- 1Department of Chemical Engineering, Faculty of Engineering, University of Waterloo, Waterloo, ON, Canada
- 2Waterloo Institute for Nanotechnology, University of Waterloo, Waterloo, ON, Canada
- 3Centre for Biotechnology and Bioengineering, University of Waterloo, Waterloo, ON, Canada
Pluripotent stem cells (PSCs) have great potential to revolutionize the fields of tissue engineering and regenerative medicine as well as stem cell therapeutics. However, the end goal of using PSCs for therapeutic use remains distant due to limitations in current PSC production. Conventional methods for PSC expansion have limited potential to be scaled up to produce the number of cells required for the end-goal of therapeutic use due to xenogenic components, high cost or low efficiency. In this mini review, we explore novel methods and emerging technologies of improving PSC expansion: the use of the two-dimensional mechanobiological strategies of topography and stiffness and the use of three-dimensional (3D) expansion methods including encapsulation, microcarrier-based culture, and suspension culture. Additionally, we discuss the limitations of conventional PSC expansion methods as well as the challenges in implementing non-conventional methods.
Introduction
Pluripotent stem cells (PSCs), including embryonic and induced pluripotent stem cells (ESCs and iPSCs, respectively), are unique for their unlimited self-renewal and ability to differentiate into any cell of the three germ layers. These potentials could revolutionize the fields of disease modeling and regenerative medicine. Conventional PSC expansion methods, including feeder layers and the addition of growth factors to feeder-free culture, have been shown to maintain the undifferentiated state of PSCs efficiently. However, using feeder layers to expand human PSCs (hPSCs) is limited by concerns of transmission of animal pathogens and immunogens for clinical applications (Villa-Diaz et al., 2013) and are laborious to work with, having to culture two types of cells. Additionally, both methods can be irreproducible due to the poorly defined xenogenic culture conditions. Although xeno-free and defined media for hPSC expansion (Chen G. et al., 2011; Baghbaderani et al., 2016; Yasuda et al., 2018) are available, such media are expensive to scale-up for clinical use (Chen et al., 2014). Thus, much research has gone into novel methods that can improve hPSC expansion such as using mechanobiological principles, including surface topography, stiffness and surface modification. Mechanobiological principles have shown promises in reducing or replacing the need for biochemical growth factors in PSC culture (Ireland and Simmons, 2015; Argentati et al., 2019). For example, the transforming growth factor-beta (TGF-β) pathway, which is essential to maintaining hPSC pluripotency (James et al., 2005), can be activated by mechanotransduction, eliminating the need for supplementing TGF-β (Eyckmans et al., 2011; Rys et al., 2016). Use of the synthetic PSC niche is motivated by their low cost and high availability (Brafman et al., 2010; Fan et al., 2015). This review will focus on two types of emerging methods for improving PSC expansion: (1) two-dimensional (2D) methods that employ mechanobiological principles (e.g. topography and stiffness) and (2) three-dimensional (3D) methods of expansion including use of encapsulation, microcarriers, and suspension. Figure 1 summarizes both conventional and emerging strategies for enhancing PSC expansion.
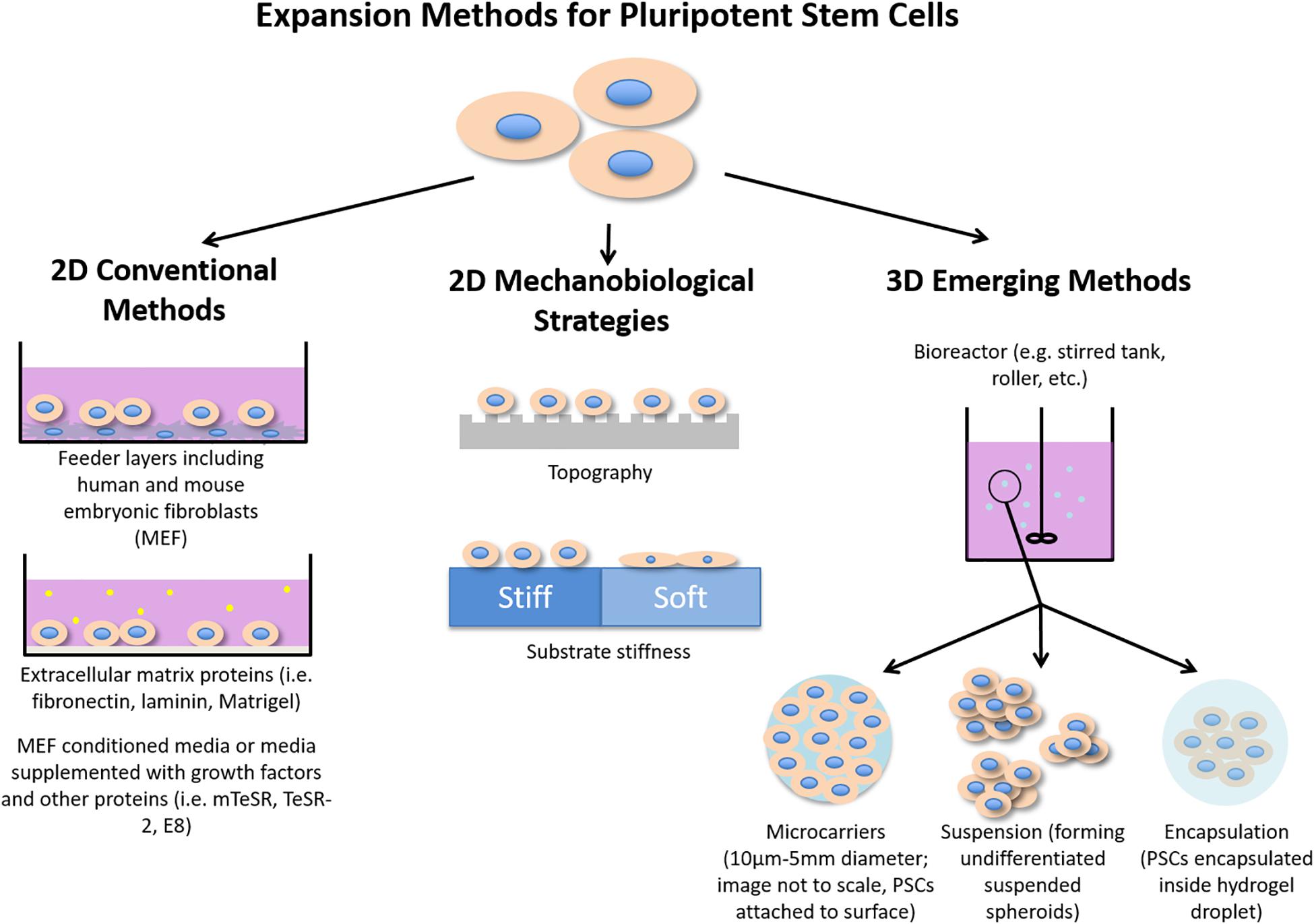
Figure 1. Illustration summarizing conventional methods of PSC expansion as well as mechanobiological strategies and emerging 3D methods for enhancing PSC expansion.
As the field is not yet mature, the majority of studies have used mouse models as groundwork for human PSC studies. It is noteworthy, however, that results are not necessarily consistent between the two species due to differences in pathways associated with maintenance and the state of pluripotency of the cell. Mouse ESCs (mESCs) are in the naïve state of pluripotency, in which there has been no lineage specification (Ying et al., 2008); while hPSCs are in the primed state of pluripotency after isolation from the blastocyte (Huang et al., 2012), though generation of naïve hPSCs has been recently achieved (Zimmerlin et al., 2016; Yang et al., 2017; Lipsitz et al., 2018) with much of the knowledge gained from studying mESCs. Although the overall goal for improvement of PSC expansion, we will discuss mPSC studies in addition to hPSC studies to highlight the importance of mechanobiology in regulating PSC fate as most work in mechanobiology relating to PSC expansion has been done in mouse PSCs (mPSCs). Due to the differences in pluripotency states, the numerous differences in patterns of pluripotency-associated gene expression, morphology, culture requirements, differentiation behavior and molecular profiles will determine different expansion methods for mPSCs and hPSCs (Nichols and Smith, 2009; Davidson et al., 2015). We suggest an excellent review by Davidson et al. (2015) for a comprehensive understanding of the differences and significances of mouse and human pluripotency.
Conventional Methods of PSC Expansion
PSCs are commonly cultured using feeder layers or feeder-free systems (Table 1) that require the use of a biological matrix supplemented with chemical growth factors. Feeder layers consist of cells that create and maintain the stem cell niche required for expanding and maintaining the pluripotency of PSCs (Johnson et al., 2008). Feeder cells provide the biochemical factors required by PSCs for self-renewal and proliferation, along with biophysical cues, including topography and stiffness (López-Fagundo et al., 2016). We suggest a comprehensive review on feeder layers by Llames et al. (2015).
The other conventional methods of culturing PSCs involve using ECM components with cell culture media supplemented with growth factors that regulate genes related to pluripotency (Srinivasan et al., 2016) – either to up-regulate promoters of pluripotency or down-regulate inhibitors of pluripotency. The growth factors used depend on the pathways to be regulated, which depend on the cell type. For example, mPSC culture depends on growth factors such as leukemia inhibitory factor (LIF) (Smith et al., 1988; Williams et al., 1988) to maintain pluripotency, while hPSC maintenance depends on fibroblast growth factor 2 (FGF2) (Dvorak et al., 2006) and Activin A (Beattie et al., 2005). Despite containing animal-derived products (bovine serum albumin), the most commonly used feeder-free media for hPSC expansion is mTeSR media and is typically used with the animal-derived Matrigel coating.
Xeno-free and chemically defined systems have been developed for PSC expansion. However, their high cost limits its use in large-scale production of PSCs (Chen G. et al., 2011). The most basic xeno-free medium for hPSC expansion is Essential 8 (E8). These media are used with a vitronectin coated culture vessel to make the expansion system completely defined and xeno-free. However, E8’s use in hPSC expansion is limited due to inconsistencies and slower growth rates (Hey et al., 2018). Therefore, it is worth exploring the use of physical and mechanical cues in PSC maintenance and expansion, which could improve the large-scale xeno-free expansion. We recommend a book chapter (Srinivasan et al., 2016) for a comprehensive review of conventional hPSC expansion, a review by Dakhore et al. (2018) that compares hPSC expansion media, and a review by Hayashi and Furue (2016) that summarizes substrates used in hPSC expansion.
Non-Conventional Methods of PSC Expansion
Due to the limitations of conventional methods PSC expansion, new expansion methods that improve PSC expansion are needed to make progress toward therapeutic use of PSCs. Additionally, these non-conventional methods aim to improve efficiency, reproducibility and cost. For clinical use, current Good Manufacturing Practice (GMP) is an important aspect to consider. However, most mechanobiological studies of PSC expansion have not covered this area yet. We recommend the recent review by Bedford et al. (2018) for a comprehensive review of cGMP for cell therapy and the review by De Sousa et al. (2016), which reviews cGMP in hPSC expansion specifically.
Two-Dimensional (2D) Non-conventional Methods
2D methods focus on surface and materials properties of the expansion substrate, which can scale-out expansion in 2D and be implemented into a 3D culture for scaling up expansion. The 2D mechanobiological strategies that have been studied include growth factor immobilization (Alberti et al., 2008; Sohi et al., 2018) and micropatterning with proteins (Mosiewicz et al., 2013; Hammad et al., 2016) or other ECM molecules (Meade et al., 2013), surface chemistry (Saha et al., 2011; Kimura et al., 2018), and nanomaterials including graphene (Chen G.Y. et al., 2012) and carbon nanotubes (Akasaka et al., 2011; Pryzhkova et al., 2014). However, we will only discuss topographical cues and stiffness in this review.
Topographical Cues for PSC Expansion
Topography plays a key role in determining PSC fate (Ankam et al., 2013, 2015, 2018; Chan et al., 2013), including maintaining pluripotency and regulating self-renewal and proliferation. Table 2 lists examples of studies of micro and nanotopographies on PSC maintenance and expansion. Studies of hPSCs suggest that smaller topographical features promote their undifferentiated state (Bae et al., 2014; Reimer et al., 2016; Ko et al., 2017). Using a TopoChip with over 1000 patterns made of tissue culture polystyrene (T), small feature size with high feature density were found to promote hPSC pluripotency best (Reimer et al., 2016). Comparatively, another study found that nano-pillars and nano-grooves of around 200 nm on polydimethylsiloxane (PDMS) promoted proliferation and maintenance of hiPSCs in feeder-free conditions (Ko et al., 2017). A study using nanopillars of 120–360 nm in diameter, found that pillars with diameters 120–170 nm retained the most pluripotency marker expression and had the least amount of colony spreading (Bae et al., 2014). Meanwhile, hESCs cultured on vitronectin-coated micro-patterns, binary colloidal crystals of 2 and 5 μm, resulted in improved maintenance of pluripotency (Wang et al., 2018), though this study did not involve nano-sized patterns. In contrast, Chen W. et al. (2012) compared hESC expansion on 150 nm rough glass surface to expansion on a smooth glass surface and found that Oct4 expression of hESCs was lower on the nanorough surface compared to the smooth surface.
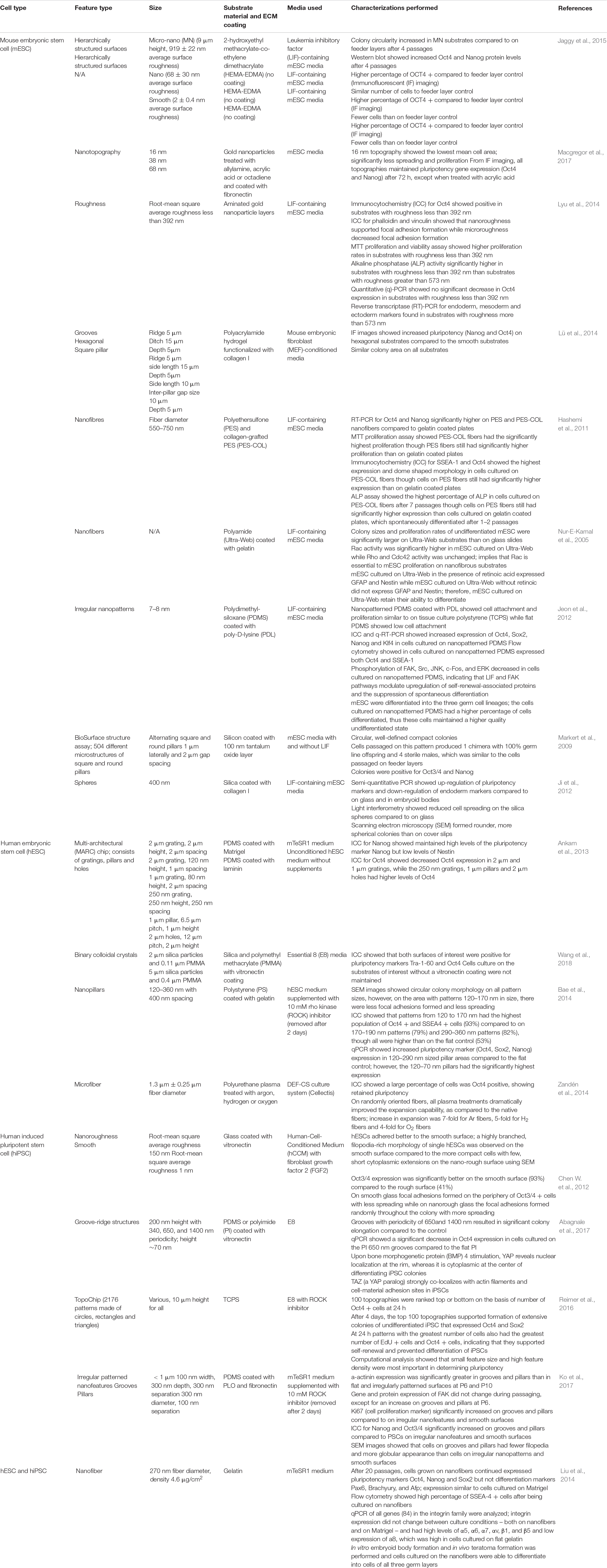
Table 2. Summary of examples of studies of human or mouse pluripotent stem cells (hPSCs and mPSCs, respectively) on topographical features and their results.
While topography undoubtedly contributes significantly to PSC maintenance, a common consensus on which topographies are the most important for PSC maintenance has not been reached. Studies have implied that surface topography alone cannot maintain pluripotency (Ankam et al., 2013; Abagnale et al., 2017; Wang et al., 2018). Interestingly, in studies of mPSCs and hPSCs, it was hypothesized that topography affects focal adhesion formation, which affects colony morphology and stem cell fate. Colonies with retained pluripotency and compact, circular morphology on patterned areas showed significantly fewer focal adhesions compared to the colonies that spread out and grew in irregular shapes on flat surfaces (Jeon et al., 2012; Ji et al., 2012; Bae et al., 2014; Ko et al., 2017; Macgregor et al., 2017). Surface topography may prevent focal adhesion formation, which reduces spreading and leads to the compact, circular colonies associated with preserved pluripotency (Hashemi et al., 2011; Jeon et al., 2012; Ji et al., 2012; Kong et al., 2013; Bae et al., 2014; Ko et al., 2017). It has also been hypothesized that topography affects ECM protein adsorption, affecting the cell adhesion, proliferation and morphology, thus synergistically maintaining PSCs (Zandén et al., 2014; Macgregor et al., 2017).
Stiffness of Substrate on PSC Expansion
Substrate stiffness plays a significant role in controlling cellular behavior and stem cell fate. Synthetic biomaterials, including hydrogels, are useful tools for studying the effects of stiffness. Hydrogels can be modified to have different stiffness, depending on the molecular weight or concentration of polymer, and the crosslinking density (Caliari and Burdick, 2016), which depends on crosslinker concentration and crosslinking time. Polyacrylamide (PA) hydrogels (Pelham and Wang, 1997; Mih et al., 2011) and polydimethylsiloxane (PDMS) (Evans et al., 2009; Sun et al., 2012), with large tunable ranges of stiffness are examples used for studying the impact of stiffness on PSCs.
Mouse ESCs were cultured on PDMS substrates with varying stiffness and showed increased cell spreading and proliferation with increasing substrate stiffness (0.041–2.7 MPa) along with increased differentiation (Evans et al., 2009). Similarly, mESCs cultured on a stiff poly-L-lysine/hyaluronic acid (PLL/HA) hydrogel film showed increased cell adhesion and proliferation, while weak cell adhesion, and round colonies retaining pluripotency were observed on the softer PLL/HA films (Blin et al., 2010). Soft PA substrates maintained mESC pluripotency better than stiff substrates including TCPS (Chowdhury et al., 2010a). When cultured on substrates similar to mESC intrinsic stiffness (0.5–0.6 kPa), mESCs had improved self-renewal and retention of pluripotency, thus proposing stiffness matching as a method for maintaining mESCs (Chowdhury et al., 2010b). Later, mESCs cultured on PA hydrogels of varying stiffness preserved their pluripotency regardless of the surface topography, with increased Oct4 and Nanog expression on all soft substrates compared to the stiff substrates; topography only influenced pluripotency on cells cultured on stiff substrates (Lü et al., 2014).
The effects of stiffness observed in mESCs are not translated to hPSCs. There is limited consensus on the effects of stiffness, with some groups finding little to no influence of stiffness on pluripotency (Keung et al., 2012; Maldonado et al., 2015; Przybyla et al., 2016; Price et al., 2017) and others finding the opposite (Musah et al., 2012; Sun et al., 2012; Kim et al., 2018; Sung et al., 2018). Nonetheless, observations made by several groups imply that stiffer substrates are more suitable for hPSC expansion. Using PDMS with effective moduli of 1.92 kPa (soft), 14.22 kPa (medium rigid), and 1218.4 kPa (rigid), hESC cytoskeleton contractility was found to increase with matrix rigidity along with maintained pluripotency (Sun et al., 2012). Consistent with these findings, hESCs and hiPSCs, cultured on PA hydrogels functionalized with a glucosaminoglycan binding peptide, attached better and formed more spread out and robust colonies on substrates coated with and without a Matrigel coating (Musah et al., 2012). The stiffest substrates (10 kPa) were found to support hESC expansion in five different hESC lines, with high levels of YAP/TAZ nuclear localization, an indicator of pluripotency. Later studies also found that YAP/TAZ nuclear localization decreases in soft substrates (Price et al., 2017; Lee et al., 2019). Although YAP/TAZ nuclear localization decreased in soft substrates, pluripotency marker expression remained similar between soft and stiff substrates with higher proliferation in stiff substrates (Price et al., 2017). Similarly, as substrate stiffness increased, cell proliferation increased, and substrate stiffness has an inverse relationship with spontaneous differentiation (Maldonado et al., 2015). Ligand density also affects how cells respond to their substrate stiffness; with the right number of functional groups, soft materials can support hPSC attachment proliferation and self-renewal similar to that of a stiff hydrogel (Lee et al., 2019). Despite the inconclusiveness of exactly how substrate stiffness affects hPSC behavior, especially as material choice also affects pluripotency and the absolute stiffness required for PSC culture, it is clear that stiffer substrates are more suitable for hPSC expansion in contrast with softer substrates for mPSC expansion.
Three-Dimensional (3D) Methods of PSC Expansion
Recently, 3D methods of stem cell culture have gained traction due to the need for scalable stem cell expansion to obtain therapeutically relevant number of cells. 3D cell culture methods offer the opportunity to significantly scale-up the expansion of hPSCs. The 3D methods of PSC culture are divided into three categories: (i) PSC encapsulation in hydrogels, (ii) microcarrier-based 3D PSC culture, and (iii) PSC suspension culture.
A growing body of literature suggests that 3D cell culture systems recapitulate the in vivo microenvironment of cells that could help to improve stem cells expansion. In a landmark study of PSC encapsulation, a defined and scalable 3D cell encapsulation system in a thermo-responsive hydrogel was developed for hPSC expansion and differentiation; it also enabled efficient retrieval of the cells from the hydrogels following expansion, without using the cell dissociation enzymes (Lei and Schaffer, 2013). The cells expanded ∼80-fold in 3D culture compared to ∼9-fold expansion in 2D over 15 days. Cumulatively, 3D cell culture led to 1072-fold expansion over 60 passages (Lei and Schaffer, 2013). PSCs are mechanosensitive as previously discussed; however, the role of biophysical signals and cell-matrix interactions in the context of 3D PSC expansion was not investigated. Scaffolds used for 3D expansion of PSCs should provide a balance between cell–cell contact and cell–matrix interactions (Li et al., 2012). An alginate-based hydrogel with tethered polypeptides comprising of a cell-binding sequence of E-cadherin for 3D PSC expansion has shown to improve the proliferation rate of PSCs without compromising pluripotency marker expression resulting in up to 23-fold higher expansion in HAV10 peptide conjugated gels (Banerjee et al., 2018). Matrix degradability and remodeling by encapsulated PSCs are other parameters that affect PSC fate. Encapsulated PSCs are known to remodel their environment during proliferation and differentiation (Khetan et al., 2013; Madl et al., 2017). However, the capacity of PSCs to remodel the environment and their effect on the pluripotency related markers are poorly understood. This should be studied in greater detail to design more informed and tailor-made 3D scaffolds for enhanced stem cell expansion.
Microcarrier-based systems are another method for PSC expansion, which combine 2D cell adhesion in microcarriers with a 3D configuration of the bioreactor system to expand the area available for PSC expansion. Microcarriers act as supporting substrates for adherent cell culture with a diameter varying from 10 μm up to 5 mm (Le and Hasegawa, 2019). The major benefit of using microcarriers is their capacity to provide large surface areas for cell growth while being compatible with adherent cell culture systems. Cells can form a confluent layer around the microporous microcarrier; while in macroporous microcarriers, they are entrapped inside the pores of the microcarriers (Badenes et al., 2014). Factors including the type of materials used to fabricate the microcarriers, the shape of the microcarrier, and the type of ECM coating used for cell adhesion influence the yield and pluripotency of the PSC culture in a microcarrier system (Chen A.K.L. et al., 2011). For instance, use of matrigel coating led to up to 18-fold higher PSC expansion compared to uncoated microcarriers (Chen A.K.L. et al., 2011). Recently, dissolvable microcarriers have been developed, which allow the retrieval of cells without using enzymatic dissociation (Badenes et al., 2014; Shekaran et al., 2016; Rodrigues et al., 2019).
By leveraging the self-aggregation property of PSCs, suspension-based cell culture systems are being developed to improve yield. Such systems promote cell-cell interactions while inhibiting the cell-matrix interactions. Usually, such systems consist of single cell culture in the presence of rho kinase (ROCK) inhibitor (Olmer et al., 2010; Abbasalizadeh et al., 2012), which supports long-term PSC survival in an undifferentiated state. PSCs grow in a monoclonal fashion and form suspended spheroids of varying sizes. Microfabrication technology has been used to further improve the homogeneity of PSC colonies (Hsiao and Palecek, 2012; Hookway et al., 2016). Optimization of bioreactor hydrodynamic conditions using combinations of static and stirred culture has enabled size-controlled aggregates of hPSCs (Abbasalizadeh et al., 2012). Traditionally, the yield of hPSCs is lower compared to mPSCs in suspension bioreactor cultures (Lipsitz et al., 2018). Recently, Lipsitz et al. found that the use of naïve hPSCs, as opposed to primed hPSCs, was a critical element for enabling high-yield expansion of PSCs (Over all 25-fold expansion; up to 5.7-fold higher compared to primed hPSCs) in suspension culture (Lipsitz et al., 2018). Despite tremendous progress in suspension-based cell culture, more research is needed in maintaining the homogeneity of cell aggregates in scalable suspension culture. Additionally, cells on the surface of the suspension aggregates experience uncontrolled shear stress, which could also lead to heterogeneous cell populations, as shear stress is known to affect stem cell fate (Toh and Voldman, 2011; Vining and Mooney, 2017).
Conclusion and Future Outlook
Conventional methods of PSC expansion have clear and significant limitations in expansion. For development of large scale, defined and xeno-free PSC expansion systems, research should look toward using approaches with mechanobiological principles and 3D strategies for enhancing cell pluripotency retention and proliferation to improve current xeno-free expansion systems. Despite great progress in these fields, studying each physical cue in isolation is difficult as they are interconnected. It is challenging to draw conclusions regarding the effects of topography and stiffness due to many variations in study parameters, while 3D culture systems have much to be optimized. Nonetheless, these non-conventional methods have shown to improve PSC yield in xeno-free systems and thus should continue to be studied. Additionally, studies suggest that mechanobiological cues used with current PSC culture methods can enhance current PSC culture methods. The knowledge obtained in organoid cultures, mechanobiology, new advances in microfabrication and stimulus-responsive materials could contribute to future development of non-conventional systems for scaling up PSC expansion and revolutionize the field of regenerative medicine.
Author Contributions
Literature search was conducted by SC and MR. Manuscript writing and editing were performed by SC, MR, and EY.
Funding
The work was generously supported by the Natural Sciences and Engineering Research Council of Canada (NSERC) Discovery (NSERC 2016040; to MR, SC, and EY) and the University of Waterloo start-up fund (to MR, SC, and EY). In addition, SC was supported by the Ontario Graduate Scholarship.
Conflict of Interest
The authors declare that the research was conducted in the absence of any commercial or financial relationships that could be construed as a potential conflict of interest.
References
Abagnale, G., Sechi, A., Steger, M., Zhou, Q., Kuo, C. C., Aydin, G., et al. (2017). Surface topography guides morphology and spatial patterning of induced pluripotent stem cell colonies. Stem Cell Rep. 9, 654–666. doi: 10.1016/j.stemcr.2017.06.016
Abbasalizadeh, S., Larijani, M. R., Samadian, A., and Baharvand, H. (2012). Bioprocess development for mass production of size-controlled human pluripotent stem cell aggregates in stirred suspension bioreactor. Tissue Eng. Part C Methods 18, 831–851. doi: 10.1089/ten.tec.2012.0161
Akasaka, T., Yokoyama, A., Matsuoka, M., Hashimoto, T., and Watari, F. (2011). Maintenance of hemiround colonies and undifferentiated state of mouse induced pluripotent stem cells on carbon nanotube-coated dishes. Carbon N. Y. 49, 2287–2299. doi: 10.1016/j.carbon.2011.01.061
Alberti, K., Davey, R. E., Onishi, K., George, S., Salchert, K., Seib, F. P., et al. (2008). Functional immobilization of signaling proteins enables control of stem cell fate. Nat. Methods 5, 645–650. doi: 10.1038/nmeth.1222
Amit, M., and Itskovitz-Eldor, J. (2006). Feeder-free culture of human embryonic stem cells. Methods Enzymol. 420, 37–49. doi: 10.1016/S0076-6879(06)20003-X
Amit, M., Margulets, V., Segev, H., Shariki, K., Laevsky, I., Coleman, R., et al. (2004). Human feeder layers for human embryonic stem cells. Biol. Reprod. 68, 2150–2156. doi: 10.1095/biolreprod.102.012583
Ankam, S., Lim, C. K., and Yim, E. K. F. (2015). Actomyosin contractility plays a role in MAP2 expression during nanotopography-directed neuronal differentiation of human embryonic stem cells. Biomaterials 47, 20–28. doi: 10.1016/j.biomaterials.2015.01.003
Ankam, S., Suryana, M., Chan, L. Y., Moe, A. A. K., Teo, B. K. K., and Law, J. B. K. (2013). Substrate topography and size determine the fate of human embryonic stem cells to neuronal or glial lineage. Acta Biomater. 9, 4535–4545. doi: 10.1016/j.actbio.2012.08.018
Ankam, S., Teo, B. K. K., Pohan, G., Ho, S. W. L., Lim, C. K., and Yim, E. K. F. (2018). Temporal changes in nucleus morphology, Lamin A/C and histone methylation during nanotopography-induced neuronal differentiation of stem cells. Front. Bioeng. Biotechnol. 6:69. doi: 10.3389/fbioe.2018.00069
Argentati, C., Morena, F., Tortorella, I., Bazzucchi, M., Porcellati, S., and Emiliani, C. (2019). Insight into mechanobiology: how stem cells feel mechanical forces and orchestrate biological functions. Int. J. Mol. Sci. 20:5337. doi: 10.3390/ijms20215337
Badenes, S. M., Fernandes, T. G., Rodrigues, C. A. V., Diogo, M. M., and Cabral Joaquim, M. S. (2014). “Scalable Expansion of Human-Induced Pluripotent Stem Cells in Xeno-Free Microcarriers,” in Stem Cells and Good Manufacturing Practices. Methods in Molecular Biology, Vol. 1283, ed. K. Turksen, (New York, NY: Humana Press).
Bae, D., Moon, S. H., Park, B. G., Park, S. J., Jung, T., Kim, J. S., et al. (2014). Nanotopographical control for maintaining undifferentiated human embryonic stem cell colonies in feeder free conditions. Biomaterials 35, 916–928. doi: 10.1016/j.biomaterials.2013.10.031
Baghbaderani, B. A., Tian, X., Cadet, J. S., Shah, K., Walde, A., Tran, H., et al. (2016). A newly defined and xeno-free culture medium supports every-other-day medium replacement in the generation and long-term cultivation of human pluripotent stem cells. PLoS One 11:e0161229. doi: 10.1371/journal.pone.0161229
Banerjee, I., Kumta, P., and Richardson, T. (2018). Peptide Conjugated Hydrogel Substrate for the Maintenance and Expansion of Human Pluripotent Stem Cells. U.S. Patent No US 2018/0171286 A1. Washington, DC: United States Patent and Trademark Office.
Bangalore, M. P., Adhikarla, S., Mukherjee, O., and Panicker, M. M. (2017). Genotoxic effects of culture media on human pluripotent stem cells. Sci. Rep. 7, 1–12. doi: 10.1038/srep42222
Beattie, G. M., Lopez, A. D., Bucay, N., Hinton, A., Firpo, M. T., King, C. C., et al. (2005). Activin a maintains pluripotency of human embryonic stem cells in the absence of feeder layers. Stem Cells 23, 489–495.
Bedford, P., Jy, J., Collins, L., and Keizer, S. (2018). Considering cell therapy product “Good Manufacturing Practice” status. Front. Med. 5:118. doi: 10.3389/fmed.2018.00118
Blin, G., Lablack, N., Louis-Tisserand, M., Nicolas, C., Picart, C., and Pucéat, M. (2010). Nano-scale control of cellular environment to drive embryonic stem cells selfrenewal and fate. Biomaterials 31, 1742–1750. doi: 10.1016/j.biomaterials.2009.11.055
Brafman, D. A., Chang, C. W., Fernandez, A., Willert, K., Varghese, S., and Chien, S. (2010). Long-term human pluripotent stem cell self-renewal on synthetic polymer surfaces. Biomaterials 31, 9135–9144. doi: 10.1016/j.biomaterials.2010.08.007
Caliari, S. R., and Burdick, J. A. (2016). A practical guide to hydrogels for cell culture. Nat. Methods 13, 405–414. doi: 10.1038/nmeth.3839
Chan, L. Y., Birch, W. R., Yim, E. K. F., and Choo, A. B. H. (2013). Temporal application of topography to increase the rate of neural differentiation from human pluripotent stem cells. Biomaterials 34, 382–392. doi: 10.1016/j.biomaterials.2012.09.033
Chen, A. K. L., Chen, X., Choo, A. B. H., Reuveny, S., and Oh, S. K. W. (2011). Critical microcarrier properties affecting the expansion of undifferentiated human embryonic stem cells. Stem Cell Res. 7, 97–111. doi: 10.1016/j.scr.2011.04.007
Chen, G., Gulbranson, D. R., Hou, Z., Bolin, J. M., Probasco, M. D., Smuga-otto, K., et al. (2011). Chemically defined conditions for human iPS cell derivation and culture. Nat. Methods 8, 424–429. doi: 10.1038/nmeth.1593
Chen, G. Y., Pang, D. W. P., Hwang, S. M., Tuan, H. Y., and Hu, Y. C. (2012). A graphene-based platform for induced pluripotent stem cells culture and differentiation. Biomaterials 33, 418–427. doi: 10.1016/j.biomaterials.2011.09.071
Chen, K. G., Mallon, B. S., McKay, R. D. G., and Robey, P. G. (2014). Human pluripotent stem cell culture: considerations for maintenance, expansion, and therapeutics. Cell Stem Cell 14, 13–26. doi: 10.1016/j.stem.2013.12.005
Chen, W., Villa-Diaz, L. G., Sun, Y., Weng, S., Kim, J. K., Lam, R. H. W., et al. (2012). Nanotopography influences adhesion, spreading, and self-renewal of human embryonic stem cells. ACS Nano 6, 4094–4103. doi: 10.1021/nn3004923
Chowdhury, F., Li, Y., Poh, Y. C., Yokohama-Tamaki, T., Wang, N., and Tanaka, T. S. (2010a). Soft substrates promote homogeneous self-renewal of embryonic stem cells via downregulating cell-matrix tractions. PLoS One 5:e15655. doi: 10.1371/journal.pone.0015655
Chowdhury, F., Na, S., Li, D., Poh, Y., Tanaka, T. S., Wang, F., et al. (2010b). Cell material property dictates stress-induced spreading and differentiation in embryonic stem cells. Nat. Mater. 9, 82–88. doi: 10.1038/nmat2563.Cell
Conner, D. A. (2000). Mouse embryo fibroblast (MEF) feeder cell preparation. Curr. Protoc. Mol. Biol. 51, 23.2.1–23.2.7. doi: 10.1002/0471142727.mb2302s51
Dakhore, S., Nayer, B., and Hasegawa, K. (2018). Human pluripotent stem cell culture: current status, challenges, and advancement. Stem Cells Int. 2018:7396905. doi: 10.1155/2018/7396905
Davidson, K. C., Mason, E. A., and Pera, M. F. (2015). The pluripotent state in mouse and human. Development 142, 3090–3099. doi: 10.1242/dev.116061
De Sousa, P. A., Downie, J. M., Tye, B. J., Bruce, K., Dand, P., Dhanjal, S., et al. (2016). Development and production of good manufacturing practice grade human embryonic stem cell lines as source material for clinical application. Stem Cell Res. 17, 379–390. doi: 10.1016/j.scr.2016.08.011
Dvorak, P., Dvorakova, D., and Hampl, A. (2006). Fibroblast growth factor signaling in embryonic and cancer stem cells. FEBS Lett. 580, 2869–2874. doi: 10.1016/j.febslet.2006.01.095
Evans, N., Minelli, C., Gentleman, E., LaPointe, V., Patankar, S., Kallivretaki, M., et al. (2009). Substrate stiffness affects early differentiation events in embryonic stem cells. Eur. Cells Mater. 18, 1–14. doi: 10.22203/ecm.v018a01
Eyckmans, J., Boudou, T., Yu, X., and Chen, C. S. (2011). A Hitchhiker’s guide to mechanobiology. Dev. Cell 21, 35–47.
Fan, Y., Wu, J., Ashok, P., Hsiung, M., and Tzanakakis, E. S. (2015). Production of human pluripotent stem cell therapeutics under defined xeno-free conditions: progress and challenges. Stem Cell Rev. Rep. 11, 96–109. doi: 10.1007/s12015-014-9544-x
Hammad, M., Rao, W., Smith, J. G. W., Anderson, D. G., Langer, R., Young, L. E., et al. (2016). Identification of polymer surface adsorbed proteins implicated in pluripotent human embryonic stem cell expansion. Biomater. Sci. 4, 1381–1391. doi: 10.1039/c6bm00214e
Hashemi, S. M., Soudi, S., Shabani, I., Naderi, M., and Soleimani, M. (2011). The promotion of stemness and pluripotency following feeder-free culture of embryonic stem cells on collagen-grafted 3-dimensional nanofibrous scaffold. Biomaterials 32, 7363–7374. doi: 10.1016/j.biomaterials.2011.06.048
Hayashi, Y., and Furue, M. K. (2016). Biological effects of culture substrates on human pluripotent stem cells. Stem Cells Int. 2016:5380560. doi: 10.1155/2016/5380560
Hey, C. A. B., Saltõkova, K. B., Bisgaard, H. C., and Møller, L. B. (2018). Comparison of two different culture conditions for derivation of early hiPSC. Cell Biol. Int. 42, 1467–1473. doi: 10.1002/cbin.10966
Hookway, T. A., Butts, J. C., Lee, E., Tang, H., and McDevitt, T. C. (2016). Aggregate formation and suspension culture of human pluripotent stem cells and differentiated progeny. Methods 101, 11–20. doi: 10.1016/j.ymeth.2015.11.027
Hovatta, O., Mikkola, M., Gertow, K., Strömberg, A. M., Inzunza, J., Hreinsson, J., et al. (2003). A culture system using human foreskin fibroblasts as feeder cells allows production of human embryonic stem cells. Hum. Reprod. 18, 1404–1409. doi: 10.1093/humrep/deg290
Hsiao, C., and Palecek, S. P. (2012). Microwell regulation of pluripotent stem cell self-renewal and differentiation. Bionanoscience 2, 266–276.
Huang, Y., Osorno, R., Tsakiridis, A., and Wilson, V. (2012). In Vivo differentiation potential of epiblast stem cells revealed by chimeric embryo formation. Cell Rep. 2, 1571–1578. doi: 10.1016/j.celrep.2012.10.022
Ireland, R. G., and Simmons, C. A. (2015). Human pluripotent stem cell mechanobiology: Manipulating the biophysical microenvironment for regenerative medicine and tissue engineering applications. Stem Cells 33, 3187–3196. doi: 10.1002/stem.2105
Jaggy, M., Zhang, P., Greiner, A. M., Autenrieth, T. J., Nedashkivska, V., Efremov, A. N., et al. (2015). Hierarchical micro-nano surface topography promotes long-term maintenance of undifferentiated mouse embryonic stem cells. Nano Lett. 15, 7146–7154. doi: 10.1021/acs.nanolett.5b03359
James, D., Levine, A. J., Besser, D., and Hemmati-Brivanlou, A. (2005). TGFβ/activin/nodal signaling is necessary for the maintenance of pluripotency in human embryonic stem cells. Development 132, 1273–1282. doi: 10.1242/dev.01706
Jeon, K., Oh, H. J., Lim, H., Kim, J. H., Lee, D. H., Lee, E. R., et al. (2012). Self-renewal of embryonic stem cells through culture on nanopattern polydimethylsiloxane substrate. Biomaterials 33, 5206–5220. doi: 10.1016/j.biomaterials.2012.04.011
Ji, L., Lapointe, V. L. S., Evans, N. D., and Stevens, M. M. (2012). Changes in embryonic stem cell colony morphology and early differentiation markers driven by colloidal crystal topographical cues. Eur. Cells Mater. 23, 135–146. doi: 10.22203/eCM.v023a10
Johnson, B. V., Shindo, N., Rathjen, P. D., Rathjen, J., and Keough, R. A. (2008). Understanding pluripotency – How embryonic stem cells keep their options open. Mol. Hum. Reprod. 14, 513–520. doi: 10.1093/molehr/gan048
Kaufman, M. H., and Evans, M. J. (1981). Establishment in culture of pluripotential cells from mouse embryos. Nature 292, 154–156.
Keung, A. J., Asuri, P., Kumar, S., and Schaffer, D. V. (2012). Soft microenvironments promote the early neurogenic differentiation but not self-renewal of human pluripotent stem cells. Integr. Biol. 4, 1049–1058. doi: 10.1039/c2ib20083j
Khetan, S., Guvendiren, M., Legant, W. R., Cohen, D. M., Chen, C. S., and Burdick, J. A. (2013). Degradation-mediated cellular traction directs stem cell fate in covalently crosslinked three-dimensional hydrogels. Nat. Mater. 12, 458–465. doi: 10.1038/nmat3586
Kim, I. G., Gil, C. H., Seo, J., Park, S. J., Subbiah, R., Jung, T. H., et al. (2018). Mechanotransduction of human pluripotent stem cells cultivated on tunable cell-derived extracellular matrix. Biomaterials 150, 100–111. doi: 10.1016/j.biomaterials.2017.10.016
Kimura, Y., Kasai, K., and Miyata, S. (2018). Feeder-free culture for mouse induced pluripotent stem cells by using UV/ozone surface-modified substrates. Mater. Sci. Eng. C 92, 280–286. doi: 10.1016/j.msec.2018.06.053
Ko, J.-Y., Oh, H.-J., Lee, J., and Im, G.-I. (2017). Nanotopographic influence on the in vitro behavior of induced pluripotent stem cells. Tissue Eng. Part A 24, 595–606. doi: 10.1089/ten.tea.2017.0144
Kong, Y. P., Tu, C. H., Donovan, P. J., and Yee, A. F. (2013). Expression of Oct4 in human embryonic stem cells is dependent on nanotopographical configuration. Acta Biomater. 9, 6369–6380. doi: 10.1016/j.actbio.2013.01.036
Le, M. N. T., and Hasegawa, K. (2019). Expansion culture of human pluripotent stem cells and production of cardiomyocytes. Bioengineering 6:48. doi: 10.3390/bioengineering6020048
Lee, S., Stanton, A. E., Tong, X., and Yang, F. (2019). Hydrogels with enhanced protein conjugation efficiency reveal stiffness-induced YAP localization in stem cells depends on biochemical cues. Biomaterials 202, 26–34. doi: 10.1016/j.biomaterials.2019.02.021
Lei, Y., and Schaffer, D. V. (2013). A fully defined and scalable 3D culture system for human pluripotent stem cell expansion and differentiation. Proc. Natl. Acad. Sci. U.S.A. 10, E5039–E5048. doi: 10.1073/pnas.1309408110
Li, L., Bennett, S. A. L., and Wang, L. (2012). Role of E-cadherin and other cell adhesion molecules in survival and differentiation of human pluripotent stem cells. Cell Adhes. Migr. 6, 59–70. doi: 10.4161/cam.6.1.19583
Lipsitz, Y. Y., Woodford, C., Yin, T., Hanna, J. H., and Zandstra, P. W. (2018). Modulating cell state to enhance suspension expansion of human pluripotent stem cells. Proc. Natl. Acad. Sci. U.S.A. 115, 6369–6374. doi: 10.1073/pnas.1714099115
Liu, L., Yoshioka, M., Nakajima, M., Ogasawara, A., Liu, J., Hasegawa, K., et al. (2014). Nanofibrous gelatin substrates for long-term expansion of human pluripotent stem cells. Biomaterials 35, 6259–6267. doi: 10.1016/j.biomaterials.2014.04.024
Llames, S., García-Pérez, E., Meana, Á, Larcher, F., and del Río, M. (2015). Feeder layer cell actions and applications. Tissue Eng. Part B Rev. 21, 345–353. doi: 10.1089/ten.teb.2014.0547
López-Fagundo, C., Livi, L. L., Ramchal, T., Darling, E. M., and Hoffman-Kim, D. (2016). A biomimetic synthetic feeder layer supports the proliferation and self-renewal of mouse embryonic stem cells. Acta Biomater. 39, 55–64. doi: 10.1016/j.actbio.2016.04.047
Lü, D., Luo, C., Zhang, C., Li, Z., and Long, M. (2014). Differential regulation of morphology and stemness of mouse embryonic stem cells by substrate stiffness and topography. Biomaterials 35, 3945–3955. doi: 10.1016/j.biomaterials.2014.01.066
Lyu, Z., Wang, H., Wang, Y., Ding, K., Liu, H., Yuan, L., et al. (2014). Maintaining the pluripotency of mouse embryonic stem cells on gold nanoparticle layers with nanoscale but not microscale surface roughness. Nanoscale 6, 6959–6969. doi: 10.1039/c4nr01540a
Macgregor, M., Williams, R., Downes, J., Bachhuka, A., and Vasilev, K. (2017). The role of controlled surface topography and chemistry on mouse embryonic stem cell attachment, growth and self-renewal. Materials (Basel) 10:1081. doi: 10.3390/ma10091081
Madl, C. M., Lesavage, B. L., Dewi, R. E., Dinh, C. B., Stowers, R. S., Khariton, M., et al. (2017). Maintenance of neural progenitor cell stemness in 3D hydrogels requires matrix remodelling. Nat. Mater. 16, 1233–1242. doi: 10.1038/nmat5020
Maldonado, M., Wong, L. Y., Echeverria, C., Ico, G., Low, K., Fujimoto, T., et al. (2015). The effects of electrospun substrate-mediated cell colony morphology on the self-renewal of human induced pluripotent stem cells. Biomaterials 50, 10–19. doi: 10.1016/j.biomaterials.2015.01.037
Markert, L. D., Lovmand, J., Foss, M., Lauridsen, R. H., Lovmand, M., Füchtbauer, E.-M., et al. (2009). Identification of distinct topographical surface microstructures favoring either undifferentiated expansion or differentiation of murine embryonic stem cells. Stem Cells Dev. 18, 1331–1342. doi: 10.1089/scd.2009.0114
Meade, K. A., White, K. J., Pickford, C. E., Holley, R. J., Marson, A., Tillotson, D., et al. (2013). Immobilization of heparan sulfate on electrospun meshes to support embryonic stem cell culture and differentiation. J. Biol. Chem. 288, 5530–5538. doi: 10.1074/jbc.M112.423012
Mih, J. D., Sharif, A. S., Liu, F., Marinkovic, A., Symer, M. M., and Tschumperlin, D. J. (2011). A multiwell platform for studying stiffness-dependent cell biology. PLoS One 6:e19929. doi: 10.1371/journal.pone.0019929
Mosiewicz, K. A., Kolb, L., Van Der Vlies, A. J., Martino, M. M., Lienemann, P. S., Hubbell, J. A., et al. (2013). In situ cell manipulation through enzymatic hydrogel photopatterning. Nat. Mater. 12, 1072–1078. doi: 10.1038/nmat3766
Musah, S., Morin, S. A., Wrighton, P. J., Zwick, D. B., Jin, S., and Kiessling, L. L. (2012). Glycosaminoglycan-binding hydrogels enable mechanical control of human pluripotent stem cell self-renewal. ACS Nano 6, 10168–10177. doi: 10.1021/nn3039148
Nichols, J., and Smith, A. (2009). Naive and primed pluripotent states. Cell Stem Cell 4, 487–492. doi: 10.1016/j.stem.2009.05.015
Nur-E-Kamal, A., Ahmed, I., Kamal, J., Schindler, M., and Meiners, S. (2005). Three-dimensional nanofibrillar surfaces promote self-renewal in mouse embryonic stem cells. Stem Cells 24, 426–433.
Olmer, R., Haase, A., Merkert, S., Cui, W., Paleèek, J., Ran, C., et al. (2010). Long term expansion of undifferentiated human iPS and ES cells in suspension culture using a defined medium. Stem Cell Res. 5, 51–64. doi: 10.1016/j.scr.2010.03.005
Pelham, R. J., and Wang, Y. L. (1997). Cell locomotion and focal adhesions are regulated by substrate flexibility. Proc. Natl. Acad. Sci. U.S.A. 94, 13661–13665. doi: 10.1073/pnas.94.25.13661
Price, A. J., Huang, E. Y., Sebastiano, V., and Dunn, A. R. (2017). A semi-interpenetrating network of polyacrylamide and recombinant basement membrane allows pluripotent cell culture in a soft, ligand-rich microenvironment. Biomaterials 121, 179–192. doi: 10.1016/j.biomaterials.2016.12.005
Pryzhkova, M. V., Aria, I., Cheng, Q., Harris, G. M., Zan, X., Gharib, M., et al. (2014). Carbon nanotube-based substrates for modulation of human pluripotent stem cell fate. Biomaterials 35, 5098–5109. doi: 10.1016/j.biomaterials.2014.03.011
Przybyla, L., Lakins, J. N., and Weaver, V. M. (2016). Tissue mechanics orchestrate wnt-dependent human embryonic stem cell differentiation. Cell Stem Cell 19, 462–475. doi: 10.1016/j.stem.2016.06.018
Reimer, A., Vasilevich, A., Hulshof, F., Viswanathan, P., Van Blitterswijk, C. A., De Boer, J., et al. (2016). Scalable topographies to support proliferation and Oct4 expression by human induced pluripotent stem cells. Sci. Rep. 6:18948. doi: 10.1038/srep18948
Richards, M., Fong, C. Y., Chan, W. K., Wong, P. C., and Bongso, A. (2002). Human feeders support prolonged undifferentiated growth of human inner cell masses and embryonic stem cells. Nat. Biotechnol. 20, 933–936. doi: 10.1038/nbt726
Rodrigues, A. L., Rodrigues, C. A. V., Gomes, A. R., Vieira, S. F., Badenes, S. M., Diogo, M. M., et al. (2019). Dissolvable microcarriers allow scalable expansion and harvesting of human induced pluripotent stem cells under xeno-free conditions. Biotechnol. J. 14, 1–12. doi: 10.1002/biot.201800461
Rys, J. P., Monteiro, D. A., and Alliston, T. (2016). Mechanobiology of TGFβ signaling in the skeleton. Matrix Biol. 5, 413–425. doi: 10.1016/j.matbio.2016.02.002
Saha, K., Mei, Y., Reisterer, C. M., Pyzocha, N. K., Yang, J., Muffat, J., et al. (2011). Surface-engineered substrates for improved human pluripotent stem cell culture under fully defined conditions. Proc. Natl. Acad. Sci. U.S.A. 108, 18714–18719. doi: 10.1073/pnas.1114854108
Shekaran, A., Lam, A., Sim, E., Jialing, L., Jian, L., Wen, J. T. P., et al. (2016). Biodegradable ECM-coated PCL microcarriers support scalable human early MSC expansion and in vivo bone formation. Cytotherapy 18, 1332–1344. doi: 10.1016/j.jcyt.2016.06.016
Smith, A. G., Heath, J. K., Donaldson, D. D., Wong, G. G., Moreau, J., and Stahl, M. (1988). Inhibition of pluripotential embryonic stem cell differentiation by purified polypeptides. Nature 336, 688–690.
Sohi, A. N., Naderi-Manesh, H., Soleimani, M., Yasaghi, E. R., Manjili, H. K., Tavaddod, S., et al. (2018). Synergistic effect of co-immobilized FGF-2 and vitronectin-derived peptide on feeder-free expansion of induced pluripotent stem cells. Mater. Sci. Eng. C 93, 157–169. doi: 10.1016/j.msec.2018.07.072
Srinivasan, A., Toh, Y. C., Loh, X. J., and Toh, W. S. (2016). Substrates and surfaces for control of pluripotent stem cell fate and function. Adv. Surfaces Stem Cell Res. 343–380. doi: 10.1002/9781119242642.ch12
Sun, Y., Villa-Diaz, L. G., Lam, R. H. W., Chen, W., Krebsbach, P. H., and Fu, J. (2012). Mechanics regulates fate decisions of human embryonic stem cells. PLoS One 7:e37178. doi: 10.1371/journal.pone.0037178
Sung, T.-C., Li, H.-F., Higuchi, A., Ling, Q.-D., Yang, J.-S., Tseng, Y.-C., Pan, C. H. P., et al. (2018). Human pluripotent stem cell culture on polyvinyl alcohol-co-itaconic acid hydrogels with varying stiffness under xeno-free conditions. J. Vis. Exp. 132:e57314. doi: 10.3791/57314
Takahashi, K., Narita, M., Yokura, M., Ichisaka, T., and Yamanaka, S. (2009). Human induced pluripotent stem cells on autologous feeders. PLoS One 4:e8067. doi: 10.1371/journal.pone.0008067
Takahashi, K., Tanabe, K., Ohnuki, M., Narita, M., Ichisaka, T., Tomoda, K., et al. (2007). Induction of pluripotent stem cells from adult human fibroblasts by defined factors. Cell 131, 861–872. doi: 10.1016/j.cell.2007.11.019
Takahashi, K., and Yamanaka, S. (2006). Induction of pluripotent stem cells from mouse embryonic and adult fibroblast cultures by defined factors. Cell 126, 663–676. doi: 10.1016/j.cell.2006.07.024
Thomson, J. A., Itskovitz-Eldor, J., Shapiro, S. S., Waknitz, M. A. J., Swiergiel, J. J., and Marshall, V. S. (1998). Embryonic stem cell lines derived from human blastocysts. Science 282, 1145–1147. doi: 10.1126/science.282.5391.1145
Toh, Y. C., and Voldman, J. (2011). Fluid shear stress primes mouse embryonic stem cells for differentiation in a self-renewing environment via heparan sulfate proteoglycans transduction. FASEB J. 25, 1208–1217. doi: 10.1096/fj.10-168971
Tomishima, M. (2014). Conditioning pluripotent stem cell media with mouse embryonic fibroblasts (MEF-CM). StemBook 20:2. doi: 10.3824/stembook.1.68.1
Villa-Diaz, L. G., Ross, A. M., Lahann, J., and Krebsbach, P. H. (2013). The evolution of human pluripotent stem cell culture: from feeder cells to synthetic coatings. Stem Cells 31, 1–7. doi: 10.1002/stem.1260
Vining, K. H., and Mooney, D. J. (2017). Mechanical forces direct stem cell behaviour in development and regeneration. Nat. Rev. Mol. Cell Biol. 18, 728–742. doi: 10.1016/j.bone.2016.06.013
Wang, P. Y., Khan, S., Nguyen, T., Kingshott, P., and Wong, R. C. B. (2018). Topographical modulation of pluripotency and differentiation of human embryonic stem cells. IEEE Trans. Nanotechnol. 17, 381–384. doi: 10.1109/TNANO.2017.2763604
Williams, R. L., Hilton, D. J., Pease, S., Willson, T. A., Stewart, C. L., Gearing, D. P., et al. (1988). Myeloid leukaemia inhibitory factor maintains the developmental potential of embryonic stem cells. Nature 336, 684–687. doi: 10.1038/336684a0
Yang, H., Qiu, Y., Zeng, X., Ding, Y., Zeng, J., Lu, K., et al. (2016). Effect of a feeder layer composed of mouse embryonic and human foreskin fibroblasts on the proliferation of human embryonic stem cells. Exp. Ther. Med. 11, 2321–2328. doi: 10.3892/etm.2016.3204
Yang, Y., Liu, B., Xu, J., Wang, J., Wu, J., and Shi, C. (2017). Derivation of pluripotent stem cells with in vivo embryonic and extraembryonic potency. Cell 169, 243.e25–257.e25. doi: 10.1016/j.cell.2017.02.005
Yasuda, S. Y., Ikeda, T., Shahsavarani, H., Yoshida, N., Nayer, B., Hino, M., et al. (2018). Chemically defined and growth-factor-free culture system for the expansion and derivation of human pluripotent stem cells. Nat. Biomed. Eng. 2, 173–182. doi: 10.1038/s41551-018-0200-7
Ying, Q. L., Wray, J., Nichols, J., Batlle-Morera, L., Doble, B., Woodgett, J., et al. (2008). The ground state of embryonic stem cell self-renewal. Nature 453, 519–523. doi: 10.1038/nature06968
Yu, J., Smuga-Otto, K., Antosiewicz-Bourget, J., Frane, J. L., Thomson, J. A., Vodyanik, M. A., et al. (2007). Induced pluripotent stem cell lines derived from human somatic cells. Science 318, 1917–1920. doi: 10.1126/science.1151526
Zandén, C., Hellström Erkenstam, N., Padel, T., Wittgenstein, J., Liu, J., and Kuhn, H. G. (2014). Stem cell responses to plasma surface modified electrospun polyurethane scaffolds. Nanomedicine 10, e949–e958. doi: 10.1016/j.nano.2014.01.010
Keywords: pluripotent stem cell culture, mechanobiology, three-dimension (3D) culture methods, topography, stiffness, encapsulation, microcarriers, suspension
Citation: Chan SW, Rizwan M and Yim EKF (2020) Emerging Methods for Enhancing Pluripotent Stem Cell Expansion. Front. Cell Dev. Biol. 8:70. doi: 10.3389/fcell.2020.00070
Received: 31 October 2019; Accepted: 27 January 2020;
Published: 14 February 2020.
Edited by:
Selwin K. Wu, National University of Singapore, SingaporeReviewed by:
David Schaffer, University of California, Berkeley, United StatesYang Xiao, Columbia University, United States
Copyright © 2020 Chan, Rizwan and Yim. This is an open-access article distributed under the terms of the Creative Commons Attribution License (CC BY). The use, distribution or reproduction in other forums is permitted, provided the original author(s) and the copyright owner(s) are credited and that the original publication in this journal is cited, in accordance with accepted academic practice. No use, distribution or reproduction is permitted which does not comply with these terms.
*Correspondence: Evelyn K. F. Yim, ZXlpbUB1d2F0ZXJsb28uY2E=
†Present address: Muhammad Rizwan, Donnelly Center for Cellular & Biomolecular Research, University of Toronto, Toronto, ON, Canada