- Department of Cell and Developmental Biology, Perelman School of Medicine, Abramson Family Cancer Research Institute, Institute for Regenerative Medicine, University of Pennsylvania, Philadelphia, PA, United States
RUNX1 is a recurrently mutated gene in sporadic myelodysplastic syndrome and leukemia. Inherited mutations in RUNX1 cause familial platelet disorder with predisposition to acute myeloid leukemia (FPD/AML). In sporadic AML, mutations in RUNX1 are usually secondary events, whereas in FPD/AML they are initiating events. Here we will describe mutations in RUNX1 in sporadic AML and in FPD/AML, discuss the mechanisms by which inherited mutations in RUNX1 could elevate the risk of AML in FPD/AML individuals, and speculate on why mutations in RUNX1 are rarely, if ever, the first event in sporadic AML.
Introduction
Acute leukemia is caused by the acquisition of mutations in hematopoietic stem and/or progenitor cells (HSPCs) that promote their clonal expansion and impair downstream differentiation. The earliest initiating step in leukemia is a mutation that generates a hematopoietic stem cell (HSC) that is, in some poorly understood way, primed for leukemic transformation by secondary mutations. The mutations in HSCs that initiate sporadic leukemia in adults generally occur in genes encoding epigenetic regulatory proteins such as DNMT3A, ASXL1, IDH2, and TET2 (Papaemmanuil et al., 2016). Disease progression is caused by the acquisition of secondary mutations in HSCs containing initiating mutations. These secondary, driver mutations involve genes encoding several functional categories of proteins including transcription factors (e.g., CEBPA, RUNX1, GATA2, and ETV6), signaling molecules (FLT3, NRAS, PTPN11, KRAS, KIT, CBL, and NF1), splicing factors (SRSF2, SF3B1, and U2AF1), and proteins with other functions (NPM1, SMC1A) (Cancer Genome Atlas Research Network et al., 2013; Papaemmanuil et al., 2016).
The order of mutations in sporadic acute leukemia in adults, where mutations in epigenetic regulators generally precede those in genes encoding other categories of proteins, is upended in individuals who have inherited a leukemia predisposition gene. Leukemia predisposition genes are constitutional mutations that greatly elevate the lifetime risk of leukemia, which in the general population is 1.5% (Howlader et al., 1975–2014), but in individuals with inherited leukemia disposition genes, the risk of myeloid malignancy is much higher depending on the mutation and other genetic, epigenetic, and environmental factors (Owen C. et al., 2008; Liew and Owen, 2011; West et al., 2014). For instance, the lifetime risk of myeloid malignancy is about 44% with germ line RUNX1 mutations; however, the lifetime risk approaches nearly 100% for germ line CEBPA mutations (Godley, 2014). The importance of these inherited mutations in leukemia, their distinct clinical features, and the implications for treatment were recently recognized by the World Health Organization in their 2016 revision to the classification scheme for myeloid neoplasms and acute leukemia, which includes a new category of “myeloid neoplasms with germ line predisposition” (Arber et al., 2016; Table 1). Of note, mutations in several of the genes that confer leukemia predisposition (e.g., CEBPA, ETV6, GATA2, NF1, RUNX1, PTPN11, CBL, and RAS) are also frequently found in sporadic acute myeloid leukemia (AML) or myelodysplastic syndrome (MDS) (Cancer Genome Atlas Research Network et al., 2013). However, an important distinction, for which the mechanistic basis is not well understood, is that these inherited mutations are rarely initiating events in sporadic AML in adults but are, by definition, the initiating events in myeloid neoplasms with germ line predisposition.
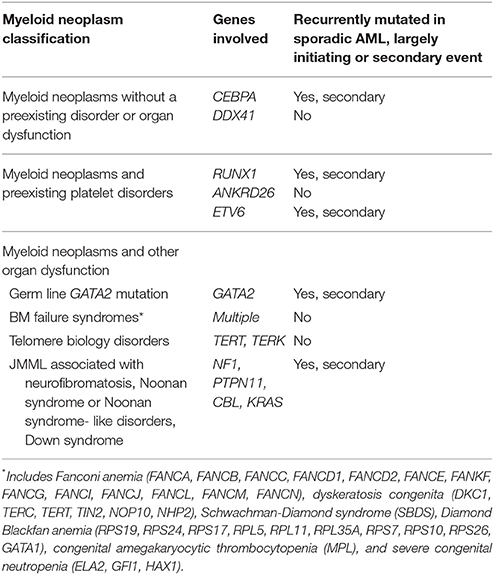
Table 1. World Health Organization classification of myeloid neoplasms with germ line predisposition.
Here we will focus on RUNX1, a leukemia predisposition gene that is also frequently mutated in sporadic leukemia. We will describe the inherited and acquired mutations in RUNX1 and speculate on why mutations in RUNX1 can be initiating events when inherited but are rarely initiating events in sporadic leukemia.
Somatic Mutations in RUNX1
RUNX1 encodes a sequence-specific transcription factor that is essential for HSC formation in the conceptus and is important for the differentiation of cells of the lymphoid, myeloid, and megakaryocytic lineages (Cai et al., 2000; Ichikawa et al., 2004; Growney et al., 2005; Tober et al., 2016). RUNX1 is a recurrent target of somatic mutations in de novo AML, myelodysplastic syndrome (MDS), acute lymphocytic leukemia (ALL), atypical chronic myeloid leukemia (aCML), and secondary AML (Mangan and Speck, 2011). There are two broad categories of mutations in RUNX1: monoallelic chromosomal translocations and mono- or biallelic somatic mutations. The most common chromosomal translocations are t(8;21)(q22;q22) in de novo AML and t(12;21)(p13;q22) in acute lymphocytic leukemia (B-ALL). Both of these translocations are initiating events in sporadic leukemia and can be acquired in utero as evidenced by their presence in Guthrie card samples from newborns diagnosed in childhood with AML or B-ALL (Wiemels et al., 1999, 2002). The t(8;21) and t(12;21) translocations generate fusion proteins (RUNX1-RUNX1T1 and ETV6-RUNX1, respectively) with neomorphic activity (Miyoshi et al., 1991, 1993; Chang et al., 1993; Nucifora et al., 1993; Golub et al., 1995; Romana et al., 1995; Shurtleff et al., 1995; Yergeau et al., 1997; Okuda et al., 1998; Wildonger and Mann, 2005; Schindler et al., 2009). Both translocations confer a favorable prognosis in their respective diseases (Grimwade et al., 1998; Byrd et al., 2002; Schlenk et al., 2003). AML with t(8;21)(q22;q22) or with inv(16)(p13;q22) or t(16)(p13;q22), which disrupt CBFB the non-DNA-binding partner of RUNX1, are included under the category of “AML with recurrent genetic abnormalities” in the 2016 WHO classification scheme (Arber et al., 2016) and together are often referred to as “core-binding factor acute myeloid leukemia” (CBF-AML).
Mono- and biallelic mutations in RUNX1 include deletions, missense, splicing, frameshift, and nonsense mutations. These mutations are mechanistically distinct from the chromosomal translocations and confer a worse prognosis (Osato et al., 1999; Imai et al., 2000; Harada et al., 2003; Steensma et al., 2005; Gelsi-Boyer et al., 2008; Kuo et al., 2009; Bejar et al., 2011; Mangan and Speck, 2011; Gaidzik et al., 2016). Some mutations truncate the RUNX1 protein N-terminal to or within the DNA-binding domain and consequently inactivate the protein. Other mutations confer weak dominant negative activity to RUNX1. For example, mutations in DNA-contacting residues that disrupt DNA binding without perturbing the structure of the DNA-binding domain behave as weakly dominant negative mutations (Michaud et al., 2002; Matheny et al., 2007; Owen C. J. et al., 2008; Preudhomme et al., 2009; Bluteau et al., 2011). The mechanism by which this occurs is not known but probably involves RUNX1 binding to and sequestering a limiting protein through an interaction that requires RUNX1 to be properly folded. A possible candidate for this limiting protein is the non-DNA-binding subunit partner of the RUNX proteins, CBFβ, which associates with the RUNX1 DNA-binding domain. Another type of dominant negative mutation removes the C-terminal transactivation domain while leaving the DNA-binding domain intact, which allows mutant RUNX1 to occupy its target sites and block occupancy and transactivation by full length RUNX proteins (RUNX1, RUNX2, and RUNX3; Harada et al., 2003; Satoh et al., 2008). For simplicity's sake, we will refer to both the weakly dominant negative and inactivating RUNX1 mutations as “mutations” to distinguish them from the chromosomal translocations. AML with mono- and biallelic RUNX1 mutations has been provisionally classified by the WHO as “AML with mutated RUNX1” (distinct from CBF-AML) to reflect the possible worse prognosis as compared to other AML types (Tang et al., 2009; Gaidzik et al., 2011, 2016; Schnittger et al., 2011; Mendler et al., 2012; Arber et al., 2016).
The order of mutation acquisition in sporadic AML can be discerned by whole-genome sequencing, which reveals not only which mutations are present but also the allelic fraction of each mutation. Clonal mutations, present in all leukemia cells, represent initiating events, while subclonal mutations are secondary events. Whereas mutations in epigenetic modifiers such as DNMT3A, ASXL1, IDH2, and TET2 have been consistently identified as early mutations in studies examining the temporal order of mutation acquisition in sporadic AML (Jan et al., 2012; Krönke et al., 2013; Corces-Zimmerman et al., 2014; Shlush et al., 2014; Hirsch et al., 2016; Papaemmanuil et al., 2016), RUNX1 mutations have rarely been identified as “early” or “initiating” mutations in these studies (Jan et al., 2012; Corces-Zimmerman et al., 2014; Shlush et al., 2014; Hirsch et al., 2016; Papaemmanuil et al., 2016). The largest and most recent analysis of the order of mutation acquisition was conducted using 1540 de novo AML samples and validated the previous identification of DNMT3A, ASXL1, IDH2, and TET2 as the earliest mutations in sporadic AML (Papaemmanuil et al., 2016). In this large analysis, as in prior smaller analyses, acquired RUNX1 mutations were not identified as the earliest mutations and were rarely clonal. In a calculation to determine the relative order of mutation acquisition based on pairwise precedences, sporadic RUNX1 mutations occurred 11th out of 28 genes analyzed. Thus, RUNX1 mutations in sporadic AML are usually intermediate secondary events that drive disease progression (Jan et al., 2012; Corces-Zimmerman et al., 2014; Shlush et al., 2014; Hirsch et al., 2016; Papaemmanuil et al., 2016).
Myelodysplastic syndrome (MDS) is a clonal hematopoietic disorder characterized by ineffective hematopoiesis and is a harbinger of AML in approximately 25–30% of patients (Tefferi and Vardiman, 2009; Steensma, 2015). MDS is characterized by cytopenias of one or more peripheral blood lineages, bone marrow cells with dysplastic features, and ≤19% blast cells (Arber et al., 2016). Mutations in RUNX1 are usually secondary events in MDS, although in a small number of patients they were identified as initiating events in clonal analyses (Papaemmanuil et al., 2013; Thota et al., 2014; da Silva-Coelho et al., 2017).
The paucity of initiating RUNX1 mutations is particularly striking in the age-related phenomenon of clonal hematopoiesis of indeterminant potential (CHIP) (Genovese et al., 2014; Jaiswal et al., 2014; Xie et al., 2014). CHIP is caused by a mutation in an HSC that causes it to selectively expand in the bone marrow relative to normal HSCs. The presence of CHIP is detected by an increase in the fraction of a mutated allele in peripheral blood (Busque et al., 2012; Genovese et al., 2014; Jaiswal et al., 2014; Xie et al., 2014; McKerrell et al., 2015). Despite having an expanded HSC clone, individuals with CHIP have normal hematopoiesis. The prevalence of CHIP increases with age—it is rare in individuals less than 40 years of age, increases to approximately 5–10% incidence in people over the age of 70, and reaches an incidence of 20% in individuals ≥90 years of age (Genovese et al., 2014; Jaiswal et al., 2014; McKerrell et al., 2015). CHIP confers a modestly elevated risk of MDS and AML (0.5–1% per year). The most common mutations in CHIP are the same as those identified as initiating mutations in sporadic adult AML: DNMT3A, TET2, and ASXL1 (Genovese et al., 2014; Jaiswal et al., 2014; Xie et al., 2014). CHIP is thought to represent a first step in leukemogenesis in adults that at a low, but discernably elevated, rate progresses to leukemia. Strikingly, in three separate studies not a single mutation was found in RUNX1 in individuals with CHIP (Genovese et al., 2014; Jaiswal et al., 2014; Xie et al., 2014). Mutations in several other germ line leukemia predisposition genes (CEBPA, GATA2, ETV6) were also rare or undetected.
RUNX1 mutations are more common in clonal cytopenia of undetermined significance (CCUS) (Kwok et al., 2015; Malcovati et al., 2017). CCUS is a subcategory of idiopathic cytopenias of undetermined significance (ICUS) (Valent et al., 2012; Cargo et al., 2015; Kwok et al., 2015), which include unexplained cytopenias that fail to meet the diagnostic criteria for MDS. CCUS is distinct from CHIP by virtue of its abnormal hematopoiesis. Approximately 2–4% of CCUS patients had RUNX1 mutations (Kwok et al., 2015; Malcovati et al., 2017).
In summary, mutations in RUNX1 are rarely initiating events in AML, have not been observed in CHIP, and have been identified at a low frequency in MDS and CCUS.
Inherited Mutations in RUNX1
Inherited mono-allelic RUNX1 mutations are associated with “familial platelet disorder with predisposition to AML” (FPD/AML) (Song et al., 1999). FPD/AML individuals usually present with mild to moderate thrombocytopenia and bleeding disorders (epistaxis, easy bruising, excessive bleeding during minor surgery, menorrhagia) (Luddy et al., 1978). The bleeding disorder is caused by impaired proplatelet formation, functional aspirin-like platelet activation defects, and abnormal megakaryocyte differentiation and polyploidization. FPD/AML individuals also have a strikingly elevated (~44% lifetime risk) of MDS or acute leukemia (AML and to a lesser extent T-ALL) (Godley, 2014). Since the bleeding disorder is usually manageable and in most individuals does not greatly compromise the quality of life, bone marrow transplantation from a non-affected family member or an unrelated matched donor is generally not recommended unless the patient has leukemia (University of Chicago Hematopoietic Malignancies Cancer Risk Team, 2016).
The ability to monitor HSC expansion by changes in variant allele frequencies in FPD/AML individuals by next generation sequencing may offer the opportunity to intervene at the pre-leukemic stage, prior to the appearance of overt MDS or leukemia. Knowing when to intervene and treat a healthy individual at an early stage of disease, however, requires knowing which secondary mutations are likely to lead to disease progression. In sporadic leukemia, it is assumed that the most potent cooperating mutations are those that most frequently co-occur due to selection. For RUNX1 in sporadic leukemia, these include mutations in ASXL1, BCOR, KMD2A, PHF6, and SRFS2, and less significantly in IDH2, STAG2, SF3B1, and trisomy 13 (Gaidzik et al., 2016; Papaemmanuil et al., 2016). On the other hand, RUNX1 mutations are inversely correlated with mutations in CEBPA, NPM1, TP53, t(8;21)(q22;q22), inv(16)(p13;q22)/t(16)(p13;q22), and t(15;17)(q24;q21), presumably because the mutations are either functionally redundant or antagonistic. Other common MDS or AML mutations (e.g., in DNMT3A) were neither enriched nor inversely correlated with RUNX1 mutations.
Unfortunately, the rarity of FPD/AML makes definitive large-scale sequencing studies impossible, and the handful of smaller studies that have been conducted reported somewhat different results. One group sequenced AML samples from 9 Japanese FPD/AML patients and reported somatic mutations in CDC25C in 4 patients, concluding that somatic mutations in CDC25C (which are rarely observed in sporadic AML) were the most common genetic event in AML arising from FPD/AML (Yoshimi et al., 2014; Sakurai et al., 2016). A second group analyzed somatic mutations in 8 leukemia patients from four French FPD/AML families, identified loss of function mutations in the second RUNX1 allele in 6 patients, and concluded that mutation of the remaining wild type RUNX1 allele was the most common secondary event (Antony-Debré et al., 2016). Strikingly, the French group found no mutations in CDC25C. A third group (Churpek et al.) from the United States analyzed somatic mutations in 7 FPD/AML patients with MDS or AML and found no mutations in either CDC25C or in the second RUNX1 allele, but instead, identified mutations in a collection of other genes including BCOR, PHF6, DNMT3A, TET2, CREBBP, U2AF1, NUP214, SMC3, and PDS5B (Churpek et al., 2015).
In the Churpek et al. study, the peripheral blood of 9 FPD/AML individuals with no evidence of leukemia was also analyzed (Churpek et al., 2015). Six of these 9 individuals had clonal hematopoiesis, and a missense mutation in DNMT3A was found in one person (Churpek et al., 2015). A DNMT3A mutation was also identified in an asymptomatic FPD/AML individual in a separate study (Antony-Debré et al., 2016). The asymptomatic FPD/AML individuals with evidence of clonal hematopoiesis ranged in age from 8 to 54 (Churpek et al., 2015), whereas CHIP in the general population is extremely rare in this age group (Genovese et al., 2014; Jaiswal et al., 2014; Xie et al., 2014). It appears that the acquisition of secondary mutations is greatly accelerated in FPD/AML individuals and presumably contributes to the elevated risk of leukemia in these patients. An important question raised by these studies is why clonal hematopoiesis is so greatly accelerated in individuals with FPD/AML. In the next several sections we will describe experimental data that begin to address this question.
Defects in DNA Repair Pathways in RUNX1 Mutant Cells
Inefficient DNA repair could contribute to the rapid accumulation of mutations seen in non-leukemic FPD/AML patients. This could result from the dysregulated expression or activity of proteins in DNA repair pathways, which include mismatch repair (MMR), base excision (BER), nucleotide excision (NER), double-strand break repair [homologous recombination (HR), non-homologous end joining (NHEJ), alternative non-homologous end-joining (Alt-NHEJ)], and interstrand DNA crosslink repair by the Fanconi Anemia (FA) / BReast CAncer susceptibility (BRCA) pathway (Cheung and Taniguchi, 2017). Thus far, RUNX1 has been implicated in regulating the BER, HR, and FA/BRCA pathways.
Most evidence for RUNX1's role in DNA repair has been generated using cells expressing the neomorphic RUNX1-RUNX1T1 (AML1-ETO) protein. Several studies showed that expression of RUNX1-RUNX1T1 in primary HSPCs or cell lines resulted in down regulation of a number of genes involved in BER, including OGG1 which encodes the primary enzyme responsible for excising oxidized bases (Alcalay et al., 2003; Krejci et al., 2008; Liddiard et al., 2010; Forster et al., 2016). RUNX1-RUNX1T1 was shown to occupy the OGG1 gene and presumably directly regulates OGG1 expression (Krejci et al., 2008; Forster et al., 2016). Functional defects in BER were observed in RUNX1-RUNX1T1 expressing cells, such as slow repair of DNA damage by alkylating agents (Alcalay et al., 2003) and accumulation of somatic mutations more rapidly over time (Forster et al., 2016).
Inefficient DNA repair by the HR pathway has also been documented in RUNX1-RUNX1T1 expressing cells. RUNX1-RUNX1T1 expressing cells are very sensitive to poly (ADP-ribose) polymerase (PARP) inhibitors (Esposito et al., 2015), which have potent activity specifically on cells with defective HR repair (Helleday, 2011). Treatment with PARP inhibitors suppressed the colony forming ability of RUNX1-RUNX1T1 expressing cells, consistent with a defect in HR repair (Esposito et al., 2015). A very early event in the repair of DNA double-strand breaks and a surrogate marker for breaks is phosphorylation of the histone variant H2AX (the phosphorylated form is γ-H2AX) by the ATM serine-threonine kinase (Mah et al., 2010). DNA double-strand breaks trigger a cascade of events that recruit DNA repair proteins, including the recombinase RAD51 through BRCA1 and BRCA2. RUNX1-RUNX1T1 expressing cells cultured in vitro gradually acquired more γ-H2AX foci than normal cells over time, indicating that they more rapidly accumulated unrepaired double-strand breaks (Krejci et al., 2008; Wichmann et al., 2015). Following acute induction of DNA damage, the γ-H2AX foci that formed disappeared more slowly, indicative of slower resolution or repair of γ-H2AX+ double strand breaks (Esposito et al., 2015). The slow repair of DNA correlated with decreased recruitment of the recombinase RAD51 to the DNA damage sites (Esposito et al., 2015). This deficiency in HR in RUNX1-RUNX1T1 expressing cells was associated with lower expression of genes responsible for HR, including BRCA1, BRCA2, ATM, and RAD51 (Esposito et al., 2015). Whether RUNX1-RUNX1T1 regulates these DNA repair genes directly or indirectly is unclear.
Defects in DNA repair have also been observed with the types of RUNX1 mutations found in FPD/AML. Retroviral transduction of a dominant negative truncated RUNX1 protein lacking its transactivation domain into HSPCs resulted in a greater proportion of cells with evidence of DNA damage, as detected by the presence of an increased number of cells containing γ-H2AX+ foci and slower decay of γ-H2AX+ foci following DNA damage induction (Satoh et al., 2012). The defect in DNA repair was accompanied by the downregulation of several DNA repair genes, including RAD51 and the growth arrest and DNA damage gene GADD45. The RUNX proteins may also directly regulate the FA/BRCA pathway (Krejci et al., 2008; Wang et al., 2014). The FA/BRCA pathway is essential for repairing interstrand crosslinks in DNA. Inherited mutations in multiple FA/BRCA pathway genes cause bone marrow failure and predispose to myeloid leukemia and other cancers (Cheung and Taniguchi, 2017; Table 1). The FA/BRCA pathway is strongly activated by the crosslinking agent mitomycin C. When RUNX1 and its homolog RUNX3 were together deleted or knocked down, the sensitivity of cells to mitomycin C increased, indicating inefficient repair of the damage (Wang et al., 2014). RUNX1 was shown to co-immunoprecipitate with FANCD2, a protein in the FA/BRCA pathway that localizes to sites on interstrand crosslinks (Wang et al., 2014). Recruitment of FANCD2 to DNA decreased upon combined knockdown of RUNX1 and RUNX3, and repair was depressed (Wang et al., 2014).
In addition to direct effects on DNA repair pathways, RUNX1 mutations may promote the survival of HSCs with somatic mutations. For example, lower p53 levels have been documented in RUNX1 deficient HSPCs (Cai et al., 2015). The p53 transcription factor protects cells from DNA damage by arresting the cell cycle to allow DNA repair to take place and by inducing apoptosis and senescence of unrepaired cells. The reduction in p53 protein levels in RUNX1 deficient HSCs was not accompanied by reduced Tp53 mRNA levels; therefore, the mechanism of the reduction is post-transcriptional (Cai et al., 2015). Other studies showed that RUNX1 forms a complex with p300-p53 to acetylate p53 and thereby activate p53 target genes (Wu et al., 2013). Hence loss of RUNX1 could affect both p53 levels and activity. RUNX1 deficient HSCs contained a decreased percentage of apoptotic cells, both in the absence and presence of DNA damaging agents (Motoda et al., 2007; Cai et al., 2011, 2015). Thus, inefficient elimination of cells that have acquired DNA damage through impaired p53 activity (Cai et al., 2015) could contribute to the accumulation of HSCs with unrepaired DNA damage and acquired secondary mutations in FPD/AML. Other survival pathways and apoptotic pathways are also likely to be dysregulated in RUNX1 deficient HSCs, as increasing p53 levels by inhibiting its interaction with MDM2 using the MDM2:p53 inhibitor nutlin-3 did not completely correct the low apoptotic phenotype (Cai et al., 2015). All of the above-mentioned studies were performed with cells that have more severe perturbations in functional RUNX1 than would be expected with the mono-allelic mutations in FPD/AML, and the DNA repair and apoptotic defects are likely to be more profound in experimental models. Nevertheless, the median age for leukemia development in FPD/AML individuals is 33 years (Owen C. J. et al., 2008). Thus, even minor defects in DNA repair could contribute to the increased accumulation of mutations over a period of several decades.
Forces that May Drive Clonal Selection of HSCs with Secondary Mutations in FPD/AML
For an HSC with a somatic mutation to clonally expand, there must be selective pressures favoring that HSC that provide it with a competitive advantage. The nature of selective pressures can change with age; for example, the selective pressure that drives the expansion of somatically mutated cells is thought to increase with age and contribute to the age-associated increased incidence of cancer (Rozhok and DeGregori, 2016). An aged inflammatory niche, for instance, was a critical determinant in selecting for HSPCs with oncogenic driver mutations in mice (Henry et al., 2015). The ability of HSPCs transduced with oncogenes (NRASV12, BCR-ABL, and MYC) to promote leukemogenesis was greatly enhanced by transplantation into old recipient mice, whereas when transplanted into young hosts, leukemogenesis was largely suppressed (Henry et al., 2015). The expansion of the transduced HSPCs in the aged bone marrow niche could be prevented by transgene expression of the anti-inflammatory mediators α-1-antitrypsin (AAT), or interleukin-37 (IL-37) (Henry et al., 2015). These findings suggest that not only do mutations need to be acquired in HSCs but also that the leukemic clone must be in the inflammatory environment of an aged bone marrow for the mutations to provide a fitness advantage that can be selected for. Consistent with this, recent studies showed that inflammatory cytokines can promote the selective in vitro growth of human AML cells. In a large functional screen of primary human AML samples, several inflammatory cytokines, including IL-1, promoted the growth of AML progenitors while suppressing the growth of normal HSPCs (Carey et al., 2017). Strikingly, despite genetic and clinical heterogeneity, the growth of 40 out of 60 primary AML samples was stimulated by IL-1, suggesting that selective growth driven by inflammatory cytokines is a common feature of many AML cells (Carey et al., 2017). Differentiated myeloid cells were identified as the primary source of IL-1, highlighting the critical role that an inflammatory niche may play in leukemogenesis (Carey et al., 2017).
Increased production of inflammatory cytokines by myeloid cells has been observed in individuals with CHIP and in mouse models of the mutations found in CHIP. Evidence of inflammation and increased serum levels of IL-8 were identified in CHIP associated with mutations in TET2 (Jaiswal et al., 2017). Myeloid cells in mice were shown to be a source of inflammatory cytokines, as a myeloid cell specific disruption of Tet2 with Lyz2-Cre elevated mRNAs encoding multiple chemokines and inflammatory cytokines; furthermore, endotoxin stimulation of Tet2 deficient macrophages resulted in more robust secretion of IL-1β and IL-6 (Cull et al., 2017; Fuster et al., 2017; Jaiswal et al., 2017). Hence, mutations in HSCs may increase the production of inflammatory cytokines by downstream myeloid lineage cells, which could potentially feedback on and selectively promote the growth of the mutated HSCs.
Clinical evidence hints that elevated inflammation may contribute to disease severity in FPD/AML individuals. In one FPD/AML pedigree, affected family members were reported to have eczema, the severity of which correlated directly with the severity of thrombocytopenia (Sorrell et al., 2012). Strikingly, eczema was most severe in those family members who went on to develop frank leukemia (Sorrell et al., 2012). In another study, hypersensitivity to G-CSF was observed in peripheral blood mononuclear cells from an FPD/AML patient (Chin et al., 2016). This correlates with findings in mouse models, where increased sensitivity of HSPCs to G-CSF upon mono- or biallelic deletion of Runx1 was proposed to result from an increase in STAT3 signaling (Chin et al., 2016; Lam et al., 2016).
Further suggestive evidence that mutations in RUNX1 may elevate inflammation can be seen in studies of its homolog RUNX3, which binds the same DNA sequence as RUNX1 and is a well-documented negative regulator of inflammation. As is well reviewed elsewhere, genome wide association studies (GWAS) linked RUNX3 with diseases of inflammation in humans including ulcerative colitis, celiac disease, ankylosing spondylitis, psoriasis, and asthma (Lotem et al., 2015). Runx3 knockout mice exhibit spontaneous colitis and lung inflammation, in part due to dysregulated TGF-β signaling (Brenner et al., 2004; Fainaru et al., 2004). RUNX1 also negatively regulates inflammatory signaling in the lung (Tang et al., 2017). Deletion of Runx1 in lung epithelium resulted in constitutive activation of NF-κB pro-inflammatory signaling and increased susceptibility to LPS-induced acute lung injury in vivo (Tang et al., 2017). Given these findings in lung epithelium, germ line mutations in RUNX1 could have more pleiotropic effects in patients with FPD/AML than previously appreciated.
The mechanisms by which RUNX1 regulates inflammation are unclear. RUNX1 is reported to have opposing effects on NF-κB signaling output due to interactions with different members of the NF-κB signaling pathway. Nakagawa et al. showed that RUNX1 interacted with the IκB kinase (IKK) complex in the cytoplasm, and deletion of Runx1 activated NF-κB signaling (Nakagawa et al., 2011). The ability of RUNX1 to inhibit IKK activity was lost in three RUNX1 mutants identified in MDS patients (Nakagawa et al., 2011). However, a different group reported a contrasting activity of RUNX1 in regulating NF-κB, in that knock down of RUNX1 in myeloid cell lines and activated primary mouse peritoneal macrophages attenuated NF-κB signaling (Luo et al., 2016). The latter study also showed that RUNX1 binds to p50 (a NF-κB family member) in the nucleus and may lead to enhanced NF-κB signaling via recruitment of RNA polymerase II at some p50-occupied sites (Luo et al., 2016). Further, use of a RUNX1 inhibitor provided some protection against LPS-induced septic shock in an in vivo murine model (Luo et al., 2016). Therefore, RUNX1 appears to interface with the NF-κB pathway at multiple points and to be capable of both interfering with and augmenting NF-κB signaling.
RUNX proteins can also modulate inflammatory signaling through their regulation of T cell development and function (Djuretic et al., 2009; Wong et al., 2011). RUNX1 and RUNX3 influence the development and function of TH1, TH2, TREG, and TH17 cells, which regulate autoimmunity, asthma, allergic responses, and tumor immunity (Djuretic et al., 2007, 2009; Naoe et al., 2007; Ono et al., 2007; Zhang et al., 2008; Bruno et al., 2009; Wong et al., 2011). T cell specific deletion of Runx1 caused spontaneous hyperactivation of CD4+ T cells resulting in severe lung inflammation that evolved into a lethal systemic inflammatory disease (Wong et al., 2012).
Putting all this together, we speculate that systemic inflammation may be elevated in FPD/AML and that an inflammatory bone marrow microenvironment may provide a positive selective pressure for HSCs that have acquired secondary mutations.
Effects of RUNX1 Mutations on HSCs
The mutations in epigenetic regulator genes that initiate AML and predominate in CHIP have the capability of selectively expanding HSCs. This phenomenon of clonal HSC expansion has been reproduced in several mouse models. Deletion of Dnmt3a in mice skewed the balance of self-renewal versus differentiation of HSCs, causing an accumulation of HSCs in the bone marrow, inefficient output of HSCs to differentiated hematopoietic progeny in the periphery, and a repopulation advantage of Dnmt3a deficient HSCs over normal HSCs in transplant experiments (Challen et al., 2012; Shlush et al., 2014). Mutations in Tet2 similarly conferred a competitive advantage to murine HSCs, allowing them to outcompete normal HSCs in transplantation experiments (Ko et al., 2011; Moran-Crusio et al., 2011; Quivoron et al., 2011).
RUNX1 mutant HSCs do not appear to enjoy the same competitive advantage over normal HSCs as do HSCs with Dnmt3a or Tet2 mutations. RUNX1 mutations may require the context of additional mutations in order to provide a fitness advantage for the leukemic clone. Initial reports showed that deletion of Runx1 in hematopoietic cells caused an expansion of HSPCs (lineage negative Sca1+ Kit+ cells) and committed myeloid progenitors in the bone marrow (Ichikawa et al., 2004; Growney et al., 2005). However, the majority of studies in which careful analyses were carried out on functional long-term repopulating HSCs (LT-HSCs) reported modest, 2-4-fold decreases in their frequency upon Runx1 mutation (Sun and Downing, 2004; Jacob et al., 2009; Cai et al., 2011). For example, a pan-hematopoietic homozygous deletion of Runx1 resulted in a 3-4-fold decrease in functional LT-HSCs (measured in limiting dilution transplantation experiments) in the bone marrow of both young and aged mice (Jacob et al., 2009; Cai et al., 2011). Similarly, monoallelic germ line mutations in Runx1 reduced the frequency of functional LT-HSCs in the bone marrow by about 50% (Sun and Downing, 2004). Runx1 deficient (Runx1Δ/Δ), Runx1+/−, and wild type HSCs performed equivalently well in serial transplantation experiments, in that by the fourth round of transplantation, HSCs of all three genotypes were essentially exhausted, and hence had similar self-renewal potential (Sun and Downing, 2004; Cai et al., 2011). By comparison, Dnmt3a deficient HSCs expanded with each subsequent round of transplantation (Challen et al., 2012). Therefore, the inability of RUNX1 mutations to induce clonal HSC expansion like that seen with DNMT3A and TET2 mutations may account for the rarity of RUNX1 mutations as initiating events in AML and in CHIP.
An interesting and potentially clinically consequential feature of RUNX1 mutant HSPCs is that they exhibit a greater selective advantage over normal HSPCs following exposure to radiation. Irradiation of a mixture of Runx1 deficient (Runx1Δ/Δ) and normal bone marrow cells followed by transplantation into mice resulted in a greater selective expansion of Runx1 deficient HSPCs (lineage negative Sca1+ Kit+ cells) and myeloid lineage cells in the bone marrow compared to irradiated wild type or non-irradiated Runx1 deficient cells (Cai et al., 2015). This was likely due to the increased resistance of Runx1 deficient HSPCs to endogenous and genotoxic stress (Cai et al., 2015). A very similar phenomenon of radiation-dependent HSC expansion was also observed in HSPCs with a Tp53 mutation, consistent with this interpretation (Marusyk et al., 2010). Runx1 deficient HSPCs were less apoptotic, grew more slowly than normal HSCs, had a lower rate of protein translation, and had markedly decreased ribosome biogenesis. Consistent with decreased ribosome biogenesis, Runx1 deficient HSPCs were smaller than normal HSPCs, and preliminary data suggested that this small cell phenotype was shared with HSPCs from FPD/AML individuals (Cai et al., 2015). This stress resistant, slow growth, low apoptotic phenotype could presumably confer resistance to treatment and may explain why RUNX1 mutations confer a relatively poor prognosis in AML. It may also explain the observation that RUNX1 mutations occurred at a higher frequency in MDS patients previously exposed to low-level radiation as compared to all MDS patients (Harada et al., 2003; Zharlyganova et al., 2008).
Conclusions
It was originally thought that inherited RUNX1 mutations cause leukemia predisposition because every HSC in an FPD/AML individual is already one mutation along the path to AML. However, it is likely that inherited RUNX1 mutations play a more active role in promoting leukemia progression. The dysregulation of DNA repair and decreased apoptosis of RUNX1 mutant HSCs along with an increased inflammatory microenvironment may contribute to the markedly increased incidence of MDS, AML, and T-ALL in FPD/AML individuals. We envision a model whereby RUNX1 mutations increase the rate at which secondary mutations are acquired; moreover, increased inflammatory signals delivered by RUNX1 mutant myeloid lineage cells or other cells in the RUNX1 mutant bone marrow microenvironment provide a selective pressure that confers a competitive advantage to FPD/AML HSCs that have acquired secondary mutations (Figure 1). On the other hand, in a normal individual a single HSC with a RUNX1 mutation does not have a competitive advantage over normal HSCs and hence does not expand and initiate leukemia.
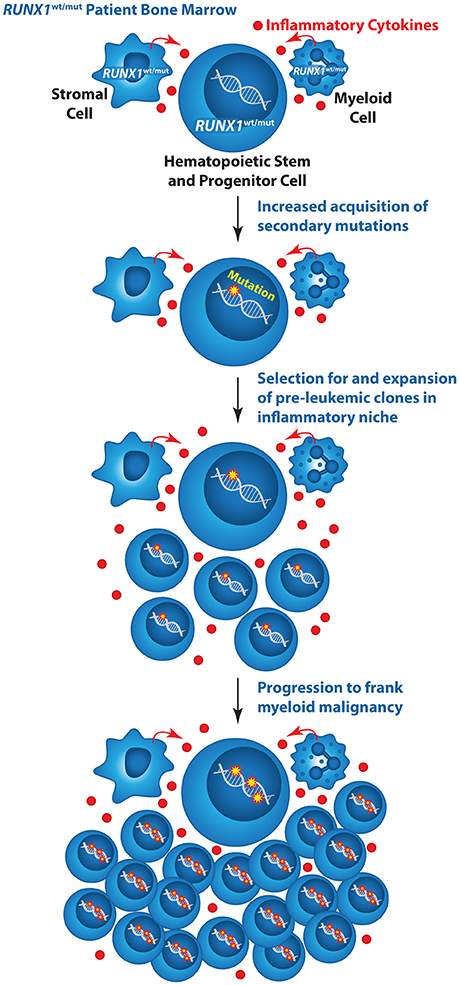
Figure 1. Model illustrating the mechanism by which inherited mutations in RUNX1 could elevate the risk of AML in FPD/AML individuals. Wildtype (wt), mutant (mut).
The initiation and evolution of leukemia in FPD/AML individuals can be carefully followed by next generation sequencing. Individuals diagnosed with FPD/AML could be monitored on a regular basis for evidence of clonal HSC expansion driven by acquired secondary mutations and treated before they progress to frank AML. However, we still do not know which secondary mutations are the most deleterious and whether they are the same as or different than the co-occurring mutations in sporadic AML. For example, DNMT3A mutations have been reported in two asymptomatic FPD/AML individuals (Churpek et al., 2015; Antony-Debré et al., 2016). Does the presence of a DNMT3A mutation, which in a normal individual only modestly increases the risk of leukemia, confer a much greater risk in the context of an inherited RUNX1 mutation? More data and longitudinal studies correlating the stepwise accumulation of mutations in individuals with FPD/AML with clinical outcome are needed to predict which mutations most likely portend a rapid progression to MDS or AML. Mechanistic studies in animal and cell models will also be necessary to understand what is driving leukemia progression in FPD/AML and to identify strategies that can avert, delay, or reverse this progression.
Author Contributions
All authors listed have made a substantial, direct and intellectual contribution to the work, and approved it for publication.
Funding
National Institutes of Health grants R01CA149976 (NS), T32HL007439 (DB), and F30CA196123 (DB) as well as the Abramson Family Cancer Research Institute's Patel Scholar Award (DB) supported this work. Work on this project is also funded by the Babich Family Foundation.
Conflict of Interest Statement
The authors declare that the research was conducted in the absence of any commercial or financial relationships that could be construed as a potential conflict of interest.
References
Alcalay, M., Meani, N., Gelmetti, V., Fantozzi, A., Fagioli, M., Orleth, A., et al. (2003). Acute myeloid leukemia fusion proteins deregulate genes involved in stem cell maintenance and DNA repair. J. Clin. Invest. 112, 1751–1761. doi: 10.1172/JCI17595
Antony-Debré, I., Duployez, N., Bucci, M., Geffroy, S., Micol, J. B., Renneville, A., et al. (2016). Somatic mutations associated with leukemic progression of familial platelet disorder with predisposition to acute myeloid leukemia. Leukemia 30, 999–1002. doi: 10.1038/leu.2015.236
Arber, D. A., Orazi, A., Hasserjian, R., Thiele, J., Borowitz, M. J., Le Beau, M. M., et al. (2016). The 2016 revision to the World Health Organization classification of myeloid neoplasms and acute leukemia. Blood 127, 2391–2405. doi: 10.1182/blood-2016-03-643544
Bejar, R., Stevenson, K., Abdel-Wahab, O., Galili, N., Nilsson, B., Garcia-Manero, G., et al. (2011). Clinical effect of point mutations in myelodysplastic syndromes. N. Engl. J. Med. 364, 2496–2506. doi: 10.1056/NEJMoa1013343
Bluteau, D., Gilles, L., Hilpert, M., Antony-Debré, I., James, C., Debili, N., et al. (2011). Down-regulation of the RUNX1-target gene NR4A3 contributes to hematopoiesis deregulation in familial platelet disorder/acute myelogenous leukemia. Blood 118, 6310–6320. doi: 10.1182/blood-2010-12-325555
Brenner, O., Levanon, D., Negreanu, V., Golubkov, O., Fainaru, O., Woolf, E., et al. (2004). Loss of Runx3 function in leukocytes is associated with spontaneously developed colitis and gastric mucosal hyperplasia. Proc. Natl. Acad. Sci. U.S.A. 101, 16016–16021. doi: 10.1073/pnas.0407180101
Bruno, L., Mazzarella, L., Hoogenkamp, M., Hertweck, A., Cobb, B. S., Sauer, S., et al. (2009). Runx proteins regulate Foxp3 expression. J. Exp. Med. 206, 2329–2337. doi: 10.1084/jem.20090226
Busque, L., Patel, J. P., Figueroa, M. E., Vasanthakumar, A., Provost, S., Hamilou, Z., et al. (2012). Recurrent somatic TET2 mutations in normal elderly individuals with clonal hematopoiesis. Nat. Genet. 44, 1179–1181. doi: 10.1038/ng.2413
Byrd, J. C., Mrózek, K., Dodge, R. K., Carroll, A. J., Edwards, C. G., Arthur, D. C., et al. (2002). Pretreatment cytogenetic abnormalities are predictive of induction success, cumulative incidence of relapse, and overall survival in adult patients with de novo acute myeloid leukemia: results from Cancer and Leukemia Group B (CALGB 8461). Blood 100, 4325–4336. doi: 10.1182/blood-2002-03-0772
Cai, X., Gao, L., Teng, L., Ge, J., Oo, Z., Kumar, A. R., et al. (2015). Runx1 deficiency decreases ribosome biogenesis and confers stress resistance to hematopoietic stem and progenitor cells. Cell Stem Cell 17, 165–177. doi: 10.1016/j.stem.2015.06.002
Cai, X., Gaudet, J. J., Mangan, J. K., Chen, M. J., De Obaldia, M. E., Oo, Z., et al. (2011). Runx1 loss minimally impacts long-term hematopoietic stem cells. PLoS ONE 6:e28430. doi: 10.1371/journal.pone.0028430
Cai, Z., de Bruijn, M. F. T. R., Ma, X., Dortland, B., Luteijn, T., Downing, J. R., et al. (2000). Haploinsufficiency of AML1/CBFA2 affects the embryonic generation of mouse hematopoietic stem cells. Immunity 13, 423–431. doi: 10.1016/S1074-7613(00)00042-X
Cancer Genome Atlas Research Network, Ley, T. J., Miller, C., Ding, L., Raphael, B. J., Mungall, A. J., et al. (2013). Genomic and epigenomic landscapes of adult de novo acute myeloid leukemia. N. Engl. J. Med. 368, 2059–2074. doi: 10.1056/NEJMoa1301689
Carey, A., Edwards, D. K., Eide, C. A., Newell, L., Traer, E., Medeiros, B. C., et al. (2017). Identification of Interleukin-1 by functional screening as a key mediator of cellular expansion and disease progression in acute Myeloid Leukemia. Cell Rep. 18, 3204–3218. doi: 10.1016/j.celrep.2017.03.018
Cargo, C. A., Rowbotham, N., Evans, P. A., Barrans, S. L., Bowen, D. T., Crouch, S., et al. (2015). Targeted sequencing identifies patients with preclinical MDS at high risk of disease progression. Blood 126, 2362–2365. doi: 10.1182/blood-2015-08-663237
Challen, G. A., Sun, D., Jeong, M., Luo, M., Jelinek, J., Berg, J. S., et al. (2012). Dnmt3a is essential for hematopoietic stem cell differentiation. Nat. Genet. 44, 23–31. doi: 10.1038/ng.1009
Chang, K. S., Fan, Y. H., Stass, S. A., Estey, E. H., Wang, G., Trujillo, J. M., et al. (1993). Expression of AML1-ETO fusion transcripts and detection of minimal residual disease in t(8;21)-positive acute myeloid leukemia. Oncogene 8, 983–988.
Cheung, R. S., and Taniguchi, T. (2017). Recent insights into the molecular basis of Fanconi anemia: genes, modifiers, and drivers. Int. J. Hematol. 106, 335–344. doi: 10.1007/s12185-017-2283-4
Chin, D. W., Sakurai, M., Nah, G. S., Du, L., Jacob, B., Yokomizo, T., et al. (2016). RUNX1 haploinsufficiency results in granulocyte colony-stimulating factor hypersensitivity. Blood Cancer J. 6:e379. doi: 10.1038/bcj.2015.105
Churpek, J. E., Pyrtel, K., Kanchi, K. L., Shao, J., Koboldt, D., Miller, C. A., et al. (2015). Genomic analysis of germ line and somatic variants in familial myelodysplasia/acute myeloid leukemia. Blood 126, 2484–2490. doi: 10.1182/blood-2015-04-641100
Corces-Zimmerman, M. R., Hong, W. J., Weissman, I. L., Medeiros, B. C., and Majeti, R. (2014). Preleukemic mutations in human acute myeloid leukemia affect epigenetic regulators and persist in remission. Proc. Natl. Acad. Sci. U.S.A. 111, 2548–2553. doi: 10.1073/pnas.1324297111
Cull, A. H., Snetsinger, B., Buckstein, R., Wells, R. A., and Rauh, M. J. (2017). Tet2 restrains inflammatory gene expression in macrophages. Exp. Hematol. 55, 56–70.e13. doi: 10.1016/j.exphem.2017.08.001
da Silva-Coelho, P., Kroeze, L. I., Yoshida, K., Koorenhof-Scheele, T. N., Knops, R., van de Locht, L. T., et al. (2017). Clonal evolution in myelodysplastic syndromes. Nat. Commun. 8:15099. doi: 10.1038/ncomms15099
Djuretic, I. M., Cruz-Guilloty, F., and Rao, A. (2009). Regulation of gene expression in peripheral T Cells by Runx Transcription Factors. Adv. Immunol. 104, 1–23. doi: 10.1016/s0065-2776(08)04001-7
Djuretic, I. M., Levanon, D., Negreanu, V., Groner, Y., Rao, A., and Ansel, K. M. (2007). Transcription factors T-bet and Runx3 cooperate to activate Ifng and silence Il4 in T helper type 1 cells. Nat. Immunol. 8, 145–153. doi: 10.1038/ni1424
Esposito, M. T., Zhao, L., Fung, T. K., Rane, J. K., Wilson, A., Martin, N., et al. (2015). Synthetic lethal targeting of oncogenic transcription factors in acute leukemia by PARP inhibitors. Nat. Med. 21, 1481–1490. doi: 10.1038/nm.3993
Fainaru, O., Woolf, E., Lotem, J., Yarmus, M., Brenner, O., Goldenberg, D., et al. (2004). Runx3 regulates mouse TGF-β-mediated dendritic cell function and its absence results in airway inflammation. EMBO J. 23, 969–979. doi: 10.1038/sj.emboj.7600085
Forster, V. J., Nahari, M. H., Martinez-Soria, N., Bradburn, A. K., Ptasinska, A., Assi, S. A., et al. (2016). The leukemia-associated RUNX1/ETO oncoprotein confers a mutator phenotype. Leukemia 30, 250–253. doi: 10.1038/leu.2015.133
Fuster, J. J., MacLauchlan, S., Zuriaga, M. A., Polackal, M. N., Ostriker, A. C., Chakraborty, R., et al. (2017). Clonal hematopoiesis associated with TET2 deficiency accelerates atherosclerosis development in mice. Science 355, 842–847. doi: 10.1126/science.aag1381
Gaidzik, V. I., Bullinger, L., Schlenk, R. F., Zimmermann, A. S., Röck, J., Paschka, P., et al. (2011). RUNX1 mutations in acute myeloid leukemia: results from a comprehensive genetic and clinical analysis from the AML study group. J. Clin. Oncol. 29, 1364–1372. doi: 10.1200/JCO.2010.30.7926
Gaidzik, V. I., Teleanu, V., Papaemmanuil, E., Weber, D., Paschka, P., Hahn, J., et al. (2016). RUNX1 mutations in acute myeloid leukemia are associated with distinct clinico-pathologic and genetic features. Leukemia. 30:2282. doi: 10.1038/leu.2016.126
Gelsi-Boyer, V., Trouplin, V., Adélaïde, J., Aceto, N., Remy, V., Pinson, S., et al. (2008). Genome profiling of chronic myelomonocytic leukemia: frequent alterations of RAS and RUNX1 genes. BMC Cancer 8:299. doi: 10.1186/1471-2407-8-299
Genovese, G., Kähler, A. K., Handsaker, R. E., Lindberg, J., Rose, S. A., Bakhoum, S. F., et al. (2014). Clonal hematopoiesis and blood-cancer risk inferred from blood DNA sequence. N. Engl. J. Med. 371, 2477–2487. doi: 10.1056/NEJMoa1409405
Godley, L. A. (2014). Inherited predisposition to acute myeloid leukemia. Semin. Hematol. 51, 306–321. doi: 10.1053/j.seminhematol.2014.08.001
Golub, T. R., Barker, G. F., Bohlander, S. K., Hiebert, S., Ward, D. C., Bray-Ward, P., et al. (1995). Fusion of the TEL gene on 12p13 to the AML1 gene on 21q22 in acute lymphoblastic leukemia. Proc. Natl. Acad. Sci. U.S.A. 92, 4917–4921.
Grimwade, D., Walker, H., Oliver, F., Wheatley, K., Harrison, C., Harrison, G., et al. (1998). The importance of diagnostic cytogenetics on outcome in AML: analysis of 1,612 patients entered into the MRC AML 10 trial. The Medical Research Council Adult and Children's Leukaemia Working Parties. Blood 92, 2322–2333
Growney, J. D., Shigematsu, H., Li, Z., Lee, B. H., Adelsperger, J., Rowan, R., et al. (2005). Loss of Runx1 perturbs adult hematopoiesis and is associated with a myeloproliferative phenotype. Blood 106, 494–504. doi: 10.1182/blood-2004-08-3280
Harada, H., Harada, Y., Tanaka, H., Kimura, A., and Inaba, T. (2003). Implications of somatic mutations in the AML1 gene in radiation-associated and therapy-related myelodysplastic syndrome/acute myeloid leukemia. Blood 101, 673–680. doi: 10.1182/blood-2002-04-1010
Helleday, T. (2011). The underlying mechanism for the PARP and BRCA synthetic lethality: clearing up the misunderstandings. Mol. Oncol. 5, 387–393. doi: 10.1016/j.molonc.2011.07.001
Henry, C. J., Casás-Selves, M., Kim, J., Zaberezhnyy, V., Aghili, L., Daniel, A. E., et al. (2015). Aging-associated inflammation promotes selection for adaptive oncogenic events in B cell progenitors. J. Clin. Invest. 125, 4666–4680. doi: 10.1172/JCI83024
Hirsch, P., Zhang, Y., Tang, R., Joulin, V., Boutroux, H., Pronier, E., et al. (2016). Genetic hierarchy and temporal variegation in the clonal history of acute myeloid leukaemia. Nat. Commun. 7:12475. doi: 10.1038/ncomms12475
Howlader, N., Noone, A. M., Krapcho, M., Miller, D., Bishop, K., Kosary, C. L., et al. (eds.). (1975–2014). SEER Cancer Statistics Review. Bethesda, MD: National Cancer Institute. Available online at: https://seer.cancer.gov/csr/1975_2014/
Ichikawa, M., Asai, T., Saito, T., Yamamoto, G., Seo, S., Yamazaki, I., et al. (2004). AML-1 is required for megakaryocytic maturation and lymphocytic differentiation, but not for maintenance of hematopoietic stem cells in adult hematopoiesis. Nat. Med. 10, 299–304. doi: 10.1038/nm997
Imai, Y., Kurokawa, M., Izutsu, K., Hangaishi, A., Takeuchi, K., Maki, K., et al. (2000). Mutations of the AML1 gene in myelodysplastic syndrome and their functional implications in leukemogenesis. Blood 96, 3154–3160.
Jacob, B., Osato, M., Yamashita, N., Wang, C. Q., Taniuchi, I., Littman, D. R., et al. (2009). Stem cell exhaustion due to Runx1 deficiency is prevented by Evi5 activation in leukemogenesis. Blood 115, 1610–1620. doi: 10.1182/blood-2009-07-232249
Jaiswal, S., Fontanillas, P., Flannick, J., Manning, A., Grauman, P. V., Mar, B. G., et al. (2014). Age-related clonal hematopoiesis associated with adverse outcomes. N. Engl. J. Med. 371, 2488–2498. doi: 10.1056/NEJMoa1408617
Jaiswal, S., Natarajan, P., Silver, A. J., Gibson, C. J., Bick, A. G., Shvartz, E., et al. (2017). Clonal hematopoiesis and risk of atherosclerotic Cardiovascular disease. N. Engl. J. Med. 377, 111–121. doi: 10.1056/NEJMoa1701719
Jan, M., Snyder, T. M., Corces-Zimmerman, M. R., Vyas, P., Weissman, I. L., Quake, S. R., et al. (2012). Clonal evolution of preleukemic hematopoietic stem cells precedes human acute myeloid leukemia. Sci. Transl. Med. 4:149ra18. doi: 10.1126/scitranslmed.3004315
Ko, M., Bandukwala, H. S., An, J., Lamperti, E. D., Thompson, E. C., Hastie, R., et al. (2011). Ten-Eleven-Translocation 2 (TET2) negatively regulates homeostasis and differentiation of hematopoietic stem cells in mice. Proc. Natl. Acad. Sci. U.S.A. 108, 14566–14571. doi: 10.1073/pnas.1112317108
Krejci, O., Wunderlich, M., Geiger, H., Chou, F. S., Schleimer, D., Jansen, M., et al. (2008). p53 signaling in response to increased DNA damage sensitizes AML1-ETO cells to stress-induced death. Blood 111, 2190–2199. doi: 10.1182/blood-2007-06-093682
Krönke, J., Bullinger, L., Teleanu, V., Tschürtz, F., Gaidzik, V. I., Kühn, M. W. M., et al. (2013). Clonal evolution in relapsed NPM1-mutated acute myeloid leukemia. Blood 122, 100–108. doi: 10.1182/blood-2013-01-479188
Kuo, M. C., Liang, D. C., Huang, C. F., Shih, Y. S., Wu, J. H., Lin, T. L., et al. (2009). RUNX1 mutations are frequent in chronic myelomonocytic leukemia and mutations at the C-terminal region might predict acute myeloid leukemia transformation. Leukemia 23, 1426–1431. doi: 10.1038/leu.2009.48
Kwok, B., Hall, J. M., Witte, J. S., Xu, Y., Reddy, P., Lin, K., et al. (2015). MDS-associated somatic mutations and clonal hematopoiesis are common in idiopathic cytopenias of undetermined significance. Blood 126, 2355–2361. doi: 10.1182/blood-2015-08-667063
Lam, K., Muselman, A., Du, R., Yan, M., Matsuura, S., and Zhang, D. E. (2016). Loss of RUNX1 function results in enhanced granulocyte-colony-stimulating factor-mediated mobilization. Blood Cancer J. 6:e407. doi: 10.1038/bcj.2016.20
Liddiard, K., Hills, R., Burnett, A. K., Darley, R. L., and Tonks, A. (2010). OGG1 is a novel prognostic indicator in acute myeloid leukaemia. Oncogene 29, 2005–2012. doi: 10.1038/onc.2009.462
Liew, E., and Owen, C. (2011). Familial myelodysplastic syndromes - a review of the literature. Haematologica. 96, 1536–1542. doi: 10.3324/haematol.2011.043422
Lotem, J., Levanon, D., Negreanu, V., Bauer, O., Hantisteanu, S., Dicken, J., et al. (2015). Runx3 at the interface of immunity, inflammation and cancer. Biochim. Biophys. Acta 1855, 131–143. doi: 10.1016/j.bbcan.2015.01.004
Luddy, R. E., Champion, L. A., and Schwartz, A. D. (1978). A fatal myeloproliferative syndrome in a family with thrombocytopenia and platelet dysfunction. Cancer 41, 1959–1963.
Luo, M. C., Zhou, S. Y., Feng, D. Y., Xiao, J., Li, W. Y., Xu, C. D., et al. (2016). Runt-related Transcription Factor 1 (RUNX1) Binds to p50 in Macrophages and Enhances TLR4-triggered Inflammation and Septic Shock. J. Biol. Chem. 291, 22011–22020. doi: 10.1074/jbc.M116.715953
Mah, L. J., El-Osta, A., and Karagiannis, T. C. (2010). gammaH2AX: a sensitive molecular marker of DNA damage and repair. Leukemia 24, 679–686. doi: 10.1038/leu.2010.6
Malcovati, L., Gallì, A., Travaglino, E., Ambaglio, I., Rizzo, E., Molteni, E., et al. (2017). Clinical significance of somatic mutation in unexplained blood cytopenia. Blood 129, 3371–3378. doi: 10.1182/blood-2017-01-763425
Mangan, J. K., and Speck, N. A. (2011). RUNX1 mutations in clonal myeloid disorders: from conventional cytogenetics to next generation sequencing, a story 40 years in the making. Crit. Rev. Oncog. 16, 77–91. doi: 10.1615/CritRevOncog.v16.i1-2.80
Marusyk, A., Porter, C. C., Zaberezhnyy, V., and DeGregori, J. (2010). Irradiation selects for p53-deficient hematopoietic progenitors. PLoS Biol. 8:e1000324. doi: 10.1371/journal.pbio.1000324
Matheny, C. J., Speck, M. E., Cushing, P. R., Zhou, Y., Corpora, T., Regan, M., et al. (2007). Disease mutations in RUNX1 and RUNX2 create nonfunctional, dominant-negative, or hypomorphic alleles. EMBO J. 26, 1163–1175. doi: 10.1038/sj.emboj.7601568
McKerrell, T., Park, N., Moreno, T., Grove, C. S., Ponstingl, H., Stephens, J., et al. (2015). Leukemia-associated somatic mutations drive distinct patterns of age-related clonal hemopoiesis. Cell Rep. 10, 1239–1245. doi: 10.1016/j.celrep.2015.02.005
Mendler, J. H., Maharry, K., Radmacher, M. D., Mrózek, K., Becker, H., Metzeler, K. H., et al. (2012). RUNX1 mutations are associated with poor outcome in younger and older patients with cytogenetically normal acute myeloid leukemia and with distinct gene and MicroRNA expression signatures. J. Clin. Oncol. 30, 3109–3118. doi: 10.1200/JCO.2011.40.6652
Michaud, J., Wu, F., Osato, M., Cottles, G. M., Yanagida, M., Asou, N., et al. (2002). In vitro analyses of known and novel RUNX1/AML1 mutations in dominant familial platelet disorder with predisposition to acute myelogenous leukemia: implications for mechanisms of pathogenesis. Blood 99, 1364–1372. doi: 10.1182/blood.V99.4.1364
Miyoshi, H., Kozu, T., Shimizu, K., Enomoto, K., Maseki, N., Kaneko, Y., et al. (1993). The t(8;21) translocation in acute myeloid leukemia results in production of an AML1-MTG8 fusion transcript. EMBO J. 12, 2715–2721.
Miyoshi, H., Shimizu, K., Kozu, T., Maseki, N., Kaneko, Y., and Ohki, M. (1991). t(8;21) breakpoints on chromosome 21 in acute myeloid leukemia are clustered within a limited region of a single gene, AML1. Proc. Natl. Acad. Sci. U.S.A. 88, 10431–10434.
Moran-Crusio, K., Reavie, L., Shih, A., Abdel-Wahab, O., Ndiaye-Lobry, D., Lobry, C., et al. (2011). Tet2 loss leads to increased hematopoietic stem cell self-renewal and myeloid transformation. Cancer Cell 20, 11–24. doi: 10.1016/j.ccr.2011.06.001
Motoda, L., Osato, M., Yamashita, N., Jacob, B., Chen, L. Q., Yanagida, M., et al. (2007). Runx1 protects hematopoietic stem/progenitor cells from oncogenic insult. Stem Cells 25, 2976–2986. doi: 10.1634/stemcells.2007-0061
Nakagawa, M., Shimabe, M., Watanabe-Okochi, N., Arai, S., Yoshimi, A., Shinohara, A., et al. (2011). AML1/RUNX1 functions as a cytoplasmic attenuator of NF-kappaB signaling in the repression of myeloid tumors. Blood 118, 6626–6637. doi: 10.1182/blood-2010-12-326710
Naoe, Y., Setoguchi, R., Akiyama, K., Muroi, S., Kuroda, M., Hatam, F., et al. (2007). Repression of interleukin-4 in T helper type 1 cells by Runx/Cbf beta binding to the Il4 silencer. J. Exp. Med. 204, 1749–1755. doi: 10.1084/jem.20062456
Nucifora, G., Birn, D. J., Erickson, P., Gao, J., LeBeau, M. M., Drabkin, H. A., et al. (1993). Detection of DNA rearrangement in the AML1 and ETO loci and of an AML1/ETO fusion mRNA in patients with t(8;21). Blood 81:883.
Okuda, T., Cai, Z., Yang, S., Lenny, N., Lyu, C., van Deursen, J. M. A., et al. (1998). Expression of a knocked-in AML1-ETO leukemia gene inhibits the establishment of normal definitive hematopoiesis and directly generates dysplastic hematopoietic progenitors. Blood 91, 3134–3143.
Ono, M., Yaguchi, H., Ohkura, N., Kitabayashi, I., Nagamura, Y., Nomura, T., et al. (2007). Foxp3 controls regulatory T-cell function by interacting with AML1/Runx1. Nature 446, 685–689. doi: 10.1038/nature05673
Osato, M., Asou, N., Abdalla, E., Hoshino, K., Yamasaki, H., Okubo, T., et al. (1999). Biallelic and heterozygous point mutations in the Runt domain of the AML1/PEBP2aB gene associated with myeloblastic leukemias. Blood 93, 1817–1824.
Owen, C. J., Toze, C. L., Koochin, A., Forrest, D. L., Smith, C. A., Stevens, J. M., et al. (2008). Five new pedigrees with inherited RUNX1 mutations causing familial platelet disorder with propensity to myeloid malignancy. Blood 112, 4639–4645. doi: 10.1182/blood-2008-05-156745
Owen, C., Barnett, M., and Fitzgibbon, J. (2008). Familial myelodysplasia and acute myeloid leukaemia–a review. Br. J. Haematol. 140, 123–132. doi: 10.1111/j.1365-2141.2007.06909.x
Papaemmanuil, E., Gerstung, M., Bullinger, L., Gaidzik, V. I., Paschka, P., Roberts, N. D., et al. (2016). Genomic classification and prognosis in acute Myeloid Leukemia. N. Engl. J. Med. 374, 2209–2221. doi: 10.1056/NEJMoa1516192
Papaemmanuil, E., Gerstung, M., Malcovati, L., Tauro, S., Gundem, G., Van Loo, P., et al. (2013). Clinical and biological implications of driver mutations in myelodysplastic syndromes. Blood 122, 3616–3627. doi: 10.1182/blood-2013-08-518886
Preudhomme, C., Renneville, A., Bourdon, V., Philippe, N., Roche-Lestienne, C., Boissel, N., et al. (2009). High frequency of RUNX1 biallelic alteration in acute myeloid leukemia secondary to familial platelet disorder. Blood 113, 5583–5587. doi: 10.1182/blood-2008-07-168260
Quivoron, C., Couronné, L., Della Valle, V., Lopez, C. K., Plo, I., Wagner-Ballon, O., et al. (2011). TET2 inactivation results in pleiotropic hematopoietic abnormalities in mouse and is a recurrent event during human lymphomagenesis. Cancer Cell 20, 25–38. doi: 10.1016/j.ccr.2011.06.003
Romana, S. P., Mauchauffé, M., Le Coniat, M., Chumakow, I., Le Paslier, D., Berger, R., et al. (1995). The t(12;21) of acute lymphoblastic leukemia results in a tel-AML1 gene fusion. Blood 85. 3662–3670.
Rozhok, A. I., and DeGregori, J. (2016). The evolution of lifespan and age-dependent cancer risk. Trends Cancer 2, 552–560. doi: 10.1016/j.trecan.2016.09.004
Sakurai, M., Kasahara, H., Yoshida, K., Yoshimi, A., Kunimoto, H., Watanabe, N., et al. (2016). Genetic basis of myeloid transformation in familial platelet disorder/acute myeloid leukemia patients with haploinsufficient RUNX1 allele. Blood Cancer J. 6:e392. doi: 10.1038/bcj.2015.81
Satoh, Y., Matsumura, I., Tanaka, H., Ezoe, S., Fukushima, K., Tokunaga, M., et al. (2008). AML1/RUNX1 works as a negative regulator of c-Mpl in hematopoietic stem cells. J. Biol. Chem. 283, 30045–30056. doi: 10.1074/jbc.M804768200
Satoh, Y., Matsumura, I., Tanaka, H., Harada, H., Harada, Y., Matsui, K., et al. (2012). C-terminal mutation of RUNX1 attenuates the DNA-damage repair response in hematopoietic stem cells. Leukemia 26, 303–311. doi: 10.1038/leu.2011.202
Schindler, J. W., Van Buren, D., Foudi, A., Krejci, O., Qin, J., Orkin, S. H., et al. (2009). TEL-AML1 corrupts hematopoietic stem cells to persist in the bone marrow and initiate leukemia. Cell Stem Cell 5, 43–53. doi: 10.1016/j.stem.2009.04.019
Schlenk, R. F., Benner, A., Hartmann, F., del Valle, F., Weber, C., Pralle, H., et al. (2003). Risk-adapted postremission therapy in acute myeloid leukemia: results of the German multicenter AML HD93 treatment trial. Leukemia 17, 1521–1528. doi: 10.1038/sj.leu.2403009
Schnittger, S., Dicker, F., Kern, W., Wendland, N., Sundermann, J., Alpermann, T., et al. (2011). RUNX1 mutations are frequent in de novo AML with non complex karyotype and confer an unfavourable prognosis. Blood 117, 2348–2357. doi: 10.1182/blood-2009-11-255976
Shlush, L. I., Zandi, S., Mitchell, A., Chen, W. C., Brandwein, J. M., Gupta, V., et al. (2014). Identification of pre-leukaemic haematopoietic stem cells in acute leukaemia. Nature 506, 328–333. doi: 10.1038/nature13038
Shurtleff, S. A., Buijs, A., Behm, F. G., Rubnitz, J. E., Raimondi, S. C., Hancock, M. L., et al. (1995). TEL/AML1 fusion resulting from a cryptic t(12;21) is the most common genetic lesion in pediatric ALL and defines a subgroup of patients with an excellent prognosis. Leukemia 9, 1985–1989.
Song, W.-J., Sullivan, M. G., Legare, R. D., Hutchings, S., Tan, X., Kufrin, D., et al. (1999). Haploinsufficiency of CBFA2 (AML1) causes familial thrombocytopenia with propensity to develop acute myelogenous leukamia (FPD/AML). Nat. Genet. 23, 166–175.
Sorrell, A., Espenschied, C., Wang, W., Weitzel, J., Chu, S., Parker, P., et al. (2012). Hereditary leukemia due to rare RUNX1c splice variant (L472X) presents with eczematous phenotype. Int. J. Clin. Med. 3, 607–613. doi: 10.4236/ijcm.2012.37110
Steensma, D. P. (2015). Myelodysplastic syndromes: diagnosis and treatment. Mayo Clin. Proc. 90, 969–983. doi: 10.1016/j.mayocp.2015.04.001
Steensma, D. P., Gibbons, R. J., Mesa, R. A., Tefferi, A., and Higgs, D. R. (2005). Somatic point mutations in RUNX1/CBFA2/AML1 are common in high-risk myelodysplastic syndrome, but not in myelofibrosis with myeloid metaplasia. Eur. J. Haematol. 74, 47–53. doi: 10.1111/j.1600-0609.2004.00363.x
Sun, W., and Downing, J. R. (2004). Haploinsufficiency of AML1 results in a decrease in the number of LTR-HSCs while simultaneously inducing an increase in more mature progenitors. Blood 104, 3565–3572. doi: 10.1182/blood-2003-12-4349
Tang, J. L., Hou, H. A., Chen, C. Y., Liu, C. Y., Chou, W. C., Tseng, M. H., et al. (2009). AML1/RUNX1 mutations in 470 adult patients with de novo acute myeloid leukemia: prognostic implication and interaction with other gene alterations. Blood 114, 5352–5361. doi: 10.1182/blood-2009-05-223784
Tang, X., Sun, L., Jin, X., Chen, Y., Zhu, H., Liang, Y., et al. (2017). Runt-related transcription factor 1 regulates LPS-Induced acute lung injury via NF-κB signaling. Am. J. Respir. Cell Mol. Biol. 57, 174–183. doi: 10.1165/rcmb.2016-0319OC
Tefferi, A., and Vardiman, J. W. (2009). Myelodysplastic syndromes. N. Engl. J. Med. 361, 1872–1885. doi: 10.1056/NEJMra0902908
Thota, S., Viny, A. D., Makishima, H., Spitzer, B., Radivoyevitch, T., Przychodzen, B., et al. (2014). Genetic alterations of the cohesin complex genes in myeloid malignancies. Blood 124, 1790–1798. doi: 10.1182/blood-2014-04-567057
Tober, J., Maijenburg, M. W., and Speck, N. A. (2016). Taking the Leap: Runx1 in the Formation of Blood from Endothelium. Curr. Top. Dev. Biol. 118:113–162. doi: 10.1016/bs.ctdb.2016.01.008
University of Chicago Hematopoietic Malignancies Cancer Risk Team (2016). How I diagnose and manage individuals at risk for inherited myeloid malignancies. Blood 128, 1800–1813. doi: 10.1182/blood-2016-05-670240
Valent, P., Bain, B. J., Bennett, J. M., Wimazal, F., Sperr, W. R., Mufti, G., et al. (2012). Idiopathic cytopenia of undetermined significance (ICUS) and idiopathic dysplasia of uncertain significance (IDUS), and their distinction from low risk MDS. Leuk. Res. 36, 1–5. doi: 10.1016/j.leukres.2011.08.016
Wang, C. Q., Krishnan, V., Tay, L. S., Chin, D. W., Koh, C. P., Chooi, J. Y., et al. (2014). Disruption of Runx1 and Runx3 leads to bone marrow failure and leukemia predisposition due to transcriptional and DNA repair defects. Cell Rep. 8, 767–782. doi: 10.1016/j.celrep.2014.06.046
West, A. H., Godley, L. A., and Churpek, J. E. (2014). Familial myelodysplastic syndrome/acute leukemia syndromes: a review and utility for translational investigations. Ann. N.Y. Acad. Sci. 1310, 111–118. doi: 10.1111/nyas.12346
Wichmann, C., Quagliano-Lo Coco, I., Yildiz, Ö., Chen-Wichmann, L., Weber, H., Syzonenko, T., et al. (2015). Activating c-KIT mutations confer oncogenic cooperativity and rescue RUNX1/ETO-induced DNA damage and apoptosis in human primary CD34+ hematopoietic progenitors. Leukemia 29, 279–289. doi: 10.1038/leu.2014.179
Wiemels, J. L., Cazzaniga, G., Daniotti, M., Eden, O. B., Addison, G., Masera, G., et al. (1999). Prenatal origin of acute lymphoblastic leukaemia in children. Lancet 354, 1499–1503.
Wiemels, J. L., Xiao, Z., Buffler, P. A., Maia, A. T., Ma, X., Dicks, B. M., et al. (2002). In utero origin of t(8;21) AML1-ETO translocations in childhood acute myeloid leukemia. Blood 99, 3801–3805. doi: 10.1182/blood.V99.10.3801
Wildonger, J., and Mann, R. S. (2005). The t(8;21) translocation converts AML1 into a constitutive transcriptional repressor. Development 132, 2263–2272. doi: 10.1242/dev.01824
Wong, W. F., Kohu, K., Chiba, T., Sato, T., and Satake, M. (2011). Interplay of transcription factors in T-cell differentiation and function: the role of Runx. Immunology 132, 157–164. doi: 10.1111/j.1365-2567.2010.03381.x
Wong, W. F., Kohu, K., Nakamura, A., Ebina, M., Kikuchi, T., Tazawa, R., et al. (2012). Runx1 deficiency in CD4+ T cells causes fatal autoimmune inflammatory lung disease due to spontaneous hyperactivation of cells. J. Immunol. 188, 5408–5420. doi: 10.4049/jimmunol.1102991
Wu, D., Ozaki, T., Yoshihara, Y., Kubo, N., and Nakagawara, A. (2013). Runt-related transcription factor 1 (RUNX1) stimulates tumor suppressor p53 protein in response to DNA damage through complex formation and acetylation. J. Biol. Chem. 288, 1353–1364. doi: 10.1074/jbc.M112.402594
Xie, M., Lu, C., Wang, J., McLellan, M. D., Johnson, K. J., Wendl, M. C., et al. (2014). Age-related mutations associated with clonal hematopoietic expansion and malignancies. Nat. Med. 20, 1472–1478. doi: 10.1038/nm.3733
Yergeau, D. A., Hetherington, C. J., Wang, Q., Zhang, P., Sharpe, A. H., Binder, M., et al. (1997). Embryonic lethality and impairment of haematopoiesis in mice heterozygous for an AML1-ETO fusion gene. Nat. Genet. 15, 303–306.
Yoshimi, A., Toya, T., Kawazu, M., Ueno, T., Tsukamoto, A., Iizuka, H., et al. (2014). Recurrent CDC25C mutations drive malignant transformation in FPD/AML. Nat. Commun. 5:4770. doi: 10.1038/ncomms5770
Zhang, F., Meng, G., and Strober, W. (2008). Interactions among the transcription factors Runx1, RORgammat and Foxp3 regulate the differentiation of interleukin 17-producing T cells. Nat. Immunol. 9, 1297–1306. doi: 10.1038/ni.1663
Keywords: RUNX1, leukemia, myeloid neoplasms, leukemia predisposition, familial platelet disorder with predisposition for acute myeloid leukemia, pre-leukemia
Citation: Bellissimo DC and Speck NA (2017) RUNX1 Mutations in Inherited and Sporadic Leukemia. Front. Cell Dev. Biol. 5:111. doi: 10.3389/fcell.2017.00111
Received: 15 September 2017; Accepted: 04 December 2017;
Published: 20 December 2017.
Edited by:
Keisuke Ito, Albert Einstein College of Medicine, United StatesReviewed by:
Motomi Osato, National University of Singapore, SingaporeJames C. Neil, University of Glasgow, United Kingdom
Copyright © 2017 Bellissimo and Speck. This is an open-access article distributed under the terms of the Creative Commons Attribution License (CC BY). The use, distribution or reproduction in other forums is permitted, provided the original author(s) or licensor are credited and that the original publication in this journal is cited, in accordance with accepted academic practice. No use, distribution or reproduction is permitted which does not comply with these terms.
*Correspondence: Nancy A. Speck, bmFuY3lhc0B1cGVubi5lZHU=