, 885–892. doi: 10.1038/nmeth.3507
Pawlowski, M., Ortmann, D., Bertero, A., Tavares, J. M., Pedersen, R. A., Vallier, L., et al. (2017). Inducible and deterministic forward programming of human pluripotent stem cells into neurons, skeletal myocytes, and oligodendrocytes. Stem Cell Rep. 8, 803–812. doi: 10.1016/j.stemcr.2017.02.016
Pepin, M., Schwarze, U., Superti-Furga, A., and Byers, P. H. (2000). Clinical and genetic features of ehlers-danlos syndrome type IV, the vascular type. N. Engl. J. Med. 342, 673–680. doi: 10.1056/nejm200003093421001
Pereira, L., Lee, S. Y., Gayraud, B., Andrikopoulos, K., Shapiro, S. D., Bunton, T., et al. (1999). Pathogenetic sequence for aneurysm revealed in mice underexpressing fibrillin-1. Proc. Natl. Acad. Sci. U.S.A. 96, 3819–3823. doi: 10.1073/pnas.96.7.3819
Pinard, A., Jones, G. T., and Milewicz, D. M. (2019). Genetics of thoracic and abdominal aortic diseases. Circ. Res. 124, 588–606. doi: 10.1161/CIRCRESAHA.118.312436
Popp, B., Krumbiegel, M., Grosch, J., Sommer, A., Uebe, S., Kohl, Z., et al. (2018). Need for high-resolution Genetic Analysis in iPSC: results and lessons from the ForIPS consortium. Sci. Rep. 8:17201.
Pyeritz, R. E., and KcKusick, V. A. (1979). The Marfan Syndrome: diagnosis and Management. N. Engl. J. Med. 300, 772–777. doi: 10.1056/NEJM197904053001406
Qiu, J., Zheng, Y., Hu, J., Liao, D., Gregersen, H., Deng, X., et al. (2013). Biomechanical regulation of vascular smooth muscle cell functions: from in vitro to in vivo understanding. J. R. Soc. Interface 11:20130852. doi: 10.1098/rsif.2013.0852
Quarto, N., Leonard, B., Li, S., Marchand, M., Anderson, E., Behr, B., et al. (2012a). Skeletogenic phenotype of human Marfan embryonic stem cells faithfully phenocopied by patient-specific induced-pluripotent stem cells. Proc. Natl. Acad. Sci. U.S.A. 109, 215–220. doi: 10.1073/pnas.1113442109
Quarto, N., Li, S., Renda, A., and Longaker, M. T. (2012b). Exogenous activation of BMP-2 signaling overcomes TGFβ-mediated inhibition of osteogenesis in marfan embryonic stem cells and marfan patient-specific induced pluripotent stem cells. Stem Cells 30, 2709–2719. doi: 10.1002/stem.1250
Raphel, L., Talasila, A., Cheung, C., and Sinha, S. (2012). Myocardin overexpression is sufficient for promoting the development of a mature smooth muscle cell-like phenotype from human embryonic stem cells. PLoS One 7:e44052. doi: 10.1371/journal.pone.0044052
Rodríguez-Vita, J., Sánchez-López, E., Esteban, V., Rupérez, M., Egido, J., and Ruiz-Ortega, M. (2005). Angiotensin II activates the Smad pathway in vascular smooth muscle cells by a transforming growth factor-β-independent mechanism. Circulation 111, 2509–2517. doi: 10.1161/01.CIR.0000165133.84978.E2
Rossi, A., Gabbrielli, E., Villano, M., Messina, M., Ferrara, F., and Weber, E. (2010). Human microvascular lymphatic and blood endothelial cells produce fibrillin: deposition patterns and quantitative analysis. J. Anat. 217, 705–714. doi: 10.1111/j.1469-7580.2010.01306.x
Rovner, A. S., Murphy, R. A., and Owens, G. K. (1986). Expression of smooth muscle and nonmuscle myosin heavy chains in cultured vascular smooth muscle cells. J. Biol. Chem. 261, 14740–14745.
Row, S., Santandreu, A., Swartz, D. D., and Andreadis, S. T. (2017). Cell-free vascular grafts: recent developments and clinical potential. Technology 5, 13–20. doi: 10.1142/S2339547817400015
Sachlos, E., and Auguste, D. T. (2008). Embryoid body morphology influences diffusive transport of inductive biochemicals: a strategy for stem cell differentiation. Biomaterials 29, 4471–4480. doi: 10.1016/j.biomaterials.2008.08.012
Sato, T., Arakawa, M., Tashima, Y., Tsuboi, E., Burdon, G., Trojan, J., et al. (2018). Statins reduce thoracic aortic aneurysm growth in marfan syndrome mice via inhibition of the ras-induced ERK (Extracellular signal-regulated kinase) signaling pathway. J. Am. Heart Assoc. 7:e008543. doi: 10.1161/JAHA.118.008543
Sawada, H., Rateri, D. L., Moorleghen, J. J., Majesky, M. W., and Daugherty, A. (2017). Smooth muscle cells derived from second heart field and cardiac neural crest reside in spatially distinct domains in the media of the ascending aorta - brief report. Arterioscler. Thromb. Vasc. Biol. 37, 1722–1726. doi: 10.1161/ATVBAHA.117.309599
Schrijver, I., Liu, W., Brenn, T., Furthmayr, H., and Francke, U. (1999). Cysteine substitutions in epidermal growth factor-like domains of fibrillin-1: distinct effects on biochemical and clinical phenotypes. Am. J. Hum. Genet. 65, 1007–1020. doi: 10.1086/302582
Schrijver, I., Liu, W., Odom, R., Brenn, T., Oefner, P., Furthmayr, H., et al. (2002). Premature termination mutations in FBN1: distinct effects on differential allelic expression and on protein and clinical phenotypes. Am. J. Hum. Genet. 71, 223–237. doi: 10.1086/341581
Segura, A. M., Luna, R. E., Horiba, K., Stetler-Stevenson, W. G., McAllister, H. A., Willerson, J. T., et al. (1998). Immunohistochemistry of matrix metalloproteinases and their inhibitors in thoracic aortic aneurysms and aortic valves of patients with Marfan’s syndrome. Circulation 98, II331–II337.
Sendzik, J., Shakibaei, M., Schäfer-Korting, M., Lode, H., and Stahlmann, R. (2010). Synergistic effects of dexamethasone and quinolones on human-derived tendon cells. Int. J. Antimicrob. Agents 35, 366–374. doi: 10.1016/j.ijantimicag.2009.10.009
Serrano, F., Bernard, W. G., Granata, A., Iyer, D., Steventon, B., Kim, M., et al. (2019). A novel human pluripotent stem cell-derived neural crest model of treacher collins syndrome shows defects in cell death and migration. Stem Cells Dev. 28, 81–100. doi: 10.1089/scd.2017.0234
Sharma, A., Burridge, P. W., McKeithan, W. L., Serrano, R., Shukla, P., Sayed, N., et al. (2017). High-throughput screening of tyrosine kinase inhibitor cardiotoxicity with human induced pluripotent stem cells. Sci. Transl. Med. 9:eaaf2584. doi: 10.1126/scitranslmed.aaf2584
Shi, Z. D., Abraham, G., and Tarbell, J. M. (2010). Shear stress modulation of smooth muscle cell marker genes in 2-D and 3-D depends on mechanotransduction by heparan sulfate Proteoglycans and ERK1/2. PLoS One 5:e12196. doi: 10.1371/journal.pone.0012196
Shi, Z.-D., and Tarbell, J. M. (2011). Fluid flow mechanotransduction in vascular smooth muscle cells and fibroblasts. Ann. Biomed. Eng. 39, 1608–1619. doi: 10.1007/s10439-011-0309-2
Sinha, S., Wamhoff, B. R., Hoofnagle, M. H., Thomas, J., Neppl, R. L., Deering, T., et al. (2006). Assessment of contractility of purified smooth muscle cells derived from embryonic stem cells. Stem Cells 24, 1678–1688. doi: 10.1634/stemcells.2006-0002
Skovrind, I., Harvald, E. B., Juul Belling, H., Jørgensen, C. D., Lindholt, J. S., and Andersen, D. C. (2019). Concise review: patency of small-diameter tissue-engineered vascular grafts: a meta-analysis of preclinical trials. Stem Cells Transl. Med. 8, 671–680. doi: 10.1002/sctm.18-0287
Solan, A., Dahl, S. L. M., and Niklason, L. E. (2009). Effects of mechanical stretch on collagen and cross-linking in engineered blood vessels. Cell Transplant. 18, 915–921. doi: 10.3727/096368909x471161
Song, H. H. G., Rumma, R. T., Ozaki, C. K., Edelman, E. R., and Chen, C. S. (2018). Vascular tissue engineering: progress, challenges, and clinical promise. Cell Stem Cell 22, 340–354. doi: 10.1016/j.stem.2018.02.009
Song, J., Rolfe, B. E., Hayward, I. P., Campbell, G. R., and Campbell, J. H. (2001). Reorganization of structural proteins in vascular smooth muscle cells grown in collagen gel and basement membrane matrices (Matrigel): a comparison with their in situ counterparts. J. Struct. Biol. 133, 43–54. doi: 10.1006/jsbi.2001.4327
Stenzel, D., Nye, E., Nisancioglu, M., Adams, R. H., Yamaguchi, Y., and Gerhardt, H. (2009). Peripheral mural cell recruitment requires cell-autonomous heparan sulfate. Blood 114, 915–924. doi: 10.1182/blood-2008-10-186239
Suchorska, W. M., Augustyniak, E., Richter, M., and Trzeciak, T. (2017). Comparison of four protocols to generate chondrocyte-like cells from human induced pluripotent stem cells (hiPSCs). Stem Cell Rev. Rep. 13, 299–308. doi: 10.1007/s12015-016-9708-y
Sugiura, T., Matsumura, G., Miyamoto, S., Miyachi, H., Breuer, C. K., and Shinoka, T. (2018). Tissue-engineered vascular grafts in children with congenital heart disease: intermediate term follow-up. Semin. Thorac. Cardiovasc. Surg. 30, 175–179. doi: 10.1053/j.semtcvs.2018.02.002
Sundaram, S., One, J., Siewert, J., Teodosescu, S., Zhao, L., Dimitrievska, S., et al. (2014). Tissue-engineered vascular grafts created from human induced pluripotent stem cells. Stem Cells Transl. Med. 3, 1535–1543. doi: 10.5966/sctm.2014-0065
Takahashi, K., and Yamanaka, S. (2006). Induction of pluripotent stem cells from mouse embryonic and adult fibroblast cultures by defined factors. Cell 126, 663–676. doi: 10.1016/j.cell.2006.07.024
Teixido-Tura, G., Forteza, A., Rodríguez-Palomares, J., González Mirelis, J., Gutiérrez, L., Sánchez, V., et al. (2018). Losartan versus Atenolol for prevention of aortic dilation in patients with Marfan syndrome. J. Am. Coll. Cardiol. 72, 1613–1618. doi: 10.1016/j.jacc.2018.07.052
Tidball, A. M., Dang, L. T., Glenn, T. W., Kilbane, E. G., Klarr, D. J., Margolis, J. L., et al. (2017). Rapid generation of human genetic loss-of-function iPSC lines by simultaneous reprogramming and gene editing. Stem Cell Rep. 9, 725–731. doi: 10.1016/j.stemcr.2017.07.003
Tiscornia, G., Vivas, E. L., and Belmonte, J. C. I. (2011). Diseases in a dish: modeling human genetic disorders using induced pluripotent cells. Nat. Med. 17, 1570–1576. doi: 10.1038/nm.2504
Topouzis, S., and Majesky, M. W. (1996). Smooth muscle lineage diversity in the chick embryo. Two types of aortic smooth muscle cell differ in growth and receptor-mediated transcriptional responses to transforming growth factor-β. Dev. Biol. 178, 430–445. doi: 10.1006/dbio.1996.0229
Touboul, T., Hannan, N. R. F., Corbineau, S., Martinez, A., Martinet, C., Branchereau, S., et al. (2010). Generation of functional hepatocytes from human embryonic stem cells under chemically defined conditions that recapitulate liver development. Hepatology 51, 1754–1765. doi: 10.1002/hep.23506
Touyz, R. M., and Schiffrin, E. L. (1997). Angiotensin II regulates vascular smooth muscle cell pH, contraction, and growth via tyrosine kinase–dependent signaling pathways. Hypertension 30, 222–229. doi: 10.1161/01.hyp.30.2.222
Trillhaase, A., Haferkamp, U., Rangnau, A., Märtens, M., Schmidt, B., Trilck, M., et al. (2018). Differentiation of human iPSCs into VSMCs and generation of VSMC-derived calcifying vascular cells. Stem Cell Res. 31, 62–70. doi: 10.1016/j.scr.2018.07.008
Tsai, M. C., Chen, L., Zhou, J., Tang, Z., Hsu, T. F., Wang, Y., et al. (2009). Shear stress induces synthetic-to-contractile phenotypic modulation in smooth muscle cells via peroxisome proliferator-activated receptor α/δ activations by prostacyclin released by sheared endothelial cells. Circ. Res. 105, 471–480. doi: 10.1161/CIRCRESAHA.109.193656
Tsai, W. C., Hsu, C. C., Chen, C. P. C., Chang, H. N., Wong, A. M. K., Lin, M. S., et al. (2010). Ciprofloxacin up-regulates tendon cells to express matrix metalloproteinase-2 with degradation of type i collagen. J. Orthop. Res. 29, 67–73. doi: 10.1002/jor.21196
Ueba, H., Kawakami, M., and Yaginuma, T. (1997). Shear stress as an inhibitor of vascular smooth muscle cell proliferation. Arterioscler. Thromb. Vasc. Biol. 17, 1512–1516. doi: 10.1161/01.atv.17.8.1512
van den Akker, J., Tuna, B. G., Pistea, A., Sleutel, A. J. J., Bakker, E. N. T. P., and van Bavel, E. (2012). Vascular smooth muscle cells remodel collagen matrices by long-distance action and anisotropic interaction. Med. Biol. Eng. Comput. 50, 701–715. doi: 10.1007/s11517-012-0916-6
van Meer, B. J., Krotenberg, A., Sala, L., Davis, R. P., Eschenhagen, T., Denning, C., et al. (2019). Simultaneous measurement of excitation-contraction coupling parameters identifies mechanisms underlying contractile responses of hiPSC-derived cardiomyocytes. Nat. Commun. 10:4325.
Volpato, V., Smith, J., Sandor, C., Ried, J. S., Baud, A., Handel, A., et al. (2018). Reproducibility of molecular phenotypes after long-term differentiation to human ipsc-derived neurons: a multi-site omics study. Stem Cell Rep. 11, 897–911. doi: 10.1016/j.stemcr.2018.08.013
Wallace, C. S., Strike, S. A., and Truskey, G. A. (2007). Smooth muscle cell rigidity and extracellular matrix organization influence endothelial cell spreading and adhesion formation in coculture. Am. J. Physiol. Heart Circ. Physiol. 293, 1978–1986. doi: 10.1152/ajpheart.00618.2007
Wang, Y., Ait-Oufella, H., Herbin, O., Bonnin, P., Ramkhelawon, B., Taleb, S., et al. (2010). TGF-β activity protects against inflammatory aortic aneurysm progression and complications in angiotensin II-infused mice. J. Clin. Invest. 120, 422–432. doi: 10.1172/JCI38136.422
Wanjare, M., Kuo, F., and Gerecht, S. (2013). Derivation and maturation of synthetic and contractile vascular smooth muscle cells from human pluripotent stem cells. Cardiovasc. Res. 97, 321–330. doi: 10.1093/cvr/cvs315
Warren, C. R., O’Sullivan, J. F., Friesen, M., Becker, C. E., Zhang, X., Liu, P., et al. (2017). Induced pluripotent stem cell differentiation enables functional validation of GWAS variants in metabolic disease. Cell Stem Cell 20, 547–557.e7. doi: 10.1016/j.stem.2017.01.010
Wasteson, P., Johansson, B. R., Jukkola, T., Breuer, S., Akydsurek, L. M., Partanen, J., et al. (2008). Developmental origin of smooth muscle cells in the descending aorta in mice. Development 135, 1823–1832. doi: 10.1242/dev.020958
Weber, E., Rossi, A., Solito, R., Sacchi, G., Agliano’, M., and Gerli, R. (2002). Focal adhesion molecules expression and fibrillin deposition by lymphatic and blood vessel endothelial cells in culture. Microvasc. Res. 64, 47–55. doi: 10.1006/mvre.2002.2397
Wei, H., Hu, J. H., Angelov, S. N., Fox, K., Yan, J., Enstrom, R., et al. (2017). Aortopathy in a mouse model of Marfan syndrome is not mediated by altered transforming growth factor β signaling. J. Am. Heart Assoc. 6:e004968. doi: 10.1161/JAHA.116.004968
Weinberg, C. B., and Bell, E. (1986). A blood vessel model constructed from collagen and cultured vascular cells. Science 231, 397–400. doi: 10.1126/science.2934816
Whiteman, P., and Handford, P. A. (2003). Defective secretion of recombinant fragments of fibrillin-1: implications of protein misfolding for the pathogenesis of Marfan syndrome and related disorders. Hum. Mol. Genet. 12, 727–737. doi: 10.1093/hmg/ddg081
Williams, J. A., Loeys, B. L., Nwakanma, L. U., Dietz, H. C., Spevak, P. J., Patel, N. D., et al. (2007). Early surgical experience with loeys-dietz: a new syndrome of aggressive thoracic aortic aneurysm disease. Ann. Thorac. Surg. 83, S757–S763. doi: 10.1016/j.athoracsur.2006.10.091
Wise, S. G., Byrom, M. J., Waterhouse, A., Bannon, P. G., Weiss, A. S., and Ng, M. K. C. (2011). A multilayered synthetic human elastin/polycaprolactone hybrid vascular graft with tailored mechanical properties. Acta Biomater. 7, 295–303. doi: 10.1016/j.actbio.2010.07.022
Xie, C. Q., Zhang, J., Villacorta, L., Cui, T., Huang, H., and Chen, Y. E. (2007). A highly efficient method to differentiate smooth muscle cells from human embryonic stem cells [2]. Arterioscler. Thromb. Vasc. Biol. 27, 311–312. doi: 10.1161/ATVBAHA.107.154260
Xiong, W., Gong, J., Chen, Y. E., and Yang, B. (2017). Abstract 19200: a highly efficient in vitro smooth muscle cells differentiation system from human pluripotent stem cells-derived neural crest stem cells. Circulation 136:A19200.
Xiong, W., Meisinger, T., Knispel, R., Worth, J. M., and Baxter, B. T. (2012). MMP-2 Regulates Erk1/2 phosphorylation and aortic dilatation in Marfan syndrome. Circ. Res. 110, 92–101. doi: 10.1161/CIRCRESAHA.112.268268
Yeung, K. K., Bogunovic, N., Keekstra, N., Beunders, A. A. M., Pals, J., van der Kuij, K., et al. (2017). Transdifferentiation of human dermal fibroblasts to smooth muscle-like cells to study the effect of MYH11 and ACTA2 mutations in aortic aneurysms. Hum. Mutat. 38, 439–450. doi: 10.1002/humu.23174
Yu, K., Zheng, B., Han, M., and Wen, J. K. (2011). ATRA activates and PDGF-BB represses the SM22α promoter through KLF4 binding to, or dissociating from, its cis-DNA elements. Cardiovasc. Res. 90, 464–474. doi: 10.1093/cvr/cvr017
Zhang, H., Xiong, Z. M., and Cao, K. (2014). Mechanisms controlling the smooth muscle cell death in progeria via down-regulation of poly(ADP-ribose) polymerase 1. Proc. Natl. Acad. Sci. U.S.A. 111, E2261–E2270. doi: 10.1073/pnas.1320843111
Zhang, J., Lian, Q., Zhu, G., Zhou, F., Sui, L., Tan, C., et al. (2011). A human iPSC model of hutchinson gilford progeria reveals vascular smooth muscle and mesenchymal stem cell defects. Cell Stem Cell 8, 31–45. doi: 10.1016/j.stem.2010.12.002
Zhang, J., McIntosh, B. E., Wang, B., Brown, M. E., Probasco, M. D., Webster, S., et al. (2019). A human pluripotent stem cell-based screen for smooth muscle cell differentiation and maturation identifies inhibitors of Intimal hyperplasia. Stem Cell Rep. 12, 1269–1281. doi: 10.1016/j.stemcr.2019.04.013
Zhang, S., Liu, X., Bawa-Khalfe, T., Lu, L.-S., Lyu, Y. L., Liu, L. F., et al. (2012). Identification of the molecular basis of doxorubicin-induced cardiotoxicity. Nat. Med. 18, 1639–1642. doi: 10.1038/nm.2919
Zhang, Y. E. (2017). Non-Smad signaling pathways of the TGF-β family. Cold Spring Harb. Perspect. Biol. 9:a022129. doi: 10.1101/cshperspect.a022129
Zhu, J.-H., Chen, C.-L., Flavahan, S., Harr, J., Su, B., and Flavahan, N. A. (2011). Cyclic stretch stimulates vascular smooth muscle cell alignment by redox-dependent activation of Notch3. Am. J. Physiol. Heart Circ. Physiol. 300, H1770–H1780. doi: 10.1152/ajpheart.00535.2010
Zhu, L., Vranckx, R., Van Kien, P. K., Lalande, A., Boisset, N., Mathieu, F., et al. (2006). Mutations in myosin heavy chain 11 cause a syndrome associating thoracic aortic aneurysm/aortic dissection and patent ductus arteriosus. Nat. Genet. 38, 343–349. doi: 10.1038/ng1721
Keywords: induced pluripotent stem cell, aortic aneurysm, Marfan, Loeys-Dietz, vascular smooth muscle, disease-in-a-dish
Citation: Davaapil H, Shetty DK and Sinha S (2020) Aortic “Disease-in-a-Dish”: Mechanistic Insights and Drug Development Using iPSC-Based Disease Modeling. Front. Cell Dev. Biol. 8:550504. doi: 10.3389/fcell.2020.550504
Received: 09 April 2020; Accepted: 08 October 2020;
Published: 28 October 2020.
Edited by:
Masayuki Yazawa, Columbia University, United StatesReviewed by:
Zi-Bing Jin, Capital Medical University, ChinaCopyright © 2020 Davaapil, Shetty and Sinha. This is an open-access article distributed under the terms of the Creative Commons Attribution License (CC BY). The use, distribution or reproduction in other forums is permitted, provided the original author(s) and the copyright owner(s) are credited and that the original publication in this journal is cited, in accordance with accepted academic practice. No use, distribution or reproduction is permitted which does not comply with these terms.
*Correspondence: Sanjay Sinha, c3M2NjFAY2FtLmFjLnVr
Disclaimer: All claims expressed in this article are solely those of the authors and do not necessarily represent those of their affiliated organizations, or those of the publisher, the editors and the reviewers. Any product that may be evaluated in this article or claim that may be made by its manufacturer is not guaranteed or endorsed by the publisher.
Research integrity at Frontiers
Learn more about the work of our research integrity team to safeguard the quality of each article we publish.