- 1Division of Allergy and Immunology, Department of Pediatrics, Duke University Medical Center, Durham, NC, USA
- 2Institute of Biotherapy, School of Biotechnology, Southern Medical University, Guangzhou, China
- 3Department of Immunology, Duke University Medical Center, Durham, NC, USA
- 4Hematologic Malignancies and Cellular Therapies Program, Duke Cancer Institute, Duke University Medical Center, Durham, NC, USA
Diacylglycerol kinases (DGKs) are a family of enzymes that regulate the relative levels of diacylglycerol (DAG) and phosphatidic acid (PA) in cells by phosphorylating DAG to produce PA. Both DAG and PA are important second messengers cascading T cell receptor (TCR) signal by recruiting multiple effector molecules, such as RasGRP1, PKCθ, and mTOR. Studies have revealed important physiological functions of DGKs in the regulation of receptor signaling and the development and activation of immune cells. In this review, we will focus on recent progresses in our understanding of two DGK isoforms, α and ζ, in CD8 T effector and memory cell differentiation, regulatory T cell development and function, and invariant NKT cell development and effector lineage differentiation.
Introduction
Diacylglycerol (DAG) and phosphatidic acid (PA) are two key lipid second messengers that facilitate efficient receptor-mediated signaling in immune cells along with many other cells. They regulate numerous intracellular signaling molecules to control cell differentiation, proliferation, survival, and function. Following T cell receptor (TCR) engagement, DAG is produced through the activation of Phospholipase Cγ1 (PLCγ1), which hydrolyzes membrane phosphatidylinositol bisphosphate (PIP2) to DAG and inositol trisphosphate (IP3). DAG, together with other signal events, recruits downstream effector molecules to the membrane through their C1 domains and allosterically activates these effectors, with protein kinase Cθ (PKCθ), Ras guanyl–releasing protein 1 (RasGRP1), protein kinase D (PKD), Munc13s, and chimaerins being important for T cell development and/or function (Krishna and Zhong, 2013a; Merida et al., 2015).
DAG plays an important role in recruiting PKCθ to the plasma membrane and immune synapse in T cells (Diaz-Flores et al., 2003; Carrasco and Merida, 2004). The activation of PKCθ leads to TCR-mediated NF-κB and mammalian/mechanistic target of rapamycin complex 1 (mTORC1) activation in T cells (Sun et al., 2000; Isakov and Altman, 2002; Hamilton et al., 2014), which affects key processes, including T cell activation and survival (Manicassamy et al., 2006; Hayashi and Altman, 2007), IL-2 production (Werlen et al., 1998), TH2 responses (Cannons et al., 2004; Marsland et al., 2004), TH17 responses (Kwon et al., 2012), invariant NKT (iNKT) cell development and activation (Schmidt-Supprian et al., 2004; Fang et al., 2012), and Treg development (Gupta et al., 2008; Barnes et al., 2009; Medoff et al., 2009).
Ras guanyl–releasing protein 1 (RasGRP1) is another downstream molecule that is recruited to the cytoplasm membrane by DAG (Jones et al., 2002; Carrasco and Merida, 2004). RasGRP1 promotes activation of Ras by exchanging GDP for GTP, leading to the activation of the RAF1-MEK1/2-ERK1/2 pathway (Ebinu et al., 1998; Dower et al., 2000; Roose et al., 2005). Additionally, RasGRP1-Ras-Erk1/2 pathway functions upstream for TCR-induced mTORC1, mTORC2, and PI3K activation in T cells (Gorentla et al., 2011). RasGRP1 plays an essential role in conventional αβ T cell development (Dower et al., 2000; Fuller et al., 2012), particularly for the selection of thymocytes that express weak TCR signals (Priatel et al., 2002) and for early iNKT cell development (Shen et al., 2011a). While RasGRP1 appears dispensable for overall γδT cell development, it ensures IL-17 expressing γδT17 lineage differentiation and TCR-induced γδT cell activation (Chen et al., 2012). More recently, it was also found that RasGRP1, together with RasGRP3, promotes early thymic precursor generation (Golec et al., 2016). Additionally, RasGRP1 may play a role in promoting antigen-induced CD8 cell expansion by lowering the threshold of T cell activation (Priatel et al., 2010).
PKDs are recruited by both DAG and DAG-activated PKCs. Upon stimulation, inactive PKDs translocate from the cytosol to the plasma membrane in response to membrane DAG production, where they are then activated by novel PKCs (Rozengurt et al., 2005; Spitaler et al., 2006). PKDs have been shown to exert different effects on VDJ recombination at the TCRβ locus and on CD4 and CD8 expression during T cell development based on their localization at the cytosol or plasma membrane (Marklund et al., 2003; Spitaler et al., 2006). Additionally, PKD2 acts as a sensitive digital amplifier of TCR engagement, enabling CD8 T cells to match the production of inflammatory cytokines to the quality and quantity of TCR ligands (Navarro et al., 2014).
Munc13 proteins are mammalian homologs of the C. elegans Unc13, which are important for neurotransmitter secretion (Brose and Rosenmund, 2002). Munc13-1, Munc13-2, and Munc13-3 isoforms bind to DAG with high affinity. The Munc13-4 isoform lacks a C1 domain (Koch et al., 2000; Shirakawa et al., 2004), but it is involved in granule maturation and exocytosis in NK cells and cytotoxic T lymphocytes (CTLs) (Feldmann et al., 2003; Menager et al., 2007), phagosomal maturation, and the killing of intracellular bacteria in neutrophils (Johnson et al., 2011; Monfregola et al., 2012). Deficiency of Munc13-4 causes primary immune deficiency in patients (Feldmann et al., 2003; Cichocki et al., 2014).
Chimaerins possess Rac-specific GTPase Activating Protein (GAP) activity (Caloca et al., 1999; Yang and Kazanietz, 2007). Chimaerin isoforms α2 and β2 are expressed at different levels in T cells and have been shown to translocate to the immune synapse and to both participate in TCR signaling and receive regulation from it (Caloca et al., 2008; Siliceo and Merida, 2009). Chimaerins have been found to inhibit TCR-mediated NFAT activation and DAG-dependent actin polymerization to regulate T cell adhesion and chemotaxis (Siliceo et al., 2006).
Phosphatidic acid (PA) is produced both by the activity of DAG kinases (DGKs) and by the phospholipase D (PLD) family of enzymes in T cells. DGKs phosphorylate DAG to convert it to PA, while PLDs mediate the hydrolysis of phosphatidylcholine (Jenkins and Frohman, 2005; Zhong et al., 2008). The removal of PA is mediated by lipins, which can turn off PA-mediated signaling through dephosphorylation, and they have been shown to regulate mast cell function in the immune system (Csaki and Reue, 2010; Shin et al., 2013b). Intracellular levels of PA change dynamically in response to environmental stimuli (Wang et al., 2006). The downstream effector molecules of PA include a multitude of kinases, such as mTOR (Chen and Fang, 2002), phosphatidylinositol-4-phosphate 5-kinase (PIP5K) (Galandrini et al., 2005; Jarquin-Pardo et al., 2007; Micucci et al., 2008; Cockcroft, 2009; Yoon et al., 2011), spingosine kinase (SPHK ½), RAF1 (Ghosh et al., 1996; Shome et al., 1997; Rizzo et al., 1999, 2000; Andresen et al., 2002), and other molecules, such as Src homology region 2 domain-containing phosphatase 1 (SHP1) (Frank et al., 1999), kinase suppressor of Ras 1 (KSR1, a scaffolding protein that interacts with several components of the Raf-MEK-ERK cascade) (Morrison, 2001; Kraft et al., 2008), and Sos, another guanine nucleotide exchange factor for Ras activation (Zhao et al., 2007). Both PLD and DGK-derived PA has been shown to directly activate mTOR in non-T cells (Chen and Fang, 2002; Avila-Flores et al., 2005). In these cells, PA can also activate mTOR indirectly via ERK (Winter et al., 2010), but such a mechanism has not been examined in T cells. In T cells, DGKα and ζ mainly inhibit TCR-induced mTOR signaling by negative control of DAG-mediated RasGRP1 and likely PKCθ activation (Gorentla et al., 2011; Hamilton et al., 2014). However, DGK-derived PA has been shown to promote T cell maturation in the thymus (Guo et al., 2008) and to regulate innate immune responses (Liu et al., 2007). Future studies should determine the direct downstream of the effector(s) of PA that mediate its functions in these immune cells.
The diverse and important functions of DAG—and PA-mediated signaling suggest their levels must be tightly controlled temporally and spatially. DGKs switch from DAG-mediated signals to PA-mediated signals to dynamically regulate downstream pathways in response to the engagement of the TCR and many other receptors (Merida et al., 2008; Cai et al., 2009; Zhong et al., 2011). In mammals, there are ten DGK isoforms encoded by different genes, some of which also contain splicing variants, adding complexity to this family of enzymes. All DGKs contain a kinase domain and at least two cysteine-rich C1 domains but differ in the homology of their other structural domains as well as their interaction with other biomolecules. Based on their structural distinction and homology, DGKs are classified into five types that may differ in subcellular localization, function, and regulation. The existence of multiple isoforms poses a significant challenge in studying the physiological roles of any specific isoforms in cellular development and functions due to functional redundancies, a fact demonstrated in conventional αβ T cell and iNKT cell development in mice deficient in both DGKα and DGKζ (Guo et al., 2008; Shen et al., 2011b). Of these ten isoforms, DGKα and DGKζ as well as DGKδ are the major isoforms expressed in T cells (Zhong et al., 2002; Olenchock et al., 2006a; Sakane et al., 2007). Both DGKα and ζ have been found to regulate multiple signaling pathways downstream from the TCR (Zhong et al., 2002, 2003; Sanjuan et al., 2003; Baldanzi et al., 2011; Gharbi et al., 2011; Gorentla et al., 2011), such as the RasGRP1-Ras-Erk1/2 pathway, the PKCθ-IKK-NFκB pathway, mTOR signaling (Gorentla et al., 2011), and MAP kinase-interacting serine/threonine kinase (Mnk) 1 and 2 signaling (Gorentla et al., 2013). They control T cell development (Outram et al., 2002; Guo et al., 2008; Almena et al., 2013), activation and anergy (Zhong et al., 2003; Olenchock et al., 2006a; Zha et al., 2006; Baldanzi et al., 2011), survival (Baldanzi et al., 2011; Ruffo et al., 2016), secretion (Alonso et al., 2007, 2011; Chauveau et al., 2014), and effector function (Shin et al., 2012; Yang et al., 2016b). Besides T cells, DGKζ also regulates the development, survival, and function of mast cells (Olenchock et al., 2006b), B cells (Wheeler et al., 2013), dendritic cells and macrophages (Liu et al., 2007), osteoclasts (Zamani et al., 2015), and NK cells (Yang et al., 2016a). Extensive reviews about DGKs in immune cells have been published recently (Merida et al., 2008, 2015; Zhong et al., 2008; Krishna and Zhong, 2013b). Here, we will focus on recent literature concerning DGKs in T cell tolerance, iNKT cell development and function, and CD8 T cell-mediated antimicrobial and antitumor immunity.
DGKα and DGKζ in T Cell Tolerance
Clonal deletion of highly self-reactive T cells in the thymic medulla, generation of properly functioning regulatory T cells (Treg), and T cell anergy are among the most important mechanisms of T cell tolerance that prevent autoimmune diseases (Metzger and Anderson, 2011; Xing and Hogquist, 2012). Although DGKα and ζ synergistically promote T cell maturation from the CD4+CD8+ double positive (DP) to the CD4+CD8− or CD4−CD8+ single positive (SP) stage, no direct evidence has implicated DGKα and ζ in interference with negative selection in establishing central tolerance (Guo et al., 2008).
Regulatory T cells generated in the thymus (tTregs) dominantly suppress T cells and other immune cells to prevent autoimmune diseases. However, they also negatively regulate antitumor and antipathogen immune responses. tTregs are derived from CD4 SP thymocytes in the thymic medulla after relatively strong but transient TCR-MHC/peptide engagement and signaling (Mahmud et al., 2014; Li and Rudensky, 2016). They express Foxp3, a key transcription factor that is critical for their development, maintenance, and function. TCR signaling is not only essential for tTreg generation but also required for tTreg homeostasis and function (Kim et al., 2009; Delpoux et al., 2014; Levine et al., 2014; Vahl et al., 2014). Multiple DAG-mediated signaling pathways are involved in tTreg development and function, indicated by the impaired tTreg development and function in mice deficient in either RasGRP1-Ras or PKCθ-IKK-NFκB signaling. Both NFκB and AP1 are involved in transcriptional activation of Foxp3 expression and possibly in regulating other tTreg properties (Schmidt-Supprian et al., 2004; Willoughby et al., 2007; Chen et al., 2008; Gupta et al., 2008; Barnes et al., 2009; Medoff et al., 2009). Both the percentage and number of tTregs in the CD4+ population are increased in DGKζ-deficient (but not DGKα-deficient) thymocytes and splenocytes, compared to wild-type (WT) controls (Table 1). Additionally, Foxp3−CD25+ cells within the CD4 SP thymocytes are increased in a DGKζ-deficient thymus, suggesting that DGKζ negatively controls early tTreg development. The inhibitory effect of DGKζ on tTreg development is found to be dependent on its negative control of the NFκB/c-Rel and RasGRP1-Ras-Erk pathways (Joshi et al., 2013; Schmidt et al., 2013). Of note are reports that DGKα and ζ manifest differential effects on TNFα-induced NFκB activation in tumor cells and fibroblasts, with DGKα positively regulating PKCζ-mediated p65/RelA at serine 311 residue (Yanagisawa et al., 2007; Kai et al., 2009), while DGKζ inhibits TNFα-induced NFκB activation via decreasing NFkB phosphorylation at Ser468/536, its nuclear localization, and its association with CBP (Tsuchiya et al., 2015). It would be interesting to investigate whether such mechanisms also operate in T cells or downstream of TCR to contribute to DGKα and ζ function in tTreg differentiation. It also remains unclear if DGKα and ζ act redundantly or synergistically to control Treg differentiation and function.
T cell anergy is a form of peripheral tolerance whereby T cells that recognize self-antigens in the absence of co-stimulatory signals are rendered functionally inactive (Schwartz, 2003; Powell, 2006; Fathman and Lineberry, 2007; Chappert and Schwartz, 2010; Kalekar et al., 2016). In anergic T cells, DAG-mediated signaling, including Ras/Erk1/2, NFκB, and mTOR activation, is diminished, while Ca++-mediated signaling and NFAT are selectively elevated or unhindered (Powell, 2006; Chappert and Schwartz, 2010; Xie et al., 2012; Figure 1). Both DGKα and ζ are expressed at higher levels in anergic T cells than in activated T cells (Macian et al., 2002; Olenchock et al., 2006a; Zha et al., 2006). Deficiency of either DGKα or ζ or inhibition of DGK activity contributes T cell resistance to anergic induction (Olenchock et al., 2006a; Zha et al., 2006), while overexpression of DGKα promotes T cell anergy (Zha et al., 2006). Because DAG and IP3 are produced at an equimolar ratio by PLCγ1 from PIP2, the elevated DGKα and ζ expression in anergic T cells may shift the equilibrium of IP3 and DAG toward the predominance of IP3-Ca++-NFAT signaling over DAG signaling and subsequent AP1 induction. NFAT forms a NFAT/AP1 dimer to promote T cell activation, but it also functions as a monomer to induce transcription of anergy-promoting molecules, such as Cbl-b and TRAIL (Macian et al., 2002; Wu et al., 2006). It is postulated that elevated DGK activity may lead to NFAT monomer predominance over NFAT/AP1 dimer for anergy induction (Zhong et al., 2008; Krishna and Zhong, 2013a), although experimental evidence has not yet been presented.
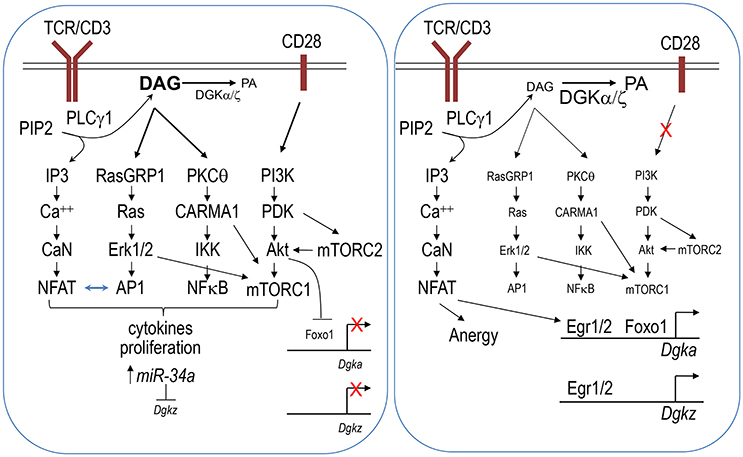
Figure 1. DGKα and DGKζ in T cell activation and anergy. Engagement of the TCR in the presence of co-stimulation results in strong activation of the PI3K-PDK1-Akt pathway (left panel). This pathway leads to mTORC2 signaling. Together with activation of the RasGRP1/Ras-Erk1/2 and PKCθ-CARMA1 pathways, they leads to mTORC1 activation. mTORC2 also promotes Akt activation via phosphorylation. Activated Akt phosphorylates Foxo1, leading to it sequestration in the cytosol and failure to activate DGKα transcription. In activated T cells, miR-34a is upregulated, which in turn downregulates DGKζ expression. Decreased DGKα and ζ expression leads to strong DAG-mediated signaling including increases of AP-1 and NFκB activity. AP-1 associates with NFAT to promote T cell activation. At the same time, AP-1 reduces monomeric NFAT to prevent it from inducing anergy promoting molecules. Strong DAG signaling together with IP3-CaN (calcineurin)-NFAT signaling allows full activation of T cells. In contrast, engagement of TCR in the absence of co-stimulation decreases PI3K-Akt-mTOR signaling, leading to increased nuclear Foxo1 and DGKα transcription (right panel). miR-34a mediated repression of DGKζ might also be lost under anergy inducing conditions. Increased DGKα and ζ expression may lead to a skewed balance between IP3 and DAG toward strong or selective Ca++-NFAT signaling and induction of Egr1/2, which further induce transcription of DGKα and DGKζ as well as other anergy promoting molecules. Selective IP3-Ca++-NFAT signaling in the presence of weak DAG-mediated signaling induces T cells to enter an anergic state.
An important issue is how DGKα and ζ expression is regulated. The transcription factor early growth response gene 2 (Egr2) is upregulated in anergic T cells and plays an important role in T cell anergy (Zheng et al., 2012). It binds directly to both Dgka and Dgkz promoters to increase the expression of these genes as well as several other anergy-promoting genes (Zheng et al., 2012, 2013). Another transcription factor, Foxo1, also directly promotes Dgka transcription (Martinez-Moreno et al., 2012). Foxo1 function, which is regulated by its subcellular localization between the cytosol and nuclei, is sequestered in the cytosolic compartment following Akt-mediated phosphorylation, which prevents it from association with target genes. In naïve or unstimulated T cells, nuclear Foxo1 activates Dgka expression. TCR engagement in the presence of CD28 costimulation induces strong PI3K/Akt activation, which may reduce nuclear Foxo1 and subsequent DGKα expression to ensure full T cell activation and avoidance of anergy (Martinez-Moreno et al., 2012). DGKζ expression has also been found to be regulated by microRNA. Two conserved sequences that match to the miR-34a seed sequence are located in the coding region and 3′ untranslated region (3′ UTR) of Dgkz. miR-34a expression is greatly upregulated in activated T cells. miR-34a directly represses DGKζ expression through targeting both Dgkz 3′ UTR and the coding region to promote T cell activation (Shin et al., 2013a).
DGKs in iNKT Cell Development and Function
Invariant NKT (iNKT) cells express the invariant Vα14Jα18 TCR, which recognizes lipid antigens presented by MHC class I-like CD1d molecules (Kawano et al., 1997; Mendiratta et al., 1997; Gapin et al., 2001). They are derived from a unique innate-like lymphoid cell lineage and can rapidly respond to agonist stimulation in both innate and adaptive immune responses via production of cytokines, such as IL-4, IL-17, IL-10, IL-13, IFNγ, and TNFα (Bendelac et al., 2007; Coquet et al., 2008; Godfrey et al., 2010; Milpied et al., 2011; Brennan et al., 2013; Salio et al., 2014). iNKT cells participate in host defense against microbial infection, antitumor immunity, and many diseases, such as allergies, asthma, graft-vs.-host disease, and obesity (Osman et al., 2000; Terashima et al., 2008; Van Kaer et al., 2013; Berzins and Ritchie, 2014).
Based on surface CD24, CD44, and NK1.1 expression, iNKT cells are traditionally defined by four developmental stages in the thymus: stage 0 (CD24+CD44−NK1.1−), stage 1 (CD24−CD44−NK1.1−), stage 2 (CD24−CD44+NK1.1−), and stage 3 (CD24−CD44+NK1.1+) (Bendelac et al., 2007; Godfrey et al., 2010; Figure 2). Recently, iNKT cells have also been defined into multiple terminally differentiated effector lineages, such as IFN-γ-producing iNKT1, IL-4-producing iNKT2, and IL-17-producing iNKT17 lineage (Matsuda et al., 2006; Michel et al., 2007, 2008). In addition, IL-10-producing iNKT10, T follicular helper (Tfh)-like iNKT cells (iNKTFH), and regulatory T cell (Treg)-like iNKT cells have also recently been described (Chang et al., 2012; Tonti et al., 2012; Sag et al., 2014; Lynch et al., 2015; Rampuria and Lang, 2015). iNKT1 and iNKT17 cells mostly reside in the CD44+NK1.1+ and the CD44+NK1.1−ICOS+ populations, respectively (Watarai et al., 2012; Constantinides and Bendelac, 2013; Lee et al., 2013; Wu et al., 2014b).
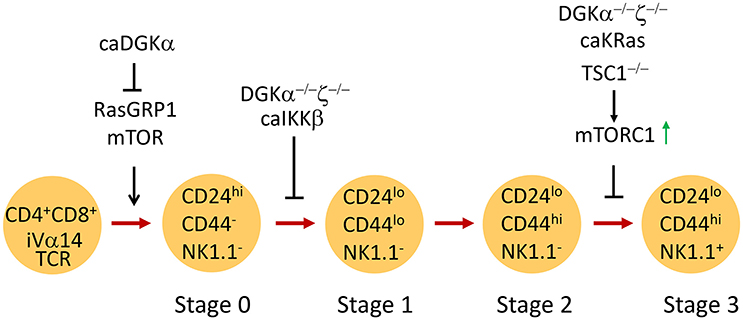
Figure 2. Regulation of iNKT cell development by DGKα and DGKζ. CD4+CD8+ DP thymocytes expressing the iVα14TCR undergo positive selection to become iNKT cells. RasGRP1/mTOR signaling is critical for generation of stage 0 iNKT cells. Constitutive DGKα inhibits iNKT generation possibly by inhibiting RasGRP1/Erk1/2 activation. DGKα and ζ double deficiency or overactivation of IKKβ causes similar blockade of early iNKT cell development. Overactivation of mTORC1 due to TSC1 deficiency leads to blockade of iNKT terminal maturation. DGKα and ζ double deficiency or expression of a constitutively active KRas also results in impaired iNKT terminal maturation, correlated with elevated mTORC1 activation.
Both the RasGRP1-Ras-Erk1/2 and PKCθ-IKK-NFκB pathways have been shown to play important roles in iNTK cell development (Yang et al., 2015). Although it was initially thought that Ras and Erk1/2 activation were dispensable for iNKT cell ontogeny, two recent studies have provided evidence that the RasGRP1-Ras-Mek1/2-Erk1/2 pathway is critical for early iNKT cell development (Hu et al., 2011; Shen et al., 2011a). In RasGRP1-deficient mice, stage 0 iNKT cells as well as total iNKT cell count are significantly decreased, suggesting defective positive selection (Shen et al., 2011a). In concordance with these observations, mice expressing dominant negative Ras in developing thymocytes demonstrated iNKT cell developmental defects (Hu et al., 2011). The RasGRP1-Ras-Erk1/2 pathway activates mTORC1 and mTORC2 signaling as well as Mnk1/2 in developing thymocytes (Gorentla et al., 2011, 2013). Both mTORC1 and mTORC2, but not Mnk1/2, are important for early iNKT cell development (Gorentla et al., 2013; Shin et al., 2014; Wei et al., 2014; Zhang et al., 2014; Prevot et al., 2015), revealing a RasGRP1-Ras-Erk1/2-mTOR signal cascade in iNKT cells for their development. mTORC1, but not mTORC2, promotes PLZF nuclear localization, which may ensure iNKT cell maturation in stage 1 and differentiation to cytokine-producing cells (Shin et al., 2014; Prevot et al., 2015). In iNKT cells, both the DAG and the SLAM (signaling lymphocytic-activation molecule)-SAP (SLAM adaptor protein)-FynT pathway are involved in PKCθ and subsequent NFκB activation. The PKCθ-IKK-NFκB pathway is essential in the ontogeny of iNKT cells, at least in part by increasing expression of antiapoptotic proteins, such as Bcl-xL (Elewaut et al., 2003; Sivakumar et al., 2003; Schmidt-Supprian et al., 2004; Stanic et al., 2004; Chung et al., 2005; Nichols et al., 2005; Pasquier et al., 2005; Griewank et al., 2007; Fang et al., 2012), but it is independent of CARMA1 and Malt1 (Mucosa-associated lymphoid tissue lymphoma translocation protein 1) (Medoff et al., 2009). CARMA1 contributes to TCR-induced mTORC1 activation in T cells (Hamilton et al., 2014). Given the minimal requirement of CARMA1 for iNKT cell development, it would be interesting to determine if TCR-induced mTORC1 activation in iNKT cells would be independent of CARMA1.
Emerging evidence demonstrates that tight regulation of DAG-mediated signaling by DGK activity is critical for the development of iNKT cells. Elevated DGKα activity brought about by expressing a membrane-targeted caDGKα in thymocytes under the control of the proximal Lck promoter caused reduced Erk1/2 activation in thymocytes and a 50% decrease of thymic iNKT cells (Almena et al., 2013). Germline deletion of either DGKα or ζ did not significantly alter iNKT cell numbers in mice. However, simultaneous ablation of both enzymes resulted in a drastic decrease in the number of iNKT cells in the thymus and in peripheral lymphoid organs (Shen et al., 2011b), correlated with prolonged DAG accumulation, elevated Ras-Erk1/2 and PKCθ-IKK signaling, and enhanced activation of both mTORC1 and mTORC2 activities in DP thymocytes (Guo et al., 2008; Gorentla et al., 2011). In DGKα and ζ double knockout mice, there was a decrease in the number of stage 1 to stage 3 iNKT cells. Stage 0 iNKT cells were not examined. The remaining iNKT cells in these mice were mostly CD44+NK1.1− stage 2 cells, suggesting that DGKα and ζ promote both early and terminal iNKT cell maturation (Shen et al., 2011b). Interestingly, expression of constitutive active (CA) IKKβ in developing thymocytes caused a severe reduction in the number of stage 1–3 iNKT cells. Thus, DGKα and ζ double deficiency may cause dysregulation of the PKCθ-IKK-NFκB pathway, leading to early iNKT cell developmental blockage. Different from CA-IKKβ, expression of CA-KRas in thymocytes caused a selective blockage of the transition from stage 2 to 3 of iNKT cells and was associated with decreased T-bet expression (Shen et al., 2011b). Because CA-KRas and DGKα and ζ double deficiency caused elevated mTORC1 signaling (Gorentla et al., 2011) and overactivation of mTORC1 in the absence of TSC1 also resulted in a similar iNKT cell terminal maturation defect (Wu et al., 2014b), DGKα and ζ may synergistically promote iNKT cell terminal maturation at least in part by preventing overactivation of the RasGRP1-Ras-Erk1/2-mTORC1 signaling cascade.
The role of DGKs in iNKT effector functions, however, is less clear. DAG-mediated signaling pathways play important roles in T cell activation, effector lineage differentiation, and tolerance (Chen et al., 2012). They are thus expected to be important in iNKT activation and function. For example, PKCθ is essential for iNKT-mediated liver inflammation (Fang et al., 2012). In germline DGKζ-deficient mice, iNKT17, but not iNKT1 cell number, was selectively decreased. Interestingly, iNKT-17 defects caused by DGKζ deficiency can be corrected in chimeric mice reconstituted with mixed WT and DGKζ-deficient bone marrow cells, suggesting that DGKζ controls iNKT-17 differentiation via an extrinsic mechanism (Wu et al., 2013). Future investigation should define the type of cells that provide such a DGKζ-regulated extrinsic control of iNKT-17 development. Additionally, mTORC1 deficient iNKT cells are defective in activation and are not able to inflict liver damage (Shin et al., 2014). Overactivation of mTORC1 due to TSC1 deficiency shapes iNKT cell effector lineage fates and contributes to their resistance to anergy and enhanced antitumor immunity (Wu et al., 2014a,b). Given the ability of DGKs in regulating mTOR and PKCθ signaling, future studies should determine if DGKs intrinsically regulate iNKT cell functions and effector lineage differentiation under steady state and in various pathologic conditions.
DGKα and ζ in CD8 T Cell-Mediated Antipathogen Immune Responses
CD8 T cells play important roles in immune responses against pathogens, particularly intracellular pathogens. Upon microbial infection, naïve CD8 T cells are activated after engagement of their TCRs with pathogen-derived peptides presented by antigen-presenting cells. They massively expand and differentiate into cytotoxic T cells that are equipped to kill pathogen-infected target cells and secrete proinflammatory cytokines. A typical antigen-specific CD8 T cell-mediated response includes an expansion phase in which CD8 cells proliferate rapidly and differentiate into effector cells, a contraction phase in which 90–95% of effector CD8 cells die due to apoptosis, and a memory maintenance phase in which the remaining 5–10% of cells are retained as fast-responding memory cells (Williams et al., 2006; Harty and Badovinac, 2008; Zhang and Bevan, 2011). During the expansion phase, effector CD8 T cells differentiate into short-lived effector cells (SLECs, CD127lowKLRG1hi) and memory precursor effector cells (MPECs, CD127hiKLRG1low) (Kaech et al., 2003; Sarkar et al., 2008). SLECs produce high levels of cytokines but are prone to death, while MPECs have high potential to differentiate to long-lived memory cells.
Engagement of the TCR on naïve CD8 T cells provides a critical signal that initiates their activation and expansion. TCR signal strength and quality regulate both the magnitude of expansion and the effector fates of CD8 T cells (Zehn et al., 2009; Iborra et al., 2013; Marchingo et al., 2014; Fulton et al., 2015) through the Ras-Erk1/2-AP1 and PKCθ-IKK-NFκB signaling pathways (Sun et al., 2000; Priatel et al., 2002; Zhong et al., 2008; Merida et al., 2015). An initial study found that DGKζ-deficient mice mounted an enhanced antiviral immune response following lymphocytic choriomeningitis virus (LCMV) infection. These mice showed enhanced expansion of viral-specific effector CD4 and CD8 T cells that contained higher percentages of IFNγ-producing cells 7 days after LCMV infection, which resulted in a quicker clearance of the virus than in WT mice (Zhong et al., 2003). A subsequent study further revealed that DGKα and ζ differentially regulate effector and memory CD8 T cell differentiation. While a deficiency of either DGKα or ζ resulted in enhanced effector CD8 T cell expansion, it slightly decreased memory CD8 T cell formation and response to LCMV infection, which correlated with elevated mTORC1 signaling in these cells (Shin et al., 2012).
Although deficiency of either DGKα or ζ enhances antiviral immune responses, DGKα and ζ double deficiency actually caused severe impairment of CD8 T cell-mediated responses to Listeria monocytogenes (LM) infection (Yang et al., 2016b). In an ovalbumin (OVA) specific OT1 TCR transgenic model and newly generated floxed DGKζ conditional-deficient mice where DGKα and ζ activity can be selectively deleted in naïve and memory CD8 T cells, it was found that ablation of both DGKα and ζ, but not of the individual DGKα or ζ isoform, impaired primary CD8 T cell responses (Table 1). At the earliest hours after LM-OVA infection, DGKα and ζ double deficient CD8 T cells expressed decreased levels of chemokine receptors CCR4, CCR5, and CXCR3 and showed impaired migration to the draining lymph nodes (dLNs). Cells that migrated to the dLNs were compromised in their proliferative ability due to not yet defined mechanism(s). In contrast to this in vivo setting, DGKα and ζ double deficient CD8 T cells proliferated more vigorously than WT controls in vitro following antigen stimulation, suggesting that the defect in proliferation was not due to intrinsic defects. It would be interesting to determine if DGKα and ζ are involved in regulating T cell/APC engagement for initiation of T cell activation. As a consequence of impaired expansion of DGKα and ζ double deficient CD8 T cells during primary immune responses, formation of memory cells was severely decreased as well. In addition, DGKα and ζ double deficiency compromised memory CD8 T cell function in homeostasis. Ablation of DGKα and ζ in preformed memory CD8 T cells accelerated the decline of these cells due to increased death and decreased homeostatic proliferative renewal (Yang et al., 2016b).
In DGKα and ζ double deficient CD8 T cells, TCR-induced NFκB nuclear localization was surprisingly diminished, although nuclear NFκB was elevated before stimulation (Yang et al., 2016b). A similar situation was also observed in T cells expressing a constitutive active IKKβ. CD8 T cells expressing a constitutive active IKKβ are defective in expansion in vivo following LM-OVA infection and are impaired in TCR-induced nuclear NFκB translocation (Krishna et al., 2012). It is likely, then, that elevated DAG levels may lead to an increase of basal activation of the PKCθ-IKK-NFκB pathway, which may trigger a negative feedback inhibition for TCR-induced activation of this pathway. Further studies should illustrate the exact negative feedback mechanism caused by DGKα and ζ double deficiency and by overactivation of IKKβ.
One consequence of decreased NFκB activation in DGKα and ζ double deficient CD8 T cells was decreased miR-155 expression and, subsequently, increased SOCS1 expression (Yang et al., 2016b). miR-155 promotes expansion of effector CD8 T cells and generation of memory CD8 T cells by targeting SOCS1 expression to ensure signaling from the common γ (γc) chain cytokine receptors (Dudda et al., 2013; Gracias et al., 2013). Common γ chain receptor signaling is known to be critical for CD8 effector and memory responses (Becker et al., 2002; Kieper et al., 2002; Carrio et al., 2004; Bachmann et al., 2007; Cui and Kaech, 2010; Sandau et al., 2010; Feau et al., 2011; Boyman and Sprent, 2012; Van Der Windt et al., 2012; Starbeck-Miller et al., 2014; Cui et al., 2015); SOCS1 negatively controls signaling from these γc-chain cytokine receptors (Cornish et al., 2003). Overexpression of miR-155 restored signaling from these receptors in DGKα and ζ double deficient CD8 T cells and partially corrected their defective responses. The data identified a DGK-NFκB-miR-155-SOCS1 axis that bridges TCR and γc-chain cytokine signaling for robust CD8 T-cell primary and memory responses to bacterial infection (Yang et al., 2016b).
DGKα and ζ Regulate CD8 T Cell and CAR-T Cell Mediated Antitumor Immunity
A tumor microenvironment suppresses T cell mediated antitumor immunity, rendering tumor-infiltrating T cells hyporesponsive or anergic (Abe and Macian, 2013; Crespo et al., 2013). DGKζ-deficient CD8 T cells contain elevated antitumor immunity. DGKζ-deficient mice subcutaneously injected with the EL-4 thymoma had reduced tumor burdens and increased tumor-specific proliferative CD8 effector T cells compared to WT controls (Riese et al., 2011, 2013). Both increased Erk1/2 activation and decreased sensitivity to the suppressive cytokine TGF-β in DGKζ-deficient CD8 T cells may be responsible for stronger activation and antitumor immunity (Arumugam et al., 2015).
Recently, chimeric antigen receptor (CAR) T cells (CAR-T cells) have demonstrated superior activity in tumor control and, in some cases, tumor eradication (Fesnak et al., 2016). However, CAR-T cells have manifested limited efficacy for solid tumors in that they are subjected to suppression by the local tumor environment and may become hyporesponsive or anergic. Such hyporesponsive or anergic tumor-infiltrating T cells or CAR-T cells show decreased Ras/Erk activation but elevated DGKα and ζ levels (Moon et al., 2014). Both type 1 and type 2 DGK inhibitors are capable of reversing such hyporesponsiveness in tumor-infiltrating CAR-T cells ex vivo, leading to increased cytotoxicity (Moon et al., 2014). Consistent with this finding, genetic ablation of DGKα, ζ, or both DGKα and ζ enhanced CD8 T cells transduced with a mesoCAR, a CAR with high affinity to the human tumor antigen mesothelin. DGKα and ζ single or double deficient mesoCAR-T cells produced elevated IFNγ production and demonstrated stronger antitumor cytotoxicity than WT controls, which correlated with reduced sensitivity to TGFβ and increased expression of FasL and TRAIL, ligands for the death receptors FAS and TRAIL-RI/RII. Importantly, DGK-deficient mesoCAR-T cells controlled mesothelioma in vivo better than WT controls (Riese et al., 2013). The enhancement of CAR-T function by DGKα and ζ double deficiency sharply contrasts with the defective anti-LM responses of DGKα and ζ double deficient CD8 T cells, suggesting differential requirements of DAG-mediated signaling downstream of CARs and TCR and for CAR-T and conventional CD8 T cell activation.
Summary
Over the past few years, our understanding of the DGK family of enzymes in immune cells has been significantly advanced. DGKα and ζ act individually to negatively control T cell activation, effector CD8 T cell differentiation and function during antimicrobial and antitumor immune responses, and tTreg generation. DGKα and ζ also manifest functional redundancy in promoting conventional αβ T cell and iNKT cell development and in enhancing CAR-T cell function. The unexpected severe impairment of CD8 T cell-mediated immune responses to microbial infection in the absence of both DGKα and ζ underscores the importance of fine-tuning DAG levels and also suggests potential negative feedback mechanisms triggered by deregulated DAG-mediated signaling. Defining such mechanisms should shed additional light on the regulation of DAG-mediated signaling pathways. Additional efforts are also needed to illustrate the underlying mechanisms of differential effects of DGKα and ζ double deficiency on CD8 T cells during antitumor and antipathogen immune responses. While DGKα and ζ perform similar or redundant functions, a more prominent role of DGKζ than DGKα in certain aspects of T cell biology, such as effector CD8 T cell differentiation and Treg, development has been noted (Table 1); however, determinants of such differences between DGKα and ζ remain unclear. The drastic differences observed between DGKα and ζ double and single deficient CD8 T cells during immune responses beg for development of DGK isoform-specific inhibitors. Such inhibitors used individually or in combination may provide great advantages over pan-DGK inhibitors in modulating immune responses for therapeutic purposes in different disease settings to minimize undesirable side effects. Key elements, such as transcription factors, microRNAs, and posttranslational modifications that control the dynamic individual and synergistic functions of DGK isoforms in T cells are beginning to be appreciated and require further exploration for better understanding of their physiological importance and the development of novel strategies enabling selective modulation of DGK α and ζ expression and activities for treating autoimmune diseases, viral infections, and cancer.
Author Contributions
SC, ZH, and X-PZ are involved in preparation of the manuscript.
Conflict of Interest Statement
The authors declare that the research was conducted in the absence of any commercial or financial relationships that could be construed as a potential conflict of interest.
Acknowledgments
This work is supported by the National Institutes of Health (R01AI079088 and R01AI101206) for X-PZ.
References
Abe, B. T., and Macian, F. (2013). Uncovering the mechanisms that regulate tumor-induced T-cell anergy. Oncoimmunol 2:e22679. doi: 10.4161/onci.22679
Almena, M., Andrada, E., Liebana, R., and Merida, I. (2013). Diacylglycerol metabolism attenuates T-cell receptor signaling and alters thymocyte differentiation. Cell Death Dis. 4:e912. doi: 10.1038/cddis.2013.396
Alonso, R., Mazzeo, C., Mérida, I., and Izquierdo, M. (2007). A new role of diacylglycerol kinase α on the secretion of lethal exosomes bearing Fas ligand during activation-induced cell death of T lymphocytes. Biochimie 89, 213–221. doi: 10.1016/j.biochi.2006.07.018
Alonso, R., Mazzeo, C., Rodriguez, M. C., Marsh, M., Fraile-Ramos, A., Calvo, V., et al. (2011). Diacylglycerol kinase α regulates the formation and polarisation of mature multivesicular bodies involved in the secretion of Fas ligand-containing exosomes in T lymphocytes. Cell Death Differ. 18, 1161–1173. doi: 10.1038/cdd.2010.184
Andresen, B. T., Rizzo, M. A., Shome, K., and Romero, G. (2002). The role of phosphatidic acid in the regulation of the Ras/MEK/Erk signaling cascade. FEBS Lett. 531, 65–68. doi: 10.1016/S0014-5793(02)03483-X
Arumugam, V., Bluemn, T., Wesley, E., Schmidt, A. M., Kambayashi, T., Malarkannan, S., et al. (2015). TCR signaling intensity controls CD8+ T cell responsiveness to TGF-β. J. Leukoc. Biol. 98, 703–712. doi: 10.1189/jlb.2HIMA1214-578R
Avila-Flores, A., Santos, T., Rincón, E., and Mérida, I. (2005). Modulation of the mammalian target of rapamycin pathway by diacylglycerol kinase-produced phosphatidic acid. J. Biol. Chem. 280, 10091–10099. doi: 10.1074/jbc.M412296200
Bachmann, M. F., Wolint, P., Walton, S., Schwarz, K., and Oxenius, A. (2007). Differential role of IL-2R signaling for CD8+ T cell responses in acute and chronic viral infections. Eur. J. Immunol. 37, 1502–1512. doi: 10.1002/eji.200637023
Baldanzi, G., Pighini, A., Bettio, V., Rainero, E., Traini, S., Chianale, F., et al. (2011). SAP-mediated inhibition of diacylglycerol kinase α regulates TCR-induced diacylglycerol signaling. J. Immunol. 187, 5941–5951. doi: 10.4049/jimmunol.1002476
Barnes, M. J., Krebs, P., Harris, N., Eidenschenk, C., Gonzalez-Quintial, R., Arnold, C. N., et al. (2009). Commitment to the regulatory T cell lineage requires CARMA1 in the thymus but not in the periphery. PLoS Biol. 7:e51. doi: 10.1371/journal.pbio.1000051
Becker, T. C., Wherry, E. J., Boone, D., Murali-Krishna, K., Antia, R., Ma, A., et al. (2002). Interleukin 15 is required for proliferative renewal of virus-specific memory CD8 T cells. J. Exp. Med. 195, 1541–1548. doi: 10.1084/jem.20020369
Bendelac, A., Savage, P. B., and Teyton, L. (2007). The biology of NKT cells. Annu. Rev. Immunol. 25, 297–336. doi: 10.1146/annurev.immunol.25.022106.141711
Berzins, S. P., and Ritchie, D. S. (2014). Natural killer T cells: drivers or passengers in preventing human disease? Nat. Rev. Immunol. 14, 640–646. doi: 10.1038/nri3725
Boyman, O., and Sprent, J. (2012). The role of interleukin-2 during homeostasis and activation of the immune system. Nat. Rev. Immunol. 12, 180–190. doi: 10.1038/nri3156
Brennan, P. J., Brigl, M., and Brenner, M. B. (2013). Invariant natural killer T cells: an innate activation scheme linked to diverse effector functions. Nat. Rev. Immunol. 13, 101–117. doi: 10.1038/nri3369
Brose, N., and Rosenmund, C. (2002). Move over protein kinase C, you've got company: alternative cellular effectors of diacylglycerol and phorbol esters. J. Cell Sci. 115, 4399–4411. doi: 10.1242/jcs.00122
Cai, J., Abramovici, H., Gee, S. H., and Topham, M. K. (2009). Diacylglycerol kinases as sources of phosphatidic acid. Biochim. Biophys. Acta 1791, 942–948. doi: 10.1016/j.bbalip.2009.02.010
Caloca, M. J., Delgado, P., Alarcón, B., and Bustelo, X. R. (2008). Role of chimaerins, a group of Rac-specific GTPase activating proteins, in T-cell receptor signaling. Cell. Signal. 20, 758–770. doi: 10.1016/j.cellsig.2007.12.015
Caloca, M. J., Garcia-Bermejo, M. L., Blumberg, P. M., Lewin, N. E., Kremmer, E., Mischak, H., et al. (1999). β2-chimaerin is a novel target for diacylglycerol: binding properties and changes in subcellular localization mediated by ligand binding to its C1 domain. Proc. Natl. Acad. Sci. U.S.A. 96, 11854–11859. doi: 10.1073/pnas.96.21.11854
Cannons, J. L., Yu, L. J., Hill, B., Mijares, L. A., Dombroski, D., Nichols, K. E., et al. (2004). SAP regulates T(H)2 differentiation and PKC-θ-mediated activation of NF-κB1. Immunity 21, 693–706. doi: 10.1016/j.immuni.2004.09.012
Carrasco, S., and Merida, I. (2004). Diacylglycerol-dependent binding recruits PKCθ and RasGRP1 C1 domains to specific subcellular localizations in living T lymphocytes. Mol. Biol. Cell 15, 2932–2942. doi: 10.1091/mbc.E03-11-0844
Carrio, R., Bathe, O. F., and Malek, T. R. (2004). Initial antigen encounter programs CD8+ T cells competent to develop into memory cells that are activated in an antigen-free, IL-7- and IL-15-rich environment. J. Immunol. 172, 7315–7323. doi: 10.4049/jimmunol.172.12.7315
Chang, P. P., Barral, P., Fitch, J., Pratama, A., Ma, C. S., Kallies, A., et al. (2012). Identification of Bcl-6-dependent follicular helper NKT cells that provide cognate help for B cell responses. Nat. Immunol. 13, 35–43. doi: 10.1038/ni.2166
Chappert, P., and Schwartz, R. H. (2010). Induction of T cell anergy: integration of environmental cues and infectious tolerance. Curr. Opin. Immunol. 22, 552–559. doi: 10.1016/j.coi.2010.08.005
Chauveau, A., Le Floc'h, A., Bantilan, N. S., Koretzky, G. A., and Huse, M. (2014). Diacylglycerol kinase α establishes T cell polarity by shaping diacylglycerol accumulation at the immunological synapse. Sci. Signal. 7:ra82. doi: 10.1126/scisignal.2005287
Chen, J., and Fang, Y. (2002). A novel pathway regulating the mammalian target of rapamycin (mTOR) signaling. Biochem. Pharmacol. 64, 1071–1077. doi: 10.1016/S0006-2952(02)01263-7
Chen, X., Priatel, J. J., Chow, M. T., and Teh, H. S. (2008). Preferential development of CD4 and CD8 T regulatory cells in RasGRP1-deficient mice. J. Immunol. 180, 5973–5982. doi: 10.4049/jimmunol.180.9.5973
Chen, Y., Ci, X., Gorentla, B., Sullivan, S. A., Stone, J. C., Zhang, W., et al. (2012). Differential requirement of RasGRP1 for γδ T cell development and activation. J. Immunol. 189, 61–71. doi: 10.4049/jimmunol.1103272
Chung, B., Aoukaty, A., Dutz, J., Terhorst, C., and Tan, R. (2005). Signaling lymphocytic activation molecule-associated protein controls NKT cell functions. J. Immunol. 174, 3153–3157. doi: 10.4049/jimmunol.174.6.3153
Cichocki, F., Schlums, H., Li, H., Stache, V., Holmes, T., Lenvik, T. R., et al. (2014). Transcriptional regulation of Munc13-4 expression in cytotoxic lymphocytes is disrupted by an intronic mutation associated with a primary immunodeficiency. J. Exp. Med. 211, 1079–1091. doi: 10.1084/jem.20131131
Cockcroft, S. (2009). Phosphatidic acid regulation of phosphatidylinositol 4-phosphate 5-kinases. Biochim. Biophys. Acta 1791, 905–912. doi: 10.1016/j.bbalip.2009.03.007
Constantinides, M. G., and Bendelac, A. (2013). Transcriptional regulation of the NKT cell lineage. Curr. Opin. Immunol. 25, 161–167. doi: 10.1016/j.coi.2013.01.003
Coquet, J. M., Chakravarti, S., Kyparissoudis, K., Mcnab, F. W., Pitt, L. A., Mckenzie, B. S., et al. (2008). Diverse cytokine production by NKT cell subsets and identification of an IL-17-producing CD4-NK1.1- NKT cell population. Proc. Natl. Acad. Sci. U.S.A. 105, 11287–11292. doi: 10.1073/pnas.0801631105
Cornish, A. L., Chong, M. M., Davey, G. M., Darwiche, R., Nicola, N. A., Hilton, D. J., et al. (2003). Suppressor of cytokine signaling-1 regulates signaling in response to interleukin-2 and other γ c-dependent cytokines in peripheral T cells. J. Biol. Chem. 278, 22755–22761. doi: 10.1074/jbc.M303021200
Crespo, J., Sun, H., Welling, T. H., Tian, Z., and Zou, W. (2013). T cell anergy, exhaustion, senescence, and stemness in the tumor microenvironment. Curr. Opin. Immunol. 25, 214–221. doi: 10.1016/j.coi.2012.12.003
Csaki, L. S., and Reue, K. (2010). Lipins: multifunctional lipid metabolism proteins. Annu. Rev. Nutr. 30, 257–272. doi: 10.1146/annurev.nutr.012809.104729
Cui, G., Staron, M. M., Gray, S. M., Ho, P. C., Amezquita, R. A., Wu, J., et al. (2015). IL-7-Induced Glycerol Transport and TAG Synthesis Promotes Memory CD8+ T Cell Longevity. Cell 161, 750–761. doi: 10.1016/j.cell.2015.03.021
Cui, W., and Kaech, S. M. (2010). Generation of effector CD8+ T cells and their conversion to memory T cells. Immunol. Rev. 236, 151–166. doi: 10.1111/j.1600-065X.2010.00926.x
Delpoux, A., Yakonowsky, P., Durand, A., Charvet, C., Valente, M., Pommier, A., et al. (2014). TCR signaling events are required for maintaining CD4 regulatory T cell numbers and suppressive capacities in the periphery. J. Immunol. 193, 5914–5923. doi: 10.4049/jimmunol.1400477
Díaz-Flores, E., Siliceo, M., Martínez, A. C., and Mérida, I. (2003). Membrane translocation of protein kinase Cθ during T lymphocyte activation requires phospholipase C-γ-generated diacylglycerol. J. Biol. Chem. 278, 29208–29215. doi: 10.1074/jbc.M303165200
Dower, N. A., Stang, S. L., Bottorff, D. A., Ebinu, J. O., Dickie, P., Ostergaard, H. L., et al. (2000). RasGRP is essential for mouse thymocyte differentiation and TCR signaling. Nat. Immunol. 1, 317–321. doi: 10.1038/80799
Dudda, J. C., Salaun, B., Ji, Y., Palmer, D. C., Monnot, G. C., Merck, E., et al. (2013). MicroRNA-155 is required for effector CD8+ T cell responses to virus infection and cancer. Immunity 38, 742–753. doi: 10.1016/j.immuni.2012.12.006
Ebinu, J. O., Bottorff, D. A., Chan, E. Y., Stang, S. L., Dunn, R. J., and Stone, J. C. (1998). RasGRP, a Ras guanyl nucleotide- releasing protein with calcium- and diacylglycerol-binding motifs. Science 280, 1082–1086. doi: 10.1126/science.280.5366.1082
Elewaut, D., Shaikh, R. B., Hammond, K. J., De Winter, H., Leishman, A. J., Sidobre, S., et al. (2003). NIK-dependent RelB activation defines a unique signaling pathway for the development of Vα 14i NKT cells. J. Exp. Med. 197, 1623–1633. doi: 10.1084/jem.20030141
Fang, X., Wang, R., Ma, J., Ding, Y., Shang, W., and Sun, Z. (2012). Ameliorated ConA-induced hepatitis in the absence of PKC-theta. PLoS ONE 7:e31174. doi: 10.1371/journal.pone.0031174
Fathman, C. G., and Lineberry, N. B. (2007). Molecular mechanisms of CD4+ T-cell anergy. Nat. Rev. Immunol. 7, 599–609. doi: 10.1038/nri2131
Feau, S., Arens, R., Togher, S., and Schoenberger, S. P. (2011). Autocrine IL-2 is required for secondary population expansion of CD8+ memory T cells. Nat. Immunol. 12, 908–913. doi: 10.1038/ni.2079
Feldmann, J., Callebaut, I., Raposo, G., Certain, S., Bacq, D., Dumont, C., et al. (2003). Munc13-4 is essential for cytolytic granules fusion and is mutated in a form of familial hemophagocytic lymphohistiocytosis (FHL3). Cell 115, 461–473. doi: 10.1016/S0092-8674(03)00855-9
Fesnak, A. D., June, C. H., and Levine, B. L. (2016). Engineered T cells: the promise and challenges of cancer immunotherapy. Nat. Rev. Cancer 16, 566–581. doi: 10.1038/nrc.2016.97
Frank, C., Keilhack, H., Opitz, F., Zschörnig, O., and Böhmer, F. D. (1999). Binding of phosphatidic acid to the protein-tyrosine phosphatase SHP-1 as a basis for activity modulation. Biochemistry 38, 11993–12002. doi: 10.1021/bi982586w
Fuller, D. M., Zhu, M., Song, X., Ou-Yang, C. W., Sullivan, S. A., Stone, J. C., et al. (2012). Regulation of RasGRP1 function in T cell development and activation by its unique tail domain. PLoS ONE 7:e38796. doi: 10.1371/journal.pone.0038796
Fulton, R. B., Hamilton, S. E., Xing, Y., Best, J. A., Goldrath, A. W., Hogquist, K. A., et al. (2015). The TCR's sensitivity to self peptide-MHC dictates the ability of naive CD8+ T cells to respond to foreign antigens. Nat. Immunol. 16, 107–117. doi: 10.1038/ni.3043
Galandrini, R., Micucci, F., Tassi, I., Cifone, M. G., Cinque, B., Piccoli, M., et al. (2005). Arf6: a new player in FcgammaRIIIA lymphocyte-mediated cytotoxicity. Blood 106, 577–583. doi: 10.1182/blood-2004-10-4100
Gapin, L., Matsuda, J. L., Surh, C. D., and Kronenberg, M. (2001). NKT cells derive from double-positive thymocytes that are positively selected by CD1d. Nat. Immunol. 2, 971–978. doi: 10.1038/ni710
Gharbi, S. I., Rincon, E., Avila-Flores, A., Torres-Ayuso, P., Almena, M., Cobos, M. A., et al. (2011). Diacylglycerol kinase ζ controls diacylglycerol metabolism at the immunological synapse. Mol. Biol. Cell 22, 4406–4414. doi: 10.1091/mbc.E11-03-0247
Ghosh, S., Strum, J. C., Sciorra, V. A., Daniel, L., and Bell, R. M. (1996). Raf-1 kinase possesses distinct binding domains for phosphatidylserine and phosphatidic acid. Phosphatidic acid regulates the translocation of Raf-1 in 12-O-tetradecanoylphorbol-13-acetate-stimulated Madin-Darby canine kidney cells. J. Biol. Chem. 271, 8472–8480. doi: 10.1074/jbc.271.14.8472
Godfrey, D. I., Stankovic, S., and Baxter, A. G. (2010). Raising the NKT cell family. Nat. Immunol. 11, 197–206. doi: 10.1038/ni.1841
Golec, D. P., Henao Caviedes, L. M., and Baldwin, T. A. (2016). RasGRP1 and RasGRP3 Are required for efficient generation of early thymic progenitors. J. Immunol. 197, 1743–1753. doi: 10.4049/jimmunol.1502107
Gorentla, B. K., Krishna, S., Shin, J., Inoue, M., Shinohara, M. L., Grayson, J. M., et al. (2013). Mnk1 and 2 are dispensable for T cell development and activation but important for the pathogenesis of experimental autoimmune encephalomyelitis. J. Immunol. 190, 1026–1037. doi: 10.4049/jimmunol.1200026
Gorentla, B. K., Wan, C. K., and Zhong, X. P. (2011). Negative regulation of mTOR activation by diacylglycerol kinases. Blood 117, 4022–4031. doi: 10.1182/blood-2010-08-300731
Gracias, D. T., Stelekati, E., Hope, J. L., Boesteanu, A. C., Doering, T. A., Norton, J., et al. (2013). The microRNA miR-155 controls CD8+ T cell responses by regulating interferon signaling. Nat. Immunol. 14, 593–602. doi: 10.1038/ni.2576
Griewank, K., Borowski, C., Rietdijk, S., Wang, N., Julien, A., Wei, D. G., et al. (2007). Homotypic interactions mediated by Slamf1 and Slamf6 receptors control NKT cell lineage development. Immunity 27, 751–762. doi: 10.1016/j.immuni.2007.08.020
Guo, R., Wan, C. K., Carpenter, J. H., Mousallem, T., Boustany, R. M., Kuan, C. T., et al. (2008). Synergistic control of T cell development and tumor suppression by diacylglycerol kinase α and ζ. Proc. Natl. Acad. Sci. U.S.A. 105, 11909–11914. doi: 10.1073/pnas.0711856105
Gupta, S., Manicassamy, S., Vasu, C., Kumar, A., Shang, W., and Sun, Z. (2008). Differential requirement of PKC-θ in the development and function of natural regulatory T cells. Mol. Immunol. 46, 213–224. doi: 10.1016/j.molimm.2008.08.275
Hamilton, K. S., Phong, B., Corey, C., Cheng, J., Gorentla, B., Zhong, X., et al. (2014). T cell receptor-dependent activation of mTOR signaling in T cells is mediated by Carma1 and MALT1, but not Bcl10. Sci. Signal. 7:ra55. doi: 10.1126/scisignal.2005169
Harty, J. T., and Badovinac, V. P. (2008). Shaping and reshaping CD8+ T-cell memory. Nat. Rev. Immunol. 8, 107–119. doi: 10.1038/nri2251
Hayashi, K., and Altman, A. (2007). Protein kinase C theta (PKCθ): a key player in T cell life and death. Pharmacol. Res. 55, 537–544. doi: 10.1016/j.phrs.2007.04.009
Hu, T., Gimferrer, I., Simmons, A., Wiest, D., and Alberola-Ila, J. (2011). The Ras/MAPK pathway is required for generation of iNKT cells. PLoS ONE 6:e19890. doi: 10.1371/journal.pone.0019890
Iborra, S., Ramos, M., Arana, D. M., Lazaro, S., Aguilar, F., Santos, E., et al. (2013). N-ras couples antigen receptor signaling to Eomesodermin and to functional CD8+ T cell memory but not to effector differentiation. J. Exp. Med. 210, 1463–1479. doi: 10.1084/jem.20112495
Isakov, N., and Altman, A. (2002). protein kinase cθ in T cell activation. Annu. Rev. Immunol. 20, 761–794. doi: 10.1146/annurev.immunol.20.100301.064807
Jarquin-Pardo, M., Fitzpatrick, A., Galiano, F. J., First, E. A., and Davis, J. N. (2007). Phosphatidic acid regulates the affinity of the murine phosphatidylinositol 4-phosphate 5-kinase-Iβ for phosphatidylinositol-4-phosphate. J. Cell. Biochem. 100, 112–128. doi: 10.1002/jcb.21027
Jenkins, G. M., and Frohman, M. A. (2005). Phospholipase D: a lipid centric review. Cell. Mol. Life Sci. 62, 2305–2316. doi: 10.1007/s00018-005-5195-z
Johnson, J. L., Hong, H., Monfregola, J., Kiosses, W. B., and Catz, S. D. (2011). Munc13-4 restricts motility of Rab27a-expressing vesicles to facilitate lipopolysaccharide-induced priming of exocytosis in neutrophils. J. Biol. Chem. 286, 5647–5656. doi: 10.1074/jbc.M110.184762
Jones, D. R., Sanjuan, M. A., Stone, J. C., and Merida, I. (2002). Expression of a catalytically inactive form of diacylglycerol kinase α induces sustained signaling through RasGRP. FASEB J. 16, 595–597. doi: 10.1096/fj.01-0762fje
Joshi, R. P., Schmidt, A. M., Das, J., Pytel, D., Riese, M. J., Lester, M., et al. (2013). The ζ isoform of diacylglycerol kinase plays a predominant role in regulatory T cell development and TCR-mediated ras signaling. Sci. Signal. 6:ra102. doi: 10.1126/scisignal.2004373
Kaech, S. M., Tan, J. T., Wherry, E. J., Konieczny, B. T., Surh, C. D., and Ahmed, R. (2003). Selective expression of the interleukin 7 receptor identifies effector CD8 T cells that give rise to long-lived memory cells. Nat. Immunol. 4, 1191–1198. doi: 10.1038/ni1009
Kai, M., Yasuda, S., Imai, S., Toyota, M., Kanoh, H., and Sakane, F. (2009). Diacylglycerol kinase α enhances protein kinase Cζ-dependent phosphorylation at Ser311 of p65/RelA subunit of nuclear factor-κB. FEBS Lett. 583, 3265–3268. doi: 10.1016/j.febslet.2009.09.017
Kalekar, L. A., Schmiel, S. E., Nandiwada, S. L., Lam, W. Y., Barsness, L. O., Zhang, N., et al. (2016). CD4+ T cell anergy prevents autoimmunity and generates regulatory T cell precursors. Nat. Immunol. 17, 304–314. doi: 10.1038/ni.3331
Kawano, T., Cui, J., Koezuka, Y., Toura, I., Kaneko, Y., Motoki, K., et al. (1997). CD1d-restricted and TCR-mediated activation of Vα14 NKT cells by glycosylceramides. Science 278, 1626–1629. doi: 10.1126/science.278.5343.1626
Kieper, W. C., Tan, J. T., Bondi-Boyd, B., Gapin, L., Sprent, J., Ceredig, R., et al. (2002). Overexpression of interleukin (IL)-7 leads to IL-15-independent generation of memory phenotype CD8+ T cells. J. Exp. Med. 195, 1533–1539. doi: 10.1084/jem.20020067
Kim, J. K., Klinger, M., Benjamin, J., Xiao, Y., Erle, D. J., Littman, D. R., et al. (2009). Impact of the TCR signal on regulatory T cell homeostasis, function, and trafficking. PLoS ONE 4:e6580. doi: 10.1371/journal.pone.0006580
Koch, H., Hofmann, K., and Brose, N. (2000). Definition of Munc13-homology-domains and characterization of a novel ubiquitously expressed Munc13 isoform. Biochem. J. 349, 247–253. doi: 10.1042/bj3490247
Kraft, C. A., Garrido, J. L., Fluharty, E., Leiva-Vega, L., and Romero, G. (2008). Role of phosphatidic acid in the coupling of the ERK cascade. J. Biol. Chem. 283, 36636–36645. doi: 10.1074/jbc.M804633200
Krishna, S., Xie, D., Gorentla, B., Shin, J., Gao, J., and Zhong, X. P. (2012). Chronic activation of the kinase IKKβ impairs T cell function and survival. J. Immunol. 189, 1209–1219. doi: 10.4049/jimmunol.1102429
Krishna, S., and Zhong, X. (2013a). Role of diacylglycerol kinases in T cell development and function. Crit. Rev. Immunol. 33, 97–118.
Krishna, S., and Zhong, X. P. (2013b). Regulation of lipid signaling by diacylglycerol kinases during T cell development and function. Front. Immunol. 4:178. doi: 10.3389/fimmu.2013.00178
Kwon, M. J., Ma, J., Ding, Y., Wang, R., and Sun, Z. (2012). Protein kinase C-θ promotes Th17 differentiation via upregulation of Stat3. J. Immunol. 188, 5887–5897. doi: 10.4049/jimmunol.1102941
Lee, Y. J., Holzapfel, K. L., Zhu, J., Jameson, S. C., and Hogquist, K. A. (2013). Steady-state production of IL-4 modulates immunity in mouse strains and is determined by lineage diversity of iNKT cells. Nat. Immunol. 14, 1146–1154. doi: 10.1038/ni.2731
Levine, A. G., Arvey, A., Jin, W., and Rudensky, A. Y. (2014). Continuous requirement for the TCR in regulatory T cell function. Nat. Immunol. 15, 1070–1078. doi: 10.1038/ni.3004
Li, M. O., and Rudensky, A. Y. (2016). T cell receptor signalling in the control of regulatory T cell differentiation and function. Nat. Rev. Immunol. 16, 220–233. doi: 10.1038/nri.2016.26
Liu, C. H., Machado, F. S., Guo, R., Nichols, K. E., Burks, A. W., Aliberti, J. C., et al. (2007). Diacylglycerol kinase ζ regulates microbial recognition and host resistance to Toxoplasma gondii. J. Exp. Med. 204, 781–792. doi: 10.1084/jem.20061856
Lynch, L., Michelet, X., Zhang, S., Brennan, P. J., Moseman, A., Lester, C., et al. (2015). Regulatory iNKT cells lack expression of the transcription factor PLZF and control the homeostasis of T(reg) cells and macrophages in adipose tissue. Nat. Immunol. 16, 85–95. doi: 10.1038/ni.3047
Macian, F., Garcia-Cozar, F., Im, S. H., Horton, H. F., Byrne, M. C., and Rao, A. (2002). Transcriptional mechanisms underlying lymphocyte tolerance. Cell 109, 719–731. doi: 10.1016/S0092-8674(02)00767-5
Mahmud, S. A., Manlove, L. S., Schmitz, H. M., Xing, Y., Wang, Y., Owen, D. L., et al. (2014). Costimulation via the tumor-necrosis factor receptor superfamily couples TCR signal strength to the thymic differentiation of regulatory T cells. Nat. Immunol. 15, 473–481. doi: 10.1038/ni.2849
Manicassamy, S., Gupta, S., Huang, Z., and Sun, Z. (2006). Protein kinase C-θ-mediated signals enhance CD4+ T cell survival by up-regulating Bcl-xL. J. Immunol. 176, 6709–6716. doi: 10.4049/jimmunol.176.11.6709
Marchingo, J. M., Kan, A., Sutherland, R. M., Duffy, K. R., Wellard, C. J., Belz, G. T., et al. (2014). T cell signaling. Antigen affinity, costimulation, and cytokine inputs sum linearly to amplify T cell expansion. Science 346, 1123–1127. doi: 10.1126/science.1260044
Marklund, U., Lightfoot, K., and Cantrell, D. (2003). Intracellular location and cell context-dependent function of protein kinase D. Immunity 19, 491–501. doi: 10.1016/S1074-7613(03)00260-7
Marsland, B. J., Soos, T. J., Spath, G., Littman, D. R., and Kopf, M. (2004). Protein kinase Cθ is critical for the development of in vivo T helper (Th)2 cell but not Th1 cell responses. J. Exp. Med. 200, 181–189. doi: 10.1084/jem.20032229
Martinez-Moreno, M., Garcia-Lievana, J., Soutar, D., Torres-Ayuso, P., Andrada, E., Zhong, X. P., et al. (2012). FoxO-dependent regulation of diacylglycerol kinase α gene expression. Mol. Cell. Biol. 32, 4168–4180. doi: 10.1128/MCB.00654-12
Matsuda, Y., Toda, M., Kato, T., Kuribayashi, K., and Kakimi, K. (2006). Fulminant liver failure triggered by therapeutic antibody treatment in a mouse model. Int. J. Oncol. 29, 1119–1125. doi: 10.3892/ijo.29.5.1119
Medoff, B. D., Sandall, B. P., Landry, A., Nagahama, K., Mizoguchi, A., Luster, A. D., et al. (2009). Differential requirement for CARMA1 in agonist-selected T-cell development. Eur. J. Immunol. 39, 78–84. doi: 10.1002/eji.200838734
Menager, M. M., Menasche, G., Romao, M., Knapnougel, P., Ho, C. H., Garfa, M., et al. (2007). Secretory cytotoxic granule maturation and exocytosis require the effector protein hMunc13-4. Nat. Immunol. 8, 257–267. doi: 10.1038/ni1431
Mendiratta, S. K., Martin, W. D., Hong, S., Boesteanu, A., Joyce, S., and Van Kaer, L. (1997). CD1d1 mutant mice are deficient in natural T cells that promptly produce IL-4. Immunity 6, 469–477. doi: 10.1016/S1074-7613(00)80290-3
Merida, I., Andrada, E., Gharbi, S. I., and Avila-Flores, A. (2015). Redundant and specialized roles for diacylglycerol kinases α and ζ in the control of T cell functions. Sci. Signal. 8:re6. doi: 10.1126/scisignal.aaa0974
Merida, I., Avila-Flores, A., and Merino, E. (2008). Diacylglycerol kinases: at the hub of cell signalling. Biochem. J. 409, 1–18. doi: 10.1042/BJ20071040
Metzger, T. C., and Anderson, M. S. (2011). Control of central and peripheral tolerance by Aire. Immunol. Rev. 241, 89–103. doi: 10.1111/j.1600-065X.2011.01008.x
Michel, M. L., Keller, A. C., Paget, C., Fujio, M., Trottein, F., Savage, P. B., et al. (2007). Identification of an IL-17-producing NK1.1(neg) iNKT cell population involved in airway neutrophilia. J. Exp. Med. 204, 995–1001. doi: 10.1084/jem.20061551
Michel, M. L., Mendes-Da-Cruz, D., Keller, A. C., Lochner, M., Schneider, E., Dy, M., et al. (2008). Critical role of ROR-gammat in a new thymic pathway leading to IL-17-producing invariant NKT cell differentiation. Proc. Natl. Acad. Sci. U.S.A. 105, 19845–19850. doi: 10.1073/pnas.0806472105
Micucci, F., Capuano, C., Marchetti, E., Piccoli, M., Frati, L., Santoni, A., et al. (2008). PI5KI-dependent signals are critical regulators of the cytolytic secretory pathway. Blood 111, 4165–4172. doi: 10.1182/blood-2007-08-108886
Milpied, P., Massot, B., Renand, A., Diem, S., Herbelin, A., Leite-De-Moraes, M., et al. (2011). IL-17-producing invariant NKT cells in lymphoid organs are recent thymic emigrants identified by neuropilin-1 expression. Blood 118, 2993–3002. doi: 10.1182/blood-2011-01-329268
Monfregola, J., Johnson, J. L., Meijler, M. M., Napolitano, G., and Catz, S. D. (2012). MUNC13-4 protein regulates the oxidative response and is essential for phagosomal maturation and bacterial killing in neutrophils. J. Biol. Chem. 287, 44603–44618. doi: 10.1074/jbc.M112.414029
Moon, E. K., Wang, L. C., Dolfi, D. V., Wilson, C. B., Ranganathan, R., Sun, J., et al. (2014). Multifactorial T-cell hypofunction that is reversible can limit the efficacy of chimeric antigen receptor-transduced human T cells in solid tumors. Clin. Cancer Res. 20, 4262–4273. doi: 10.1158/1078-0432.CCR-13-2627
Navarro, M. N., Feijoo-Carnero, C., Arandilla, A. G., Trost, M., and Cantrell, D. A. (2014). Protein kinase D2 is a digital amplifier of T cell receptor-stimulated diacylglycerol signaling in naive CD8+ T cells. Sci. Signal. 7:ra99. doi: 10.1126/scisignal.2005477
Nichols, K. E., Hom, J., Gong, S. Y., Ganguly, A., Ma, C. S., Cannons, J. L., et al. (2005). Regulation of NKT cell development by SAP, the protein defective in XLP. Nat. Med. 11, 340–345. doi: 10.1038/nm1189
Olenchock, B. A., Guo, R., Carpenter, J. H., Jordan, M., Topham, M. K., Koretzky, G. A., et al. (2006a). Disruption of diacylglycerol metabolism impairs the induction of T cell anergy. Nat. Immunol. 7, 1174–1181. doi: 10.1038/ni1400
Olenchock, B. A., Guo, R., Silverman, M. A., Wu, J. N., Carpenter, J. H., Koretzky, G. A., et al. (2006b). Impaired degranulation but enhanced cytokine production after FcεRI stimulation of diacylglycerol kinase ζ-deficient mast cells. J. Exp. Med. 203, 1471–1480. doi: 10.1084/jem.20052424
Osman, Y., Kawamura, T., Naito, T., Takeda, K., Van Kaer, L., Okumura, K., et al. (2000). Activation of hepatic NKT cells and subsequent liver injury following administration of α-galactosylceramide. Eur. J. Immunol. 30, 1919–1928. doi: 10.1002/1521-4141(200007)30:7<1919::AID-IMMU1919>3.0.CO;2-3
Outram, S. V., Crompton, T., Merida, I., Varas, A., and Martinez, A. C. (2002). Diacylglycerol kinase α activity promotes survival of CD4+CD8+ double positive cells during thymocyte development. Immunology 105, 391–398. doi: 10.1046/j.1365-2567.2002.01385.x
Pasquier, B., Yin, L., Fondaneche, M. C., Relouzat, F., Bloch-Queyrat, C., Lambert, N., et al. (2005). Defective NKT cell development in mice and humans lacking the adapter SAP, the X-linked lymphoproliferative syndrome gene product. J. Exp. Med. 201, 695–701. doi: 10.1084/jem.20042432
Powell, J. D. (2006). The induction and maintenance of T cell anergy. Clin. Immunol. 120, 239–246. doi: 10.1016/j.clim.2006.02.004
Prevot, N., Pyaram, K., Bischoff, E., Sen, J. M., Powell, J. D., and Chang, C. H. (2015). Mammalian target of rapamycin complex 2 regulates invariant NKT cell development and function independent of promyelocytic leukemia zinc-finger. J. Immunol. 194, 223–230. doi: 10.4049/jimmunol.1401985
Priatel, J. J., Chen, X., Huang, Y. H., Chow, M. T., Zenewicz, L. A., Coughlin, J. J., et al. (2010). RasGRP1 regulates antigen-induced developmental programming by naive CD8 T cells. J. Immunol. 184, 666–676. doi: 10.4049/jimmunol.0803521
Priatel, J. J., Teh, S. J., Dower, N. A., Stone, J. C., and Teh, H. S. (2002). RasGRP1 transduces low-grade TCR signals which are critical for T cell development, homeostasis, and differentiation. Immunity 17, 617–627. doi: 10.1016/S1074-7613(02)00451-X
Rampuria, P., and Lang, M. L. (2015). CD1d-dependent expansion of NKT follicular helper cells in vivo and in vitro is a product of cellular proliferation and differentiation. Int. Immunol. 27, 253–263. doi: 10.1093/intimm/dxv007
Riese, M. J., Grewal, J., Das, J., Zou, T., Patil, V., Chakraborty, A. K., et al. (2011). Decreased diacylglycerol metabolism enhances ERK activation and augments CD8+ T cell functional responses. J. Biol. Chem. 286, 5254–5265. doi: 10.1074/jbc.M110.171884
Riese, M. J., Wang, L. C., Moon, E. K., Joshi, R. P., Ranganathan, A., June, C. H., et al. (2013). Enhanced effector responses in activated CD8+ T cells deficient in diacylglycerol kinases. Cancer Res. 73, 3566–3577. doi: 10.1158/0008-5472.CAN-12-3874
Rizzo, M. A., Shome, K., Vasudevan, C., Stolz, D. B., Sung, T. C., Frohman, M. A., et al. (1999). Phospholipase D and its product, phosphatidic acid, mediate agonist-dependent raf-1 translocation to the plasma membrane and the activation of the mitogen-activated protein kinase pathway. J. Biol. Chem. 274, 1131–1139. doi: 10.1074/jbc.274.2.1131
Rizzo, M. A., Shome, K., Watkins, S. C., and Romero, G. (2000). The recruitment of Raf-1 to membranes is mediated by direct interaction with phosphatidic acid and is independent of association with Ras. J. Biol. Chem. 275, 23911–23918. doi: 10.1074/jbc.M001553200
Roose, J. P., Mollenauer, M., Gupta, V. A., Stone, J., and Weiss, A. (2005). A diacylglycerol-protein kinase C-RasGRP1 pathway directs Ras activation upon antigen receptor stimulation of T cells. Mol. Cell. Biol. 25, 4426–4441. doi: 10.1128/MCB.25.11.4426-4441.2005
Rozengurt, E., Rey, O., and Waldron, R. T. (2005). Protein kinase D signaling. J. Biol. Chem. 280, 13205–13208. doi: 10.1074/jbc.R500002200
Ruffo, E., Malacarne, V., Larsen, S. E., Das, R., Patrussi, L., Wulfing, C., et al. (2016). Inhibition of diacylglycerol kinase α restores restimulation-induced cell death and reduces immunopathology in XLP-1. Sci. Transl. Med. 8:321ra327. doi: 10.1126/scitranslmed.aad1565
Sag, D., Krause, P., Hedrick, C. C., Kronenberg, M., and Wingender, G. (2014). IL-10-producing NKT10 cells are a distinct regulatory invariant NKT cell subset. J. Clin. Invest. 124, 3725–3740. doi: 10.1172/JCI72308
Sakane, F., Imai, S., Kai, M., Yasuda, S., and Kanoh, H. (2007). Diacylglycerol kinases: why so many of them? Biochim. Biophys. Acta 1771, 793–806. doi: 10.1016/j.bbalip.2007.04.006
Salio, M., Silk, J. D., Jones, E. Y., and Cerundolo, V. (2014). Biology of CD1- and MR1-restricted T cells. Annu. Rev. Immunol. 32, 323–366. doi: 10.1146/annurev-immunol-032713-120243
Sandau, M. M., Kohlmeier, J. E., Woodland, D. L., and Jameson, S. C. (2010). IL-15 regulates both quantitative and qualitative features of the memory CD8 T cell pool. J. Immunol. 184, 35–44. doi: 10.4049/jimmunol.0803355
Sanjuan, M. A., Pradet-Balade, B., Jones, D. R., Martinez, A. C., Stone, J. C., Garcia-Sanz, J. A., et al. (2003). T cell activation in vivo targets diacylglycerol kinase α to the membrane: a novel mechanism for Ras attenuation. J. Immunol. 170, 2877–2883. doi: 10.4049/jimmunol.170.6.2877
Sarkar, S., Kalia, V., Haining, W. N., Konieczny, B. T., Subramaniam, S., and Ahmed, R. (2008). Functional and genomic profiling of effector CD8 T cell subsets with distinct memory fates. J. Exp. Med. 205, 625–640. doi: 10.1084/jem.20071641
Schmidt, A. M., Lu, W., Sindhava, V. J., Huang, Y., Burkhardt, J. K., Yang, E., et al. (2015). Regulatory T cells require TCR signaling for their suppressive function. J. Immunol. 194, 4362–4370. doi: 10.4049/jimmunol.1402384
Schmidt, A. M., Zou, T., Joshi, R. P., Leichner, T. M., Pimentel, M. A., Sommers, C. L., et al. (2013). Diacylglycerol kinase ζ limits the generation of natural regulatory T cells. Sci. Signal. 6:ra101. doi: 10.1126/scisignal.2004411
Schmidt-Supprian, M., Tian, J., Grant, E. P., Pasparakis, M., Maehr, R., Ovaa, H., et al. (2004). Differential dependence of CD4+CD25+ regulatory and natural killer-like T cells on signals leading to NF-κB activation. Proc. Natl. Acad. Sci. U.S.A. 101, 4566–4571. doi: 10.1073/pnas.0400885101
Schwartz, R. H. (2003). T cell anergy. Annu. Rev. Immunol. 21, 305–334. doi: 10.1146/annurev.immunol.21.120601.141110
Shen, S., Chen, Y., Gorentla, B. K., Lu, J., Stone, J. C., and Zhong, X. P. (2011a). Critical roles of RasGRP1 for invariant NKT cell development. J. Immunol. 187, 4467–4473. doi: 10.4049/jimmunol.1003798
Shen, S., Wu, J., Srivatsan, S., Gorentla, B. K., Shin, J., Xu, L., et al. (2011b). Tight regulation of diacylglycerol-mediated signaling is critical for proper invariant NKT cell development. J. Immunol. 187, 2122–2129. doi: 10.4049/jimmunol.1100495
Shin, J., O'brien, T. F., Grayson, J. M., and Zhong, X. P. (2012). Differential regulation of primary and memory CD8 T cell immune responses by diacylglycerol kinases. J. Immunol. 188, 2111–2117. doi: 10.4049/jimmunol.1102265
Shin, J., Wang, S., Deng, W., Wu, J., Gao, J., and Zhong, X. P. (2014). Mechanistic target of rapamycin complex 1 is critical for invariant natural killer T-cell development and effector function. Proc. Natl. Acad. Sci. U.S.A. 111, E776–E783. doi: 10.1073/pnas.1315435111
Shin, J., Xie, D., and Zhong, X. P. (2013a). MicroRNA-34a enhances T cell activation by targeting diacylglycerol kinase ζ. PLoS ONE 8:e77983. doi: 10.1371/journal.pone.0077983
Shin, J., Zhang, P., Wang, S., Wu, J., Guan, Z., and Zhong, X. P. (2013b). Negative control of mast cell degranulation and the anaphylactic response by the phosphatase lipin1. Eur. J. Immunol. 43, 240–248. doi: 10.1002/eji.201242571
Shirakawa, R., Higashi, T., Tabuchi, A., Yoshioka, A., Nishioka, H., Fukuda, M., et al. (2004). Munc13-4 is a GTP-Rab27-binding protein regulating dense core granule secretion in platelets. J. Biol. Chem. 279, 10730–10737. doi: 10.1074/jbc.M309426200
Shome, K., Vasudevan, C., and Romero, G. (1997). ARF proteins mediate insulin-dependent activation of phospholipase D. Curr. Biol. 7, 387–396. doi: 10.1016/S0960-9822(06)00186-2
Siliceo, M., Garcia-Bernal, D., Carrasco, S., Diaz-Flores, E., Coluccio Leskow, F., Teixido, J., et al. (2006). β2-chimaerin provides a diacylglycerol-dependent mechanism for regulation of adhesion and chemotaxis of T cells. J. Cell Sci. 119, 141–152. doi: 10.1242/jcs.02722
Siliceo, M., and Merida, I. (2009). T cell receptor-dependent tyrosine phosphorylation of β2-chimaerin modulates its Rac-GAP function in T cells. J. Biol. Chem. 284, 11354–11363. doi: 10.1074/jbc.M806098200
Sivakumar, V., Hammond, K. J., Howells, N., Pfeffer, K., and Weih, F. (2003). Differential requirement for Rel/nuclear factor κB family members in natural killer T cell development. J. Exp. Med. 197, 1613–1621. doi: 10.1084/jem.20022234
Spitaler, M., Emslie, E., Wood, C. D., and Cantrell, D. (2006). Diacylglycerol and protein kinase D localization during T lymphocyte activation. Immunity 24, 535–546. doi: 10.1016/j.immuni.2006.02.013
Stanic, A. K., Bezbradica, J. S., Park, J. J., Van Kaer, L., Boothby, M. R., and Joyce, S. (2004). Cutting edge: the ontogeny and function of Va14Ja18 natural T lymphocytes require signal processing by protein kinase Cθ and NF-κB. J. Immunol. 172, 4667–4671. doi: 10.4049/jimmunol.172.8.4667
Starbeck-Miller, G. R., Xue, H. H., and Harty, J. T. (2014). IL-12 and type I interferon prolong the division of activated CD8 T cells by maintaining high-affinity IL-2 signaling in vivo. J. Exp. Med. 211, 105–120. doi: 10.1084/jem.20130901
Sun, Z., Arendt, C. W., Ellmeier, W., Schaeffer, E. M., Sunshine, M. J., Gandhi, L., et al. (2000). PKC-θ is required for TCR-induced NF-κB activation in mature but not immature T lymphocytes. Nature 404, 402–407. doi: 10.1038/35006090
Terashima, A., Watarai, H., Inoue, S., Sekine, E., Nakagawa, R., Hase, K., et al. (2008). A novel subset of mouse NKT cells bearing the IL-17 receptor B responds to IL-25 and contributes to airway hyperreactivity. J. Exp. Med. 205, 2727–2733. doi: 10.1084/jem.20080698
Tonti, E., Fedeli, M., Napolitano, A., Iannacone, M., Von Andrian, U. H., Guidotti, L. G., et al. (2012). Follicular helper NKT cells induce limited B cell responses and germinal center formation in the absence of CD4+ T cell help. J. Immunol. 188, 3217–3222. doi: 10.4049/jimmunol.1103501
Tsuchiya, R., Tanaka, T., Hozumi, Y., Nakano, T., Okada, M., Topham, M. K., et al. (2015). Downregulation of diacylglycerol kinase ζ enhances activation of cytokine-induced NF-κB signaling pathway. Biochim. Biophys. Acta 1853, 361–369. doi: 10.1016/j.bbamcr.2014.11.011
Vahl, J. C., Drees, C., Heger, K., Heink, S., Fischer, J. C., Nedjic, J., et al. (2014). Continuous T cell receptor signals maintain a functional regulatory T cell pool. Immunity 41, 722–736. doi: 10.1016/j.immuni.2014.10.012
Van Der Windt, G. J., Everts, B., Chang, C. H., Curtis, J. D., Freitas, T. C., Amiel, E., et al. (2012). Mitochondrial respiratory capacity is a critical regulator of CD8+ T cell memory development. Immunity 36, 68–78. doi: 10.1016/j.immuni.2011.12.007
Van Kaer, L., Parekh, V. V., and Wu, L. (2013). Invariant natural killer T cells as sensors and managers of inflammation. Trends Immunol. 34, 50–58. doi: 10.1016/j.it.2012.08.009
Wang, X., Devaiah, S. P., Zhang, W., and Welti, R. (2006). Signaling functions of phosphatidic acid. Prog. Lipid Res. 45, 250–278. doi: 10.1016/j.plipres.2006.01.005
Watarai, H., Sekine-Kondo, E., Shigeura, T., Motomura, Y., Yasuda, T., Satoh, R., et al. (2012). Development and function of invariant natural killer T cells producing T(h)2- and T(h)17-cytokines. PLoS Biol. 10:e1001255. doi: 10.1371/journal.pbio.1001255
Wei, J., Yang, K., and Chi, H. (2014). Cutting edge: discrete functions of mTOR signaling in invariant NKT cell development and NKT17 fate decision. J. Immunol. 193, 4297–4301. doi: 10.4049/jimmunol.1402042
Werlen, G., Jacinto, E., Xia, Y., and Karin, M. (1998). Calcineurin preferentially synergizes with PKC-θ to activate JNK and IL-2 promoter in T lymphocytes. EMBO J. 17, 3101–3111. doi: 10.1093/emboj/17.11.3101
Wheeler, M. L., Dong, M. B., Brink, R., Zhong, X. P., and Defranco, A. L. (2013). Diacylglycerol kinase ζ limits B cell antigen receptor-dependent activation of ERK signaling to inhibit early antibody responses. Sci. Signal. 6, ra91. doi: 10.1126/scisignal.2004189
Williams, M. A., Holmes, B. J., Sun, J. C., and Bevan, M. J. (2006). Developing and maintaining protective CD8+ memory T cells. Immunol. Rev. 211, 146–153. doi: 10.1111/j.0105-2896.2006.00389.x
Willoughby, J. E., Costello, P. S., Nicolas, R. H., Robinson, N. J., Stamp, G., Powrie, F., et al. (2007). Raf signaling but not the ERK effector SAP-1 is required for regulatory T cell development. J. Immunol. 179, 6836–6844. doi: 10.4049/jimmunol.179.10.6836
Winter, J. N., Fox, T. E., Kester, M., Jefferson, L. S., and Kimball, S. R. (2010). Phosphatidic acid mediates activation of mTORC1 through the ERK signaling pathway. Am. J. Physiol. Cell Physiol. 299, C335–C344. doi: 10.1152/ajpcell.00039.2010
Wu, J., Shen, S., Yang, J., Xia, Z., and Zhong, X. P. (2013). Diacylglycerol kinase zeta positively controls the development of iNKT-17 cells. PLoS ONE 8:e75202. doi: 10.1371/journal.pone.0075202
Wu, J., Shin, J., Xie, D., Wang, H., Gao, J., and Zhong, X. P. (2014a). Tuberous sclerosis 1 promotes invariant NKT cell anergy and inhibits invariant NKT cell-mediated antitumor immunity. J. Immunol. 192, 2643–2650. doi: 10.4049/jimmunol.1302076
Wu, J., Yang, J., Yang, K., Wang, H., Gorentla, B., Shin, J., et al. (2014b). iNKT cells require TSC1 for terminal maturation and effector lineage fate decisions. J. Clin. Invest. 124, 1685–1698. doi: 10.1172/JCI69780
Wu, Y., Borde, M., Heissmeyer, V., Feuerer, M., Lapan, A. D., Stroud, J. C., et al. (2006). FOXP3 controls regulatory T cell function through cooperation with NFAT. Cell 126, 375–387. doi: 10.1016/j.cell.2006.05.042
Xie, D. L., Wu, J., Lou, Y. L., and Zhong, X. P. (2012). Tumor suppressor TSC1 is critical for T-cell anergy. Proc. Natl. Acad. Sci. U.S.A. 109, 14152–14157. doi: 10.1073/pnas.1119744109
Xing, Y., and Hogquist, K. A. (2012). T-cell tolerance: central and peripheral. Cold Spring Harb. Perspect. Biol. 4, 1–15. doi: 10.1101/cshperspect.a006957
Yanagisawa, K., Yasuda, S., Kai, M., Imai, S., Yamada, K., Yamashita, T., et al. (2007). Diacylglycerol kinase α suppresses tumor necrosis factor-alpha-induced apoptosis of human melanoma cells through NF-κB activation. Biochim. Biophys. Acta 1771, 462–474. doi: 10.1016/j.bbalip.2006.12.008
Yang, C., and Kazanietz, M. G. (2007). Chimaerins: GAPs that bridge diacylglycerol signalling and the small G-protein Rac. Biochem. J. 403, 1–12. doi: 10.1042/BJ20061750
Yang, E., Singh, B. K., Paustian, A. M., and Kambayashi, T. (2016a). Diacylglycerol Kinase ζ Is a Target To Enhance NK Cell Function. J. Immunol. 197, 934–941. doi: 10.4049/jimmunol.1600581
Yang, J., Zhang, P., Krishna, S., Wang, J., Lin, X., Huang, H., et al. (2016b). Unexpected positive control of NFκB and miR-155 by DGKα and ζ ensures effector and memory CD8+ T Cell differentiation. Oncotarget 23, 33744–33764. doi: 10.18632/oncotarget.8164
Yang, W., Gorentla, B., Zhong, X. P., and Shin, J. (2015). mTOR and its tight regulation for iNKT cell development and effector function. Mol. Immunol. 68, 536–545. doi: 10.1016/j.molimm.2015.07.022
Yoon, M. S., Sun, Y., Arauz, E., Jiang, Y., and Chen, J. (2011). Phosphatidic acid activates mammalian target of rapamycin complex 1 (mTORC1) kinase by displacing FK506 binding protein 38 (FKBP38) and exerting an allosteric effect. J. Biol. Chem. 286, 29568–29574. doi: 10.1074/jbc.M111.262816
Zamani, A., Decker, C., Cremasco, V., Hughes, L., Novack, D. V., and Faccio, R. (2015). Diacylglycerol kinase ζ (DGKζ) is a critical regulator of bone homeostasis via modulation of c-fos levels in osteoclasts. J. Bone Miner. Res. 30, 1852–1863. doi: 10.1002/jbmr.2533
Zehn, D., Lee, S. Y., and Bevan, M. J. (2009). Complete but curtailed T-cell response to very low-affinity antigen. Nature 458, 211–214. doi: 10.1038/nature07657
Zha, Y., Marks, R., Ho, A. W., Peterson, A. C., Janardhan, S., Brown, I., et al. (2006). T cell anergy is reversed by active Ras and is regulated by diacylglycerol kinase-α. Nat. Immunol. 7, 1166–1173. doi: 10.1038/ni1394
Zhang, L., Tschumi, B. O., Corgnac, S., Ruegg, M. A., Hall, M. N., Mach, J. P., et al. (2014). Mammalian target of rapamycin complex 1 orchestrates invariant NKT cell differentiation and effector function. J. Immunol. 193, 1759–1765. doi: 10.4049/jimmunol.1400769
Zhang, N., and Bevan, M. J. (2011). CD8+ T cells: foot soldiers of the immune system. Immunity 35, 161–168. doi: 10.1016/j.immuni.2011.07.010
Zhao, C., Du, G., Skowronek, K., Frohman, M. A., and Bar-Sagi, D. (2007). Phospholipase D2-generated phosphatidic acid couples EGFR stimulation to Ras activation by Sos. Nat. Cell Biol. 9, 706–712. doi: 10.1038/ncb1594
Zheng, Y., Zha, Y., Driessens, G., Locke, F., and Gajewski, T. F. (2012). Transcriptional regulator early growth response gene 2 (Egr2) is required for T cell anergy in vitro and in vivo. J. Exp. Med. 209, 2157–2163. doi: 10.1084/jem.20120342
Zheng, Y., Zha, Y., Spaapen, R. M., Mathew, R., Barr, K., Bendelac, A., et al. (2013). Egr2-dependent gene expression profiling and ChIP-Seq reveal novel biologic targets in T cell anergy. Mol. Immunol. 55, 283–291. doi: 10.1016/j.molimm.2013.03.006
Zhong, X. P., Guo, R., Zhou, H., Liu, C., and Wan, C. K. (2008). Diacylglycerol kinases in immune cell function and self-tolerance. Immunol. Rev. 224, 249–264. doi: 10.1111/j.1600-065X.2008.00647.x
Zhong, X. P., Hainey, E. A., Olenchock, B. A., Jordan, M. S., Maltzman, J. S., Nichols, K. E., et al. (2003). Enhanced T cell responses due to diacylglycerol kinase ζ deficiency. Nat. Immunol. 4, 882–890. doi: 10.1038/ni958
Zhong, X. P., Hainey, E. A., Olenchock, B. A., Zhao, H., Topham, M. K., and Koretzky, G. A. (2002). Regulation of T cell receptor-induced activation of the Ras-ERK pathway by diacylglycerol kinase ζ. J. Biol. Chem. 277, 31089–31098. doi: 10.1074/jbc.M203818200
Keywords: diacylglycerol kinase, regulatory T cells, invariant NKT cells
Citation: Chen SS, Hu Z and Zhong X-P (2016) Diacylglycerol Kinases in T Cell Tolerance and Effector Function. Front. Cell Dev. Biol. 4:130. doi: 10.3389/fcell.2016.00130
Received: 31 August 2016; Accepted: 27 October 2016;
Published: 10 November 2016.
Edited by:
Fumio Sakane, Chiba University, JapanReviewed by:
Kaoru Goto, Yamagata University School of Medicine, JapanAntonia Avila-Flores, Centro Nacional de Biotecnologia - CSIC, Spain
Copyright © 2016 Chen, Hu and Zhong. This is an open-access article distributed under the terms of the Creative Commons Attribution License (CC BY). The use, distribution or reproduction in other forums is permitted, provided the original author(s) or licensor are credited and that the original publication in this journal is cited, in accordance with accepted academic practice. No use, distribution or reproduction is permitted which does not comply with these terms.
*Correspondence: Xiao-Ping Zhong, WGlhb3BpbmcuemhvbmdAZHVrZS5lZHU=