- Department of Genetics, College of Life Sciences, Northeast Forestry University, Harbin, China
Hematopoiesis is a necessary process for development and immune defense in Drosophila from the embryonic period to adulthood. There are two main stages in this process: the first stage occurs in the head mesoderm during the embryonic stage, and the second occurs in a specialized hematopoietic organ along the dorsal vessel, the lymph gland, during the larval stage. The lymph gland consists of paired lobes, each of which has distinct regions: the cortical zone (CZ), which contains mature hemocytes; the medullary zone (MZ), which contains hematopoietic progenitors; and the posterior signaling center (PSC), which specifically expresses the early B-cell factor (EBF) transcription factor Collier (Col) and the HOX factor Antennapedia (Antp) to form a microenvironment similar to that of the mammalian bone marrow hematopoietic stem cell niche. The PSC plays a key role in regulating hematopoietic progenitor differentiation. Moreover, the PSC contributes to the cellular immune response to wasp parasitism triggered by elevated ROS levels. Two recent studies have revealed that hematopoietic progenitor maintenance is directly regulated by Col expressed in the MZ and is independent of the PSC, challenging the traditional model. In this review, we summarize the regulatory networks of PSC cell proliferation, the controversy regarding PSC-mediated regulation of hematopoietic progenitor differentiation, and the wasp egg infection response. In addition, we discuss why the PSC is an ideal model for investigating mammalian hematopoietic stem cell niches and leukemia.
Introduction
Hematopoiesis in Drosophila and vertebrates is highly conserved, and there are significant similarities in the molecular mechanisms between the cardiogenic mesoderm in Drosophila and the aorta-gonadal-mesonephros mesoderm in mammals (Medvinsky and Dzierzak, 1996; Mandal et al., 2004). Drosophila hemocytes are needed for immune defense, wound healing, tissue integrity and environmental stress responses. Because of the convenience of genetic manipulation, Drosophila are regarded as ideal models for research on the regulatory factors of hematopoiesis and the molecular mechanisms of leukemia (Robertson et al., 1988; Crozatier and Vincent, 2011; Baril et al., 2017).
Drosophila undergo two main waves of hematopoiesis (Holz et al., 2003). The first occurs in the head mesoderm at the early embryonic stage (Tepass et al., 1994). The promonocytes of this stage can differentiate into mature hemocytes that contribute to populations of sessile hemocytes attached to the cuticle and circulating hemocytes in the hemolymph at the larval stage (Lebestky et al., 2000; Kurucz et al., 2007; Honti et al., 2010). The cardiogenic mesoderm of the embryo subsequently becomes a specialized organ, the lymph gland, and another hematopoietic pool is created in the larval stage (Shrestha and Gateff, 1982; Jung et al., 2005). During metamorphosis, hematopoietic progenitors of the lymph gland differentiate into mature hemocytes and enter the circulation due to dispersal of the lymph gland (Lanot et al., 2001; Grigorian et al., 2011). The hemocytes generated from these two phases can persist into the adult stage. Moreover, a recent study has revealed four clusters of hemocytes located in the dorsal part of the adult fly abdomen, termed “adult hematopoietic hubs” (Ghosh et al., 2015). Three types of terminally differentiated cells are generated by hematopoietic progenitors (Evans et al., 2003). Plasmatocytes are the most numerous circulating hemocytes, representing 90–95% of hemocytes in circulation, and have the ability to phagocytose invading pathogens (Tepass et al., 1994; Evans et al., 2003; Jung et al., 2005). Crystal cells, so named because they contain crystalline inclusions, are other mature hemocytes (Shrestha and Gateff, 1982; Lanot et al., 2001) that can cause melanization reactions for wound healing (Rizki and Rizki, 1990; Rämet et al., 2002). The third type of mature hemocyte is the lamellocyte; these cells are rarely found in healthy larvae (Lanot et al., 2001). The main function of lamellocytes is to encapsulate foreign objects that are too large to be phagocytosed by plasmatocytes (Rizki and Rizki, 1992).
In the third larval stage, the Drosophila lymph gland matures and can be separated into three distinct zones: the cortical zone (CZ), the medullary zone (MZ) and the posterior signaling center (PSC) (Jung et al., 2005). Under normal conditions, the CZ consists of mature hemocytes, including plasmatocytes and crystal cells, whereas the MZ, located in the inner region of each lymph gland lobe, contains hematopoietic progenitors that can differentiate into mature hemocytes. The PSC comprises 30∼40 cells at the posterior tip of each primary lobe, acting as a hematopoietic niche (Mandal et al., 2007) similar to the hematopoietic niche in the bone marrow of mammals (Krzemień et al., 2007), and plays a key role in regulating progenitor homeostasis (see section “The PSC Functions as a Hematopoietic Progenitor Niche”). PSC cells are distinguished by the expression of the Notch ligand Serrate (Ser), the early B-cell factor (EBF) Collier (Col) and the HOX factor Antennapedia (Antp) (Lebestky et al., 2003; Crozatier et al., 2004; Krzemień et al., 2007; Mandal et al., 2007). Notably, wasp parasitism elevates the reactive oxygen species (ROS) level in the PSC, which is important for cellular immune responses (see section “Role of the PSC in the Cellular Immune Response to Parasitic Wasp Infection”) (Crozatier et al., 2004; Sinenko et al., 2012; Louradour et al., 2017).
Lymph Gland and the PSC
Ontogeny and Terminal Differentiation of the Lymph Gland
The lymph gland is an important hematopoietic and immune organ of Drosophila. Ontogenetically, the development of the lymph gland starts at the early embryonic phase, and the naïve lymph gland forms at the late embryonic phase. At that time, a cluster of Col+Antp+ cells remain at the posterior of the lymph gland in the PSC, providing a niche for hematopoietic progenitors. Afterward, the lymph gland further develops, reaching maturity at the third-instar larval stage. Finally, dispersion of cells in the lymph gland occurs at the metamorphosis stage.
At the early embryonic phase, lymph gland progenitors, cardioblast (CB) and pericardial (PC) cells, belong to a closed lineage and are located in the cardiogenic mesoderm (Figure 1A). Subsequently, the cardiogenic mesoderm gives rise to a part of the dorsal thoracic mesoderm and forms the origin of the lymph gland. The T1–T3 segments of the lateral thoracic mesoderm contain three pairs of cell clusters that express the transcription factor Odd-skipped (Odd) (Ward and Coulter, 2000; Mandal et al., 2007), and these cell clusters coalesce to form the lymph gland (Figure 1A; Mandal et al., 2004). Mutation of either the homeobox protein Tin or the GATA factor Pnr during this stage results in a missing lymph gland; moreover, the decapentaplegic (Dpp), heartless, Notch and wingless (Wg) pathways also participate in the formation of the lymph gland (Table 1; Mandal et al., 2004). The precursors of the PSC express Col and Antp (Mandal et al., 2007), and the separate cell clusters express Col in the T2 and T3 segments (Crozatier et al., 2004); however, Antp+ cells are located only in the T3 segment (Mandal et al., 2007). The cell clusters expressing either Col or Antp partially colocalize with cells expressing Odd and are progressively restricted to the posterior region before coalescing, at which point they become the PSC of the lymph gland in the larval stage. Other Odd+Col–Antp– cells are precursors of hematopoietic progenitors that are triggered to mature via a dpp signal provided by the PSC at the first larval stage. These cells become the MZ or differentiate into mature hemocytes at the third larval stage (Figure 1A).
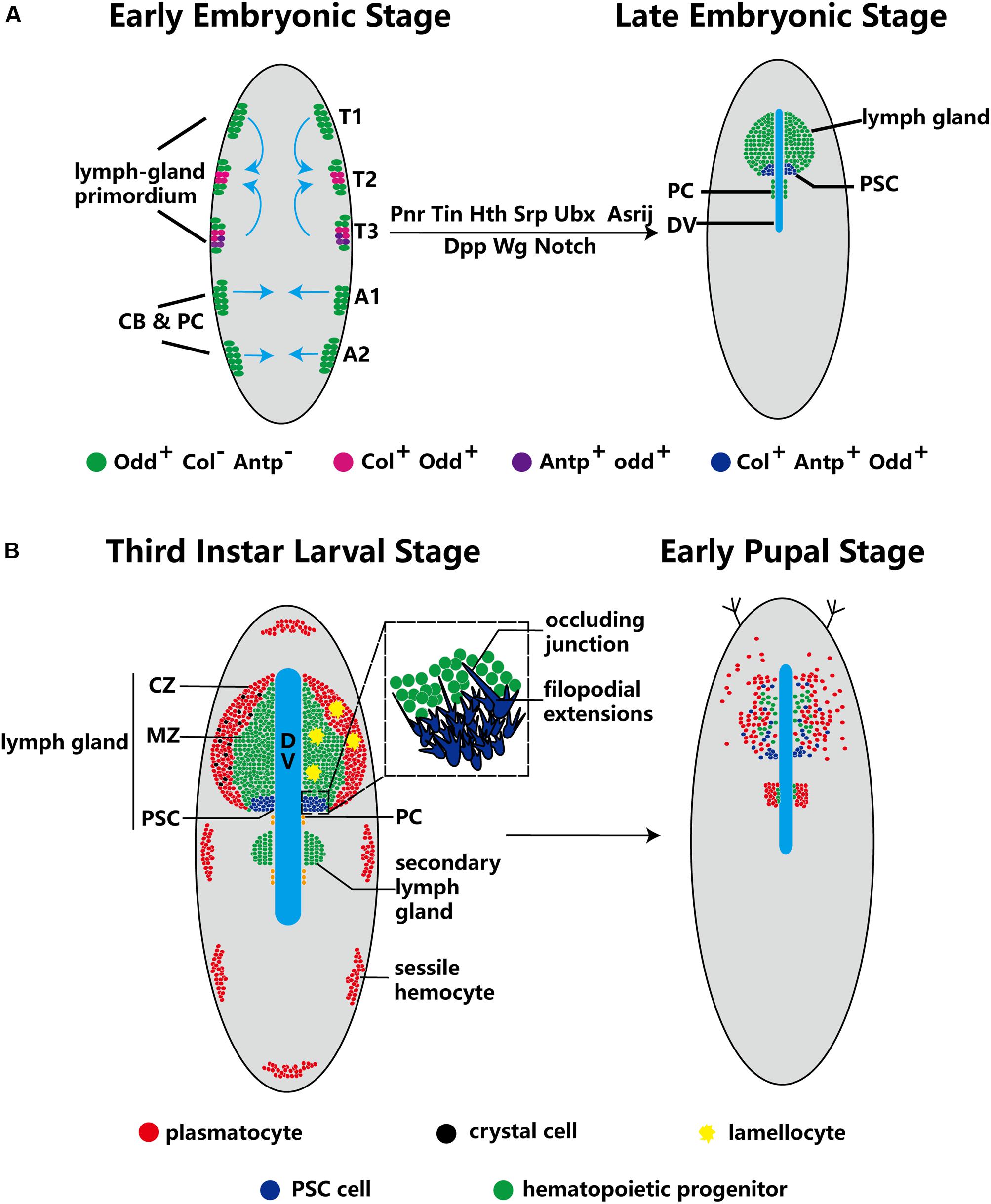
Figure 1. Ontogeny of the lymph gland and the PSC. (A) Odd+ cells form three pairs of cell clusters in the T1–T3 segments; the blue arrows indicate that these clusters coalesce to form the lymph gland. The PSC (purple) is defined by Col+ and Antp+ cells in the T2 and T3 segments. The progenitors, CBs and PCs, are closed lineages located in the cardiogenic mesoderm during the early embryo stage. At the late embryo stage, the lymph gland is along the dorsal vessel (DV), and PCs are located in the posterior lobes of the lymph gland. (B) In third-instar larvae, the lymph gland is mature and can be separated into three zones: the CZ, the MZ and the PSC. Three types of terminal mature hemocytes, plasmatocytes (red), crystal cells (black) and lamellocytes (yellow), are found in the lymph gland (lamellocytes rarely appear under normal conditions). The magnified view of PSC cells in the dotted-line box shows the occluding junction and filopodial extensions. During the early pupal stage, MZ cells show active proliferation and differentiation, and PSC cells are spread out. Subsequently, the lymph gland dissociates and releases hemocytes into the circulation.
Several studies have reported factors that influence lymph gland development. For example, loss of the GATA factor serpent (srp) causes the primordium to form pericardial cells but not lymph gland progenitors (Mandal et al., 2004). In addition, loss of the homeodomain cofactor homothorax (hth) causes failure of the formation of lymph glands; in contrast, overexpression of hth causes disappearance of the PSC (Mandal et al., 2007). On the other hand, loss of some genes causes lymph gland expansion. Several embryonic cell clusters express Ultrabithorax (Ubx), which can inhibit lymph gland formation. Upon loss of Antp, the lymph gland excessively expands into the abdominal segments at the early embryonic phase (Mandal et al., 2004). Furthermore, loss of the endocytic protein Asrij leads to excess lymph gland lobe formation at both the early embryonic and larval stages (Table 1; Kulkarni et al., 2011). These observations suggest that endosome trafficking is extremely important for lymph gland development.
During the metamorphosis stage of pupation, MZ cells actively proliferate and differentiate into terminal mature hemocytes, while PSC cells spread throughout the lymph gland (Grigorian et al., 2011). When the organism enters the pupal stage, the cells of the primary lobes of the lymph gland become large and transform into phagocytic cells. The lymph gland gradually dissociates, disappearing completely by 15 h after puparium formation, and releases hemocytes into the circulation (Figure 1B; Lanot et al., 2001; Grigorian et al., 2011).
PSC Cell Features
Lebestky et al. (2003) found that the lymph gland contains a Ser-expressing signaling center on each primary lobe, which was defined as the PSC. Subsequently, Ser+ cells have also been detected in the CZ and posterior lobes of lymph glands (Ferguson and Martinez-Agosto, 2014). Crozatier et al. (2004) observed that Col, the Drosophila ortholog of the vertebrate gene encoding EBF, contributes to the differentiation of lamellocytes during the cellular immune response to parasitization. In addition, Ser has been found to colocalize with Col at the posterior tip of the lymph gland, and the levels of Ser are dependent on those of Col (Crozatier et al., 2004). Surprisingly, Col+ cells are not unique to the PSC but are also detected in the MZ at low levels (Benmimoun et al., 2015; Oyallon et al., 2016). These results have led to controversy regarding the localization of Col in the lymph gland (see section “Necessity of the PSC for Hematopoietic Progenitor Maintenance”). Mandal et al. (2007) showed that the PSC also expresses Antp during both the embryonic and larval stages. Moreover, the expression of Col is dependent upon that of Antp, indicating that Antp acts upstream of Col and Ser (Mandal et al., 2007).
At the early embryonic stage, the PSC of the lymph gland primordium can show active proliferation (Mandal et al., 2007). However, at the larval stage, PSC cells in the mature lymph gland are mitotically inactive (Lebestky et al., 2003; Mandal et al., 2007), and the number of PSC cells is maintained within the range of 30∼40. These data suggest that a robust mechanism that maintains stable numbers of PSC cells plays a key role in lymph gland homeostasis.
In addition, extensions of filopodia have been observed on PSC cells (Figure 1B). Knockdown of the EBF col, the GATA factor srp, the BAP chromatin-remodeling complex osa, and other important genes causes failure of filopodial extension in the PSC (Krzemień et al., 2007; Tokusumi et al., 2010, 2012); however, the detailed mechanisms have not been carefully characterized and require extensive further study. Khadilkar et al. reported the presence of occluding junctions between PSC cells. These occluding junctions establish a permeability barrier, the function of which is to regulate the communication of signals derived from the PSC to prohemocytes (hematopoietic progenitors) and to participate in the immune response (Khadilkar et al., 2017).
Autonomous Regulation of PSC Cells
The number of PSC cells is stable at the third-instar larval stage, suggesting that autonomous or non-autonomous regulation prevents PSC cell proliferation (Table 2). In the 2000s, based on the initial definition of the PSC associated with the Notch ligand Ser, the Notch pathway was proposed to be a potential important regulator of the PSC. Early research published in 2004 indicated that the Col protein is required for the expression of Ser (Crozatier et al., 2004). However, research published in 2007 showed that the dominant-negative forms of both Ser and Notch cause the disappearance of Col-expressing cells (Krzemień et al., 2007). These studies indicate that there is an interdependence between Ser and Col expression in the regulation of PSC function.
Drosophila Myc (dMyc), the ortholog of the mammalian protooncogene cMyc that encodes a transcriptional activator, regulates cellular growth and proliferation (Bouchard et al., 1998). Overexpression of dMyc in the PSC increases the number of PSC cells (to ∼100 cells) (Pennetier et al., 2012). Jumeau (Jumu) is a member of the forkhead (Fkh) transcription factor family and can bind the FKH domain of dMyc. Knockdown of jumu significantly decreases the number of PSC cells; in contrast, overexpression of jumu in the PSC significantly increases the number of PSC cells (Hao and Jin, 2017).
Both the Wg/Wnt and Dpp/bone morphogenetic protein (BMP) pathways are reported to be located upstream of dMyc (Gallant, 2009). Overexpression of wg increases the number of PSC cells; in contrast, expression of the inactive forms of the Wg pathway receptors Fz and DFz2 significantly reduces the number of PSC cells (Sinenko et al., 2009). Moreover, the increase in PSC cell number caused by overexpression of wg can be reversed by knockdown of dmyc (Pennetier et al., 2012). Therefore, the Wg/Wnt pathway indirectly regulates the proliferation of PSC cells by affecting dMyc levels. In contrast to Wg, Dpp/BMP limits the proliferation of PSC cells. Col downregulates dMyc by enhancing Dpp signaling, and knockdown of col significantly increases PSC cell numbers. Therefore, Col is also a self-autonomous negative regulator of PSC cells (Pennetier et al., 2012). In addition, depletion of the permeability barrier through knockdown of occluding junction components increases the number of PSC cells. When there is no permeability barrier, the strength of both Dpp and Wg signaling is decreased in PSC cells; however, the decrease in Dpp signaling overrides aberrant Wg levels and elevates dMyc expression (Khadilkar et al., 2017). These observations suggest that permeability contributes to the regulation of PSC cell numbers through Dpp and Wg signaling.
The Drosophila insulin/insulin-like growth factor signaling (IIS) pathway is related to metabolism, translation and protein synthesis (Sousa-Nunes et al., 2011; Tennessen and Thummel, 2011). The target of rapamycin (TOR) pathway is another protein synthesis-regulating signaling pathway that engages in crosstalk with the IIS pathway through Akt1. Both the IIS and TOR pathways have been shown to be involved in dMyc expression and regulation, suggesting that both pathways and their related factors are candidates for autonomous regulation of PSC cells. As expected, increased IIS signaling in the PSC through activation of phosphoinositide-3 kinase (PI3K), loss of FoxO or phosphatase and tensin homolog (PTEN), and overexpression of the insulin receptor (InR) or Akt1 can induce PSC expansion (Benmimoun et al., 2012; Tokusumi et al., 2012). Notably, vesicle trafficking is important for signal transduction. ADP ribosylation factor 1 (ARF1) is required for coat assembly, the Golgi architecture and endosome trafficking. ARF1 balances the IIS pathway in PSC cells by partially affecting PI3K. Furthermore, ARF1 can regulate Asrij and participate in lymph gland development by switching between GDP- and GTP-binding forms. Low levels of ARF1 in the PSC can inactivate Asrij and cause complete loss of the PSC (Khadilkar et al., 2014). In addition, Bantam miRNA regulates IIS signaling by affecting Akt1 and is activated by dMyc. Loss of bantam significantly reduces the number of PSC cells; however, this effect can be reversed by overexpression of dmyc. Moreover, the increase in PSC cell number caused by overexpression of bantam can be reversed by knockdown of the InR pathway (Lam et al., 2014). Therefore, dMyc is located between Bantam and the InR pathway and regulates the signaling cascade and feedback loop.
eIF4E (4E)-binding protein (4EBP), which is an inhibitor of the elF4F complex, is inhibited by the IIS pathway (Tokusumi et al., 2011, 2012). Furthermore, Bag of Marbles (Bam), a negative regulator of the elF4F complex, inhibits the proliferation of PSC cells (Tokusumi et al., 2011). Retinoblastoma-family protein (Rbf), a suppressor of cell proliferation regulated by the Bam protein, can suppress the levels of E2F, one of several transcription factors that regulate dMyc levels. Accordingly, Rbf inhibits the proliferation of PSC cells (Tokusumi et al., 2015). These results indicate that the IIS pathway may regulate dMyc through both Bantam and the regulatory relationship between the F4F complex and E2F; however, the mechanisms involved in these interactions and the regulation of PSC development need to be further investigated.
Enhancement of TOR signaling through knockdown of tuberous sclerosis complex 2 (TSC2) causes PSC expansion; in contrast, attenuation of TOR signaling through knockdown of rictor, raptor, Tor, Rheb or S6K reduces PSC cell numbers. In addition, 4EBP is a downstream target of both the IIS and TOR pathways; therefore, the TOR pathway regulates PSC cells by inhibiting 4EBP. Both the IIS and TOR pathways are related to nutrient status. As expected, starvation decreases IIS and TOR pathway signaling and induces loss of the PSC (Shim et al., 2012, 2013; Tokusumi et al., 2012). Therefore, the IIS and TOR pathways, along with their related factors and dMyc, establish a complex interdependent regulatory network. In summary, dMyc, a hub gene, can autonomously regulate the network of PSC cells, as illustrated in Figure 2A.
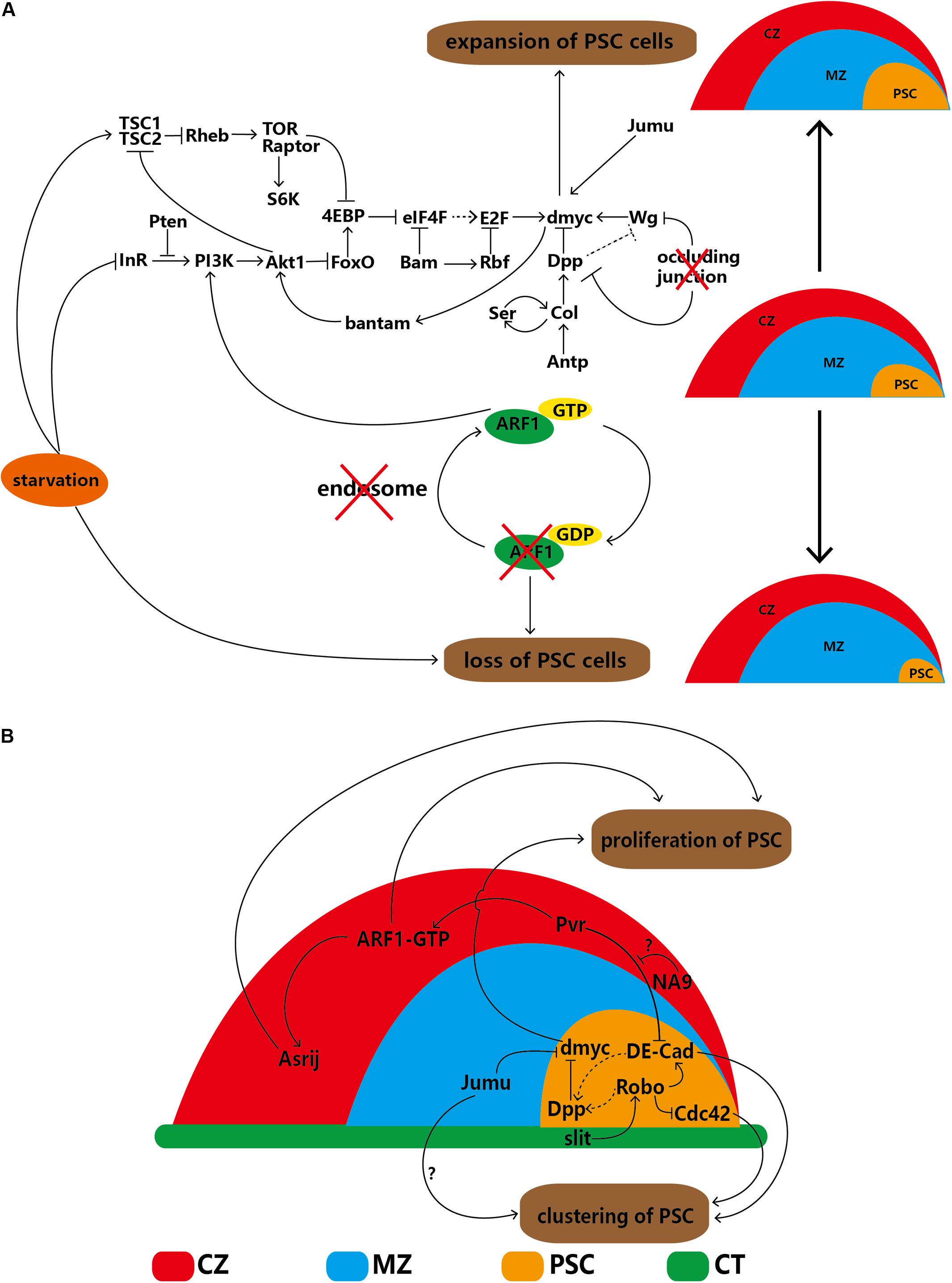
Figure 2. Regulatory network of PSC cells in the lymph gland. (A) PSC cells are self-autonomously regulated by several factors that lead to increases or decreases in cell number. (B) The proliferation and clustering of PSC cells are regulated by extrinsic factors of the CZ, MZ and DV. The solid arrows indicate positive regulation, the ‘T’-shaped arrows indicate negative regulation, the dotted arrows indicate a putative regulatory relationship, the red cross indicates that the component below is disturbed, and the question marks (‘?’) indicate that the detailed regulatory mechanism is uncertain. CZ, cortical zone; MZ, medullary zone; CT, cardiac tube.
Non-autonomous Regulation of PSC Cells
In-depth study in recent years has revealed that some cells adjacent to the PSC can regulate the proliferation or clustering of PSC cells, indicating that non-autonomous regulation exists. Cardiac tube (CT) cells and the slit/Roundabout (Robo) pathway were first discovered because the PSC is located alongside the CT. Robo, the receptor of Slit, can be activated by Slit, which is derived from the CT. Activated Robo promotes Dpp pathway signaling directly and indirectly via DE-cadherin (DE-Cad), thereby negatively regulating the proliferation of PSC cells. Under normal conditions, the number of PSC cell clusters is maintained through the dual functions of DE-Cad and Cdc42. Knockdown of Robo in the PSC significantly increases the number of PSC cells and splits the cells into several cell clusters; knockdown of slit in the CT results in an identical phenotype (Morin-Poulard et al., 2016), indicating the existence of communication between the CT and lymph gland (Figure 2B).
Knockdown of jumu in MZ cells elevates dMyc levels in the PSC through an unknown mechanism, not only significantly increasing PSC cell numbers but also splitting PSC cells into several clusters. However, the DE-Cad and Cdc42 levels remain unchanged (Hao and Jin, 2017). In the CZ, specific knockdown of the ARF1 guanine nucleotide exchange factor (Gartenzwerg) or overexpression of a GTPase-activating protein that keeps Gartenzwerg in its inactive form results in significant loss of PSC cells (Khadilkar et al., 2014). In addition, overexpression of NUP98-HOXA9 (NA9) causes significant expansion of PSC cells, and the candidate mechanism involves signaling mediated by platelet-derived growth factor (PDGF)/vascular endothelial growth factor (VEGF)-related receptor (Pvr) from mature hemocytes to DE-Cad in PSC cells. This observation reinforces Pvr as a regulator of PSC cell numbers and clustering. However, NA9 in the CZ cannot regulate Pvr expression directly (Baril et al., 2017). The differentiated cells of the CZ can control progenitors, which are triggered by binding of PDGF/VEGF-related factor 1 (Pvf1) of the PSC to Pvr on CZ cells (Mondal et al., 2011) (see details in section “The PSC Indirectly Maintains the Homeostasis of Progenitors via Hemocyte Differentiation”). Both ARF1 and NA9 in the CZ interact with Pvr (Baril et al., 2017); however, the detailed mechanism by which Pvr regulates DE-Cad in PSC cells requires clarification (Figure 2B).
The Psc Functions as a Hematopoietic Progenitor Niche
Undoubtedly, the balance between hematopoietic progenitor maintenance and differentiation is very important. PSC cells, which compose a hematopoietic progenitor niche, contribute to maintaining this balance via direct and indirect maintenance of progenitor regulation. In addition, PSC cells are able to convert hematopoietic progenitor precursors into mature hematopoietic progenitors at the first larval stage. However, two studies in 2015 and 2016 sparked controversy regarding the necessity of PSC cells for the maintenance of hematopoietic progenitors in the MZ.
The PSC Directly Maintains the Homeostasis of Hematopoietic Progenitors
PSC cells can secrete ligands directly into the MZ, which activates a corresponding signaling pathway related to the maintenance of hematopoietic progenitors in the activated MZ. The Hedgehog (Hh) signaling pathway is related to cell growth and differentiation (Lum and Beachy, 2004). Hh signaling has been widely studied in the Drosophila lymph gland and is viewed as a major maintenance process of the hematopoietic progenitor pathway directly activated by the PSC because all PSC cells express the ligand Hh. Loss of Hh signaling promotes hematopoietic progenitor differentiation into plasmatocytes and crystal cells; in contrast, increased Hh secretion from the PSC inhibits the differentiation of lamellocytes (Mandal et al., 2007). The progenitors of the MZ have two receptors: Patched (Ptc) and Smoothened (Smo) (Mandal et al., 2007; Giordani et al., 2016; Baldeosingh et al., 2018). In the absence of Hh, Ptc and Smo mediate Cubitus interruptus (Ci) in a repressor form (CiR) via phosphorylation and degradation (Akimaru et al., 1997; Aza-Blanc et al., 1997; Chen et al., 1999). In contrast, the PSC-secreted Hh protein can be transferred to the MZ and bind with Ptc to trigger the Hh signaling pathway in the lymph gland. Inactivation of Ptc can release the receptor Smo (Mandal et al., 2007; Tokusumi et al., 2018) and prevent Ci phosphorylation and degradation by protein kinase complexes of protein kinase A (PKA), casein kinase 1 (CK1) and glycogen synthase kinase-3 (GSK3) to maintain Ci in its activated form (CiA), thereby maintaining hematopoietic progenitors (Figure 3A; Chen et al., 1998; Price and Kalderon, 2002; Lum et al., 2003; Zhang et al., 2006).
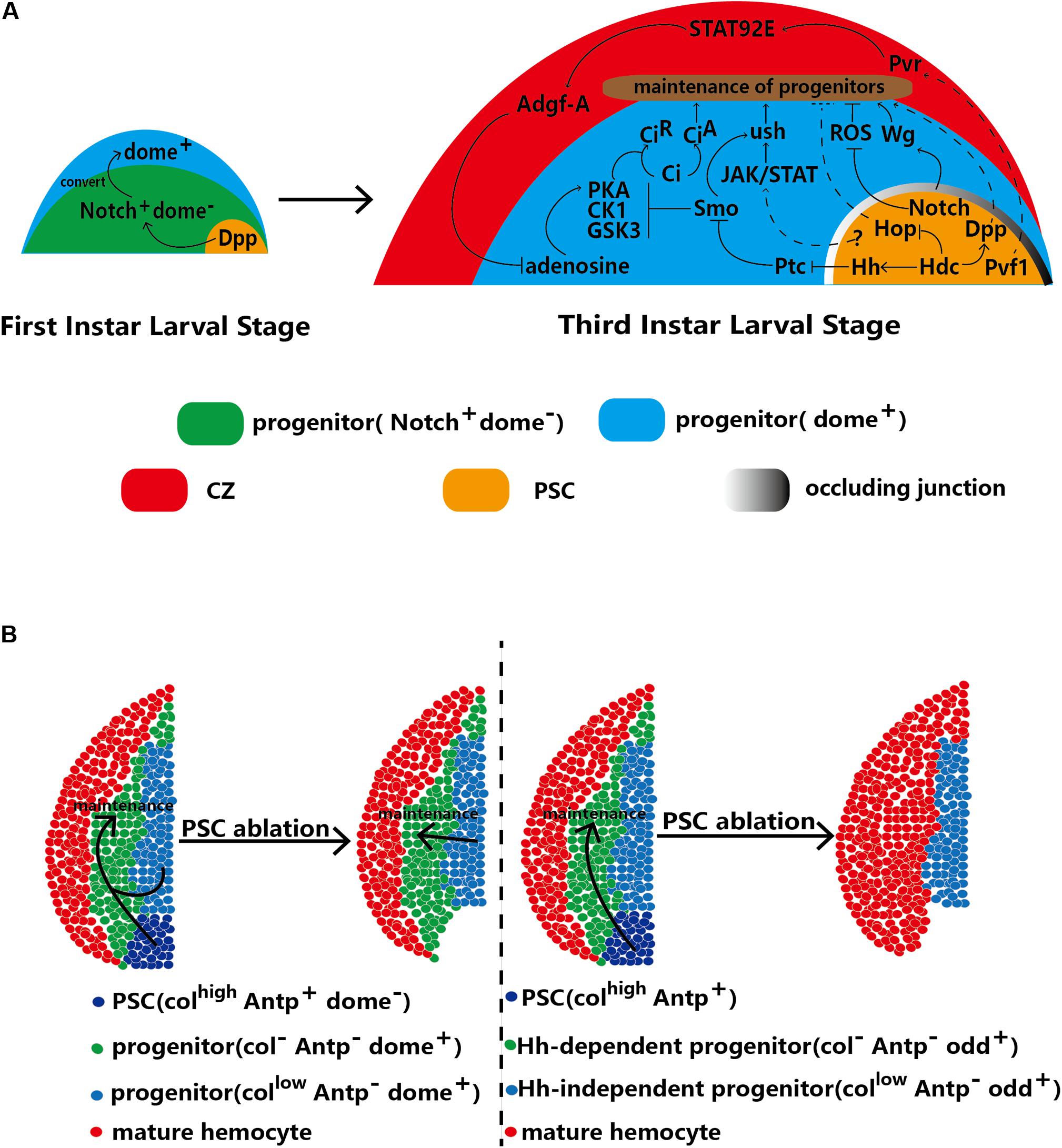
Figure 3. The PSC regulates the homeostasis of hematopoietic progenitors. (A) In first-instar larvae, the PSC regulates the conversion of Notch+/Dome– progenitors into Dome+ progenitors. At the third larval stage, the PSC maintains the homeostasis of hematopoietic progenitors through direct and indirect mechanisms. (B) Two contradictory models with differing views on the requirement of the PSC for hematopoietic progenitor maintenance. The left model indicates that the PSC (defined by Antp+ cells) is redundant and that progenitors with low Col expression are sufficient for the maintenance of hematopoietic progenitors. The right model indicates that Hh-dependent (Odd+ Col–) progenitors are maintained by the PSC. Upon ablation of the PSC, the progenitors in the subset differentiate into mature hemocytes, which results in a decrease in MZ size. Other Hh-independent progenitors are kept quiescent by autonomous maintenance.
Notably, Hh signaling is not induced until 18 h after egg hatching (AEH). This timing implies that before 18 h AEH, other signaling pathways play a role similar to that of the Hh pathway. There are no mature hemocytes in the lymph glands of early first-instar larvae (13 h AEH); only two types of hematopoietic progenitors, Notch+/domeless (Dome)– and Notch–/Dome+ cells, exist. Recently, PSC-secreted Dpp has been suggested to potentially play a role in progenitor maintenance. Dpp is a ligand of the BMP pathway that is secreted from PSC cells and contributes to converting early progenitors (progenitor precursors) into mature progenitors. For example, Dpp promotes the conversion of Notch+/Dome– progenitors into Dome+ progenitors at 30 h AEH. Then, these Dome+ progenitors form the MZ of the lymph gland at the third-instar larval stage. Weak Dpp secretion from PSC cells of first-instar larvae causes loss of Dome+ progenitors in the lymph gland at the third-instar stage (Dey et al., 2016; Figure 3A). In addition, knockdown of Headcase (Hdc), the homolog of the tumor suppressor HECA, in the PSC has been shown to enhance Dpp secretion from PSC cells and inhibit lamellocyte differentiation (Varga et al., 2019).
In the second and third larval stages, the Hh and Antp proteins are specifically expressed only in the PSC (Mandal et al., 2007). In general, the number of PSC cells reflects the strength of Hh signaling (Mandal et al., 2007; Benmimoun et al., 2012; Lam et al., 2014; Tokusumi et al., 2015), and an increase in PSC cell number strongly inhibits the differentiation of progenitors caused by high levels of Hh. However, some studies have produced results inconsistent with these findings. For example, blockade of occluding junctions in the PSC has been found to promote the proliferation of PSC cells but not to increase the levels of Hh, which can promote the differentiation of progenitors (Khadilkar et al., 2017). Col has been found to inhibit the proliferation of PSC cells; however, low levels of Col increase PSC cell numbers without inhibiting the differentiation of progenitors and decrease MZ size (Pennetier et al., 2012). The GATA factor Srp is a positive regulator of Hh signaling; however, knockdown of srp specifically in the PSC does not change PSC cell numbers (Tokusumi et al., 2010). Similarly, PSC-specific overexpression of Drosophila friend of GATA transcriptional regulator, U-shaped (ush), a negative regulator of Hh, has no effect on PSC cell numbers.
In addition to PSC-secreted Hh and Dpp, aberrant PSC impacts the maintenance of hematopoietic progenitors signaling. The Janus kinase (JAK)/signal transducer and activator of transcription (STAT) receptor Dome is regarded as a marker in hematopoietic progenitors of the MZ. JAK/STAT signaling maintains hematopoietic progenitors via Ush (Gao H. et al., 2009; Tokusumi et al., 2010). Moreover, Ush is regulated by Smo, a receptor of the Hh pathway (Baldeosingh et al., 2018). In addition, Krzemień et al. observed that PSC cells activate JAK/STAT signaling in the MZ. Loss of Col expression induces PSC cell loss and reduces Dome+ cell numbers in the MZ (Krzemień et al., 2007; Pennetier et al., 2012). In summary, the PSC, JAK/STAT pathway and Ush constitute a regulatory network that controls the differentiation of hematopoietic progenitors (Figure 3A). In addition, the JAK/STAT pathway in PSC cells regulates the differentiation of progenitors via an unknown mechanism. Knockdown of hdc induces lamellocyte differentiation, which can be reversed through knockdown of hop, a kinase for ligands of the JAK/STAT pathway in PSC cells (Varga et al., 2019). However, whether the JAK/STAT pathway in PSC cells contributes to the differentiation of MZ cells by affecting the JAK/STAT levels of progenitors needs to be further examined.
Wg signals also maintain hematopoietic progenitors (Sinenko et al., 2009). The permeability barrier consists of occluding junctions between PSC cells, which suggests that the Wg levels of PSC cells can extend to progenitors. Depletion of the permeability barrier reduces Wg signaling in both PSC cells and progenitors, thereby promoting the differentiation of progenitors (Khadilkar et al., 2017).
Notch signaling in hematopoietic progenitors promotes the differentiation of crystal cells (Ferguson and Martinez-Agosto, 2014). In addition, the Notch ligand Ser is regarded as another marker of PSC cells (Lebestky et al., 2003; Yu et al., 2018), and loss of ser decreases PSC cell numbers and promotes the differentiation of progenitors (Krzemień et al., 2007). However, recent studies have shown that there is no relationship between the strength of the Notch signal in the PSC and that in the MZ or CZ of the lymph gland (Small et al., 2014). Therefore, communication between the PSC and MZ through Notch signaling needs to be further defined. In 2009, ROS caused by oxidative stress were proven to promote differentiation of hematopoietic progenitors in the MZ (Owusu-Ansah and Banerjee, 2009). Small et al. showed that ROS in the MZ are inhibited by Notch signals of the PSC through an unknown pathway. Knockdown of the Notch signal in PSC cells causes high levels of ROS to accumulate in the MZ and numerous lamellocytes (Figure 3A; Small et al., 2014).
The PSC Indirectly Maintains the Homeostasis of Progenitors via Hemocyte Differentiation
Pvf1 originates from the PSC and is transported into the CZ, which contains Pvr (Mondal et al., 2011). Binding of Pvf1 to Pvr in the CZ results in a signaling cascade between the CZ and MZ in lymph glands. STAT92E is activated by Pvr and induces overexpression of adenosine deaminase-related growth factor A (Adgf-A). Next, Adgf-A downregulates adenosine in adjacent MZ cells, the function of which is to promote PKA to phosphorylate and degrade the key Hh signaling pathway component Ci into the active form CiA (Chen et al., 1998; Mondal et al., 2011), which contributes to the maintenance of hematopoietic progenitors. This cross-region regulatory pathway, called the equilibrium signaling pathway, is analogous to the Hh signaling pathway (Figure 3A; Mondal et al., 2011, 2014; Ferguson and Martinez-Agosto, 2017).
Necessity of the PSC for Hematopoietic Progenitor Maintenance
The PSC has traditionally been thought to be required for the maintenance of hematopoietic progenitors. However, in 2015, Benmimoun et al. raised doubts about the previous understanding of the relationship between the MZ and PSC (Crozatier et al., 2004; Benmimoun et al., 2015). These researchers found that a low level of Col expression in the MZ functioned similarly to that in the PSC by inhibiting the differentiation of hematopoietic progenitors. Furthermore, Col expression in the MZ and Col expression in the PSC were found to be independent. Antp is specifically expressed in the PSC at the third-instar larval stage. Surprisingly, neither ablation of antp through null mutation nor apoptosis of PSC cells driven by the proapoptotic protein Reaper (rpr) decreases the numbers of prohemocytes (cells in the MZ marked by Dome-GFP and Tep4). However, col null mutation promotes the differentiation of progenitors (Benmimoun et al., 2015). A follow-up study has also confirmed that PSC cells are not required for the maintenance of progenitors. Decreasing the number of PSC cells by knocking down Wg signaling or inhibiting the Hh signaling pathway of the PSC by knocking down Srp does not induce prohemocyte differentiation (Figure 3B; Oyallon et al., 2016). In this model, Col in MZ cells is sufficient for the maintenance of hematopoietic progenitors, and PSC cells appear redundant. Therefore, the traditional model in which the PSC functions to regulate hematopoietic progenitors has been questioned.
More recent studies have further confused the matter, leading to controversy. In 2018, Baldeosingh et al. examined the effects of specific ablation of PSC cells driven by rpr; however, their results contradicted those of the previous study. They analyzed the colocalization of Col and Odd in the MZ upon ablation of the PSC and found that the Col–Odd+ subpopulation of prohemocytes differentiated into mature hemocytes; however, other Odd+ prohemocytes expressing low levels of Col were maintained (Baldeosingh et al., 2018; Figure 3B). Sharma et al. (2019) split the MZ into Hh-independent and Hh-dependent progenitors, supporting this finding. These results may be more trustworthy than the former because prohemocytes were detected with various markers, such as Odd, E-cadherin, Dome-MESO-LacZ and Ci (Jung et al., 2005; Yu et al., 2018). In addition, two studies have shown that the Hh protein derived from PSC cells is a key component that regulates hematopoietic homeostasis (Mandal et al., 2007; Tokusumi et al., 2018). The potential redundancy of the PSC in the maintenance of progenitors calls into question the functions of signaling pathways derived from the PSC. However, to date, no alternative mechanism to the signaling pathways of the PSC has been defined. In this model, hematopoietic progenitors are classified into two subpopulations: Col-low and Col-negative progenitors. However, only Col-negative progenitors are required for PSC maintenance.
In summary, studies examining the regulatory functions of PSC cells have yielded several contradictory results; therefore, this topic requires deeper investigation. Interestingly, all of the studies discussed here have consistently shown that PSC cells are required for lamellocyte differentiation in response to wasp parasitism. When the PSC is ablated, progenitors fail to differentiate into lamellocytes in response to wasp parasitism (Crozatier et al., 2004; Krzemień et al., 2007; Benmimoun et al., 2015).
Role of the Psc in the Cellular Immune Response to Parasitic Wasp Infection
The parasitoid wasp Leptopilina boulardi is commonly used to infect fruit flies in order to study the cellular immune response to invasion (Carton and Nappi, 2001). The dedicated cellular immune response promotes the proliferation of hemocytes and induces massive differentiation into lamellocytes, whose function is to encapsulate wasp eggs (Lanot et al., 2001). At the end of infection, the lymph gland is disrupted and releases many lamellocytes (Krzemień et al., 2007). Notably, the PSC is more sensitive to wasp infection than other regions; the Toll/nuclear factor κB (NFκB) immune signaling pathway is activated in PSC cells even under normal conditions (Gueguen et al., 2013), suggesting that the high Toll/NFκB levels of the PSC allow this region to be constantly prepared to resist environmental infection. As described above, PSC undoubtedly plays a key role in the cellular immune response to parasitic wasps.
Wasp infection causes high levels of ROS to accumulate in the PSC, which can trigger the secretion of Spitz (s.spi), a ligand for epidermal growth factor receptor (EGFR), into the MZ, subsequently activating the EGFR signaling pathway of anterior lobe cells and causing massive lamellocyte differentiation in the lymph glands (Sinenko et al., 2012; Figure 4). In 2017, follow-up research showed that another signaling pathway triggered by high ROS levels in PSC cells contributes to the immune response to wasp infection (Louradour et al., 2017). ROS can promote cleavage of the Toll/NFκB pathway ligand Spätzle (Spz) by Spätzle-processing enzyme (SPE), therefore activating the Toll/NFκB pathway in the PSC (Jang et al., 2006). The activated NFκB pathway of the PSC induces breakdown of the permeability barrier (made of occluding junctions), which enables the immune signal to extend into the MZ (Khadilkar et al., 2017). Next, the Toll/NF-kB pathway of the MZ is activated and promotes the differentiation of lamellocytes. Finally, high levels of Dif and Toll pathway transcription factors contribute to the dispersion of mature hemocytes from the lymph gland into the circulation. These findings suggest that the EGFR and Toll/NFκB pathways may act in parallel in response to wasp infection in PSC cells to regulate lamellocyte differentiation. However, although the EGFR and Toll/NFκB pathways have similar functions and triggering mechanisms in hematopoiesis, the relationship between the two pathways is unclear (Sinenko et al., 2012; Louradour et al., 2017; Figure 4).
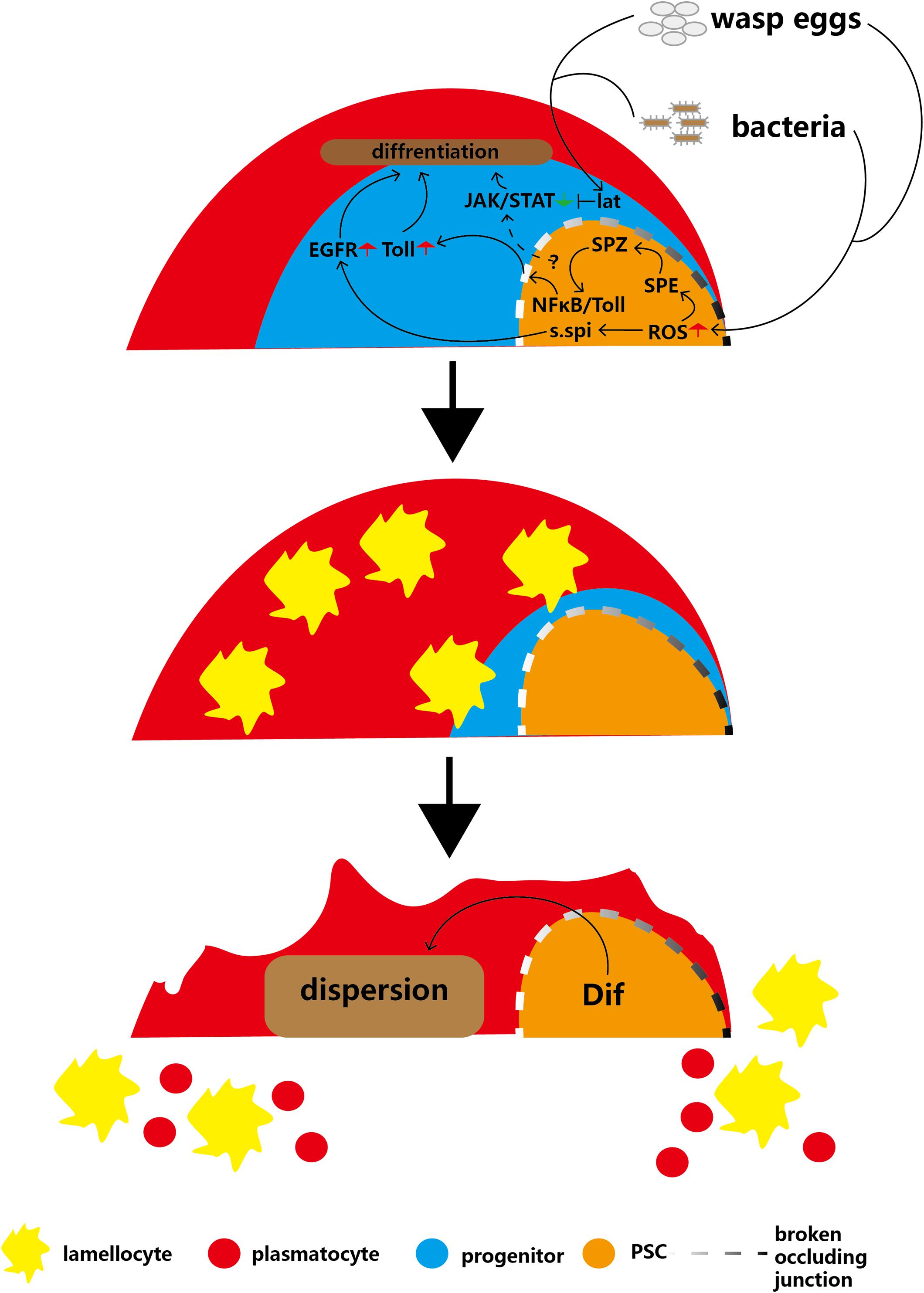
Figure 4. Infection with parasitic wasps or bacteria induces production of lamellocytes and disruption of the lymph gland. Parasitic wasp or bacterial infection induces a decrease in MZ size or an increase in ROS levels in the PSC, respectively, and causes activation of the EGFR and Toll pathways in the MZ. In addition, infections inhibit the JAK/STAT pathway through Lat, which overrides PSC maintenance JAK/STAT levels. These two pathways cause massive differentiation of lamellocytes. The Dif-dependent Toll/NFκB pathway contributes to the disruption of the lymph gland to release mature hemocytes into circulation.
In addition, PSC cells maintain JAK/STAT pathway signaling in hematopoietic progenitors to inhibit prohemocyte differentiation (Krzemień et al., 2007; Gao H. et al., 2009). After wasp infection, the massive prohemocyte differentiation into mature hemocytes suggests that JAK/STAT signaling in progenitors is deactivated because PSC cells lose the capacity to maintain the JAK/STAT pathway. In addition, a short type I cytokine-related receptor, latran (lat), is activated by wasp infection. Next, activated lat in hematopoietic progenitors inhibits the JAK/STAT pathway to promote the differentiation of prohemocytes into lamellocytes (Figure 4; Makki et al., 2010). This indicates that after wasp infection, inhibition of lat can override the PSC cell-mediated maintenance of the hematopoietic progenitor JAK/STAT pathway.
Psc of the Drosophila Lymph Gland as a Model for Investigating the Mammalian Bone Marrow Hematopoietic Stem Cell Niche
Similar HSC Niche Regulatory Mechanisms in Drosophila and Mammals
Mammalian hematopoietic stem cells (HSCs), which form the basis for mammalian hematopoietic processes, have the capability to produce all mature blood cell lineages. In Drosophila, the development of HSCs occurs in two main waves: the embryonic stage and the adult stage. During mammalian embryogenesis, HSC precursors migrate through different embryonic niches to develop into mature HSCs, which is reminiscent of the function of the Drosophila lymph gland PSC at the first larval stage (Mikkola and Orkin, 2006; Dey et al., 2016) (see section “The PSC Directly Maintains the Homeostasis of Hematopoietic Progenitors”). In adults, the main HSCs reside in the bone marrow niche. An HSC niche can be defined as a location from which housing and maintenance regulatory signals are derived. HSC niche regulatory signals cause HSCs to remain quiescent under normal conditions and to differentiate or proliferate during immune responses (Suda et al., 2005; Pinho and Frenette, 2019).
Two types of mammalian HSC niches have been identified: the endosteal niche and the perivascular niche. HSCs are attached to osteoblasts of trabecular bone in the endosteal niche and to the fenestrated endothelia of bone marrow sinusoids in the perivascular niche (Wilson and Trumpp, 2006; Kiel and Morrison, 2008; Celso et al., 2009; Xie et al., 2009). Two long-term problems in the field of mammalian HSC research are the difficulty in dissecting niches located at various anatomical sites and the lack of specific markers for identification of hematopoietic niches (Mikkola and Orkin, 2006; Pinho and Frenette, 2019). Compared to mammalian niches, the PSC of the Drosophila lymph gland is a unique HSC niche and expresses several specific markers (see section “PSC Cell Features”). In summary, the Drosophila PSC is an ideal model for investigating the mammalian HSC niche regulatory mechanism.
As described above, the Hh, Dpp/BMP, wingless, Notch and Slit/Robo signaling pathways regulate hematopoietic progenitors via the PSC. Hh signaling has been conserved throughout evolution, including in mammalian HSCs (Gao J. et al., 2009; Hofmann et al., 2009). Moreover, loss of BMP receptor 1A (BMPR1A) in the mouse bone marrow stroma increases the numbers of both osteoblasts and repopulating HSCs; this effect is similar to the phenotype induced by loss of the BMP signal in the Drosophila PSC (Zhang et al., 2003; Pennetier et al., 2012). Wnt in mammals is analogous to Wg in Drosophila (Siegfried and Perrimon, 1994; Cavallo et al., 1998; Packard et al., 2002). Overexpression of Wg in PSC cells promotes PSC cell proliferation and blocks hematopoietic progenitor differentiation (Sinenko et al., 2009; Pennetier et al., 2012), and similar observations have been made in mammals. Overexpression of Ctnnb1, a primary regulatory target of Wnt signaling, increases the HSC pool by blocking HSC differentiation (Kirstetter et al., 2006; Scheller et al., 2006). Notch also participates in developmental signaling pathways. The Drosophila PSC can be defined by a cell cluster expressing the Notch ligand Ser (Lebestky et al., 2003). Similarly, mammalian HSC niche osteoblasts express Notch1 and Notch3 and the ligands Jagged1 and Dll1 (Li et al., 1998; Stier et al., 2002; Dando et al., 2005). In addition, the Notch pathway in both mammalian HSC niches and the Drosophila PSC can affect hematopoietic differentiation. However, the detailed regulatory mechanism in the mammalian HSC niche, like that in the Drosophila PSC, remains unclear (Renström et al., 2010; Small et al., 2014). In Drosophila, Slit, secreted by the CT, binds to its receptor Robo in the PSC to activate the Slit/Robo signaling pathway (Morin-Poulard et al., 2016). Similarly, slit2-Robo4 regulates HSC localization in the bone marrow perivascular niche (Smith-Berdan et al., 2011, 2012). During infection, Toll-like receptors are expressed by mammalian HSCs and can sense pathogens. Upon activation, NFκB/Toll induces HSCs to differentiate into immune cells (Nagai et al., 2006; Zhao et al., 2014). Analogous to the case in mammals, elevations in ROS levels in Drosophila PSC cells caused by pathogen infection can activate NFκB/Toll signaling in hematopoietic progenitors and promote the differentiation of plasmatocytes and lamellocytes (see section “Role of the PSC in the Cellular Immune Response to Parasitic Wasp Infection”).
However, there are still some regulatory mechanisms of niche HSCs that are specific to either Drosophila or mammals. In mammals, loss of membrane-bound stem cell factor (SCF) decreases the numbers of osteoblasts and HSCs (McCulloch et al., 1965; Lyman and Jacobsen, 1998), and knockout of core-binding factor subunit α1 (CBFα1) causes complete loss of osteoblasts and HSCs (Komori et al., 1997; Otto et al., 1997; Deguchi et al., 1999). Although the Drosophila ortholog lozenge (lz) participates in crystal cell differentiation, there is no evidence that lz contributes to the regulation of niche HSCs (Evans et al., 2003). Constitutive activation of parathyroid hormone (PTH) or the PTH/PTH-related protein receptor (PPR) specifically in osteoblasts can increase both osteoblast and HSC populations in mouse bone marrow (Calvi et al., 2003). Furthermore, Cxcl12 and its receptor Cxcr4 have been shown to contribute to niche HSC regulation (Zou et al., 1998; Sugiyama et al., 2006; Tzeng et al., 2011).
Drosophila PSC and Leukemia
Leukemia is a kind of malignant disease whose symptoms include increased numbers of leucocytes in the blood or bone marrow. As observed in human leukemia, melanotic tumors, which are composed of aggregated hemocytes and large numbers of abnormal lamellocytes, can appear in Drosophila (Boulet et al., 2018). Leukemic stem/progenitor cells (LSCs) or leukemia-initiating cells (LICs) are the root mediators of leukemia initiation, drug resistance and relapse (Zhou and Xu, 2015; Pulikkan and Castilla, 2018). Reducing the maintenance of LSCs and LICs through niche regulatory signaling is a therapeutic strategy. The genetically conserved Hh pathway has been demonstrated to be required for leukemia-initiating cell differentiation (Dagklis et al., 2015; Lim et al., 2015), and Drosophila Hh signaling is derived from PSC cells (Mandal et al., 2007). In PSC cells, Hh signaling inhibits Ush expression, and direct knockdown of Hh signaling via Hh RNAi can result in a leukemia-like phenotype (Mandal et al., 2007; Baldeosingh et al., 2018). Furthermore, Hh signaling pathway inhibitors can be applied for clinical therapy of Bcr-Abl leukemia (Helgason et al., 2010). Feeding of PF-04449913, an inhibitor of the human Hh pathway receptor Smo, to Drosophila larvae significantly promotes LSC differentiation and blocks relapse (Giordani et al., 2016).
In mammals, LSCs can modulate and hijack the hematopoietic niche to outcompete normal HSCs (Nwajei and Konopleva, 2013; Pinho and Frenette, 2019), and similar capabilities have been observed in Drosophila. Notably, HOX transcription factors have been observed to be dysregulated in acute myeloid leukemia (AML). Misexpression of NA9 in mature Drosophila hemocytes using the UAS/Gal4 system not only promotes hemocyte proliferation and hyperplastic growth of the lymph gland but also induces PSC expansion (Baril et al., 2017). In addition, misexpression of NA9 can interfere with Pvr signaling, which is involved in leukemia (Stamm et al., 2018; Hattori et al., 2019). These observations suggest that proliferation of PSC cells is related to the leukemia-like phenotype.
Conclusion and Perspectives
The Drosophila lymph gland is a powerful model for studying hematopoiesis, and the PSC has become an ideal model for studying niche interactions with HSCs. However, some questions about the PSC remain to be answered. For example, whether the PSC is required for the maintenance of hematopoietic progenitors or whether Col-positive progenitors are sufficient for this maintenance remains an important question. In addition, whether the number of PSC cells represents the strength of the Hh signaling pathway is unclear. Furthermore, the detailed functions of filopodial extensions of PSC cells have yet to be elucidated. Finally, although the PSC is undoubtedly the unique niche of hematopoietic progenitors in the lymph gland, hematopoietic progenitors are very rarely found among circulating hemocytes (Osman et al., 2009; Sinenko et al., 2010); thus, whether some cells provide a niche for circulating hematopoietic progenitors deserves to be studied.
Overall, we have summarized several important points regarding the Drosophila lymph gland PSC: (1) that autonomous and non-autonomous regulatory networks maintain stable numbers of PSC cells; (2) that PSC cells regulate the homeostasis of hematopoietic progenitors not only directly but also indirectly via mature hemocyte equilibrium signals; (3) that the requirement of the PSC for the maintenance of hematopoietic progenitors is currently controversial; (4) that PSC cells play a key role in the immune response via elevations in ROS levels; and (5) that the Drosophila lymph gland PSC can be used to investigate the relationship between leukemia and the mammalian bone marrow hematopoietic niche.
Author Contributions
FL and SY contributed to the writing of this review article. LJ approved the final version of the manuscript. FL, SY, and LJ are accountable for the entire contents.
Funding
This work was supported by the National Natural Science Foundation of China (31772521).
Conflict of Interest
The authors declare that the research was conducted in the absence of any commercial or financial relationships that could be construed as a potential conflict of interest.
References
Akimaru, H., Chen, Y., Dai, P., Hou, D.-X., Nonaka, M., Smolik, S. M., et al. (1997). Drosophila CBP is a co-activator of cubitus interruptus in hedgehog signalling. Nature 386, 735–738. doi: 10.1038/386735a0
Aza-Blanc, P., Ramírez-Weber, F.-A., Laget, M.-P., Schwartz, C., and Kornberg, T. B. (1997). Proteolysis that is inhibited by hedgehog targets Cubitus interruptus protein to the nucleus and converts it to a repressor. Cell 89, 1043–1053. doi: 10.1016/s0092-8674(00)80292-5
Baldeosingh, R., Gao, H., Wu, X., and Fossett, N. (2018). Hedgehog signaling from the posterior signaling center maintains U-shaped expression and a prohemocyte population in Drosophila. Dev. Biol. 441, 132–145. doi: 10.1016/j.ydbio.2018.06.020
Baril, C., Gavory, G., Bidla, G., Knævelsrud, H., Sauvageau, G., and Therrien, M. (2017). Human NUP98-HOXA9 promotes hyperplastic growth of hematopoietic tissues in Drosophila. Dev. Biol. 421, 16–26. doi: 10.1016/j.ydbio.2016.11.003
Benmimoun, B., Polesello, C., Haenlin, M., and Waltzer, L. (2015). The EBF transcription factor Collier directly promotes Drosophila blood cell progenitor maintenance independently of the niche. Proc. Natl. Acad. Sci. U.S.A. 112, 9052–9057. doi: 10.1073/pnas.1423967112
Benmimoun, B., Polesello, C., Waltzer, L., and Haenlin, M. (2012). Dual role for Insulin/TOR signaling in the control of hematopoietic progenitor maintenance in Drosophila. Development 139, 1713–1717. doi: 10.1242/dev.080259
Bouchard, C., Staller, P., and Eilers, M. (1998). Control of cell proliferation by Myc. Trends Cell Biol. 8, 202–206.
Boulet, M., Miller, M., Vandel, L., and Waltzer, L. (2018). “From drosophila blood cells to human leukemia,” in Drosophila Models for Human Diseases, Ed. M. Yamaguchi, (Berlin: Springer), 195–214. doi: 10.1007/978-981-13-0529-0_11
Calvi, L., Adams, G., Weibrecht, K., Weber, J., Olson, D., Knight, M., et al. (2003). Osteoblastic cells regulate the haematopoietic stem cell niche. Nature 425, 841–846. doi: 10.1038/nature02040
Carton, Y., and Nappi, A. J. (2001). Immunogenetic aspects of the cellular immune response of Drosophila against parasitoids. Immunogenetics 52, 157–164. doi: 10.1007/s002510000272
Cavallo, R. A., Cox, R. T., Moline, M. M., Roose, J., Polevoy, G. A., Clevers, H., et al. (1998). Drosophila Tcf and Groucho interact to repress Wingless signalling activity. Nature 395, 604–608. doi: 10.1038/26982
Celso, C. L., Fleming, H. E., Wu, J. W., Zhao, C. X., Miake-Lye, S., Fujisaki, J., et al. (2009). Live-animal tracking of individual haematopoietic stem/progenitor cells in their niche. Nature 457, 92–96. doi: 10.1038/nature07434
Chen, C.-H., Von Kessler, D. P., Park, W., Wang, B., Ma, Y., and Beachy, P. A. (1999). Nuclear trafficking of Cubitus interruptus in the transcriptional regulation of Hedgehog target gene expression. Cell 98, 305–316. doi: 10.1016/s0092-8674(00)81960-1
Chen, Y., Gallaher, N., Goodman, R. H., and Smolik, S. M. (1998). Protein kinase A directly regulates the activity and proteolysis of cubitus interruptus. Proc. Natl. Acad. Sci. U.S.A. 95, 2349–2354. doi: 10.1073/pnas.95.5.2349
Crozatier, M., Ubeda, J.-M., Vincent, A., and Meister, M. (2004). Cellular immune response to parasitization in Drosophila requires the EBF orthologue collier. PLoS Biol. 2:e196. doi: 10.1371/journal.pbio.0020196
Crozatier, M., and Vincent, A. (2011). Drosophila: a model for studying genetic and molecular aspects of haematopoiesis and associated leukaemias. Dis. Models Mech. 4, 439–445. doi: 10.1242/dmm.007351
Dagklis, A., Pauwels, D., Lahortiga, I., Geerdens, E., Bittoun, E., Cauwelier, B., et al. (2015). Hedgehog pathway mutations in T-cell acute lymphoblastic leukemia. Haematologica 100:e102.
Dando, J. S., Tavian, M., Catelain, C., Poirault, S., Bennaceur-Griscelli, A., Sainteny, F., et al. (2005). Notch/Delta4 interaction in human embryonic liver CD34+ CD38- cells: positive influence on BFU-E production and LTC-IC potential maintenance. Stem Cells 23, 550–560. doi: 10.1634/stemcells.2004-0205
Deguchi, K., Yagi, H., Inada, M., Yoshizaki, K., Kishimoto, T., and Komori, T. (1999). Excessive extramedullary hematopoiesis in Cbfa1-deficient mice with a congenital lack of bone marrow. Biochem. Biophys. Res. Commun. 255, 352–359. doi: 10.1006/bbrc.1999.0163
Dey, N. S., Ramesh, P., Chugh, M., Mandal, S., and Mandal, L. (2016). Dpp dependent Hematopoietic stem cells give rise to Hh dependent blood progenitors in larval lymph gland of Drosophila. eLife 5:e18295. doi: 10.7554/eLife.18295
Evans, C. J., Hartenstein, V., and Banerjee, U. (2003). Thicker than blood: conserved mechanisms in Drosophila and vertebrate hematopoiesis. Dev. Cell 5, 673–690. doi: 10.1016/s1534-5807(03)00335-6
Ferguson, G. B., and Martinez-Agosto, J. A. (2014). Yorkie and Scalloped signaling regulates Notch-dependent lineage specification during Drosophila hematopoiesis. Curr. Biol. 24, 2665–2672. doi: 10.1016/j.cub.2014.09.081
Ferguson, G. B., and Martinez-Agosto, J. A. (2017). The TEAD family transcription factor Scalloped regulates blood progenitor maintenance and proliferation in Drosophila through PDGF/VEGFR receptor (Pvr) signaling. Dev. Biol. 425, 21–32. doi: 10.1016/j.ydbio.2017.03.016
Gallant, P. (2009). Drosophila Myc. Adv. Cancer Res. 103, 111–144. doi: 10.1016/S0065-230X(09)03005-X
Gao, H., Wu, X., and Fossett, N. (2009). Upregulation of the Drosophila Friend of GATA gene U-shaped by JAK/STAT signaling maintains lymph gland prohemocyte potency. Mol. Cell. Biol. 29, 6086–6096. doi: 10.1128/MCB.00244-09
Gao, J., Graves, S., Koch, U., Liu, S., Jankovic, V., Buonamici, S., et al. (2009). Hedgehog signaling is dispensable for adult hematopoietic stem cell function. Cell Stem Cell 4, 548–558. doi: 10.1016/j.stem.2009.03.015
Ghosh, S., Singh, A., Mandal, S., and Mandal, L. (2015). Active hematopoietic hubs in Drosophila adults generate hemocytes and contribute to immune response. Dev. Cell 33, 478–488. doi: 10.1016/j.devcel.2015.03.014
Giordani, G., Barraco, M., Giangrande, A., Martinelli, G., Guadagnuolo, V., Simonetti, G., et al. (2016). The human Smoothened inhibitor PF-04449913 induces exit from quiescence and loss of multipotent Drosophila hematopoietic progenitor cells. Oncotarget 7:55313. doi: 10.18632/oncotarget.10879
Grigorian, M., Mandal, L., and Hartenstein, V. (2011). Hematopoiesis at the onset of metamorphosis: terminal differentiation and dissociation of the Drosophila lymph gland. Dev. Genes Evol. 221, 121–131. doi: 10.1007/s00427-011-0364-6
Gueguen, G., Kalamarz, M. E., Ramroop, J., Uribe, J., and Govind, S. (2013). Polydnaviral ankyrin proteins aid parasitic wasp survival by coordinate and selective inhibition of hematopoietic and immune NF-kappa B signaling in insect hosts. PLoS Pathog. 9:e1003580. doi: 10.1371/journal.ppat.1003580
Hao, Y., and Jin, L. H. (2017). Dual role for Jumu in the control of hematopoietic progenitors in the Drosophila lymph gland. eLife 6:e25094. doi: 10.7554/eLife.25094
Hattori, N., Kawaguchi, Y., Sasaki, Y., Shimada, S., Murai, S., Abe, M., et al. (2019). Monitoring TIGIT/DNAM-1 and PVR/PVRL2 immune checkpoint expression levels in allogeneic stem cell transplantation for acute myeloid leukemia. Biol. Blood Marrow Transplant. 25, 861–867. doi: 10.1016/j.bbmt.2019.01.013
Helgason, G. V., Young, G. A., and Holyoake, T. L. (2010). Targeting chronic myeloid leukemia stem cells. Curr. Hematol. Malignancy Rep. 5, 81–87. doi: 10.1007/s11899-010-0043-0
Hofmann, I., Stover, E. H., Cullen, D. E., Mao, J., Morgan, K. J., Lee, B. H., et al. (2009). Hedgehog signaling is dispensable for adult murine hematopoietic stem cell function and hematopoiesis. Cell Stem Cell 4, 559–567. doi: 10.1016/j.stem.2009.03.016
Holz, A., Bossinger, B., Strasser, T., Janning, W., and Klapper, R. (2003). The two origins of hemocytes in Drosophila. Development 130, 4955–4962. doi: 10.1242/dev.00702
Honti, V., Csordás, G., Márkus, R., Kurucz, É, Jankovics, F., and Andó, I. (2010). Cell lineage tracing reveals the plasticity of the hemocyte lineages and of the hematopoietic compartments in Drosophila melanogaster. Mol. Immunol. 47, 1997–2004. doi: 10.1016/j.molimm.2010.04.017
Jang, I.-H., Chosa, N., Kim, S.-H., Nam, H.-J., Lemaitre, B., Ochiai, M., et al. (2006). A Spätzle-processing enzyme required for toll signaling activation in Drosophila innate immunity. Dev. Cell 10, 45–55. doi: 10.1016/j.devcel.2005.11.013
Jung, S.-H., Evans, C. J., Uemura, C., and Banerjee, U. (2005). The Drosophila lymph gland as a developmental model of hematopoiesis. Development 132, 2521–2533. doi: 10.1242/dev.01837
Khadilkar, R. J., Rodrigues, D., Mote, R. D., Sinha, A. R., Kulkarni, V., Magadi, S. S., et al. (2014). ARF1–GTP regulates Asrij to provide endocytic control of Drosophila blood cell homeostasis. Proc. Natl. Acad. Sci. U.S.A. 111, 4898–4903. doi: 10.1073/pnas.1303559111
Khadilkar, R. J., Vogl, W., Goodwin, K., and Tanentzapf, G. (2017). Modulation of occluding junctions alters the hematopoietic niche to trigger immune activation. eLife 6:e28081. doi: 10.7554/eLife.28081
Kiel, M. J., and Morrison, S. J. (2008). Uncertainty in the niches that maintain haematopoietic stem cells. Nat. Rev. Immunol. 8, 290–301. doi: 10.1038/nri2279
Kirstetter, P., Anderson, K., Porse, B. T., Jacobsen, S. E. W., and Nerlov, C. (2006). Activation of the canonical Wnt pathway leads to loss of hematopoietic stem cell repopulation and multilineage differentiation block. Nat. Immunol. 7, 1048–1056. doi: 10.1038/ni1381
Komori, T., Yagi, H., Nomura, S. A., Sasaki, K., Deguchi, K., et al. (1997). Targeted disruption of Cbfa1 results in a complete lack of bone formation owing to maturational arrest of osteoblasts. Cell 89, 755–764. doi: 10.1016/s0092-8674(00)80258-5
Krzemień, J., Dubois, L., Makki, R., Meister, M., Vincent, A., and Crozatier, M. (2007). Control of blood cell homeostasis in Drosophila larvae by the posterior signalling centre. Nature 446, 325–328. doi: 10.1038/nature05650
Kulkarni, V., Khadilkar, R. J., Srivathsa, M., and Inamdar, M. S. (2011). Asrij maintains the stem cell niche and controls differentiation during Drosophila lymph gland hematopoiesis. PLoS One 6:e27667. doi: 10.1371/journal.pone.0027667
Kurucz, É, Váczi, B., Márkus, R., Laurinyecz, B., Vilmos, P., Zsámboki, J., et al. (2007). Definition of Drosophila hemocyte subsets by cell-type specific antigens. Acta Biol. Hung. 58, 95–111. doi: 10.1556/ABiol.58.2007.Suppl.8
Lam, V., Tokusumi, T., Tokusumi, Y., and Schulz, R. A. (2014). bantam miRNA is important for Drosophila blood cell homeostasis and a regulator of proliferation in the hematopoietic progenitor niche. Biochem. Biophys. Res. Commun. 453, 467–472. doi: 10.1016/j.bbrc.2014.09.109
Lanot, R., Zachary, D., Holder, F., and Meister, M. (2001). Postembryonic hematopoiesis in Drosophila. Dev. Biol. 230, 243–257.
Lebestky, T., Chang, T., Hartenstein, V., and Banerjee, U. (2000). Specification of Drosophila hematopoietic lineage by conserved transcription factors. Science 288, 146–149. doi: 10.1126/science.288.5463.146
Lebestky, T., Jung, S.-H., and Banerjee, U. (2003). A Serrate-expressing signaling center controls Drosophila hematopoiesis. Genes Dev. 17, 348–353. doi: 10.1101/gad.1052803
Li, L., Milner, L. A., Deng, Y., Iwata, M., Banta, A., Graf, L., et al. (1998). The human homolog of rat Jagged1expressed by marrow stroma inhibits differentiation of 32D cells through interaction with Notch1. Immunity 8, 43–55. doi: 10.1016/s1074-7613(00)80457-4
Lim, Y., Gondek, L., Li, L., Wang, Q., Ma, H., Chang, E., et al. (2015). Integration of Hedgehog and mutant FLT3 signaling in myeloid leukemia. Sci. Transl. Med. 7:291ra296. doi: 10.1126/scitranslmed.aac9303
Louradour, I., Sharma, A., Morin-Poulard, I., Letourneau, M., Vincent, A., Crozatier, M., et al. (2017). Reactive oxygen species-dependent Toll/NF-κB activation in the Drosophila hematopoietic niche confers resistance to wasp parasitism. eLife 6:e25496. doi: 10.7554/eLife.25496
Lum, L., and Beachy, P. A. (2004). The Hedgehog response network: sensors, switches, and routers. Science 304, 1755–1759. doi: 10.1126/science.1098020
Lum, L., Yao, S., Mozer, B., Rovescalli, A., Von Kessler, D., Nirenberg, M., et al. (2003). Identification of Hedgehog pathway components by RNAi in Drosophila cultured cells. Science 299, 2039–2045. doi: 10.1126/science.1081403
Lyman, S. D., and Jacobsen, S. E. W. (1998). c-kit ligand and Flt3 ligand: stem/progenitor cell factors with overlapping yet distinct activities. Blood J. Am. Soc. Hematol. 91, 1101–1134.
Makki, R., Meister, M., Pennetier, D., Ubeda, J.-M., Braun, A., Daburon, V., et al. (2010). A short receptor downregulates JAK/STAT signalling to control the Drosophila cellular immune response. PLoS Biol. 8:e1000441. doi: 10.1371/journal.pbio.1000441
Mandal, L., Banerjee, U., and Hartenstein, V. (2004). Evidence for a fruit fly hemangioblast and similarities between lymph-gland hematopoiesis in fruit fly and mammal aorta-gonadal-mesonephros mesoderm. Nat. Genet. 36, 1019–1023. doi: 10.1038/ng1404
Mandal, L., Martinez-Agosto, J. A., Evans, C. J., Hartenstein, V., and Banerjee, U. (2007). A Hedgehog-and Antennapedia-dependent niche maintains Drosophila haematopoietic precursors. Nature 446, 320–324. doi: 10.1038/nature05585
McCulloch, E. A., Siminovitch, L., Till, J. E., Russell, E. S., and Bernstein, S. E. (1965). The cellular basis of the genetically determined hemopoietic defect in anemic mice of genotype Sl/Sld. Blood 26, 399–410. doi: 10.1182/blood-2016-05-716142
Medvinsky, A., and Dzierzak, E. (1996). Definitive hematopoiesis is autonomously initiated by the AGM region. Cell 86, 897–906. doi: 10.1016/s0092-8674(00)80165-8
Mikkola, H. K., and Orkin, S. H. (2006). The journey of developing hematopoietic stem cells. Development 133, 3733–3744. doi: 10.1242/dev.02568
Mondal, B. C., Mukherjee, T., Mandal, L., Evans, C. J., Sinenko, S. A., Martinez-Agosto, J. A., et al. (2011). Interaction between differentiating cell-and niche-derived signals in hematopoietic progenitor maintenance. Cell 147, 1589–1600. doi: 10.1016/j.cell.2011.11.041
Mondal, B. C., Shim, J., Evans, C. J., and Banerjee, U. (2014). Pvr expression regulators in equilibrium signal control and maintenance of Drosophila blood progenitors. eLife 3:e03626. doi: 10.7554/eLife.03626
Morin-Poulard, I., Sharma, A., Louradour, I., Vanzo, N., Vincent, A., and Crozatier, M. (2016). Vascular control of the Drosophila haematopoietic microenvironment by Slit/Robo signalling. Nat. Commun. 7, 1–12. doi: 10.1038/ncomms11634
Nagai, Y., Garrett, K. P., Ohta, S., Bahrun, U., Kouro, T., Akira, S., et al. (2006). Toll-like receptors on hematopoietic progenitor cells stimulate innate immune system replenishment. Immunity 24, 801–812. doi: 10.1016/j.immuni.2006.04.008
Nwajei, F., and Konopleva, M. (2013). The bone marrow microenvironment as niche retreats for hematopoietic and leukemic stem cells. Adv. Hematol. 2013:953982. doi: 10.1155/2013/953982
Osman, D., Gobert, V., Ponthan, F., Heidenreich, O., Haenlin, M., and Waltzer, L. (2009). A Drosophila model identifies calpains as modulators of the human leukemogenic fusion protein AML1-ETO. Proc. Natl. Acad. Sci. U.S.A. 106, 12043–12048. doi: 10.1073/pnas.0902449106
Otto, F., Thornell, A. P., Crompton, T., Denzel, A., Gilmour, K. C., Rosewell, I. R., et al. (1997). Cbfa1, a candidate gene for cleidocranial dysplasia syndrome, is essential for osteoblast differentiation and bone development. Cell 89, 765–771. doi: 10.1016/s0092-8674(00)80259-7
Owusu-Ansah, E., and Banerjee, U. (2009). Reactive oxygen species prime Drosophila haematopoietic progenitors for differentiation. Nature 461, 537–541. doi: 10.1038/nature08313
Oyallon, J., Vanzo, N., Krzemień, J., Morin-Poulard, I., Vincent, A., and Crozatier, M. (2016). Two independent functions of Collier/Early B cell factor in the control of Drosophila blood cell homeostasis. PLoS One 11:e0148978. doi: 10.1371/journal.pone.0148978
Packard, M., Koo, E. S., Gorczyca, M., Sharpe, J., Cumberledge, S., and Budnik, V. (2002). The Drosophila Wnt, wingless, provides an essential signal for pre-and postsynaptic differentiation. Cell 111, 319–330. doi: 10.1016/s0092-8674(02)01047-4
Pennetier, D., Oyallon, J., Morin-Poulard, I., Dejean, S., Vincent, A., and Crozatier, M. (2012). Size control of the Drosophila hematopoietic niche by bone morphogenetic protein signaling reveals parallels with mammals. Proc. Natl. Acad. Sci. U.S.A. 109, 3389–3394. doi: 10.1073/pnas.1109407109
Pinho, S., and Frenette, P. S. (2019). Haematopoietic stem cell activity and interactions with the niche. Nat. Rev. Mol. Cell Biol. 20, 303–320. doi: 10.1038/s41580-019-0103-9
Price, M. A., and Kalderon, D. (2002). Proteolysis of the Hedgehog signaling effector Cubitus interruptus requires phosphorylation by Glycogen Synthase Kinase 3 and Casein Kinase 1. Cell 108, 823–835. doi: 10.1016/s0092-8674(02)00664-5
Pulikkan, J. A., and Castilla, L. H. (2018). Preleukemia and leukemia-initiating cell activity in inv (16) acute myeloid leukemia. Front. Oncol. 8:129. doi: 10.3389/fonc.2018.00129
Rämet, M., Lanot, R., Zachary, D., and Manfruelli, P. (2002). JNK signaling pathway is required for efficient wound healing in Drosophila. Dev. Biol. 241, 145–156. doi: 10.1006/dbio.2001.0502
Renström, J., Kröger, M., Peschel, C., and Oostendorp, R. A. (2010). How the niche regulates hematopoietic stem cells. Chem. Biol. Interact 184, 7–15. doi: 10.1016/j.cbi.2009.11.012
Rizki, R. M., and Rizki, T. (1990). Encapsulation of parasitoid eggs in phenoloxidase-deficient mutants of Drosophila melanogaster. J. Insect Physiol. 36, 523–529. doi: 10.1016/0014-4894(90)90131-u
Rizki, T., and Rizki, R. M. (1992). Lamellocyte differentiation in Drosophila larvae parasitized by Leptopilina. Dev. Comp. Immunol. 16, 103–110. doi: 10.1016/0145-305x(92)90011-z
Robertson, H. M., Preston, C. R., Phillis, R. W., Johnson-Schlitz, D. M., Benz, W. K., and Engels, W. R. (1988). A stable genomic source of P element transposase in Drosophila melanogaster. Genetics 118, 461–470.
Scheller, M., Huelsken, J., Rosenbauer, F., Taketo, M. M., Birchmeier, W., Tenen, D. G., et al. (2006). Hematopoietic stem cell and multilineage defects generated by constitutive β-catenin activation. Nat. Immunol. 7, 1037–1047. doi: 10.1038/ni1387
Sharma, S. K., Ghosh, S., Geetha, A. R., Mandal, S., and Mandal, L. (2019). Cell adhesion-mediated actomyosin assembly regulates the activity of cubitus interruptus for hematopoietic progenitor maintenance in Drosophila. Genetics 212, 1279–1300. doi: 10.1534/genetics.119.302209
Shim, J., Gururaja-Rao, S., and Banerjee, U. (2013). Nutritional regulation of stem and progenitor cells in Drosophila. Development 140, 4647–4656. doi: 10.1242/dev.079087
Shim, J., Mukherjee, T., and Banerjee, U. (2012). Direct sensing of systemic and nutritional signals by haematopoietic progenitors in Drosophila. Nat. Cell Biol. 14, 394–400. doi: 10.1038/ncb2453
Shrestha, R., and Gateff, E. (1982). Ultrastructure and cytochemistry of the cell types in the larval hematopoietic organs and hemolymph of drosophila melanogaster. Dev. Growth Differ. 24, 65–82.
Siegfried, E., and Perrimon, N. (1994). Drosophila wingless: a paradigm for the function and mechanism of Wnt signaling. Bioessays 16, 395–404. doi: 10.1002/bies.950160607
Sinenko, S. A., Hung, T., Moroz, T., Tran, Q., Sidhu, S., Cheney, M. D., et al. (2010). Genetic manipulation of AML1-ETO–induced expansion of hematopoietic precursors in a Drosophila model. Blood 116, 4612–4620. doi: 10.1182/blood-2010-03-276998
Sinenko, S. A., Mandal, L., Martinez-Agosto, J. A., and Banerjee, U. (2009). Dual role of wingless signaling in stem-like hematopoietic precursor maintenance in Drosophila. Dev. Cell 16, 756–763. doi: 10.1016/j.devcel.2009.03.003
Sinenko, S. A., Shim, J., and Banerjee, U. (2012). Oxidative stress in the haematopoietic niche regulates the cellular immune response in Drosophila. EMBO Rep. 13, 83–89. doi: 10.1038/embor.2011.223
Small, C., Ramroop, J., Otazo, M., Huang, L. H., Saleque, S., and Govind, S. (2014). An unexpected link between notch signaling and ROS in restricting the differentiation of hematopoietic progenitors in Drosophila. Genetics 197, 471–483. doi: 10.1534/genetics.113.159210
Smith-Berdan, S., Nguyen, A., Hassanein, D., Zimmer, M., Ugarte, F., Ciriza, J., et al. (2011). Robo4 cooperates with CXCR4 to specify hematopoietic stem cell localization to bone marrow niches. Cell Stem Cell 8, 72–83. doi: 10.1016/j.stem.2010.11.030
Smith-Berdan, S., Schepers, K., Ly, A., Passegué, E., and Forsberg, E. C. (2012). Dynamic expression of the Robo ligand Slit2 in bone marrow cell populations. Cell Cycle 11, 675–682. doi: 10.4161/cc.11.4.19146
Sousa-Nunes, R., Yee, L. L., and Gould, A. P. (2011). Fat cells reactivate quiescent neuroblasts via TOR and glial insulin relays in Drosophila. Nature 471, 508–512. doi: 10.1038/nature09867
Stamm, H., Klingler, F., Grossjohann, E.-M., Muschhammer, J., Vettorazzi, E., Heuser, M., et al. (2018). Immune checkpoints PVR and PVRL2 are prognostic markers in AML and their blockade represents a new therapeutic option. Oncogene 37, 5269–5280. doi: 10.1038/s41388-018-0288-y
Stier, S., Cheng, T., Dombkowski, D., Carlesso, N., and Scadden, D. T. (2002). Notch1 activation increases hematopoietic stem cell self-renewal in vivo and favors lymphoid over myeloid lineage outcome. Blood J. Am. Soc. Hematol. 99, 2369–2378. doi: 10.1182/blood.v99.7.2369
Suda, T., Arai, F., and Hirao, A. (2005). Hematopoietic stem cells and their niche. Trends Immunol. 26, 426–433.
Sugiyama, T., Kohara, H., Noda, M., and Nagasawa, T. (2006). Maintenance of the hematopoietic stem cell pool by CXCL12-CXCR4 chemokine signaling in bone marrow stromal cell niches. Immunity 25, 977–988. doi: 10.1016/j.immuni.2006.10.016
Tennessen, J. M., and Thummel, C. S. (2011). Coordinating growth and maturation—insights from Drosophila. Curr. Biol. 21, R750–R757. doi: 10.1016/j.cub.2011.06.033
Tepass, U., Fessler, L. I., Aziz, A., and Hartenstein, V. (1994). Embryonic origin of hemocytes and their relationship to cell death in Drosophila. Development 120, 1829–1837.
Tokusumi, T., Tokusumi, Y., Hopkins, D. W., and Schulz, R. A. (2015). Bag of Marbles controls the size and organization of the Drosophila hematopoietic niche through interactions with the Insulin-like growth factor pathway and Retinoblastoma-family protein. Development 142, 2261–2267. doi: 10.1242/dev.121798
Tokusumi, T., Tokusumi, Y., Hopkins, D. W., Shoue, D. A., Corona, L., and Schulz, R. A. (2011). Germ line differentiation factor Bag of Marbles is a regulator of hematopoietic progenitor maintenance during Drosophila hematopoiesis. Development 138, 3879–3884. doi: 10.1242/dev.069336
Tokusumi, T., Tokusumi, Y., and Schulz, R. A. (2018). The mir-7 and bag of marbles genes regulate Hedgehog pathway signaling in blood cell progenitors in Drosophila larval lymph glands. Genesis 56:e23210. doi: 10.1002/dvg.23210
Tokusumi, Y., Tokusumi, T., Shoue, D. A., and Schulz, R. A. (2012). Gene regulatory networks controlling hematopoietic progenitor niche cell production and differentiation in the Drosophila lymph gland. PLoS One 7:e41604. doi: 10.1371/journal.pone.0041604
Tokusumi, Y., Tokusumi, T., Stoller-Conrad, J., and Schulz, R. A. (2010). Serpent, suppressor of hairless and U-shaped are crucial regulators of hedgehog niche expression and prohemocyte maintenance during Drosophila larval hematopoiesis. Development 137, 3561–3568. doi: 10.1242/dev.053728
Tzeng, Y.-S., Li, H., Kang, Y.-L., Chen, W.-C., Cheng, W.-C., and Lai, D.-M. (2011). Loss of Cxcl12/Sdf-1 in adult mice decreases the quiescent state of hematopoietic stem/progenitor cells and alters the pattern of hematopoietic regeneration after myelosuppression. Blood J. Am. Soc. Hematol. 117, 429–439. doi: 10.1182/blood-2010-01-266833
Varga, G. I., Csordás, G., Cinege, G., Jankovics, F., Sinka, R., and Kurucz, É, et al. (2019). Headcase is a Repressor of Lamellocyte Fate in Drosophila melanogaster. Genes 10:173. doi: 10.3390/genes10030173
Ward, E. J., and Coulter, D. E. (2000). odd-skipped is expressed in multiple tissues during Drosophila embryogenesis. Mech. Dev. 96, 233–236. doi: 10.1016/s0925-4773(00)00389-0
Wilson, A., and Trumpp, A. (2006). Bone-marrow haematopoietic-stem-cell niches. Nat. Rev. Immunol. 6, 93–106. doi: 10.1038/nri1779
Xie, Y., Yin, T., Wiegraebe, W., He, X. C., Miller, D., Stark, D., et al. (2009). Detection of functional haematopoietic stem cell niche using real-time imaging. Nature 457, 97–101. doi: 10.1038/nature07639
Yu, S., Luo, F., and Jin, L. H. (2018). The Drosophila lymph gland is an ideal model for studying hematopoiesis. Dev. Compar. Immunol. 83, 60–69. doi: 10.1016/j.dci.2017.11.017
Zhang, J., Niu, C., Ye, L., Huang, H., He, X., Tong, W.-G., et al. (2003). Identification of the haematopoietic stem cell niche and control of the niche size. Nature 425, 836–841. doi: 10.1038/nature02041
Zhang, L., Jia, J., Wang, B., Amanai, K., Wharton, K. A. Jr., and Jiang, J. (2006). Regulation of wingless signaling by the CKI family in Drosophila limb development. Dev. Biol. 299, 221–237. doi: 10.1016/j.ydbio.2006.07.025
Zhao, J. L., Ma, C., O’Connell, R. M., Mehta, A., DiLoreto, R., Heath, J. R., et al. (2014). Conversion of danger signals into cytokine signals by hematopoietic stem and progenitor cells for regulation of stress-induced hematopoiesis. Cell Stem Cell 14, 445–459. doi: 10.1016/j.stem.2014.01.007
Zhou, H., and Xu, R. (2015). Leukemia stem cells: the root of chronic myeloid leukemia. Protein Cell 6, 403–412. doi: 10.1007/s13238-015-0143-7
Keywords: Drosophila, lymph gland, posterior signaling center, hematopoietic stem cells niche, differentiation, signaling network, immune response, leukemia
Citation: Luo F, Yu S and Jin LH (2020) The Posterior Signaling Center Is an Important Microenvironment for Homeostasis of the Drosophila Lymph Gland. Front. Cell Dev. Biol. 8:382. doi: 10.3389/fcell.2020.00382
Received: 19 February 2020; Accepted: 28 April 2020;
Published: 21 May 2020.
Edited by:
Atsushi Asakura, University of Minnesota Twin Cities, United StatesCopyright © 2020 Luo, Yu and Jin. This is an open-access article distributed under the terms of the Creative Commons Attribution License (CC BY). The use, distribution or reproduction in other forums is permitted, provided the original author(s) and the copyright owner(s) are credited and that the original publication in this journal is cited, in accordance with accepted academic practice. No use, distribution or reproduction is permitted which does not comply with these terms.
*Correspondence: Li Hua Jin, lhjin2000@hotmail.com; lhjin@nefu.edu.cn