- 1Department of Pharmacology, University of Oxford, Oxford, United Kingdom
- 2Department of Pharmaceutical Sciences, College of Pharmacy, King Saud Bin Abdulaziz University for Health Sciences, Riyadh, Saudi Arabia
Ca2+ homeostasis is dysregulated in cancer cells and affects processes such as tumorigenesis, angiogenesis, autophagy, progression, and metastasis. Emerging evidence has suggested that endolysosomal cation channels sustain several cancer hallmarks involving proliferation, metastasis, and angiogenesis. Here, we investigate the role of TPC1-2, TRPML1-3, and P2×4 in cancer, with a particular focus on the role of TPC2 in cancer development, melanoma, and other cancer types as well as its endogenous and exogenous modulators. It has become evident that TPC2 plays a role in cancer; however, the precise mechanisms underlying its exact role remain elusive. TPC2 is a potential candidate for cancer biomarkers and a druggable target for future cancer therapy.
Introduction
Cancer is a major public health problem and is one of the leading causes of death worldwide. It is a disease associated with abnormal tissue growth that involves dysregulation of fundamental cellular processes such as apoptosis, proliferation, differentiation, autophagy, and migration. According to Cancer Research United Kingdom, someone in the United Kingdom dies from cancer every four minutes (Cancer Statistics for the UK, 2018). The treatment options for cancer are surgery, radiotherapy, chemotherapy, immunotherapy, targeted therapy, hormone therapy, and stem cell transplants. Significant advances have been made in the treatment of both solid and hematologic malignancies, thus giving many patients the hope of a cure. However, current therapies are associated with severe side effects and a low response rate. Therefore, new tumor hallmarks and mechanisms of tumorigenesis must be identified to develop new treatment strategies.
Calcium is a ubiquitous secondary messenger, and intracellular calcium ions (Ca2+) play a significant role in various physiological processes. They act as regulators of gene transcription and cell proliferation, differentiation, migration, and death (Cui et al., 2017). Alterations of Ca2+ signaling via changes in the expression or post-translational modification of Ca2+ transporters and Ca2+ binding proteins play a role in cancer development from tumor initiation to migration as well as in chemotherapy resistance (Xu et al., 2018). Accumulated data have shown that Ca2+ homeostasis is dysregulated in cancer cells and has an impact on processes such as tumorigenesis, angiogenesis, autophagy, progression, and metastasis (Cui et al., 2017). Therefore, the regulation of spatiotemporal Ca2+ signals in the form of waves or oscillations has emerged as a topic of research relevant to the diagnosis and treatment of cancer. Ironically, the ubiquity of Ca2+ signaling pathways in normal cellular functions makes it challenging to specifically target the molecular components of Ca2+ signaling pathways, as they are also fundamental for normal vital physiological processes. One relevant strategy in this respect would be to target a specific intracellular Ca2+ store. The endolysosomal system has recently become recognized as an important new type of intracellular Ca2+ store (Morgan et al., 2011). The role of lysosomal Ca2+ has been demonstrated in cell growth and cancer (Parrington et al., 2015). The major endolysosomal Ca2+ channels are two-pore channels (TPCs), transient receptor potential cation channels, mucolipin subfamily (TRPML), and P2×4 adenosine triphosphate (ATP)-activated cation channels.
Two-pore channel 2 (TPC2 or TPCN2) is a eukaryotic ion channel located in the lysosome. The release of Ca2+ from this channel is regulated by the second messengers nicotinic acid adenine dinucleotide phosphate (NAADP) and phosphatidylinositol 3,5-bisphosphate (PI(3,5)P2). However, much remains to be established about how TPC2 interacts with NAADP. Additionally, Jha et al. (2014) have discovered novel regulators of TPC2: Mg2+ and the mitogen activated protein kinases (MAPKs) c-jun N-terminal kinase (JNK) and P38. Importantly, it has been found that the mechanistic target of rapamycin complex 1 (m-TORC1) controls lysosomal Ca2+ release via TPC2 (Ogunbayo et al., 2018). Recently, a growing body of literature has identified potential links between TPC2 and cancer. The cellular mechanisms underlying the regulation of Ca2+ signaling via TPC2 in different cancer cells and pathophysiological processes have not yet been deeply investigated, and research in this area is still in its infancy. In this article, we review the role of endolysosomal cation channels in cancer, particularly the association between TPC2 and key pathophysiological processes involved in cancer development, to uncover the roles of TPC2 in different stages of cancer from tumor initiation to tumor cell migration as well as its potential as a novel hallmark of tumors and a druggable target for future cancer therapy.
Endolysosomal Ca2+ Channels and Cancer
The endolysosomal system involves lysosomes; early, late, and recycling endosomes; and autophagosomes. Cation channels located in the endolysosomal system consist of TPCs, TRPML, and ATP-regulated P2×4 channels. A high Ca2+ concentration of ∼600 μM is found in the lysosomal lumen (Lloyd-Evans et al., 2008). Several studies have highlighted the difference between the functions of lysosomes in cancer cells compared to those in normal cells (Hämälistö and Jäättelä, 2016). The lysosome-cancer link has been established in different fundamental processes involving tumor progression and metastasis of cancer and chemotherapy resistance (Fehrenbacher and Jäättelä, 2005; Dozynkiewicz et al., 2012; Zhitomirsky and Assaraf, 2016).
Transient receptor potential cation channels include TRPML1, TRPML2, and TRPML3, all of which are located in the endolysosomal system. TRPML-mediated Ca2+ signaling plays a role in vesicular trafficking events involved in autophagy and lysosomal exocytosis (Li et al., 2018). Phosphatidylinositol 3,5-bisphosphate (PI(3,5)P2) activated lysosomal Ca2+ releases from TRPML in a pH-dependent manner for TRPML1 and TRPML3 (Xu et al., 2007; Li et al., 2017; Zhou et al., 2017). Besides, (PI(3,5)P2) reactive oxygen species (ROS) triggers Ca2+ signals via TRPML1; both are known as endogenous agonists (Li et al., 2018). The importance of targeting TRPML channels has led to the development of several synthetic modulators such as mucolipin synthetic agonist 1 (ML-SA1), MK6-83, TRPML synthetic inhibitor (ML-SI), and ML-SI3 which pharmacologically modulates TRPML channels, whereas ML2-SA1, SN-2, SF-21, and SF-22 activate TRPML2, TRPML3, TRPML2 and TRPML3, and TRPML1 and TRPML3, respectively (Grimm et al., 2010, 2014a; Chen et al., 2014; Li et al., 2018; Plesch et al., 2018; Zhang et al., 2019).
TRPML1 acts as a regulator of mTOR and TEFB signaling (Li et al., 2016). Ca2+ signaling through TRPML1 modulates mTORC1 activity, TFEB localization, and autophagosome-lysosome fusion to control autophagy (Di Paola et al., 2018). The dysregulation of autophagy is associated with carcinogenesis and chemoresistance (Owusu-Brackett et al., 2019). mTOR is involved in PI3K/AKT/mTOR signaling, which plays a crucial role in the pathogenesis of cancer (Li et al., 2016). A recent study illustrated that the dysregulation of TEFB expression or activity is correlated with pancreatic cell proliferation (Marchand et al., 2015). TFEB acts as a positive regulator of post-ischemic angiogenesis via AMPKα signaling and autophagy in endothelial cells. Furthermore, TFEB has been found to upregulate TRPML1 expression at the mRNA level and to bind to its promoter (Fan et al., 2018). According to these data, we can infer that mucolipin-1 (MCOLN1) might act as a mediator of tumor angiogenesis. MCOLN1 is upregulated in triple-negative breast cancer cell lines, and it mediates tumor development via the mTORC1 pathway and lysosomal ATP release (Xu et al., 2019). TRPML-1 modulation either by genetic downregulation or by pharmacological inhibition using ML-S11 was shown to hamper tumor growth and impair cell migration and invasion (Xu et al., 2019). Similarly, MCOLN1 is upregulated in HRAS-expressing cancer cells and plays a role in HRAS-positive-tumor proliferation. Low expression of TRPML-1 was correlated with significantly enhanced survival in HRAS mutant patients with bladder urothelial carcinoma or head and neck squamous cell carcinoma (Jung et al., 2019). A strong association has been shown between loss of/reduced TRPML-1 mRNA-expression and poor survival, revealing the potential application of MCOLN1 as a prognostic biomarker in glioblastoma patients (Morelli et al., 2019). TRPML2 overexpression promotes glioma cell proliferation (Morelli et al., 2016). TRPML3 is involved in biological processes including trafficking, autophagy, and endocytosis (Grimm et al., 2017). TRPML-mediated lysosomal Ca2+ release sustains several cancer hallmarks, including proliferation and autophagy. The protumorigenic function of TRPML warrants further investigation and is a candidate for a novel therapeutic approach to intercepting the pathological process related to cancer development.
Adenosine triphosphate-gated P2×4 cation channels are located in the late endosome, lysosome, and plasma membranes (Li et al., 2018). Luminal ATP and lysosomal pH modulate Ca2+ release from P2×4 (Li et al., 2018). P2×4-mediated lysosomal Ca2+ release leads to lysosomal membrane fusion in a calmodulin-dependent manner (Yang et al., 2019). Accumulated data show that P2×4 plays a principal role in cancer-associated pain, suggesting P2×4 modulation as a potential strategy for developing new treatments to alleviate cancer-related pain (Franceschini and Adinolfi, 2014; Yan et al., 2019).
Two-pore channels, including TPC1 and TPC2, are ubiquitously expressed in the endolysosomal system of mammalian cells. NAADP and phosphatidylinositol 3,5-bisphosphate (PI(3,5)P2) mediate the release of lysosomal Ca2+ (Li et al., 2018). TPCs modulate membrane trafficking, which plays a role in several physiological processes involving endocytosis, exocytosis, autophagy, and viral infection (Li et al., 2018). Recent evidence has linked TPCs to cancer development and progression, suggesting that TPCs are druggable targets that can interfere with tumorigenesis, angiogenesis, and metastasis (Brailoiu et al., 2009; Favia et al., 2014; Jha et al., 2014; Pafumi et al., 2017; Sterea et al., 2018). Other findings have highlighted the link between TPC1 expression dysregulation and tumorigenicity, demonstrating that TPC1 transcripts were approximately three to eightfold higher than TPC2 ones in the SKBR3 human breast cancer cell line (Brailoiu et al., 2009). Another recent study showed that NAADP-mediated TPC1 lysosomal calcium release triggers ERK and the PI3K/AKT signaling pathways, thereby promoting the proliferation of metastatic colorectal cancer (mCRC) cells. In this regard, TPC1 might be a suitable biological target that may reduce cancer growth in mCRC patients, warranting further investigation in vivo (Faris et al., 2019). Figure 1 summarizes the likely roles of TPC2, from tumor initiation to tumor cell migration.
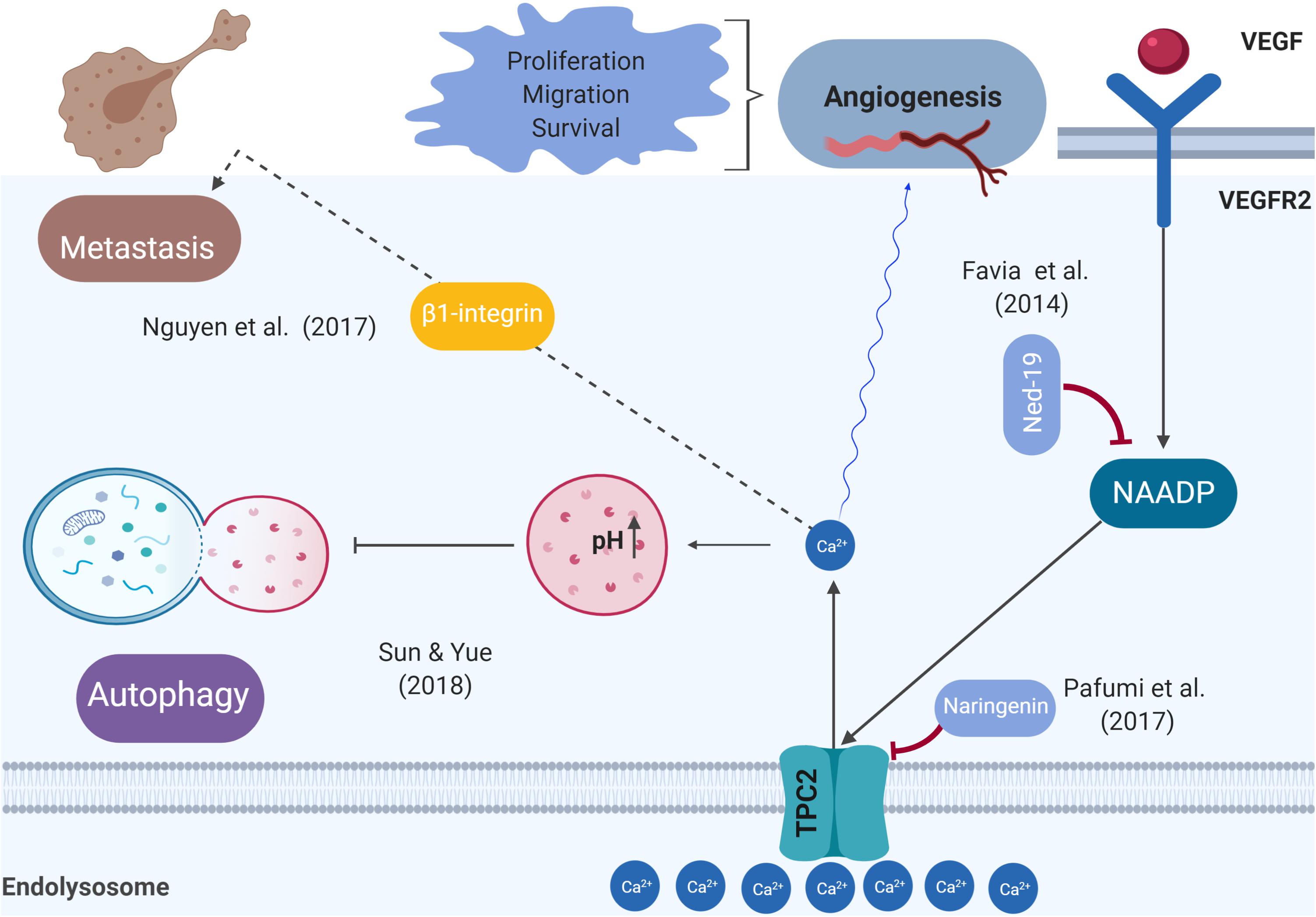
Figure 1. Schematic representation of the role of TPC2 in the pathophysiological processes related to cancer. Previous studies demonstrated that the VEGFR2/NAADP/TPC2/Ca2+ signaling pathway is critical for VEGF-induced angiogenesis in vitro and in vivo.
TPC2, Tumorigenesis, and Tumor Progression Involving Angiogenesis
Ca2+ signaling pathways are emerging as playing a key role in tumor initiation, involving increased proliferation and apoptosis resistance, two of cancer’s hallmarks (Xu et al., 2018). Alterations of Ca2+ homeostasis may behave more as a driver than as a passenger in tumorigenesis (Cui et al., 2017). TPC2 is found on chromosome 11, region 13.2 (TPCN2, NCBI Gene ID: 219931) (TPCN2 two pore segment channel 2 [Homo sapiens (human)], 2018). Data from several sources have shown that 11q13-q14 amplifications are associated with cancer (Wilkerson and Reis-Filho, 2012). This finding is consistent with that of Huang et al. (2018), who observed that TPC2 is overexpressed in oral squamous cell carcinoma cell lines (Huang et al., 2018). These findings raise intriguing questions regarding the roles of TPC2 and a number of other nearby genes as drivers of oncogenesis. Recently, Wei Sun et al. drew attention to the role of TPC2 in cancer cell proliferation. They showed that TPC2 knockdown cells proliferated more slowly than the control or TPC2 overexpressing cells (Sun and Yue, 2018). It is therefore likely that such connections exist between TPC2 and tumor growth. Further research should be undertaken to investigate the possibility that TPC2 acts as a driver to enable a cell to reach the tumorigenic state and as a promoter of tumor growth. Many unanswered questions remain about how precisely TPC2 regulates tumorigenesis and tumor progression at the molecular level. Angiogenesis is a process crucial for cancer progression and a key step in the transition of a tumor’s state from benign to malignant, whereby endothelial cells give rise to new blood vessels. Tumor cells release vascular endothelial growth factor (VEGF) that triggers signal transduction to facilitate Ca2+ signaling, leading to endothelial cell proliferation (Fehrenbacher and Jäättelä, 2005). A previous study demonstrated that the VEGFR2/NAADP/TPC2/Ca2+ signaling pathway is critical for VEGF-induced angiogenesis in vitro and in vivo (Favia et al., 2014). In vivo vascularization meditated by VEGF was abolished by Ned-19 (a TPC2 antagonist) and also in TPC2 knockout mice (Favia et al., 2014). A recent study revealed that naringenin inhibits VEGF-induced angiogenesis via TPC2 (Pafumi et al., 2017). An implication of this is the possibility that targeting TPC2 to develop anti-angiogenics is a strategy for cancer treatment.
TPC2 and Autophagy in Cancer
Autophagy captures, degrades, and recycles proteins and organelles in lysosomes to maintain metabolism and cellular homeostasis (Santana-Codina et al., 2017). Autophagy plays a dual role in cancer: it constrains tumorigenesis in normal tissue and promotes tumorigenesis in cancer tissue by overcoming microenvironmental stresses and conferring resistance to chemotherapy (Mathew et al., 2007). Previous studies have shown that intracellular Ca2+ signaling regulates both basal and induced autophagy (Kondratskyi, 2013). A much-debated question is whether TPC2 promotes or hampers autophagy at an early or late stage of the autophagic process (Pereira et al., 2011; Kayala et al., 2012; García-Rúa et al., 2016; Bootman et al., 2018). Starvation was shown to increase autophagy flux and to exacerbate autophagosome accumulation in response to colchicine (a microtubule inhibitor) in the skeletal muscle of TPC2 knockout mice (Lin et al., 2015). In contrast, a recent study investigating the role of TPC2 in the autophagy of cancer cells reported that overexpression of TPC2 in the Hela human cervical cancer cell line and in a 4T1 mouse breast cancer cell line diminished autophagosomal–lysosomal fusion, resulting in the accumulation of microtubule-associated protein light chain 3 (LC3)-II and syntaxin 17 (STX17)-positive autophagosomes (Sun and Yue, 2018). Overall, these findings indicate that TPC2 mediates autophagy. Therefore, the pharmacological modulation of TPC2 to inhibit autophagy is an attractive therapeutic strategy for enhancing cancer therapy, particularly in advanced stages, because there is a lack of drugs that diminish autophagy and exhibit selective antitumor effects. However, a better understanding of the molecular mechanisms underlying the TPC2 modulation of autophagy and the identification of related, novel, and specific downstream targets for drugs require further investigation.
TPC2, Metastasis, and Cancer Invasion
Metastatic cancer causes the majority of cancer-related mortalities, accounting for ∼90% of such deaths (Chaffer and Weinberg, 2011). Tumor metastases occur only after several stages: the key steps are the loss of cell-cell connections, transformation of primary cancer cells into migratory mesenchymal cells, and invasion of tumor cells (Xu et al., 2018). Chaffer and Weinberg (2011) discussed metastasis and summarized it as a two-phase process. The first phase involves the ability of cancer cells to physically translocate to the “site of dissemination,” and the second phase is represented by the primary tumor cells gaining the ability to colonize secondary sites and develop metastatic lesions (Chaffer and Weinberg, 2011). An in vitro model using siRNA to silence TPC1 or TPC2 and pharmacologic inhibition of TPC activity with Ned-19 or tetrandrine to investigate the role of TPCs in cancer migration indicated that the adhesion and formation of leading edges as well as migration of cancer cells were reduced (Nguyen et al., 2017). When TPC2 function was impaired by siRNA, Ned-19, or tetrandrine, this minimized the formation of lung metastases in an in vivo mouse model of mammary cancer cells (Nguyen et al., 2017). These results highlight the requirement for TPC1 and TPC2 in two critical steps of metastasis formation: adhesion and migration. A possible explanation for these results may be that the disruption of TPC function is hindered by the trafficking of β1-integrin, which is a protein that is prominently involved in tumor migration. Its promigratory trafficking occurs via the endolysosomal system, leading to its accumulation in endocytic vesicles (Nguyen et al., 2017). Consistent with the literature, disturbances in Ca2+ homeostasis alter trafficking and lead to the fusion of endocytic vesicles (Ruas et al., 2010; Nguyen et al., 2017). Similarly, endolysosomal Ca2+ signaling plays a role in the migration of neural crest cells (Patel, 2018). To develop a full picture of the role of TPC2 in regulating metastasis, additional studies of the in vivo TPC2 knockout mouse model are needed. Targeting integrins or the downstream protein(s) that interacts with TPC2 may be a promising strategy for treating metastatic carcinomas.
TPC2 in Melanoma
Melanoma is a type of skin cancer that emerges from pigmented melanocytes (Regad, 2013). Melanosomes are lysosomal organelles that synthesize melanin. Alterations in melanin synthesis increase a person’s risk of developing skin cancer (Bellono et al., 2016). Many studies have begun to provide links between TPC2, cellular pigmentation, and melanoma. Lipid phosphatidylinositol bisphosphate (PI(3,5)P2) mediates TPC2 activity, which acts as a regulator of pigmentation, by controlling melanosomal pH and membrane potential (Bellono et al., 2016). In Xenopus oocytes, TPC2 overexpression evoked pigmentation defects, and its interactivity with Rab GTPases underpinned NAADP-evoked Ca2+ signals (Lin-Moshier et al., 2014). Polymorphisms in the TPC2N gene were identified (rs35264875 and rs3829241) and were associated with a shift from brown to blond hair (Sulem et al., 2008). These two non-synonymous genetic variants were functionally characterized by endolysosomal patch-clamp techniques; this revealed that they were associated with a gain of TPC2 function by independent mechanisms (Chao et al., 2017). Therefore, it seems that TPC2 overexpression generally induced a decrease in melanin production and increased susceptibility to skin cancer.
Despite these promising results, further work is required to confirm the link between TPC2 and melanoma in mouse models and to reveal molecular mechanisms. However, the above findings suggest that TPC2 might represent a viable therapeutic target for the treatment of skin cancer.
TPC2 in Other Cancer Types
In contrast to previous findings that had identified TPC2 overexpression as a potential risk factor for skin cancer, TPCN2 expression was found to be significantly associated with increased survival in bladder cancer (P-value = 3.56E–02). Further investigation is needed to investigate its clinical utility as a potential prognostic marker for bladder cancer (Shivakumar et al., 2017). Two TPCs (TPC1 and TPC2) were expressed in all malignant and benign breast cancer lines. Therefore, they are a feature of all breast cancer subtypes and not specific to one such subtype (Jahidin et al., 2016). The knockdown of TPC2 (although not of TPC1) significantly diminishes epidermal growth factor-induced vimentin expression in MDA-MB-468, a triple-negative breast cancer cell line. TPCN2 is reported to be one of the six gene signatures correlated with prostate cancer to predict postoperative biochemical recurrence (Li et al., 2019). The exploration of the links between TPC2 and different types of cancer requires further work to identify any significant genetic variants associated with such cancers. It would then be interesting to develop in vitro and in vivo models to examine the role of these TPCN2 mutations during tumor formation (involving neoangiogenesis) and cancer-cell migration as well as their clinical utility as diagnostic or prognostic biomarkers in different cancers.
TPC2 Endogenous Agonists, Endogenous and Exogenous Inhibitors, and Interactomes
TPC2 endogenous agonists include (PI(3,5)P2) and NAADP, whereas torin-2 is an exogenous stimulator that mediates lysosomal Ca2 + release via mTOR inhibition. The endogenous inhibitors are cytoplasmic ATP, mTOR, and Mg2+, whereas the synthetic inhibitors are Ned-19, naringenin, and tetrandrine (Pafumi et al., 2017; Li et al., 2018). A recent study was conducted for repurposing the screens of ∼1500 FDA-approved drugs, and it identified fluphenazine and raloxifene as TPC2 blockers (Penny et al., 2019). HCLS1-associated protein X-1 (Hax1), a negative regulator of autophagy and apoptosis, has been physically identified as a TPC2-interactor (Lam et al., 2013). Despite the importance of TPC2 interactomes, there remains a paucity of validated evidence about the proteins that interact with TPC2 to regulate its activity in different physiological processes. Annexin A (ANXA) 1-7, Ras-related protein Rab-7, and Ankyrin repeat domain-containing protein 27 (ANKRD27) are potential candidates for mediators of TPC2 function (Lin-Moshier et al., 2014; Krogsaeter et al., 2018). A recent review summarized how the identified TPC2 SNARE-interactomes mediate membrane fusion or vesical fusion (Krogsaeter et al., 2018). Soluble N-ethylmaleimide-sensitive factor attachment protein receptor (SNARE) proteins play a role in vesicle trafficking and membrane fusion (Han et al., 2017; Wang et al., 2017). TPC2 interacts with Q-SNARE proteins, involving syntaxin 6 (STX6), syntaxin 7 (STX7), syntaxin 12 (STX12), syntaxin 16 (STX16), syntaxin 18 (STX18), VTI1B, and SNAP23 (Grimm et al., 2014b; Lin-Moshier et al., 2014). VAMP2 and VAMP3 are identified as R-SNARE-TPC2 interactors (Huttlin et al., 2017; Krogsaeter et al., 2018). Further research to reveal the complex interplay of SNARE-TPC2 interactions is strongly recommended. Moreover, investigating the interaction between these candidates and TPC2 and then validating it by co-immunoprecipitation and other approaches would be a fruitful and novel area for further research.
Closing Remarks
In summary, with growing evidence linking endolysosomal Ca2+ signaling pathways, particularly TPC2, to cancer, this review reveals the role of such pathways, especially TPC2, in cancer development from tumor initiation to metastasis. The mechanisms that underpin the role of TPC2 in different fundamental processes of cancer and in different types of tumors are not fully understood. TPC2 modulation either by genetic approaches such as siRNA or CRISPR/Cas9, or pharmacologically, is a promising therapeutic target to treat cancer. However, there is a potential for off-target effects arising from the ubiquity of TPC2. Therefore, discovering proteins that specifically interact with TPC2 in cancer tissue instead of targeting TPC2 itself is another approach for drug development that could overcome this effect. Although it has become evident that TPC2 plays a role in cancer, the precise mechanisms underlying the action of TPC2 and its involvement in cancer remain elusive and unclear. Further research in this field would be of great help in expanding our knowledge of TPC2’s action at the molecular level.
Author Contributions
AA has contributed to the mini-review-research question and designing the study, data collection, and interpretation, writing manuscript, designing and creating the figure, and addressing the reviewers’ comments. JP has revised the manuscript.
Conflict of Interest
The authors declare that the research was conducted in the absence of any commercial or financial relationships that could be construed as a potential conflict of interest.
Acknowledgments
AA is a recipient of a Saudi Ministry of Education scholarship. Furthermore, the Saudi Ministry of Education is supporting AA’s graduate studies. The figure was created with BioRender.
References
Bellono, N., Escobar, I., and Oancea, E. (2016). A melanosomal two-pore sodium channel regulates pigmentation. Sci. Rep. 6:26570
Bootman, M. D., Chehab, T., Bultynck, G., Parys, J. B., and Rietdorf, K. (2018). The regulation of autophagy by calcium signals: do we have a consensus? Cell Calcium 70, 32–46. doi: 10.1016/j.ceca.2017.08.005
Brailoiu, E., Churamani, D., Cai, X., Schrlau, M. G., Brailoiu, G. C., Gao, X., et al. (2009). Essential requirement for two-pore channel 1 in NAADP-mediated calcium signaling. J. Cell Biol. 186, 201–209. doi: 10.1083/jcb.200904073
Cancer Statistics for the UK (2018). Cancer Research UK. 2018. Available at: https://www.cancerresearchuk.org/health-professional/cancer-statistics-for-the-uk (accessed December 14, 2018).
Chaffer, C., and Weinberg, R. (2011). A perspective on cancer cell metastasis. Science 331, 1559–1564. doi: 10.1126/science.1203543
Chao, Y., Schludi, V., Chen, C., Butz, E., Nguyen, O., Müller, M., et al. (2017). TPC2 polymorphisms associated with a hair pigmentation phenotype in humans result in gain of channel function by independent mechanisms. Proc. Natl. Acad. Sci. U.S.A. 114, E8595–E8602. doi: 10.1073/pnas.1705739114
Chen, C. C., Keller, M., Hess, M., Schiffmann, R., Urban, N., Wolfgardt, A., et al. (2014). A small molecule restores function to TRPML1 mutant isoforms responsible for mucolipidosis type IV. Nat. Commun. 14:4681. doi: 10.1038/ncomms5681
Cui, C., Merritt, R., Fu, L., and Pan, Z. (2017). Targeting calcium signaling in cancer therapy. Acta Pharm. Sin. B 7, 3–17 doi: 10.1016/j.apsb.2016.11.001
Di Paola, S., Scotto-Rosato, A., and Medina, D. L. (2018). TRPML1: the Ca (2+) retaker of the lysosome. Cell Calcium 69, 112–121. doi: 10.1016/j.ceca.2017.06.006
Dozynkiewicz, M. A., Jamieson, N. B., Macpherson, I., Grindlay, J., van den Berghe, P. V., von Thun, A., et al. (2012). Rab25 and CLIC3 collaborate to promote integrin recycling from late endosomes/lysosomes and drive cancer progression. Dev. Cell 22, 131–145. doi: 10.1016/j.devcel.2011.11.008
Fan, Y., Lu, H., Liang, W., Garcia-Barrio, MT., Guo, Y., Zhang, J., et al. (2018). Endothelial TFEB (Transcription Factor EB) positively regulates postischemic angiogenesis. Circ. Res. 122, 945–957. doi: 10.1161/CIRCRESAHA.118.312672
Faris, P., Pellavio, G., Ferulli, F., Di Nezza, F., Shekha, M., Lim, D., et al. (2019). Nicotinic acid adenine dinucleotide phosphate (NAADP) induces intracellular Ca2+ release through the two-pore channel TPC1 in metastatic colorectal cancer cells. Cancers 11:E542. doi: 10.3390/cancers11040542
Favia, A., Desideri, M., Gambara, G., D’Alessio, A., Ruas, M., Esposito, B., et al. (2014). VEGF-induced neoangiogenesis is mediated by NAADP and two-pore channel-2-dependent Ca2+ signaling. Proc. Natl. Acad. Sci. U.S.A. 111, E4706–E4715. doi: 10.1073/pnas.1406029111
Fehrenbacher, N., and Jäättelä, M. (2005). Lysosomes as targets for cancer therapy. Cancer Res. 65, 2993–2995. doi: 10.1158/0008-5472.can-05-0476
Franceschini, A., and Adinolfi, E. (2014). P2X receptors: new players in cancer pain. World J. Biol. Chem. 5, 429–436. doi: 10.4331/wjbc.v5.i4.429
García-Rúa, V., Feijóo-Bandín, S., Rodríguez-Penas, D., Mosquera-Leal, A., Abu-Assi, E., Beiras, A., et al. (2016). Endolysosomal two-pore channels regulate autophagy in cardiomyocytes. J. Physiol. 594, 3061–3077. doi: 10.1113/JP271332
Grimm, C., Barthmes, M., and Wahl-Schott, C. (2014a) “TRPML3,” in Mammalian Transient Receptor Potential (TRP) Cation Channels. Handbook of Experimental Pharmacology, eds B. Nilius, and V. Flockerzi. (Springer: Heidelberg).
Grimm, C., Holdt, L. M., Chen, C. C., Hassan, S., Müller, C., Jörs, S., et al. (2014b). High susceptibility to fatty liver disease in two-pore channel 2-deficient mice. Nat. commun. 5:4699. doi: 10.1038/ncomms5699
Grimm, C., Butz, E., Chen, C. C., Wahl-Schott, C., and Biel, M. (2017). From mucolipidosis type IV to ebola: TRPML and two-pore channels at the crossroads of endo-lysosomal trafficking and disease. Cell Calcium 67, 148–155. doi: 10.1016/j.ceca.2017.04.003
Grimm, C., Jörs, S., Saldanha, S. A., Obukhov, A. G., Pan, B., Oshima, K., et al. (2010). Small molecule activators of TRPML3. Chem. Biol. 17, 135–148. doi: 10.1016/j.chembiol.2009.12.016
Hämälistö, S., and Jäättelä, M. (2016). Lysosomes in cancer—living on the edge (of the cell). Curr. Opin. Cell Biol. 39, 69–76. doi: 10.1016/j.ceb.2016.02.009
Han, J., Pluhackova, K., and Böckmann, R. A. (2017). The multifaceted role of SNARE proteins in membrane fusion. Front. Physiol. 8:5. doi: 10.3389/fphys.2017.00005
Huang, X., Godfrey, T., Gooding, W., McCarty, K., and Gollin, S. (2018). Comprehensive genome and transcriptome analysis of the 11q13 amplicon in human oral cancer and synteny to the 7F5 amplicon in murine oral carcinoma. Genes Chromosomes Cancer 45, 1058–1069. doi: 10.1002/gcc.20371
Huttlin, E. L., Bruckner, R. J., Paulo, J. A., Cannon, J. R., Ting, L., and Baltier, K. (2017). Architecture of the human interactome defines protein communities and disease networks. Nature 545, 505–509. doi: 10.1038/nature22366
Jahidin, A., Stewart, T., Thompson, E., Roberts-Thomson, S., and Monteith, G. (2016). Differential effects of two-pore channel protein 1 and 2 silencing in MDA-MB-468 breast cancer cells. Biochem. Biophys. Res. Commun. 477, 731–736. doi: 10.1016/j.bbrc.2016.06.127
Jha, A., Ahuja, M., Patel, S., Brailoiu, E., and Muallem, S. (2014). Convergent regulation of the lysosomal two-pore channel-2 by Mg2+, NAADP, PI(3,5)P2 and multiple protein kinases. EMBO J. 33, 501–511. doi: 10.1002/embj.201387035
Jung, J., Cho, K. J., Naji, A. K., Clemons, K. N., Wong, C. O., Villanueva, M., et al. (2019). HRAS-driven cancer cells are vulnerable to TRPML1 inhibition. EMBO Rep. 20:e46685. doi: 10.15252/embr.201846685
Kayala, K. M., Dickinson, G. D., Minassian, A., Walls, K. C., Green, K. N., and LaFerla, F. M. (2012). Presenilin-null cells have altered two-pore calcium channel expression and lysosomal calcium: implications for lysosomal function. Brain Res. 1489, 8–16. doi: 10.1016/j.brainres.2012.10.036
Kondratskyi, A. (2013). Calcium-permeable ion channels in control of autophagy and cancer. Front. Physiol. 4:272. doi: 10.3389/fphys.2013.00272
Krogsaeter, E. K., Biel, M., Wahl-Schott, C., and Grimm, C. (2018). The protein interaction networks of mucolipins and two-pore channels. Biochim. Biophys. Acta Mol. Cell Res. 1866, 1111–1123 doi: 10.1016/j.bbamcr.2018.10.020
Lam, A. K., Galione, A., Lai, F. A., and Zissimopoulos, S. (2013). Hax-1 identified as a two-pore channel (TPC)-binding protein. FEBS Lett. 587, 3782–3786. doi: 10.1016/j.febslet.2013.10.031
Li, F., Ji, J. P., Xu, Y., and Liu, R. L. (2019). identification a novel set of 6 differential expressed genes in prostate cancer that can potentially predict biochemical recurrence after curative surgery. Clin. Transl. Oncol. 12, 1–9. doi: 10.1007/s12094-018-02029-z
Li, M., Zhang, W. K., Benvin, N. M., Zhou, X., Su, D., Li, H., et al. (2017). Structural basis of dual Ca 2+/pH regulation of the endolysosomal TRPML1 channel. Nat. Struct. Mol. Biol. 24, 205–213. doi: 10.1038/nsmb.3362
Li, P., Gu, M., and Xu, H. (2018). Lysosomal ion channels as decoders of cellular signals. Trends Biochem. Sci. 44, 110–124. doi: 10.1016/j.tibs.2018.10.006
Li, R. J., Xu, J., Fu, C., Zhang, J., Zheng, Y. G., Jia, H., et al. (2016). Regulation of mTORC1 by lysosomal calcium and calmodulin. eLife 27:e19360. doi: 10.7554/eLife.19360
Lin, P. H., Duann, P., Komazaki, S., Park, K. H., Li, H., Sun, M., et al. (2015). Lysosomal two-pore channel subtype 2 (TPC2) regulates skeletal muscle autophagic signaling. J. Biol. Chem. 290, 3377–3389. doi: 10.1074/jbc.M114.608471
Lin-Moshier, Y., Keebler, M. V., Hooper, R., Boulware, M. J., Liu, X., Churamani, D., et al. (2014). The two-pore channel (TPC) interactome unmasks isoform-specific roles for TPCs in endolysosomal morphology and cell pigmentation. Proc. Natl. Acad. Sci. U.S.A. 111, 13087–13092. doi: 10.1073/pnas.1407004111
Lloyd-Evans, E., Morgan, A. J., He, X., Smith, D. A., Elliot-Smith, E., Sillence, D. J., et al. (2008). Niemann–pick disease type C1 is a sphingosine storage disease that causes deregulation of lysosomal calcium. Nat. Med. 14, 1247–1255. doi: 10.1038/nm.1876
Marchand, B., Arsenault, D., Raymond-Fleury, A., Boisvert, F. M., and Boucher, M. J. (2015). Glycogen synthase kinase-3 (GSK3) inhibition induces prosurvival autophagic signals in human pancreatic cancer cells. J. Biol. Chem. 290, 5592–5605. doi: 10.1074/jbc.M114.616714
Mathew, R., Karantza-Wadsworth, V., and White, E. (2007). Role of autophagy in cancer. Nat. Rev. Cancer 7, 961–967.
Morelli, M. B., Amantini, C., Tomassoni, D., Nabissi, M., Arcella, A., and Santoni, G. (2019). Transient receptor potential mucolipin-1 channels in glioblastoma: role in patient’s survival. Cancers 11:E525. doi: 10.3390/cancers11040525
Morelli, M. B., Nabissi, M., Amantini, C., Tomassoni, D., Rossi, F., Cardinali, C., et al. (2016). Overexpression of transient receptor potential mucolipin-2 ion channels in gliomas: role in tumor growth and progression. Oncotarget 7, 43654–43668. doi: 10.18632/oncotarget.9661
Morgan, A. J., Platt, F. M., Lloyd-Evans, E., and Galione, A. (2011). Molecular mechanisms of endolysosomal Ca2+ signalling in health and disease. Biochem. J. 439, 349–374. doi: 10.1042/BJ20110949
Nguyen, O. N., Grimm, C., Schneider, L. S., Chao, Y. K., Atzberger, C., Bartel, K., et al. (2017). Two-pore channel function is crucial for migration of invasive cancer cells. Cancer Res. 77, 1427–1438. doi: 10.1158/0008-5472.CAN-16-0852
Ogunbayo, O., Duan, J., Xiong, J., Wang, Q., Feng, X., Ma, J., et al. (2018). mTORC1 controls lysosomal Ca2+release through the two-pore channel TPC2. Sci. Signal. 11:eaao5775. doi: 10.1126/scisignal.aao5775
Owusu-Brackett, N., Shariati, M., and Meric-Bernstam, F. (2019). Role of PI3K/AKT/mTOR in Cancer Signaling. InPredictive Biomarkers in Oncology Cham: Springer. 263–270.
Pafumi, I., Festa, M., Papacci, F., Lagostena, L., Giunta, C., Gutla, V., et al. (2017). Naringenin impairs two-pore channel 2 activity and inhibits VEGF-induced angiogenesis. Sci. Rep. 7:5121 doi: 10.1038/s41598-017-04974-1
Parrington, J., Lear, P., and Hachem, A. (2015). Calcium signals regulated by NAADP and two-pore channels – their role in development, differentiation and cancer. Int. J. Dev. Biol. 59, 341–355. doi: 10.1387/ijdb.150211jp
Patel, S. (2018). Ins and outs of Ca2+ transport by acidic organelles and cell migration. Commun. Integr. Biol. 11:e1331800. doi: 10.1080/19420889.2017.1331800
Penny, C. J., Vassileva, K., Jha, A., Yuan, Y., Chee, X., Yates, E., et al. (2019). Mining of Ebola virus entry inhibitors identifies approved drugs as two-pore channel pore blockers. Biochim. Biophys. Acta Mol. Cell Res. 1866, 1151–1161 doi: 10.1016/j.bbamcr.2018.10.022
Pereira, G. J., Hirata, H., Fimia, G. M., Do Carmo, L. G., Bincoletto, C., Han, S. W., et al. (2011). Nicotinic acid adenine dinucleotide phosphate (NAADP) regulates autophagy in cultured astrocytes. J. Biol. Chem. 286, 27875–27881. doi: 10.1074/jbc.C110.216580
Plesch, E., Chen, C. C., Butz, E., Rosato, A. S., Krogsaeter, E. K., Yinan, H., et al. (2018). Selective agonist of TRPML2 reveals direct role in chemokine release from innate immune cells. eLife 27:e39720. doi: 10.7554/eLife.39720
Regad, T. (2013). Molecular and cellular pathogenesis of melanoma initiation and progression. Cell Mol. Life Sci. 70, 4055–4065. doi: 10.1007/s00018-013-1324-2
Ruas, M., Rietdorf, K., Arredouani, A., Davis, L. C., Lloyd-Evans, E., Koegel, H., et al. (2010). Purified TPC isoforms form NAADP receptors with distinct roles for Ca(2+) signaling and endolysosomal trafficking. Curr. Biol. 20, 703–709. doi: 10.1016/j.cub.2010.02.049
Santana-Codina, N., Mancias, J. D., and Kimmelman, A. C. (2017). The role of autophagy in cancer. Annu. Rev. Cancer Biol. 6, 19–39.
Shivakumar, M., Lee, Y., Bang, L., Garg, T., Sohn, K., and Kim, D. (2017). Identification of epigenetic interactions between miRNA and DNA methylation associated with gene expression as potential prognostic markers in bladder cancer. BMC Med. Genomics 10:30. doi: 10.1186/s12920-017-0269-y
Sterea, A. M., Almasi, S., and El Hiani, Y. (2018). The hidden potential of lysosomal ion channels: a new era of oncogenes. Cell Calcium 72, 91–103. doi: 10.1016/j.ceca.2018.02.006
Sulem, P., Gudbjartsson, D., Stacey, S., Helgason, A., Rafnar, T., Jakobsdottir, M., et al. (2008). Two newly identified genetic determinants of pigmentation in Europeans. Nat. Genet. 40, 835–837 doi: 10.1038/ng.160
Sun, W., and Yue, J. (2018). TPC2 mediates autophagy progression and extracellular vesicle secretion in cancer cells. Exp. Cell Res. 370, 478–489. doi: 10.1016/j.yexcr.2018.07.013
TPCN2 two pore segment channel 2 [Homo sapiens (human)] (2018). Gene–NCBI. Ncbi.nlm.nih.gov. Available at: https://www.ncbi.nlm.nih.gov/gene/219931 (accessed December 14, 2018).
Wang, T., Li, L., and Hong, W. (2017). SNARE proteins in membrane trafficking. Traffic 18, 767–775. doi: 10.1111/tra.12524
Wilkerson, P., and Reis-Filho, J. (2012). The 11q13-q14 amplicon: clinicopathological correlations and potential drivers. Genes Chromosomes Cancer 52, 333–355. doi: 10.1002/gcc.22037
Xu, M., Seas, A., Kiyani, M., Ji, K., and Bell, H. (2018). A temporal examination of calcium signaling in cancer- from tumorigenesis, to immune evasion, andmetastasis. Cell Biosci. 8:25. doi: 10.1186/s13578-018-0223-5
Xu, H., Delling, M., Li, L., Dong, X., and Clapham, D. E. (2007). Activating mutation in a mucolipin transient receptor potential channel leads to melanocyte loss in varitint–waddler mice. Proc. Natl Acad. Sci. U.S.A. 104, 18321–18326. doi: 10.1073/pnas.0709096104
Xu, M., Almasi, S., Yang, Y., Yan, C., Sterea, A. M., Syeda, A. K., et al. (2019). The lysosomal TRPML1 channel regulates triple negative breast cancer development by promoting mTORC1 and purinergic signaling pathways. Cell Calcium 79, 80–88. doi: 10.1016/j.ceca.2019.02.010
Yan, Y., Liang, Y., Ding, T., and Chu, H. (2019). PI3K/Akt signaling pathway may be involved in MCP-1-induced P2X4R expression in cultured microglia and cancer-induced bone pain rats. Neurosci. Lett. 701, 100–105. doi: 10.1016/j.neulet.2019.02.024
Yang, J., Zhao, Z., Gu, M., Feng, X., and Xu, H. (2019). Release and uptake mechanisms of vesicular Ca 2+ stores. Protein Cell 10, 8–19. doi: 10.1007/s13238-018-0523-x
Zhang, X., Chen, W., Gao, Q., Yang, J., Yan, X., Zhao, H., et al. (2019). Rapamycin directly activates lysosomal mucolipin TRP channels independent of mTOR. PLoS Biol. 17:e3000252. doi: 10.1371/journal.pbio.3000252
Zhitomirsky, B., and Assaraf, Y. G. (2016). Lysosomes as mediators of drug resistance in cancer. Drug Resist. Updat. 24, 23–33. doi: 10.1016/j.drup.2015.11.004
Keywords: endolysosomal, calcium, Ca2+ signals, TPC2, cancer
Citation: Alharbi AF and Parrington J (2019) Endolysosomal Ca2+ Signaling in Cancer: The Role of TPC2, From Tumorigenesis to Metastasis. Front. Cell Dev. Biol. 7:302. doi: 10.3389/fcell.2019.00302
Received: 24 September 2019; Accepted: 08 November 2019;
Published: 04 December 2019.
Edited by:
Jan B. Parys, KU Leuven, BelgiumReviewed by:
Christian Grimm, Ludwig Maximilian University of Munich, GermanyJonathan Marchant, Medical College of Wisconsin, United States
Copyright © 2019 Alharbi and Parrington. This is an open-access article distributed under the terms of the Creative Commons Attribution License (CC BY). The use, distribution or reproduction in other forums is permitted, provided the original author(s) and the copyright owner(s) are credited and that the original publication in this journal is cited, in accordance with accepted academic practice. No use, distribution or reproduction is permitted which does not comply with these terms.
*Correspondence: Abeer F. Alharbi, YWJlZXIuYWxoYXJiaUBwaGFybS5veC5hYy51aw==