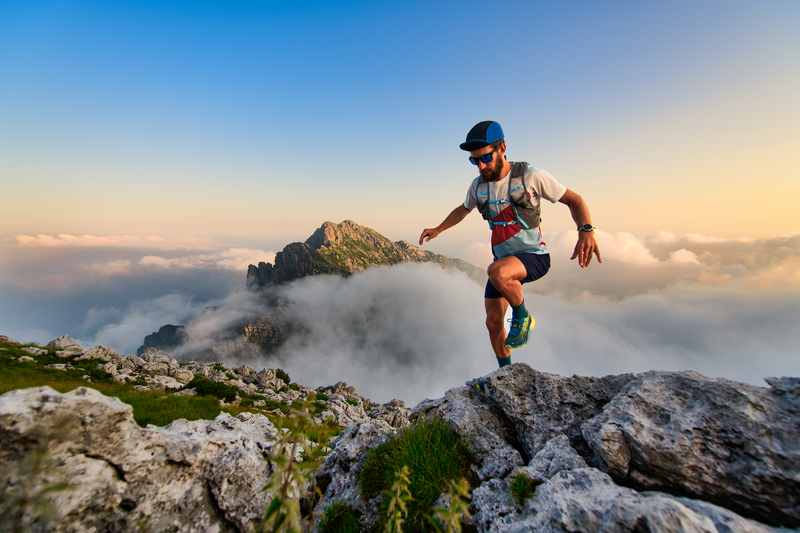
94% of researchers rate our articles as excellent or good
Learn more about the work of our research integrity team to safeguard the quality of each article we publish.
Find out more
REVIEW article
Front. Cell Dev. Biol. , 21 February 2020
Sec. Membrane Traffic and Organelle Dynamics
Volume 8 - 2020 | https://doi.org/10.3389/fcell.2020.00086
This article is part of the Research Topic 2021: Highlights in Membrane Traffic View all 19 articles
Parkinson’s disease (PD) is the second most prevalent neurodegenerative disease in the world, after Alzheimer’s disease (AD), affecting approximately 1% of people over 65 years of age. Exosomes were once considered to be cellular waste and functionless. However, our understanding about exosome function has increased, and exosomes have been found to carry specific proteins, lipids, functional messenger RNAs (mRNAs), high amounts of non-coding RNAs (including microRNAs, lncRNAs, and circRNAs) and other bioactive substances. Exosomes have been shown to be involved in many physiological processes in vivo, including intercellular communication, cell migration, angiogenesis, and anti-tumor immunity. Moreover, exosomes may be pivotal in the occurrence and progression of various diseases. Therefore, exosomes have several diverse potential applications due to their unique structure and function. For instance, exosomes may be used as biological markers for the diagnosis and prognosis of various diseases, or as a natural carrier of drugs for clinical treatment. Here, we review the potential roles of exosomes in the pathogenesis, diagnosis, treatment, and prognosis of PD.
Parkinson’s disease (PD) is the second most common neurodegenerative disease in the world, after Alzheimer’s disease (AD) (Kalia and Lang, 2015). PD affects 0.3% of the whole population, and the percentage rises to 1% of the population above 65 years of age (De Lau and Breteler, 2006; Ascherio and Schwarzschild, 2016). The clinical symptoms of PD patients include resting tremor, muscular rigidity, bradykinesia, and postural instability. Prior to the eventual appearance of motor symptoms, non-motor symptoms can be observed in PD patients, including hyposmia, constipation, urinary dysfunction, depression, anxiety, and rapid eye movement sleep behavior disorder (RBD) (Schapira et al., 2017). Most cases of PD are idiopathic, although 10–15% of cases are genetic (Deng et al., 2018). Thus far, 23 genes related to PD (PARK genes) have been identified. Mutations in SNCA, LRRK2, and VSP32 cause autosomal dominant inheritance of PD, whereas mutations in PRKN, PINK1, and DJ-1 demonstrate autosomal recessive inheritance. Moreover, a mutation in glucocerebrosidase 1 (GBA1) which causes Gaucher disease has been identified as a potential genetic risk factor for PD (Nichols et al., 2009), and both Gaucher disease patients and obligate carriers are predisposed to PD. In addition to genetic factors, environmental factors are also crucial in PD pathogenesis. For example, personal exposure to noxious chemicals such as 1-methyl-4-phenyl-1,2,3,6-tetrahydropyridine (MPTP) (Langston et al., 1983), rotenone (Betarbet et al., 2000), and paraquat (Elbaz et al., 2009; Tanner et al., 2011) can trigger PD symptoms. The risks for developing PD associated with heavy mental exposure were also investigated, but the correlation remained unclear. Notably, some studies have reported a reduced risk of developing PD among smokers (Hernán et al., 2001) and coffee drinkers (Ross et al., 2000; Palacios et al., 2012).
The hallmarks of PD pathology are lesions in the substantia nigra pars compacta (SNpc) and striatum, including the presence of cytoplasmic inclusion bodies known as Lewy bodies (LBs), and progressive loss of dopaminergic neurons (Dickson, 2012). LBs are mainly composed of filamentous α-synuclein (α-syn), a small protein of indefinite function that is ubiquitously expressed in the brain. In PD patients, α-syn becomes abnormally phosphorylated and aggregated (Spillantini et al., 1998). PD movement disorders become more and more severe as the disease progresses due to the continuing death of dopaminergic neurons in SNpc, even though other brain regions are also affected. Although α-syn misfolding and aggregation are central to the development of the disease, several other mechanisms are implicated in PD pathogenesis (Goedert et al., 2013). Indeed, mitochondrial dysfunction (Bose and Beal, 2016), neuroinflammation (Hirsch and Hunot, 2009), and abnormal protein clearance systems, including the ubiquitin-proteasome system (UPS) (McKinnon and Tabrizi, 2014) and the autophagy-lysosomal pathway (ALP) (Tanji et al., 2011), all play a role in PD pathogenesis. Although all of these processes are known to participate in the onset and progression of PD, the relationship between these pathways remains unknown.
Extracellular vesicles (EVs) were first described in plasma by Wolf some 50 years ago as “platelet dust” (Wolf, 1967). There are three main types of EVs and these are distinguished by their size and their different mechanisms of biogenesis and release. Microvesicles (MVs) and apoptotic bodies are 50–500 nm in diameter, and both are secreted directly from the cytoplasmic membrane by living or dying cells (Colombo et al., 2014). Exosomes, the smallest EVs, are 50–150 nm in diameter and are secreted from the cytoplasmic membrane into the extracellular environment via fusion with late endosomes or multivesicular bodies (MVBs). This type of vesicle was first identified in the rat (Harding et al., 1983) and sheep (Pan et al., 1985) reticulocytes and subsequently labeled as “exosomes” by Johnstone et al. (1987) and Johnstone (2005). Exosomes can be released by a wide range of cells, including neurons, blood cells, epithelial cells, immune cells, and cancer cells. Exosomes have been isolated from a variety of biological fluids, including cerebrospinal fluid (CSF), plasma, serum, saliva, urine, semen, breast milk, amniotic fluid, and ascites fluid. Besides, exosomes have been isolated in vitro from cell culture supernatants (Théry et al., 2006). This review aimed to examine the potential roles of exosomes in the pathogenesis, diagnosis, treatment, and prognosis of PD. Since circulating exosomes cannot definitively be distinguished from MVs using currently available purification methods, we refer to EVs rather than exosomes when referring to biomarker studies in this review.
Exosome biogenesis begins with the endosome system. The cytoplasmic membrane invaginates to form early endosomes, and while some are recycled by the Golgi apparatus, the majority of early endosomes develop into late endosomes (also called MVBs). ILVs are formed during the inward budding of the endosomal membrane into the lumen of MVBs (Klumperman and Raposo, 2014) (Figure 1). The whole process can be divided into ESCRT-dependent and ESCRT-independent mechanisms; the molecules involved in the biogenesis and release of exosomes are summarized in Table 1.
Figure 1. Biogenesis and secretion of exosomes. Exosome biogenesis begins in the endosome pathway. The cytoplasmic membrane invaginates to form early endosomes, parts of early endosomes are recycled by the Golgi apparatus, but the majority of them mature into late endosomes (also called MVBs). The intraluminal vesicles (ILVs) in MVBs either secrete to the extracellular environment to form exosomes or are targeted to lysosomes for degradation. The autophagy pathway is proposed to interact with the endosome pathway, and the autophagosomes and MVBs can fuse to form amphisomes, which can be degraded by lysosomes or secreted extracellularly.
The Endosomal Sorting Complex Required for Transport (ESCRT) mechanism was first identified in the 2000s (Katzmann et al., 2001). The ESCRT system is composed of four complexes ESCRT-0, -I, -II, -III, and their associated proteins. ESCRT-0 proteins HRS and STAM are involved in cargo sorting of ubiquitinated proteins (Hanson and Cashikar, 2012; Henne et al., 2013). ESCRT-I proteins TSG101 and ESCRT-II are responsible for bud formation. ESCRT-III induces vesicle scission.
In the ESCRT system, dissociation and recycling of vesicles is driven by the accessory proteins ALIX and VPS4. ALIX was recently found to interact with syntenin, which acts as a cytoplasmic adaptor of heparan sulfate proteoglycan receptors. VPS4 is an ATPase associated with various cellular activities (AAA + ATPase) and is involved in the final step of ILV formation.
In addition to ESCRT-dependent mechanisms, some studies have shown that exosome biogenesis can occur in the absence of ESCRT proteins. Lipids, tetraspanins, and heat shock proteins are all involved in ESCRT-independent mechanisms (Stuffers et al., 2009). After inhibiting the expression of ESCRT proteins, exosomes expressing proteolipid protein (PLP) are normally released by oligodendroglia cells. Sphingomyelinase, an enzyme involved in producing ceramide from sphingomyelin, plays a role in exosome biogenesis and secretion in oligodendroglia cells (Trajkovic et al., 2008). These findings are consistent with the high levels of ceramide in exosomes. Ceramides are proposed to facilitate inward budding of MVBs to form ILVs. Phospholipase D2 (PLD2), an enzyme involved in synthesizing the signal molecule phosphatidic acid (PA) from phospholipids, also influences exosome biogenesis (Laulagnier et al., 2004). As observed with ceramide, PA can also induce inward curvature of the MVB membrane to form ILVs. Lipids such as cholesterol are also enriched in exosomes (Strauss et al., 2010).
In addition to lipids, the Tetraspanin family of four transmembrane domain proteins, which are enriched in exosomes, have also been proposed to participate in sorting cargoes for exosome secretion. Finally, the chaperone Hsc70 also plays a role in cargo sorting for exosomes. Hsc70 mediates the association between cytosolic constituents and exosomal membrane proteins (Géminard et al., 2004). Recently, Hsc70 was shown to bind cytosolic proteins containing a KFERQ-motif, and to induce their selective transport to ILVs (Sahu et al., 2011).
Although the biogenesis of exosomes is divided into ESCRT-dependent and ESCRT-independent pathways, the two pathways cannot be totally separated. Moreover, multiple mechanisms may simultaneously occur in a single MVB, and different populations of exosomes may depend on different pathways.
After ILVs have formed in MVBs, the MVBs can either be transported to lysosomes for degradation or directed to the cytoplasmic membrane for exosome secretion. Transportation of MVBs to the cytoplasmic membrane requires actin and microtubule cytoskeletons and associated molecular motors, such as kinesins and myosins (Villarroya-Beltri et al., 2014). Silencing or overexpressing cortactin, an actin-binding protein, was confirmed to reduce or increase the exosome secretion, respectively (Sinha et al., 2016).
Members of the Rab GTPase family, the largest family of small GTPase proteins, play vital roles in several critical MVB transportation processes, including vesicles membrane budding, trafficking of vesicles with tubulin and actin, docking of vesicles to targeted compartments, and fusion of vesicles with the cytoplasmic membrane (Stenmark, 2009).
Upon transportation of MVBs to the cytoplasmic membrane, MVBs fuse with the cytoplasmic membrane. During this process, numerous interactions between proteins and lipids can reduce the energy barrier and facilitate the fusion. Soluble N-ethylmaleimide-sensitive factor attachment proteins receptors (SNAREs) complexes are intimately involved in fusion with intracellular membranes (Zylbersztejn and Galli, 2011). The SNAREs family is comprised of vesicle-associated SNAREs (v-SNAREs) and target membrane-associated SNAREs (t-SNAREs). Generally, during the membrane fusion process, v-SNAREs interact with t-SNAREs to form a trans-SNARE complex (Wang et al., 2017). SNARE proteins including vesicle-associated membrane protein 7 (VAMP7), vesicle-associated membrane protein 8 (VAMP8), and SNAP-23 are involved in exocytosis of lysosomes regulated by Ca2+ (Puri and Roche, 2008). However, whether SNAREs induce the fusion of MVBs with the cytoplasmic membrane is controversial.
Cell type and cellular homeostasis also have an essential effect on the pathways of exosome secretion. MVBs are targeted to lysosomes for degradation or to the cytoplasmic membrane for secretion depending on cellular homeostasis. Under stress conditions, such as hypoxia (King et al., 2012), irradiation (Lehmann et al., 2008), and ER stress (Kanemoto et al., 2016), the number of intracellular MVBs increase and more exosomes are released. Thus, exosome secretion may be an alternative route for stressful cells to clear waste products. The secreted exosomes may be degraded by phagocytes, or may communicate with neighboring cells to induce pathological conditions. Similarly, an association between exosome secretion and autophagy has been proposed. Autophagy is a degradative pathway for the elimination of cellular waste, damaged organelles, and aggregated proteins, and functions to maintain cellular homeostasis. The cargoes to be excreted are first packaged into autophagosomes, and the autophagosomes are then either directly targeted to lysosomes for degradation or fused with MVBs to form amphisomes. The amphisomes can be degraded in lysosomes or secreted extracellularly (Boya et al., 2013). The two pathways do somewhat overlap. However, the induction of autophagy by starvation can decrease exosome secretion. Moreover, inhibition of autophagic degradation induced by inhibiting the fusion of lysosomes with both autophagosomes and MVBs could enhance the secretion of exosomes (Fader et al., 2008).
Parkinson’s disease is characterized by the histopathological formation of LBs which predominantly contain misfolded α-syn. The conspicuous motor symptoms occur because of the progressive loss of dopaminergic neurons in the SNpc and striatum. However, preceding motor symptoms, patients can display some non-motor symptoms including hyposmia, gastrointestinal dysfunction, and sleep disorders (Khoo et al., 2013). Although LBs are initially found in the periphery, they gradually spread to the brain stem, and ultimately to the cerebral cortex. Following these observations, a hypothesis has been proposed that PD may begin in the enteric nervous system or olfactory bulbs and then spread to other brain regions during disease progression (Braak et al., 2003b; Del Tredici and Braak, 2016). Currently, PD progression is unexplained, but α-syn has been thought to propagate in a prion-like process (Olanow and Brundin, 2013). Moreover, exosomes are proposed to play an important role in the progression of PD (Figure 2).
Figure 2. Exosomes as mediators for cell-to-cell communication in the pathogenesis of PD. Exosomal α-syn is readily transmitted between neurons and neuroglia cells. Exosomes provide an environment for α-syn aggregation, and can potentially promote the propagation of α-syn oligomers in the CNS. Activated neuroglia cells increase the release of exosomes and pro-inflammatory cytokines, thus exacerbating neuroinflammation and the progression of PD.
Several experiments showed that α-syn is directly released into the extracellular environment or packaged into exosomes via the endosome pathway (Lee et al., 2005). However, the underlying mechanisms by which α-syn is sorted into exosomes remains unclear. In addition, α-syn reportedly interacts with synaptic vesicles to facilitate SNAREs assembly and promote neurotransmitter release (Alvarez-Erviti et al., 2010). The synaptic vesicles containing α-syn can be sorted into early endosomes by the Golgi apparatus or clathrin-mediated endocytosis (Alvarez-Erviti et al., 2011b). Next, with the assistance of VPS4 and SUMO (Small Ubiquitin-like Modifier) proteins, α-syn containing endosomes transition into MVBs and fuse with the plasma membrane for secretion as exosomal cargoes (Cabin et al., 2002). Alternatively, α-syn containing endosomes can be sorted into recycling endosomes and be exocytosed as secretory granules in a Rab11a-dependent manner (Ben Gedalya et al., 2009). In all processes, the secretion of exosomal α-syn from cells is regulated by the intracellular calcium concentration (Emmanouilidou et al., 2010) (Figure 3).
Figure 3. Proposed pathway for sorting α-syn into exosomes. Exosomes participate in the secretion of α-syn in an endosome-dependent mechanism. First, α-syn is packaged into early endosomes. Next, α-syn containing exosomes can be secreted by two pathways. With the assistance of VPS4 and SUMO, it can be secreted as exosomal cargoes upon fusion of MVBs with the cytoplasmic membrane. Alternatively, α-syn containing exosomes can be sorted into recycling endosomes and be exocytosed as secretory granules in a Rab11a-dependent way.
Although the levels of α-syn contained in exosomes are low, recent studies suggest that exosomes provide an ideal environment for α-syn to aggregate, and potentially promote the propagation of PD pathology (Grey et al., 2015). It is widely accepted that oligomeric α-syn is the toxic form of α-syn, responsible for neuronal death. Danzer et al. (2012) identified the presence of oligomeric α-syn in exosomes and demonstrated that α-syn in exosomes is more easily taken up by recipient cells than free α-syn (Danzer et al., 2012). Stuendl et al. (2016) reported that exosomes from the CSF of patients with PD and dementia with Lewy bodies (DLB) could induce the formation of α-syn oligomers (Stuendl et al., 2016). Together, these findings prove that exosomal α-syn is intimately involved in the transmission of α-syn oligomers between cells.
Exosomes may function as intercellular carriers allowing α-syn to propagate in PD patients. Li et al. (2008) found LBs formation in transplanted neurons within recipients with PD, suggesting disease propagation from host-to-graft (Li et al., 2008). In the long-term embryonic nigral transplants in PD, Kordower et al. (2008) observed LB-like pathology (Kordower et al., 2008). Moreover, Recasens et al. (2014) extracted LBs from the brains of PD patients and transplanted them into mice and monkeys, eventually triggering α-syn aggregation and neurodegeneration (Recasens et al., 2014). In in vitro experiments, α-syn in exosomes were isolated from the conditioned medium of SH-SY5Y cells following overexpression of wild-type α-syn. Subsequently, Alvarez-Erviti et al. (2011b) demonstrated that exosomes isolated from SH-SY5Y cells which overexpress α-syn could transfer α-syn to normal SH-SY5Y cells (Alvarez-Erviti et al., 2011b). Finally, Emmanouilidou et al. (2010) were able to show that α-syn containing exosomes can promote the cell death of recipient neuronal cells, providing support for the hypothesis that α-syn propagation between neurons facilitates PD progression (Emmanouilidou et al., 2010).
Many different pathways are involved in the secretion of α-syn by exosomes. One of the characteristics of PD pathology is the failure of protein clearance by the UPS and the ALP. If either of the two pathways is compromised, intracellular α-syn aggregation occurs (Alvarez-Erviti et al., 2011b). Inhibition of ALP has been found to increase exosomal α-syn release, while concomitantly reducing α-syn aggregation in the cell (Lee et al., 2013). Although inhibition of ALP can protect cells by the reduction of intracellular α-syn levels and an increase in exosomal α-syn secretion, it can also promote the intercellular transfer of α-syn by exosomes and lead to the propagation of PD pathology (Poehler et al., 2014).
In addition to neuron-to-neuron communication, exosomes can also communicate between neurons and neuroglia cells. It has been reported that α-syn secreted from neurons can be phagocytosed by microglial cells (Alvarez-Erviti et al., 2011a) and astrocytes (Lee et al., 2010) in order to eliminate the toxic α-syn. However, the overload of α-syn in neuroglia cells can trigger a neuroinflammatory response. Chang et al. (2013) reported that α-syn could induce increased exosome release by BV-2 microglial cells, and this can cause increased cell apoptosis when co-cultured with cortical neurons (Chang et al., 2013). In addition, microglial cells and monocytes isolated from young (but not old) mice showed increased phagocytosis of exosomal α-syn oligomers and decreased secretion of the pro-inflammatory cytokines TNF-α (Bliederhaeuser et al., 2016). These studies suggest that microglia cells in the aged brain can exacerbate neurodegeneration by being unable to eliminate α-syn oligomers or by accelerating the secretion of exosomal α-syn oligomers. All these findings suggest that exosomes secreted by activated microglia cells may be vital factors in the neurodegeneration and progression of PD.
Inflammation is a critical process in PD progression (Panaro and Cianciulli, 2012). A proper inflammatory response is essential for tissue repair, but an excessive and delayed inflammatory response may lead to a malignant cycle of neuroinflammation and propagation of the disease (Gao and Hong, 2008). Exosomes may participate in different stages of the inflammatory process, including the activation stage, through neuron-to-neuroglia communication, and the exacerbation stage, through neuroglia-to-neuroglia communication (Figure 2). Thus far, the exact role of exosomes in neuroinflammation has not been completely elucidated, and more research is essential.
MicroRNAs (miRNAs) are notoriously involved in PD pathogenesis, and can be packaged in exosomes. Exosomes and miRNAs form a network that, both individually and synergistically, participates in the pathogenesis of PD among several other diseases (Mirzaei et al., 2017; Li et al., 2018; Saeedi Borujeni et al., 2018; Pourhanifeh et al., 2019).
Of note, certain miRNAs target PD-related genes. McMillan et al. (2017) observed that miR-7 can combine with the 3′-untranslated region (UTR) of SNCA mRNA to inhibit its transcription, and loss of miR-7 leads to α-syn aggregation and dopaminergic neuronal loss in the brains of PD patients (McMillan et al., 2017). Chen et al. (2017) found significantly upregulated expression of miR-4639-5p in PD patients, which negatively regulated the post-transcription level of the PD-associated gene DJ-1, eventually causing severe oxidative stress and neuronal death (Chen et al., 2017).
Jiang Y. et al. (2019) reported that exosomal microRNA-137 (miR-137) is upregulated and plays a vital role in the induction of oxidative stress of neurons in PD. miR-137 was found to directly target oxidation resistance 1 (OXR1) and negatively regulate its expression, thus inducing oxidative stress in PD (Jiang Y. et al., 2019). In a manganese-induced PD cell model, the levels of 12 miRNAs were significantly increased in EVs, including miR-210-5p, miR-128-1-5p, miR-505-5p, miR-325-5p, miR-16-5p, miR-1306-5p, miR-669b-5p, miR-125b-5p, miR-450b-3p, miR-24-2-5p, miR-6516-3p, and miR-1291. These miRNAs were shown to regulate key pathways in the pathogenesis of PD, including protein aggregation, autophagy, and inflammation (Harischandra et al., 2018). Toll-like receptor (TLR) is a type of innate immune receptor that when activated can cause release of inflammatory cytokines. Winkler et al. (2014) reported that exosomes can transport miRNA let-7 to activate TLR7 in neuronal cells and consequently lead to neurodegenerative diseases (Winkler et al., 2014).
Although the role of exosomes in the pathogenesis of PD has been confirmed, it is still necessary to explore the molecular mechanisms which control and regulate exosome biogenesis, secretion, and communication with recipient cells both in vivo and in vitro. Additional research concerning cell-to-cell transfer and propagation of α-syn, inflammatory mediators, and microRNAs between neurons and neuroglia cells, can broaden our understanding of the mechanisms of PD occurrence and progression, and allow for the development of new strategies for the diagnosis and treatment for PD and other neurodegenerative diseases.
Nowadays, PD is mainly diagnosed by the appearance of noticeable clinical motor symptoms (Postuma et al., 2015). However, prior to the occurrence of motor symptoms, some non-motor symptoms are evident (Chaudhuri et al., 2006). There is currently no useful diagnosis for early stage PD. The development of a method to diagnose PD early would be an important breakthrough. Previous analysis of disease-related constituents of EVs (including exosomes) isolated from the blood or cerebrospinal fluid (CSF) of PD patients suggests that EVs can be efficient biomarkers of PD (Vella et al., 2016). The potential biomarkers in EVs of PD are summarized in Table 2 (ROC: Receiver Operating Characteristic Curve; AUV: Area Under the Curve).
α-syn aggregation in LBs is a hallmark of PD pathology (Braak et al., 2003a). Although α-syn can be separated from blood and CSF, it cannot be usefully as a significant biomarker of PD due to its low abundance. In the CSF of PD patients, α-syn has consistently been shown to be present at a lower level compared to healthy controls. Stuendl et al. (2016) reported that levels of EV α-syn are also lower in the CSF of PD patients, which is consistent with the low levels of total α-syn in CSF (Stuendl et al., 2016). However, the use of plasma and serum α-syn as a biomarker has proved to be ineffective and inconsistent, because peripheral blood cells can also produce α-syn. Shi et al. (2014) reported that α-syn can easily be transported from the CSF to blood, and that some α-syn was packaged into EVs expressing neural cell adhesion molecule L1 (L1CAM), which is the central nervous system (CNS) specific (Shi et al., 2014). Moreover, they were able to demonstrate that levels of EV α-syn in plasma derived from the CNS are significantly higher in PD patients and the levels are related to the degree of the disease (Shi et al., 2014). In view of this, the authors suggest that CNS-derived EV α-syn in plasma can be used as a biomarker for PD with high specificity and sensitivity (Shi et al., 2014). Recently, Cao et al. (2019) found that the absolute levels of α-syn oligomers and the α-syn oligomers/total α-syn ratio are both higher in salivary EVs isolated from PD patients compared with healthy controls (Cao et al., 2019). Rani et al. (2019) were able to show that CNS-derived salivary EVs increased and that the levels of phosphorylated α-syn in these salivary EVs are significantly higher in PD patients than in healthy controls (Rani et al., 2019). Although Tau is mainly thought to be important in AD, Shi et al. (2016) reported that tau in CNS-derived EVs isolated from human plasma was significantly higher in PD patients compared with AD patients (Shi et al., 2016).
Kitamura et al. (2018) isolated EVs from the plasma of PD patients at Hoehn and Yahr (HY) stage II and III to identify candidate biomarkers for PD progression. They found that levels of clusterin, apolipoprotein A1, and complement C1r subcomponent were prominently decreased in PD patients at HY stage II and III compared with healthy controls. In particular, in PD patients at HY stage III, the levels of apolipoprotein A1 were substantially decreased compared with PD patients at HY stage II. Therefore, these three EV proteins may be candidate biomarkers for PD, it should be noted that apolipoprotein A1 levels are also relevant to the progression of PD (Kitamura et al., 2018). Similarly, Jiang R. et al. (2019) isolated EV proteins from the serum of PD patients, and observed that the expression of afamin, apolipoprotein D and J, and pigmented epithelium-derived factor, were prominently increased, whereas the levels of complement C1q and protein Immunoglobulin Lambda Variable 1-33 (IGLV1-33) Cluster -33 were decreased in PD patients (Jiang R. et al., 2019).
Mutations in DJ-1 and LRRK2 have been related to familial and sporadic PD. Zhao et al. (2018) reported that the levels of DJ-1 in EVs derived from CNS, and the ratio of EV DJ-1 to total DJ-1 derived from CNS, were substantially higher in the plasma of PD patients compared to healthy controls (Zhao et al., 2018). Ho et al. (2014) found that the levels of DJ-1 and LRRK2 in urine EVs in Korean PD patients are dependent on gender. Thus, DJ-1 levels were prominently higher in male PD patients and the levels increased in an age-dependent manner. However, the sample size in their study was small (Ho et al., 2014). Fraser et al. (2016a) examined whether the levels of auto-phosphorylated Ser(P)-1292 LRRK2 in urine EVs could predict LRRK2 mutation carriers (LRRK2+) and non-carriers (LRRK2-) with or without PD. The results revealed that these levels could predict LRRK2 mutation status using the elevated ratio of Ser(P)-1292 LRRK2 to total LRRK2 in urine EVs. Furthermore, patients with PD demonstrated a higher ratio than those without PD among carriers with the LRRK2 mutation (Fraser et al., 2016a). Overall, Fraser et al. (2016a) measured the levels of auto-phosphorylated Ser(P)-1292 LRRK2 in urine EVs in 79 idiopathic PD patients and 79 healthy controls. They found that the Ser(P)-1292 LRRK2 levels were higher in men compared to women and that they increased in idiopathic PD patients in contrast to healthy controls (Fraser et al., 2016a). Ser(P)-1292 LRRK2 levels were also shown to be related to the severity of cognitive impairment (Fraser et al., 2016b). Therefore, Ser(P)-1292 LRRK2 may be used as a biomarker for both familial and idiopathic PD.
In addition to proteins, various RNA species are present in EVs. Gui et al. (2015) isolated EV miRNAs from CSF, and revealed that 16 miRNAs were higher and 11 miRNAs were lower in PD patients in contrast to healthy controls. Among those, miR-153, miR-409-3p, miR-10a-5p, and let-7g-3p were prominently increased in CSF EVs from PD patients, while miR-1 and miR-19b-3p were prominently decreased (Gui et al., 2015). Cao et al. (2017) collected serum samples and isolated EV miRNAs from serum. They found that miR-24 and miR-195 were prominently upregulated, whereas miR-19b was prominently downregulated in serum EVs isolated from PD patients, as compared with healthy controls (Cao et al., 2017). Yao et al. (2018) found that the expression of circulating EV miR-331-5p was higher in the plasma of PD patients, while the expression of circulating EV miR-505 was lower, as compared with healthy controls (Yao et al., 2018).
The above studies suggest that EVs may be useful tools for the diagnosis of PD. However, large-scale clinical trials should be conducted to verify the specificity and sensitivity. Moreover, new techniques to isolate higher numbers of pure exosomes while excluding contaminants are required to improve the diagnostic value of exosomes.
Currently, treatments for PD do not cure the disease, although many drugs can relieve the motor symptoms. However, as PD progresses, these drugs can produce adverse effects (Müller, 2012). Therefore, there is an urgent need for the discovery of new drugs or methods to treat PD.
Most of the drugs trialed for CNS diseases failed during clinical trials because that they cannot cross the blood-brain barrier(BBB) (Pardridge, 2012). However, exosomes can cross the BBB as natural nano-scaled vesicles, and they can be used as drug-delivery vehicles (Zhuang et al., 2011; Lai and Breakefield, 2012) (Figure 4). Qu et al. (2018) isolated exosomes from human blood, and loaded exosomes with a saturated solution of dopamine. In in vivo and in vitro experiments, the authors showed that blood exosomes could cross the BBB and deliver dopamine into the brain via an interaction between transferrin and transferrin receptor. The exosomes loaded with dopamine had a better therapeutic effect in the PD mice model and showed less toxicity than free dopamine by intravenously systemic administration (Qu et al., 2018). Haney et al. (2015) developed a new exosome delivery system loaded with catalase, which is a potent antioxidant, using monocytes and macrophages. These exosomes were taken up by neurons, and the catalase released could ameliorate neural inflammation and increase neural survival in in vivo or in vitro PD models (Haney et al., 2015). In addition, Kojima et al. (2018) reported a set of EXOsomal transfer into cells (EXOtic) devices that produces designer exosomes in engineered mammalian cells to deliver therapeutic catalase mRNA to the brain (Kojima et al., 2018).
Figure 4. Exosomes as nano-delivery vehicles for PD treatment. It is proposed that exosomes can be used as nano-delivery vehicles for therapeutic drugs, proteins, siRNAs, shRNAs, and miRNAs. Exosomes are obtained from natural human cells or artificially synthesized, and then modified with therapeutic components in vitro, and finally re-injected into circulation. Exosomes can cross the BBB and reach the targeted cells to release their functional cargoes for therapeutic purposes (Aryani and Denecke, 2016).
Moreover, exosomes carrying specific exogenous small interfering RNAs (siRNAs) can be therapeutic for PD. In S129D α-syn transgenic mice, systemic injection of modified exosomes loaded with siRNA to α-syn can reduce the amount of α-syn mRNA transcription and protein translation (Cooper et al., 2014). Due to the short-term efficacy of siRNA, shRNA minicircles (shRNA-MCs) were designed by Izco et al. (2019). RVG-exosomes were used to deliver anti-α-syn shRNA-MCs to the PD mouse model induced by preformed α-syn fibrils. They found this treatment reduced the aggregation of α-syn, decreased the death of dopaminergic neurons, and ameliorated the clinical symptoms (Izco et al., 2019).
Exosomes derived from mesenchymal stem cells (MSCs) have been considered as an effective tool for treatment, and there use has been shown to be beneficial in different pathological conditions, including osteoarthritis (Mianehsaz et al., 2019), multiple sclerosis (Li et al., 2019), and PD. MSC-derived exosomes were discovered to rescue dopaminergic neurons in 6-OHDA mouse models of PD, providing a potential treatment for PD (Vilaca-Faria et al., 2019). MSC-derived exosomes can also carry beneficial miRNAs and interact with neuronal cells to reduce neuroinflammation and promote neurogenesis in PD animal models. miR-21 and miR-143 in MSC-derived exosomes are found to play a significant role in immune modulation and neuronal death. Moreover, as one of the miRNAs downregulated in PD, miR-133b in MSC-derived exosomes can be transmitted to neuronal cells to promote neurite outgrowth. In addition, by modifying MSC-derived exosomes with mimic-miR-7, it is possible to inhibit α-syn aggregation and suppress NLRP3 inflammasome activation in SNpc and striatum, thereby ameliorating the neuroinflammation response in PD. Modification with antago-miR-155 can also reduce microglia cell activation and neuroinflammation, and may be therapeutic for PD. Considering the above results, transfer of genetic materials such as miRNAs within MSC-derived exosomes, is indeed beneficial to PD animal models. Therefore, understanding how the miRNAs from MSC-derived exosomes interact with the cells and molecules in PD is of great importance.
Mounting evidence has been proved that exosomes separated from various types of cells can be modified to target specific neurons and specific regions of the brain and can be therapeutic for PD and many other neurodegenerative diseases (Tomlinson et al., 2015). Although the advantages of exosomes for therapy are apparent, some limitations exist. First, we cannot obtain pure exosomes using current technology, and so it is important for us to construct a minimum exosome delivery system to contain the therapeutic molecules and little else. Second, the adverse effects of using different sources of exosomes should be examined. Finally, we need to sort out the best cellular source for exosomes (Sarko and McKinney, 2017).
Not only do exosomes participate in the pathogenesis, diagnosis, and treatment of PD, exosomes can also be used as biomarker outcomes to show treatment response in PD clinical trials. In a single-center Exenatide-PD trial, 60 idiopathic PD patients were randomly assigned to subcutaneous administration of 2 mg exenatide (n = 31) or placebo (n = 29) once weekly for 48 weeks, followed by 12-week drug withdrawal. Blood samples were collected at week 0, 24, 48, and 60, and then neuronal-derived EVs with L1CAM were selectively isolated for insulin signaling proteins quantification. Athauda et al. (2019) found that patients receiving exenatide had enhanced brain insulin signaling with increased phosphorylation of insulin receptor substrate 1 (IRS-1) at 48 and 60 weeks, as compared with placebo controls. Moreover, the expression of downstream pathway substrates was increased, such as total phosphoinositide 3-kinase-protein kinase B (Akt) and phosphorylated mechanistic target of rapamycin (mTOR). In addition, they found that changes in levels of EV biomarkers including IRS-1 p-S616, t-mTOR, and p-mTOR S2448 were correlated with changes in Movement Disorders Society Unified Parkinson’s Disease Rating Scale (MDS-UPDRS) Part 3 scores at 48 weeks. At 60 weeks, the changes in MDS-UPDRS Part 3 scores were closely associated with changes in t-mTOR. These findings suggest that exenatide can improve the MDS-UPDRS Part 3 scores, and scores were significantly related to changes in these biomarkers in EVs (Athauda et al., 2019).
This research showed that changes in EV biomarkers were significantly associated with clinical improvements in an Exenatide-PD trial, and that EV biomarkers could potentially be used to evaluate treatment response and prognosis. Furthermore, neuronal-derived EVs could be a novel tool to assess target engagement for drugs in clinical trials in PD and other CNS diseases.
Our in-depth analysis of exosomes reveals that these subcellular components participate in the onset, propagation and progression of PD, by spreading the harmful molecules, such as misfolded α-syn and inflammatory mediators. Isolation and identification of EV cargoes have been used to discover novel biomarkers for the diagnosis of PD. In addition, their ability to cross the BBB and the low immunogenic activity make exosomes ideal drug-delivery systems for the treatment of PD. Recent research suggests that neuronal-derived EVs isolated from peripheral blood can be used as biomarkers to assess prognosis and elucidate drug targets in clinical trials.
However, further investigations to elaborate the molecular mechanisms of exosomes in PD pathophysiology are warranted. What’s the direct mechanism for sorting α-syn into exosomes? In view of low levels of α-syn in exosomes, how many exosomes are required to propagate and cause pathology in in vivo or in vitro PD models? Are exosomes involved in the core pathogenesis of PD onset and progression, or are they released only as a result of PD pathophysiology? Most importantly, more accurate and standardized purification methods should be developed to isolate purer exosomes for diagnostic, therapeutic, and prognostic purposes. In 2011, the International Society of EVs was launched to tackle these challenges. We foretell that future research will shed light on these intriguing questions.
JF conceived, designed, and revised this review. HY was responsible for the original manuscript writing. JA and LW searched and collected the related references. FL, ZB, and YC drew the figures. TS completed the tables in this review.
This review was supported by the National Natural Science Foundation of China (No. 81771271).
The authors declare that the research was conducted in the absence of any commercial or financial relationships that could be construed as a potential conflict of interest.
Abrami, L., Brandi, L., Moayeri, M., Brown, M. J., Krantz, B. A., Leppla, S. H., et al. (2013). Hijacking multivesicular bodies enables long-term and exosome-mediated long-distance action of anthrax toxin. Cell Rep. 5, 986–996. doi: 10.1016/j.celrep.2013.10.019
Alvarez-Erviti, L., Couch, Y., Richardson, J., Cooper, J., and Wood, M. (2011a). Alpha-synuclein release by neurons activates the inflammatory response in a microglial cell line. Neurosci. Res. 69, 337–342. doi: 10.1016/j.neures.2010.12.020
Alvarez-Erviti, L., Seow, Y., Schapira, A., Gardiner, C., Sargent, I., Wood, M., et al. (2011b). Lysosomal dysfunction increases exosome-mediated alpha-synuclein release and transmission. Neurobiol. Dis. 42, 360–367. doi: 10.1016/j.nbd.2011.01.029
Alvarez-Erviti, L., Rodriguez-Oroz, M. C., Cooper, J. M., Caballero, C., Ferrer, I., Obeso, J. A., et al. (2010). Chaperone-mediated autophagy markers in Parkinson disease brains. Arch. Neurol. 67, 1464–1472. doi: 10.1001/archneurol.2010.198
Aryani, A., and Denecke, B. (2016). Exosomes as a nanodelivery system: a key to the future of neuromedicine? Mol. Neurobiol. 53, 818–834. doi: 10.1007/s12035-014-9054-5
Ascherio, A., and Schwarzschild, M. A. (2016). The epidemiology of Parkinson’s disease: risk factors and prevention. Lancet Neurol. 15, 1257–1272. doi: 10.1016/s1474-4422(16)30230-7
Athauda, D., Gulyani, S., Karnati, H. K., Li, Y., Tweedie, D., Mustapic, M., et al. (2019). Utility of neuronal-derived exosomes to examine molecular mechanisms that affect motor function in patients with Parkinson disease: a secondary analysis of the exenatide-PD trial. JAMA Neurol. 76, 420–429. doi: 10.1001/jamaneurol.2018.4304
Baietti, M. F., Zhang, Z., Mortier, E., Melchior, A., Degeest, G., Geeraerts, A., et al. (2012). Syndecan-syntenin-ALIX regulates the biogenesis of exosomes. Nat. Cell Biol. 14, 677–685. doi: 10.1038/ncb2502
Ben Gedalya, T., Loeb, V., Israeli, E., Altschuler, Y., Selkoe, D. J., and Sharon, R. (2009). Alpha-synuclein and polyunsaturated fatty acids promote clathrin-mediated endocytosis and synaptic vesicle recycling. Traffic 10, 218–234. doi: 10.1111/j.1600-0854.2008.00853.x
Betarbet, R., Sherer, T. B., MacKenzie, G., Garcia-Osuna, M., Panov, A. V., and Greenamyre, J. T. (2000). Chronic systemic pesticide exposure reproduces features of Parkinson’s disease. Nat. Neurosci. 3, 1301–1306. doi: 10.1038/81834
Bliederhaeuser, C., Grozdanov, V., Speidel, A., Zondler, L., Ruf, W., Bayer, H., et al. (2016). Age-dependent defects of alpha-synuclein oligomer uptake in microglia and monocytes. Acta Neuropathol. 131, 379–391. doi: 10.1007/s00401-015-1504-2
Bose, A., and Beal, M. (2016). Mitochondrial dysfunction in Parkinson’s disease. J. Neurochem. 139, 216–231. doi: 10.1111/jnc.13731
Boya, P., Reggiori, F., and Codogno, P. (2013). Emerging regulation and functions of autophagy. Nat. Cell Biol. 15, 713–720. doi: 10.1038/ncb2788
Braak, H., Del Tredici, K., Rüb, U., De Vos, R., Jansen Steur, E., and Braak, E. (2003a). Staging of brain pathology related to sporadic Parkinson’s disease. Neurobiol. Aging 24, 197–211. doi: 10.1016/s0197-4580(02)00065-9
Braak, H., Rüb, U., Gai, W., and Del Tredici, K. (2003b). Idiopathic Parkinson’s disease: possible routes by which vulnerable neuronal types may be subject to neuroinvasion by an unknown pathogen. J. Neural Transm. 110, 517–536. doi: 10.1007/s00702-002-0808-2
Cabin, D. E., Shimazu, K., Murphy, D., Cole, N. B., Gottschalk, W., McIlwain, K. L., et al. (2002). Synaptic vesicle depletion correlates with attenuated synaptic responses to prolonged repetitive stimulation in mice lacking alpha-synuclein. J. Neurosci. 22, 8797–8807. doi: 10.1523/jneurosci.22-20-08797.2002
Cao, X., Lu, J., Zhao, Z., Li, M., Lu, T., An, X., et al. (2017). MicroRNA biomarkers of Parkinson’s disease in serum exosome-like microvesicles. Neurosci. Lett. 644, 94–99. doi: 10.1016/j.neulet.2017.02.045
Cao, Z., Wu, Y., Liu, G., Jiang, Y., Wang, X., Wang, Z., et al. (2019). α-Synuclein in salivary extracellular vesicles as a potential biomarker of Parkinson’s disease. Neurosci. Lett. 696, 114–120. doi: 10.1016/j.neulet.2018.12.030
Chairoungdua, A., Smith, D. L., Pochard, P., Hull, M., and Caplan, M. J. (2010). Exosome release of β-catenin: a novel mechanism that antagonizes Wnt signaling. J. Cell Biol. 190, 1079–1091. doi: 10.1083/jcb.201002049
Chang, C., Lang, H., Geng, N., Wang, J., Li, N., and Wang, X. (2013). Exosomes of BV-2 cells induced by alpha-synuclein: important mediator of neurodegeneration in PD. Neurosci. Lett. 548, 190–195. doi: 10.1016/j.neulet.2013.06.009
Chaudhuri, K., Healy, D., and Schapira, A. (2006). Non-motor symptoms of Parkinson’s disease: diagnosis and management. Lancet Neurol. 5, 235–245. doi: 10.1016/S1474-4422(06)70373-8
Chen, Y., Gao, C., Sun, Q., Pan, H., Huang, P., Ding, J., et al. (2017). MicroRNA-4639 is a regulator of DJ-1 expression and a potential early diagnostic marker for Parkinson’s disease. Front. Aging Neurosci. 9:232. doi: 10.3389/fnagi.2017.00232
Colombo, M., Moita, C., van Niel, G., Kowal, J., Vigneron, J., Benaroch, P., et al. (2013). Analysis of ESCRT functions in exosome biogenesis, composition and secretion highlights the heterogeneity of extracellular vesicles. J. Cell Sci. 126(Pt 24), 5553–5565. doi: 10.1242/jcs.128868
Colombo, M., Raposo, G., and Théry, C. (2014). Biogenesis, secretion, and intercellular interactions of exosomes and other extracellular vesicles. Annu. Rev. Cell Dev. Biol. 30, 255–289. doi: 10.1146/annurev-cellbio-101512-122326
Cooper, J., Wiklander, P., Nordin, J., Al-Shawi, R., Wood, M., Vithlani, M., et al. (2014). Systemic exosomal siRNA delivery reduced alpha-synuclein aggregates in brains of transgenic mice. Mov. Disord. 29, 1476–1485. doi: 10.1002/mds.25978
Danzer, K., Kranich, L., Ruf, W., Cagsal-Getkin, O., Winslow, A., Zhu, L., et al. (2012). Exosomal cell-to-cell transmission of alpha synuclein oligomers. Mol. Neurodegener. 7:42. doi: 10.1186/1750-1326-7-42
De Lau, L. M., and Breteler, M. M. (2006). Epidemiology of Parkinson’s disease. Lancet Neurol. 5, 525–535. doi: 10.1016/s1474-4422(06)70471-9
Del Tredici, K., and Braak, H. (2016). Review: Sporadic Parkinson’s disease: development and distribution of α-synuclein pathology. Neuropathol. Appl. Neurobiol. 42, 33–50. doi: 10.1111/nan.12298
Deng, H., Wang, P., and Jankovic, J. (2018). The genetics of Parkinson disease. Ageing Res. Rev. 42, 72–85. doi: 10.1016/j.arr.2017.12.007
Dickson, D. (2012). Parkinson’s disease and Parkinsonism: neuropathology. Cold Spring Harb. Perspect. Med. 2:a009258. doi: 10.1101/cshperspect.a009258
Elbaz, A., Clavel, J., Rathouz, P. J., Moisan, F., Galanaud, J. P., Delemotte, B., et al. (2009). Professional exposure to pesticides and Parkinson disease. Ann. Neurol. 66, 494–504. doi: 10.1002/ana.21717
Emmanouilidou, E., Melachroinou, K., Roumeliotis, T., Garbis, S., Ntzouni, M., Margaritis, L., et al. (2010). Cell-produced alpha-synuclein is secreted in a calcium-dependent manner by exosomes and impacts neuronal survival. J. Neurosci. 30, 6838–6851. doi: 10.1523/JNEUROSCI.5699-09.2010
Fader, C. M., Sánchez, D., Furlán, M., and Colombo, M. I. (2008). Induction of autophagy promotes fusion of multivesicular bodies with autophagic vacuoles in k562 cells. Traffic 9, 230–250. doi: 10.1111/j.1600-0854.2007.00677.x
Fader, C. M., Sánchez, D. G., Mestre, M. B., and Colombo, M. I. (2009). TI-VAMP/VAMP7 and VAMP3/cellubrevin: two v-SNARE proteins involved in specific steps of the autophagy/multivesicular body pathways. Biochim. Biophys. Acta 1793, 1901–1916. doi: 10.1016/j.bbamcr.2009.09.011
Fraser, K., Moehle, M., Alcalay, R., and West, A. (2016a). Urinary LRRK2 phosphorylation predicts parkinsonian phenotypes in G2019S LRRK2 carriers. Neurology 86, 994–999. doi: 10.1212/WNL.0000000000002436
Fraser, K., Rawlins, A. B., Clark, R., Alcalay, R., Standaert, D., Liu, N., et al. (2016b). Ser(P)-1292 LRRK2 in urinary exosomes is elevated in idiopathic Parkinson’s disease. Mov. Disord. 31, 1543–1550. doi: 10.1002/mds.26686
Friand, V., David, G., and Zimmermann, P. (2015). Syntenin and syndecan in the biogenesis of exosomes. Biol. Cell 107, 331–341. doi: 10.1111/boc.201500010
Frühbeis, C., Fröhlich, D., Kuo, W. P., Amphornrat, J., Thilemann, S., Saab, A. S., et al. (2013). Neurotransmitter-triggered transfer of exosomes mediates oligodendrocyte-neuron communication. PLoS Biol. 11:e1001604. doi: 10.1371/journal.pbio.1001604
Gao, H., and Hong, J. (2008). Why neurodegenerative diseases are progressive: uncontrolled inflammation drives disease progression. Trends Immunol. 29, 357–365. doi: 10.1016/j.it.2008.05.002
Géminard, C., De Gassart, A., Blanc, L., and Vidal, M. (2004). Degradation of AP2 during reticulocyte maturation enhances binding of hsc70 and Alix to a common site on TFR for sorting into exosomes. Traffic 5, 181–193. doi: 10.1111/j.1600-0854.2004.0167.x
Goedert, M., Spillantini, M. G., Del Tredici, K., and Braak, H. (2013). 100 years of Lewy pathology. Nat. Rev. Neurol. 9, 13–24. doi: 10.1038/nrneurol.2012.242
Grey, M., Dunning, C., Gaspar, R., Grey, C., Brundin, P., Sparr, E., et al. (2015). Acceleration of α-synuclein aggregation by exosomes. J. Biol. Chem. 290, 2969–2982. doi: 10.1074/jbc.M114.585703
Gross, J. C., Chaudhary, V., Bartscherer, K., and Boutros, M. (2012). Active Wnt proteins are secreted on exosomes. Nat. Cell Biol. 14, 1036–1045. doi: 10.1038/ncb2574
Gui, Y., Liu, H., Zhang, L., Lv, W., and Hu, X. (2015). Altered microRNA profiles in cerebrospinal fluid exosome in Parkinson disease and Alzheimer disease. Oncotarget 6, 37043–37053. doi: 10.18632/oncotarget.6158
Haney, M. J., Klyachko, N. L., Zhao, Y., Gupta, R., Plotnikova, E. G., He, Z., et al. (2015). Exosomes as drug delivery vehicles for Parkinson’s disease therapy. J. Control. Release 207, 18–30. doi: 10.1016/j.jconrel.2015.03.033
Hanson, P. I., and Cashikar, A. (2012). Multivesicular body morphogenesis. Annu. Rev. Cell Dev. Biol. 28, 337–362. doi: 10.1146/annurev-cellbio-092910-154152
Harding, C., Heuser, J., and Stahl, P. (1983). Receptor-mediated endocytosis of transferrin and recycling of the transferrin receptor in rat reticulocytes. J. Cell Biol. 97, 329–339. doi: 10.1083/jcb.97.2.329
Harischandra, D. S., Ghaisas, S., Rokad, D., Zamanian, M., Jin, H., Anantharam, V., et al. (2018). Environmental neurotoxicant manganese regulates exosome-mediated extracellular miRNAs in cell culture model of Parkinson’s disease: relevance to α-synuclein misfolding in metal neurotoxicity. Neurotoxicology 64, 267–277. doi: 10.1016/j.neuro.2017.04.007
Henne, W. M., Stenmark, H., and Emr, S. D. (2013). Molecular mechanisms of the membrane sculpting ESCRT pathway. Cold Spring Harb. Perspect. Biol. 5:a016766. doi: 10.1101/cshperspect.a016766
Hernán, M. A., Zhang, S. M., Rueda-deCastro, A. M., Colditz, G. A., Speizer, F. E., and Ascherio, A. (2001). Cigarette smoking and the incidence of Parkinson’s disease in two prospective studies. Ann. Neurol. 50, 780–786. doi: 10.1002/ana.10028
Hirsch, E., and Hunot, S. (2009). Neuroinflammation in Parkinson’s disease: a target for neuroprotection? Lancet Neurol. 8, 382–397. doi: 10.1016/S1474-4422(09)70062-6
Ho, D., Yi, S., Seo, H., Son, I., and Seol, W. (2014). Increased DJ-1 in urine exosome of Korean males with Parkinson’s disease. Biomed Res. Int. 2014:704678. doi: 10.1155/2014/704678
Hoshino, D., Kirkbride, K. C., Costello, K., Clark, E. S., Sinha, S., Grega-Larson, N., et al. (2013). Exosome secretion is enhanced by invadopodia and drives invasive behavior. Cell Rep. 5, 1159–1168. doi: 10.1016/j.celrep.2013.10.050
Hsu, C., Morohashi, Y., Yoshimura, S., Manrique-Hoyos, N., Jung, S., Lauterbach, M. A., et al. (2010). Regulation of exosome secretion by Rab35 and its GTPase-activating proteins TBC1D10A-C. J. Cell Biol. 189, 223–232. doi: 10.1083/jcb.200911018
Izco, M., Blesa, J., Schleef, M., Schmeer, M., Porcari, R., Al-Shawi, R., et al. (2019). Systemic exosomal delivery of shRNA minicircles prevents Parkinsonian pathology. Mol. Ther. 27, 30371–30375. doi: 10.1016/j.ymthe.2019.08.010
Jiang, R., Rong, C., Ke, R., Meng, S., Yan, X., Ke, H., et al. (2019). Differential proteomic analysis of serum exosomes reveals alterations in progression of Parkinson disease. Medicine 98:e17478. doi: 10.1097/md.0000000000017478
Jiang, Y., Liu, J., Chen, L., Jin, Y., Zhang, G., Lin, Z., et al. (2019). Serum secreted miR-137-containing exosomes affects oxidative stress of neurons by regulating OXR1 in Parkinson’s disease. Brain Res. 1722:146331. doi: 10.1016/j.brainres.2019.146331
Johnstone, R. M. (2005). Revisiting the road to the discovery of exosomes. Blood Cells Mol. Dis. 34, 214–219. doi: 10.1016/j.bcmd.2005.03.002
Johnstone, R. M., Adam, M., Hammond, J. R., Orr, L., and Turbide, C. (1987). Vesicle formation during reticulocyte maturation, Association of plasma membrane activities with released vesicles (exosomes). J. Biol. Chem. 262, 9412–9420.
Kalia, L. V., and Lang, A. E. (2015). Parkinson’s disease. Lancet 386, 896–912. doi: 10.1016/s0140-6736(14)61393-3
Kanemoto, S., Nitani, R., Murakami, T., Kaneko, M., Asada, R., Matsuhisa, K., et al. (2016). Multivesicular body formation enhancement and exosome release during endoplasmic reticulum stress. Biochem. Biophys. Res. Commun. 480, 166–172. doi: 10.1016/j.bbrc.2016.10.019
Katzmann, D. J., Babst, M., and Emr, S. D. (2001). Ubiquitin-dependent sorting into the multivesicular body pathway requires the function of a conserved endosomal protein sorting complex, ESCRT-I. Cell 106, 145–155. doi: 10.1016/s0092-8674(01)00434-2
Khoo, T., Yarnall, A., Duncan, G., Coleman, S., O’Brien, J., Brooks, D., et al. (2013). The spectrum of nonmotor symptoms in early Parkinson disease. Neurology 80, 276–281. doi: 10.1212/WNL.0b013e31827deb74
King, H. W., Michael, M. Z., and Gleadle, J. M. (2012). Hypoxic enhancement of exosome release by breast cancer cells. BMC Cancer 12:421. doi: 10.1186/1471-2407-12-421
Kitamura, Y., Kojima, M., Kurosawa, T., Sasaki, R., Ichihara, S., Hiraku, Y., et al. (2018). Proteomic profiling of exosomal proteins for blood-based biomarkers in Parkinson’s disease. Neuroscience 392, 121–128. doi: 10.1016/j.neuroscience.2018.09.017
Klumperman, J., and Raposo, G. (2014). The complex ultrastructure of the endolysosomal system. Cold Spring Harb. Perspect. Biol. 6:a016857. doi: 10.1101/cshperspect.a016857
Kojima, R., Bojar, D., Rizzi, G., Hamri, G. C.-E., El-Baba, M. D., Saxena, P., et al. (2018). Designer exosomes produced by implanted cells intracerebrally deliver therapeutic cargo for Parkinson’s disease treatment. Nat. Commun. 9:1305. doi: 10.1038/s41467-018-03733-8
Kordower, J., Chu, Y., Hauser, R., Freeman, T., and Olanow, C. (2008). Lewy body-like pathology in long-term embryonic nigral transplants in Parkinson’s disease. Nat. Med. 14, 504–506. doi: 10.1038/nm1747
Lai, C., and Breakefield, X. (2012). Role of exosomes/microvesicles in the nervous system and use in emerging therapies. Front. Physiol. 3:228. doi: 10.3389/fphys.2012.00228
Langston, J. W., Ballard, P., Tetrud, J. W., and Irwin, I. (1983). Chronic Parkinsonism in humans due to a product of meperidine-analog synthesis. Science 219, 979–980. doi: 10.1126/science.6823561
Laulagnier, K., Grand, D., Dujardin, A., Hamdi, S., Vincent-Schneider, H., Lankar, D., et al. (2004). PLD2 is enriched on exosomes and its activity is correlated to the release of exosomes. FEBS Lett. 572, 11–14. doi: 10.1016/j.febslet.2004.06.082
Lee, H., Cho, E., Lee, K., Kim, J., Cho, S., and Lee, S. (2013). Autophagic failure promotes the exocytosis and intercellular transfer of α-synuclein. Exp. Mol. Med. 45:e22. doi: 10.1038/emm.2013.45
Lee, H., Patel, S., and Lee, S. (2005). Intravesicular localization and exocytosis of alpha-synuclein and its aggregates. J. Neurosci. 25, 6016–6024. doi: 10.1523/JNEUROSCI.0692-05.2005
Lee, H., Suk, J., Patrick, C., Bae, E., Cho, J., Rho, S., et al. (2010). Direct transfer of alpha-synuclein from neuron to astroglia causes inflammatory responses in synucleinopathies. J. Biol. Chem. 285, 9262–9272. doi: 10.1074/jbc.M109.081125
Lehmann, B. D., Paine, M. S., Brooks, A. M., McCubrey, J. A., Renegar, R. H., Wang, R., et al. (2008). Senescence-associated exosome release from human prostate cancer cells. Cancer Res. 68, 7864–7871. doi: 10.1158/0008-5472.Can-07-6538
Li, D., Li, Y.-P., Li, Y.-X., Zhu, X.-H., Du, X.-G., Zhou, M., et al. (2018). Effect of regulatory network of exosomes and microRNAs on neurodegenerative diseases. Chin. Med. J. 131, 2216–2225. doi: 10.4103/0366-6999.240817
Li, J., Englund, E., Holton, J., Soulet, D., Hagell, P., Lees, A., et al. (2008). Lewy bodies in grafted neurons in subjects with Parkinson’s disease suggest host-to-graft disease propagation. Nat. Med. 14, 501–503. doi: 10.1038/nm1746
Li, Z., Liu, F., He, X., Yang, X., Shan, F., and Feng, J. (2019). Exosomes derived from mesenchymal stem cells attenuate inflammation and demyelination of the central nervous system in EAE rats by regulating the polarization of microglia. Int. Immunopharmacol. 67, 268–280. doi: 10.1016/j.intimp.2018.12.001
McKinnon, C., and Tabrizi, S. J. (2014). The ubiquitin-proteasome system in neurodegeneration. Antioxid. Redox Signal. 21, 2302–2321. doi: 10.1089/ars.2013.5802
McMillan, K. J., Murray, T. K., Bengoa-Vergniory, N., Cordero-Llana, O., Cooper, J., Buckley, A., et al. (2017). Loss of microRNA-7 regulation leads to α-synuclein accumulation and dopaminergic neuronal loss in vivo. Mol. Ther. 25, 2404–2414. doi: 10.1016/j.ymthe.2017.08.017
Mianehsaz, E., Mirzaei, H. R., Mahjoubin-Tehran, M., Rezaee, A., Sahebnasagh, R., Pourhanifeh, M. H., et al. (2019). Mesenchymal stem cell-derived exosomes: a new therapeutic approach to osteoarthritis? Stem Cell Res. Ther. 10:340. doi: 10.1186/s13287-019-1445-0
Mirzaei, H., Sahebkar, A., Jaafari, M. R., Goodarzi, M., and Mirzaei, H. R. (2017). Diagnostic and therapeutic potential of exosomes in cancer: The beginning of a new tale? J. Cell. Physiol. 232, 3251–3260. doi: 10.1002/jcp.25739
Müller, T. (2012). Drug therapy in patients with Parkinson’s disease. Transl. Neurodegener. 1:10. doi: 10.1186/2047-9158-1-10
Nazarenko, I., Rana, S., Baumann, A., McAlear, J., Hellwig, A., Trendelenburg, M., et al. (2010). Cell surface tetraspanin Tspan8 contributes to molecular pathways of exosome-induced endothelial cell activation. Cancer Res. 70, 1668–1678. doi: 10.1158/0008-5472.Can-09-2470
Nichols, W. C., Pankratz, N., Marek, D. K., Pauciulo, M. W., Elsaesser, V. E., Halter, C. A., et al. (2009). Mutations in GBA are associated with familial Parkinson disease susceptibility and age at onset. Neurology 72, 310–316. doi: 10.1212/01.wnl.0000327823.81237.d1
Olanow, C., and Brundin, P. (2013). Parkinson’s disease and alpha synuclein: is Parkinson’s disease a prion-like disorder? Mov. Disord. 28, 31–40. doi: 10.1002/mds.25373
Ostrowski, M., Carmo, N. B., Krumeich, S., Fanget, I., Raposo, G., Savina, A., et al. (2010). Rab27a and Rab27b control different steps of the exosome secretion pathway. Nat. Cell Biol. 12, 19–30. doi: 10.1038/ncb2000
Palacios, N., Gao, X., McCullough, M. L., Schwarzschild, M. A., Shah, R., Gapstur, S., et al. (2012). Caffeine and risk of Parkinson’s disease in a large cohort of men and women. Mov. Disord. 27, 1276–1282. doi: 10.1002/mds.25076
Pan, B. T., Teng, K., Wu, C., Adam, M., and Johnstone, R. M. (1985). Electron microscopic evidence for externalization of the transferrin receptor in vesicular form in sheep reticulocytes. J. Cell Biol. 101, 942–948. doi: 10.1083/jcb.101.3.942
Panaro, M., and Cianciulli, A. (2012). Current opinions and perspectives on the role of immune system in the pathogenesis of Parkinson’s disease. Curr. Pharm. Des. 18, 200–208. doi: 10.2174/138161212799040574
Pardridge, W. (2012). Drug transport across the blood-brain barrier. J. Cereb. Blood Flow Metab. 32, 1959–1972. doi: 10.1038/jcbfm.2012.126
Perez-Hernandez, D., Gutiérrez-Vázquez, C., Jorge, I., López-Martín, S., Ursa, A., Sánchez-Madrid, F., et al. (2013). The intracellular interactome of tetraspanin-enriched microdomains reveals their function as sorting machineries toward exosomes. J. Biol. Chem. 288, 11649–11661. doi: 10.1074/jbc.M112.445304
Poehler, A., Xiang, W., Spitzer, P., May, V., Meixner, H., Rockenstein, E., et al. (2014). Autophagy modulates SNCA/α-synuclein release, thereby generating a hostile microenvironment. Autophagy 10, 2171–2192. doi: 10.4161/auto.36436
Postuma, R., Berg, D., Stern, M., Poewe, W., Olanow, C., Oertel, W., et al. (2015). MDS clinical diagnostic criteria for Parkinson’s disease. Mov. Disord. 30, 1591–1601. doi: 10.1002/mds.26424
Pourhanifeh, M. H., Mahjoubin-Tehran, M., Shafiee, A., Hajighadimi, S., Moradizarmehri, S., Mirzaei, H., et al. (2019). MicroRNAs and exosomes: Small molecules with big actions in multiple myeloma pathogenesis. IUBMB Life. doi: 10.1002/iub.2211 [Epub ahead of print].
Proux-Gillardeaux, V., Raposo, G., Irinopoulou, T., and Galli, T. (2007). Expression of the Longin domain of TI-VAMP impairs lysosomal secretion and epithelial cell migration. Biol. Cell 99, 261–271. doi: 10.1042/bc20060097
Puri, N., and Roche, P. A. (2008). Mast cells possess distinct secretory granule subsets whose exocytosis is regulated by different SNARE isoforms. Proc. Natl. Acad. Sci. U.S.A. 105, 2580–2585. doi: 10.1073/pnas.0707854105
Qu, M., Lin, Q., Huang, L., Fu, Y., Wang, L., He, S., et al. (2018). Dopamine-loaded blood exosomes targeted to brain for better treatment of Parkinson’s disease. J. Control. Release 287, 156–166. doi: 10.1016/j.jconrel.2018.08.035
Rani, K., Mukherjee, R., Singh, E., Kumar, S., Sharma, V., Vishwakarma, P., et al. (2019). Neuronal exosomes in saliva of Parkinson’s disease patients: a pilot study. Parkinsonism Relat. Disord. 67, 21–23. doi: 10.1016/j.parkreldis.2019.09.008
Recasens, A., Dehay, B., Bové, J., Carballo-Carbajal, I., Dovero, S., Pérez-Villalba, A., et al. (2014). Lewy body extracts from Parkinson disease brains trigger α-synuclein pathology and neurodegeneration in mice and monkeys. Ann. Neurol. 75, 351–362. doi: 10.1002/ana.24066
Ross, G. W., Abbott, R. D., Petrovitch, H., Morens, D. M., Grandinetti, A., Tung, K. H., et al. (2000). Association of coffee and caffeine intake with the risk of Parkinson disease. JAMA 283, 2674–2679. doi: 10.1001/jama.283.20.2674
Saeedi Borujeni, M. J., Esfandiary, E., Taheripak, G., Codoñer-Franch, P., Alonso-Iglesias, E., and Mirzaei, H. (2018). Molecular aspects of diabetes mellitus: resistin, microRNA, and exosome. J. Cell. Biochem. 119, 1257–1272. doi: 10.1002/jcb.26271
Sahu, R., Kaushik, S., Clement, C. C., Cannizzo, E. S., Scharf, B., Follenzi, A., et al. (2011). Microautophagy of cytosolic proteins by late endosomes. Dev. Cell 20, 131–139. doi: 10.1016/j.devcel.2010.12.003
Sarko, D., and McKinney, C. (2017). Exosomes: origins and therapeutic potential for neurodegenerative disease. Front. Neurosci. 11:82. doi: 10.3389/fnins.2017.00082
Savina, A., Vidal, M., and Colombo, M. I. (2002). The exosome pathway in K562 cells is regulated by Rab11. J. Cell Sci. 115(Pt 12), 2505–2515.
Schapira, A. H. V., Chaudhuri, K. R., and Jenner, P. (2017). Non-motor features of Parkinson disease. Nat. Rev. Neurosci. 18, 435–450. doi: 10.1038/nrn.2017.62
Shi, M., Kovac, A., Korff, A., Cook, T. J., Ginghina, C., Bullock, K. M., et al. (2016). CNS tau efflux via exosomes is likely increased in Parkinson’s disease but not in Alzheimer’s disease. Alzheimers Dement. 12, 1125–1131. doi: 10.1016/j.jalz.2016.04.003
Shi, M., Liu, C., Cook, T. J., Bullock, K. M., Zhao, Y., Ginghina, C., et al. (2014). Plasma exosomal α-synuclein is likely CNS-derived and increased in Parkinson’s disease. Acta Neuropathol. 128, 639–650. doi: 10.1007/s00401-014-1314-y
Sinha, S., Hoshino, D., Hong, N. H., Kirkbride, K. C., Grega-Larson, N. E., Seiki, M., et al. (2016). Cortactin promotes exosome secretion by controlling branched actin dynamics. J. Cell Biol. 214, 197–213. doi: 10.1083/jcb.201601025
Spillantini, M. G., Crowther, R. A., Jakes, R., Hasegawa, M., and Goedert, M. (1998). alpha-Synuclein in filamentous inclusions of Lewy bodies from Parkinson’s disease and dementia with lewy bodies. Proc. Natl. Acad. Sci. U.S.A. 95, 6469–6473. doi: 10.1073/pnas.95.11.6469
Stenmark, H. (2009). Rab GTPases as coordinators of vesicle traffic. Nat. Rev. Mol. Cell Biol. 10, 513–525. doi: 10.1038/nrm2728
Strauss, K., Goebel, C., Runz, H., Möbius, W., Weiss, S., Feussner, I., et al. (2010). Exosome secretion ameliorates lysosomal storage of cholesterol in Niemann-Pick type C disease. J. Biol. Chem. 285, 26279–26288. doi: 10.1074/jbc.M110.134775
Stuendl, A., Kunadt, M., Kruse, N., Bartels, C., Moebius, W., Danzer, K., et al. (2016). Induction of α-synuclein aggregate formation by CSF exosomes from patients with Parkinson’s disease and dementia with Lewy bodies. Brain 139, 481–494. doi: 10.1093/brain/awv346
Stuffers, S., Sem Wegner, C., Stenmark, H., and Brech, A. (2009). Multivesicular endosome biogenesis in the absence of ESCRTs. Traffic 10, 925–937. doi: 10.1111/j.1600-0854.2009.00920.x
Tamai, K., Tanaka, N., Nakano, T., Kakazu, E., Kondo, Y., Inoue, J., et al. (2010). Exosome secretion of dendritic cells is regulated by Hrs, an ESCRT-0 protein. Biochem. Biophys. Res. Commun. 399, 384–390. doi: 10.1016/j.bbrc.2010.07.083
Tanji, K., Mori, F., Kakita, A., Takahashi, H., and Wakabayashi, K. (2011). Alteration of autophagosomal proteins (LC3, GABARAP and GATE-16) in Lewy body disease. Neurobiol. Dis. 43, 690–697. doi: 10.1016/j.nbd.2011.05.022
Tanner, C. M., Kamel, F., Ross, G. W., Hoppin, J. A., Goldman, S. M., Korell, M., et al. (2011). Rotenone, paraquat, and Parkinson’s disease. Environ. Health Perspect. 119, 866–872. doi: 10.1289/ehp.1002839
Théry, C., Amigorena, S., Raposo, G., and Clayton, A. (2006). Isolation and characterization of exosomes from cell culture supernatants and biological fluids. Curr. Protoc. Cell Biol. 30, 3.22.1–3.22.29. doi: 10.1002/0471143030.cb0322s30
Tomlinson, P., Zheng, Y., Fischer, R., Heidasch, R., Gardiner, C., Evetts, S., et al. (2015). Identification of distinct circulating exosomes in Parkinson’s disease. Ann. Clin. Transl. Neurol. 2, 353–361. doi: 10.1002/acn3.175
Trajkovic, K., Hsu, C., Chiantia, S., Rajendran, L., Wenzel, D., Wieland, F., et al. (2008). Ceramide triggers budding of exosome vesicles into multivesicular endosomes. Science 319, 1244–1247. doi: 10.1126/science.1153124
Van Niel, G., Charrin, S., Simoes, S., Romao, M., Rochin, L., Saftig, P., et al. (2011). The tetraspanin CD63 regulates ESCRT-independent and -dependent endosomal sorting during melanogenesis. Dev. Cell 21, 708–721. doi: 10.1016/j.devcel.2011.08.019
Vella, L., Hill, A., and Cheng, L. (2016). Focus on extracellular vesicles: exosomes and their role in protein trafficking and biomarker potential in Alzheimer’s and Parkinson’s disease. Int. J. Mol. Sci. 17:173. doi: 10.3390/ijms17020173
Vilaca-Faria, H., Salgado, A. J., and Teixeira, F. G. (2019). Mesenchymal stem cells-derived exosomes: a new possible therapeutic strategy for Parkinson’s disease? Cells 8:E118. doi: 10.3390/cells8020118
Villarroya-Beltri, C., Baixauli, F., Gutiérrez-Vázquez, C., Sánchez-Madrid, F., and Mittelbrunn, M. (2014). Sorting it out: regulation of exosome loading. Semin. Cancer Biol. 28, 3–13. doi: 10.1016/j.semcancer.2014.04.009
Wang, S., Liu, Z., Ye, T., Mabrouk, O. S., Maltbie, T., Aasly, J., et al. (2017). Elevated LRRK2 autophosphorylation in brain-derived and peripheral exosomes in LRRK2 mutation carriers. Acta Neuropathol. Commun. 5, 86. doi: 10.1186/s40478-017-0492-y
Winkler, C. W., Taylor, K. G., and Peterson, K. E. (2014). Location is everything: let-7b microRNA and TLR7 signaling results in a painful TRP. Sci. Signal. 7:e14. doi: 10.1126/scisignal.2005407
Wolf, P. (1967). The nature and significance of platelet products in human plasma. Br. J. Haematol. 13, 269–288. doi: 10.1111/j.1365-2141.1967.tb08741.x
Yao, Y. F., Qu, M. W., Li, G. C., Zhang, F. B., and Rui, H. C. (2018). Circulating exosomal miRNAs as diagnostic biomarkers in Parkinson’s disease. Eur. Rev. Med. Pharmacol. Sci. 22, 5278–5283. doi: 10.26355/eurrev_201808_15727
Zhao, Z. H., Chen, Z. T., Zhou, R. L., Zhang, X., Ye, Q. Y., and Wang, Y. Z. (2018). Increased DJ-1 and alpha-synuclein in plasma neural-derived exosomes as potential markers for Parkinson’s disease. Front. Aging Neurosci. 10:438. doi: 10.3389/fnagi.2018.00438
Zhuang, X., Xiang, X., Grizzle, W., Sun, D., Zhang, S., Axtell, R., et al. (2011). Treatment of brain inflammatory diseases by delivering exosome encapsulated anti-inflammatory drugs from the nasal region to the brain. Mol. Ther. 19, 1769–1779. doi: 10.1038/mt.2011.164
Keywords: exosomes, Parkinson’s disease, neurodegeneration, neuroinflammation, pathogenesis, diagnosis, treatment, prognosis
Citation: Yu H, Sun T, An J, Wen L, Liu F, Bu Z, Cui Y and Feng J (2020) Potential Roles of Exosomes in Parkinson’s Disease: From Pathogenesis, Diagnosis, and Treatment to Prognosis. Front. Cell Dev. Biol. 8:86. doi: 10.3389/fcell.2020.00086
Received: 31 October 2019; Accepted: 30 January 2020;
Published: 21 February 2020.
Edited by:
Daniel Ungar, University of York, United KingdomReviewed by:
Hamed Mirzaei, Kashan University of Medical Sciences, IranCopyright © 2020 Yu, Sun, An, Wen, Liu, Bu, Cui and Feng. This is an open-access article distributed under the terms of the Creative Commons Attribution License (CC BY). The use, distribution or reproduction in other forums is permitted, provided the original author(s) and the copyright owner(s) are credited and that the original publication in this journal is cited, in accordance with accepted academic practice. No use, distribution or reproduction is permitted which does not comply with these terms.
*Correspondence: Juan Feng, anVhbmZlbmdAY211LmVkdS5jbg==; ZmVuZ2p1YW5kckAxNjMuY29t
Disclaimer: All claims expressed in this article are solely those of the authors and do not necessarily represent those of their affiliated organizations, or those of the publisher, the editors and the reviewers. Any product that may be evaluated in this article or claim that may be made by its manufacturer is not guaranteed or endorsed by the publisher.
Research integrity at Frontiers
Learn more about the work of our research integrity team to safeguard the quality of each article we publish.