- Cardiac and Skeletal Muscle Research Group, Department of Experimental Biology, University of Jaén, Jaén, Spain
The adult pumping heart is formed by distinct tissue layers. From inside to outside, the heart is composed by an internal endothelial layer, dubbed the endocardium, a thick myocardial component which supports the pumping capacity of the heart and exteriorly covered by a thin mesothelial layer named the epicardium. Cardiac insults such as coronary artery obstruction lead to ischemia and thus to an irreversible damage of the myocardial layer, provoking in many cases heart failure and death. Thus, searching for new pathways to regenerate the myocardium is an urgent biomedical need. Interestingly, the capacity of heart regeneration is present in other species, ranging from fishes to neonatal mammals. In this context, several lines of evidences demonstrated a key regulatory role for the epicardial layer. In this manuscript, we provide a state-of-the-art review on the developmental process leading to the formation of the epicardium, the distinct pathways controlling epicardial precursor cell specification and determination and current evidences on the regenerative potential of the epicardium to heal the injured heart.
The development of the heart is a complex process. The primitive heart tube is formed from cardiogenic mesoderm of the cardiac crescents, i.e., first heart field (FHF), while anterior and venous poles are derived from a subsequent subset of cardiogenic cells located medial to the cardiac crescents, dubbed second heart field (SHF; Kelly et al., 2001; Kelly and Buckingham, 2002). In addition, external cellular contributions to the developing heart will take place from this stage onwards. On the one hand, cardiac neural crest will colonize the most anterior parts of the heart playing a pivotal role on aortico-pulmonary septation (Kirby and Waldo, 1990, 1995). On the other hand, cell originating from the proepicardium (PE) will cover and infiltrate into the developing heart leading to distinct cellular subpopulations, such as endothelial and smooth muscle cells forming the coronary vasculature, endocardial cushion mesenchyme, cardiac fibroblasts, and of course the adult epicardial lining (Winter and Gittenberger-de Groot, 2007; Gittenberger-de Groot et al., 2012). In this manuscript we will provide a state-of-the-art review on the developmental process leading to the formation of the PE/epicardium, the signaling pathways providing cell specification and fate determination to those epicardial precursor cells including the upcoming role of non-coding RNAs, and current evidences on the regenerative role of the epicardium as to heal the injured heart.
Initial Phases of the Proepicardial (PE) and Epicardial Formation; A Journey to the Developing Embryonic Heart
The proepicardium (PE) is a small protuberance that progressively develops within limiting boundaries between the hepatic and cardiac primordia. It is composed of an external epithelial lining configured as a cauliflower structure and an internal mesenchymal component (Virágh et al., 1993; Kálmán et al., 1995; Ratajska et al., 2008). A single PE anlage is observed at early developmental stages in zebrafish (Serluca, 2008) while in the sturgeon and in mice bilateral PE buds are formed subsequently merging into a single midline structure (Schulte et al., 2007; Icardo et al., 2009). Curiously, in chicken two PE primordia are formed, but interestingly the right PE anlage develops before the left one is visible (Schulte et al., 2007). These data suggest divergent evolutionary trends on the formation of the PE primordia and furthermore advocate that embryonic left-right signaling might play a role controlling PE formation (Schlueter and Brand, 2012).
Transcriptional heterogeneity is widely documented for the PE anlage, and in addition, cell specific markers for several of the PE/epicardial cell derivatives, such as endothelial (Poelmann et al., 1993; Mikawa and Gourdie, 1996; Cossette and Misra, 2011; Niderla-Bielińska et al., 2015) and smooth muscle (Valder and Olson, 1994) cells have also been documented, suggesting an early heterogeneous compartmentalization. Subsequently after the formation of the PE a process of delamination and migration of the proepicardial cells occurs. This process will lead to external covering of the atrioventricular canal and the entire atrial and ventricular myocardial chambers as demonstrated by seminal studies using quail-chicken embryos (Pérez-Pomares et al., 1998, 2002; Vrancken Peeters et al., 1999; Figure 1). In zebrafish, this process is dependent on the pericardial fluid currents (Peralta et al., 2013, 2014; Plavicki et al., 2013, 2014). In mice, proepicardial cells are detached from the PE forming cysts that migrate to the developing cardiac chambers through the pericardial cavity (Männer et al., 2001; Hirose et al., 2006). These cysts randomly attach to the ventricular and atrial chambers and progressively expand until the final full coverage of the cardiac chambers is completed.
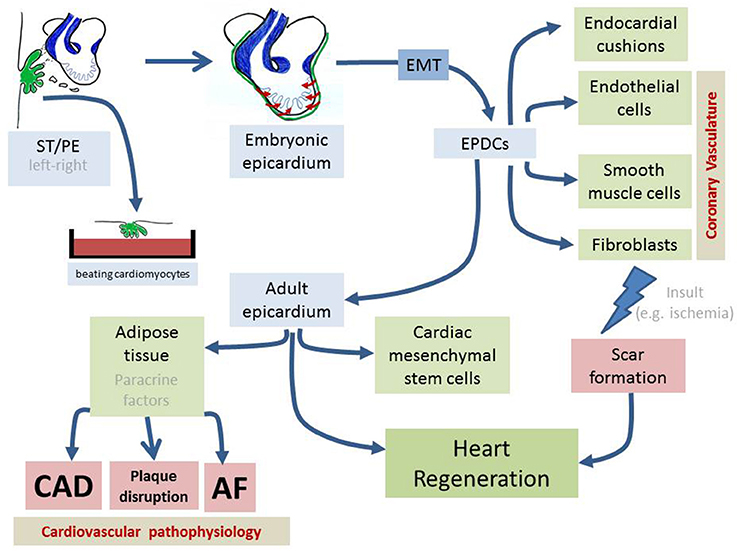
Figure 1. Schematic representation of the distinct developmental stages of the proepicardium (PE)/septum transversum (ST) formation as well as on the distinct lineage contribution of the embryonic and adult epicardium.
Once the PE cells migrate and cover the surface of the developing embryonic myocardium an epicardial-myocardial signaling crosstalk is initiated. This process is crucial for the correct development of both cardiac tissue layers. The epicardium is instructed to initiate an epithelial-to-mesenchymal transformation (EMT), detaching from the epithelial epicardial layer and migrating first into the subepicardial space. These cells subsequently invade the myocardial walls, giving rise to the epicardial derived cells (EPDCs) (Dettman et al., 1998). An additional source of subepicardial cells of hematopoietic origin is provided during embryonic development which further contributes to the heterogeneity of the embryonic and postnatal epicardium (Balmer et al., 2014). In the following chapters we provide a state-of-the-art review on the differential contribution of the embryonic epicardium in cardiovascular development and disease.
Cell Fate and Contribution of the Embryonic Epicardium to the Mature Heart
Epicardial derived cells once they go through the subepicardial space continue their journey into the developing heart. Seminal approaches using quail-chick chimeras demonstrated that quail EPDCs contribute to distinct cardiac cell lineages, such as endothelial and smooth muscle cells in the coronary vasculature, endocardial mesenchymal cells in the atrioventricular cushions and also cardiac fibroblasts (Poelmann et al., 1993; Dettman et al., 1998; Figure 1). Since the experimental model used was a heterologous chimera, multiple criticisms were arising as which was indeed the real contribution of these cells. Supporting evidences were generated using retroviral-defective cell lineage tracing experiments in chicken hearts providing similar results (Mikawa and Gourdie, 1996); i.e., vascular endothelial, smooth muscle, and cardiac fibroblasts. Contribution to endocardial cushions is scarce, although it has been proposed that these cells are important for the correct development of the atrioventricular junction and the annulus fibrosus (Lie-Venema et al., 2008; Zhou et al., 2010; Lockhart et al., 2014). More recently, a contribution to cardiac resident stem cells (mesenchymal-like) has also been reported (Chong et al., 2011). In all cases, contribution to the developing myocardium was never observed (Poelmann et al., 1993; Mikawa and Gourdie, 1996; Pérez-Pomares et al., 2002). Surprisingly, in vitro PE culture experiments demonstrated that cardiomyocytes could be derived from these precursor cell pools (Kruithof et al., 2006).
With the advent of the molecular era, genetic lineage tracing in mice assaulted the quest to understand the contribution of the PE/embryonic epicardium to the mature murine heart. Several lineage tracing approaches were documented, in most cases, using Cre/loxP conditional activation of the reporter genes. In this setting, Tbx18-lineage tracing demonstrated a contribution to all the previously reported EPDC-derived lineages but surprisingly, also to the cardiomyocyte lineage. Whereas, these studies claimed that epicardial Tbx18+ cells contributed in vivo to ventricular cardiomyocytes (Cai et al., 2008), it was previously reported that fetal cardiomyocytes also expressed Tbx18 (Franco et al., 2006; Christoffels et al., 2009; Zeng et al., 2011) and thus those Tbx18+ epicardial lineage tracing experiments were dubious.
Epicardial Wt1+ derived cells have also been reported to contribute to endothelial cells and to the myocardium (Zhou et al., 2008; Zhou and Pu, 2011). Evidence for Wt1+ cells in the embryonic heart has also been reported but excluding cardiomyocytes (Zeng et al., 2011) yet more recent evidence demonstrated that Wt1-derived cardiomyoctes can be traced in the developing heart before PE/epicardial formation (Rudat and Kispert, 2012; Cano et al., 2016), thus questioning the epicardial contribution to the developing cardiac muscle. On the other hand, prove that epicardial cells do not contribute to myocardium in zebrafish comes from Tcf21-tracing (Kikuchi et al., 2011) and transplant experiments (González-Rosa et al., 2012), in which a contribution to the perivascular beds is reported. While these data might support the notion that epicardial cells can contribute to the formation of cardiomyocytes in vivo, yet these evidences remain controversial, mainly because the limitation on the use of Cre-based techniques as a bone fide fate mapping approach (Christoffels et al., 2009).
Additional controversies have also arisen regarding the contribution of EPDCs to other vascular components. To date, it seems clear that EPDCs mostly contribute to cardiac fibroblasts and vascular smooth muscle cells, but their contribution to vascular endothelial cells have also been challenged by additional Cre-based fate mapping experiments. In fact, epicardial-derived Cre based lineage tracing in mice failed to provide substantial contribution to the developing vascular endothelium in mice (Merki et al., 2005; Cai et al., 2008; Zhou et al., 2008). Red-Horse et al. (2010) described that coronary endothelial lining was mostly entirely derived from the sinus venosus endothelium as revealed by an Apelin-Cre mice (Red-Horse et al., 2010; Tian et al., 2013), a process that is VEGF-dependent (Chen et al., 2014). However, additional evidences reported that ventricular endocardial cells also can contribute to the coronary vasculature (Wu et al., 2012) as revealed by Nfatc1-Cre lineage tracing. Furthermore, by the usage of novel proepicardial lineage tracing markers such as Scleraxis-Cre, Semaphorin-3D-Cre, and Fabp4-CreER drivers (Katz et al., 2012; He et al., 2014) a contribution to the coronary vasculature was also reported. In fact, reconciling evidences reported by Chen et al. (2014) determined that sinus-venous (SV) derived coronary vasculature mostly contributed to the dorsal and lateral coronary vasculature (~70%) whereas the ventral aspects were mostly endocardial derived (~70–80%), with just a small (~20%) but uniform contribution from the epicardium. These data are in line with a recent report that similarly estimated a 20% contribution from the proepicardium (Cano et al., 2016). Interestingly, a significant proportion of SV-derived and endocardial-derived cells displayed overlapping patterns with PE-derived cells, suggesting a common lineage origin. These data support the notion that multiple precursor cell populations contribute to the formation of the cardiac vasculature in mice, in contrast to avian hearts, in which the epicardial-derived contribution is large and undisputed. Lineage relationships between these three distinct coronary vasculature components remain nonetheless to be fully elucidated in mice.
Over the last decade our understanding of the molecular regulation of epicardial derived cells has largely increased with the usage of conditional spatio-temporal deletion of discrete signaling pathways. Epicardial cells display distinct divergent and overlapping expression patterns of Wt1, Nfatc1, Tbx18, and Pod1 in the chicken and murine hearts (Braitsch et al., 2012), providing a heterogeneous panel of potentially distinct cardiac stem cells. Whereas, to date it remains elusive when and how epicardial cells becomes specific to their prospective lineage, it is increasing clear that multiple factors play pivotal roles in this process as summarized in Table 1. In particular, PDGFRβ is important for epicardial migration and for the development of coronary vascular smooth muscle cells (Mellgren et al., 2008; Bax et al., 2009; Smith et al., 2011), retinoic acid and VEGF primes endothelial vs. smooth muscle differentiation (Guadix et al., 2006; Tomanek et al., 2006; Azambuja et al., 2010) and Fgf signaling (Guadix et al., 2006), mainly through Fgf10 and Fgfr2b are essential for cardiac fibroblast formation (Vega-Hernández et al., 2011). In addition, Pod1/Tcf21 is regulated by retinoic acid and inhibits differentiation of EPDCs into smooth muscle cells in chicken and mice (Braitsch et al., 2012) while Wnt signaling is also important for epicardial specification, as Dkk1 and Dkk2 mouse mutants display impaired epicardial development (Phillips et al., 2011). Similarly PCP disruption is also critical in this context (Phillips et al., 2008) as well as MAPK kinase genetic inactivation (Liberatore and Yutzey, 2004; Craig et al., 2010a,b). Other signaling pathways, such as CXCL12/CXCR4 are also crucial for cardiac vascular development (Cavallero et al., 2015). Furthermore, Hippo signaling, mediated by Yap/Taz modulates Tbx18 and Wt1 expression in the epicardium controlling their contribution to the coronary vasculature (Singh et al., 2016). Several other molecules have also been reported to be critical for coronary artery formation, such as tenascin C (Ando et al., 2011) and nephrin (Wagner et al., 2011) particularly for smooth muscle recruitment to those cardiac vessels. Overall these findings highlight the complexity of distinct signaling pathways and molecules governing the coronary vasculature development.
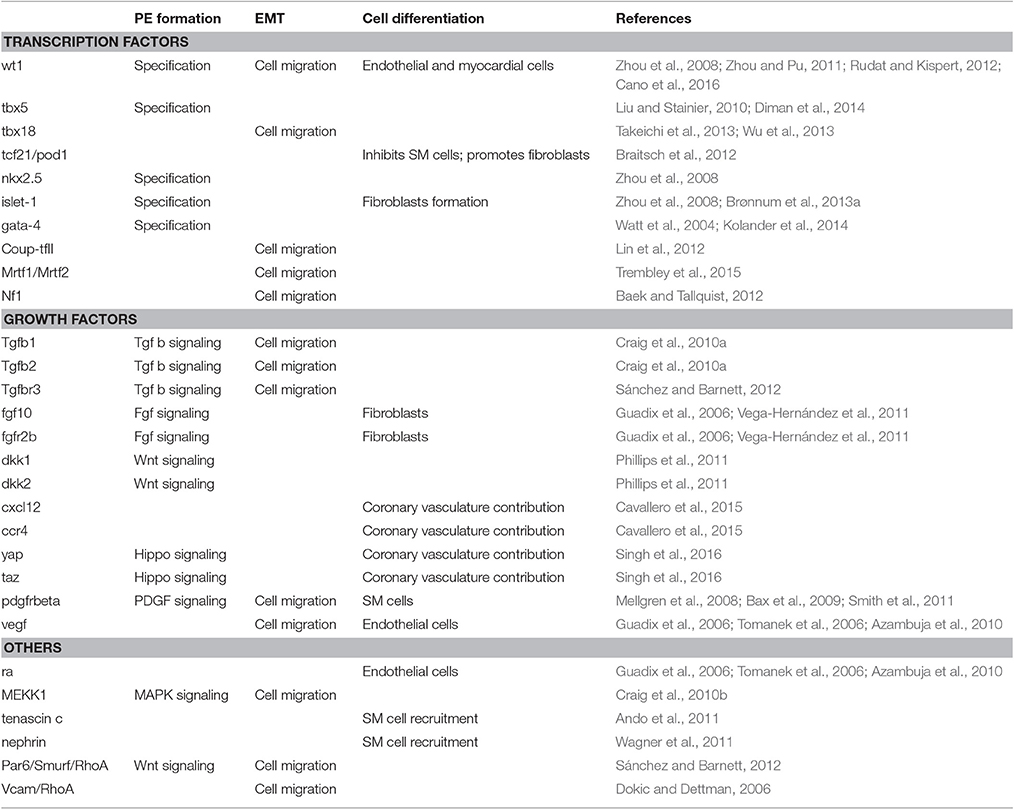
Table 1. List of transcription factors, growth factors are other distinct molecules involved in distinct phases of proepicardium/epicardium development.
The Role of The Postnatal Epicardium in the Injured Heart
Within the adult heart, the epicardium represents the outermost layer, which is a simple epithelial layer. For many years, the functional role of this layer has been neglected as it was considered as an external cover devoid of any functional meaning. The discovery that the epicardial precursors can differentiate to beating cardiomyocytes has branded the epicardium as a source of cardiac stem cells with great therapeutic potential (Wessels and Pérez-Pomares, 2004; Pérez-Pomares et al., 2006; Winter et al., 2009). In addition, it has been reported that the adult epicardium plays a pivotal role in cardiac regeneration (Bollini et al., 2011, 2015; Schlueter and Brand, 2012; Masters and Riley, 2014; Kennedy-Lydon and Rosenthal, 2015; Figure 1) as detailed below.
Seminal work by Kruithof et al. (2006) described that the embryonic chicken PE if placed in appropriate cell culture conditions, was capable of giving rise to beating cardiomyocytes. Such in vitro conditions could be further promoted by Bmp administration and blocked by Fgf signaling. Thus, these data opened out the possibility that the epicardium could serve as an in vivo source of potential cardiomyocytes if the appropriate signals would be instructed in vivo. Importantly, Smart et al. (2011) demonstrated that adult epicardial derived cells, if previously primed with thymosinβ4, eventually generated functionally beating cardiomyocytes in an ischemic heart, yet the proportion of de novo integrated cells was rather spurious and its instructive mechanism remains rather obscure (Gajzer et al., 2013). Nonetheless, as a proof of principle approach it was highly valuable. This work introduced a novel concept of an activated epicardium, a condition by which embryonic epicardial markers such as Wt1 and Tbx18 are re-expressed in the adult epicardium (Huang et al., 2012; van Wijk et al., 2012; Braitsch et al., 2013; Bollini et al., 2014; Aguiar and Brunt, 2015) in response to distinct biological stimuli such as thymosinβ4 (Smart et al., 2012; Smart and Riley, 2012), stem cell factor (SCF; Xiang et al., 2014), and prokineticins (Urayama et al., 2008) among others. In addition, this activated epicardium secretes paracrine factors that modulate myocardial injury response (Zhou et al., 2011; Foglio et al., 2015).
While it is documented that the human heart has a limited capacity to regenerate (Bergmann et al., 2009), it is also highly acknowledged that the newt heart can also widely regenerate by other means (Becker et al., 1974; Oberpriller and Oberpriller, 1974). Furthermore, the adult zebrafish heart can also regenerate (Poss et al., 2002) and the epicardium provides a pivotal role during this regeneration process (Gemberling et al., 2015; Wang et al., 2015). Molecular analyses have demonstrated that the epicardium becomes activated as soon as the heart is injured and such activation provides instructive signals that promote cardiomyocyte proliferation, revascularization, and tissue repair (Lien et al., 2006, 2012; Marín-Juez et al., 2016). During this process a transitory scar stage occurs and is subsequently replaced by fully functional and integrated cardiomyocytes (González-Rosa et al., 2011; Mercer et al., 2013; Itou et al., 2014; Marro et al., 2016).
Further analyses in this front identified that Wnt1/β-catenin is crucial promoting formation of cardiac fibroblasts and hence cardiac repair (Duan et al., 2012). Several studies have identified key molecules modulating this regeneration capacity. For example, Nrg1 acts as a mitogenic agent in cardiomyocytes following injury during cardiac zebrafish regeneration (Gemberling et al., 2015). Notch (Zhao et al., 2014), Raldh2 (Itou et al., 2014), and myocardial NF-κB (Karra et al., 2015) are also essential for heart regeneration in zebrafish. Hydrogen peroxide (Han et al., 2014) has been reported to prime heart regeneration and telomerase has been identified as instrumental for zebrafish regeneration (Bednarek et al., 2015), but still it remains to be established if these factors are modulated by the epicardium. More recently, it has been demonstrated that epicardial regeneration is guided by the cardiac outflow tract and hedgehog signaling (Wang et al., 2015) and single cell transcriptome of the epicardium has identified caveolin1 as an essential factor in regenerating zebrafish heart (Cao and Poss, 2016). Moreover, re-expression of epicardial developmental genes and enhanced EMT in response to injury has been widely demonstrated (Lepilina et al., 2006; Kim et al., 2010; González-Rosa et al., 2011; Schnabel et al., 2011). These data suggest that complex regulatory networks control zebrafish regeneration (Rodius et al., 2016) positioning the epicardium as a key tissue layer for regeneration. Thus, these data will be highly instrumental to search for novel ways to heal the injured heart.
In adult mice, the regenerative capacity is lost and the injured heart responds by generating a fibrous scar which is derived from pre-existing epicardial cells (Zhou et al., 2008; Duan et al., 2012) as well as de novo recruited bone marrow-borne circulating cells (Ruiz-Villalba et al., 2015). Interestingly, full regeneration is achieved at early developmental stages, i.e., on the first week of life, in which the epicardium (Porrello et al., 2011) is also a highly instructive player and thymosinβ4 priming increases the time window for mammalian heart regeneration (Rui et al., 2014). In addition a role for Wnt signaling has also been identified in the regenerating heart in mice (Mizutani et al., 2016). Recent evidence demonstrated that exosomal signaling from the epicardium is essential for myocardial maturation highlighting a pivotal role for clustering in this process (Foglio et al., 2015). All these efforts have provided the bases of heart regeneration. A giant step was recently reported by Wei et al. (2015) whom used reconstitution of epicardial follistatin-like1 expression in biomaterial patches to heal the adult injured heart, opening a novel way to regenerate the adult mammalian heart.
An Unexpected Epicardial Derivative with Paracrine Signaling Leading to CAD and AF
While it is highly acknowledged that the epicardial precursor cells, within the PE, and subsequently the EPDCs will give rise to distinct cardiovascular embryonic cell lineages, it has remained unexplored if the adult epicardium can generate additional cellular subpopulations. Recent evidences have demonstrated that intramyocardial adipose tissue is derived from the endocardium (Zhang et al., 2016), whereas adipose tissue around the heart, mainly at the venous, arterial connections, and atrial appendages is an adult epicardium derivative (Yamaguchi et al., 2015). Furthermore, cardiac adipose tissue deposition has recently been associated to distinct cardiovascular pathologies (Figure 1), such as coronary arteries diseases (Iwayama et al., 2014), atherosclerosis plaque disruption (Talman et al., 2014; Yamashita et al., 2014), and atrial fibrillation (Batal et al., 2010; Nakanishi et al., 2012; Gaborit et al., 2013). Although, these are early days to fully understand the molecular mechanisms linking epicardium, adipose tissue deposition, and cardiovascular pathologies, supporting evidences suggest that these cells can act as paracrine signaling center that, if impaired, can be the source of cardiovascular diseases (Langlois et al., 2010; Greulich et al., 2012).
Non-Coding RNAs in the PE/Epicardium
Over the last decade we have witnessed a revolution in the concept of the control of gene expression with the discovery of non-coding RNAs. Non-coding RNAs can be broadly classified according to the transcript size into long non-coding RNAs (lncRNAs) and small non-coding RNAs. Our current understanding of lncRNAs is still in its infancy with just a limited number of reports in the developing heart (Grote et al., 2013; Klattenhoff et al., 2013; Sauvageau et al., 2013; Zhu et al., 2014; Kurian et al., 2015). On the other hand, our knowledge on the functional role of small non-coding RNAs, in particular microRNAs, has been largely increased (Callis and Wang, 2008; Chen and Wang, 2012; Bonet et al., 2013; Philippen et al., 2015; Yan and Jiao, 2016). microRNAs are small non-coding RNA of 18–24 nt in length that by homologous base-priming are capable of blocking translation or degrading mRNA transcripts. microRNAs are transcribed by RNA polymerase II, 5′ capped and 3′ polyadenylated leading to mature microRNA by RNA endonucleases such as Drosha and Dicer (Aranega and Franco, 2015; Towler et al., 2015). Mature microRNAs are loaded into the RISC complex which can thereafter search for mRNA transcript base complementarity (Hammond, 2015; Shen and Hung, 2015). To date more than a 1,000 distinct microRNAs have been identified in humans, which are quite conserved among evolution. A seminal study by Singh et al. (2011) demonstrated that conditional ablation of Dicer, an RNAse processing enzyme, in the epicardium provoked impaired epicardial formation, thin-walled myocardium, and aberrant coronary vasculature formation. Thus, this study demonstrated a pivotal role for microRNAs in PE/epicardium development. A large array of studies have been reported in key developmental processes by which the PE/epicardium is formed, such as epithelial-to-mesenchymal transition in cancer (see for recent reviews; Behbahani et al., 2016; Peng et al., 2016; Sulaiman et al., 2016; Zou et al., 2016) and also within the heart (Stankunas et al., 2010; Bonet et al., 2015) and cardiac regeneration (Porrello et al., 2013) but surprisingly only a short list of studies have been reported in PE/epicardium formation. miR-21 has been reported in numerous studies promoting fibrogenesis both during cardiac development and disease (Thum et al., 2008; Adam et al., 2012; Derda et al., 2015; Gupta et al., 2016). Brønnum et al. (2013a) has recently reported that miR-21 promotes fibrogenic EMT in epicardial cells by modulating Pcd4 and Sprouty-1 and these authors (Brønnum et al., 2013b) have also reported that islet-1 can influence miR-21 expression and therefore modulate cardiac fibrogenic EMT. Seeger et al. (2016) demonstrated that let-7 inhibition enhances the recruitment of epicardial cells after myocardial infarction promoting an improved cardiac function. Overall these studies demonstrate a nascent role for microRNAs in PE/epicardium formation, which might provide novel approaches to activate and prime epicardial cells for cardiac regeneration.
Conclusions and Perspectives
Over the last decade our understanding of the cellular contribution of the PE/epicardium has largely increased. Seminal works using quail-chick chimeras demonstrated a large plasticity for the EPCDs, contributing to the cardiac fibrous skeleton, the coronary vasculature and the developing atrioventricular valves (Poelmann et al., 1993; Wessels and Pérez-Pomares, 2004; Figure 1). However, with the advent of molecular tracing tools, multiple evidences demonstrated a rather more complex contribution and architecture to the coronary vasculature in mice. Cre-driven fate mapping can be pervasive and promiscuous tools, deriving in complex and in many cases controversial findings. We hope that either retrospective clonal analysis as previously reported for myocardial components (Meilhac et al., 2003, 2004a,b) or genuine prospective lineage tracing would serve to reconcile these findings in the PE/epicardial context. With no doubt one of the seminal work that prompted the interest of the epicardial lining in the context of cardiac stem cell and cardiac regeneration was reported by Kruithof et al. (2006) demonstrating that PE/epicardial cells could be generating cardiomyocyte in vitro opening the possibilities to unlock the myocardial lineage commitment in vivo. Thymosin beta4 was the first of these unlocking tools, providing an entry site to regenerate the heart using the epicardium as a cell source (Smart et al., 2011; Smart and Riley, 2012). In addition, bridging epicardial activation by follistatin-like1 into biomaterials provided additional convincing evidences on the feasibility of these approaches (Wei et al., 2015). New tools will be discovered in the near future.
In recent years a novel link between the epicardium and epicardial derived structures is emerging (Figure 1). Intriguingly, adipose fat deposition within the pericardiac regions has been linked to cardiac pathophysiologies such as coronary artery atherosclerosis and atrial fibrillation. To date the casual relationship remains enigmatic, yet a plausible embryonic link might be present since epicardial cells can differentiate into adipose tissue (Zhang et al., 2016) and epicardial cells contribute to both endothelial and smooth muscle components of the coronary vessels (Pérez-Pomares et al., 2002; Cano et al., 2016). However, our current understanding is still in its infancy and for sure we will witness additional cellular and molecular evidences deciphering the interplay between these rather apparent distinct cardiovascular entities.
While our cellular and molecular understanding of PE/epicardium/EPDC has greatly advanced in recently years, the discovery of novel levels of gene regulations, in particular those exerted by the non-coding RNAs, is called to change our molecular and signaling pathways schemes. The discovery that microRNAs are crucial to epicardial development is simply demonstrating the equally pivotal roles of these tiny molecules in other cardiovascular developmental contexts (Cordes and Srivastava, 2009; Chinchilla et al., 2011; Bonet et al., 2015). In addition to microRNAs, long-non-coding RNAs are also called to play pivotal role in cardiogenesis (Grote et al., 2013; Klattenhoff et al., 2013) and thus similarly in epicardial development. In coming years, additional routes would be discovered demonstrating the essential role of these new players in epicardial biology both during development and disease.
Author Contributions
AD searched for literature records and wrote part of the manuscript. DF wrote part of the manuscript, editing, and approved the final manuscript version. AA read and suggested editing comments to the manuscript.
Funding
DF and AA are partially funding by a grant of the Ministerio de Economia y Competitividad (BFU2015-67131-P) of the Spanish Government and the Consejeria de IEconomia y Conocimiento (CTS-446) of the Junta de Andalucia Regional Council.
Conflict of Interest Statement
The authors declare that the research was conducted in the absence of any commercial or financial relationships that could be construed as a potential conflict of interest.
Abbreviations
Bmp, Bone morphogenetic protein; CXCR4, Chemokine (C-X-C motif) receptor 4; Cre, Cre recombinase; CXCL12, Chemokine (C-X-C motif) ligand 12; Dkk1, Dickkopf-related protein 1; Dkk2, Dickkopf-related protein 2; EMT, Epitelial-to-mesenchymal transition; EPDCs, Epicardial derived cells; Fabp4, Fatty acid binding protein 4; Fgf, Fibroblast growth factor; Fgf10, Fibroblast growth factor 10; Fgfr2b, Fibroblast growth factor receptor 2b; FHF, First heart field; lcnRNA, Long non-coding RNA; MAPK, Mitogen-Activated Protein Kinase; Nfatc1, Nuclear factor of activated T-cells, cytoplasmic 1; NF-κB, Nuclear Factor κB; Nrg1, Neuregulin 1; Pcd4, Programmed Cell Death 4; PCP, Planar cell polarity; PE, Proepicardium; Pod1/Tcf21, Podocyte-expressed 1/Transcription factor 21; Raldh2, Aldehyde dehydrogenase family 1, subfamily A2; SHF, Second heart field; Tbx18, T-box homeobox 18; Tcf21/Pod1, Transcription factor 21/Podocyte-expressed 1; VEGF, Vascular endothelial growth factor; Wt1, Wilms tumor protein; Yap/Taz, Yes associated protein 1/Transcriptional coactivator with PDZ-binding motif.
References
Adam, O., Löhfelm, B., Thum, T., Gupta, S. K., Puhl, S. L., Schäfers, H. J., et al. (2012). Role of miR-21 in the pathogenesis of atrial fibrosis. Basic Res. Cardiol. 107:278. doi: 10.1007/s00395-012-0278-0
Aguiar, C., and Brunt, K. R. (2015). Wilms' tumor 1 (re)activation in evidence for both epicardial progenitor and endothelial cells for cardiovascular regeneration. J. Mol. Cell. Cardiol. 84, 112–115. doi: 10.1016/j.yjmcc.2015.04.001
Ando, K., Takahashi, M., Yamagishi, T., Miyagawa-Tomita, S., Imanaka-Yoshida, K., Yoshida, T., et al. (2011). Tenascin C may regulate the recruitment of smooth muscle cells during coronary artery development. Differentiation 81, 299–306. doi: 10.1016/j.diff.2011.03.002
Aranega, A. E., and Franco, D. (2015). “Post-transcriptional regulation by proteins and non-coding RNAs,” in Congenital Heart Diseases: The Broken Heart, eds S. Rickert-Sperling, R. G. Kelly, D. J. Driscoll (Wien: Springer), 153–172.
Azambuja, A. P., Portillo-Sánchez, V., Rodrigues, M. V., Omae, S. V., Schechtman, D., Strauss, B. E., et al. (2010). Retinoic acid and VEGF delay smooth muscle relative to endothelial differentiation to coordinate inner and outer coronary vessel wall morphogenesis. Circ. Res. 107, 204–216. doi: 10.1161/circresaha.109.214650
Baek, S. T., and Tallquist, M. D. (2012). Nf1 limits epicardial derivative expansion by regulating epithelial to mesenchymal transition and proliferation. Development 139, 2040–2049. doi: 10.1242/dev.074054
Balmer, G. M., Bollini, S., Dubé, K. N., Martinez-Barbera, J. P., Williams, O., and Riley, P. R. (2014). Dynamic haematopoietic cell contribution to the developing and adult epicardium. Nat. Commun. 5:4054. doi: 10.1038/ncomms5054
Batal, O., Schoenhagen, P., Shao, M., Ayyad, A. E., Van Wagoner, D. R., Halliburton, S. S., et al. (2010). Left atrial epicardial adiposity and atrial fibrillation. Circ. Arrhythm. Electrophysiol. 3, 230–236. doi: 10.1161/CIRCEP.110.957241
Bax, N. A., Lie-Venema, H., Vicente-Steijn, R., Bleyl, S. B., Van Den Akker, N. M., Maas, S., et al. (2009). Platelet-derived growth factor is involved in the differentiation of second heart field-derived cardiac structures in chicken embryos. Dev. Dyn. 238, 2658–2669. doi: 10.1002/dvdy.22073
Becker, R. O., Chapin, S., and Sherry, R. (1974). Regeneration of the ventricular myocardium in amphibians. Nature 248, 145–147.
Bednarek, D., González-Rosa, J. M., Guzmán-Martínez, G., Gutiérrez-Gutiérrez, Ó., Aguado, T., Sánchez-Ferrer, C., et al. (2015). Telomerase is essential for zebrafish heart regeneration. Cell Rep. 12, 1691–1703. doi: 10.1016/j.celrep.2015.07.064
Behbahani, G. D., Ghahhari, N. M., Javidi, M. A., Molan, A. F., Feizi, N., and Babashah, S. (2016). MicroRNA-mediated post-transcriptional regulation of epithelial to mesenchymal transition in cancer. Pathol. Oncol. Res. 23, 1–12. doi: 10.1007/s12253-016-0101-6
Bergmann, O., Bhardwaj, R. D., Bernard, S., Zdunek, S., Barnabé-Heider, F., Walsh, S., et al. (2009). Evidence for cardiomyocyte renewal in humans. Science 324, 98–102. doi: 10.1126/science.1164680
Bollini, S., Riley, P. R., and Smart, N. (2015). Thymosin β4: multiple functions in protection, repair and regeneration of the mammalian heart. Expert Opin. Biol. Ther. 15(Suppl. 1), S163–S174. doi: 10.1517/14712598.2015.1022526
Bollini, S., Smart, N., and Riley, P. R. (2011). Resident cardiac progenitor cells: at the heart of regeneration. J. Mol. Cell. Cardiol. 50, 296–303. doi: 10.1016/j.yjmcc.2010.07.006
Bollini, S., Vieira, J. M., Howard, S., Dubè, K. N., Balmer, G. M., Smart, N., et al. (2014). Re-activated adult epicardial progenitor cells are a heterogeneous population molecularly distinct from their embryonic counterparts. Stem Cells Dev. 23, 1719–1730. doi: 10.1089/scd.2014.0019
Bonet, F., Dueñas, Á., López-Sánchez, C., García-Martínez, V., Aránega, A. E., and Franco, D. (2015). MiR-23b and miR-199a impair epithelial-to-mesenchymal transition during atrioventricular endocardial cushion formation. Dev. Dyn. 244, 1259–1275. doi: 10.1002/dvdy.24309
Bonet, F., Hernandez-Torres, F., Esteban, F. J., Aranega, A., and Franco, D. (2013). Comparative analyses of microRNA microarrays during cardiogenesis: functional perspectives. Microarrays 2, 81–96. doi: 10.3390/microarrays2020081
Braitsch, C. M., Combs, M. D., Quaggin, S. E., and Yutzey, K. E. (2012). Pod1/Tcf21 is regulated by retinoic acid signaling and inhibits differentiation of epicardium-derived cells into smooth muscle in the developing heart. Dev. Biol. 368, 345–357. doi: 10.1016/j.ydbio.2012.06.002
Braitsch, C. M., Kanisicak, O., van Berlo, J. H., Molkentin, J. D., and Yutzey, K. E. (2013). Differential expression of embryonic epicardial progenitor markers and localization of cardiac fibrosis in adult ischemic injury and hypertensive heart disease. J. Mol. Cell. Cardiol. 65, 108–119. doi: 10.1016/j.yjmcc.2013.10.005
Brønnum, H., Andersen, D. C., Schneider, M., Nossent, A. Y., Nielsen, S. B., and Sheikh, S. P. (2013a). Islet-1 is a dual regulator of fibrogenic epithelial-to-mesenchymal transition in epicardial mesothelial cells. Exp. Cell Res. 319, 424–435. doi: 10.1016/j.yexcr.2012.12.019
Brønnum, H., Andersen, D. C., Schneider, M., Sandberg, M. B., Eskildsen, T., Nielsen, S. B., et al. (2013b). miR-21 promotes fibrogenic epithelial-to-mesenchymal transition of epicardial mesothelial cells involving Programmed Cell Death 4 and Sprouty-1. PLoS ONE 8:e56280. doi: 10.1371/journal.pone.0056280
Cai, C. L., Martin, J. C., Sun, Y., Cui, L., Wang, L., Ouyang, K., et al. (2008). A myocardial lineage derives from Tbx18 epicardial cells. Nature 454, 104–108. doi: 10.1038/nature06969
Callis, T. E., and Wang, D. Z. (2008). Taking microRNAs to heart. Trends Mol. Med. 14, 254–260. doi: 10.1016/j.molmed.2008.03.006
Cano, E., Carmona, R., Ruiz-Villalba, A., Rojas, A., Chau, Y. Y., Wagner, K. D., et al. (2016). Extracardiac septum transversum/proepicardial endothelial cells pattern embryonic coronary arterio-venous connections. Proc. Natl. Acad. Sci. U.S.A. 113, 656–661. doi: 10.1073/pnas.1509834113
Cao, J., and Poss, K. D. (2016). Explant culture of adult zebrafish hearts for epicardial regeneration studies. Nat. Protoc. 11, 872–881. doi: 10.1038/nprot.2016.049
Cavallero, S., Shen, H., Yi, C., Lien, C. L., Kumar, S. R., and Sucov, H. M. (2015). CXCL12 signaling is essential for maturation of the ventricular coronary endothelial plexus and establishment of functional coronary circulation. Dev. Cell 33, 469–477. doi: 10.1016/j.devcel.2015.03.018
Chen, H. I., Sharma, B., Akerberg, B. N., Numi, H. J., Kivelä, R., Saharinen, P., et al. (2014). The sinus venosus contributes to coronary vasculature through VEGFC-stimulated angiogenesis. Development 141, 4500–4512. doi: 10.1242/dev.113639
Chen, J., and Wang, D. Z. (2012). microRNAs in cardiovascular development. J. Mol. Cell. Cardiol. 52, 949–957. doi: 10.1016/j.yjmcc.2012.01.012
Chinchilla, A., Lozano, E., Daimi, H., Esteban, F. J., Crist, C., Aranega, A. E., et al. (2011). MicroRNA profiling during mouse ventricular maturation: a role for miR-27 modulating Mef2c expression. Cardiovasc. Res. 89, 98–108. doi: 10.1093/cvr/cvq264
Chong, J. J., Chandrakanthan, V., Xaymardan, M., Asli, N. S., Li, J., Ahmed, I., et al. (2011). Adult cardiac-resident MSC-like stem cells with a proepicardial origin. Cell Stem Cell 9, 527–540. doi: 10.1016/j.stem.2011.10.002
Christoffels, V. M., Grieskamp, T., Norden, J., Mommersteeg, M. T., Rudat, C., and Kispert, A. (2009). Tbx18 and the fate of epicardial progenitors. Nature 458, E8–E9; discussion: E9–E10. doi: 10.1038/nature07916
Cordes, K. R., and Srivastava, D. (2009). MicroRNA regulation of cardiovascular development. Circ. Res. 104, 724–732. doi: 10.1161/CIRCRESAHA.108.192872
Cossette, S., and Misra, R. (2011). The identification of different endothelial cell populations within the mouse proepicardium. Dev. Dyn. 240, 2344–2353. doi: 10.1002/dvdy.22724
Craig, E. A., Austin, A. F., Vaillancourt, R. R., Barnett, J. V., and Camenisch, T. D. (2010a). TGFβ2-mediated production of hyaluronan is important for the induction of epicardial cell differentiation and invasion. Exp. Cell Res. 316, 3397–3405. doi: 10.1016/j.yexcr.2010.07.006
Craig, E. A., Parker, P., Austin, A. F., Barnett, J. V., and Camenisch, T. D. (2010b). Involvement of the MEKK1 signaling pathway in the regulation of epicardial cell behavior by hyaluronan. Cell. Signal. 22, 968–976. doi: 10.1016/j.cellsig.2010.02.004
Derda, A. A., Thum, S., Lorenzen, J. M., Bavendiek, U., Heineke, J., Keyser, B., et al. (2015). Blood-based microRNA signatures differentiate various forms of cardiac hypertrophy. Int. J. Cardiol. 196, 115–122. doi: 10.1016/j.ijcard.2015.05.185
Dettman, R. W., Denetclaw, W. Jr., Ordahl, C. P., and Bristow, J. (1998). Common epicardial origin of coronary vascular smooth muscle, perivascular fibroblasts, and intermyocardial fibroblasts in the avian heart. Dev. Biol. 193, 169–181. doi: 10.1006/dbio.1997.8801
Diman, N. Y., Brooks, G., Kruithof, B. P., Elemento, O., Seidman, J. G., Seidman, C. E., et al. (2014). Tbx5 is required for avian and Mammalian epicardial formation and coronary vasculogenesis. Circ. Res. 115, 834–844. doi: 10.1161/CIRCRESAHA.115.304379
Dokic, D., and Dettman, R. W. (2006). VCAM-1 inhibits TGFbeta stimulated epithelial-mesenchymal transformation by modulating Rho activity and stabilizing intercellular adhesion in epicardial mesothelial cells. Dev. Biol. 299, 489–504. doi: 10.1016/j.ydbio.2006.08.054
Duan, J., Gherghe, C., Liu, D., Hamlett, E., Srikantha, L., Rodgers, L., et al. (2012). Wnt1/βcatenin injury response activates the epicardium and cardiac fibroblasts to promote cardiac repair. EMBO J. 31, 429–442. doi: 10.1038/emboj.2011.418
Foglio, E., Puddighinu, G., Fasanaro, P., D'Arcangelo, D., Perrone, G. A., Mocini, D., et al. (2015). Exosomal clusterin, identified in the pericardial fluid, improves myocardial performance following MI through epicardial activation, enhanced arteriogenesis and reduced apoptosis. Int. J. Cardiol. 197, 333–347. doi: 10.1016/j.ijcard.2015.06.008
Franco, D., Meilhac, S. M., Christoffels, V. M., Kispert, A., Buckingham, M., and Kelly, R. G. (2006). Left and right ventricular contributions to the formation of the interventricular septum in the mouse heart. Dev. Biol. 294, 366–375. doi: 10.1016/j.ydbio.2006.02.045
Gaborit, B., Abdesselam, I., and Dutour, A. (2013). Epicardial fat: more than just an “epi” phenomenon? Horm. Metab. Res. 45, 991–1001. doi: 10.1055/s-0033-1358669
Gajzer, D. C., Balbin, J., and Chaudhry, H. W. (2013). Thymosin β4 and cardiac regeneration: are we missing a beat? Stem Cell Rev. 9, 303–312. doi: 10.1007/s12015-012-9378-3
Gemberling, M., Karra, R., Dickson, A. L., and Poss, K. D. (2015). Nrg1 is an injury-induced cardiomyocyte mitogen for the endogenous heart regeneration program in zebrafish. elife 4:e05871. doi: 10.7554/eLife.05871
Gittenberger-de Groot, A. C., Winter, E. M., Bartelings, M. M., Goumans, M. J., DeRuiter, M. C., and Poelmann, R. E. (2012). The arterial and cardiac epicardium in development, disease and repair. Differentiation 84, 41–53. doi: 10.1016/j.diff.2012.05.002
González-Rosa, J. M., Martín, V., Peralta, M., Torres, M., and Mercader, N. (2011). Extensive scar formation and regression during heart regeneration after cryoinjury in zebrafish. Development 138, 1663–1674. doi: 10.1242/dev.060897
González-Rosa, J. M., Peralta, M., and Mercader, N. (2012). Pan-epicardial lineage tracing reveals that epicardium derived cells give rise to myofibroblasts and perivascular cells during zebrafish heart regeneration. Dev. Biol. 370, 173–186. doi: 10.1016/j.ydbio.2012.07.007
Greulich, F., Farin, H. F., Schuster-Gossler, K., and Kispert, A. (2012). Tbx18 function in epicardial development. Cardiovasc. Res. 96, 476–483. doi: 10.1093/cvr/cvs277
Grote, P., Wittler, L., Hendrix, D., Koch, F., Währisch, S., Beisaw, A., et al. (2013). The tissue-specific lncRNA Fendrr is an essential regulator of heart and body wall development in the mouse. Dev. Cell 24, 206–214. doi: 10.1016/j.devcel.2012.12.012
Guadix, J. A., Carmona, R., Muñoz-Chápuli, R., and Pérez-Pomares, J. M. (2006). In vivo and in vitro analysis of the vasculogenic potential of avian proepicardial and epicardial cells. Dev. Dyn. 235, 1014–1026. doi: 10.1002/dvdy.20685
Gupta, S. K., Itagaki, R., Zheng, X., Batkai, S., Thum, S., Ahmad, F., et al. (2016). miR-21 promotes fibrosis in an acute cardiac allografttransplantation model. Cardiovasc. Res. 110, 215–226. doi: 10.1093/cvr/cvw030
Hammond, S. M. (2015). An overview of microRNAs. Adv. Drug Deliv. Rev. 87, 3–14. doi: 10.1016/j.addr.2015.05.001
Han, P., Zhou, X. H., Chang, N., Xiao, C. L., Yan, S., Ren, H., et al. (2014). Hydrogen peroxide primes heart regeneration with a derepression mechanism. Cell Res. 24, 1091–1107. doi: 10.1038/cr.2014.108
He, L., Tian, X., Zhang, H., Wythe, J. D., and Zhou, B. (2014). Fabp4-CreER lineage tracing reveals two distinctive coronary vascular populations. J. Cell. Mol. Med. 18, 2152–2156. doi: 10.1111/jcmm.12415
Hirose, T., Karasawa, M., Sugitani, Y., Fujisawa, M., Akimoto, K., Ohno, S., et al. (2006). PAR3 is essential for cyst-mediated epicardial development by establishing apical cortical domains. Development 133, 1389–1398. doi: 10.1242/dev.02294
Huang, G. N., Thatcher, J. E., McAnally, J., Kong, Y., Qi, X., Tan, W., et al. (2012). C/EBP transcription factors mediate epicardial activation during heart development and injury. Science 338, 1599–1603. doi: 10.1126/science.1229765
Icardo, J. M., Guerrero, A., Durán, A. C., Colvee, E., Domezain, A., and Sans-Coma, V. (2009). The development of the epicardium in the sturgeon Acipenser naccarii. Anat. Rec. (Hoboken). 292, 1593–1601. doi: 10.1002/ar.20939
Itou, J., Akiyama, R., Pehoski, S., Yu, X., Kawakami, H., and Kawakami, Y. (2014). Regenerative responses after mild heart injuries for cardiomyocyte proliferation in zebrafish. Dev. Dyn. 243, 1477–1486. doi: 10.1002/dvdy.24171
Iwayama, T., Nitobe, J., Watanabe, T., Ishino, M., Tamura, H., Nishiyama, S., et al. (2014). Role of epicardial adipose tissue in coronary artery disease in non-obese patients. J. Cardiol. 63, 344–349. doi: 10.1016/j.jjcc.2013.10.002
Kálmán, F., Virágh, S., and Módis, L. (1995). Cell surface glycoconjugates and theextracellular matrix of the developing mouse embryo epicardium. Anat. Embryol. 191, 451–464. doi: 10.1007/BF00304430
Karra, R., Knecht, A. K., Kikuchi, K., and Poss, K. D. (2015). Myocardial NF-κB activation is essential for zebrafish heart regeneration. Proc. Natl. Acad. Sci. U.S.A. 112, 13255–13260. doi: 10.1073/pnas.1511209112
Katz, T. C., Singh, M. K., Degenhardt, K., Rivera-Feliciano, J., Johnson, R. L., Epstein, J. A., et al. (2012). Distinct compartments of the proepicardial organ give rise to coronary vascular endothelial cells. Dev. Cell 22, 639–650. doi: 10.1016/j.devcel.2012.01.012
Kelly, R. G., and Buckingham, M. E. (2002). The anterior heart-forming field: voyage to the arterial pole of the heart. Trends Genet. 18, 210–216. doi: 10.1016/S0168-9525(02)02642-2
Kelly, R. G., Brown, N. A., and Buckingham, M. E. (2001). The arterial pole of the mouse heart forms from Fgf10-expressing cells in pharyngeal mesoderm. Dev. Cell 1, 435–440. doi: 10.1016/S1534-5807(01)00040-5
Kennedy-Lydon, T., and Rosenthal, N. (2015). Cardiac regeneration: epicardial mediated repair. Proc. Biol. Sci. 282:20152147. doi: 10.1098/rspb.2015.2147
Kikuchi, K., Gupta, V., Wang, J., Holdway, J. E., Wills, A. A., Fang, Y., et al. (2011). tcf21+ epicardial cells adopt non-myocardial fates during zebrafish heart development and regeneration. Development 138, 2895–2902. doi: 10.1242/dev.067041
Kim, J., Wu, Q., Zhang, Y., Wiens, K. M., Huang, Y., Rubin, N., et al. (2010). PDGF signaling is required for epicardial function and blood vessel formation in regenerating zebrafish hearts. Proc. Natl. Acad. Sci. U.S.A. 107, 17206–17210. doi: 10.1073/pnas.0915016107
Kirby, M. L., and Waldo, K. L. (1990). Role of neural crest in congenital heart disease. Circulation 82, 332–340. doi: 10.1161/01.CIR.82.2.332
Kirby, M. L., and Waldo, K. L. (1995). Neural crest and cardiovascular patterning. Circ. Res. 77, 211–215. doi: 10.1161/01.RES.77.2.211
Klattenhoff, C. A., Scheuermann, J. C., Surface, L. E., Bradley, R. K., Fields, P. A., Steinhauser, M. L., et al. (2013). Braveheart, a long noncoding RNA required for cardiovascular lineage commitment. Cell 152, 570–583. doi: 10.1016/j.cell.2013.01.003
Kolander, K. D., Holtz, M. L., Cossette, S. M., Duncan, S. A., and Misra, R. P. (2014). Epicardial GATA factors regulate early coronary vascular plexus formation. Dev. Biol. 386, 204–215. doi: 10.1016/j.ydbio.2013.12.033
Kruithof, B. P., van Wijk, B., Somi, S., Kruithof-de Julio, M., Pérez Pomares, J. M., Weesie, F., et al. (2006). BMP and FGF regulate the differentiation of multipotential pericardial mesoderm into the myocardial or epicardial lineage. Dev. Biol. 295, 507–522. doi: 10.1016/j.ydbio.2006.03.033
Kurian, L., Aguirre, A., Sancho-Martinez, I., Benner, C., Hishida, T., Nguyen, T. B., et al. (2015). Identification of novel long noncoding RNAs underlying vertebrate cardiovascular development. Circulation 131, 1278–1290. doi: 10.1161/CIRCULATIONAHA.114.013303
Langlois, D., Hneino, M., Bouazza, L., Parlakian, A., Sasaki, T., Bricca, G., et al. (2010). Conditional inactivation of TGF-β type II receptor in smooth muscle cells and epicardium causes lethal aortic and cardiac defects. Transgenic Res. 19, 1069–1082. doi: 10.1007/s11248-010-9379-4
Lepilina, A., Coon, A. N., Kikuchi, K., Holdway, J. E., Roberts, R. W., Burns, C. G., et al. (2006). A dynamic epicardial injury response supports progenitor cell activity during zebrafish heart regeneration. Cell 127, 607–619. doi: 10.1016/j.cell.2006.08.052
Liberatore, C. M., and Yutzey, K. E. (2004). MAP kinase activation in avian cardiovascular development. Dev. Dyn. 230, 773–780. doi: 10.1002/dvdy.20092
Lien, C. L., Harrison, M. R., Tuan, T. L., and Starnes, V. A. (2012). Heart repair and regeneration: recent insights from zebrafish studies. Wound Repair Regen. 20, 638–646. doi: 10.1111/j.1524-475X.2012.00814.x
Lien, C. L., Schebesta, M., Makino, S., Weber, G. J., and Keating, M. T. (2006). Gene expression analysis of zebrafish heart regeneration. PLoS Biol. 4:e260. doi: 10.1371/journal.pbio.0040260
Lie-Venema, H., Eralp, I., Markwald, R. R., van den Akker, N. M., Wijffels, M. C., Kolditz, D. P., et al. (2008). Periostin expression by epicardium-derived cells is involved in the development of the atrioventricular valves and fibrous heart skeleton. Differentiation 76, 809–819. doi: 10.1111/j.1432-0436.2007.00262.x
Lin, F. J., You, L. R., Yu, C. T., Hsu, W. H., Tsai, M. J., and Tsai, S. Y. (2012). Endocardial cushion morphogenesis and coronary vessel development require chicken ovalbumin upstream promoter-transcription factor II. Arterioscler. Thromb. Vasc. Biol. 32, e135–e146. doi: 10.1161/ATVBAHA.112.300255
Liu, J., and Stainier, D. Y. (2010). Tbx5 and Bmp signaling are essential for proepicardium specification in zebrafish. Circ. Res. 106, 1818–1828. doi: 10.1161/CIRCRESAHA.110.217950
Lockhart, M. M., Phelps, A. L., van den Hoff, M. J., and Wessels, A. (2014). The epicardium and the development of the atrioventricular junction in the murine heart. J. Dev. Biol. 2, 1–17. doi: 10.3390/jdb2010001
Männer, J., Pérez-Pomares, J. M., Macías, D., and Muñoz-Chápuli, R. (2001). The origin, formation and developmental significance of the epicardium: a review. Cells Tissues Organs 169, 89–103. doi: 10.1159/000047867
Marín-Juez, R., Marass, M., Gauvrit, S., Rossi, A., Lai, S. L., Materna, S. C., et al. (2016). Fast revascularization of the injured area is essential to support zebrafish heart regeneration. Proc. Natl. Acad. Sci. U.S.A. 113, 11237–11242. doi: 10.1073/pnas.1605431113
Marro, J., Pfefferli, C., de Preux Charles, A. S., Bise, T., and Jaźwińska, A. (2016). Collagen XII contributes to epicardial and connective tissues in the zebrafish heart during ontogenesis and regeneration. PLoS ONE 11:e0165497. doi: 10.1371/journal.pone.0165497
Masters, M., and Riley, P. R. (2014). The epicardium signals the way towards heart regeneration. Stem Cell Res. 13(3 Pt B), 683–692. doi: 10.1016/j.scr.2014.04.007
Meilhac, S. M., Esner, M., Kelly, R. G., Nicolas, J. F., and Buckingham, M. E. (2004a). The clonal origin of myocardial cells in different regions of the embryonic mouse heart. Dev. Cell 6, 685–698. doi: 10.1016/S1534-5807(04)00133-9
Meilhac, S. M., Esner, M., Kerszberg, M., Moss, J. E., and Buckingham, M. E. (2004b). Oriented clonal cell growth in the developing mouse myocardium underlies cardiac morphogenesis. J. Cell Biol. 164, 97–109. doi: 10.1083/jcb.200309160
Meilhac, S. M., Kelly, R. G., Rocancourt, D., Eloy-Trinquet, S., Nicolas, J. F., and Buckingham, M. E. (2003). A retrospective clonal analysis of the myocardium reveals two phases of clonal growth in the developing mouse heart. Development 130, 3877–3889. doi: 10.1242/dev.00580
Mellgren, A. M., Smith, C. L., Olsen, G. S., Eskiocak, B., Zhou, B., Kazi, M. N., et al. (2008). Platelet-derived growth factor receptor beta signaling is required for efficient epicardial cell migration and development of two distinct coronary vascular smooth muscle cell populations. Circ. Res. 103, 1393–1401. doi: 10.1161/CIRCRESAHA.108.176768
Mercer, S. E., Odelberg, S. J., and Simon, H. G. (2013). A dynamic spatiotemporal extracellular matrix facilitates epicardial-mediated vertebrate heart regeneration. Dev. Biol. 382, 457–469. doi: 10.1016/j.ydbio.2013.08.002
Merki, E., Zamora, M., Raya, A., Kawakami, Y., Wang, J., Zhang, X., et al. (2005). Epicardial retinoid X receptor alpha is required for myocardial growth and coronary artery formation. Proc. Natl. Acad. Sci. U.S.A. 102, 18455–18460. doi: 10.1073/pnas.0504343102
Mikawa, T., and Gourdie, R. G. (1996). Pericardial mesoderm generates a population of coronary smooth muscle cells migrating into the heart along with ingrowth of the epicardial organ. Dev. Biol. 174, 221–232. doi: 10.1006/dbio.1996.0068
Mizutani, M., Wu, J. C., and Nusse, R. (2016). Fibrosis of the neonatal mouse heart after cryoinjury is accompanied by wnt signaling activation and epicardial-to-mesenchymal transition. J. Am. Heart Assoc. 5:e002457. doi: 10.1161/JAHA.115.002457
Nakanishi, K., Fukuda, S., Tanaka, A., Otsuka, K., Sakamoto, M., Taguchi, H., et al. (2012). Peri-atrial epicardial adipose tissue is associated with new-onset nonvalvular atrial fibrillation. Circ. J. 76, 2748–2754. doi: 10.1253/circj.CJ-12-0637
Niderla-Bielińska, J., Gula, G., Flaht-Zabost, A., Jankowska-Steifer, E., Czarnowska, E., Radomska-Leśniewska, D. M., et al. (2015). 3-D reconstruction and multiple marker analysis of mouse proepicardial endothelial cell population. Microvasc. Res. 102, 54–69. doi: 10.1016/j.mvr.2015.08.007
Oberpriller, J. O., and Oberpriller, J. C. (1974). Response of the adult newt ventricle to injury. J. Exp. Zool. 187, 249–253. doi: 10.1002/jez.1401870208
Peng, F., Xiong, L., Tang, H., Peng, C., and Chen, J. (2016). Regulation of epithelial-mesenchymal transition through microRNAs: clinical and biological significance of microRNAs in breast cancer. Tumour Biol. 37, 14463–14477. doi: 10.1007/s13277-016-5334-1
Peralta, M., González-Rosa, J. M., Marques, I. J., and Mercader, N. (2014). The epicardium in the embryonic and adult zebrafish. J. Dev. Biol. 2, 101–116. doi: 10.3390/jdb2020101
Peralta, M., Steed, E., Harlepp, S., González-Rosa, J. M., Monduc, F., Ariza-Cosano, A., et al. (2013). Heartbeat-driven pericardiac fluid forces contribute to epicardium morphogenesis. Curr. Biol. 23, 1726–1735. doi: 10.1016/j.cub.2013.07.005
Pérez-Pomares, J. M., Macías, D., García-Garrido, L., and Muñoz-Chápuli, R. (1998). The origin of the subepicardial mesenchyme in the avian embryo: an immunohistochemical and quail-chick chimera study. Dev. Biol. 200, 57–68. doi: 10.1006/dbio.1998.8949
Pérez-Pomares, J. M., Mironov, V., Guadix, J. A., Macías, D., Markwald, R. R., and Muñoz-Chápuli, R. (2006). In vitro self-assembly of proepicardial cell aggregates: an embryonic vasculogenic model for vascular tissue engineering. Anat. Rec. A Discov. Mol. Cell. Evol. Biol. 288, 700–713. doi: 10.1002/ar.a.20338
Pérez-Pomares, J. M., Phelps, A., Sedmerova, M., Carmona, R., González-Iriarte, M., Muñoz-Chápuli, R., et al. (2002). Experimental studies on the spatiotemporal expression of WT1 and RALDH2 in the embryonic avian heart: a model for the regulation of myocardial and valvuloseptal development by epicardially derived cells (EPDCs). Dev. Biol. 247, 307–326. doi: 10.1006/dbio.2002.0706
Philippen, L. E., Dirkx, E., da Costa-Martins, P. A., and De Windt, L. J. (2015). Non-coding RNA in control of gene regulatory programs in cardiac development and disease. J. Mol. Cell. Cardiol. 89(Pt A), 51–58. doi: 10.1016/j.yjmcc.2015.03.014
Phillips, H. M., Hildreth, V., Peat, J. D., Murdoch, J. N., Kobayashi, K., Chaudhry, B., et al. (2008). Non-cell-autonomous roles for the planar cell polarity gene Vangl2 in development of the coronary circulation. Circ. Res. 102, 615–623. doi: 10.1161/CIRCRESAHA.107.160861
Phillips, M. D., Mukhopadhyay, M., Poscablo, C., and Westphal, H. (2011). Dkk1 and Dkk2 regulate epicardial specification during mouse heart development. Int. J. Cardiol. 150, 186–192. doi: 10.1016/j.ijcard.2010.04.007
Plavicki, J., Hofsteen, P., Peterson, R. E., and Heideman, W. (2013). Dioxin inhibits zebrafish epicardium and proepicardium development. Toxicol. Sci. 131, 558–567. doi: 10.1093/toxsci/kfs301
Plavicki, J. S., Hofsteen, P., Yue, M. S., Lanham, K. A., Peterson, R. E., and Heideman, W. (2014). Multiple modes of proepicardial cell migration require heartbeat. BMC Dev. Biol. 14:18. doi: 10.1186/1471-213X-14-18
Poelmann, R. E., Gittenberger-de Groot, A. C., Mentink, M. M., Bökenkamp, R., and Hogers, B. (1993). Development of the cardiac coronary vascular endothelium, studied with antiendothelial antibodies, in chicken-quail chimeras. Circ. Res. 73, 559–568. doi: 10.1161/01.RES.73.3.559
Porrello, E. R., Mahmoud, A. I., Simpson, E., Hill, J. A., Richardson, J. A., Olson, E. N., et al. (2011). Transient regenerative potential of the neonatal mouse heart. Science 331, 1078–1080. doi: 10.1126/science.1200708
Porrello, E. R., Mahmoud, A. I., Simpson, E., Johnson, B. A., Grinsfelder, D., Canseco, D., et al. (2013). Regulation of neonatal and adult mammalian heart regeneration by the miR-15 family. Proc. Natl. Acad. Sci. U.S.A. 110, 187–192. doi: 10.1073/pnas.1208863110
Poss, K. D., Wilson, L. G., and Keating, M. T. (2002). Heart regeneration in zebrafish. Science 298, 2188–2190. doi: 10.1126/science.1077857
Ratajska, A., Czarnowska, E., and Ciszek, B. (2008). Embryonic development of the proepicardium and coronary vessels. Int. J. Dev. Biol. 52, 229–236. doi: 10.1387/ijdb.072340ar
Red-Horse, K., Ueno, H., Weissman, I. L., and Krasnow, M. A. (2010). Coronary arteries form by developmental reprogramming of venous cells. Nature 464, 549–553. doi: 10.1038/nature08873
Rodius, S., Androsova, G., Götz, L., Liechti, R., Crespo, I., Merz, S., et al. (2016). Analysis of the dynamic co-expression network of heart regeneration in the zebrafish. Sci. Rep. 6:26822. doi: 10.1038/srep26822
Rudat, C., and Kispert, A. (2012). Wt1 and epicardial fate mapping. Circ. Res. 111, 165–169. doi: 10.1161/CIRCRESAHA.112.273946
Rui, L., Yu, N., Hong, L., Feng, H., Chunyong, H., Jian, M., et al. (2014). Extending the time window of mammalian heart regeneration by thymosin beta 4. J. Cell. Mol. Med. 18, 2417–2424. doi: 10.1111/jcmm.12421
Ruiz-Villalba, A., Simón, A. M., Pogontke, C., Castillo, M. I., Abizanda, G., Pelacho, B., et al. (2015). Interacting resident epicardium-derived fibroblasts and recruited bone marrow cells form myocardial infarction scar. J. Am. Coll. Cardiol. 65, 2057–2066. doi: 10.1016/j.jacc.2015.03.520
Sánchez, N. S., and Barnett, J. V. (2012). TGFβ and BMP-2 regulate epicardial cell invasion via TGFβR3 activation of the Par6/Smurf1/RhoA pathway. Cell. Signal. 24, 539–548. doi: 10.1016/j.cellsig.2011.10.006
Sauvageau, M., Goff, L. A., Lodato, S., Bonev, B., Groff, A. F., Gerhardinger, C., et al. (2013). Multiple knockout mouse models reveal lincRNAs are required for life and brain development. elife 2:e01749. doi: 10.7554/eLife.01749
Schlueter, J., and Brand, T. (2012). Epicardial progenitor cells in cardiac development and regeneration. J. Cardiovasc. Transl. Res. 5, 641–653. doi: 10.1007/s12265-012-9377-4
Schnabel, K., Wu, C. C., Kurth, T., and Weidinger, G. (2011). Regeneration of cryoinjury induced necrotic heart lesions in zebrafish is associated with epicardial activation and cardiomyocyte proliferation. PLoS ONE 6:e18503. doi: 10.1371/journal.pone.0018503
Schulte, I., Schlueter, J., Abu-Issa, R., Brand, T., and Männer, J. (2007). Morphological and molecular left-right asymmetries in the development of the proepicardium: a comparative analysis on mouse and chick embryos. Dev. Dyn. 236, 684–695. doi: 10.1002/dvdy.21065
Seeger, T., Xu, Q. F., Muhly-Reinholz, M., Fischer, A., Kremp, E. M., Zeiher, A. M., et al. (2016). Inhibition of let-7 augments the recruitment of epicardial cells and improves cardiac function after myocardial infarction. J. Mol. Cell. Cardiol. 94, 145–152. doi: 10.1016/j.yjmcc.2016.04.002
Serluca, F. C. (2008). Development of the proepicardial organ in the zebrafish. Dev. Biol. 315, 18–27. doi: 10.1016/j.ydbio.2007.10.007
Shen, J., and Hung, M. C. (2015). Signaling-mediated regulation of MicroRNA processing. Cancer Res. 75, 783–791. doi: 10.1158/0008-5472.CAN-14-2568
Singh, A., Ramesh, S., Cibi, D. M., Yun, L. S., Li, J., Li, L., et al. (2016). Hippo signaling mediators yap and taz are required in the epicardium for coronary vasculature development. Cell Rep. 15, 1384–1393. doi: 10.1016/j.celrep.2016.04.027
Singh, M. K., Lu, M. M., Massera, D., and Epstein, J. A. (2011). MicroRNA-processing enzyme Dicer is required in epicardium for coronary vasculature development. J. Biol. Chem. 286, 41036–41045. doi: 10.1074/jbc.M111.268573
Smart, N., and Riley, P. R. (2012). The epicardium as a candidate for heart regeneration. Future Cardiol. 8, 53–69. doi: 10.2217/fca.11.87
Smart, N., Bollini, S., Dubé, K. N., Vieira, J. M., Zhou, B., Davidson, S., et al. (2011). De novo cardiomyocytes from within the activated adult heart after injury. Nature 474, 640–644. doi: 10.1038/nature10188
Smart, N., Bollini, S., Dubé, K. N., Vieira, J. M., Zhou, B., Riegler, J., et al. (2012). Myocardial regeneration: expanding the repertoire of thymosin β4 in the ischemic heart. Ann. N.Y. Acad. Sci. 1269, 92–101. doi: 10.1111/j.1749-6632.2012.06708.x
Smith, C. L., Baek, S. T., Sung, C. Y., and Tallquist, M. D. (2011). Epicardial-derived cell epithelial-to-mesenchymal transition and fate specification require PDGF receptor signaling. Circ. Res. 108, e15–e26. doi: 10.1161/CIRCRESAHA.110.235531
Stankunas, K., Ma, G. K., Kuhnert, F. J., Kuo, C. J., and Chang, C. P. (2010). VEGF signaling has distinct spatiotemporal roles during heart valve development. Dev. Biol. 347, 325–336. doi: 10.1016/j.ydbio.2010.08.030
Sulaiman, S. A., Ab Mutalib, N. S., and Jamal, R. (2016). miR-200c regulation of metastases in ovarian cancer: potential role in epithelial and mesenchymal transition. Front. Pharmacol. 7:271. doi: 10.3389/fphar.2016.00271
Takeichi, M., Nimura, K., Mori, M., Nakagami, H., and Kaneda, Y. (2013). The transcription factors Tbx18 and Wt1 control the epicardial epithelial-mesenchymal transition through bi-directional regulation of Slug in murine primary epicardial cells. PLoS ONE 8:e57829. doi: 10.1371/journal.pone.0057829
Talman, A. H., Psaltis, P. J., Cameron, J. D., Meredith, I. T., Seneviratne, S. K., and Wong, D. T. (2014). Epicardial adipose tissue: far more than a fat depot. Cardiovasc. Diagn. Ther. 4, 416–429. doi: 10.3978/j.issn.2223-3652.2014.11.05
Thum, T., Gross, C., Fiedler, J., Fischer, T., Kissler, S., Bussen, M., et al. (2008). MicroRNA-21 contributes to myocardial disease by stimulating MAP kinase signalling in fibroblasts. Nature 456, 980–984. doi: 10.1038/nature07511
Tian, X., Hu, T., Zhang, H., He, L., Huang, X., Liu, Q., et al. (2013). Subepicardial endothelial cells invade the embryonic ventricle wall to form coronary arteries. Cell Res. 23, 1075–1090. doi: 10.1038/cr.2013.83
Tomanek, R. J., Ishii, Y., Holifield, J. S., Sjogren, C. L., Hansen, H. K., and Mikawa, T. (2006). VEGF family members regulate myocardial tubulogenesis and coronary artery formation in the embryo. Circ. Res. 98, 947–953. doi: 10.1161/01.RES.0000216974.75994.da
Towler, B. P., Jones, C. I., and Newbury, S. F. (2015). Mechanisms of regulation of mature miRNAs. Biochem. Soc. Trans. 43, 1208–1214. doi: 10.1042/BST20150157
Trembley, M. A., Velasquez, L. S., de Mesy Bentley, K. L., and Small, E. M. (2015). Myocardin-related transcription factors control the motility of epicardium-derived cells and the maturation of coronary vessels. Development 142, 21–30. doi: 10.1242/dev.116418
Urayama, K., Guilini, C., Turkeri, G., Takir, S., Kurose, H., Messaddeq, N., et al. (2008). Prokineticin receptor-1 induces neovascularization and epicardial-derived progenitor cell differentiation. Arterioscler. Thromb. Vasc. Biol. 28, 841–849. doi: 10.1161/ATVBAHA.108.162404
Valder, A. M., and Olson, M. D. (1994). Immuno localization of vascular smooth muscle precursors during coronary vasculogenesis in the embryonic quail heart. FASEB J. 8:A394.
van Wijk, B., Gunst, Q. D., Moorman, A. F., and van den Hoff, M. J. (2012). Cardiac regeneration from activated epicardium. PLoS ONE 7:e44692. doi: 10.1371/journal.pone.0044692
Vega-Hernández, M., Kovacs, A., De Langhe, S., and Ornitz, D. M. (2011). FGF10/FGFR2b signaling is essential for cardiac fibroblast development and growth of the myocardium. Development 138, 3331–3340. doi: 10.1242/dev.064410
Virágh, S., Gittenberger-de Groot, A. C., Poelmann, R. E., and Kálmán, F. (1993). Early development of quail heart epicardium and associated vascular and glandular structures. Anat. Embryol. 188, 381–393. doi: 10.1007/BF00185947
Vrancken Peeters, M. P., Gittenberger-de Groot, A. C., Mentink, M. M., and Poelmann, R. E. (1999). Smooth muscle cells and fibroblasts of the coronary arteries derive from epithelial-mesenchymal transformation of the epicardium. Anat. Embryol. 199, 367–378. doi: 10.1007/s004290050235
Wagner, N., Morrison, H., Pagnotta, S., Michiels, J. F., Schwab, Y., Tryggvason, K., et al. (2011). The podocyte protein nephrin is required for cardiac vessel formation. Hum. Mol. Genet. 20, 2182–2194. doi: 10.1093/hmg/ddr106
Wang, J., Cao, J., Dickson, A. L., and Poss, K. D. (2015). Epicardial regeneration is guided by cardiac outflow tract and Hedgehog signalling. Nature 522, 226–230. doi: 10.1038/nature14325
Watt, A. J., Battle, M. A., Li, J., and Duncan, S. A. (2004). GATA4 is essential for formation of the proepicardium and regulates cardiogenesis. Proc. Natl. Acad. Sci. U.S.A. 101, 12573–12578. doi: 10.1073/pnas.0400752101
Wei, K., Serpooshan, V., Hurtado, C., Diez-Cuñado, M., Zhao, M., Maruyama, S., et al. (2015). Epicardial FSTL1 reconstitution regenerates the adult mammalian heart. Nature 525, 479–485. doi: 10.1038/nature15372
Wessels, A., and Pérez-Pomares, J. M. (2004). The epicardium and epicardially derived cells (EPDCs) as cardiac stem cells. Anat. Rec. A Discov. Mol. Cell. Evol. Biol. 276, 43–57. doi: 10.1002/ar.a.10129
Winter, E. M., and Gittenberger-de Groot, A. C. (2007). Epicardium-derived cells in cardiogenesis and cardiac regeneration. Cell. Mol. Life Sci. 64, 692–703. doi: 10.1007/s00018-007-6522-3
Winter, E. M., van Oorschot, A. A., Hogers, B., van der Graaf, L. M., Doevendans, P. A., Poelmann, R. E., et al. (2009). A new direction for cardiac regeneration therapy: application of synergistically acting epicardium-derived cells and cardiomyocyte progenitor cells. Circ. Heart Fail. 2, 643–653. doi: 10.1161/CIRCHEARTFAILURE.108.843722
Wu, S. P., Dong, X. R., Regan, J. N., Su, C., and Majesky, M. W. (2013). Tbx18 regulates development of the epicardium and coronary vessels. Dev. Biol. 383, 307–320. doi: 10.1016/j.ydbio.2013.08.019
Wu, Y. C., Liu, C. Y., Chen, Y. H., Chen, R. F., Huang, C. J., and Wang, I. J. (2012). Blood vessel epicardial substance (Bves) regulates epidermal tight junction integrity through atypical protein kinase C. J. Biol. Chem. 287, 39887–39897. doi: 10.1074/jbc.M112.372078
Xiang, F. L., Liu, Y., Lu, X., Jones, D. L., and Feng, Q. (2014). Cardiac-specific overexpression of human stem cell factor promotes epicardial activation and arteriogenesis after myocardial infarction. Circ. Heart Fail. 7, 831–842. doi: 10.1161/CIRCHEARTFAILURE.114.001423
Yamaguchi, Y., Cavallero, S., Patterson, M., Shen, H., Xu, J., Kumar, S. R., et al. (2015). Adipogenesis and epicardial adipose tissue: a novel fate of the epicardium induced by mesenchymal transformation and PPARγ activation. Proc. Natl. Acad. Sci. U.S.A. 112, 2070–2075. doi: 10.1073/pnas.1417232112
Yamashita, K., Yamamoto, M. H., Ebara, S., Okabe, T., Saito, S., Hoshimoto, K., et al. (2014). Association between increased epicardial adipose tissue volume and coronary plaque composition. Heart Vessels 29, 569–577. doi: 10.1007/s00380-013-0398-y
Yan, S., and Jiao, K. (2016). Functions of miRNAs during mammalian heart development. Int. J. Mol. Sci. 17:789. doi: 10.3390/ijms17050789
Zeng, B., Ren, X. F., Cao, F., Zhou, X. Y., and Zhang, J. (2011). Developmental patterns and characteristics of epicardial cell markers Tbx18 and Wt1 in murine embryonic heart. J. Biomed. Sci. 18:67. doi: 10.1186/1423-0127-18-67
Zhang, H., Pu, W., Liu, Q., He, L., Huang, X., Tian, X., et al. (2016). Endocardium contributes to cardiac fat. Circ. Res. 118, 254–265. doi: 10.1161/CIRCRESAHA.115.307202
Zhao, L., Borikova, A. L., Ben-Yair, R., Guner-Ataman, B., MacRae, C. A., Lee, R. T., et al. (2014). Notch signaling regulates cardiomyocyte proliferation during zebrafish heart regeneration. Proc. Natl. Acad. Sci. U.S.A. 111, 1403–1408. doi: 10.1073/pnas.1311705111
Zhou, B., Honor, L. B., He, H., Ma, Q., Oh, J. H., Butterfield, C., et al. (2011). Adult mouse epicardium modulates myocardial injury by secreting paracrine factors. J. Clin. Invest. 121, 1894–1904. doi: 10.1172/JCI45529
Zhou, B., Ma, Q., Rajagopal, S., Wu, S. M., Domian, I., Rivera-Feliciano, J., et al. (2008). Epicardial progenitors contribute to the cardiomyocyte lineage in the developing heart. Nature 454, 109–113. doi: 10.1038/nature07060
Zhou, B., and Pu, W. T. (2011). Epicardial epithelial-to-mesenchymal transition in injured heart. J. Cell. Mol. Med. 15, 2781–2783. doi: 10.1111/j.1582-4934.2011.01450.x
Zhou, B., von Gise, A., Ma, Q., Hu, Y. W., and Pu, W. T. (2010). Genetic fate mapping demonstrates contribution of epicardium-derived cells to the annulus fibrosis of the mammalian heart. Dev. Biol. 338, 251–261. doi: 10.1016/j.ydbio.2009.12.007
Zhu, J. G., Shen, Y. H., Liu, H. L., Liu, M., Shen, Y. Q., Kong, X. Q., et al. (2014). Long noncoding RNAs expression profile of the developing mouse heart. J. Cell. Biochem. 115, 910–918. doi: 10.1002/jcb.24733
Keywords: epicardium, proepicardium, non-coding RNAs, heart development, regeneration
Citation: Dueñas A, Aranega AE and Franco D (2017) More than Just a Simple Cardiac Envelope; Cellular Contributions of the Epicardium. Front. Cell Dev. Biol. 5:44. doi: 10.3389/fcell.2017.00044
Received: 31 January 2017; Accepted: 10 April 2017;
Published: 01 May 2017.
Edited by:
Juan Jose Sanz-Ezquerro, Consejo Superior de Investigaciones Científicas, SpainReviewed by:
Nicola Smart, University of Oxford, UKManvendra K. Singh, DUKE-NUS Medical School, Singapore
Nadia Mercader, University of Bern, Switzerland
Copyright © 2017 Dueñas, Aranega and Franco. This is an open-access article distributed under the terms of the Creative Commons Attribution License (CC BY). The use, distribution or reproduction in other forums is permitted, provided the original author(s) or licensor are credited and that the original publication in this journal is cited, in accordance with accepted academic practice. No use, distribution or reproduction is permitted which does not comply with these terms.
*Correspondence: Diego Franco, ZGZyYW5jb0B1amFlbi5lcw==