- Laboratory of Neurobiology, Nencki Institute of Experimental Biology, Polish Academy of Sciences, Warsaw, Poland
Chemical synapses are specialized interfaces between neurons in the brain that transmit and modulate information, thereby integrating cells into multiplicity of interacting neural circuits. Cell adhesion molecules (CAMs) might form trans-synaptic complexes that are crucial for the appropriate identification of synaptic partners and further for the establishment, properties, and dynamics of synapses. When affected, trans-synaptic adhesion mechanisms play a role in synaptopathies in a variety of neuropsychiatric disorders including epilepsy. This review recapitulates current understanding of trans-synaptic interactions in pathophysiology of interneuronal connections. In particular, we discuss here the possible implications of trans-synaptic adhesion dysfunction for epilepsy.
Introduction
Synaptic connections are important defining features of neurons. They continue to be formed and eliminated throughout the development and postnatal period allowing neurons spreading and processing complex patterns of impulses that underlie cognition. Synapses must maintain and strictly regulate the positioning of two opposite molecular architectures – their receptor and channel specification at the postsynaptic site, and the highly dynamic system of neurotransmitter-containing vesicles at the presynaptic site. Moreover, the alignment of both synaptic constituents must be coordinated in time during junction formation, thus neurotransmitters released from the pre-synaptic membrane could act on receptors located at the post-synaptic site in order to ensure fine flow of information between neurons (Harris and Littleton, 2015).
The biochemical and ultrastructural studies of a synapse suggest that adhesion might be critical for the proper assembly and molecular composition of pre- and postsynaptic specializations (Lučić et al., 2005; Zuber et al., 2005). In fact, electron microscopy revealed that upon biochemical synaptosome (isolated nerve terminal) fractionation, both pre- and postsynaptic constituents remain intact (Evans, 2015). The concept of adhesion-mediated synapse stabilization has been supported by demonstrations that synaptic formation can be facilitated by synaptically localized cell adhesion molecules (CAMs) that cooperate across the synaptic junction (Perez de Arce et al., 2015). Trans-synaptic CAMs govern different aspects of synaptic plasticity. They organize synapse formation, control synapse morphology, and receptor function, as well as are engaged in synaptic elimination (Bhaskar et al., 2010; Siddiqui and Craig, 2011). An aim of this review is to recapitulate current understanding of trans-synaptic interactions in pathophysiology of inter-neuronal connections that underlies epileptogenesis and epilepsy.
The crucial role of trans-synaptic CAMs in shaping synapses is also reflected by the fact that many of these molecules have been linked to synaptopathies in neuropsychiatric disorders, including epilepsy. Epilepsy is a condition that has an impact on more than 60 million people throughout the world (Moshé et al., 2015). The disorder is characterized by the presence of recurrent seizures and such comorbidities as cognitive deficits. The mechanisms that underlie these epileptic abnormalities remain largely unknown (Yuen et al., 2018). Since both seizures and cognition require transfer of information throughout synapses, it is justified to formulate the hypothesis that any dysregulation of trans-synaptic complexes might potentially contribute to development and maintenance of epilepsy and its comorbidities. The experimental evidence to support this notion is growing and gains increasing attention, therefore, another aim of the review is to summarize the studies demonstrating possible role of trans-synaptic adhesion in epilepsy. Importantly, epilepsy and its development named epileptogenesis, provide particularly attractive neuropsychiatric condition to study as they can be effectively investigated using animal models (Lévesque et al., 2016).
At least to some extent, plasticity of interneuronal connections seems to be based on synaptically implemented and strictly controlled enzymatic digestion of trans-synaptic CAMs and their adhesive contacts by extracellular proteases (Ethell and Ethell, 2007; Benson and Huntley, 2012; Shinoe and Goda, 2015; Vafadari et al., 2016). The biological consequences of regulated trans-synaptic CAMs cleavage on physiological and pathological changes remains insufficiently understood. We wish to acknowledge here the role of different extracellular proteases in cleavage of trans-synaptic CAMs and describe their contribution to epileptogenesis and epilepsy.
Pathophysiology of Trans-Synaptic CAMs
Each chemical synapse is composed of a patch of presynaptic cell membrane from one neuron facing a patch of postsynaptic cell membrane from a second neuron. The space between them is called a synaptic cleft. The presynaptic side of the synapse harbors the molecular machinery essential for a turnover of neurotransmitter-containing vesicles. Its role is to initiate synaptic responses. The postsynaptic side of the synapse contains receptors and ion channels and triggers downstream cellular signals. During synaptic activity presynaptic neuron executes the release of neurotransmitter molecules into the synaptic cleft, then their binding to the postsynaptic receptors activates channels and evokes molecular responses in the postsynaptic neuron. Apart from signal transmission a role of synapses is to wire neurons into specific functional circuits. Synaptic connections are very dynamic and as a result of synaptic activity they undergo structural and functional changes – a process that is called synaptic plasticity. Synaptic plasticity involves alterations of molecular components present at a synapse, which leads to changes in synaptic geometry and communication efficacy. The phenomenon of synaptic plasticity is widely involved in synapse formation, maintenance and elimination and underlies learning, memory and regenerative processes (Harris and Littleton, 2015). Understanding of how a complex molecular signature of an appropriate synaptic connection is established, maintained, and modified over time remains a major research challenge.
The trans-synaptic CAMs play particularly important role in regulation of pre- and postsynaptic molecular assembly during diverse steps of synapse lifespan. During contact establishment at the site of future synapse, the trans-synaptic CAMs mediate recognition of partner cells. Then, they ensure functional specification of developing synaptic contact by the recruitment of molecular components to active zones, and postsynaptic densities, which eventually leads to the different types of functional synapses with distinct physiological properties (Washbourne et al., 2004).
Dynamic Reorganization of the Synaptic CAMs by Local Proteolysis
The use of trans-synaptic adhesion term here might be, however, misleading because these molecules are not only static structural elements of adhesion, but also dynamic modulators of continual morphological and functional synaptic plasticity (Holtmaat and Svoboda, 2009; Kasai et al., 2010; Chen et al., 2014; Gjørlund et al., 2017). Trans-synaptic CAMs modulate the synapse structure and function with final effect on efficacy of interneuronal connections in line with Konorski/Hebb theorem (Konorski, 1948; Hebb, 1949; Huber and Menzel, 2004). This suggests that trans-synaptic dimers are natural effective means to facilitate molecular changes in synaptic contacts in response to neuronal activity, which might ensure specific retrograde and anterograde modulation of synaptic signal transmission. Indeed, studies on perisynaptic proteolytic cleavage of CAMs provide the promising insights into the possible mechanisms in this regard. Several, possibly complementary, mechanisms have been proposed, that involve regulated proteolysis of trans-synaptic CAMs, including reduced synaptic stability, production of the truncated signaling ectodomains, and increased concentration of transcription-influencing intracellular fragments (Nagappan-Chettiar et al., 2017).
There are several classes of extracellularly acting proteases that are modulated by synaptic activity and are well established to be engaged in synaptic plasticity (Qian et al., 1993; Lüthi et al., 1997; Lukasiuk et al., 2011; Huntley, 2012). The most extensively studied in the context of trans-synaptic CAMs cleavage are the families of matrix metalloproteinases (MMPs) and ADAM metalloproteinases, both belonging to the class of zinc-dependent proteases (metzincins) (Mott and Werb, 2004; Rivera et al., 2010; Huntley, 2012). Among MMPs, MMP-9 has been particularly intensively investigated in synapse function (Huntley, 2012; Vafadari et al., 2016). One of the first associations between MMP-9 and trans-synaptic machinery emerged upon experimental identification of β-dystroglycan (postsynaptic CAM identified as neurexin partner in the brain) (Sugita et al., 2001; Michaluk et al., 2007; Figure 1).
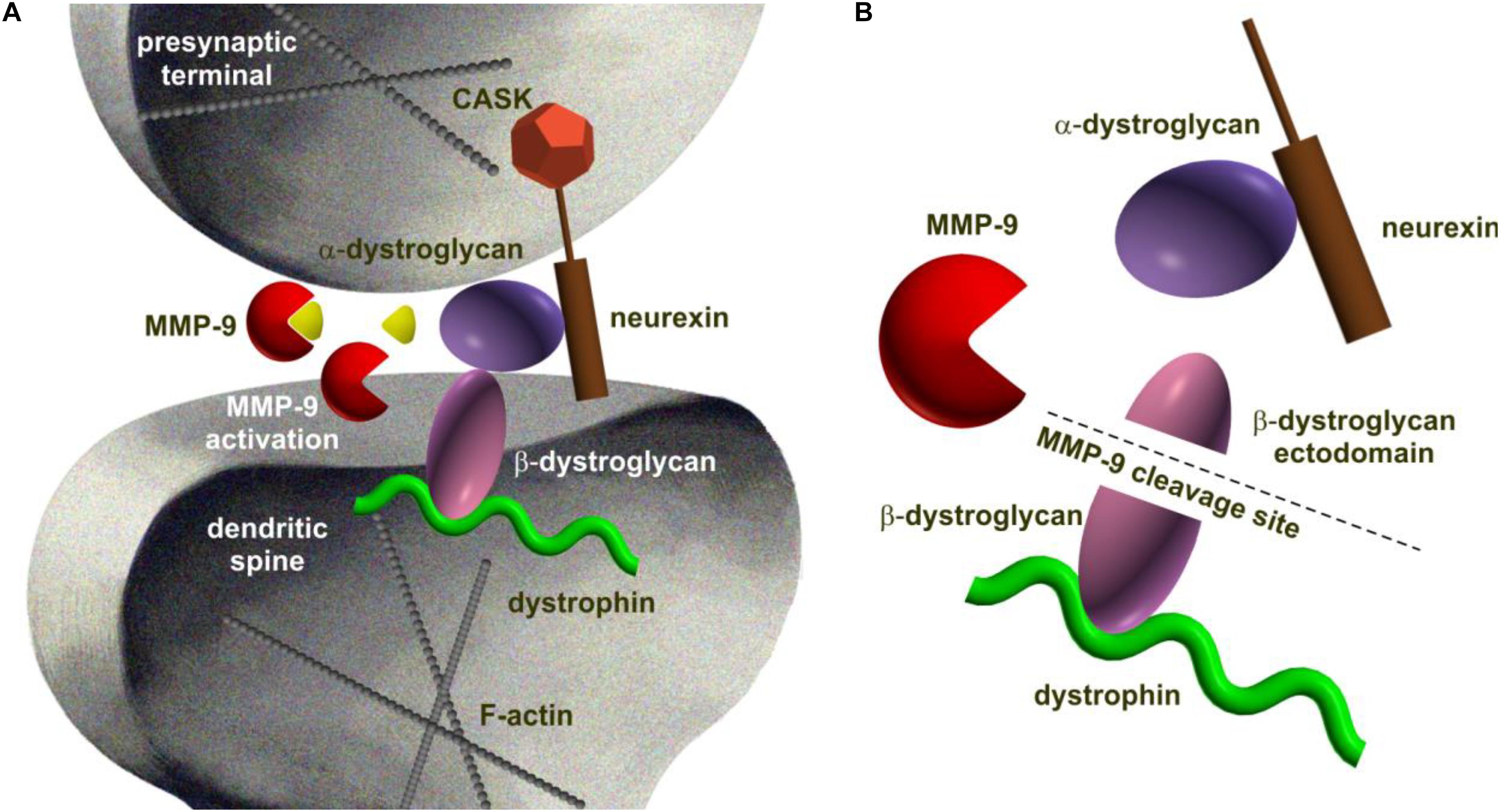
FIGURE 1. Schematic representation of dystroglycan complex being processed by MMP-9 at the synapse as an example of proteolytic cleavage of trans-synaptic interaction. (A) Dystroglycan is an ubiquitous membrane protein associated to dystrophin. It is composed of α- and β-subunits. The α-dystroglycan is a highly glycosylated extracellular component, whereas transmembrane β-dystroglycan forms a bridge between α-subunit and the cytoskeleton. Like neuroligin, dystroglycan binds specifically to neurexins (Sugita et al., 2001). (B) MMP-9-mediated β-dystrogycan proteolysis occurs in response to synaptic activity (Michaluk et al., 2007). MMP-9 catalyzes the cleavage within the β-dystroglycan ectodomain producing a 30 kDa C-terminal fragment and an N-terminal region that is further processed (Bozzi et al., 2015). The consequences of β-dystroglycan cleavage are not known, however, a growing body of evidence suggests a functional role for the entire dystrophin-glycoprotein complex at central synapses and in their plasticity since deletion of this protein is critically associated with attenuated high frequency stimulation-induced long-term potentiation at certain synapses (Satz et al., 2010).
Apart from metaloproteinases, trypsin-type serine proteases are also well-evidenced to be involved in synaptic plasticity, with a particular involvement of neuropsin (KLK8) in modulation of trans-synaptic complexes (Baranes et al., 1998; Tamura et al., 2006).
One can easily notice that any alterations in this complex and proteolytically modulated trans-synaptic engineering might result in serious neuronal dysfunctions in developing or mature synapses. Indeed, scientific investigations on neuronal cultures and in vivo animal models have identified several potentially important trans-synaptic CAMs and their associated proteases and linked them to synaptic dysfunctions. Due to the large number of molecules and considering the fact that experimental evidence supporting the involvement of some direct trans-synaptic interactions and their digestion in synapse function is sometimes compelling, we wish to acknowledge here only the most exemplary ones (summarized in Table 1).
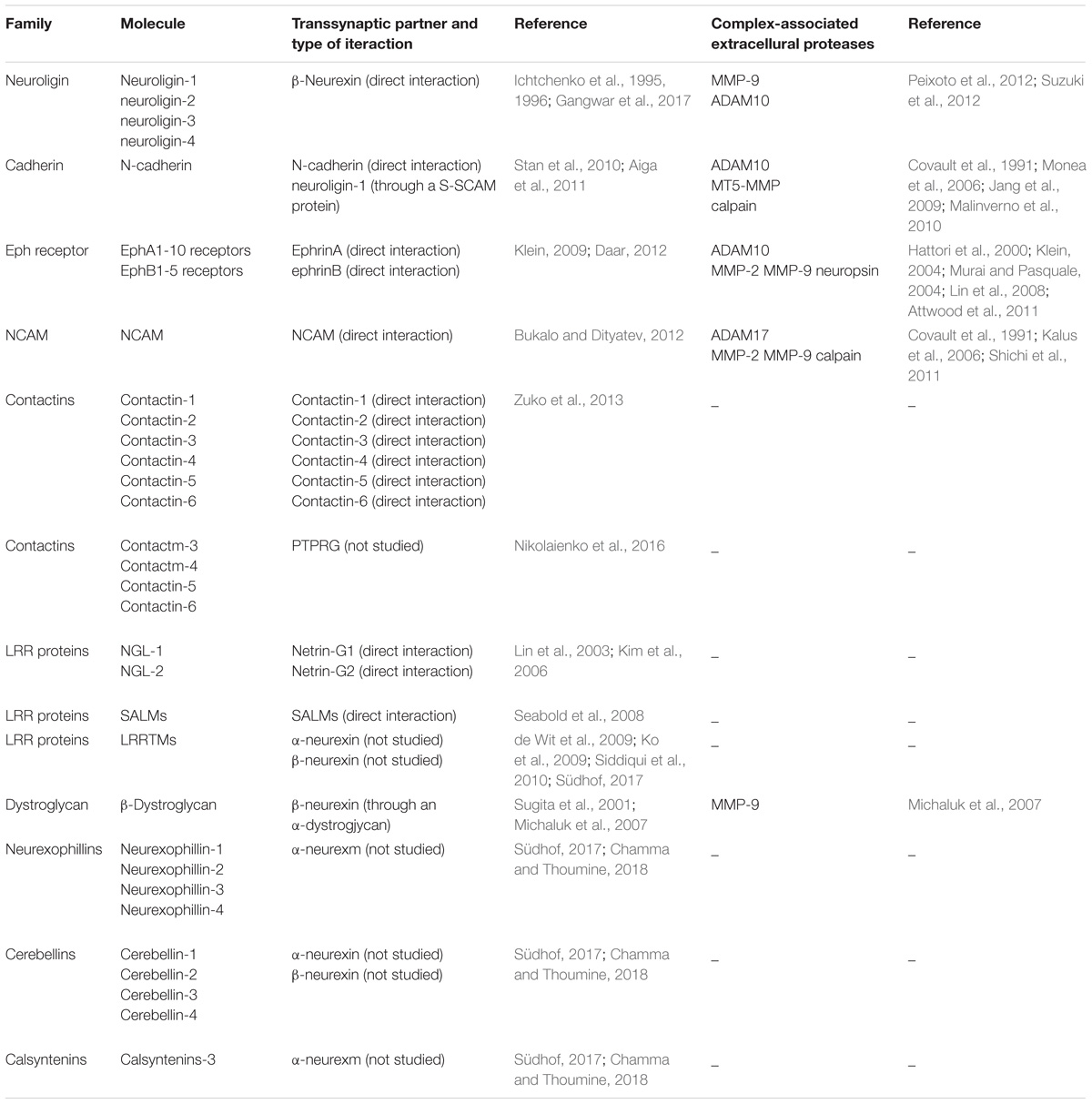
TABLE 1. Trans-synaptic molecules, their partner proteins, and associated proteases involved in synaptic function.
Neuroligins and Neurexins
Trans-synaptic adhesion system is based on either a homo- or a heterophilic interactions between CAMs over the width of synaptic cleft (Yamada and Nelson, 2007). A canonical example of heterophilic interaction is the neuroligin/neurexin complex (Ichtchenko et al., 1995). Neurexins are presynaptic cell surface proteins that are expressed in neurons in plethora of alternatively spliced and regulated isoforms (Krueger et al., 2012). In vertebrates, neurexins genes possess two promoters that independently guide synthesis of α-neurexins and β-neurexins (Missler and Südhof, 1998). For ligand binding, the extracellular tail of α-neurexins contains six LNS (laminin-neurexin-sex hormone binding globulin) domains, as well as three EGF (epidermal growth factor-like) domains. On the other hand, the ectodomain of β-neurexins is composed of unique LNS motif (Reissner et al., 2013). Neuroligins are a family of four type-1 membrane postsynaptic CAMs (named and numbered as neuroligin-1 to neuroligin-4) that contribute to synapse specification. Neuroligins are composed of several domains, including extracellular region, a single transmembrane domain, and cytoplasmic tail. The extracellular region of neuroligins contains a cholinesterase-like domain, responsible for binding to certain splice variants of neurexins through their LNS domain (Lisé and El-Husseini, 2006). The cytoplasmic domains of neuroligins and neurexins contain a four amino acid-long C-terminal motif for binding PDZ domains of scaffolding proteins. Via this motif, neuroligins bind to postsynaptic intracellular scaffolding proteins, including PSD95 and many others (Ichtchenko et al., 1996; Irie et al., 1997; Meyer et al., 2004; Gangwar et al., 2017). The same time the presynaptic differentiation is coordinated through the PDZ-domain-binding motif of β-neurexin which interacts with the CASK (Hata et al., 1996) and Mint (Biederer and Südhof, 2000) proteins at the presynaptic site -both associated with Munc18 protein that is substantial for release of neurotransmitter (Verhage et al., 2000; Figure 2).
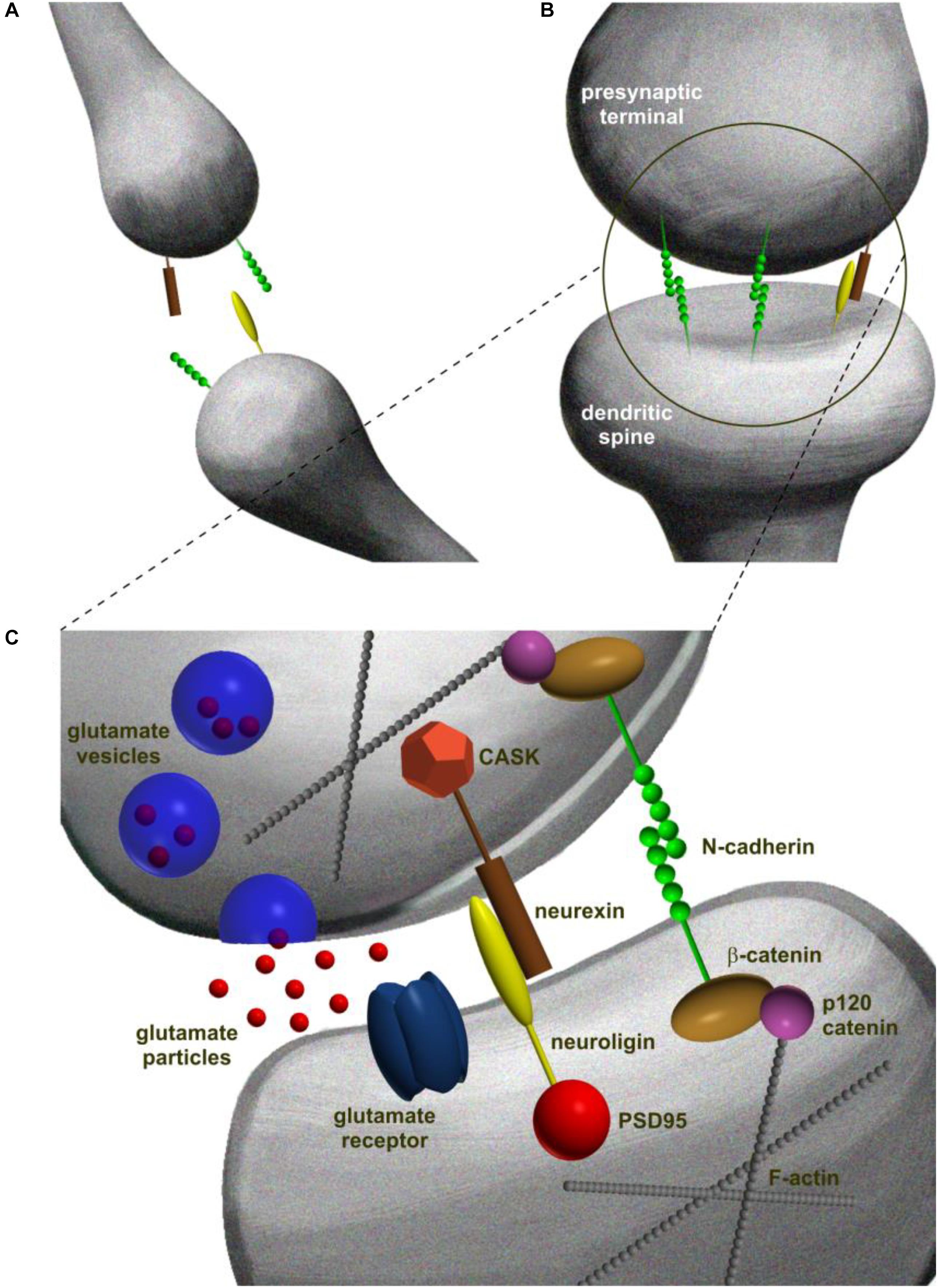
FIGURE 2. Schematic representation of different steps in trans-synaptic complex formation in excitatory synapse development. (A) Synaptogenesis is initiated at the points of contacts between dendritic filopodia and axons. At this stage, synaptic CAMs are distributed evenly at the contact point. (B) Contact point is stabilized by dimerization of the opposing CAM molecules that form trans-synaptic complexes spanning the partner cells and creating mechanical adhesion points. (C) Adhesion points develop into mature synapses that consist of presynaptic terminal with neurotransmitter vesicles and a postsynaptic density characterized by the presence of receptors. The molecular diversity of pre- and postsynaptic constituents is built upon C-terminal interactions of trans-synaptic complexes linking the trans-synaptic dimer to the scaffolding proteins and filamentous actin (McMahon and Díaz, 2011).
As demonstrated by elegant studies on heterogenic cultures, the aforementioned interactions seem to be sufficient for the synaptic arrangement. For instance expression of neuroligins in non-neuronal cells evoked differentiation of presynaptic constituent at the axonal processes of co-cultured neurons. On the other hand, expression of β-neurexin in non-neuronal cells triggered development of postsynaptic specification at the dendrites of neighboring neurons (Gokce and Südhof, 2013). Moreover, elevated expression of neuroligins in neuronal culture increased the number of synapses in respect of synapse type and neuroligin isoform (Chih et al., 2004, 2005; Varoqueaux et al., 2004; Levinson and El-Husseini, 2005). Furthermore, in vivo studies on knockout (KO) animals demonstrated that neuroligins as well as neurexins are crucial for the formation of synaptic contacts and their function (Missler et al., 2003; Varoqueaux et al., 2006). Later on, it was clarified that neuroligins have a significant role in balancing the number of excitatory and inhibitory neuronal contacts. To be precise, Song et al. (1999) showed by in vitro experiments that neuroligin-1 is specific for excitatory while Varoqueaux et al. (2004) demonstrated that neuroligin-2 is specific for inhibitory synapses. On the other hand, neuroligin-3 and -4 seem to be present on both types of synaptic junctions (Budreck and Scheiffele, 2007; Hammer et al., 2015).
Multiple pieces of data on neuroligin/neurexin complex role at synapses come from loss of function studies. For instance, it was shown that knocking out neuroligin-1 in mice attenuates the strength of excitatory synapses, whereas neurons lacking neuroligin-2 are characterized by attenuated efficacy of inhibitory connections. Moreover, the triple KO of neuroligin-1–3 in mice occurred to be neonatally lethal, and considerable synaptic deficits had been reported based on both in vitro and in situ studies of these animals (Varoqueaux et al., 2006). Moreover, silencing neuroligin-1 in mice blocks the storage of fear memory by reducing NMDAR-mediated currents and affects the expression of long-term potentiation (LTP) (Kim et al., 2008). In addition, NMDAR/AMPAR current ratio and LTP were found reduced in neuroligin-1 KO mice (Chubykin et al., 2007). On the other hand, overexpressing neuroligin-1 also leads to the learning and memory deficits and impaired synaptic plasticity (Dahlhaus et al., 2009). Neuroligin-2 overexpressing mice show increased synaptic contact area and enlarged pool of reserve vesicles in synapses, and alterations in the excitation to inhibition ratio which seems to result in anxiety and loss of social interactions (Hines et al., 2008). In addition, neurexins in conjunction with neuroligins also contribute to the synaptic plasticity throughout the activity-dependent molecular pathways (Varoqueaux et al., 2004; Jedlicka et al., 2015). Experimental data show that the extracellular domain of neuroligin-1 can be cleaved by MMP-9 and ADAM10, and the remaining membrane-bound peptide is subsequently processed by γ-secretase. The shedding of neuroligin-1 requires NMDA receptor activity (Peixoto et al., 2012; Suzuki et al., 2012).
Studies have demonstrated that besides neuroligins, neurexins create trans-synaptic interactions also with several other proteins, particularly, neurexophilins, cerebellins, calsyntenins, as well as dystroglycans, and LRRTMs (Südhof, 2017; Chamma and Thoumine, 2018). Briefly, neurexophilins comprise a family of four secreted glycoproteins (neurexophilin-1 to neurexophilin-4) (Missler et al., 1998) that play an essential function in synaptic plasticity (Born et al., 2014; Neupert et al., 2015). They were initially discovered as an α-neurexin partners by affinity chromatography (Petrenko et al., 1996). Cerebellins are a group of secreted glycoproteins that were classified to the C1q and TNF family (Kishore et al., 2004). There are four vertebrate cerebellins family members (named cerebellin-1 to cerebellin-4) that are abundantly expressed in the brain. They critically contribute to the synaptic function and plasticity through the trans-synaptic interactions with presynaptic neurexins and postsynaptic glutamate receptors (Ito-Ishida et al., 2008; Uemura et al., 2010; Matsuda and Yuzaki, 2011; Seigneur and Südhof, 2017). Their important role in synaptic organization has been very recently confirmed with studies on a single, double, and triple constitutive cerrebellin KO mice (Seigneur and Südhof, 2018). Calsyntenins are members of the cadherin superfamily, type I transmembrane proteins containing two cadherin repeats, laminin, neurexin, and LNS domains (Vogt et al., 2001; Araki et al., 2003). Among them, novel synaptic organizer named calsyntenin-3 has been recently reported by Pettem et al. (2013). Calsystenin-3 localizes predominantly postsynaptically (Hintsch et al., 2002) and was characterized by its ability to bind to α- but not β-neurexins (Lu et al., 2014). The protein seems to organize both excitatory and inhibitory synaptic assembly as demonstrated by co-culture assay (Pettem et al., 2013). This action was suppressed once calsyntenin-3 extracellular domain was cleaved (Pettem et al., 2013). Due to broadly documented contribution to trans-synaptic interactions dystroglycans and LRRTMs were described in a separate sections of this review.
Cadherins
A common example of prototypic homophilic interactions is cadherin/cadherin complex. Cadherins are large family of glycosylated transmembrane cell–cell adhesion molecules conserved among multicellular organisms, which play essential roles in tissue morphogenesis (Hulpiau and van Roy, 2011).
N-cadherin (neural cadherin) is one of the most abundant cadherins in synapses. It belongs to the classic cadherins – a single-pass transmembrane proteins containing five extracellular cadherin domains, a transmembrane motif, and a cytoplasmic domain. The protein homophilic adhesion is calcium-dependent (Takeichi, 2007). Molecular determinants of N-cadherin association with the synapse are based on extracellular domain of the protein which binds to the other N-cadherin molecule on juxtaposed cell membrane and highly conserved C-terminus binding β-catenin and p120-catenin (Rubio et al., 2005; Elia et al., 2006; Takeichi, 2007). β-catenin recruits α-catenin (a binding partner of filamentous actin) to the complex and plethora of PDZ domain-containing proteins (Arikkath and Reichardt, 2008). This mesh of interactions is considered to guide structural and functional organization of synaptic assembly linking N- cadherin to cytoskeletal elements (Yamada et al., 2005), ensuring the adhesion of synaptic membranes, organizing the presynaptic vesicle clusters (Bamji et al., 2003; Bozdagi et al., 2004), and postsynaptic membrane machinery (Togashi et al., 2002; Tai et al., 2008) together with the glutamate receptors at the synapse (Schnell et al., 2002; Danielson et al., 2012; Figure 2). The above notions have been supported by many studies on neuronal cultures. For instance, cells lacking either N-cadherin or β-catenin showed multiple synaptic malformations such as more filopodia-like thinner spines with parallel decrease of total spine number (Okuda et al., 2007; Saglietti et al., 2007; Mendez et al., 2010).
Alteration of N-cadherin function induces detectable synaptic deficits that clearly demonstrate structural and functional association of N-cadherin with the processes of synapse stabilization, synaptic transmission and plasticity at both sides of the synapses. For example conditional KO mice are characterized by reduced LTP and impaired spine enlargement as demonstrated by Bozdagi et al. (2010) in the CA1 hippocampal region. The LTP-induced long-term stabilization of synapses was reported to be impaired when N-cadherin is knocked down (Mendez et al., 2010). The lack of N-cadherin markedly diminished short-term facilitation and depression at excitatory synapses (Jungling et al., 2006). Moreover, presynaptic N-cadherin adhesion, when reduced, leads to the altered recycling of vesicles (Togashi et al., 2002). On the other hand, overexpression of the N-terminal domain of N-cadherin seems to be responsible for the increased frequency of miniature excitatory postsynaptic currents (mEPSCs) (Saglietti et al., 2007). Interestingly, cooperation between neuroligin-1 and N-cadherin had also been inspected and reported to be important for the formation of excitatory synapses and control of vesicle dynamics at synapses (Stan et al., 2010; Aiga et al., 2011). N-cadherin extracellular domain can be processed by ADAM10 (Malinverno et al., 2010) or membrane type 5 MMP (MT5-MMP) in an AMPA-dependent manner (Monea et al., 2006). The continuation of that molecular events is the cleavage of N-cadherin intracellular fragment which is processed by γ-secretase in order to release a C-terminal-derived motif that affects CREB dependent transcription (Marambaud et al., 2003).
Eph Receptors and Ephrins
Eph receptors are family of receptor tyrosine kinases, containing an extracellular region with the ephrin ligand-binding domain, a single transmembrane segment, and a cytoplasmic domain containing PDZ-binding motif (Pasquale, 2004, 2005). The family is comprised of 10 EphA and 5 EphB receptors which have been studied in various physiological processes (Kullander and Klein, 2002; Himanen, 2012). EphA and EphB receptors are characterized by the affinity to transmembrane glycosylphosphatidylinositol-anchored proteins ephrinA, and ephrinB, respectively. Eph receptors and ephrins were found at both pre- and postsynaptic sites (Klein, 2009). Resultant Eph receptor/ephrin interaction mediates adhesion and bidirectional communication between the receptor-expressing membrane and the ephrin-expressing partner membrane (Daar, 2012). EphrinA-mediated EphA signaling modulates the synaptic plasticity (Hruska and Dalva, 2012). In particular, the activation of EphA4 downregulates the synaptic GluR1, and attenuates amplitude of mEPSC (Fu et al., 2011). Moreover, the EphA4-deficiency in hippocampal CA1 region was found by Grunwald et al. (2004) to alter maturation of dendritic spines (Murai et al., 2003), to impair LTP as well as long-term depression (LTD). Also EphrinB-mediated EphB signaling seems to be important for synaptic functions. In particular, the EphB1-3 receptors expression reduces the number of glutamergic synapses and the recruitment of NMDARs and AMPARs (Henkemeyer et al., 2003). Also in another study localization of the AMPARs was shown to be modulated by EphB2 (Kayser et al., 2006). As demonstrated in cultured neurons, siRNA-mediated downregulation of EphB2 expression in the postsynaptic neuron reduced mEPSCs frequency at postsynaptic cell (Kayser et al., 2006). In addition, mice deficient in EphB2 are characterized by reduced NMDAR-mediated currents and impaired LTP (Henderson et al., 2001). Later on, it occurred that EphrinB2 is essential for maintaining both LTP and LTD through the C-terminal PDZ interaction (Bouzioukh et al., 2007). In CA1 neurons of ephrinB3-deficient mice, mEPSCs show reduced amplitude. The same time NMDAR/AMPAR ratio is increased in this animal (Antion et al., 2010). In CA3 pyramidal cells extracellular application of soluble ephrinsB reduces mossy fiber LTP (Contractor et al., 2002). Replacement of ephrinB3 intracellular domain with β-galactosidase blocks the LTP at mossy fibers (Armstrong et al., 2006). The Eph receptors/ephrins interactions can be decomposed as a result of proteolytic cleavage of ephrinA2 and ephrinB2 by ADAM10 (Hattori et al., 2000). Moreover, it has been demonstrated in vitro that EphB2 receptor can also be cleaved by MMP-2 and MMP-9 in dissociated hippocampal neuronal cultures (Lin et al., 2008). Finally, the Ephrin/Eph receptor complex was demonstrated to be involved in cognition when cleaved by MMP-9 following induction of hippocampal LTP (Klein, 2004; Murai and Pasquale, 2004). Another study reported neuropsin-mediated cleavage of EphB2 in amygdala which was related to stress-induced synaptic plasticity and anxiety (Attwood et al., 2011).
NCAM
The neural cell adhesion molecule (NCAM) is a glycoprotein which expression was reported at both pre- and postsynaptic constituents. The N-terminal region of NCAM binds to the partner NCAM molecule and contains five Ig domains followed by a region containing two fibronectin type III-like repeats (Kasper et al., 2000). Numerous alternatively spliced variants of NCAM were identified in rat brain (Reyes et al., 1991) and studies have demonstrated that NCAM has an influence on synapse structural and functional maturation through both homo- and heterophilic interactions (Bukalo and Dityatev, 2012). For instance, postsynaptic NCAM forms a complex that is responsible for the recruitment of NMDARs to synapses, and is crucial for NMDAR-dependent forms of plasticity such as LTP and LTD (Müller et al., 1996; Bukalo et al., 2004; Sytnyk et al., 2006). NCAM might be polysialylated. Polysialylation is a posttranslational modification of the NCAM. In the adult brain, polysialylated NCAM (PSA-NCAM) is restricted to regions of neuroplasticity where it affects homophilic NCAM binding and modulates NCAM-mediated adhesion (Rutishauser and Landmesser, 1996; Kiss and Rougon, 1997). The ablation of NCAM results in reduction of the number of synapses (Dityatev et al., 2000). Moreover, suppression of NCAM-mediated adhesion with either synthetic peptides or function-interfering antibodies reduced LTP in CA1 region, along with impaired hippocampal-dependent learning. Hinkle et al. (2006) have revealed that NCAM might be processed by ADAM17, MMP-2, and MMP-9.
Contactins
Contactins are Ig-CAMs that contain six N-terminal Ig-like domains (Buttiglione et al., 1996; Perrin et al., 2001). There are six members of this subfamily that are typically expressed in the nervous system: contactin-1 (contactin/F3), contactin-2 (also named TAG-1), contactin-3, and contactin-4 (BIG-1 and BIG-2, respectively), and contactin-5, contactin-6 (NB-2 and NB-3) (Shimoda and Watanabe, 2009). Contactins function most probably at pre- and postsynaptic sites through predominantly heterophilic interactions with distinct members of contactin family and other trans-synaptic CAMs such as protein-tyrosine phosphatase receptor type G (PTPRG) (Zuko et al., 2013; Nikolaienko et al., 2016), however, complete recognition of their trans-synaptic activities requires detailed studies. Among contactins, contactin-1 has been extensively studied in context of synaptic function. Contactin-1 has been identified at postsynaptic density of CA1 pyramidal cells and its inactivation impairs paired-pulse facilitation and LTD. Synapses deficient in contactin-1 show decreased EPSCs amplitudes upon low-frequency stimulation (Murai et al., 2002). Contactin-4 and 5 were found to be involved at early stages of development in synapse differentiation (Mercati et al., 2013) whereas contactin-6 deficient mice show reduced number of excitatory synapses but not inhibitory ones (Sakurai et al., 2010). Very few if any studies directly address the prospective proteolytic cleavage of contactins at the synapse. Thus further investigation is required to find out their enzymatic partners.
LRR Proteins
Several types of LRR (leucine-rich repeats) proteins were identified at synapses, in particular NGLs (netrin-G ligands), SALMs (synaptic adhesion-like molecules), and LRRTM (leucine rich repeat transmembrane) proteins. The three NGLs expressed in vertebrates (NGL1-3) are type I membrane proteins localized to postsynaptic sites. Their name comes from the NGLs characteristic potential to bind to presynaptic netrins (netrin-G1 and -G2) (Lin et al., 2003; Kim et al., 2006). Crucial for this heterophilic interactions are NGLs extracellular leucine-rich repeats, and their single extracellular Ig-domain. At the intracellular side a short cytoplasmic tail of NGLs is capable of interacting with PSD-95 (Woo et al., 2009). On the other hand netrin-G1 and -G2 isoforms are associated with axonal terminals by a GPI anchor (Nakashiba et al., 2002). Mice lacking netrin-G/NGL trans-synaptic system components develop behavioral deficits (Zhang et al., 2008).
Synapse-organizing functions were also reported for SALMs (Nam et al., 2011). Like NGLs, SALMs are type I membrane proteins. They contain crucial amino-terminal leucine-rich repeats, and a single Ig-domain. The complete role of SALMs in synapses remains unclear, however, it has been reported that at the extracellular side a subset of SALMs form homophilic complexes (Seabold et al., 2008), and they may bind AMPAR and NMDAR receptors or PSD-95 (Ko et al., 2006; Wang et al., 2006).
LRRTMs have also been reported to play a synaptic function. A plausible role of LRRTM proteins in synaptogenesis was first identified bioinformatically (Laurén et al., 2003), and then confirmed experimentally during synapse formation (Linhoff et al., 2009). The mechanisms of LRRTM-based trans-synaptic interactions remain underinvestigated. Studies revealed that LRRTM proteins bind to neurexins in a selective manner (de Wit et al., 2009; Ko et al., 2009; Siddiqui et al., 2010). Deficits of LRRTM proteins do not seem to exert any considerable phenotypes (Linhoff et al., 2009). Nevertheless knockdown of LRRTMs expression in vitro and in vivo is associated with decreased AMPAR trafficking (Ko et al., 2011; Soler-Llavina et al., 2011). There is limited experimental data on LLR protein cleavage at the synapse. Therefore that phenomenon must be further addressed.
Dystroglycan
Dystroglycan is an ubiquitous membrane protein and part of dystrophin-glycoprotein complex. It links the cytoskeleton to the extracellular matrix and stabilizes the dystrophin-glycoprotein complex at the membrane (Figure 1). Dystroglycan is a duplex of α- and β-subunits. The α-dystroglycan is a highly glycosylated extracellular component, whereas β-dystroglycan intermediates in interaction between α-subunit and the cytoskeleton. Dystroglycan, like neuroligin, also binds specifically to a subset of the LNS domains of neurexins (Sugita et al., 2001). The dystroglycan complex has been found to colocalize with GABAA receptors in the CNS and it was demonstrated that the surface expression of GABA receptors increases upon neuronal hyperactivity in a dystroglycan-dependent manner (Lévi et al., 2002; Pribiag et al., 2014).
Trans-Synaptic Cams in Epileptogenesis and Epilepsy in Patients and Animal Models
The epilepsy comprises a very heterogeneous group of neuropsychiatric disorders. They are defined by the occurrence of recurrent seizure episodes (Fisher et al., 2014). In addition to seizures, epilepsy is usually associated with cognitive impairments (Berg and Scheffer, 2011). Epilepsy is also common comorbidity of various mental conditions, such as autism spectrum disorders or schizophrenia. The classification of epilepsies has been recently revised by the International League against Epilepsy (Berg et al., 2010; Fisher et al., 2017).
Comprehensive understanding of these mechanisms cannot be fully acquired without use of animal models. The animal models of epilepsy provide a clinically relevant context for exploring the seizure-related structural and functional changes of neuronal networks during the course of epileptogenesis. Different animal models represent different forms of human epilepsies. Extensively used are models mimicking temporal lobe epilepsy (TLE) the most common form of epilepsy in adults. Rodents with recurrent TLE seizures are typically generated with use of chemoconvulsants such as pilocarpine, or kainate (Leite et al., 2002). Animals treated with the chemoconvulsive drugs display initial seizure insult (status epilepticus) that leads to a latent period and eventually to the occurrence of chronic manifestation of unprovoked tonic-clonic seizures associated with histopathological changes that are characteristic for TLE (Lothman and Bertram, 1993; Mathern et al., 2002). Like status epilepticus-induced TLE, electric kindling may also reproduce chronic epileptogenic features in temporal lobe. Electric kindling is an epileptiform plasticity phenomenon in which repetitive administration of sub-convulsant electrical stimuli in a specific brain region can lead to progressive development of seizures, culminating in generalized seizure activity (McNamara, 1984). Kindling is also often induced chemically by the repeated administration of subconvulsive dose of pentylenetetrazol (PTZ).
Post-traumatic epilepsy (PTE) is one of the leading causes for acquired epilepsies in adults. PTE develops often as a result of traumatic brain injury (TBI) caused by external mechanical force. This initial brain insult triggers cascade of molecular changes that leads to the appearance of spontaneous recurrent seizures (Pitkänen and Lukasiuk, 2011). Considering the high heterogeneity of TBI in humans, TBI animal models are very diverse in order to recapitulate human syndrome as completely as possible (Pitkänen et al., 2009).
Over the last years, many genes with effect on human epilepsy have been reported providing important evidence for heritability of the condition (Ottman et al., 2010). There are several genetic animal models that resemble idiopathic epilepsy in humans. Genetically established mice and rat strains have been created by selective breeding of seizure-susceptible individuals (Frankel, 2009). As a result in these genetic animal models, seizures are either induced by specific sensory stimulation or occur spontaneously (Serikawa et al., 2015).
Distinct origins of the condition and associated seizure types are reflected by the very diverse and complex molecular mechanisms underlying epilepsies. Given the accumulating research evidence based on animal models epilepsy is now clearly recognized also as a synaptopathy. Synaptic alterations contribute to the epilepsy development, i.e., epileptogenesis, recurrent seizure initiation and propagation, and associated cognitive deficits. This notion is further supported by clinical studies suggesting that several of these synaptic mechanisms might involve dysfunctions in trans-synaptic adhesion.
Neuroligins and Neurexins
Since neuroligin/neurexin interaction regulates the proper balance between the number of excitatory and inhibitory synapses, it is not surprising that their alterations are important epileptic hallmarks. For instance, it was shown that neuroligin-1 and its binding partner neurexin-1β are intensively expressed in epileptic foci of human patients with TLE (Fang et al., 2016). Further, the animal studies revealed that neuroligin-1 knockdown prolonged chronic seizure onset and reduced seizure severity in epileptic rats. In addition patch-clamp recordings of whole-cell in brain slices from neuroligin-1 knockdown epileptic rats revealed a decrease frequency of action potentials and miniature postsynaptic currents generation in excitatory cells of hippocampal network. In this neuron the amplitude of NMDAR-dependent excitatory postsynaptic currents were also attenuated and general NMDAR1 subunit expression was found reduced in the hippocampus of epileptic rats (Fang et al., 2016). Moreover genomic microarray test detected the neuroligin-1 deletion in a patient with seizure disorder (Millson et al., 2012) and mutations in neuroligin-4 have been also associated with convulsions and synaptic dysfunctions (Laumonnier et al., 2004). Mutations in neurexin-1, a neuroligin partner were also found in patients with epilepsy. Neurexin-1 deletions result in an early onset epilepsy associated with severe recessive mental retardation (Harrison et al., 2011). Furthermore, a genome-wide study on patients with idiopathic generalized epilepsy had revealed that the risk of syndrome is increased upon exon-disrupting deletions of neurexin-1 (Møller et al., 2013). Studies on animals revealed that the level of neurexin-2 expression was enhanced in the dentate gyrus upon seizures (Górecki et al., 1999).
Cadherins
The involvement of classical cadherins in epileptogenesis and epilepsy has not apparently been examined experimentally. Nevertheless, studies on animal models revealed a potentially pathogenic mechanism related to N-cadherin. N-cadherin expression was reported to be increased in hippocampus up to 1 month following pilocarpine-induced seizure and was found consistent with the induction of mossy fiber sprouting (Shan et al., 2002).
Most of the epilepsy incidents are idiopathic or acquired, however, there is a strong evidence for heritability of the condition. Important non-classic cadherin implicated in genetic cases of epilepsy is protocadherin-19 (PCDH19). Protocadherins possess strong structural similarity to classic cadherins particularly, they contain extracellular motifs that are homologous to those of classic cadherins. The name protocadherin suggest an evolutionary old group of molecules, however, protocadherins have very late origin and for the first time appear in primitive vertebrates (Weiner and Jontes, 2013). The PCDH19 mutations are responsible for female-restricted epilepsy with mental retardation (EFMR) (for a review, see Depienne and LeGuern, 2012) that has been classified as infantile familial or sporadic epilepsy in female patients with or without intellectual disability (Dibbens et al., 2008). Due to its female-limited expression PCDH19 gene escaped epilepsy mapping until systematic sequencing of X-chromosome exons clearly revealed mutations of the PCDH19 gene as the cause of the disorder. PCDH19 alterations affect heterozygous females while hemizygous males are spared (Dibbens et al., 2008). For example, in this context genomic deletions of PCDH19 have been clinically identified in two unrelated girls with seizures (Vincent et al., 2012). Most typically PCDH19-related epilepsy is characterized by early-onset of seizures (6–36 months) followed by recurrent seizures appearing throughout childhood (Scheffer et al., 2008).
The strong association of PCDH19 with epilepsy inheritance is also stressed by genetic screening demonstrating that 11 out of 45 patients suffering from Dravet syndrome (severe myoclonic epilepsy of infancy) carried mutations in PCDH19 (Depienne et al., 2009). Moreover, mutations in PCDH19 have also been identified in many female patients with febrile seizures and in a wide spectrum of other cases (Jamal et al., 2010; Marini et al., 2010; Specchio et al., 2011a,b; Higurashi et al., 2012).
Eph Receptors and Ephrins
The expression pattern and cellular distribution of EphB receptor/ephrinB complex was investigated in patients with intractable TLE and correlated with data obtained from lithium-pilocarpine-treated rats (Huang et al., 2016). In comparison to control groups, ephrinB3 and EphB3 mRNA expressions were significantly up-regulated in patients and rats with chronic TLE seizure. Western blot analysis and semi-quantitative immunohistochemistry showed that ephrinB3 and EphB3 protein level were up-regulated in both patients and affected animals. Similarly, increased expression of EphA10 receptor as well as ephrinA4 was reported upon pilocarpine induced acute seizures, suggesting EphA10, ephrinA4 involvement in epileptogenesis (Xia et al., 2013). In addition to that, EphA5 and ephrin-A3 were reported to delay development of chronic seizures induced with stimulation of performant path fibers (Xu et al., 2003).
NCAM
NCAM-1 concentration in cerebrospinal fluid was found elevated in epilepsy patients in comparison to healthy individuals. Intensive NCAM-1 content was also detected in drug-resistant epilepsy patients when compared to the drug-effective patients (Wang et al., 2012). Therefore, NCAM-1 concentration in cerebrospinal fluid could be considered a biomarker for epilepsy. NCAM association with epilepsy was also confirmed in animal studies. Particularly, it was revealed that NCAM-positive neurons were observed in hippocampus of rats that had experienced repeated kindled generalized seizures (Sato et al., 2003).
To explore the role of polysialylated-NCAM in epilepsy, endoneuraminidase-based inactivation of PSA-NCAM was performed by administration of the drug into the contralateral ventricle of mice with chronic seizure. That action induced neurodegeneration of cells in dentate gyrus and CA3 region of hippocampus and promoted the early onset of focal seizures (Duveau and Fritschy, 2010). Another studies focused on the impact of PSA-NCAM on pathophysiology of epilepsy demonstrated that loss of PSA-NCAM decreased the number of hippocampal newborn cells in kindling-associated changes of hippocampal neuronal network. The downregulation of the hippocampal cell proliferation rate as a consequence of PSA removal did not affect the kindling progression which occurred to be comparable in rats with and without removal of PSA. On the other hand, loss of PSA increased acute seizure susceptibility as indicated by reduced seizure thresholds before kindling (Pekcec et al., 2007). Continuation of the studies on the role of PSA-NCAM utilized an electrically induced poststatus epilepticus (SE) rat model. That approach reviled that loss of PSA counterbalanced the SE-induced increase in neurogenesis in a significant manner. In addition, transient lack of PSA during induction of SE or during the initial period of epileptogenesis exhibited a gentle cognition alteration as revealed by the trials in Morris water maze (Pekcec et al., 2008).
Contactins
Contactin-6 deletions in humans were reported to be associated with various neurological syndromes including seizures as a comorbidity (Hu et al., 2015; Juan-Perez et al., 2018). This strongly suggests implication of contactin-6 in epilepsy, however, further studies on animal models are essential to test this hypothesis.
LRR Proteins
SALM3 has been lately implicated in epileptogenesis and epilepsy. The study by Li et al. (2017) showed that SALM3 was significantly overexpressed in epileptic rats in two distinct animal models (lithium-pilocarpine model, and PTZ kindling model) when compared to control animals. Further Inhibition of SALM3 by SALM3 shRNA ameliorated status epilepticus in the acute phase and decreased spontaneous recurrent seizures evoked by pilocarpine. Inhibition of SALM3 also prolonged the latent period in the PTZ kindling model. The aforementioned results suggest that SALM3 plays a role in epileptogenesis and that its suppression might exert therapeutic effect.
Dystroglycan
Initially, Kaczmarek et al. (2002) reported that β-dystroglycan undergoes limited cleavage in response to kainate treatment in a model of rat temporal lobe epileptogenesis. This finding was further elaborated by Michaluk et al. (2007), who demonstrated that seizure-evoked limited proteolytic cleavage of β-dystroglycan was MMP-9 dependent. Furthermore, Gondo et al. (2014) demonstrated in vitro that abnormal epileptiform activation of neurons with prolonged zero magnesium conditions decreased the expression of β-dystroglycan in mice cortical brain slices. The authors also showed that the β-dystroglycan deficit was associated with the dysfunctions of astrocytic endfeet which suggests epileptogenic downregulation of β-dystroglycan at blood-brain interface. Interestingly, clinical studies have identified two girls with alpha-dystroglycanopathy who presented severe and farmaco-resistant epilepsy characterized with electrographic myoclonic seizures (Di Rosa et al., 2011).
Trans-Synaptic CAMs-Related Proteases in Epilepsy
Among extracellular proteases the most intensively studied in epilepsy pathogenesis are metzincin metalloproteinases. Some of them have already had an identified trans-synaptic target which makes metzincins potential contributors to aberrant synaptic plasticity that occurs in epilepsy development.
The best examined metzincin that is associated with trans-synaptic CAM and plays the significant role in different models and types of epilepsy is MMP-9 (Bronisz and Kurkowska-Jastrzêbska, 2016; Vafadari et al., 2016). The very first reports concerning the participation of MMP-9 in epilepsy were provided by Zhang et al. (1998, 2000), who presented that MMP-9 levels increase in brain of the rats subjected to kainate treatment. Later on, Szklarczyk et al. (2002) showed that MMP-9 at the level of mRNA, protein and enzymatic activity were markedly increased by kainate in the hippocampus, the brain region spared from the cell loss and instead undergoing extensive aberrant plasticity, contributing to the epileptogenesis. In fact, this study founded the field of MMP-9 in the plasticity. Finally, Wilczynski et al. (2008) established the functional role of MMP-9 in epilepsy, demonstrating that: (i) MMP-9 protein levels and enzymatic activity were strongly increased at the synapse upon seizures; (ii) sensitivity to pentylenetetrazol-based kindling was decreased in MMP-9 KO mice, but was increased in transgenic rats with neuronal overexpression of MMP-9; (iii) MMP-9 deficiency diminished kainate-evoked epileptiform changes in reviring of hippocampal network.
Subsequently, the presumed role of MMP-9 in epileptogenesis was confirmed with use of other models namely treatment with either pilocarpine or 4-aminopyridine (Kim et al., 2009; Takács et al., 2010). Recently, Pijet et al. (2018) have demonstrated that MMP-9 deficiency decreased the seizure appearance whereas overexpression of MMP-9 increased the number of mice with spontaneous seizures in posttraumatic injury model. Studies on animals were supplemented with clinical data demonstrating that in human patients with different types of epilepsy, occurrence of chronic seizures is correlated with the elevated levels of MMP-9 in serum, plasma, and cerebrospinal fluid as well as in the brain (Leppert et al., 2000; Suenaga et al., 2008; Li et al., 2012; Romi et al., 2012; Konopka et al., 2013; Zybura-Broda et al., 2016). A role of MMP-9 in the development of epilepsy has been also supported by studies with genetic model based on Wistar Glaxo Rijswijk (WAG/Rij) rats (Kim et al., 2009; Takács et al., 2010).
The role of other MMPs in epilepsy development has been so far poorly investigated. Experimental data indicates the significant increase of MMP-2 activity in animal models of TLE after systemic injection of pilocarpine (Hoehna et al., 2012) or kainate (Zhang et al., 1998, 2000). Gorter et al. (2007) also demonstrated enhancement in the expression of MMP-2 and -14 induced by generalized seizure evoked by electrical stimulation of the hippocampus. In kainate-evoked chronic seizures, also MMP-3 expression was reported to be elevated (Penkowa et al., 2005; Lee et al., 2010). Interestingly, serum concentration of MMP-3 in epileptic patients was in contrast found to be decreased (Wang et al., 2016).
Apart from MMPs, other proteases targeting CAMs were also implicated in epileptogenesis and epilepsy. For instance, ADAM9 and ADAM10 expression were reported to be induced in dentate gyrus of hippocampus following kainite-induced status epilepticus (Ortiz et al., 2005). Moreover, mice with intensive ADAM10 overexpression experience more frequent seizures than either control animals, or mice with moderate ADAM10 overexpression (Clement et al., 2008). In addition, ADAM22- and ADAM23-deficient mice are characterized by occurrence of multiple spontaneous seizures (Sagane et al., 2005; Owuor et al., 2009; Fukata et al., 2010). Also ADAMTS1 expression was demonstrated to be increased after status epilepticus-evoked seizures (Gorter et al., 2007). Neuropsin (KLK8) downregulation has been reported to be associated with seizure burden in animal model of kainate-induced epilepsy (Missault et al., 2017).
There are also other types of proteases definitely implicated in cleavage of trans-synaptic CAMs, and with strong relation to epileptogenesis and epilepsy. Particularly worth to mention is for instance calpain -a protein that belongs to the family of calcium-dependent cysteine proteases (Saido et al., 1992) that was demonstrated to truncate N-cadherin and NCAM (Covault et al., 1991; Jang et al., 2009) and its functional role in epilepsy development was confirmed by a recent work of Lam et al. (2017). Nevertheless, these proteases are predominantly intracellular thus their detailed description is not in the scope of this review.
Concluding Remarks and Perspectives
Although evidence implicating different trans-synaptic complexes in the formation and maintenance of synaptic structure is considerable and their role in synaptic dysfunction convincing, further experimental data is required in order to understand the biological consequences and significance of the proteolytic trans-synaptic CAMs cleavage in modulation of synaptic function. Initial studies have already started to shed light on the dynamics of the key trans-synaptic adhesion molecules cleavage products, in response to synaptic activity. Such experimental data will be essential for an in-depth understanding of the function of adhesion molecules in synaptopathies. Particularly in epilepsy where the trans-synaptic CAMs seem to be crucial for many epileptogenic mechanisms and therefore comprise promising targets for the future pharmacological therapeutic interventions.
Author Contributions
All authors listed have made a substantial, direct and intellectual contribution to the work, and approved it for publication.
Funding
This work was supported by the National Science Centre (NCN, Poland, Grant No. 2015/19/D/NZ7/02402) and National Centre for Research and Development (NCBiR, Poland, Grant No. PBS/A8/36/2015).
Conflict of Interest Statement
The authors declare that the research was conducted in the absence of any commercial or financial relationships that could be construed as a potential conflict of interest.
References
Aiga, M., Levinson, J. N., and Bamji, S. X. (2011). N-cadherin and Neuroligins cooperate to regulate synapse formation in hippocampal cultures. J. Biol. Chem. 286, 851–858. doi: 10.1074/jbc.M110.176305
Antion, M. D., Christie, L. A., Bond, A. M., Dalva, M. B., and Contractor, A. (2010). Ephrin-B3 regulates glutamate receptor signaling at hippocampal synapses. Mol. Cell. Neurosci. 45, 378–388. doi: 10.1016/j.mcn.2010.07.011
Araki, Y., Tomita, S., Yamaguchi, H., Miyagi, N., Sumioka, A., Kirino, Y., et al. (2003). Novel cadherin-related membrane proteins, Alcadeins, enhance the X11-like protein-mediated stabilization of amyloid beta-protein precursor metabolism. J. Biol. Chem. 278, 49448–49458. doi: 10.1074/jbc.M306024200
Arikkath, J., and Reichardt, L. F. (2008). Cadherins and catenins at synapses: roles in synaptogenesis and synaptic plasticity. Trends Neurosci. 31, 487–494. doi: 10.1016/j.tins.2008.07.001
Armstrong, J. N., Saganich, M. J., Xu, N.-J., Henkemeyer, M., Heinemann, S. F., and Contractor, A. (2006). B-ephrin reverse signaling is required for NMDA-independent long-term potentiation of mossy fibers in the hippocampus. J. Neurosci. 26, 3474–3481. doi: 10.1523/JNEUROSCI.4338-05.2006
Attwood, B. K., Bourgognon, J.-M., Patel, S., Mucha, M., Schiavon, E., Skrzypiec, A. E., et al. (2011). Neuropsin cleaves EphB2 in the amygdala to control anxiety. Nature 473, 372–375. doi: 10.1038/nature09938
Bamji, S. X., Shimazu, K., Kimes, N., Huelsken, J., Birchmeier, W., Lu, B., et al. (2003). Role of β-Catenin in synaptic vesicle localization and presynaptic assembly. Neuron 40, 719–731. doi: 10.1016/S0896-6273(03)00718-9
Baranes, D., Lederfein, D., Huang, Y. Y., Chen, M., Bailey, C. H., and Kandel, E. R. (1998). Tissue plasminogen activator contributes to the late phase of LTP and to synaptic growth in the hippocampal mossy fiber pathway. Neuron 21, 813–825. doi: 10.1016/S0896-6273(00)80597-8
Benson, D. L., and Huntley, G. W. (2012). Building and remodeling synapses. Hippocampus 22, 954–968. doi: 10.1002/hipo.20872
Berg, A. T., Berkovic, S. F., Brodie, M. J., Buchhalter, J., Cross, J. H., van Emde Boas, W., et al. (2010). Revised terminology and concepts for organization of seizures and epilepsies: report of the ILAE Commission on Classification and Terminology, 2005-2009. Epilepsia 51, 676–685. doi: 10.1111/j.1528-1167.2010.02522.x
Berg, A. T., and Scheffer, I. E. (2011). New concepts in classification of the epilepsies: entering the 21st century. Epilepsia 52, 1058–1062. doi: 10.1111/j.1528-1167.2011.03101.x
Bhaskar, K., Konerth, M., Kokiko-Cochran, O. N., Cardona, A., Ransohoff, R. M., and Lamb, B. T. (2010). Regulation of tau pathology by the microglial fractalkine receptor. Neuron 68, 19–31. doi: 10.1016/j.neuron.2010.08.023
Biederer, T., and Südhof, T. C. (2000). Mints as adaptors. Direct binding to neurexins and recruitment of munc18. J. Biol. Chem. 275, 39803–39806. doi: 10.1074/jbc.C000656200
Born, G., Breuer, D., Wang, S., Rohlmann, A., Coulon, P., Vakili, P., et al. (2014). Modulation of synaptic function through the α-neurexin-specific ligand neurexophilin-1. Proc. Natl. Acad. Sci. U.S.A. 111, E1274–E1283. doi: 10.1073/pnas.1312112111
Bouzioukh, F., Wilkinson, G. A., Adelmann, G., Frotscher, M., Stein, V., and Klein, R. (2007). Tyrosine phosphorylation sites in ephrinB2 are required for hippocampal long-term potentiation but not long-term depression. J. Neurosci. 27, 11279–11288. doi: 10.1523/JNEUROSCI.3393-07.2007
Bozdagi, O., Valcin, M., Poskanzer, K., Tanaka, H., and Benson, D. L. (2004). Temporally distinct demands for classic cadherins in synapse formation and maturation. Mol. Cell. Neurosci. 27, 509–521. doi: 10.1016/J.MCN.2004.08.008
Bozdagi, O., Wang, X.-B., Nikitczuk, J. S., Anderson, T. R., Bloss, E. B., Radice, G. L., et al. (2010). Persistence of coordinated long-term potentiation and dendritic spine enlargement at mature hippocampal CA1 synapses requires N-cadherin. J. Neurosci. 30, 9984–9989. doi: 10.1523/JNEUROSCI.1223-10.2010
Bozzi, M., Sciandra, F., and Brancaccio, A. (2015). Role of gelatinases in pathological and physiological processes involving the dystrophin–glycoprotein complex. Matrix Biol. 4, 130–137. doi: 10.1016/j.matbio.2015.02.005
Bronisz, E., and Kurkowska-Jastrzêbska, I. (2016). Matrix Metalloproteinase 9 in epilepsy:the role of neuroinflammation in seizure development. Mediators Inflamm. 2016:7369020. doi: 10.1155/2016/7369020
Budreck, E. C., and Scheiffele, P. (2007). Neuroligin-3 is a neuronal adhesion protein at GABAergic and glutamatergic synapses. Eur. J. Neurosci. 26,1738–1748. doi: 10.1111/j.1460-9568.2007.05842.x
Bukalo, O., and Dityatev, A. (2012). ““Synaptic cell adhesion molecules,” in Advances in Experimental Medicine and Biology, eds I. R. Ohen, A. Lajtha, J. D. Lambris, R. Paoletti, and H. Rezaei (Berlin: Springer), 97–128. doi: 10.1007/978-3-7091-0932-8_5
Bukalo, O., Fentrop, N., Lee, A. Y., Salmen, B., Law, J. W., Wotjak, C. T., et al. (2004). Conditional ablation of the neural cell adhesion molecule reduces precision of spatial learning, long-term potentiation, and depression in the CA1 subfield of mouse Hippocampus. J. Neurosci. 24, 1565–1577. doi: 10.1523/JNEUROSCI.3298-03.2004
Buttiglione, M., Revest, J.-M., Rougon, G., and Faivre-Sarrailh, C. (1996). F3 neuronal adhesion molecule controls outgrowth and fasciculation of cerebellar granule cell neurites: a cell-type-specific effect mediated by the Ig-like domains. Mol. Cell. Neurosci. 8, 53–69. doi: 10.1006/mcne.1996.0043
Chamma, I., and Thoumine, O. (2018). Dynamics, nanoscale organization, and function of synaptic adhesion molecules. Mol. Cell. Neurosci. doi: 10.1016/j.mcn.2018.04.007 [Epub ahead of print].
Chen, C.-C., Lu, J., and Zuo, Y. (2014). Spatiotemporal dynamics of dendritic spines in the living brain. Front. Neuroanat. 8:28. doi: 10.3389/fnana.2014.00028
Chih, B., Afridi, S. K., Clark, L., and Scheiffele, P. (2004). Disorder-associated mutations lead to functional inactivation of neuroligins. Hum. Mol. Genet. 13, 1471–1477. doi: 10.1093/hmg/ddh158
Chih, B., Engelman, H., and Scheiffele, P. (2005). Control of excitatory and inhibitory synapse formation by neuroligins. Science 307, 1324–1328. doi: 10.1126/science.1107470
Chubykin, A. A., Atasoy, D., Etherton, M. R., Brose, N., Kvalali, E. T., Gibson, J. R., et al. (2007). Activity-dependent validation of excitatory vs. inhibitory synapses by neuroligin-1 vs. neuroligin-2. Neuron 54, 919–931. doi: 10.1016/j.neuron.2007.05.029
Clement, A. B., Hanstein, R., Schröder, A., Nagel, H., Endres, K., Fahrenholz, F., et al. (2008). Effects of neuron-specific ADAM10 modulation in an in vivo model of acute excitotoxic stress. Neuroscience 152, 459–468. doi: 10.1016/j.neuroscience.2007.10.060
Contractor, A., Rogers, C., Maron, C., Henkemeyer, M., Swanson, G. T., and Heinemann, S. F. (2002). Trans-synaptic Eph receptor-ephrin signaling in hippocampal mossy fiber LTP. Science 296, 1864–1869. doi: 10.1126/science.1069081
Covault, J., Liu, Q. Y., and el-Deeb, S. (1991). Calcium-activated proteolysis of intracellular domains in the cell adhesion molecules NCAM and N-cadherin. Mol. Brain Res. 11, 11–16. doi: 10.1016/0169-328X(91)90015-P
Daar, I. O. (2012). Non-SH2/PDZ reverse signaling by ephrins. Semin. Cell Dev. Biol. 23, 65–74. doi: 10.1016/j.semcdb.2011.10.012
Dahlhaus, R., Hines, R. M., Eadie, B. D., Kannangara, T. S., Hines, D. J., Brown, C. E., et al. (2009). Overexpression of the cell adhesion protein neuroligin-1 induces learning deficits and impairs synaptic plasticity by altering the ratio of excitation to inhibition in the hippocampus. Hippocampus 20, 305–322. doi: 10.1002/hipo.20630
Danielson, E., Metallo, J., and Lee, S. H. (2012). Role of TARP interaction in S-SCAM-mediated regulation of AMPA receptors. Channels 6, 393–397. doi: 10.4161/chan.21301
de Wit, J., Sylwestrak, E., O’Sullivan, M. L., Otto, S., Tiglio, K., Savas, J. N., et al. (2009). LRRTM2 interacts with neurexin1 and regulates excitatory synapse formation. Neuron 64, 799–806. doi: 10.1016/j.neuron.2009.12.019
Depienne, C., Bouteiller, D., Keren, B., Cheuret, E., Poirier, K., Trouillard, O., et al. (2009). Sporadic infantile epileptic encephalopathy caused by mutations in PCDH19 resembles dravet syndrome but mainly affects females. PLoS Genet. 5:e1000381. doi: 10.1371/journal.pgen.1000381
Depienne, C., and LeGuern, E. (2012). PCDH19 -related infantile epileptic encephalopathy: an unusual X-linked inheritance disorder. Hum. Mutat. 33, 627–634. doi: 10.1002/humu.22029
Di Rosa, G., Messina, S., D’Amico, A., Bertini, E., Pustorino, G., Spanò, M., et al. (2011). A new form of alpha-dystroglycanopathy associated with severe drug-resistant epilepsy and unusual EEG features. Epileptic Disord. 13, 259–262. doi: 10.1684/epd.2011.0461
Dibbens, L. M., Tarpey, P. S., Hynes, K., Bayly, M. A., Scheffer, I. E., Smith, R., et al. (2008). X-linked protocadherin 19 mutations cause female-limited epilepsy and cognitive impairment. Nat. Genet. 40, 776–781. doi: 10.1038/ng.149
Dityatev, A., Dityateva, G., and Schachner, M. (2000). Synaptic strength as a function of post- versus presynaptic expression of the neural cell adhesion molecule NCAM. Neuron 26, 207–217. doi: 10.1016/S0896-6273(00)81151-4
Duveau, V., and Fritschy, J.-M. (2010). PSA-NCAM-dependent GDNF signaling limits neurodegeneration and epileptogenesis in temporal lobe epilepsy. Eur. J. Neurosci. 32, 89–98. doi: 10.1111/j.1460-9568.2010.07272.x
Elia, L. P., Yamamoto, M., Zang, K., and Reichardt, L. F. (2006). p120 catenin regulates dendritic spine and synapse development through Rho-family GTPases and cadherins. Neuron 51, 43–56. doi: 10.1016/j.neuron.2006.05.018
Ethell, I. M., and Ethell, D. W. (2007). Matrix metalloproteinases in brain development and remodeling: synaptic functions and targets. J. Neurosci. Res. 85, 2813–2823. doi: 10.1002/jnr.21273
Evans, G. J. (2015). The synaptosome as a model system for studying synaptic physiology. Cold Spring Harb. Protoc. 2015, 421–424. doi: 10.1101/pdb.top074450
Fang, M., Wei, J.-L., Tang, B., Liu, J., Chen, L., Tang, Z.-H., et al. (2016). Neuroligin-1 knockdown suppresses seizure activity by regulating neuronal hyperexcitability. Mol. Neurobiol. 53, 270–284. doi: 10.1007/s12035-014-8999-8
Fisher, R. S., Acevedo, C., Arzimanoglou, A., Bogacz, A., Cross, J. H., Elger, C. E., et al. (2014). ILAE official report: a practical clinical definition of epilepsy. Epilepsia 55, 475–482. doi: 10.1111/epi.12550
Fisher, R. S., Cross, J. H., French, J. A., Higurashi, N., Hirsch, E., Jansen, F. E., et al. (2017). Operational classification of seizure types by the International League Against Epilepsy: position Paper of the ILAE Commission for Classification and Terminology. Epilepsia 58, 522–530. doi: 10.1111/epi.13670
Frankel, W. N. (2009). Genetics of complex neurological disease: challenges and opportunities for modeling epilepsy in mice and rats. Trends Genet. 25,361–367. doi: 10.1016/j.tig.2009.07.001
Fu, A. K., Hung, K.-W., Fu, W.-Y., Shen, C., Chen, Y., Xia, J., et al. (2011). APCCdh1 mediates EphA4-dependent downregulation of AMPA receptors in homeostatic plasticity. Nat. Neurosci. 14, 181–189. doi: 10.1038/nn.2715
Fukata, Y., Lovero, K. L., Iwanaga, T., Watanabe, A., Yokoi, N., Tabuchi, K., et al. (2010). Disruption of LGI1-linked synaptic complex causes abnormal synaptic transmission and epilepsy. Proc. Natl. Acad. Sci. U.S.A. 107, 3799–3804. doi: 10.1073/pnas.0914537107
Gangwar, S. P., Zhong, X., Seshadrinathan, S., Chen, H., Machius, M., and Rudenko, G. (2017). Molecular mechanism of MDGA1: regulation of neuroligin 2: neurexin trans-synaptic bridges. Neuron 94, 1132.e4–1141.e4. doi: 10.1016/j.neuron.2017.06.009
Gjørlund, M. D., Carlsen, E. M. M., Kønig, A. B., Dmytrieva, O., Petersen, A. V., Jacobsen, J., et al. (2017). Soluble ectodomain of neuroligin 1 decreases synaptic activity by activating metabotropic glutamate receptor 2. Front. Mol. Neurosci. 10:116. doi: 10.3389/fnmol.2017.00116
Gokce, O., and Südhof, T. C. (2013). Membrane-tethered monomeric neurexin LNS-domain triggers synapse formation. J. Neurosci. 33, 14617–14628. doi: 10.1523/JNEUROSCI.1232-13.2013
Gondo, A., Shinotsuka, T., Morita, A., Abe, Y., Yasui, M., and Nuriya, M. (2014). Sustained down-regulation of β-dystroglycan and associated dysfunctions of astrocytic endfeet in epileptic cerebral cortex. J. Biol. Chem. 289, 30279–30288. doi: 10.1074/jbc.M114.588384
Górecki, D. C., Szklarczyk, A., Lukasiuk, K., Kaczmarek, L., and Simons, J. P. (1999). Differential seizure-induced and developmental changes of neurexin expression. Mol. Cell. Neurosci. 13, 218–227. doi: 10.1006/MCNE.1999.0740
Gorter, J. A., Van Vliet, E. A., Rauwerda, H., Breit, T., Stad, R., van Schaik, L., et al. (2007). Dynamic changes of proteases and protease inhibitors revealed by microarray analysis in CA3 and entorhinal cortex during epileptogenesis in the rat. Epilepsia 48, 53–64. doi: 10.1111/j.1528-1167.2007.01290.x
Grunwald, I. C., Korte, M., Adelmann, G., Plueck, A., Kullander, K., Adams, R. H., et al. (2004). Hippocampal plasticity requires postsynaptic ephrinBs. Nat. Neurosci. 7, 33–40. doi: 10.1038/nn1164
Hammer, M., Krueger-Burg, D., Patrick, L., Varoqueaux, F., Rhee, J.-S., Brose, N., et al. (2015). Perturbed hippocampal synaptic inhibition and γ- oscillations in a Neuroligin-4 knockout mouse model of autism perturbed hippocampal synaptic inhibition and γ-oscillations in a neuroligin-4 knockout mouse model of autism. Cell Rep. 13, 516–523. doi: 10.1016/j.celrep.2015.09.011
Harris, K. P., and Littleton, J. T. (2015). Transmission, development, and plasticity of synapses. Genetics 201, 345–375. doi: 10.1534/genetics.115.176529
Harrison, V., Connell, L., Hayesmoore, J., McParland, J., Pike, M. G., and Blair, E. (2011). Compound heterozygous deletion of NRXN1 causing severe developmental delay with early onset epilepsy in two sisters. Am. J. Med. Genet. Part A 155, 2826–2831. doi: 10.1002/ajmg.a.34255
Hata, Y., Butz, S., and Südhof, T. C. (1996). CASK: a novel dlg/PSD95 homolog with an N-terminal calmodulin-dependent protein kinase domain identified by interaction with neurexins. J. Neurosci. 16, 2488–2494. doi: 10.1523/JNEUROSCI.16-08-02488.1996
Hattori, M., Osterfield, M., and Flanagan, J. G. (2000). Regulated cleavage of a contact-mediated axon repellent. Science 289, 1360–1365. doi: 10.1126/science.289.5483.1360
Hebb, D. (1949). The Organization of Behavior: A Neuropsychological Theory. New York, NY: JOHN WILEY if SONS, Inc, 335.
Henderson, J. T., Georgiou, J., Jia, Z., Robertson, J., Elowe, S., Roder, J. C., et al. (2001). The receptor tyrosine kinase EphB2 regulates NMDA-dependent synaptic function. Neuron 32, 1041–1056. doi: 10.1016/S0896-6273(01)00553-0
Henkemeyer, M., Itkis, O. S., Ngo, M., Hickmott, P. W., and Ethell, I. M. (2003). Multiple EphB receptor tyrosine kinases shape dendritic spines in the hippocampus. J. Cell Biol. 163, 1313–1326. doi: 10.1083/jcb.200306033
Higurashi, N., Shi, X., Yasumoto, S., Oguni, H., Sakauchi, M., Itomi, K., et al. (2012). PCDH19 mutation in Japanese females with epilepsy. Epilepsy Res. 99, 28–37. doi: 10.1016/j.eplepsyres.2011.10.014
Himanen, J. P. (2012). Ectodomain structures of Eph receptors. Semin. Cell Dev. Biol. 23, 35–42. doi: 10.1016/j.semcdb.2011.10.025
Hines, R. M., Wu, L., Hines, D. J., Steenland, H., Mansour, S., Dahlhaus, R., et al. (2008). Synaptic imbalance, stereotypies, and impaired social interactions in mice with altered neuroligin 2 expression. J. Neurosci. 28, 6055–6067. doi: 10.1523/JNEUROSCI.0032-08.2008
Hinkle, C. L., Diestel, S., Lieberman, J., and Maness, P. F. (2006). Metalloprotease-induced ectodomain shedding of neural cell adhesion molecule (NCAM). J. Neurobiol. 66, 1378–1395. doi: 10.1002/neu.20257
Hintsch, G., Zurlinden, A., Meskenaite, V., Steuble, M., Fink-Widmer, K., Kinter, J., et al. (2002). The calsyntenins—a family of postsynaptic membrane proteins with distinct Neuronal Expression Patterns. Mol. Cell. Neurosci. 21, 393–409. doi: 10.1006/MCNE.2002.1181
Hoehna, Y., Uckermann, O., Luksch, H., Stefovska, V., Marzahn, J., Theil, M., et al. (2012). Matrix metalloproteinase 9 regulates cell death following pilocarpine-induced seizures in the developing brain. Neurobiol. Dis. 48, 339–347. doi: 10.1016/j.nbd.2012.06.023
Holtmaat, A., and Svoboda, K. (2009). Erratum: experience-dependent structural synaptic plasticity in the mammalian brain. Nat. Rev. Neurosci. 10, 759–759. doi: 10.1038/nrn2721
Hruska, M., and Dalva, M. B. (2012). Ephrin regulation of synapse formation, function and plasticity. Mol. Cell. Neurosci. 50, 35–44. doi: 10.1016/j.mcn.2012.03.004
Hu, J., Liao, J., Sathanoori, M., Kochmar, S., Sebastian, J., Yatsenko, S. A., et al. (2015). CNTN6 copy number variations in 14 patients: a possible candidate gene for neurodevelopmental and neuropsychiatric disorders. J. Neurodev. Disord. 7:26. doi: 10.1186/s11689-015-9122-9
Huang, H., Li, R., Yuan, J., Zhou, X., Liu, X., Ou, S., et al. (2016). Up-regulated ephrinB3/EphB3 expression in intractable temporal lobe epilepsy patients and pilocarpine induced experimental epilepsy rat model. Brain Res. 1639, 1–12. doi: 10.1016/j.brainres.2016.02.035
Huber, L., and Menzel, R. (2004). Structural basis of long-trm potentiation in single dendritic spines. Nature 429, 761–766. doi: 10.1038/nature02594.1
Hulpiau, P., and van Roy, F. (2011). New insights into the evolution of metazoan cadherins. Mol. Biol. Evol. 28, 647–657. doi: 10.1093/molbev/msq233
Huntley, G. W. (2012). Synaptic circuit remodelling by matrix metalloproteinases in health and disease. Nat. Rev. Neurosci. 13, 743–757. doi: 10.1038/nrn3320
Ichtchenko, K., Hata, Y., Nguyen, T., Ullrich, B., Missler, M., Moomaw, C., et al. (1995). Neuroligin 1: asplice site-specific ligand for β-neurexins. Cell 81, 435–443. doi: 10.1016/0092-8674(95)90396-8
Ichtchenko, K., Nguyen, T., and Südhof, T. C. (1996). Structures, alternative splicing, and neurexin binding of multiple neuroligins. J. Biol. Chem. 271, 2676–2682. doi: 10.1074/JBC.271.5.2676
Irie, M., Hata, Y., Takeuchi, M., Ichtchenko, K., Toyoda, A., Hirao, K., et al. (1997). Binding of neuroligins to PSD-95. Science 277, 1511–1515. doi: 10.1126/science.277.5331.1511
Ito-Ishida, A., Miura, E., Emi, K., Matsuda, K., Iijima, T., Kondo, T., et al. (2008). Cbln1 regulates rapid formation and maintenance of excitatory synapses in mature cerebellar Purkinje cells in vitro and in vivo. J. Neurosci. 28, 5920–5930. doi: 10.1523/JNEUROSCI.1030-08.2008
Jamal, S. M., Basran, R. K., Newton, S., Wang, Z., and Milunsky, J. M. (2010). Novel de novo PCDH19 mutations in three unrelated females with epilepsy female restricted mental retardation syndrome. Am. J. Med. Genet. A 152A, 2475–2481. doi: 10.1002/ajmg.a.33611
Jang, Y.-N., Jung, Y.-S., Lee, S. H., Moon, C.-H., Kim, C.-H., and Baik, E. J. (2009). Calpain-Mediated N-cadherin proteolytic processing in brain injury. J. Neurosci. 29, 5974–5984. doi: 10.1523/JNEUROSCI.6178-08.2009
Jedlicka, P., Vnencak, M., Krueger, D. D., Jungenitz, T., Brose, N., and Schwarzacher, S. W. (2015). Neuroligin-1 regulates excitatory synaptic transmission, LTP and EPSP-spike coupling in the dentate gyrus in vivo. Brain Struct. Funct. 220, 47–58. doi: 10.1007/s00429-013-0636-1
Juan-Perez, C., Farrand, S., and Velakoulis, D. (2018). Schizophrenia and epilepsy as a result of maternally inherited CNTN6 copy number variant. Schizophr. Res. doi: 10.1016/j.schres.2018.06.062 [Epub ahead of print].
Jungling, K., Eulenburg, V., Moore, R., Kemler, R., Lessmann, V., and Gottmann, K. (2006). N-Cadherin transsynaptically regulates short-term plasticity at glutamatergic synapses in embryonic stem cell-derived neurons. J. Neurosci. 26, 6968–6978. doi: 10.1523/JNEUROSCI.1013-06.2006
Kaczmarek, L., Lapinska-Dzwonek, J., and Szymczak, S. (2002). Matrix metalloproteinases in the adult brain physiology: a link between c-Fos, AP-1 and remodeling of neuronal connections? EMBO J. 21, 6643–6648. doi: 10.1093/emboj/cdf676
Kalus, I., Bormann, U., Mzoughi, M., Schachner, M., and Kleene, R. (2006). Proteolytic cleavage of the neural cell adhesion molecule by ADAM17/TACE is involved in neurite outgrowth. J. Neurochem. 98, 78–88. doi: 10.1111/j.1471-4159.2006.03847.x
Kasai, H., Fukuda, M., Watanabe, S., Hayashi-Takagi, A., and Noguchi, J. (2010). Structural dynamics of dendritic spines in memory and cognition. Trends Neurosci. 33, 121–129. doi: 10.1016/j.tins.2010.01.001
Kasper, C., Rasmussen, H., Kastrup, J. S., Ikemizu, S., Jones, E. Y., Berezin, V., et al. (2000). Structural basis of cell-cell adhesion by NCAM. Nat. Struct. Biol. 7, 389–393. doi: 10.1038/75165
Kayser, M. S., McClelland, A. C., Hughes, E. G., and Dalva, M. B. (2006). Intracellular and trans-synaptic regulation of glutamatergic synaptogenesis by EphB receptors. J. Neurosci. 26, 12152–12164. doi: 10.1523/JNEUROSCI.3072-06.2006
Kim, G. W., Kim, H.-J., Cho, K.-J., Kim, H.-W., Cho, Y.-J., and Lee, B. I. (2009). The role of MMP-9 in integrin-mediated hippocampal cell death after pilocarpine-induced status epilepticus. Neurobiol. Dis. 36, 169–180. doi: 10.1016/j.nbd.2009.07.008
Kim, J., Jung, S.-Y., Lee, Y. K., Park, S., Choi, J.-S., Lee, C. J., et al. (2008). Neuroligin-1 is required for normal expression of LTP and associative fear memory in the amygdala of adult animals. Proc. Natl. Acad. Sci. U.S.A. 105, 9087–9092. doi: 10.1073/pnas.0803448105
Kim, S., Burette, A., Chung, H. S., Kwon, S.-K., Woo, J., Lee, H. W., et al. (2006). NGL family PSD-95-interacting adhesion molecules regulate excitatory synapse formation. Nat. Neurosci. 9, 1294–1301. doi: 10.1038/nn1763
Kishore, U., Gaboriaud, C., Waters, P., Shrive, A. K., Greenhough, T. J., Reid, K. B. M., et al. (2004). C1q and tumor necrosis factor superfamily: modularity and versatility. Trends Immunol. 25, 551–561. doi: 10.1016/J.IT.2004.08.006
Kiss, J. Z., and Rougon, G. (1997). Cell biology of polysialic acid. Curr. Opin. Neurobiol. 7, 640–646. doi: 10.1016/S0959-4388(97)80083-9
Klein, R. (2004). Eph/ephrin signaling in morphogenesis, neural development and plasticity. Curr. Opin. Cell Biol. 16, 580–589. doi: 10.1016/j.ceb.2004.07.002
Klein, R. (2009). Bidirectional modulation of synaptic functions by Eph/ephrin signaling. Nat. Neurosci. 12, 15–20. doi: 10.1038/nn.2231
Ko, J., Fuccillo, M. V., Malenka, R. C., and Südhof, T. C. (2009). LRRTM2 Functions as a Neurexin ligand in promoting excitatory synapse formation. Neuron 64, 791–798. doi: 10.1016/j.neuron.2009.12.012
Ko, J., Kim, S., Chung, H. S., Kim, K., Han, K., Kim, H., et al. (2006). SALM synaptic cell adhesion-like molecules regulate the differentiation of excitatory synapses. Neuron 50, 233–245. doi: 10.1016/j.neuron.2006.04.005
Ko, J., Soler-Llavina, G. J., Fuccillo, M. V., Malenka, R. C., and Südhof, T. C. (2011). Neuroligins/LRRTMs prevent activity- and Ca 2+ /calmodulin-dependent synapse elimination in cultured neurons. J. Cell Biol. 194, 323–334. doi: 10.1083/jcb.201101072
Konopka, A., Grajkowska, W., Ziemiańska, K., Roszkowski, M., Daszkiewicz, P., Rysz, A., et al. (2013). Matrix metalloproteinase-9 (MMP-9) in human intractable epilepsy caused by focal cortical dysplasia. Epilepsy Res. 104, 45–58. doi: 10.1016/j.eplepsyres.2012.09.018
Konorski, J. (1948). Conditioned Reflexes and Neuron Organization. Cambridge: University Press, doi: 10.1002/1097-4679
Krueger, D. D., Tuffy, L. P., Papadopoulos, T., and Brose, N. (2012). The role of neurexins and neuroligins in the formation, maturation, and function of vertebrate synapses. Curr. Opin. Neurobiol. 22, 412–422. doi: 10.1016/J.CONB.2012.02.012
Kullander, K., and Klein, R. (2002). Mechanisms and functions of Eph and ephrin signalling. Nat. Rev. Mol. Cell Biol. 3, 475–486. doi: 10.1038/nrm856
Lam, P. M., Carlsen, J., and González, M. I. (2017). A calpain inhibitor ameliorates seizure burden in an experimental model of temporal lobe epilepsy. Neurobiol. Dis. 102, 1–10. doi: 10.1016/j.nbd.2017.02.003
Laumonnier, F., Bonnet-Brilhault, F., Gomot, M., Blanc, R., David, A., Moizard, M.-P., et al. (2004). X-linked mental retardation and autism are associated with a mutation in the NLGN4 gene, a member of the neuroligin family. Am. J. Hum. Genet. 74, 552–557. doi: 10.1086/382137
Laurén, J., Airaksinen, M. S., Saarma, M., and Timmusk, T. (2003). A novel gene family encoding leucine-rich repeat transmembrane proteins differentially expressed in the nervous system. Genomics 81, 411–421. doi: 10.1016/S0888-7543(03)00030-2
Lee, J., Lim, E., Kim, Y., Li, E., and Park, S. (2010). Ghrelin attenuates kainic acid-induced neuronal cell death in the mouse hippocampus. J. Endocrinol. 205, 263–270. doi: 10.1677/JOE-10-0040
Leite, J., Garcia-Cairasco, N., and Cavalheiro, E. (2002). New insights from the use of pilocarpine and kainate models. Epilepsy Res. 50, 93–103. doi: 10.1016/S0920-1211(02)00072-4
Leppert, D., Leib, S. L., Grygar, C., Miller, K. M., Schaad, U. B., and Holländer, G. A. (2000). Matrix metalloproteinase (MMP)-8 and MMP-9 in cerebrospinal fluid during bacterial meningitis: association with blood-brain barrier damage and neurological sequelae. Clin. Infect. Dis. 31, 80–84. doi: 10.1086/313922
Lévesque, M., Avoli, M., and Bernard, C. (2016). Animal models of temporal lobe epilepsy following systemic chemoconvulsant administration. J. Neurosci. Methods 260, 45–52. doi: 10.1016/j.jneumeth.2015.03.009
Lévi, S., Grady, R. M., Henry, M. D., Campbell, K. P., Sanes, J. R., and Craig, A. M. (2002). Dystroglycan is selectively associated with inhibitory GABAergic synapses but is dispensable for their differentiation. J. Neurosci. 22, 4274–4285. doi: 10.1523/JNEUROSCI.22-11-04274.2002
Levinson, J. N., and El-Husseini, A. (2005). Building excitatory and inhibitory synapses: balancing neuroligin partnerships. Neuron 48, 171–174. doi: 10.1016/j.neuron.2005.09.017
Li, J., Chen, L., Wang, N., Jiang, G., Wu, Y., and Zhang, Y. (2017). Effect of synaptic adhesion-like molecule 3 on epileptic seizures: evidence from animal models. Epilepsy Behav. 69, 18–23. doi: 10.1016/j.yebeh.2016.11.023
Li, S., Yu, S., Zhang, C., Shu, H., Liu, S., An, N., et al. (2012). Increased expression of matrix metalloproteinase 9 in cortical lesions from patients with focal cortical dysplasia type IIb and tuberous sclerosis complex. Brain Res. 1453, 46–55. doi: 10.1016/j.brainres.2012.03.009
Lin, J. C., Ho, W.-H., Gurney, A., and Rosenthal, A. (2003). The netrin-G1 ligand NGL-1 promotes the outgrowth of thalamocortical axons. Nat. Neurosci. 6, 1270–1276. doi: 10.1038/nn1148
Lin, K.-T., Sloniowski, S., Ethell, D. W., and Ethell, I. M. (2008). Ephrin-B2-induced cleavage of EphB2 receptor is mediated by matrix metalloproteinases to trigger cell repulsion. J. Biol. Chem. 283, 28969–28979. doi: 10.1074/jbc.M804401200
Linhoff, M. W., Laurén, J., Cassidy, R. M., Dobie, F. A., Takahashi, H., Nygaard, H. B., et al. (2009). An unbiased expression screen for synaptogenic proteins identifies the LRRTM protein family as synaptic organizers. Neuron 61,734–749. doi: 10.1016/j.neuron.2009.01.017
Lisé, M. F., and El-Husseini, A. (2006). The neuroligin and neurexin families: from structure to function at the synapse. Cell. Mol. Life Sci. 63, 1833–1849. doi: 10.1007/s00018-006-6061-3
Lothman, E. W., and Bertram, E. H. (1993). Epileptogenic effects of status epilepticus. Epilepsia 34(Suppl. 1), S59–S70. doi: 10.1111/j.1528-1157.1993.tb05907.x
Lu, Z., Wang, Y., Chen, F., Tong, H., Reddy, M. V., Luo, L., et al. (2014). Calsyntenin-3 molecular architecture and interaction with neurexin 1α. J. Biol. Chem. 289, 34530–34542. doi: 10.1074/jbc.M114.606806
Lučić, V., Yang, T., Schweikert, G., Förster, F., and Baumeister, W. (2005). Morphological characterization of molecular complexes present in the synaptic cleft. Structure 13, 423–434. doi: 10.1016/j.str.2005.02.005
Lukasiuk, K., Wilczynski, G. M., and Kaczmarek, L. (2011). Extracellular proteases in epilepsy. Epilepsy Res. 96, 191–206. doi: 10.1016/j.eplepsyres.2011.08.002
Lüthi, A., Van der Putten, H., Botteri, F. M., Mansuy, I. M., Meins, M., Frey, U., et al. (1997). Endogenous serine protease inhibitor modulates epileptic activity and hippocampal long-term potentiation. J. Neurosci. 17, 4688–4699. doi: 10.1038/372777a0
Malinverno, M., Carta, M., Epis, R., Marcello, E., Verpelli, C., Cattabeni, F., et al. (2010). Synaptic localization and activity of ADAM10 regulate excitatory synapses through N-cadherin cleavage. J. Neurosci. 30, 16343–16355. doi: 10.1523/JNEUROSCI.1984-10.2010
Marambaud, P., Wen, P. H., Dutt, A., Shioi, J., Takashima, A., Siman, R., et al. (2003). A CBP binding transcriptional repressor produced by the PS1/epsilon-cleavage of N-cadherin is inhibited by PS1 FAD mutations. Cell 114, 635–645. doi: 10.1016/j.cell.2003.08.008
Marini, C., Mei, D., Parmeggiani, L., Norci, V., Calado, E., Ferrari, A., et al. (2010). Protocadherin 19 mutations in girls with infantile-onset epilepsy. Neurology 75, 646–653. doi: 10.1212/WNL.0b013e3181ed9e67
Mathern, G. W., Adelson, P. D., Cahan, L. D., and Leite, J. P. (2002). Hippocampal neuron damage in human epilepsy: Meyer’s hypothesis revisited. Prog. Brain Res. 135, 237–251. doi: 10.1016/S0079-6123(02)35023-4
Matsuda, K., and Yuzaki, M. (2011). Cbln family proteins promote synapse formation by regulating distinct neurexin signaling pathways in various brain regions. Eur. J. Neurosci. 33, 1447–1461. doi: 10.1111/j.1460-9568.2011.07638.x
McMahon, S. A., and Díaz, E. (2011). Mechanisms of excitatory synapse maturation by trans-synaptic organizing complexes. Curr. Opin. Neurobiol. 21, 221–227. doi: 10.1016/J.CONB.2010.12.005
McNamara, J. O. (1984). Role of neurotransmitters in seizure mechanisms in the kindling model of epilepsy. Fed. Proc. 43, 2516–2520.
Mendez, P., De Roo, M., Poglia, L., Klauser, P., and Muller, D. (2010). N-cadherin mediates plasticity-induced long-term spine stabilization. J. Cell Biol. 189, 589–600. doi: 10.1083/jcb.201003007
Mercati, O., Danckaert, A., Andre-Leroux, G., Bellinzoni, M., Gouder, L., Watanabe, K., et al. (2013). Contactin 4, -5 and -6 differentially regulate neuritogenesis while they display identical PTPRG binding sites. Biol. Open 2, 324–334. doi: 10.1242/bio.20133343
Meyer, G., Varoqueaux, F., Neeb, A., Oschlies, M., and Brose, N. (2004). The complexity of PDZ domain-mediated interactions at glutamatergic synapses: a case study on neuroligin. Neuropharmacology 47, 724–733. doi: 10.1016/J.NEUROPHARM.2004.06.023
Michaluk, P., Kolodziej, L., Mioduszewska, B., Wilczynski, G. M., Dzwonek, J., Jaworski, J., et al. (2007). β-dystroglycan as a target for MMP-9, in response to enhanced neuronal activity. J. Biol. Chem. 282, 16036–16041. doi: 10.1074/jbc.M700641200
Millson, A., LaGrave, D., Willis, M. J. H., Rowe, L. R., Lyon, E., and South, S. T. (2012). Chromosomal loss of 3q26.3-3q26.32, involving a partial neuroligin 1 deletion, identified by genomic microarray in a child with microcephaly, seizure disorder, and severe intellectual disability. Am. J. Med. Genet. Part A 158A, 159–165. doi: 10.1002/ajmg.a.34349
Missault, S., Peeters, L., Amhaoul, H., Thomae, D., Van Eetveldt, A., Favier, B., et al. (2017). Decreased levels of active uPA and KLK8 assessed by [111 In]MICA-401 binding correlate with the seizure burden in an animal model of temporal lobe epilepsy. Epilepsia 58, 1615–1625. doi: 10.1111/epi.13845
Missler, M., Hammer, R. E., and Südhof, T. C. (1998). Neurexophilin binding to alpha-neurexins. A single LNS domain functions as an independently folding ligand-binding unit. J. Biol. Chem. 273, 34716–34723. doi: 10.1074/JBC.273.52.34716
Missler, M., and Südhof, T. C. (1998). Neurexins: three genes and 1001 products. Trends Genet. 14, 20–26. doi: 10.1016/S0168-9525(97)01324-3
Missler, M., Zhang, W., Rohlmann, A., Kattenstroth, G., Hammer, R. E., Gottmann, K., et al. (2003). α-Neurexins couple Ca2+ channels to synaptic vesicle exocytosis. Nature 423, 939–948. doi: 10.1038/nature01755
Møller, R. S., Weber, Y. G., Klitten, L. L., Trucks, H., Muhle, H., Kunz, W. S., et al. (2013). Exon-disrupting deletions of NRXN1 in idiopathic generalized epilepsy. Epilepsia 54, 256–264. doi: 10.1111/epi.12078
Monea, S., Jordan, B. A., Srivastava, S., DeSouza, S., and Ziff, E. B. (2006). Membrane localization of membrane type 5 matrix metalloproteinase by AMPA receptor binding protein and cleavage of cadherins. J. Neurosci. 26, 2300–2312. doi: 10.1523/JNEUROSCI.3521-05.2006
Moshé, S. L., Perucca, E., Ryvlin, P., and Tomson, T. (2015). Epilepsy: new advances. Lancet 385, 884–898. doi: 10.1016/S0140-6736(14)60456-6
Mott, J. D., and Werb, Z. (2004). Regulation of matrix biology by matrix metalloproteinases. Curr. Opin. Cell Biol. 16, 558–564. doi: 10.1016/j.ceb.2004.07.010
Müller, B. M., Kistner, U., Kindler, S., Chung, W. J., Kuhlendahl, S., Fenster, S. D., et al. (1996). SAP102, a novel postsynaptic protein that interacts with NMDA receptor complexes in vivo. Neuron 17, 255–265. doi: 10.1016/S0896-6273(00)80157-9
Murai, K. K., Misner, D., and Ranscht, B. (2002). Contactin supports synaptic plasticity associated with hippocampal long-term depression but not potentiation. Curr. Biol. 12, 181–190. doi: 10.1016/S0960-9822(02)00680-2
Murai, K. K., Nguyen, L. N., Irie, F., Yamaguchi, Y., and Pasquale, E. B. (2003). Control of hippocampal dendritic spine morphology through ephrin-A3/EphA4 signaling. Nat. Neurosci. 6, 153–160. doi: 10.1038/nn994
Murai, K. K., and Pasquale, E. B. (2004). Eph receptors, ephrins, and synaptic function. Neuroscientist 10, 304–314. doi: 10.1177/1073858403262221
Nagappan-Chettiar, S., Johnson-Venkatesh, E. M., and Umemori, H. (2017). Activity-dependent proteolytic cleavage of cell adhesion molecules regulates excitatory synaptic development and function. Neurosci. Res. 116, 60–69. doi: 10.1016/J.NEURES.2016.12.003
Nakashiba, T., Nishimura, S., Ikeda, T., and Itohara, S. (2002). Complementary expression and neurite outgrowth activity of netrin-G subfamily members. Mech. Dev. 111, 47–60. doi: 10.1016/S0925-4773(01)00600-1
Nam, J., Mah, W., and Kim, E. (2011). The SALM/Lrfn family of leucine-rich repeat-containing cell adhesion molecules. Semin. Cell Dev. Biol. 22, 492–498. doi: 10.1016/j.semcdb.2011.06.005
Neupert, C., Schneider, R., Klatt, O., Reissner, C., Repetto, D., Biermann, B., et al. (2015). Regulated dynamic trafficking of neurexins inside and outside of synaptic terminals. J. Neurosci. 35, 13629–13647. doi: 10.1523/JNEUROSCI.4041-14.2015
Nikolaienko, R. M., Hammel, M., Dubreuil, V., Zalmai, R., Hall, D. R., Mehzabeen, N., et al. (2016). Structural basis for interactions between contactin family members and protein-tyrosine phosphatase receptor type G in neural tissues. J. Biol. Chem. 291, 21335–21349. doi: 10.1074/jbc.M116.742163
Okuda, T., Yu, L. M. Y., Cingolani, L. A., Kemler, R., and Goda, Y. (2007). beta-Catenin regulates excitatory postsynaptic strength at hippocampal synapses. Proc. Natl. Acad. Sci. U.S.A. 104, 13479–13484. doi: 10.1073/pnas.0702334104
Ortiz, R. M., Kärkkäinen, I., Huovila, A.-P. J., and Honkaniemi, J. (2005). ADAM9, ADAM10, and ADAM15 mRNA levels in the rat brain after kainic acid-induced status epilepticus. Brain Res. Mol. Brain Res. 137, 272–275. doi: 10.1016/j.molbrainres.2005.03.008
Ottman, R., Hirose, S., Jain, S., Lerche, H., Lopes-Cendes, I., Noebels, J. L., et al. (2010). Genetic testing in the epilepsies-report of the ILAE genetics commission. Epilepsia 51, 655–670. doi: 10.1111/j.1528-1167.2009.02429.x
Owuor, K., Harel, N. Y., Englot, D. J., Hisama, F., Blumenfeld, H., and Strittmatter, S. M. (2009). LGI1-associated epilepsy through altered ADAM23-dependent neuronal morphology. Mol. Cell. Neurosci. 42, 448–457. doi: 10.1016/j.mcn.2009.09.008
Pasquale, E. B. (2004). Eph–ephrin promiscuity is now crystal clear. Nat. Neurosci. 7, 417–418. doi: 10.1038/nn0504-417
Pasquale, E. B. (2005). Eph receptor signalling casts a wide net on cell behaviour. Nat. Rev. Mol. Cell Biol. 6, 462–475. doi: 10.1038/nrm1662
Peixoto, R. T., Kunz, P. A., Kwon, H., Mabb, A. M., Sabatini, B. L., Philpot, B. D., et al. (2012). Transsynaptic signaling by activity-dependent cleavage of Neuroligin-1. Neuron 76, 396–409. doi: 10.1016/j.neuron.2012.07.006
Pekcec, A., Fuest, C., Mühlenhoff, M., Gerardy-Schahn, R., and Potschka, H. (2008). Targeting epileptogenesis-associated induction of neurogenesis by enzymatic depolysialylation of NCAM counteracts spatial learning dysfunction but fails to impact epilepsy development. J. Neurochem. 105, 389–400. doi: 10.1111/j.1471-4159.2007.05172.x
Pekcec, A., Mühlenhoff, M., Gerardy-Schahn, R., and Potschka, H. (2007). Impact of the PSA-NCAM system on pathophysiology in a chronic rodent model of temporal lobe epilepsy. Neurobiol. Dis. 27, 54–66. doi: 10.1016/j.nbd.2007.04.002
Penkowa, M., Florit, S., Giralt, M., Quintana, A., Molinero, A., Carrasco, J., et al. (2005). Metallothionein reduces central nervous system inflammation, neurodegeneration, and cell death following kainic acid-induced epileptic seizures. J. Neurosci. Res. 79, 522–534. doi: 10.1002/jnr.20387
Perez de Arce, K., Schrod, N., Metzbower, S. W. R., Allgeyer, E., Kong, G. K. W., et al. (2015). Topographic mapping of the synaptic cleft into adhesive nanodomains. Neuron 88, 1165–1172. doi: 10.1016/j.neuron.2015.11.011
Perrin, F. E., Rathjen, F. G., and Stoeckli, E. T. (2001). Distinct subpopulations of sensory afferents require F11 or axonin-1 for growth to their target layers within the spinal cord of the chick. Neuron 30, 707–723. doi: 10.1016/S0896-6273(01)00315-4
Petrenko, A. G., Ullrich, B., Missler, M., Krasnoperov, V., Rosahl, T. W., and Südhof, T. C. (1996). Structure and evolution of neurexophilin. J. Neurosci. 16, 4360–4369. doi: 10.1523/JNEUROSCI.16-14-04360.1996
Pettem, K. L., Yokomaku, D., Luo, L., Linhoff, M. W., Prasad, T., Connor, S. A., et al. (2013). The specific α-neurexin interactor calsyntenin-3 promotes excitatory and inhibitory synapse development. Neuron 80, 113–128. doi: 10.1016/J.NEURON.2013.07.016
Pijet, B., Stefaniuk, M., Kostrzewska-Ksiezyk, A., Tsilibary, P.-E., Tzinia, A., and Kaczmarek, L. (2018). Elevation of MMP-9 levels promotes epileptogenesis after traumatic brain injury. Mol. Neurobiol. doi: 10.1007/s12035-018-1061-5 [Epub ahead of print].
Pitkänen, A., Immonen, R. J., Gröhn, O. H. J., and Kharatishvili, I. (2009). From traumatic brain injury to posttraumatic epilepsy: what animal models tell us about the process and treatment options. Epilepsia 50, 21–29. doi: 10.1111/j.1528-1167.2008.02007.x
Pitkänen, A., and Lukasiuk, K. (2011). Mechanisms of epileptogenesis and potential treatment targets. Lancet Neurol. 10, 173–186. doi: 10.1016/S1474-4422(10)70310-0
Pribiag, H., Peng, H., Shah, W. A., Stellwagen, D., and Carbonetto, S. (2014). Dystroglycan mediates homeostatic synaptic plasticity at GABAergic synapses. Proc. Natl. Acad. Sci. U.S.A. 111, 6810–6815. doi: 10.1073/pnas.1321774111
Qian, Z., Gilbert, M. E., Colicos, M. A., Kandel, E. R., and Kuhl, D. (1993). Tissue-plasminogen activator is induced as an immediate–early gene during seizure, kindling and long-term potentiation. Nature 361, 453–457. doi: 10.1038/361453a0
Reissner, C., Runkel, F., and Missler, M. (2013). Neurexins. Genome Biol. 14:213. doi: 10.1186/gb-2013-14-9-213
Reyes, A. A., Small, S. J., and Akeson, R. (1991). At least 27 alternatively spliced forms of the neural cell adhesion molecule mRNA are expressed during rat heart development. Mol. Cell. Biol. 11, 1654–1661.
Rivera, S., Khrestchatisky, M., Kaczmarek, L., Rosenberg, G. A., and Jaworski, D. M. (2010). Metzincin proteases and their inhibitors: foes or friends in nervous system physiology? J. Neurosci. 30, 15337–15357. doi: 10.1523/JNEUROSCI.3467-10.2010
Romi, F., Helgeland, G., and Gilhus, N. E. (2012). Serum levels of matrix metalloproteinases: implications in clinical neurology. Eur. Neurol. 67,121–128. doi: 10.1159/000334862
Rubio, M. E., Curcio, C., Chauvet, N., and Brusés, J. L. (2005). Assembly of the N-cadherin complex during synapse formation involves uncoupling of p120-catenin and association with presenilin 1. Mol. Cell. Neurosci. 30, 118–130. doi: 10.1016/j.mcn.2005.06.005
Rutishauser, U., and Landmesser, L. (1996). Polysialic acid in the vertebrate nervous system: a promoter of plasticity in cell-cell interactions. Trends Neurosci. 19, 422–427. doi: 10.1016/0166-2236(96)10041-2
Sagane, K., Hayakawa, K., Kai, J., Hirohashi, T., Takahashi, E., Miyamoto, N., et al. (2005). Ataxia and peripheral nerve hypomyelination in ADAM22-deficient mice. BMC Neurosci. 6:33. doi: 10.1186/1471-2202-6-33
Saglietti, L., Dequidt, C., Kamieniarz, K., Rousset, M.-C., Valnegri, P., Thoumine, O., et al. (2007). Extracellular interactions between GluR2 and N-cadherin in spine regulation. Neuron 54, 461–477. doi: 10.1016/j.neuron.2007.04.012
Saido, T. C., Nagao, S., Shiramine, M., Tsukaguchi, M., Sorimachi, H., Murofushi, H., et al. (1992). Autolytic transition of mu-calpain upon activation as resolved by antibodies distinguishing between the pre- and post-autolysis forms. J. Biochem. 111, 81–86. doi: 10.1093/oxfordjournals.jbchem.a123723
Sakurai, K., Toyoshima, M., Takeda, Y., Shimoda, Y., and Watanabe, K. (2010). Synaptic formation in subsets of glutamatergic terminals in the mouse hippocampal formation is affected by a deficiency in the neural cell recognition molecule NB-3. Neurosci. Lett. 473, 102–106. doi: 10.1016/J.NEULET.2010.02.027
Sato, K., Iwai, M., Zhang, W. R., Kamada, H., Ohta, K., Omori, N., et al. (2003). Highly polysialylated neural cell adhesion molecule (PSA-NCAM) positive cells are increased and change localization in rat hippocampus by exposure to repeated kindled seizures. Acta Neurochir. Suppl. 86, 575–579.
Satz, J. S., Ostendorf, A. P., Hou, S., Turner, A., Kusano, H., Lee, J. C., et al. (2010). Distinct functions of glial and neuronal dystroglycan in the developing and adult Mouse Brain. J. Neurosci. 30, 14560–14572. doi: 10.1523/JNEUROSCI.3247-10.2010
Scheffer, I. E., Turner, S. J., Dibbens, L. M., Bayly, M. A., Friend, K., Hodgson, B., et al. (2008). Epilepsy and mental retardation limited to females: an under-recognized disorder. Brain 131, 918–927. doi: 10.1093/brain/awm338
Schnell, E., Sizemore, M., Karimzadegan, S., Chen, L., Bredt, D. S., and Nicoll, R. A. (2002). Direct interactions between PSD-95 and stargazin control synaptic AMPA receptor number. Proc. Natl. Acad. Sci. U.S.A. 99, 13902–13907. doi: 10.1073/pnas.172511199
Seabold, G. K., Wang, P. Y., Chang, K., Wang, C.-Y., Wang, Y.-X., Petralia, R. S., et al. (2008). The SALM family of adhesion-like molecules forms heteromeric and homomeric complexes. J. Biol. Chem. 283, 8395–8405. doi: 10.1074/jbc.M709456200
Seigneur, E., and Südhof, T. C. (2017). Cerebellins are differentially expressed in selective subsets of neurons throughout the brain. J. Comp. Neurol. 525, 3286–3311. doi: 10.1002/cne.24278
Seigneur, E., and Südhof, T. C. (2018). Genetic ablation of all cerebellins reveals synapse organizer functions in multiple regions throughout the brain. J. Neurosci. 38, 4774–4790. doi: 10.1523/JNEUROSCI.0360-18.2018
Serikawa, T., Mashimo, T., Kuramoro, T., Voigt, B., Ohno, Y., and Sasa, M. (2015). Advances on genetic rat models of epilepsy. Exp. Anim. 64, 1–7. doi: 10.1538/expanim.14-0066
Shan, W., Yoshida, M., Wu, X.-R., Huntley, G. W., and Colman, D. R. (2002). Neural (N-) cadherin, a synaptic adhesion molecule, is induced in hippocampal mossy fiber axonal sprouts by seizure. J. Neurosci. Res. 69, 292–304. doi: 10.1002/jnr.10305
Shichi, K., Fujita-Hamabe, W., Harada, S., Mizoguchi, H., Yamada, K., Nabeshima, T., et al. (2011). Involvement of matrix metalloproteinase-mediated proteolysis of neural cell adhesion molecule in the development of cerebral ischemic neuronal damage. J. Pharmacol. Exp. Ther. 338, 701–710. doi: 10.1124/jpet.110.178079
Shimoda, Y., and Watanabe, K. (2009). Contactins: emerging key roles in the development and function of the nervous system. Cell Adh. Migr. 3, 64–70.
Shinoe, T., and Goda, Y. (2015). Tuning synapses by proteolytic remodeling of the adhesive surface. Curr. Opin. Neurobiol. 35, 148–155. doi: 10.1016/J.CONB.2015.08.005
Siddiqui, T. J., and Craig, A. M. (2011). Synaptic organizing complexes. Curr. Opin. Neurobiol. 21, 132–143. doi: 10.1016/j.conb.2010.08.016
Siddiqui, T. J., Pancaroglu, R., Kang, Y., Rooyakkers, A., and Craig, A. M. (2010). LRRTMs and neuroligins bind neurexins with a differential code to cooperate in glutamate Synapse Development. J. Neurosci. 30, 7495–7506. doi: 10.1523/JNEUROSCI.0470-10.2010
Soler-Llavina, G. J., Fuccillo, M. V., Ko, J., Sudhof, T. C., and Malenka, R. C. (2011). The neurexin ligands, neuroligins and leucine-rich repeat transmembrane proteins, perform convergent and divergent synaptic functions in vivo. Proc. Natl. Acad. Sci. U.S.A. 108, 16502–16509. doi: 10.1073/pnas.1114028108
Song, J. Y., Ichtchenko, K., Südhof, T. C., and Brose, N. (1999). Neuroligin 1 is a postsynaptic cell-adhesion molecule of excitatory synapses. Proc. Natl. Acad. Sci. U.S.A. 96, 1100–1105. doi: 10.1073/pnas.96.3.1100
Specchio, N., Fusco, L., and Vigevano, F. (2011a). Acute-onset epilepsy triggered by fever mimicking FIRES (febrile infection-related epilepsy syndrome): the role of protocadherin 19 (PCDH19) gene mutation. Epilepsia 52, e172–e175. doi: 10.1111/j.1528-1167.2011.03193.x
Specchio, N., Marini, C., Terracciano, A., Mei, D., Trivisano, M., Sicca, F., et al. (2011b). Spectrum of phenotypes in female patients with epilepsy due to protocadherin 19 mutations. Epilepsia 52, 1251–1257. doi: 10.1111/j.1528-1167.2011.03063.x
Stan, A., Pielarski, K. N., Brigadski, T., Wittenmayer, N., Fedorchenko, O., Gohla, A., et al. (2010). Essential cooperation of N-cadherin and neuroligin-1 in the transsynaptic control of vesicle accumulation. Proc. Natl. Acad. Sci. U.S.A. 107, 11116–11121. doi: 10.1073/pnas.0914233107
Südhof, T. C. (2017). Synaptic Neurexin Complexes: a molecular code for the logic of neural circuits. Cell 171, 745–769. doi: 10.1016/j.cell.2017.10.024
Suenaga, N., Ichiyama, T., Kubota, M., Isumi, H., Tohyama, J., and Furukawa, S. (2008). Roles of matrix metalloproteinase-9 and tissue inhibitors of metalloproteinases 1 in acute encephalopathy following prolonged febrile seizures. J. Neurol. Sci. 266, 126–130. doi: 10.1016/j.jns.2007.09.011
Sugita, S., Saito, F., Tang, J., Satz, J., Campbell, K., and Südhof, T. C. (2001). A stoichiometric complex of neurexins and dystroglycan in brain. J. Cell Biol. 154, 435–445. doi: 10.1083/JCB.200105003
Suzuki, K., Hayashi, Y., Nakahara, S., Kumazaki, H., Prox, J., Horiuchi, K., et al. (2012). Activity-dependent proteolytic cleavage of neuroligin-1. Neuron 76, 410–422. doi: 10.1016/j.neuron.2012.10.003
Sytnyk, V., Leshchyns’ka, I., Nikonenko, A. G., and Schachner, M. (2006). NCAM promotes assembly and activity-dependent remodeling of the postsynaptic signaling complex. J. Cell Biol. 174, 1071–1085. doi: 10.1083/jcb.200604145
Szklarczyk, A., Lapinska, J., Rylski, M., McKay, R. D. G., and Kaczmarek, L. (2002). Matrix metalloproteinase-9 undergoes expression and activation during dendritic remodeling in adult hippocampus. J. Neurosci. 22, 920–930. doi: 10.1523/JNEUROSCI.22-03-00920.2002
Tai, C.-Y., Kim, S. A., and Schuman, E. M. (2008). Cadherins and synaptic plasticity. Curr. Opin. Cell Biol. 20, 567–575. doi: 10.1016/J.CEB.2008.06.003
Takács, E., Nyilas, R., Szepesi, Z., Baracskay, P., Karlsen, B., Røsvold, T., et al. (2010). Matrix metalloproteinase-9 activity increased by two different types of epileptic seizures that do not induce neuronal death: a possible role in homeostatic synaptic plasticity. Neurochem. Int. 56, 799–809. doi: 10.1016/j.neuint.2010.03.003
Takeichi, M. (2007). The cadherin superfamily in neuronal connections and interactions. Nat. Rev. Neurosci. 8, 11–20. doi: 10.1038/nrn2043
Tamura, H., Ishikawa, Y., Hino, N., Maeda, M., Yoshida, S., Kaku, S., et al. (2006). Neuropsin is essential for early processes of memory acquisition and Schaffer collateral long-term potentiation in adult mouse hippocampus in vivo. J. Physiol. 570, 541–551. doi: 10.1113/jphysiol.2005.098715
Togashi, H., Abe, K., Mizoguchi, A., Takaoka, K., Chisaka, O., and Takeichi, M. (2002). Cadherin regulates dendritic spine morphogenesis. Neuron 35, 77–89. doi: 10.1016/S0896-6273(02)00748-1
Uemura, T., Lee, S.-J., Yasumura, M., Takeuchi, T., Yoshida, T., Ra, M., et al. (2010). Trans-Synaptic interaction of GluRδ2 and neurexin through Cbln1 mediates synapse formation in the cerebellum. Cell 141, 1068–1079. doi: 10.1016/j.cell.2010.04.035
Vafadari, B., Salamian, A., and Kaczmarek, L. (2016). MMP-9 in translation: from molecule to brain physiology, pathology, and therapy. J. Neurochem. 139, 91–114. doi: 10.1111/jnc.13415
Varoqueaux, F., Aramuni, G., Rawson, R. L., Mohrmann, R., Missler, M., Gottmann, K., et al. (2006). Neuroligins determine synapse maturation and function. Neuron 51, 741–754. doi: 10.1016/j.neuron.2006.09.003
Varoqueaux, F., Jamain, S., and Brose, N. (2004). Neuroligin 2 is exclusively localized to inhibitory synapses. Eur. J. Cell Biol. 83, 449–456. doi: 10.1078/0171-9335-00410
Verhage, M., Maia, A. S., Plomp, J. J., Brussaard, A. B., Heeroma, J. H., Vermeer, H., et al. (2000). Synaptic assembly of the brain in the absence of neurotransmitter secretion. Science 287, 864–869. doi: 10.1126/science.287.5454.864
Vincent, A. K., Noor, A., Janson, A., Minassian, B. A., Ayub, M., Vincent, J. B., et al. (2012). Identification of genomic deletions spanning the PCDH19 gene in two unrelated girls with intellectual disability and seizures. Clin. Genet. 82, 540–545. doi: 10.1111/j.1399-0004.2011.01812.x
Vogt, L., Schrimpf, S. P., Meskenaite, V., Frischknecht, R., Kinter, J., Leone, D. P., et al. (2001). Calsyntenin-1, a proteolytically processed postsynaptic membrane protein with a cytoplasmic calcium-binding domain. Mol. Cell. Neurosci. 17, 151–166. doi: 10.1006/mcne.2000.0937
Wang, C.-Y., Chang, K., Petralia, R. S., Wang, Y.-X., Seabold, G. K., and Wenthold, R. J. (2006). A novel family of adhesion-like molecules that interacts with the NMDA Receptor. J. Neurosci. 26, 2174–2183. doi: 10.1523/JNEUROSCI.3799-05.2006
Wang, R., Zeng, G. Q., Liu, X., Tong, R. Z., Zhou, D., and Hong, Z. (2016). Evaluation of serum matrix metalloproteinase-3 as a biomarker for diagnosis of epilepsy. J. Neurol. Sci. 367, 291–297. doi: 10.1016/j.jns.2016.06.031
Wang, W., Wang, L., Luo, J., Xi, Z., Wang, X., Chen, G., et al. (2012). Role of a neural cell adhesion molecule found in cerebrospinal fluid as a potential biomarker for epilepsy. Neurochem. Res. 37, 819–825. doi: 10.1007/s11064-011-0677-x
Washbourne, P., Dityatev, A., Scheiffele, P., Biederer, T., Weiner, J. A., Christopherson, K. S., et al. (2004). Cell adhesion molecules in synapse formation. J. Neurosci. 24, 9244–9249. doi: 10.1523/JNEUROSCI.3339-04.2004
Weiner, J. A., and Jontes, J. D. (2013). Protocadherins, not prototypical: a complex tale of their interactions, expression, and functions. Front. Mol. Neurosci. 6:4. doi: 10.3389/fnmol.2013.00004
Wilczynski, G. M., Konopacki, F. A., Wilczek, E., Lasiecka, Z., Gorlewicz, A., and Michaluk, P. (2008). Important role of matrix metalloproteinase 9 in epileptogenesis. J. Cell Biol. 180, 1021–1035. doi: 10.1083/jcb.200708213
Woo, J., Kwon, S.-K., and Kim, E. (2009). The NGL family of leucine-rich repeat-containing synaptic adhesion molecules. Mol. Cell. Neurosci. 42, 1–10. doi: 10.1016/j.mcn.2009.05.008
Xia, Y., Luo, C., Dai, S., and Yao, D. (2013). Increased EphA/ephrinA expression in hippocampus of pilocarpine treated mouse. Epilepsy Res. 105, 20–29. doi: 10.1016/j.eplepsyres.2013.01.001
Xu, B., Li, S., Brown, A., Gerlai, R., Fahnestock, M., and Racine, R. J. (2003). EphA/ephrin-A interactions regulate epileptogenesis and activity-dependent axonal sprouting in adult rats. Mol. Cell. Neurosci. 24, 984–999. doi: 10.1016/j.mcn.2003.08.003
Yamada, S., and Nelson, W. J. (2007). Synapses: sites of cell recognition, adhesion, and functional specification. Annu. Rev. Biochem. 76, 267–294. doi: 10.1146/annurev.biochem.75.103004.142811
Yamada, S., Pokutta, S., Drees, F., Weis, W. I., and Nelson, W. J. (2005). Deconstructing the cadherin-catenin-actin complex. Cell 123, 889–901. doi: 10.1016/j.cell.2005.09.020
Yuen, A. W. C., Keezer, M. R., and Sander, J. W. (2018). Epilepsy is a neurological and a systemic disorder. Epilepsy Behav. 78, 57–61. doi: 10.1016/j.yebeh.2017.10.010
Zhang, J. W., Deb, S., and Gottschall, P. E. (1998). Regional and differential expression of gelatinases in rat brain after systemic kainic acid or bicuculline administration. Eur. J. Neurosci. 10, 3358–3368. doi: 10.1046/j.1460-9568.1998.00347.x
Zhang, J. W., Deb, S., and Gottschall, P. E. (2000). Regional and age-related expression of gelatinases in the brains of young and old rats after treatment with kainic acid. Neurosci. Lett. 295, 9–12. doi: 10.1016/S0304-3940(00)01582-2
Zhang, W., Rajan, I., Savelieva, K. V., Wang, C.-Y., Vogel, P., and Kelly, M. (2008). Netrin-G2 and netrin-G2 ligand are both required for normal auditory responsiveness. Genes Brain Behav. 7, 385–392. doi: 10.1111/j.1601-183X.2007.00361.x
Zuber, B., Nikonenko, I., Klauser, P., Muller, D., and Dubochet, J. (2005). The mammalian central nervous synaptic cleft contains a high density of periodically organized complexes. Proc. Natl. Acad. Sci. U.S.A. 102,19192–19197. doi: 10.1073/pnas.0509527102
Zuko, A., Kleijer, K. T. E., Oguro-Ando, A., Kas, M. J. H., van Daalen, E., van der Zwaag, B., et al. (2013). Contactins in the neurobiology of autism. Eur. J. Pharmacol. 719, 63–74. doi: 10.1016/j.ejphar.2013.07.016
Keywords: synaptic plasticity, autism spectrum disorders, epileptogenesis, neuronal development, neuropsychiatric disorders
Citation: Gorlewicz A and Kaczmarek L (2018) Pathophysiology of Trans-Synaptic Adhesion Molecules: Implications for Epilepsy. Front. Cell Dev. Biol. 6:119. doi: 10.3389/fcell.2018.00119
Received: 13 May 2018; Accepted: 30 August 2018;
Published: 21 September 2018.
Edited by:
Mitsugu Fujita, Kindai University, JapanReviewed by:
Iryna Leshchyns’Ka, University of New South Wales, AustraliaGabriele Loers, Universitätsklinikum Hamburg-Eppendorf, Germany
Copyright © 2018 Gorlewicz and Kaczmarek. This is an open-access article distributed under the terms of the Creative Commons Attribution License (CC BY). The use, distribution or reproduction in other forums is permitted, provided the original author(s) and the copyright owner(s) are credited and that the original publication in this journal is cited, in accordance with accepted academic practice. No use, distribution or reproduction is permitted which does not comply with these terms.
*Correspondence: Adam Gorlewicz, YS5nb3JsZXdpY3pAbmVuY2tpLmdvdi5wbA==