- 1Ministry of Education Key Laboratory of Biosystems Homeostasis and Protection and Innovation Center for Cell Signaling Network, Life Sciences Institute, Zhejiang University, Hangzhou, Zhejiang, China
- 2The Second Affiliated Hospital, School of Medicine, Zhejiang University, Hangzhou, Zhejiang, China
Endoplasmic reticulum–mitochondria contact sites (ERMCSs) are dynamic contact regions with a distance of 10–30 nm between the endoplasmic reticulum and mitochondria. Endoplasmic reticulum–mitochondria contact sites regulate various biological processes, including lipid transfer, calcium homeostasis, autophagy, and mitochondrial dynamics. The dysfunction of ERMCS is closely associated with various neurodegenerative diseases, including Parkinson’s disease, Alzheimer’s disease, and amyotrophic lateral sclerosis. In this review, we will summarize the current knowledge of the components and organization of ERMCSs, the methods for monitoring ERMCSs, and the physiological functions of ERMCSs in different model systems. Additionally, we will emphasize the current understanding of the malfunction of ERMCSs and their potential roles in neurodegenerative diseases.
Introduction
Endoplasmic reticulum (ER) forms interconnected networks of membrane tubules and sacs that play a major role in the synthesis, modification, and transport of proteins and lipids in the eukaryotic cells (Schwarz and Blower, 2016). Mitochondrion serves as a powerhouse and metabolic center for the production of ATP and precursors of macromolecules, such as proteins, lipids, DNA, and RNA (Devine and Kittler, 2018). The contact sites between ER and mitochondria are not only critical for the communications between these two important organelles but also serve as a platform to regulate other cellular events (Cohen et al., 2018). Since the early 1970s, ER–mitochondria contact sites (ERMCSs) have been observed through ultrastructural electron microscopy. The subcellular fractionation studies also indicated a close association between the membranes of ER and mitochondria. Although ERMCSs are conserved structures in all eukaryotic cells, the tethering molecules are diverse across species. Recent studies have developed many methods to monitor ERMCS and identified multiple tethering complexes that mediate ERMCS formation. Previous studies have extensively investigated the functions of ERMCS at the cellular levels. Several diseases, including neurogenerative disorders, are associated with abnormal ERMCSs (Paillusson et al., 2016). Since there are many high-quality reviews published recently, we will briefly give an introduction to the organization and functions of ERMCSs in this review (Phillips and Voeltz, 2016; Lackner, 2019; Moltedo et al., 2019).
The Organization of ERMCSs
In the last few decades, several pairs of protein complexes have been identified that bridge the ER and mitochondria. The mitochondria and ER membrane components mediate the formation of ERMCSs with the help of some cytosolic proteins to promote the exchange of cellular components and signals between the ER and mitochondria (Figure 1).
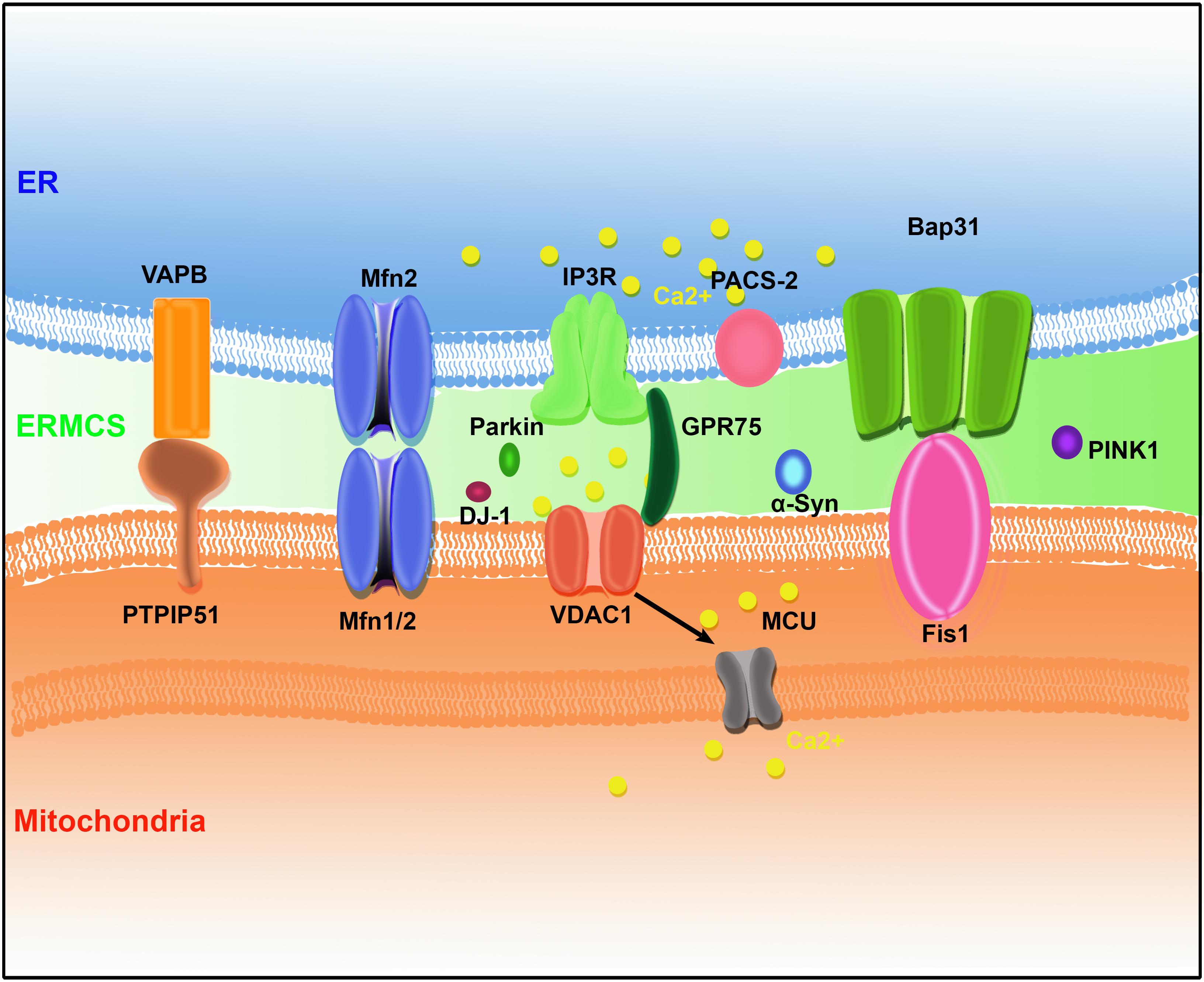
Figure 1. The protein organization at ER–mitochondria contact sites (ERMCSs) in mammalian systems. Several pairs of proteins located on mitochondria and ER surface to form tethers, including vesicle-associated membrane protein (VAMP)-associated protein (VAP) B (VAPB)–protein tyrosine phosphatase-interacting protein-51 (PTPIP51), Mfn1/2, inositol 1,4,5-trisphosphate receptor (IP3R)–glucose-regulated protein 75 (GPR75)–voltage-dependent anion channel (VDAC1) complex, and B-cell receptor-associated protein 31 (Bap31) (Bap31)–fission 1 homolog (Fis1). Neurodegenerative disease-related proteins such as α-synuclein (α-Syn), DJ-1, PINK1, and Parkin were concentrated at ERMCSs.
The Tethering Molecules in Yeast
ER–mitochondria encounter structure (ERMCS), a protein complex in yeast, was reported to mediate the contacts between ER and mitochondria. The mitochondrial outer membrane proteins (Mdm10 and Mdm34), cytosolic protein (Mdm12), and integral ER protein (Mmm1) mediate the tethering between mitochondria and ER to form ERMES. Gem1, the yeast ortholog of Miro, is enriched in the contact sites and regulates ERMES (Kornmann et al., 2011). Additionally, the ER membrane protein complex (EMC) interacts with the mitochondrial translocase of outer membrane (TOM) complex to tether ER to the mitochondria (Lahiri et al., 2014). In contrast to ERMES, EMC is conserved in higher organisms. The role of mammalian EMC in tethering ER to the mitochondria is not known. Ltc1/Lam6 is also reported to regulate the contact between the ER and mitochondria in yeast (Elbaz-Alon et al., 2015; Murley et al., 2015). Ltc1/Lam6 is conserved among the mammalian cells. However, the role of mammalian orthologs of Ltc1/Lam6 in ERMCSs is not known.
The Tethering Molecules in Mammals
Mitofusin 2
In mammals, several tether molecules are reported to mediate the formation of ERMCSs. Mitofusin 2 (MFN2) is a dynamin-like GTPase that is located not only on the mitochondrial outer membrane but also is enriched in the interface between ER and mitochondria. MFN2 can form a homotypic dimer and a heterotypic dimer with its paralog MFN1, which plays a major role in the mitochondrial outer membrane fusion. In 2008, MFN2 was reported to tether ER to the mitochondria and to regulate mitochondrial calcium uptake from ER. Mfn2 ablation in the mouse embryonic fibroblasts (MEFs) and HeLa cells increases the distance between ER and mitochondria and decreases the calcium transfer from ER to the mitochondria (De Brito and Scorrano, 2008). Several groups have reported new components that regulate ER–mitochondrial tethering via MFN2 (Cerqua et al., 2010; Sugiura et al., 2013; Daniele et al., 2014; Morales et al., 2014). However, later studies have challenged the role of MFN2 in mediating the formation of ERMCS. The loss of Mfn2 does not decrease the formation of ERMCS but increases both ERMCS formation and calcium trafficking between the ER and mitochondria (Cosson et al., 2012; Filadi et al., 2016; Leal et al., 2016). This indicated that MFN2 serves as an antagonist to ERMCS formation. Further studies are needed to elucidate the role of MFN2 in tethering ER to the mitochondria.
VAPB and PTPIP51
Vesicle-associated membrane protein (VAMP)-associated proteins (VAPs) are integral ER membrane proteins that contain an N-terminal major sperm protein (MSP) domain, a central coiled-coil region, and a C-terminal transmembrane domain. The vertebrates have two VAPs (VAPA and VAPB). Mutations in VAPB cause rare forms of spinal muscular atrophy (SMA) and amyotrophic lateral sclerosis 8 (ALS8) in patients. VAPs play an important role in membrane trafficking, lipid transfer and metabolism, unfolded protein response (UPR), and autophagy (Kaiser et al., 2005; Lev et al., 2008; Zhao et al., 2018). VAPs interact with various proteins containing the FFAT-motif, which comprises consensus EFFDAXE amino acid sequence and serves as a versatile access point for the ER (Murphy and Levine, 2016). Several FFAT-motif-containing proteins mediate the contact between ER and other organelles. Protein tyrosine phosphatase-interacting protein-51 (PTPIP51) is a mitochondrial outer membrane protein containing an FFAT-motif that binds to VAPB (De Vos et al., 2012). Overexpression of either VAPB or PTPIP51 increases the ERMCS formation and calcium transfer from ER to mitochondria. The RNA interference (RNAi)-mediated silencing of VAPB or PTPIP51 or overexpression of ALS mutant form of VAPB (VAPBP56S) decreases the ERMCS formation and disturbs the calcium exchange between these two organelles (Stoica et al., 2014). TDP-43 and FUS are two proteins that are pathologically linked to ALS and frontotemporal dementia (FTD). TDP-34 and FUS modulate the interaction between VAPB and PTPIP51 via the activation of GSK-3β protein kinase and therefore perturbs ERMCS (Stoica et al., 2014; Stoica et al., 2016). The Parkinson’s disease (PD)-related protein, α-synuclein, binds to VAPB and disrupts the interaction between VAPB and PTPIP51, which leads to the loss of contact between ER and mitochondria (Paillusson et al., 2017).
B-Cell Receptor-Associated Protein 31 and Its Binding Partners
B-cell receptor-associated protein 31 (Bap31) is an integral ER membrane protein containing an N-terminal membrane-bound region with three predicted transmembrane helices and a cytosolic C-terminal domain with one or two predicted coiled coils (Iwasawa et al., 2011). During apoptosis, the mitochondrial fission protein, fission 1 homolog (Fis1) interacts with Bap31 to bridge the ER and mitochondria and promotes the caspase-8-mediated cleavage of Bap31 into the pro-apoptotic p20Bap31 (Chandra et al., 2004). The ablation of phosphofurin acidic cluster sorting protein 2 (PACS-2), a multifunctional ER-associated vesicular sorting protein, leads to Bap31-dependent mitochondrial fragmentation and uncoupling of the ER from the mitochondria (Simmen et al., 2005). However, further studies are needed to confirm whether PACS-2 functions as a component of the Fis1–Bap31 complex or as a regulator of Fis1–Bap31 interaction.
Bap31 also interacts with Bcl-2, which is localized in the mitochondria. The interaction between Bap31 and Bcl-2 is facilitated by the interaction between CDIP1 and Bap31 in the ER during ER-stress (Namba et al., 2013).
A recent study reported that Bap31 could also form an ER–mitochondrial bridging complex by interacting with translocase of the outer mitochondrial membrane 40 (Tom40). The Bap31–Tom40 complex facilitates nuclear-encoded mitochondrial protein translocation and mitochondrial homeostasis (Namba, 2019).
VDAC1–Grp75–IP3R
The voltage-dependent anion channel (VDAC), which is located at the mitochondrial outer membrane, is a key component that mediates calcium transport to the mitochondria (Rapizzi et al., 2002). Voltage-dependent anion channel physically interacts with inositol 1,4,5-trisphosphate receptor (IP3R), the ER calcium release channel, through the molecular chaperone glucose-regulated protein 75 (Grp75). These tripartite complexes colocalize on the mitochondrial-associated membranes (MAMs) and directly enhance the mitochondrial calcium uptake (Szabadkai et al., 2006). This suggested that the VDAC1–Grp75–IP3R complex may serve as an ER–mitochondria tether. The silencing of Grp75 abolishes the mitochondrial calcium uptake. Transglutaminase type 2 (TG2) interacts with Grp75 in the MAMs. Disrupting the TG2–Grp75 interaction decreases the number of ER–mitochondria contact sites (D’eletto et al., 2018). Sigma-1 receptor (Sig-1R) binds to IP3R at MAM to regulate ER–mitochondrial interorganellar calcium signaling (Hayashi and Su, 2007). Other modulators, such as mitochondrial calcium uniporter (MCU; Xu et al., 2018) and etoposide-induced protein 2.4 (EI24; Yuan et al., 2019), were also reported to regulate the VDAC1–Grp75–IP3R scaffolds. However, IP3R ablation does not affect ER–mitochondria contacts, which indicates that VDAC1–Grp75–IP3R scaffold is not involved in physical tethering (Csordas et al., 2006).
The Functions of ERMCSs
Endoplasmic reticulum–mitochondria contact sites regulate a variety of biological processes, including calcium homeostasis, lipid transfer, autophagy, and mitochondrial dynamics (Figure 2).
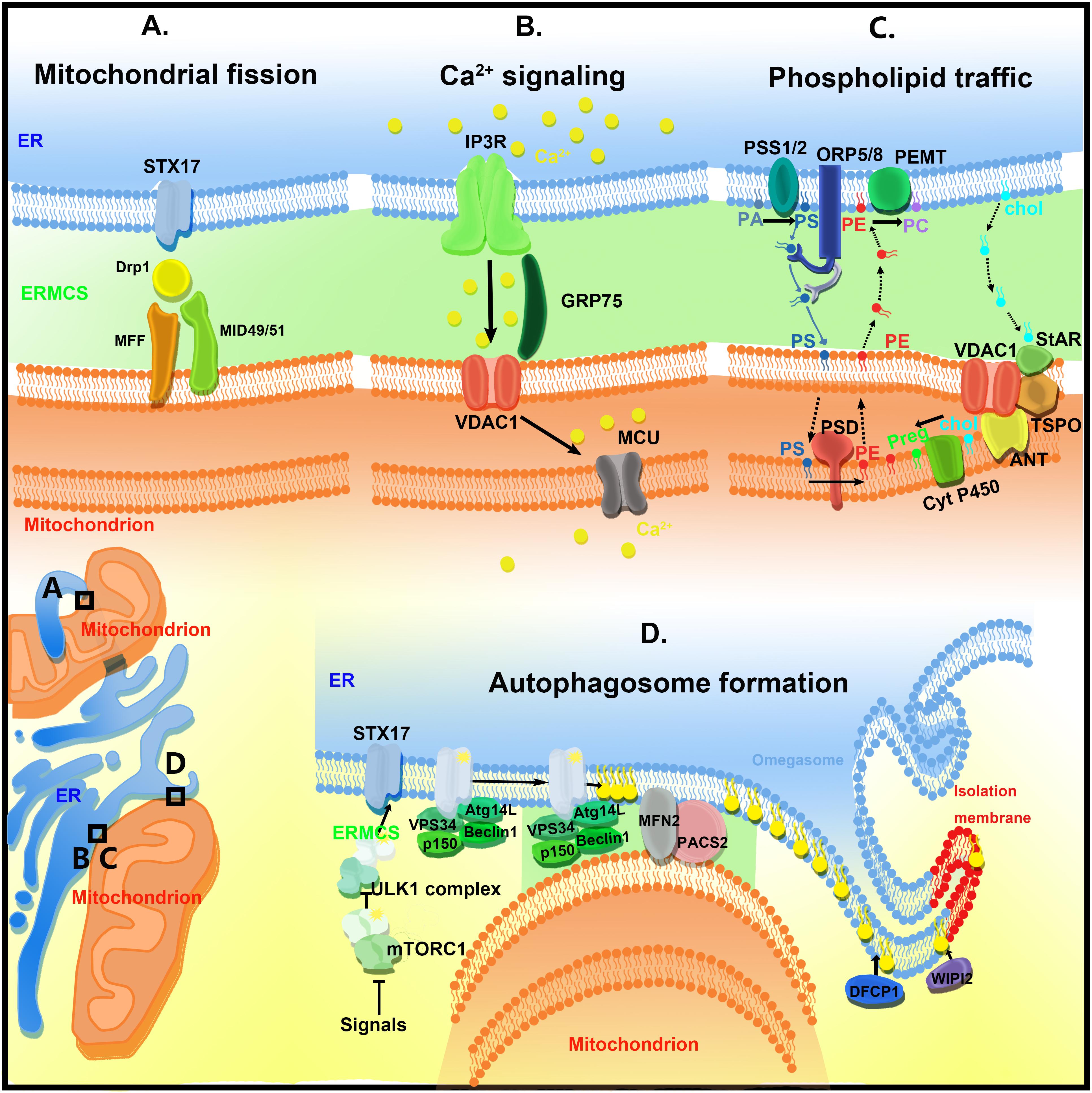
Figure 2. The functions of ER–mitochondria contact sites (ERMCSs). The typical ERMCSs are illustrated at the bottom left corner. The boxed regions are enlarged to show the detailed cellular events and molecular organizations. (A) Mitochondria fission occurs at the ERMCSs. ER-located protein Syntaxin 17 (STX17) presents at ERMCS and mediates mitochondrial division by determining the localization and activity of Drp1. (B) The VDAC1–Grp75–IP3R tripartite complex serves as the major platform that coordinates calcium transfer between ER and mitochondria. Mitochondrial inner membrane located mitochondrial calcium uniporter (MCU) takes up the calcium transported from VDAC1. (C) The ERMCSs form the platform for phospholipid traffic between ER and mitochondria. (D) The pre-autophagosome/autophagosome proteins re-localize to the ERMCS to promote the initiation of autophagosome formation.
Regulation of Mitochondrial Dynamics
Mitochondria are highly dynamic organelles that undergo fusion and fission to maintain their healthy state. Interestingly, the ER tubules define the position of mitochondrial fission sites (Friedman et al., 2011). In both yeast and mammalian cells, mitochondrial division initially occurs at the ER-mitochondrial contact sites, and the ER–mitochondrial contacts are formed before the recruitment of fission machinery proteins, such as Drp1 and Mff (Friedman et al., 2011). Inverted formin 2 (INF2), an ER-located protein, regulates actin polymerization and drives the mitochondrial constriction and division in the mammalian cells (Korobova et al., 2013). Syntaxin 17 is located on the tubular, smooth ER membranes. Syntaxin 17 is involved in the ER–Golgi intracellular trafficking and autophagy. Recently, Syntaxin 17 was reported to be present at the ER–mitochondrial contact sites and to mediate mitochondrial division by determining the localization and activity of Drp1 (Arasaki et al., 2015). Sept2, a subset of proteins localized to the mitochondrial constrictions, was reported to physically bind to Drp1 and to mediate Drp1-dependent mitochondrial division in the mammalian cells (Pagliuso et al., 2016). The contact sites not only mark the mitochondrial fission sites but also mark the replication sites of mtDNA (Lewis et al., 2016). The mtDNA is localized in the matrix. Thus, it would be interesting to determine the communication between ERMCS and mtDNA replication machinery in the mitochondrial matrix. The results of high-resolution microscopy imaging analysis indicated that some mitochondrial fusion events also occur at the ERMCSs (Guo et al., 2018). However, the molecular mechanisms underlying this process are still elusive.
Calcium Transfer
ER lumen is the major calcium store in the mammalian cells. The ER calcium level is determined by sarco-ER Ca2+ transport ATPases (SERCAs), intraluminal calcium-binding proteins, such as calreticulin, calnexin (CNX), and BiP/GRP78. SERCA2b is a ubiquitously expressed isoform of SERCAs that is critical for ER calcium uptake. Calnexin and thioredoxin-related transmembrane protein 1 (TMX1) regulate SERCA2b activity. Calnexin is enriched at the ER luminal side of the ERMCSs through palmitoylation and interaction with PACS-2. Transmembrane protein 1 belongs to the family of protein disulfide isomerases. Transmembrane protein 1 has a single transmembrane domain and is palmitoylated at the cytosolic stretch, which is required for its targeting to the ERMCS (Lynes et al., 2012). Transmembrane protein 1 inhibits the activity of SERCA2b, which can be antagonized by CNX, the positive regulator of SERCA2b. The loss of TMX1 increases the ER calcium store.
Upon stimulation, calcium is released to the cytosol through IP3R on the ER membrane. The external signals stimulate the production of Ins(1,4,5)P3 that binds and activates IP3R and triggers the calcium release from ER to the cytosol and enhances the local calcium concentration. The calcium flux from ER to mitochondria is critical for multiple mitochondrial functions. Basal calcium oscillation in mitochondria is required for multiple metabolic processes (Gellerich et al., 2010). The calcium overload in the mitochondria leads to the opening of the mitochondrial permeability transition pore and subsequentially results in cell death (Baumgartner et al., 2009). As the MCU complex has low calcium affinity, ER and mitochondria must be in close proximity for producing high local calcium concentration. Thus, ERMCS is a hot spot for calcium transfer between the ER and mitochondria.
IP3R, which is enriched in the ERMCS, interacts with VDAC1 located at the mitochondrial outer membrane via a chaperone, Grp75. The resulting VDAC1–Grp75–IP3R complex serves as the major platform and chaperone complexes that coordinate calcium transfer between ER and mitochondria (Bononi et al., 2012). MCU localized at the mitochondrial inner membrane takes up the calcium transported from VDAC1. When ER calcium level is low, Sig-1R is released from BIP/GPR78 to stabilize IP3R3 at ERMCSs and promote prolonged ER calcium release (Hayashi and Su, 2007; Figure 2B).
Lipid Synthesis and Exchange
The cellular transport and homeostasis are dependent on the extensive transport of lipids and their precursors between ER and mitochondria (Osman et al., 2011; Figure 2C). Endoplasmic reticulum is a major membrane lipid synthesis center in the cells. The mitochondrial membrane has high levels of phospholipid and low levels of sterols and sphingolipids. Sphingolipids and phospholipids, such as phosphatidic acid (PA), phosphatidylserine (PS), phosphotidylcholine (PC), and phosphatidylinositol (PI), are synthesized in the ER. Cardiolipin (CL), a diglycerophospholipid, is a mitochondrial-specific phospholipid enriched in the inner mitochondrial membrane. Cardiolipin is synthesized in the mitochondria at the matrix side of the inner membrane using PA transported from the ER (Tatsuta et al., 2014). Phosphatidylserine is converted to phosphatidylethanolamine (PE) by PS decarboxylase (PSD) on the inner mitochondrial membrane. The mitochondrion-derived PE is transferred back to the ER, where it serves as a precursor for synthesizing PC. As mitochondria are not integrated into the classical vesicular trafficking routes, non-vesicular mediated transports that occur at the contact sites of membranes play major roles in the lipid transport between the ER and mitochondria. Several key enzymes mediate phospholipid synthesis, such as phosphatidylserine synthase-1/2 (PSS1/2) and phosphatidylethanolamine N-methyltransferase 2 (PEMT2), an enzyme implicated in PC synthesis. These enzymes are localized to the ERMCSs (Cui et al., 1993). In yeast, ERMES-deficient mitochondria exhibit an altered membrane lipid composition and an impaired conversion of PS to PC (Kornmann et al., 2009). However, there is no direct evidence to show that ERMES participates in the lipid transfer directly. The EMC complex in yeast is also involved in PS shuttling from ER to mitochondria (Lahiri et al., 2014). However, the EMC proteins do not have a lipid-binding domain and, therefore, may regulate lipid transfer indirectly. In mammals, further studies are needed to elucidate the mechanism underlying lipid transfer at ERMESs. Oxysterol-binding protein (OSBP)-related protein 5 and 8 (ORP5/ORP8) were recently reported to be localized at the ERMCSs and to potentially mediate the transport of phospholipid (probably PS) between two organelles (Galmes et al., 2016).
In addition to phospholipid, key regulators of triacylglycerol synthesis and steroidogenesis, such as acyl-CoA/diacylglycerol acyltransferase 2 (DGAT2; Stone et al., 2009) and steroidogenic acute regulatory protein (StAR; Prasad et al., 2015) are enriched in the ERMCSs. Long-chain-fatty-acid-CoA ligase 4 (FACL4; Lewin et al., 2001), an enzyme that mediates the ligation of fatty acids to coenzyme A (CoA), and acyl-coenzyme A:cholesterol acyltransferase-1 (ACAT1/SOAT1), an enzyme that catalyzes the generation of cholesterol esters, is also enriched in ERMCSs (Lewin et al., 2002). These findings indicate that the ERMCSs are closely related to lipid exchange between the ER and mitochondria, and lipid metabolism.
Regulation of Autophagy Process
Autophagy is an intracellular bulk degradation process, which is tightly regulated. Autophagy dysfunction is closely associated with numerous human diseases (Mizushima and Komatsu, 2011). The initiation of autophagy involves the formation of isolation membranes, which engulf some cytosolic components and damaged organelles. The isolation membranes are sealed to form double-membraned autophagosomes, which fuse with the lysosomes where the contents are degraded. Although the ER, mitochondria, and plasma membrane are reported to contribute to the original autophagosome membrane, the origin of the autophagosomal membranes is still controversial. In 2013, Hamasaki et al. (2013) demonstrated that ERMCSs mediate autophagosome formation. Upon starvation, the pre-autophagosome/autophagosome markers, ATG14 and ATG6, re-localize to the ERMCS to initiate autophagosome formation. The disruption of the ERMCSs decreases the number of ATG14-positive autophagosomes. Meanwhile, Syntaxin 17 is redistributed to ERMCSs to mediate PI3–kinase complex recruitment by physically binding to ATG14. PTPIP51 is also reported to bind to VAPB and to regulate autophagy (Gomez-Suaga et al., 2017). Increasing ERMCS formation by overexpressing VAPB or PTPIP51 impairs autophagosome formation. Conversely, decreasing the expression of VAPB or PTPIP51 stimulates autophagy. These data indicate that ERMCS is a hot spot to regulate the autophagy process (Figure 2D).
ERMCSs and Neurodegeneration
ERMCSs have attracted widespread attention in neurodegenerative diseases, mainly because they are widely involved in processes closely related to mitochondrial function and cell survival. Several neurodegenerative disease-associated proteins are enriched in the ER–mitochondrial interface. Several studies have demonstrated that ERMCSs, which are the key regulators of lipid metabolism, Ca2+ homeostasis, and autophagosome formation, are affected by pathogenic mutations (Figure 3).
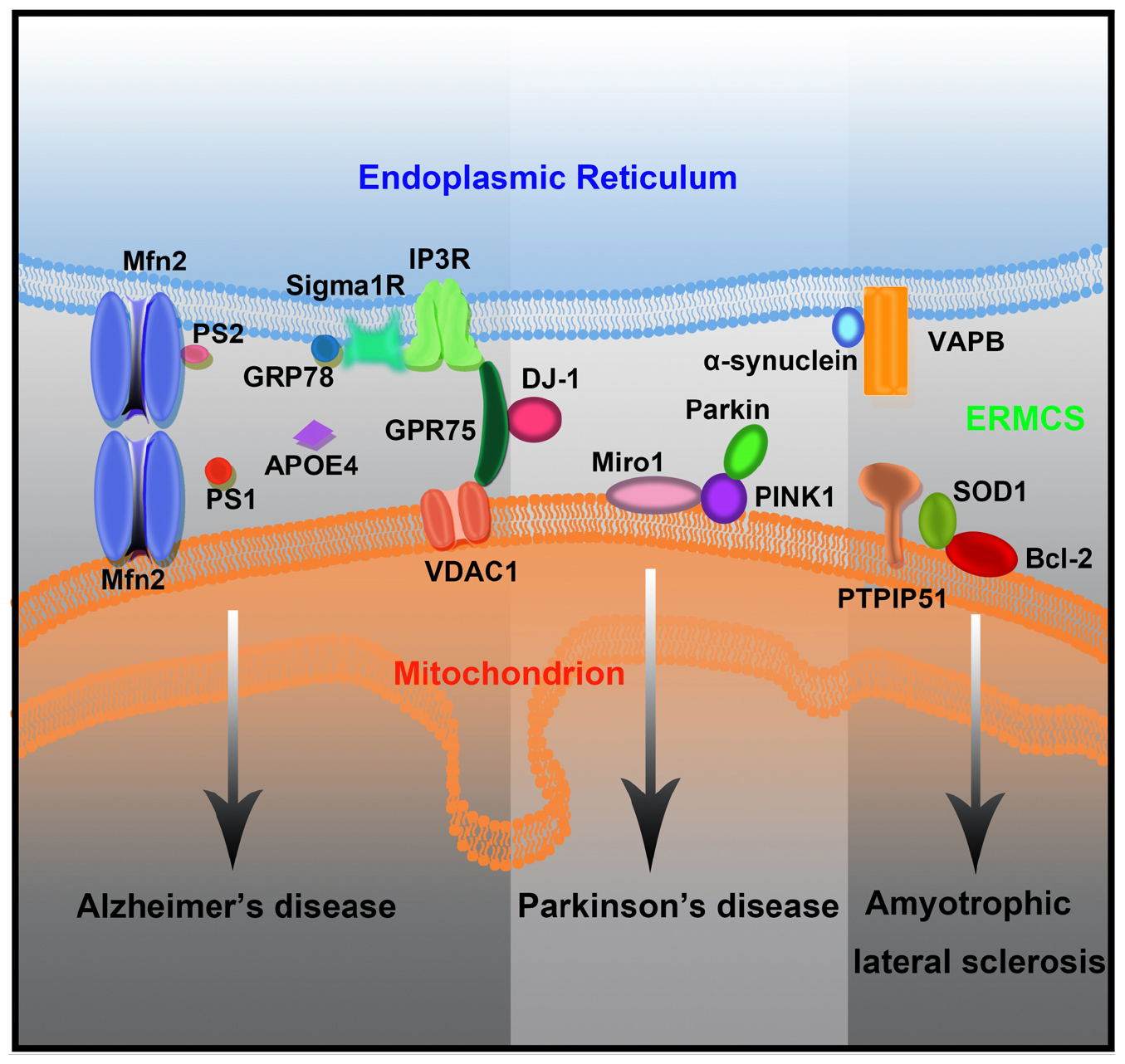
Figure 3. The mutant forms of neurodegenerative disease-associated protein lead to ERMCS defects and finally neuronal death.
Alzheimer’s Disease
Familial cases of Alzheimer’s disease (AD) are rare and are caused due to the mutations in the amyloid precursor protein (APP) or presenilins (PS1 and PS2). The APP Swe/Lon mouse model exhibit enhanced ER–mitochondria connection and enhanced mitochondrial calcium concentrations without marked changes in the lipid composition (Hedskog et al., 2013).
PS1 and PS2 are components of the γ-secretase complex, which is involved in APPβ processing (Naj and Schellenberg, 2017). Both PS1 and PS2 are enriched in the mitochondria-associated ER membranes (Area-Gomez et al., 2009). The mutations in PS2 increase ERMCS formation and enhance cholesteryl ester and phospholipid synthesis in the cellular models (Zampese et al., 2011; Area-Gomez et al., 2012). Additionally, PS2 mutations increase the ER–mitochondria connection in the fibroblasts of patients with sporadic AD (Area-Gomez et al., 2012). Although mutations in PS1 and PS2 can enhance cholesteryl ester and phospholipid synthesis, only PS2 can modulate the Ca2+ shuttling between ER and mitochondria (Zampese et al., 2011).
Sig-1R forms a Ca2+-sensitive chaperone complex with Bip/GRP78 and promotes Ca2+ release from the ER by stabilizing IP3R3. It has been proposed that Sig-1R forms part of the endogenous defense system against AD (Maurice and Goguadze, 2017). Reportedly, compounds with Sig-1R agonist activity possess neuroprotective abilities. These findings indicate that the activity of Sig-1R may prevent AD pathology, therefore, presenting a promising therapeutic target for AD (Jia et al., 2019).
Individuals carrying the ε4 allele of apolipoprotein E (ApoE4) have increased risk for developing AD compared to the ones carrying ApoE3, the most common isoform. Cells treated with ApoE4 containing astrocyte conditioned media (ACM) have increased synthesis of phospholipids and of cholesteryl esters compared to those treated with ApoE3 containing ACM, suggesting an upregulated ERMCS functions in the cells treated with ApoE4 containing ACM (Tambini et al., 2016).
Although most evidence illustrates that enhanced ERMCS activity is associated with AD, conflicting results have been suggested in other studies. It has been demonstrated that expressing a linker that can force contacts between mitochondria and the ER suppressed motor impairment and extended the lifespan in a Drosophila model of AD (Garrido-Maraver et al., 2020).
Parkinson’s Disease
Parkinson’s disease is a progressive movement disorder with selective loss of dopaminergic (DA) neurons in the substantia nigra and accumulation of Lewy bodies consisting of α-synuclein aggregates in the patient’s brains. Most PD cases are sporadic, and less than 10% are familial cases caused by genetic mutations in genes, such as SNCA, LRRK2, VPS35, PINK1, PARK2, and PARK7 (Liu et al., 2019).
α-Synuclein, a presynaptic protein encoded by SNCA, is detected in the ERMCSs. In HeLa cells, PD-associated synuclein mutations decreased ER–mitochondria connections, as well as phospholipid synthesis (Guardia-Laguarta et al., 2014). α-Synuclein binds to the ERMCS tether protein VAPB. The VAPB–PTPIP51 tethers were disrupted when either the wild type or the PD-associated mutant forms of α-synuclein were overexpressed. A similar disruption of the VAPB–PTPIP51 interaction was also observed in neurons derived from induced pluripotent stem cells from familial PD patients with an affected SNCA gene. Hence, the contacts between the ER and mitochondria were loosened, and Ca2+ signaling at neuronal ERMCSs was affected (Paillusson et al., 2017).
DJ-1 is a conserved multifunctional protein encoded by PARK7. Loss of DJ-1 leads to early-onset recessive familial PD. DJ-1 is enriched in ERMCSs and interacts with the VDAC1–Grp75–IP3R complex (Liu et al., 2019). In HeLa cells, overexpression of DJ-1 enhanced ER–mitochondrial calcium transfer and marginally increased ERMCSs (Ottolini et al., 2013). DJ-1 ablation disrupted the formation of the VDAC1–Grp75–IP3R complex, causing IP3R accumulation at ERMCSs. Furthermore, similar ERMCS defects were observed in the brain of DJ-1 knockout mice and sporadic PD patients. In DJ-1 knockout M17 cell lines, the length of ERMCSs was greatly reduced, and the ER–mitochondria calcium transfer was significantly decreased (Liu et al., 2019).
PARK2 is an important gene whose mutation is responsible for 50% of familial autosomal recessive PD cases and probably some sporadic PD cases (Wang et al., 2010). PARK2 encodes an E3 ligase Parkin that play critical roles in mitophagy. Calì et al. demonstrated that the upregulation of PARK2 can enhance the mitochondrial Ca2+ uptake from ER (Cali et al., 2013). Consistent with this study, Basso et al. observed that the tether between the ER and mitochondria decreased in PARK2 mutant human fibroblasts. Furthermore, the locomotor deficit in the Drosophila model of PD can be rescued by expressing an ER–mitochondria synthetic linker to increase ER–mitochondria tethering (Basso et al., 2018). However, Gautier et al. revealed that the ER and mitochondria are closely associated and the ER–mitochondria Ca2+ transfer was enhanced in the primary cells from patients with PD presenting PARK2 mutations (Gautier et al., 2016). The rationale underlying the differences in these findings remain unclear.
PINK1 is a protein kinase necessary for the recruitment of Parkin to the damaged mitochondria during mitophagy. In DA neurons with PINK1 mutations, ERMCSs were strengthened, and the mitochondrial Ca2+ level was increased. Miro, a protein well known for axonal transport of mitochondria, mediated the effects of PINK1 on mitochondrial calcium and morphology. Miro overexpression mimicked PINK1 mutant-induced mitochondrial Ca2+ elevation, which could be rescued by inhibiting the genes involved in calcium transfer in ERMCSs. Inhibition of Miro or components of ERMCSs rescued the defects induced by the PINK1 mutation. Surprisingly, the Miro-mediated calcium transfer was independent of its mitochondrial transport activity (Lee et al., 2018). Recently, RHOT1, the gene coding for Miro1, was found to carry mutations in patients with PD. The structure and number of ERMCSs were altered, and Ca2+ homeostasis was impaired in patient-derived fibroblasts carrying RHOT1 mutants (Berenguer-Escuder et al., 2019).
Amyotrophic Lateral Sclerosis
Amyotrophic lateral sclerosis is a fatal late-stage neurodegenerative disorder, characterized by progressive loss of motor neurons, muscle weakness, spasms, and death within a few years of diagnosis. Over 90% of ALS cases occur sporadically, and familial cases of ALS are rare. Furthermore, approximately 15% of ALS present with frontotemporal lobar dementia (ALS/FTD). Familial ALS is caused due to mutations in the genes encoding for the antioxidant protein superoxide dismutase 1 (SOD1), the TAR-DNA binding protein 43 (TDP43), ubiquilin 2 (Ubqln2), and VAPB (De Mario et al., 2017; Perrone et al., 2020).
Dominant mutations in the gene encoding SOD1 leads to familial ALS and accumulation of SOD1 mutant proteins in the ERMCSs (Bruijn et al., 2004). Reportedly, the mutant SOD1 interacts with Bcl-2, affecting calcium homeostasis (Eckenrode et al., 2010).
Accumulation of TDP-43 is a hallmark of ALS/FTD pathology. Overexpression of wild type, as well as mutant TDP-43, leads to reduced ER–mitochondria association, decreased VAPB–PTPIP51 interaction, and affects Ca2+ homeostasis (Stoica et al., 2014).
In the spinal cord of patients with ALS, VAPB mRNA levels were decreased when compared to control subjects (Anagnostou et al., 2010). The P56S mutant form of VAPB has been associated with ALS type 8 (Perrone et al., 2020). VAPB–P56S has demonstrated altered affinity to PTPIP51 and increased Ca2+ release from ER stores, consequently elevating Ca2+ uptake by the mitochondria (De Vos et al., 2012).
Tools for Studying ERMCSs
Since Copeland and Dalton first discovered ERMCSs in the pseudobranch glands of Fundulus heteroclitus by electron microscopy, various methods have been used to study the structure and function of ERMCSs. The function and structure of ERMCSs were gradually revealed after the establishment of protocols for isolating membrane contacts and high-resolution microscopy (Table 1).
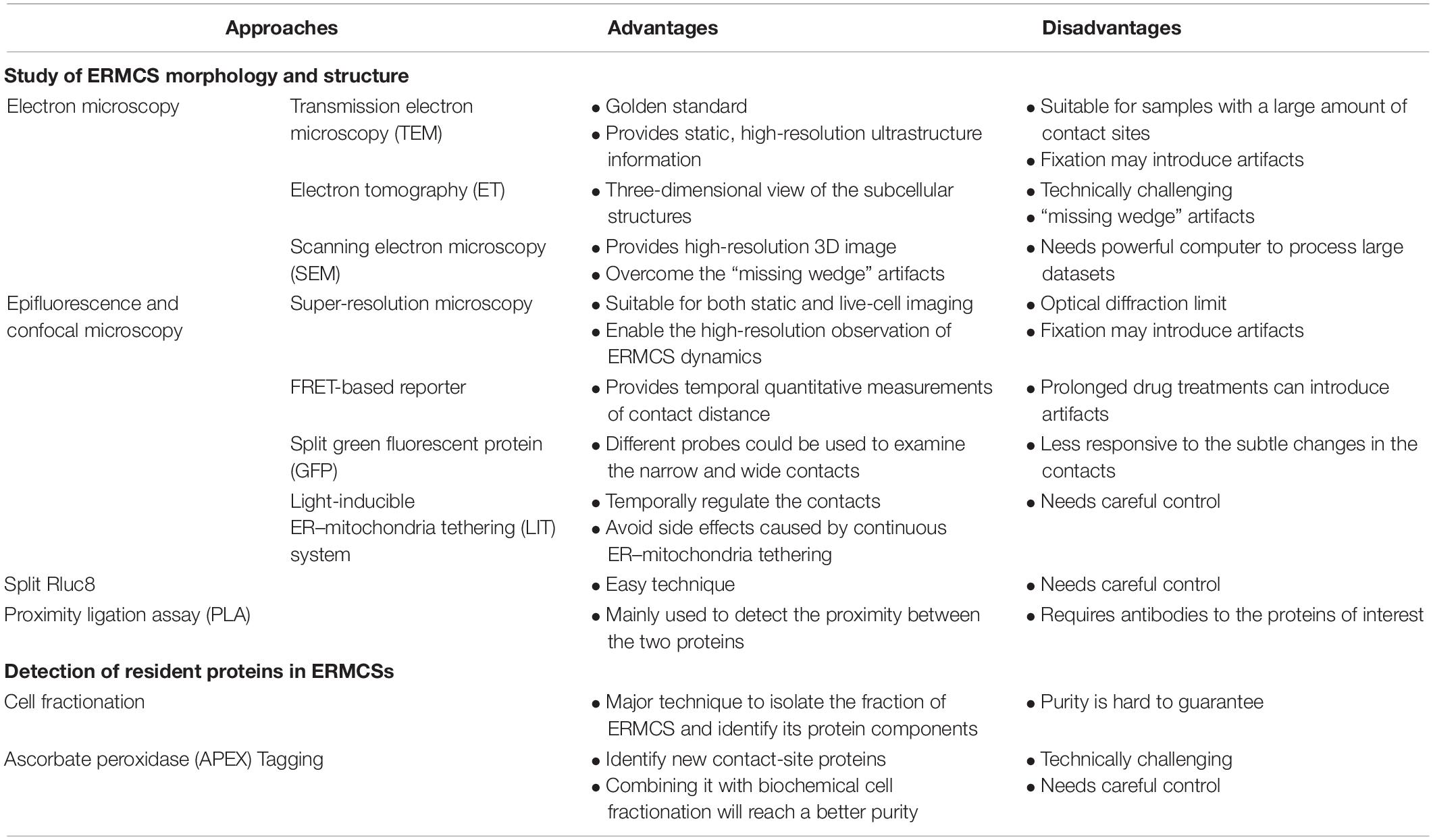
Table 1. Advantages and disadvantages of the various experimental approaches to study endoplasmic reticulum–mitochondria contact sites (ERMCSs).
Study of ERMCS Morphology and Structure
Electron Microscopy
Transmission electron microscopy (TEM) is still considered as the gold standard to study the structure of ERMCS. TEM analysis provides static, high-resolution, and ultrastructure information about the contact sites. Transmission electron microscopy is particularly suitable for samples with a large number of contact sites or those with resident proteins that can be analyzed by immune electron microscopy analysis (Honscher et al., 2014).
Electron tomography (ET) provides a three-dimensional view of the subcellular structures. In ET, a micrograph of a sample is recorded by tilting the sample in different directions and then merged into a three-dimensional structure. Electron tomography often requires serial sectioning, which could be laborious and technically challenging. Additionally, the incomplete 3D reconstructions with regions lacking information often leads to “missing wedge” artifacts (Fernández-Busnadiego, 2016).
Volume EM techniques based on scanning electron microscopy (SEM) not only provide high-resolution 3D images of large specimen volumes but also overcome the “missing wedge” artifacts (Peddie and Collinson, 2014). However, volume EM needs a powerful computer to process large datasets.
Epifluorescence and Confocal Microscopy
The most common technique to visualize the contacts is to express fluorescent proteins that label mitochondria and ER in the cells and observe the overlapping signals. This simple application is suitable for both static and live-cell imaging and is a readily available tool to study ERMCSs. However, this technique is associated with some inherent limitations because of its optical diffraction limit and some artifacts introduced by fixation (Scorrano et al., 2019). The development of super-resolution microscopy has enabled the high-resolution observation of ERMC dynamics (Guo et al., 2018). However, super-resolution microscopy does not conclusively provide information on the formation of contacts between two spatially close organelles.
A drug-inducible Forster resonance energy transfer (FRET)-based reporter was developed to visualize ERMCSs (Csordás et al., 2010). This technique involves introducing a rapamycin-induced dimerization domain, which can provide temporal quantitative measurements of contact distance. However, prolonged drug treatments can introduce artifacts. Several groups have reported the use of ERMCS reporters using split green fluorescent protein (GFP; Cieri et al., 2018; Yang et al., 2018). One half of the split GFP protein labels the ER surface, while the other labels the mitochondrial surface. The fluorescence of GFP is detected if the ER and mitochondria are spatially close enough to form contacts. The narrow (8–10 nm) probe and wide probe (10–50 nm) with short or long linker could be used to examine the narrow and wide contacts, respectively. These reporters do not artificially increase tethering between ER and mitochondria. Additionally, a light-inducible ER–mitochondria tethering (LIT) system was developed to temporally regulate the tethering between ER and mitochondria (Shi et al., 2018). This will be useful for studies that require multiple switching on–off steps and for avoiding side effects caused by continuous ER–mitochondria tethering.
Split Rluc8
Renilla luciferase (Rluc) is a bioluminescent enzyme. The reconstitution of split fragments of RLuc enables the detection of two closely associated proteins. Lim et al. (2015) developed a tool with Mito–RLuc8N and RLuc8C–ER to label the mitochondria and ER, respectively. When the ER and mitochondria are far apart, the activity of RLuc8 cannot be reconstructed. When the ER and mitochondria form contacts, Mito–RLuc8N will be close to RLuc8C-ER, and the total enzyme activity of RLuc8 is detected through the luminescence conversion of substrates (Lim et al., 2015).
Proximity Ligation Assay
Proximity ligation assay (PLA) is an antibody-based method for detecting biomolecules and their physical proximity at a single molecule level resolution. Typically, two unique proteins are recognized by primary antibodies raised in different species. The secondary antibodies conjugated with a pair of oligonucleotides (PLA probes) bind to the primary antibody. The PLA probes will hybridize with the connector oligos only if they are in close proximity to each other. The ligase forms a closed, circle DNA template, and the PLA probe acts as a primer to generate concatemeric sequences, which amplify the signals that are still tethered to the PLA probe by 1000-fold. The labeled oligos hybridize to the complementary sequences within the amplicon, which could then be analyzed by microscopy analysis. This technique was first introduced in 2002 to detect Zeptomoles (10 or 21 mol) of platelet-derived growth factor (PDGF) in the solution phase (Fredriksson et al., 2002). Currently, this assay is mainly used to detect the proximity between the two proteins (Bagchi et al., 2015). In PLA, two contact specific proteins on the ER side and mitochondria side are recognized by their antibodies, and the signals could quantitatively indicate the distance or contact range between the two membranes.
Detection of Resident Proteins in ERMCSs
Cell Fractionation
Cell fractionation mainly refers to the separation of organelles by sucrose gradient. In 1957, Lever (1958) demonstrated that the mitochondria separated by sucrose gradient were always mixed with ER, which indicated the association between ER and mitochondria. Currently, cell fractionation is still a major technique to isolate the fraction of ERMCSs and identify its protein components. The purity of the fraction obtained remains a concern.
Ascorbate Peroxidase Tagging
In situ proximity labeling method uses an engineered ascorbate peroxidase (APEX) to tag a protein of interest and evaluate its expression. The cells are then treated with hydrogen peroxide (H2O2) in the presence of biotin–phenol. The proteins proximal to the APEX fusion protein will be covalently labeled with biotin, which could then be purified and detected via mass spectrometry. Using this APEX tagging technique, the ERMCS-specific protein could be tagged with APEX, and the other proteins located at the ERMCSs could be identified (Cho et al., 2017).
In conclusion, ERMCSs are hot spots for multiple cellular events and are critical for neuronal homeostasis. Although there is no direct evidence to indicate that changes in the ERMCSs can cause neurodegeneration, further studies on ERMCs will provide valuable insights into neurodegeneration and potentially a novel therapeutic target. As mentioned earlier, there are inconsistencies regarding some findings related to the roles of ERMCSs in neurodegenerative diseases. The rationales underlying these remain elusive. Developing a standardized approach to analyze and quantify ERMCSs might be the key to resolve the inconsistencies.
Author Contributions
CT obtained financial support. All authors wrote and approved the final version of the manuscript.
Funding
CT is supported by the National Natural Science Foundation of China (91754103, 31622034, and 31571383), National Key Research and Developmental Program of China (2017YFC1001100 and 2017YFC1001500), Natural Science Foundation of Zhejiang Province, China (LR16C070001), and Fundamental research funds for the central universities. CT is a Qianjiang Scholar.
Conflict of Interest
The authors declare that the research was conducted in the absence of any commercial or financial relationships that could be construed as a potential conflict of interest.
References
Anagnostou, G., Akbar, M. T., Paul, P., Angelinetta, C., Steiner, T. J., and De Belleroche, J. (2010). Vesicle associated membrane protein B (VAPB) is decreased in ALS spinal cord. Neurobiol Aging 31, 969–985. doi: 10.1016/j.neurobiolaging.2008.07.005
Arasaki, K., Shimizu, H., Mogari, H., Nishida, N., Hirota, N., Furuno, A., et al. (2015). A role for the ancient SNARE syntaxin 17 in regulating mitochondrial division. Dev. Cell 32, 304–317. doi: 10.1016/j.devcel.2014.12.011
Area-Gomez, E., De Groof, A. J., Boldogh, I., Bird, T. D., Gibson, G. E., Koehler, C. M., et al. (2009). Presenilins are enriched in endoplasmic reticulum membranes associated with mitochondria. Am. J. Pathol. 175, 1810–1816. doi: 10.2353/ajpath.2009.090219
Area-Gomez, E., Del Carmen, Lara Castillo, M., Tambini, M. D., Guardia-Laguarta, C., De Groof, A. J., et al. (2012). Upregulated function of mitochondria-associated ER membranes in Alzheimer disease. EMBO J. 31, 4106–4123. doi: 10.1038/emboj.2012.202
Bagchi, S., Fredriksson, R., and Wallen-Mackenzie, A. (2015). In situ proximity ligation assay (PLA). Methods Mol. Biol. 1318, 149–159. doi: 10.1007/978-1-4939-2742-5_15
Basso, V., Marchesan, E., Peggion, C., Chakraborty, J., Von Stockum, S., Giacomello, M., et al. (2018). Regulation of ER-mitochondria contacts by Parkin via Mfn2. Pharmacol. Res. 138, 43–56. doi: 10.1016/j.phrs.2018.09.006
Baumgartner, H. K., Gerasimenko, J. V., Thorne, C., Ferdek, P., Pozzan, T., Tepikin, A. V., et al. (2009). Calcium elevation in mitochondria is the main Ca2+ requirement for mitochondrial permeability transition pore (mPTP) opening. J. Biol. Chem. 284, 20796–20803. doi: 10.1074/jbc.m109.025353
Berenguer-Escuder, C., Grossmann, D., Massart, F., Antony, P., Burbulla, L. F., Glaab, E., et al. (2019). Variants in miro1 cause alterations of ER-mitochondria contact sites in fibroblasts from Parkinson’s Disease Patients. J. Clin. Med. 8:2226. doi: 10.3390/jcm8122226
Bononi, A., Missiroli, S., Poletti, F., Suski, J. M., Agnoletto, C., Bonora, M., et al. (2012). Mitochondria-associated membranes (MAMs) as hotspot Ca(2+) signaling units. Adv. Exp. Med. Biol. 740, 411–437. doi: 10.1007/978-94-007-2888-2_17
Bruijn, L. I., Miller, T. M., and Cleveland, D. W. (2004). Unraveling the mechanisms involved in motor neuron degeneration in ALS. Annu. Rev. Neurosci. 27, 723–749. doi: 10.1146/annurev.neuro.27.070203.144244
Cali, T., Ottolini, D., Negro, A., and Brini, M. (2013). Enhanced parkin levels favor ER-mitochondria crosstalk and guarantee Ca(2+) transfer to sustain cell bioenergetics. Biochim. Biophys. Acta 1832, 495–508. doi: 10.1016/j.bbadis.2013.01.004
Cerqua, C., Anesti, V., Pyakurel, A., Liu, D., Naon, D., Wiche, G., et al. (2010). Trichoplein/mitostatin regulates endoplasmic reticulum-mitochondria juxtaposition. EMBO Rep. 11, 854–860. doi: 10.1038/embor.2010.151
Chandra, D., Choy, G., Deng, X., Bhatia, B., Daniel, P., and Tang, D. G. (2004). Association of active caspase 8 with the mitochondrial membrane during apoptosis: potential roles in cleaving BAP31 and caspase 3 and mediating mitochondrion-endoplasmic reticulum cross talk in etoposide-induced cell death. Mol. Cell Biol. 24, 6592–6607. doi: 10.1128/mcb.24.15.6592-6607.2004
Cho, I.-T., Adelmant, G., Lim, Y., Marto, J. A., Cho, G., and Golden, J. A. (2017). Ascorbate peroxidase proximity labeling coupled with biochemical fractionation identifies promoters of endoplasmic reticulum–mitochondrial contacts. J. Biol. Chem. 292, 16382–16392. doi: 10.1074/jbc.m117.795286
Cieri, D., Vicario, M., Giacomello, M., Vallese, F., Filadi, R., Wagner, T., et al. (2018). SPLICS: a split green fluorescent protein-based contact site sensor for narrow and wide heterotypic organelle juxtaposition. Cell Death Differ. 25, 1131–1145. doi: 10.1038/s41418-017-0033-z
Cohen, S., Valm, A. M., and Lippincott-Schwartz, J. (2018). Interacting organelles. Curr. Opin. Cell Biol. 53, 84–91. doi: 10.1016/j.ceb.2018.06.003
Cosson, P., Marchetti, A., Ravazzola, M., and Orci, L. (2012). Mitofusin-2 independent juxtaposition of endoplasmic reticulum and mitochondria: an ultrastructural study. PLoS One 7:e46293. doi: 10.1371/journal.pone.0046293
Csordas, G., Renken, C., Varnai, P., Walter, L., Weaver, D., Buttle, K. F., et al. (2006). Structural and functional features and significance of the physical linkage between ER and mitochondria. J. Cell Biol. 174, 915–921. doi: 10.1083/jcb.200604016
Csordás, G., Várnai, P., Golenár, T., Roy, S., Purkins, G., Schneider, T. G., et al. (2010). Imaging interorganelle contacts and local calcium dynamics at the er-mitochondrial interface. Mol. Cell 39, 121–132. doi: 10.1016/j.molcel.2010.06.029
Cui, Z., Vance, J. E., Chen, M. H., Voelker, D. R., and Vance, D. E. (1993). Cloning and expression of a novel phosphatidylethanolamine N-methyltransferase. A specific biochemical and cytological marker for a unique membrane fraction in rat liver. J. Biol. Chem. 268, 16655–16663.
Daniele, T., Hurbain, I., Vago, R., Casari, G., Raposo, G., Tacchetti, C., et al. (2014). Mitochondria and melanosomes establish physical contacts modulated by Mfn2 and involved in organelle biogenesis. Curr. Biol. 24, 393–403. doi: 10.1016/j.cub.2014.01.007
De Brito, O. M., and Scorrano, L. (2008). Mitofusin 2 tethers endoplasmic reticulum to mitochondria. Nature 456, 605–610. doi: 10.1038/nature07534
De Mario, A., Quintana-Cabrera, R., Martinvalet, D., and Giacomello, M. (2017). (Neuro)degenerated mitochondria-ER contacts. Biochem. Biophys. Res. Commun. 483, 1096–1109. doi: 10.1016/j.bbrc.2016.07.056
De Vos, K. J., Morotz, G. M., Stoica, R., Tudor, E. L., Lau, K. F., Ackerley, S., et al. (2012). VAPB interacts with the mitochondrial protein PTPIP51 to regulate calcium homeostasis. Hum. Mol. Genet. 21, 1299–1311. doi: 10.1093/hmg/ddr559
D’eletto, M., Rossin, F., Occhigrossi, L., Farrace, M. G., Faccenda, D., Desai, R., et al. (2018). Transglutaminase Type 2 Regulates ER-Mitochondria Contact Sites by Interacting with GRP75. Cell Rep. 25, 3573.e4–3581.e4.
Devine, M. J., and Kittler, J. T. (2018). Mitochondria at the neuronal presynapse in health and disease. Nat. Rev. Neurosci. 19, 63–80. doi: 10.1038/nrn.2017.170
Eckenrode, E. F., Yang, J., Velmurugan, G. V., Foskett, J. K., and White, C. (2010). Apoptosis protection by Mcl-1 and Bcl-2 modulation of inositol 1,4,5-trisphosphate receptor-dependent Ca2+ signaling. J. Biol. Chem. 285, 13678–13684. doi: 10.1074/jbc.m109.096040
Elbaz-Alon, Y., Eisenberg-Bord, M., Shinder, V., Stiller, S. B., Shimoni, E., Wiedemann, N., et al. (2015). Lam6 regulates the extent of contacts between organelles. Cell Rep. 12, 7–14. doi: 10.1016/j.celrep.2015.06.022
Fernández-Busnadiego, R. (2016). Supramolecular architecture of endoplasmic reticulum–plasma membrane contact sites. Biochem. Soc. Trans. 44, 534–540. doi: 10.1042/bst20150279
Filadi, R., Greotti, E., Turacchio, G., Luini, A., Pozzan, T., and Pizzo, P. (2016). Presenilin 2 modulates endoplasmic reticulum-mitochondria coupling by tuning the antagonistic effect of mitofusin 2. Cell Rep. 15, 2226–2238. doi: 10.1016/j.celrep.2016.05.013
Fredriksson, S., Gullberg, M., Jarvius, J., Olsson, C., Pietras, K., Gustafsdottir, S. M., et al. (2002). Protein detection using proximity-dependent DNA ligation assays. Nat. Biotechnol. 20, 473–477. doi: 10.1038/nbt0502-473
Friedman, J. R., Lackner, L. L., West, M., Dibenedetto, J. R., Nunnari, J., and Voeltz, G. K. (2011). ER tubules mark sites of mitochondrial division. Science 334, 358–362. doi: 10.1126/science.1207385
Galmes, R., Houcine, A., Van Vliet, A. R., Agostinis, P., Jackson, C. L., and Giordano, F. (2016). ORP5/ORP8 localize to endoplasmic reticulum-mitochondria contacts and are involved in mitochondrial function. EMBO Rep. 17, 800–810. doi: 10.15252/embr.201541108
Garrido-Maraver, J., Loh, S. H. Y., and Martins, L. M. (2020). Forcing contacts between mitochondria and the endoplasmic reticulum extends lifespan in a Drosophila model of Alzheimer’s disease. Biol. Open 9:bio047530. doi: 10.1242/bio.047530
Gautier, C. A., Erpapazoglou, Z., Mouton-Liger, F., Muriel, M. P., Cormier, F., Bigou, S., et al. (2016). The endoplasmic reticulum-mitochondria interface is perturbed in PARK2 knockout mice and patients with PARK2 mutations. Hum. Mol. Genet. 25, 2972–2984.
Gellerich, F. N., Gizatullina, Z., Trumbeckaite, S., Nguyen, H. P., Pallas, T., Arandarcikaite, O., et al. (2010). The regulation of OXPHOS by extramitochondrial calcium. Biochim. Biophys. Acta 1797, 1018–1027. doi: 10.1016/j.bbabio.2010.02.005
Gomez-Suaga, P., Paillusson, S., Stoica, R., Noble, W., Hanger, D. P., and Miller, C. C. J. (2017). The ER-Mitochondria tethering complex VAPB-PTPIP51 regulates autophagy. Curr. Biol. 27, 371–385. doi: 10.1016/j.cub.2016.12.038
Guardia-Laguarta, C., Area-Gomez, E., Rub, C., Liu, Y., Magrane, J., Becker, D., et al. (2014). alpha-Synuclein is localized to mitochondria-associated ER membranes. J. Neurosci. 34, 249–259. doi: 10.1523/jneurosci.2507-13.2014
Guo, Y., Li, D., Zhang, S., Yang, Y., Liu, J. J., Wang, X., et al. (2018). Visualizing intracellular organelle and cytoskeletal interactions at nanoscale resolution on millisecond timescales. Cell 175, 1430.e17–1442.e17.
Hamasaki, M., Furuta, N., Matsuda, A., Nezu, A., Yamamoto, A., Fujita, N., et al. (2013). Autophagosomes form at ER-mitochondria contact sites. Nature 495, 389–393. doi: 10.1038/nature11910
Hayashi, T., and Su, T. P. (2007). Sigma-1 receptor chaperones at the ER-mitochondrion interface regulate Ca(2+) signaling and cell survival. Cell 131, 596–610. doi: 10.1016/j.cell.2007.08.036
Hedskog, L., Pinho, C. M., Filadi, R., Ronnback, A., Hertwig, L., Wiehager, B., et al. (2013). Modulation of the endoplasmic reticulum-mitochondria interface in Alzheimer’s disease and related models. Proc. Natl. Acad. Sci. U.S.A. 110, 7916–7921. doi: 10.1073/pnas.1300677110
Honscher, C., Mari, M., Auffarth, K., Bohnert, M., Griffith, J., Geerts, W., et al. (2014). Cellular metabolism regulates contact sites between vacuoles and mitochondria. Dev. Cell 30, 86–94. doi: 10.1016/j.devcel.2014.06.006
Iwasawa, R., Mahul-Mellier, A. L., Datler, C., Pazarentzos, E., and Grimm, S. (2011). Fis1 and Bap31 bridge the mitochondria-ER interface to establish a platform for apoptosis induction. EMBO J. 30, 556–568. doi: 10.1038/emboj.2010.346
Jia, H., Zhang, Y., and Huang, Y. (2019). Imaging sigma receptors in the brain: New opportunities for diagnosis of Alzheimer’s disease and therapeutic development. Neurosci. Lett. 691, 3–10. doi: 10.1016/j.neulet.2018.07.033
Kaiser, S. E., Brickner, J. H., Reilein, A. R., Fenn, T. D., Walter, P., and Brunger, A. T. (2005). Structural basis of FFAT motif-mediated ER targeting. Structure 13, 1035–1045. doi: 10.1016/j.str.2005.04.010
Kornmann, B., Currie, E., Collins, S. R., Schuldiner, M., Nunnari, J., Weissman, J. S., et al. (2009). An ER-mitochondria tethering complex revealed by a synthetic biology screen. Science 325, 477–481. doi: 10.1126/science.1175088
Kornmann, B., Osman, C., and Walter, P. (2011). The conserved GTPase Gem1 regulates endoplasmic reticulum-mitochondria connections. Proc. Natl. Acad. Sci. U.S.A. 108, 14151–14156. doi: 10.1073/pnas.1111314108
Korobova, F., Ramabhadran, V., and Higgs, H. N. (2013). An actin-dependent step in mitochondrial fission mediated by the ER-associated formin INF2. Science 339, 464–467. doi: 10.1126/science.1228360
Lackner, L. L. (2019). The expanding and unexpected functions of mitochondria contact sites. Trends Cell Biol. 29, 580–590. doi: 10.1016/j.tcb.2019.02.009
Lahiri, S., Chao, J. T., Tavassoli, S., Wong, A. K., Choudhary, V., Young, B. P., et al. (2014). A conserved endoplasmic reticulum membrane protein complex (EMC) facilitates phospholipid transfer from the ER to mitochondria. PLoS Biol. 12:e1001969. doi: 10.1371/journal.pbio.1001969
Leal, N. S., Schreiner, B., Pinho, C. M., Filadi, R., Wiehager, B., Karlstrom, H., et al. (2016). Mitofusin-2 knockdown increases ER-mitochondria contact and decreases amyloid beta-peptide production. J. Cell Mol. Med. 20, 1686–1695. doi: 10.1111/jcmm.12863
Lee, K. S., Huh, S., Lee, S., Wu, Z., Kim, A. K., Kang, H. Y., et al. (2018). Altered ER-mitochondria contact impacts mitochondria calcium homeostasis and contributes to neurodegeneration in vivo in disease models. Proc. Natl. Acad. Sci. U.S.A. 115, E8844–E8853.
Lev, S., Ben Halevy, D., Peretti, D., and Dahan, N. (2008). The VAP protein family: from cellular functions to motor neuron disease. Trends Cell Biol. 18, 282–290. doi: 10.1016/j.tcb.2008.03.006
Lever, J. D. (1958). Mitochondria Isolated from rat brown adipose tissue and liver. J. Cell Bio. 4, 287–290. doi: 10.1083/jcb.4.3.287
Lewin, T. M., Kim, J. H., Granger, D. A., Vance, J. E., and Coleman, R. A. (2001). Acyl-CoA synthetase isoforms 1, 4, and 5 are present in different subcellular membranes in rat liver and can be inhibited independently. J. Biol. Chem. 276, 24674–24679. doi: 10.1074/jbc.m102036200
Lewin, T. M., Van Horn, C. G., Krisans, S. K., and Coleman, R. A. (2002). Rat liver acyl-CoA synthetase 4 is a peripheral-membrane protein located in two distinct subcellular organelles, peroxisomes, and mitochondrial-associated membrane. Arch. Biochem. Biophys. 404, 263–270. doi: 10.1016/s0003-9861(02)00247-3
Lewis, S. C., Uchiyama, L. F., and Nunnari, J. (2016). ER-mitochondria contacts couple mtDNA synthesis with mitochondrial division in human cells. Science 353, aaf5549. doi: 10.1126/science.aaf5549
Lim, Y., Cho, I. T., Schoel, L. J., Cho, G., and Golden, J. A. (2015). Hereditary spastic paraplegia-linked REEP1 modulates endoplasmic reticulum/mitochondria contacts. Ann. Neurol. 78, 679–696. doi: 10.1002/ana.24488
Liu, Y., Ma, X., Fujioka, H., Liu, J., Chen, S., and Zhu, X. (2019). DJ-1 regulates the integrity and function of ER-mitochondria association through interaction with IP3R3-Grp75-VDAC1. Proc. Natl. Acad. Sci. U.S.A. 116, 25322–25328. doi: 10.1073/pnas.1906565116
Lynes, E. M., Bui, M., Yap, M. C., Benson, M. D., Schneider, B., Ellgaard, L., et al. (2012). Palmitoylated TMX and calnexin target to the mitochondria-associated membrane. EMBO J. 31, 457–470. doi: 10.1038/emboj.2011.384
Maurice, T., and Goguadze, N. (2017). “Role of σ1 receptors in learning and memory and Alzheimer’s disease-type dementia,” in Sigma Receptors: Their Role in Disease and as Therapeutic Targets, eds S. B. Smith and T.-P. Su (Cham: Springer International Publishing), 213–233. doi: 10.1007/978-3-319-50174-1_15
Mizushima, N., and Komatsu, M. (2011). Autophagy: renovation of cells and tissues. Cell 147, 728–741. doi: 10.1016/j.cell.2011.10.026
Moltedo, O., Remondelli, P., and Amodio, G. (2019). The mitochondria-endoplasmic reticulum contacts and their critical role in aging and age-associated diseases. Front. Cell Dev. Biol. 7:172. doi: 10.3389/fcell.2019.0017
Morales, P. E., Torres, G., Sotomayor-Flores, C., Pena-Oyarzun, D., Rivera-Mejias, P., Paredes, F., et al. (2014). GLP-1 promotes mitochondrial metabolism in vascular smooth muscle cells by enhancing endoplasmic reticulum-mitochondria coupling. Biochem. Biophys. Res. Commun. 446, 410–416. doi: 10.1016/j.bbrc.2014.03.004
Murley, A., Sarsam, R. D., Toulmay, A., Yamada, J., Prinz, W. A., and Nunnari, J. (2015). Ltc1 is an ER-localized sterol transporter and a component of ER-mitochondria and ER-vacuole contacts. J. Cell Biol. 209, 539–548. doi: 10.1083/jcb.201502033
Murphy, S. E., and Levine, T. P. (2016). VAP, a versatile access point for the endoplasmic reticulum: review and analysis of FFAT-like motifs in the VAPome. Biochim. Biophys. Acta 1861, 952–961. doi: 10.1016/j.bbalip.2016.02.009
Naj, A. C., and Schellenberg, G. D. (2017). Genomic variants, genes, and pathways of Alzheimer’s disease: An overview. Am. J. Med. Genet. Part BNeuropsychiatr. Genet. 174, 5–26. doi: 10.1002/ajmg.b.32499
Namba, T. (2019). BAP31 regulates mitochondrial function via interaction with Tom40 within ER-mitochondria contact sites. Sci. Adv. 5:eaaw1386. doi: 10.1126/sciadv.aaw1386
Namba, T., Tian, F., Chu, K., Hwang, S. Y., Yoon, K. W., Byun, S., et al. (2013). CDIP1-BAP31 complex transduces apoptotic signals from endoplasmic reticulum to mitochondria under endoplasmic reticulum stress. Cell Rep. 5, 331–339. doi: 10.1016/j.celrep.2013.09.020
Osman, C., Voelker, D. R., and Langer, T. (2011). Making heads or tails of phospholipids in mitochondria. J. Cell Biol. 192, 7–16. doi: 10.1083/jcb.201006159
Ottolini, D., Cali, T., Negro, A., and Brini, M. (2013). The Parkinson disease-related protein DJ-1 counteracts mitochondrial impairment induced by the tumour suppressor protein p53 by enhancing endoplasmic reticulum-mitochondria tethering. Hum. Mol. Genet. 22, 2152–2168. doi: 10.1093/hmg/ddt068
Pagliuso, A., Tham, T. N., Stevens, J. K., Lagache, T., Persson, R., Salles, A., et al. (2016). A role for septin 2 in Drp1-mediated mitochondrial fission. EMBO Rep. 17, 858–873. doi: 10.15252/embr.201541612
Paillusson, S., Gomez-Suaga, P., Stoica, R., Little, D., Gissen, P., Devine, M. J., et al. (2017). alpha-Synuclein binds to the ER-mitochondria tethering protein VAPB to disrupt Ca(2+) homeostasis and mitochondrial ATP production. Acta Neuropathol. 134, 129–149. doi: 10.1007/s00401-017-1704-z
Paillusson, S., Stoica, R., Gomez-Suaga, P., Lau, D. H. W., Mueller, S., Miller, T., et al. (2016). There’s something wrong with my MAM; the ER-mitochondria axis and Neurodegenerative Diseases. Trends Neurosci. 39, 146–157. doi: 10.1016/j.tins.2016.01.008
Peddie, C. J., and Collinson, L. M. (2014). Exploring the third dimension: volume electron microscopy comes of age. Micron 61, 9–19. doi: 10.1016/j.micron.2014.01.009
Perrone, M., Caroccia, N., Genovese, I., Missiroli, S., Modesti, L., Pedriali, G., et al. (2020). The role of mitochondria-associated membranes in cellular homeostasis and diseases. Int. Rev. Cell Mol. Biol. 350, 119–196. doi: 10.1016/bs.ircmb.2019.11.002
Phillips, M. J., and Voeltz, G. K. (2016). Structure and function of ER membrane contact sites with other organelles. Nat. Rev. Mol. Cell Biol. 17, 69–82.
Prasad, M., Kaur, J., Pawlak, K. J., Bose, M., Whittal, R. M., and Bose, H. S. (2015). Mitochondria-associated endoplasmic reticulum membrane (MAM) regulates steroidogenic activity via steroidogenic acute regulatory protein (StAR)-voltage-dependent anion channel 2 (VDAC2) interaction. J. Biol. Chem. 290, 2604–2616. doi: 10.1074/jbc.m114.605808
Rapizzi, E., Pinton, P., Szabadkai, G., Wieckowski, M. R., Vandecasteele, G., Baird, G., et al. (2002). Recombinant expression of the voltage-dependent anion channel enhances the transfer of Ca2+ microdomains to mitochondria. J. Cell Biol. 159, 613–624. doi: 10.1083/jcb.200205091
Schwarz, D. S., and Blower, M. D. (2016). The endoplasmic reticulum: structure, function and response to cellular signaling. Cell Mol. Life Sci. 73, 79–94. doi: 10.1007/s00018-015-2052-6
Scorrano, L., De Matteis, M. A., Emr, S., Giordano, F., Hajnoczky, G., Kornmann, B., et al. (2019). Coming together to define membrane contact sites. Nat. Commun. 10, 1287.
Shi, F., Kawano, F., Park, S. E., Komazaki, S., Hirabayashi, Y., Polleux, F., et al. (2018). Optogenetic control of endoplasmic reticulum-mitochondria tethering. ACS Synth. Biol. 7, 2–9. doi: 10.1021/acssynbio.7b00248
Simmen, T., Aslan, J. E., Blagoveshchenskaya, A. D., Thomas, L., Wan, L., Xiang, Y., et al. (2005). PACS-2 controls endoplasmic reticulum-mitochondria communication and Bid-mediated apoptosis. EMBO J. 24, 717–729. doi: 10.1038/sj.emboj.7600559
Stoica, R., De Vos, K. J., Paillusson, S., Mueller, S., Sancho, R. M., Lau, K. F., et al. (2014). ER-mitochondria associations are regulated by the VAPB-PTPIP51 interaction and are disrupted by ALS/FTD-associated TDP-43. Nat. Commun. 5:3996.
Stoica, R., Paillusson, S., Gomez-Suaga, P., Mitchell, J. C., Lau, D. H., Gray, E. H., et al. (2016). ALS/FTD-associated FUS activates GSK-3beta to disrupt the VAPB-PTPIP51 interaction and ER-mitochondria associations. EMBO Rep. 17, 1326–1342. doi: 10.15252/embr.201541726
Stone, S. J., Levin, M. C., Zhou, P., Han, J., Walther, T. C., and Farese, R. V. Jr. (2009). The endoplasmic reticulum enzyme DGAT2 is found in mitochondria-associated membranes and has a mitochondrial targeting signal that promotes its association with mitochondria. J. Biol. Chem. 284, 5352–5361. doi: 10.1074/jbc.m805768200
Sugiura, A., Nagashima, S., Tokuyama, T., Amo, T., Matsuki, Y., Ishido, S., et al. (2013). MITOL regulates endoplasmic reticulum-mitochondria contacts via Mitofusin2. Mol. Cell 51, 20–34. doi: 10.1016/j.molcel.2013.04.023
Szabadkai, G., Bianchi, K., Varnai, P., De Stefani, D., Wieckowski, M. R., Cavagna, D., et al. (2006). Chaperone-mediated coupling of endoplasmic reticulum and mitochondrial Ca2+ channels. J. Cell Biol. 175, 901–911. doi: 10.1083/jcb.200608073
Tambini, M. D., Pera, M., Kanter, E., Yang, H., Guardia-Laguarta, C., Holtzman, D., et al. (2016). ApoE4 upregulates the activity of mitochondria-associated ER membranes. EMBO Rep. 17, 27–36. doi: 10.15252/embr.201540614
Tatsuta, T., Scharwey, M., and Langer, T. (2014). Mitochondrial lipid trafficking. Trends Cell Biol. 24, 44–52. doi: 10.1016/j.tcb.2013.07.011
Wang, C., Ma, H., Feng, X., Xie, S., and Chan, P. (2010). Parkin dosage mutations in patients with early-onset sporadic and familial Parkinson’s disease in Chinese: an independent pathogenic role. Brain Res. 1358, 30–38. doi: 10.1016/j.brainres.2010.08.060
Xu, H., Guan, N., Ren, Y. L., Wei, Q. J., Tao, Y. H., Yang, G. S., et al. (2018). IP3R-Grp75-VDAC1-MCU calcium regulation axis antagonists protect podocytes from apoptosis and decrease proteinuria in an Adriamycin nephropathy rat model. BMC Nephrol. 19:140. doi: 10.1186/s12882-018-0940-3
Yang, Z., Zhao, X., Xu, J., Shang, W., and Tong, C. (2018). A novel fluorescent reporter detects plastic remodeling of mitochondria-ER contact sites. J. Cell Sci. 131:jcs208686. doi: 10.1242/jcs.208686
Yuan, L., Liu, Q., Wang, Z., Hou, J., and Xu, P. (2019). EI24 tethers endoplasmic reticulum and mitochondria to regulate autophagy flux. Cell Mol. Life Sci. 77, 1591–1606. doi: 10.1007/s00018-019-03236-9
Zampese, E., Fasolato, C., Kipanyula, M. J., Bortolozzi, M., Pozzan, T., and Pizzo, P. (2011). Presenilin 2 modulates endoplasmic reticulum (ER)-mitochondria interactions and Ca2+ cross-talk. Proc. Natl. Acad. Sci. U.S.A. 108, 2777–2782. doi: 10.1073/pnas.1100735108
Keywords: endoplasmic reticulum, mitochdonrion, contact sites, neurodegeneration, autophagy
Citation: Xu L, Wang X and Tong C (2020) Endoplasmic Reticulum–Mitochondria Contact Sites and Neurodegeneration. Front. Cell Dev. Biol. 8:428. doi: 10.3389/fcell.2020.00428
Received: 14 February 2020; Accepted: 07 May 2020;
Published: 18 June 2020.
Edited by:
Laura Lackner, Northwestern University, United StatesReviewed by:
Guangpu Li, The University of Oklahoma Health Sciences Center, United StatesFengguang Guo, Texas A&M University, United States
Copyright © 2020 Xu, Wang and Tong. This is an open-access article distributed under the terms of the Creative Commons Attribution License (CC BY). The use, distribution or reproduction in other forums is permitted, provided the original author(s) and the copyright owner(s) are credited and that the original publication in this journal is cited, in accordance with accepted academic practice. No use, distribution or reproduction is permitted which does not comply with these terms.
*Correspondence: Chao Tong, Y3RvbmdAemp1LmVkdS5jbg==