- 1Department of Environmental Health, Harvard T. H. Chan School of Public Health, Boston, MA, United States
- 2Department of Physics, Northeastern University, Boston, MA, United States
The healthy and mature epithelial layer is ordinarily quiescent, non-migratory, solid-like, and jammed. However, in a variety of circumstances the layer transitions to a phase that is dynamic, migratory, fluid-like and unjammed. This has been demonstrated in the developing embryo, the developing avian airway, the epithelial layer reconstituted in vitro from asthmatic donors, wounding, and exposure to mechanical stress. Here we examine the extent to which ionizing radiation might similarly provoke epithelial layer unjamming. We exposed primary human bronchial epithelial (HBE) cells maintained in air-liquid interface (ALI) to sub-therapeutic doses (1 Gy) of ionizing radiation (IR). We first assessed: (1) DNA damage by measuring p-H2AX, (2) the integrity of the epithelial layer by measuring transepithelial electrical resistance (TEER), and (3) the extent of epithelial cell differentiation by detecting markers of differentiated airway epithelial cells. As expected, IR exposure induced DNA damage but, surprisingly, disrupted neither normal differentiation nor the integrity of the epithelial cell layer. We then measured cell shape and cellular migration to determine the extent of the unjamming transition (UJT). IR caused cell shape elongation and increased cellular motility, both of which are hallmarks of the UJT as previously confirmed. To understand the mechanism of IR-induced UJT, we inhibited TGF-β receptor activity, and found that migratory responses were attenuated. Together, these observations show that IR can provoke epithelial layer unjamming in a TGF-β receptor-dependent manner.
Introduction
The healthy and mature epithelial layer is ordinarily quiescent, non-migratory, solid-like, and jammed. In this jammed layer, epithelial cells maintain cobblestone-like shapes and rarely rearrange with their neighbors (Bi et al., 2014; Park et al., 2015; Atia et al., 2018). However, in a variety of circumstances the layer transitions to a phase that is dynamic, migratory, fluid-like and unjammed. In the unjammed layer, epithelial cells become elongated in shape and rearrange cooperatively with their neighbors (Sadati et al., 2013; Fredberg, 2014; Park et al., 2015, 2016; Pegoraro et al., 2016; Atia et al., 2018). This phase transition from the jammed to the unjammed phase, called the unjamming transition (UJT), was discovered in a living system using well-differentiated human bronchial epithelial (HBE) cells exposed to mechanical compression that mimics the mechanical effect of bronchospasm (Park et al., 2015). Since this discovery of these dynamic and structural hallmarks of epithelial UJT (Park et al., 2015; Atia et al., 2018), work from our team and others now suggests that the UJT seems to be a normal and perhaps essential part of epithelial biology but, nonetheless, can become hijacked in disease. For example, in the healthy embryo in vivo the UJT is triggered during ventral furrow formation during gastrulation in Drosophila (Atia et al., 2018), during elongation of the vertebrate body axis in the embryonic zebrafish (Mongera et al., 2018), and during airway epithelial branching in the embryonic avian lung (Spurlin et al., 2019). The UJT is therefore observed across vastly diverse biological contexts, in normal development and disease, both in vitro and in vivo, but its mechanisms and stimuli provoking the UJT are unknown.
Radiation therapy is often used to kill or shrink tumors (Molina et al., 2008; Chaffer and Weinberg, 2011). However, a growing body of evidence indicates that radiation therapy sometimes results in increased cancer metastasis among surviving cells (Moncharmont et al., 2014; Vilalta et al., 2016). The mechanism underlying this paradoxical radiation-induced metastasis remains unidentified but may involve a fluidization of the healthy, non-cancerous stroma which normally restrains the tumor. Here, we hypothesize that this paradoxical result is due to the unwanted effect of radiation on epithelial unjamming and resulting cellular migration. To test this hypothesis, we used primary HBE cells as a well-established model of the jammed layer, which can undergo the UJT. To test if radiation can provoke the UJT, we exposed the jammed HBE cell-layer to ionizing radiation (IR).
Results
Ionizing Radiation Induces DNA Damage
Air-liquid interface (ALI) culture recapitulates the environment in which bronchial epithelial cells exist within the airway in vivo. Over 14 days in ALI, progenitor basal cells differentiate into a diverse population of epithelial cells as found in vivo (Fulcher et al., 2005). To assess DNA damage, we exposed cultures of primary HBE cells in ALI conditions to 1 Gy on ALI day 14. To determine the level of DNA damage, we performed immunofluorescent staining to detect p-H2AX, a marker for DNA-double strand breaks (DSBs) (Kuo and Yang, 2008). As previously reported in a different type of cells (Mariotti et al., 2013), we observed a maximal increase in p-H2AX at 1 h post-irradiation (data not shown). This maximal p-H2AX was reduced back to baseline by 6 h post-irradiation (data not shown). Compared to time-matched control cells, irradiated cells showed robust increases in the level of p-H2AX, indicating that exposure to IR indeed leads to DNA damage (Figure 1B). We observed positive p-H2AX in both apical and basolateral HBE cells as demonstrated by orthogonal side-view imaging (Figure 1C). We also observed increased p-H2AX protein by western blot (Figure 1D). Collectively, these data indicate that exposure of HBE cells to IR induces DNA damage.
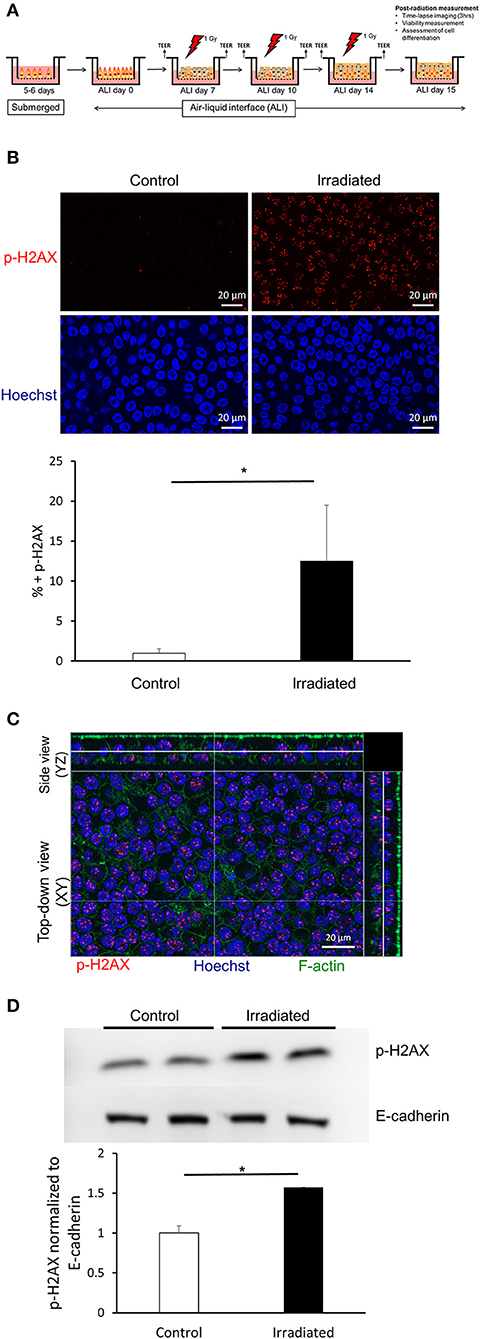
Figure 1. Ionizing radiation induces DNA damage. (A) Timeline of the experimental protocol performed to investigate epithelial cell unjamming induced by ionizing radiation. In primary HBE cells maintained in air-liquid interface culture exposed to ionizing radiation (IR), we determined DNA damage, barrier integrity, cellular viability, epithelial differentiation, as well as cellular shape and migration. (B) Representative images of p-H2AX (top, red) and nuclei stained with Hoechst (bottom, blue) from six independent experiments. IR exposure induced p-H2AX indicating increased DNA damage. Images were captured with a 63X objective (scale bars = 20 μm). The quantification of the mean area of positive p-H2AX staining is presented in the graph. Error bars represent the standard deviation from four FOVs (n = 4) from one representative experiment. (C) Representative orthogonal images of p-H2AX (red), F-actin (green), and nuclei stained with Hoechst (blue). Images were captured with a 63X objective (scale bar = 20 μm). (D) Western blotting confirms that IR induced p-H2AX. Quantified p-H2AX normalized to E-cadherin is presented in the graph. Error bars represent the standard deviation from two samples (n = 2).
Ionizing Radiation Does Not Disrupt Normal Epithelial-Cell Functions
To determine if DSBs induced by IR led to disruption of normal epithelial functions, we assessed barrier integrity, cellular viability, and epithelial differentiation. On ALI days 7, 10, and 14, barrier integrity of the HBE-cell layer was measured by transepithelial electrical resistance (TEER) at 1 h before and after each IR exposure (1 Gy) (Figure 2A). As expected for the normally maturing airway epithelial layer, TEER increased over ALI days, as previously reported (Park et al., 2015). In control HBE cells there was no difference in TEER before and after sham treatment. To our surprise, however, in irradiated cells there was also no difference in TEER before and after radiation exposure. Across four independent HBE-cell donors, TEER values post-irradiation on ALI day 14 showed no reduction compared with control (Figure 2B), thus indicating that repeated exposure to IR (1 Gy) did not compromise barrier function. At 24 h after the final exposure to radiation, we also examined cell viability by dead-cell staining with ethidium homodimer (EthD-1) that is only permeable to dead cells (Somodi and Guthoff, 1995). As a positive control for cell death, we treated cells with 70% methanol and detected robust reductions in cellular viability as expected. However, we observed no significant difference in cellular viability between the control and the irradiated cells, (Figure 2C) further indicating that repeated exposure to radiation (1 Gy) did not affect cellular viability.
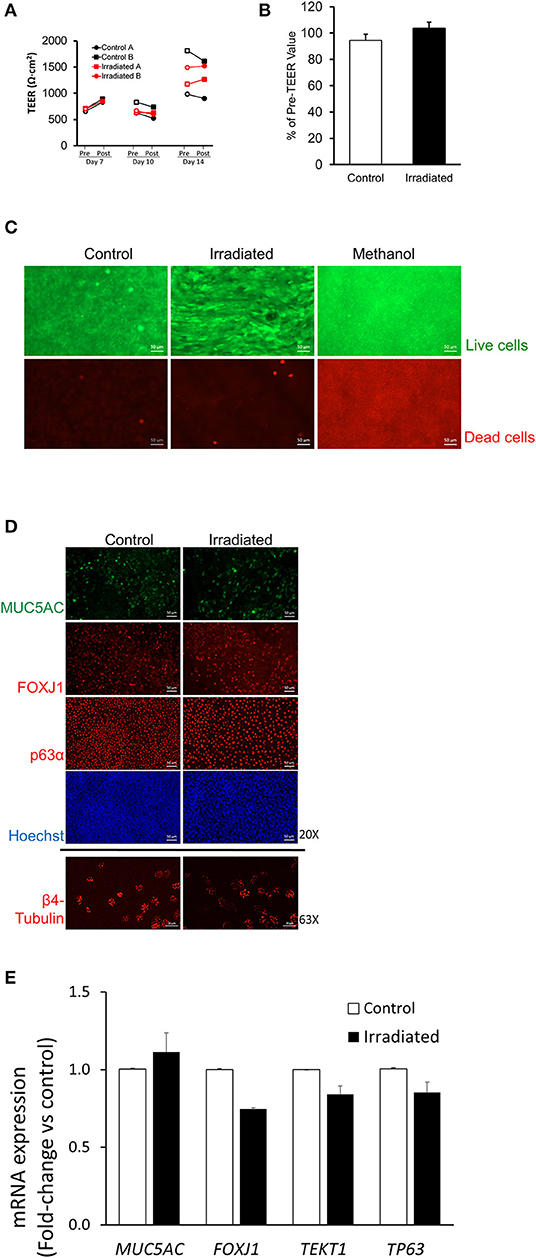
Figure 2. Ionizing radiation does not disrupt normal epithelial cell functions. (A) On ALI days 7, 10, and 14, TEER measured 1 h before (pre-exposure, open symbols) and 1 h after (post-exposure, closed symbols) indicates that IR exposure did not reduce TEER. Black lines represent TEER measured from two independent wells (Control A and Control B) of control and red lines represent TEER measured from two independent wells (Irradiated A and Irradiated B) of irradiated cells from one donor. (B) On ALI day 14, the percent change in TEER comparing 1 h pre-, and 1 h post-IR exposure showed no difference between control (white bar) and irradiated (black bar) cells, indicating that IR did not reduce TEER. Error bars represent the standard error of the mean from four independent donors (n = 4). (C) Methanol treatment used as a positive control for cell death resulted in prominent cell death as indicated by EthD-1 staining (red), whereas neither control nor IR exposure induced cell death (scale bars = 50 μm). Representative images (D) of MUC5AC, FOXJ1, p63α (20X), and β4-tubulin (63X). RT-qPCR (E) showing mRNA expression of MUC5AC, FOXJ1, TEKT1, and TP63 indicate no differences in cellular differentiation between control and irradiated cells. Scale bar = 50 μm (top 8 panels), 20 μm (bottom 2 panels) in (D) and error bars represent the standard deviation from two representative donors (n = 2) in (E).
During ALI culture conditions, basal HBE cells differentiate into goblet and ciliated cells (Stewart et al., 2012; Figure 2D). In control and irradiated cells, we observed a similar degree of differential populations of HBE cells by immunofluorescence staining for epithelial cell-type markers: MUC5AC for goblet cells; FOXJ1 and β4-tubulin for ciliated cells; p63α for basal cells (Figure 2D). Higher magnification, orthogonal side-view images of each cell marker shows that irradiated cells indeed differentiate, as exhibited by their expected localization in the baso-lateral plane (Supplementary Figure 1).
Quantification of the differential cell markers is technically challenging in the pseudostratified epithelium. Therefore, we analyzed mRNA expression of differentiated epithelial cell-type markers by RT-qPCR analysis (Figure 2E). We also measured mRNA expression of TEKT1, a gene encoding tektin-1 protein expressed in cilia (Yoshisue et al., 2004; Park and Tschumperlin, 2009). In control and irradiated cells, there was no difference in the levels of mRNA expression of MUC5AC, FOXJ1, TEKT1, and TP63. Collectively, these data demonstrate that repeated exposure of the primary HBE cells to radiation (1 Gy) induces DNA damage but does not affect epithelial layer integrity, cellular viability, or cellular differentiation.
Ionizing Radiation Induces Epithelial Unjamming in a TGF-β Receptor-Dependent Manner
To determine UJT in HBE cells, we measured cellular shape and motility. Because structural signatures reflect dynamic process of the UJT, we first used cell shape analysis to assess UJT (Park et al., 2015; Atia et al., 2018). We fixed cells at 24 h after the final exposure to IR and stained for F-actin to mark cell boundaries (Figure 3A). Control cells maintained a cobblestone-like shape whereas irradiated cells became elongated (Figure 3A). To determine systematic differences of cell shapes between control and irradiated cells, we measured cellular aspect ratio (AR) (Mashburn et al., 2012). Mean AR showed a significant difference between control and irradiated cells (Figure 3B), suggesting that irradiation induced cell shape elongation. In addition, we took time-lapse images of the pseudostratified epithelial layers starting at 5 h post-irradiation and analyzed cell motility and dynamics. Through optical flow analysis, we computed root-mean-square (RMS) displacements over 3 h. Compared to the time-matched controls, exposure to IR significantly increased the average RMS displacement of the HBE cells (Control: 0.79 ± 0.09 μm vs. IR: 6.21 ± 1.95 μm) (Supplementary Video 1).
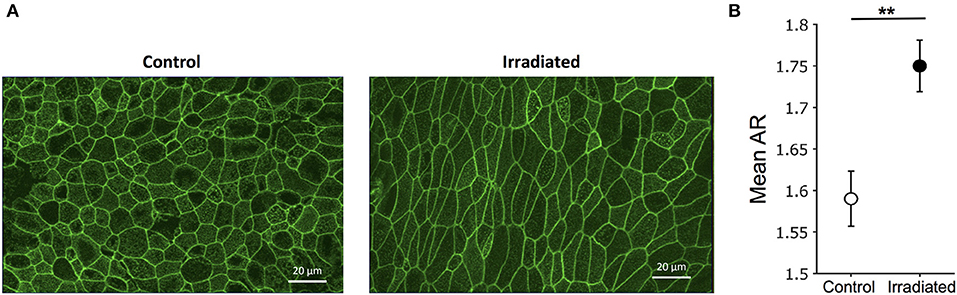
Figure 3. Ionizing radiation induces cell shape changes. (A) Representative images of F-actin staining indicate cobblestone-like shape in control (left) and elongated shape in irradiated cells (right) (scale bars = 20 μm). (B) Segmented images of F-actin staining (as shown in A) were used to calculate aspect ratio (AR = length of long axis/length of short axis). Mean aspect ratio, mean (AR), was calculated in four fields of view. Error bars represent the standard error of the mean from five independent experiments (n = 5).
To test whether inhibition of TGF-β receptor activity prevents the IR-induced UJT, HBE cells were pre-treated with the TGF-β receptor inhibitor, SB431542 (SB, 10 μM) (or vehicle) for 1 h prior to each IR exposure. Pre-treatment with the TGF-β receptor inhibitor partially attenuated the IR-increased average displacement of the cells (veh + IR: 6.21 ± 1.95 μm vs. SB + IR: 2.60 ± 0.75 μm; Figures 4A,B). Taken together, these data indicate that exposure of airway epithelial cells to IR provokes a UJT, accompanied by cell shape change. This IR-induced UJT is partially mediated via TGF-β receptor signaling. Because the activation of TGF-β receptor pathway is known to induce epithelial-to-mesenchymal transition (EMT) (Xu et al., 2009; Nieto et al., 2016), we examined if irradiated cells were undergoing EMT. While the cells treated with TGF-β1, as a positive control for the EMT, showed substantially increased expression of EMT marker genes, including FN-EDA, VIM, and ZEB1, irradiated cells were unchanged in their mRNA expressions (Supplementary Figure 2).
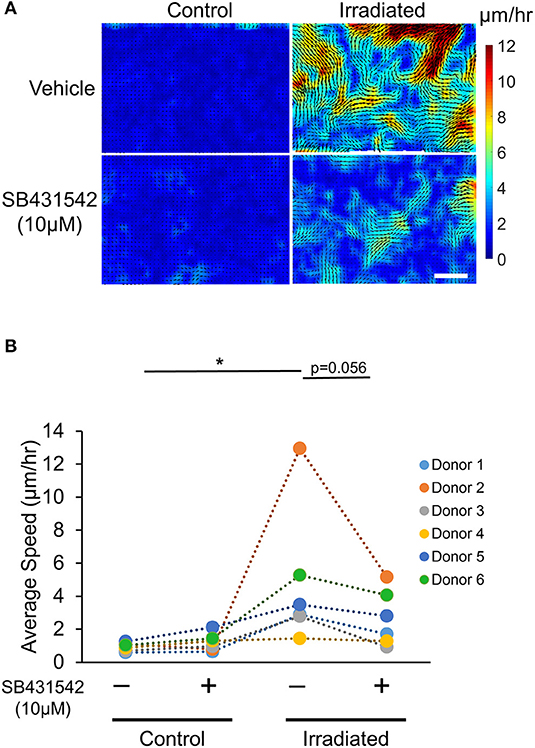
Figure 4. Irradiation-induced collective cell migration partially depends on TGF-β receptor. Representative displacement maps overlaid with velocity heat maps (A) and the quantified cellular motility (B) indicate that IR induced cellular motility, which was attenuated by SB431542 (a TGF-β receptor inhibitor) (Scale bar = 100 μm). Six donors (n = 6) were used as indicated.
Materials and Methods
Culture of Primary Human Bronchial Epithelial Cells
Primary HBE cells were obtained from the CF Center Tissue Procurement and Cell Culture Core, the University of North Carolina at Chapel Hill (courtesy of Dr. Scott Randell). As previously described (Park et al., 2015), these cells were isolated from lungs unsuitable for transplant. The lungs were obtained under protocol #03-1396 approved by the Institutional Review Board at the University of North Carolina at Chapel Hill. Informed consent was obtained from authorized representatives of all organ donors. As previously described (Park et al., 2015; Mitchel et al., 2016), HBE cells were seeded on transwells coated with 0.05 mg/ml rat tail collagen 1 and maintained in ALI culture until the cells were well-differentiated as determined by immunofluorescent staining for goblet cells marked for MUC5AC, ciliated cells marked for FOXJ1 and b4-tubulin, and basal cells marked for p63α (Figure 2D).
Exposure of Primary Airway Epithelial Cells to Ionizing Radiation
For the exposure of HBE cells to ionizing radiation, we used a RadSource RS 2000 Biological Research Irradiator (RadSource, Brentwood, TN). HBE cells were exposed to radiation over the course of ALI cultures, on days 7, 10, and 14. A dose of 1 Gy was achieved by setting the instrument to 160 kV, 25 mA for 87 s. During the radiation exposure, time-matched control (non-irradiated) cells were placed outside of the RadSource machine (Figure 1A demonstrates a timeline of the experimental procedures).
In experiments where TGF-β receptor was inhibited, SB431542 (10 μM) was added to the basolateral culture medium 1 h prior to each IR exposure. For vehicle of SB431542, DMSO (1%) was used.
Immunofluorescent Staining
HBE cells on the transwell were fixed in 4% paraformaldehyde (PFA) for 30 min and subsequently washed with PBS. Cells were then permeabilized in 0.2% Triton X-100 in phosphate-buffered saline (PBST) for 10 min. Permeabilized cells were blocked in 10% non-specific goat serum and 1% bovine serum albumin (BSA) in PBST for 1 h. Primary antibody against p-H2AX (20E3, Cell Signaling Technology, Danvers, MA) was diluted at 1:200. Secondary antibody conjugated to Alexa Fluor 594 (ThermoFisher Scientific) was diluted at 1:500. Stained cells were mounted on coverslips and imaged with a fluorescent microscope (Axio Observer, Zeiss, Germany). To observe the differentiation of the ALI cultures, we stained fixed cells with primary antibodies for MUC5AC (45M1, ThermoFisher Scientific), FOXJ1 (2A5, ThermoFisher Scientific), β4-tubulin (ONS, Millipore Sigma), and p63α (D2K8X, Cell Signaling Technology). All primary antibodies were diluted at 1:500, followed by appropriate secondary antibody (AlexaFluor mouse/rabbit). Stained cells were counter stained for F-actin using phalloidin conjugated to AlexaFluor 488 (ThermoFisher Scientific) diluted at 1:40 and with Hoechst 33342 (ThermoFisher Scientific) diluted at 1:5000.
Western Blotting
Protein lysates were separated in a 10% Mini-PROTEAN TGX pre-cast gel (Biorad). Proteins were transferred to a PVDF membrane which was subsequently blocked in 5% skim milk. The PVDF membrane was incubated with antibody against p-H2AX (20E3, Cell Signaling Technology) or E-cadherin (24E10, Cell Signaling Technology) and subsequently with secondary antibodies.
RT-qPCR
RNA was extracted from cells using RNeasy Mini Kit (Qiagen, Valencia, CA) according to the manufacturer's instructions. One μg of total RNA was used to synthesize cDNA using MultiScribe reverse transcriptase (Applied Biosystems, Foster City, CA). RT-qPCR was performed using 25 ng of cDNA, primers (Table 1) and 2X SYBR Green PCR Mastermix.
Cell Shape Analysis
To determine if cell shape changes were affected by IR exposure, cells were stained for F-actin as described above. Then, maximum intensity projections of F-actin images were created in ImageJ software (Schindelin et al., 2012) and cells were segmented using SeedWaterSegmenter (Anaconda2). As previously reported (Atia et al., 2018), cellular aspect ratio (AR = long axis/short axis) was calculated and the mean AR was computed.
Transepithelial Electrical Resistance
To determine if the integrity of airway epithelial cell layer is disrupted after radiation exposure, we used a widely accepted assay, TEER measurement, using an epithelial volt/ohm meter (EVOM2) (World Precision Instruments, Sarasota, FL), following the manufacturer's instruction, as we previously used (Park et al., 2015). In each well, TEER was measured 1 h before and 1 h after control treatment or irradiation. In some experiments, TEER was measured subsequently on ALI days 7, 10, and 14 (Figure 2A) following each exposure, whereas in other experiments (Figure 2B), TEER was measured only on ALI day 14 following the last exposure.
Live/Dead Stain
To assess the viability of the cells post-radiation, cells were stained with calcein-AM and EthD-1 (Viability/Cytotoxicity kit, ThermoFisher Scientific, Waltham, MA) was performed according to manufacturer's protocol. As a positive control for cell death, other cells from independent culture wells were incubated with 70% methanol for 30 min. Briefly, at 24 h after the final IR, cells grown on transwells were incubated in PBS containing 2 μM calcein AM and 4 μM EthD-1 for 30 min. Then, stained cells were placed on glass coverslips and imaged at a single z-plane with a fluorescent microscope (Axio Observer, Zeiss, Germany).
Time-Lapse Imaging
To monitor the motility of the cells after the final IR exposure, time-lapse images of the cells were taken at 3 min-intervals for 18 h. The imaging chamber was supplied with 37°C, 5% CO2 humidified air. A 10X objective, mounted on an Axio Observer (Zeiss, Germany) was used to collect phase contrast images. Each condition was run in duplicate and images from six fields of view per well were randomly collected. The average speed of cellular migration was assessed by Farneback optical flow analysis using Matlab (MathWorks, Natick, MA).
Statistics
Statistical analysis was performed in GraphPad Prism 8 software (GraphPad Software Inc., San Diego, CA). Data are presented as mean + SD or + SEM as indicated, except Figure 3, which is represented by Mean ± SEM. In experiments with two groups, a two-tailed, paired Student's t-test was utilized. In experiments with more than two groups, a one-way ANOVA with Tukey's post-hoc test was utilized. P-values < 0.05 were considered to be significant.
Discussion
The goal of this study is to provide the insight into the paradoxical effect of therapeutic radiation on cell motility (Moncharmont et al., 2014; Vilalta et al., 2016; Blyth et al., 2018). To address this question, we utilized in vitro culture of primary human airway epithelial cells as a model of the healthy, mature epithelium. In this epithelial cell layer system, irradiation caused DNA damage but did not disrupt normal differentiation or layer integrity. Irradiation did, however, induce cellular migration and cell shape elongation, both hallmarks of the UJT (Bi et al., 2015; Park et al., 2015; Atia et al., 2018). Our data therefore indicate that IR induced the UJT of well-differentiated, healthy HBE cells in a TGF-β receptor-dependent manner. This UJT of the non-cancerous healthy cells might create a fluidized environment, in which tumor cells might efficiently disseminate.
Under homeostatic conditions, the epithelium is typically non-migratory. However, under a variety of circumstances, the non-migratory layer becomes migratory. The transition occurs under both physiological circumstances, including wound healing, embryonic development, and pathophysiological circumstances, including tissue remodeling, cancer invasion, and metastasis. Transition of the non-migratory confluent epithelium toward the migratory state has traditionally been attributed to EMT or partial-EMT (pEMT) (Hay, 1982, 1995; Boyer et al., 1989; Savagner, 2015; Nieto et al., 2016; Brabletz et al., 2018), but in some cases can be attributed to the UJT (Sadati et al., 2013; Fredberg, 2014; Park et al., 2015, 2016; Pegoraro et al., 2016; Atia et al., 2018; Mitchel et al., 2019). The UJT together with associated fluidization of the confluent living tissue was first discovered in our laboratory (Trepat et al., 2009; Sadati et al., 2013; Park et al., 2015; Atia et al., 2018). This discovery was rooted firmly in airway biology. Since that time, the concept of cell jamming has diffused widely into the study of other collective cellular systems, and growing evidence from us and others now suggests that the capacity to jam and unjam may be an innate property of many epithelia both in normal development (Atia et al., 2018; Mongera et al., 2018; Spurlin et al., 2019) and in disease (Sadati et al., 2013; Haeger et al., 2014; Park et al., 2015; Bi et al., 2016; Gamboa Castro et al., 2016; Oswald et al., 2017; Atia et al., 2018; Mongera et al., 2018; Palamidessi et al., 2018, 2019; Fujii et al., 2019; Spurlin et al., 2019), including asthma (Angelini et al., 2011; Nnetu et al., 2012; Garcia et al., 2015; Park et al., 2015, 2016; Malinverno et al., 2017; Atia et al., 2018), and cancer (Haeger et al., 2014; Oswald et al., 2017; Palamidessi et al., 2019).
In cancer clinics, radiation is the most commonly used to kill or shrink tumors but sometimes results in the undesirable side effect of promoting migration and metastasis in surviving cancer cells. Further, tumor cells cultured in pre-irradiated stroma become highly metastatic, known as the tumor bed-effect (Monnier et al., 2008). However, these mechanism(s) remain unclear. In A549 cells (adenocarcinoma alveolar type II cells), IR (at 6 or 12 Gy) augments migration of the cells into a scratched-wound (Jung et al., 2007). This augmented migration is accompanied by cell-shape elongation (Jung et al., 2007). During cancer therapy, not only cancer cells, but also surrounding healthy epithelial cells are subjected to radiation. IR doses delivered to tumors often exceed a cumulative dose of 60 Gy, delivered over multiple treatment days (Kong et al., 2014). Delivery of high doses with minimal off-target damage is achieved by three-dimension-conformal treatment, which mitigates risk of exposure to nearby healthy tissue. Advances in radiotherapy delivery techniques have allowed for a sharp focus on the target tissue, limiting the damage to the surrounding stroma (Intensity Modulated Radiation Therapy Collaborative Working Group, 2001; Verellen et al., 2007). The resulting dose delivered to surrounding tissue is thus significantly lower than that which the tumor receives. Here, we utilized a 1 Gy dose, which is much lower than the therapeutic dose used in the clinic. Given three times over ALI culture of healthy primary HBE cells, the cumulative dose was 3 Gy. By the use of radiation at this dose, we did not observe cell-death but observed a striking migratory response, which could in turn affect physical behavior of neighboring cancer cells. Often, the radiated healthy epithelial cells are considered to promote cancer cell survival (Blyth et al., 2018). It has also been shown that low doses (0.8 Gy) of radiation may contribute to metastasis by increasing angiogenesis at the tumor site (Sofia Vala et al., 2010). However, the effect of radiation on cellular migration in healthy, mature, confluent airway epithelium had been unknown. Using primary HBE cells maintained in ALI, we examined the effects of irradiation on the homeostatic function of epithelial cells, as well as cellular migration.
Exposure of the cells to IR causes DNA damage by cleaving the phosphodiester bond in the backbone of the DNA (Lomax et al., 2013), leading to a DSB. Upon DSB, ATM Kinase phosphorylates H2AX followed by the cascade of DNA repair (Burma et al., 2001). Thus, we first determined if IR causes DSBs in HBE cells cultured at ALI. As we expected, exposure of HBE cells to IR (1 Gy) indeed caused DNA damage that is marked by p-H2AX (Figure 1C). Among the cellular events induced by IR, this observation can be particularly linked to asthma. In patients with asthma, the airway epithelium possesses an increased level of p-H2AX, suggesting that DNA damage is greater, or that DNA repair mechanisms are impaired in asthmatic airways (Chan et al., 2016). Furthermore, in a house dust mite (HDM) mouse model of allergic asthma, p-H2AX is increased in the airway epithelium (Chan et al., 2016). Surprisingly, a recent study suggests that IL-13 could be a therapeutic target for radiation-induced pulmonary fibrosis (Chung et al., 2016). IL-13 is a type 2 cytokine that is strongly associated with asthma (Rael and Lockey, 2011) and induces asthmatic airway remodeling, including increased subepithelial fibroblast proliferation (Kraft et al., 2001). Thus, there may be some common pathways that are shared between radiation-induced lung injury and asthma.
Despite substantial DNA damage marked by p-H2AX, radiation exposure at this sub-therapeutic dose (1 Gy) affected neither epithelial layer integrity nor normal epithelial differentiation, both of which are critical for maintaining proper epithelial functions (Figure 2). It is possible that radiation resulted in less p63α positive basal cells (Figure 2D), which may indicate the transition of basal into more differentiated cell types after exposure to DNA damage. However, we did not detect significant differences in TP63 mRNA expression. In irradiated cells, we observed a small reduction in both marker genes for ciliated cells, FOXJ1 and TEKT1 (Ryan et al., 2018), but it was not statistically significant (Figure 2E). Furthermore, we did not see any meaningful differences in any cell type marker proteins marked by immunofluorescent staining (Figure 2D), indicating that irradiated cells maintain their ability to differentiate similarly to non-radiated cells.
Without disruption of the epithelial barrier in the confluent epithelial layer, IR substantially induced cellular migration. To identify the mode of this increased cellular migration, we analyzed the cell shape and the speed of cellular motility to determine if this migration is attributable to the UJT. Although IR did not break cell-cell junctions as indicated by the lack of reduction in TEER (Figures 2A,B), IR caused significant changes in cell shape from cobblestone-like to elongated (Figure 3). IR also increased cellular motility (Figure 4), which was attenuated by pretreatment with a TGF-β receptor inhibitor. The TGF-β signaling pathway plays an important role in irradiation-induced fibrotic remodeling (Monson et al., 1998) and the inhibition of TGF-β signaling reduces irradiation-induced fibrosis in humans (Xavier et al., 2004). Furthermore, the increase in TGF-β expression in the mouse lung post-IR depends on IL-13, a type 2 cytokine (Chung et al., 2016), which is also tightly associated with asthma (Vignola et al., 1996; Minshall et al., 1997; Doran et al., 2017). TGF-β is a pleotropic factor that promotes a variety of asthmatic airway remodeling processes, including myofibroblast differentiation (Michalik et al., 2009), development of airway hyperresponsiveness (Leung et al., 2006), and airway smooth muscle proliferation (Makinde et al., 2007). Furthermore, TGF-β is a strong stimulator for epithelial cell migration through the EMT (Jakowlew, 2006; Al-Alawi et al., 2014; Nieto et al., 2016; Brabletz et al., 2018). In irradiated cells, our data indicate no signs of EMT (Supplementary Figure 2) but strong signs of UJT.
Conclusion
In the present study, we investigated the unjamming transition, an emergent phenomenon in epithelial cell migration, induced by radiation exposure. Despite substantial DNA damage detected in the radiated cells, the integrity of the epithelial-cell layer, cellular viability, and degree of epithelial differentiation were not disrupted by radiation. Radiation induced the UJT that is characterized by increased collective cellular migration and elongated cell shape changes. Furthermore, blocking of TGF-β receptor attenuated the degree of radiation-induced cell migration, indicating a role for TGF-β receptor signaling in HBE-cell unjamming.
Data Availability Statement
The datasets generated for this study are available on request to the corresponding author.
Author Contributions
MO'S, JM, ZN, and J-AP designed the experiments. MO'S and JM performed experiments. MO'S, JM, AD, and SK analyzed the results. MO'S, JM, AD, SK, HL, DB, ZN, and J-AP interpreted the data and revised the manuscript. MO'S, JM, and J-AP drafted the manuscript.
Funding
The authors acknowledge the funding provided by P01HL120839, U01CA244086, R01HL148152, T32HL007118, and P30DK065988.
Conflict of Interest
The authors declare that the research was conducted in the absence of any commercial or financial relationships that could be construed as a potential conflict of interest.
Acknowledgments
The authors thank Dr. Jim Butler for discussion of the data and Dr. Jeff Fredberg for constructive comments and editing of the manuscript at the Harvard T. H. Chan School of Public Health. They also thank Dr. Zhi-Min Yuan at the John B. Little Center for Radiation Sciences at the Harvard T. H. Chan School of Public Health, Dr. Jeremy Bredfeldt at the Brigham and Women's Hospital, and Dr. Michele Moreau at the Dana-Farber Cancer Institute for providing resources for irradiation exposure and Dr. Scott Randell at the Marisco Lung Institute, The University of North Carolina at Chapel Hill for providing primary human bronchial epithelial cells.
Supplementary Material
The Supplementary Material for this article can be found online at: https://www.frontiersin.org/articles/10.3389/fcell.2020.00021/full#supplementary-material
Supplementary Video 1. In control (left) and irradiated (right) cells, time-lapse images were taken between 5 and 8 h after exposure at an acquisition rate of every 3 min. In the converted movies, images are shown at 20 frames per second (one second of video is equal to 1 h of experimental time).
Supplementary Figure 1. Representative orthogonal (top-down view: X-Y and side view: Y-Z) and single z-plane (center) images from irradiated cells as shown in Figure 2D: MUC5AC (green), FOXJ1 (red), β4-tubulin (red), p63α (red), and Hoechst (blue). White line through orthogonal section indicates z-plane displayed (scale bar = 20 μm).
Supplementary Figure 2. In primary HBE cells, radiation did not induce EMT. To determine EMT, we measured mRNA expressions of EMT-related proteins, including fibronectin-EDA, vimentin and Zeb1 by RT-qPCR. In the cells exposed to TGFβ (10 ng/ml) as a positive control for the EMT, we detected a significantly increased expression of three genes, whereas in the cells exposed to radiation, we detected no meaningful increase in three genes, suggesting no sign of EMT.
References
Al-Alawi, M., Hassan, T., and Chotirmall, S. H. (2014). Transforming growth factor beta and severe asthma: a perfect storm. Respir. Med. 108, 1409–1423. doi: 10.1016/j.rmed.2014.08.008
Angelini, T. E., Hannezo, E., Trepat, X., Marquez, M., Fredberg, J. J., and Weitz, D. A. (2011). Glass-like dynamics of collective cell migration. Proc. Natl. Acad. Sci. U. S. A. 108, 4714–4719. doi: 10.1073/pnas.1010059108
Atia, L., Bi, D., Sharma, Y., Mitchel, J. A., Gweon, B., Koehler, S., et al. (2018). Geometric constraints during epithelial jamming. Nat. Phys. 14, 613–620. doi: 10.1038/s41567-018-0089-9
Bi, D., Lopez, J. H., Schwarz, J. M., and Manning, M. L. (2014). Energy barriers and cell migration in densely packed tissues. Soft Matter 10, 1885–1890. doi: 10.1039/c3sm52893f
Bi, D., Lopez, J. H., Schwarz, J. M., and Manning, M. L. (2015). A density-independent glass transition in biological tissues. Nat. Phys. 11, 1074–1079. doi: 10.1038/nphys3471
Bi, D., Yang, X., Marchetti, M. C., and Manning, M. L. (2016). Motility-driven glass and jamming transitions in biological tissues. Phys. Rev. 6:021011. doi: 10.1103/PhysRevX.6.021011
Blyth, B. J., Cole, A. J., MacManus, M. P., and Martin, O. A. (2018). Radiation therapy-induced metastasis: radiobiology and clinical implications. Clin. Exp. Metastas. 35, 223–236. doi: 10.1007/s10585-017-9867-5
Boyer, B., Tucker, G. C., Vallés, A. M., Franke, W. W., and Thiery, J. P. (1989). Rearrangements of desmosomal and cytoskeletal proteins during the transition from epithelial to fibroblastoid organization in cultured rat bladder carcinoma cells. J. Cell Biol. 109, 1495–1509. doi: 10.1083/jcb.109.4.1495
Brabletz, T., Kalluri, R., Nieto, M. A., and Weinberg, R. A. (2018). EMT in cancer. Nat. Rev. Cancer 18, 128–134. doi: 10.1038/nrc.2017.118
Burma, S., Chen, B. P., Murphy, M., Kurimasa, A., and Chen, D. J. (2001). ATM phosphorylates histone H2AX in response to DNA double-strand breaks. J. Biol. Chem. 276, 42462–42467. doi: 10.1074/jbc.C100466200
Chaffer, C. L., and Weinberg, R. A. (2011). A perspective on cancer cell metastasis. Science 331, 1559–1564. doi: 10.1126/science.1203543
Chan, T. K., Loh, X. Y., Peh, H. Y., Tan, W. N. F., Tan, W. S. D., Li, N., et al. (2016). House dust mite-induced asthma causes oxidative damage and DNA double-strand breaks in the lungs. J. Allergy Clin. Immunol. 138, 84–96 e1. doi: 10.1016/j.jaci.2016.02.017
Chu, E. K., Cheng, J., Foley, J. S., Mecham, B. H., Owen, C. A., Haley, K. J., et al. (2006). Induction of the plasminogen activator system by mechanical stimulation of human bronchial epithelial cells. Am. J. Respir. Cell Mol. Biol. 35, 628–638. doi: 10.1165/rcmb.2006-0040OC
Chung, S. I., Horton, J. A., Ramalingam, T. R., White, A. O., Chung, E. J., Hudak, K. E., et al. (2016). IL-13 is a therapeutic target in radiation lung injury. Sci. Rep. 6:39714. doi: 10.1038/srep39714
Doerner, A. M., and Zuraw, B. L. (2009). TGF-beta1 induced epithelial to mesenchymal transition (EMT) in human bronchial epithelial cells is enhanced by IL-1beta but not abrogated by corticosteroids. Respir. Res. 10:100. doi: 10.1186/1465-9921-10-100
Doran, E., Cai, F., C., Holweg, C. T. J., Wong, K., Brumm, J., Arron, J. R., et al. (2017). Interleukin-13 in asthma and other eosinophilic disorders. Front. Med. 4:139. doi: 10.3389/fmed.2017.00139
Fredberg, J. J. (2014). Power steering, power brakes, and jamming: evolution of collective cell-cell interactions. Physiology 29, 218–219. doi: 10.1152/physiol.00026.2014
Fujii, Y., Ochi, Y., Tuchiya, M., Kajita, M., Fujita, Y., Ishimoto, Y., et al. (2019). Spontaneous spatial correlation of elastic modulus in jammed epithelial monolayers observed by AFM. Biophys. J. 116, 1152–1158. doi: 10.1016/j.bpj.2019.01.037
Fulcher, M. L., Gabriel, S., Burns, K. A., Yankaskas, J. R., and Randell, S. H. (2005). Well-differentiated human airway epithelial cell cultures. Methods Mol. Med. 107, 183–206. doi: 10.1385/1-59259-861-7:183
Gamboa Castro, M., Leggett, S. E., and Wong, I. Y. (2016). Clustering and jamming in epithelial-mesenchymal co-cultures. Soft Matter 12, 8327–8337. doi: 10.1039/C6SM01287F
Garcia, S., Hannezo, E., Elgeti, J., Joanny, J. F., Silberzan, P., and Gov, N. S. (2015). Physics of active jamming during collective cellular motion in a monolayer. Proc. Natl. Acad. Sci. U. S. A. 112, 15314–15319. doi: 10.1073/pnas.1510973112
Haeger, A., Krause, M., Wolf, K., and Friedl, P. (2014). Cell jamming: collective invasion of mesenchymal tumor cells imposed by tissue confinement. Biochim. Biophys. Acta 1840, 2386–2395. doi: 10.1016/j.bbagen.2014.03.020
Hay, E. D. (1982). Interaction of embryonic surface and cytoskeleton with extracellular matrix. Am. J. Anat. 165, 1–12. doi: 10.1002/aja.1001650102
Hay, E. D. (1995). An overview of epithelio-mesenchymal transformation. Acta Anat. 154, 8–20. doi: 10.1159/000147748
Intensity Modulated Radiation Therapy Collaborative Working Group. (2001). Intensity-modulated radiotherapy: current status and issues of interest. Int. J. Radiat. Oncol. Biol. Phys. 51, 880–914. doi: 10.1016/S0360-3016(01)01749-7
Jakowlew, S. B. (2006). Transforming growth factor-beta in cancer and metastasis. Cancer Metastasis. Rev. 25, 435–457. doi: 10.1007/s10555-006-9006-2
Jung, J. W., Hwang, S. Y., Hwang, J. S., Oh, E. S., Park, S., and Han, I. O. (2007). Ionising radiation induces changes associated with epithelial-mesenchymal transdifferentiation and increased cell motility of A549 lung epithelial cells. Eur. J. Cancer 43, 1214–1224. doi: 10.1016/j.ejca.2007.01.034
Kong, F. M., Zhao, J., Wang, J., and Faivre-Finn, C. (2014). Radiation dose effect in locally advanced non-small cell lung cancer. J. Thorac. Dis. 6, 336–47. doi: 10.3978/j.issn.2072-1439.2014.01.23
Kraft, M., Lewis, C., Pham, D., and Chu, H. W. (2001). IL-4, IL-13, and dexamethasone augment fibroblast proliferation in asthma. J. Allergy Clin. Immunol. 107, 602–606. doi: 10.1067/mai.2001.113760
Kuo, L. J., and Yang, L. X. (2008). Gamma-H2AX - a novel biomarker for DNA double-strand breaks. In vivo 22, 305−309.
Leung, S. Y., Niimi, A., Noble, A., Oates, T., Williams, A. S., Medicherla, S., et al. (2006). Effect of transforming growth factor-beta receptor I kinase inhibitor 2,4-disubstituted pteridine (SD-208) in chronic allergic airway inflammation and remodeling. J. Pharmacol. Exp. Ther. 319, 586–594. doi: 10.1124/jpet.106.109314
Lomax, M. E., Folkes, L. K., and O'Neill, P. (2013). Biological consequences of radiation-induced DNA damage: relevance to radiotherapy. Clin. Oncol. 25, 578–585. doi: 10.1016/j.clon.2013.06.007
Makinde, T., Murphy, R. F., and Agrawal, D. K. (2007). The regulatory role of TGF-beta in airway remodeling in asthma. Immunol. Cell Biol. 85, 348–356. doi: 10.1038/sj.icb.7100044
Malinverno, C., Corallino, S., Giavazzi, F., Bergert, M., Li, Q., Leoni, M., et al. (2017). Endocytic reawakening of motility in jammed epithelia. Nat. Mater. 16, 587–596. doi: 10.1038/nmat4848
Mariotti, L. G., Pirovano, G., Savage, K. I., Ghita, M., Ottolenghi, A., Prise, K. M., et al. (2013). Use of the gamma-H2AX assay to investigate DNA repair dynamics following multiple radiation exposures. PLoS ONE 8:e79541. doi: 10.1371/journal.pone.0079541
Mashburn, D. N., Lynch, H. E., Ma, X., and Hutson, M. S. (2012). Enabling user-guided segmentation and tracking of surface-labeled cells in time-lapse image sets of living tissues. Cytometry A 81, 409–418. doi: 10.1002/cyto.a.22034
Michalik, M., Pierzchalska, M., Legutko, A., Ura, M., Ostaszewska, A., Soja, J., et al. (2009). Asthmatic bronchial fibroblasts demonstrate enhanced potential to differentiate into myofibroblasts in culture. Med. Sci. Monit. 15, BR194–201.
Minshall, E. M., Leung, D. Y., Martin, R. J., Song, Y. L., Cameron, L., Ernst, P., et al. (1997). Eosinophil-associated TGF-beta1 mRNA expression and airways fibrosis in bronchial asthma. Am. J. Respir. Cell Mol. Biol. 17, 326–333. doi: 10.1165/ajrcmb.17.3.2733
Mitchel, J. A., Antoniak, S., Lee, J. H., Kim, S. H., McGill, M., Kasahara, D. I., et al. (2016). IL-13 augments compressive stress-induced tissue factor expression in human airway epithelial cells. Am. J. Respir. Cell Mol. Biol. 54, 524–531. doi: 10.1165/rcmb.2015-0252OC
Mitchel, J. A., Das, A., O'Sullivan, M. J., Stancil, I. T., DeCamp, S. J., Koehler, S., et al. (2019). The unjamming transition is distinct from the epithelial-to-mesenchymal transition. bioRxiv. 665018. doi: 10.1101/665018
Molina, J. R., Yang, P., Cassivi, S. D., Schild, S. E., and Adjei, A. A. (2008). Non-small cell lung cancer: epidemiology, risk factors, treatment, and survivorship. Mayo Clin. Proc. 83, 584–594. doi: 10.1016/S0025-6196(11)60735-0
Moncharmont, C., Levy, A., Guy, J. B., Falk, A. T., Guilbert, M., Trone, J. C., et al. (2014). Radiation-enhanced cell migration/invasion process: a review. Crit. Rev. Oncol. Hematol. 92, 133–142. doi: 10.1016/j.critrevonc.2014.05.006
Mongera, A., Rowghanian, P., Gustafson, H. J., Shelton, E., Kealhofer, D. A., Carn, E. K., et al. (2018). A fluid-to-solid jamming transition underlies vertebrate body axis elongation. Nature 561, 401–405. doi: 10.1038/s41586-018-0479-2
Monnier, Y., Farmer, P., Bieler, G., Imaizumi, N., Sengstag, T., Alghisi, G. C., et al. (2008). CYR61 and alphaVbeta5 integrin cooperate to promote invasion and metastasis of tumors growing in preirradiated stroma. Cancer Res. 68, 7323–7331. doi: 10.1158/0008-5472.CAN-08-0841
Monson, J. M., Stark, P., Reilly, J. J., Sugarbaker, D. J., Strauss, G. M., Swanson, S. J., et al. (1998). Clinical radiation pneumonitis and radiographic changes after thoracic radiation therapy for lung carcinoma. Cancer. 82, 842–50. doi: 10.1002/(SICI)1097-0142(19980301)82:5<842::AID-CNCR7>3.0.CO;2-L
Nieto, M. A., Huang, R. Y., Jackson, R. A., and Thiery, J. P. (2016). Emt: 2016. Cell 166, 21–45. doi: 10.1016/j.cell.2016.06.028
Nnetu, K. D., Knorr, M., Käs, J., and Zink, M. (2012). The impact of jamming on boundaries of collectively moving weak-interacting cells. New J. Phys. 14:115012. doi: 10.1088/1367-2630/14/11/115012
Oswald, L., Grosser, S., Smith, D. M., and Käs, J. A. (2017). Jamming transitions in cancer. J. Phys. Appl. Phys. 50:483001. doi: 10.1088/1361-6463/aa8e83
Palamidessi, A., Malinverno, C., Frittoli, E., Corallino, S., Barbieri, E., Sigismund, S., et al. (2018). Unjamming overcomes kinetic and proliferation arrest in terminally differentiated cells and promotes collective motility of carcinoma. bioRxiv 388553. doi: 10.1101/388553
Palamidessi, A., Malinverno, C., Frittoli, E., Corallino, S., Barbieri, E., Sigismund, S., et al. (2019). Unjamming overcomes kinetic and proliferation arrest in terminally differentiated cells and promotes collective motility of carcinoma. Nat Mater. 18, 1252–1263. doi: 10.1038/s41563-019-0425-1
Park, J. A., Atia, L., Mitchel, J. A., Fredberg, J. J., and Butler, J. P. (2016). Collective migration and cell jamming in asthma, cancer and development. J. Cell Sci. 129, 3375–3383. doi: 10.1242/jcs.187922
Park, J. A., Kim, J. H., Bi, D., Mitchel, J. A., Qazvini, N. T., Tantisira, K., et al. (2015). Unjamming and cell shape in the asthmatic airway epithelium. Nat. Mater. 14, 1040–1048. doi: 10.1038/nmat4357
Park, J. A., and Tschumperlin, D. J. (2009). Chronic intermittent mechanical stress increases MUC5AC protein expression. Am. J. Respir. Cell Mol. Biol. 41, 459–466. doi: 10.1165/rcmb.2008-0195OC
Pegoraro, A. F., Fredberg, J. J., and Park, J. A. (2016). Problems in biology with many scales of length: cell-cell adhesion and cell jamming in collective cellular migration. Exp. Cell Res. 343, 54–59. doi: 10.1016/j.yexcr.2015.10.036
Rael, E. L., and Lockey, R. F. (2011). Interleukin-13 signaling and its role in asthma. World Allergy Organ. J. 4, 54–64. doi: 10.1097/WOX.0b013e31821188e0
Ryan, R., Failler, M., Reilly, M. L., Garfa-Traore, M., Delous, M., Filhol, E., et al. (2018). Functional characterization of tektin-1 in motile cilia and evidence for TEKT1 as a new candidate gene for motile ciliopathies. Hum. Mol. Genet. 27, 266–282. doi: 10.1093/hmg/ddx396
Sadati, M., Taheri Qazvini, N., Krishnan, R., Park, C. Y., and Fredberg, J. J. (2013). Collective migration and cell jamming. Differentiation 86, 121–5. doi: 10.1016/j.diff.2013.02.005
Savagner, P. (2015). Epithelial-mesenchymal transitions: from cell plasticity to concept elasticity. Curr. Top. Develop. Biol. 112, 273–300. doi: 10.1016/bs.ctdb.2014.11.021
Schindelin, J., Arganda-Carreras, I., Frise, E., Kaynig, V., Longair, M., Pietzsch, T., et al. (2012). Fiji: an open-source platform for biological-image analysis. Nat. Methods 9, 676–682. doi: 10.1038/nmeth.2019
Sofia Vala, I., Martins, L. R., Imaizumi, N., Nunes, R. J., Rino, J., Kuonen, F., et al. (2010). Low doses of ionizing radiation promote tumor growth and metastasis by enhancing angiogenesis. PLoS ONE 5:e11222. doi: 10.1371/journal.pone.0011222
Somodi, S., and Guthoff, R. (1995). Visualization of keratocytes in the human cornea with fluorescence microscopy. Ophthalmologe 92, 452–457.
Spurlin, J. W. 3rd, Siedlik, M. J., Nerger, B. A., Pang, M. F., Jayaraman, S., Zhang, R., et al. (2019). Mesenchymal proteases and tissue fluidity remodel the extracellular matrix during airway epithelial branching in the embryonic avian lung. Development 146:dev175257. doi: 10.1242/dev.175257
Stewart, C. E., Torr, E. E., Mohd Jamili, N. H., Bosquillon, C., and Sayers, I. (2012). Evaluation of differentiated human bronchial epithelial cell culture systems for asthma research. J. Allergy 2012:943982. doi: 10.1155/2012/943982
Tian, B., Li, X., Kalita, M., Widen, S. G., Yang, J., Bhavnani, S. K., et al. (2015). Analysis of the TGFβ-induced program in primary airway epithelial cells shows essential role of NF-κB/RelA signaling network in type II epithelial mesenchymal transition. BMC Genom. 16:529. doi: 10.1186/s12864-015-1707-x
Trepat, X., Wasserman, M., Angelini, T., Millet, E., Weitz, D., Butler, J., et al. (2009). Physical forces during collective cell migration. Nat. Phys. 5, 426–430. doi: 10.1038/nphys1269
Verellen, D., De Ridder, M., Linthout, N., Tournel, K., Soete, G., and Storme, G. (2007). Innovations in image-guided radiotherapy. Nat. Rev. Cancer 7, 949–960. doi: 10.1038/nrc2288
Vignola, A. M., Chanez, P., Chiappara, G., Merendino, A., Zinnanti, E., Bousquet, J., et al. (1996). Release of transforming growth factor-beta (TGF-beta) and fibronectin by alveolar macrophages in airway diseases. Clin. Exp. Immunol. 106, 114–119. doi: 10.1046/j.1365-2249.1996.d01-811.x
Vilalta, M., Rafat, M., and Graves, E. E. (2016). Effects of radiation on metastasis and tumor cell migration. Cell Mol. Life Sci. 73, 2999–3007. doi: 10.1007/s00018-016-2210-5
Xavier, S., Piek, E., Fujii, M., Javelaud, D., Mauviel, A., Flanders, K. C., et al. (2004). Amelioration of radiation-induced fibrosis: inhibition of transforming growth factor-beta signaling by halofuginone. J. Biol. Chem. 279, 15167–15176. doi: 10.1074/jbc.M309798200
Xu, J., Lamouille, S., and Derynck, R. (2009). TGF-beta-induced epithelial to mesenchymal transition. Cell Res. 19, 156–172. doi: 10.1038/cr.2009.5
Yoshisue, H., Puddicombe, S. M., Wilson, S. J., Haitchi, H. M., Powell, R. M., Wilson, D. I., et al. (2004). Characterization of ciliated bronchial epithelium 1, a ciliated cell-associated gene induced during mucociliary differentiation. Am. J. Respir. Cell Mol. Biol. 31, 491–500. doi: 10.1165/rcmb.2004-0050OC
Keywords: epithelium, irradiation, migration, unjamming, metastasis, cancer
Citation: O'Sullivan MJ, Mitchel JA, Das A, Koehler S, Levine H, Bi D, Nagel ZD and Park J-A (2020) Irradiation Induces Epithelial Cell Unjamming. Front. Cell Dev. Biol. 8:21. doi: 10.3389/fcell.2020.00021
Received: 01 November 2019; Accepted: 13 January 2020;
Published: 11 February 2020.
Edited by:
De Yun Wang, National University of Singapore, SingaporeReviewed by:
Hyung-Ju Cho, Yonsei University Health System, South KoreaKai Sen Tan, National University of Singapore, Singapore
Copyright © 2020 O'Sullivan, Mitchel, Das, Koehler, Levine, Bi, Nagel and Park. This is an open-access article distributed under the terms of the Creative Commons Attribution License (CC BY). The use, distribution or reproduction in other forums is permitted, provided the original author(s) and the copyright owner(s) are credited and that the original publication in this journal is cited, in accordance with accepted academic practice. No use, distribution or reproduction is permitted which does not comply with these terms.
*Correspondence: Jin-Ah Park, anBhcmtAaHNwaC5oYXJ2YXJkLmVkdQ==