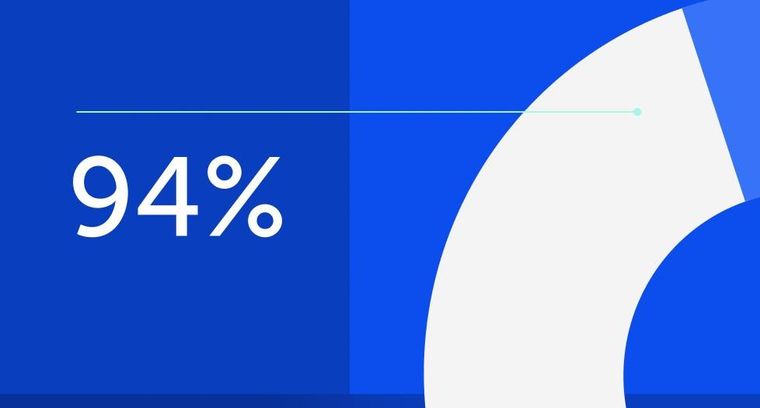
94% of researchers rate our articles as excellent or good
Learn more about the work of our research integrity team to safeguard the quality of each article we publish.
Find out more
MINI REVIEW article
Front. Cell Dev. Biol., 11 January 2016
Sec. Membrane Traffic and Organelle Dynamics
Volume 3 - 2015 | https://doi.org/10.3389/fcell.2015.00082
This article is part of the Research TopicGTPases regulate mitotic cell logisticsView all 7 articles
The small GTPase Ran regulates the interaction of transport receptors with a number of cellular cargo proteins. The high affinity binding of the GTP-bound form of Ran to import receptors promotes cargo release, whereas its binding to export receptors stabilizes their interaction with the cargo. This basic mechanism linked to the asymmetric distribution of the two nucleotide-bound forms of Ran between the nucleus and the cytoplasm generates a switch like mechanism controlling nucleo-cytoplasmic transport. Since 1999, we have known that after nuclear envelope breakdown (NEBD) Ran and the above transport receptors also provide a local control over the activity of factors driving spindle assembly and regulating other aspects of cell division. The identification and functional characterization of RanGTP mitotic targets is providing novel insights into mechanisms essential for cell division. Here we review our current knowledge on the RanGTP system and its regulation and we focus on the recent advances made through the characterization of its mitotic targets. We then briefly review the novel functions of the pathway that were recently described. Altogether, the RanGTP system has moonlighting functions exerting a spatial control over protein interactions that drive specific functions depending on the cellular context.
The first hints of the existence of a chromosome-dependent MT assembly mechanism in the dividing cell were obtained in the 1970–1980s when several groups reported that MT nucleation occurred close to or at the kinetochores (McGill and Brinkley, 1975; Telzer et al., 1975; Witt et al., 1980; De Brabander et al., 1981) and a spindle like structure formed around lambda DNA injected into metaphase arrested Xenopus eggs (Karsenti et al., 1984). In 1996, DNA coated beads were shown to trigger bipolar spindle formation when incubated in Xenopus egg extracts (Heald et al., 1996), providing further support to the idea that chromatin carries all the information required to direct MT assembly and organization in the M-phase cytoplasm. Shortly after, the identification of the small Ran GTPase as driver of chromatin-dependent MT assembly in the M-phase cytoplasm provided a major breakthrough to understand the underlying mechanism (Carazo-Salas et al., 1999; Kalab et al., 1999; Ohba et al., 1999; Wilde and Zheng, 1999; Zhang et al., 1999). Today, we know that the chromosomes drive MT assembly and organization into a bipolar spindle in a RanGTP dependent manner in most cells (Karsenti and Vernos, 2001; Rieder, 2005).
In this mini-review we will describe briefly how the RanGTP system regulates the nucleo-cytoplasmic shuttling of components in interphase and, after NEBD, the activity and/or localization of specific factors to drive spindle assembly. We will briefly review our current knowledge on the identity and function of RanGTP regulated factors and the recent advances on understanding novel mechanisms regulated by RanGTP. Finally we will provide an overview of the regulation of the RanGTP pathway itself during mitosis, its conservation in different organisms and cell types, and its role in other cellular functions. For additional information we refer the reader to excellent reviews (Ciciarello et al., 2007; O'Connell and Khodjakov, 2007; Clarke and Zhang, 2008; Kalab and Heald, 2008; Roscioli et al., 2010; Forbes et al., 2015).
Eukaryotic cells are compartmentalized and have specific transport systems for the communication between the cytoplasm and the different membrane-bound organelles. The nucleo-cytoplasmic transport system is essential to connect functionally the transcription of the genome that occurs within the nucleus, with protein translation that takes place in the cytoplasm (Figures 1A,B). The transport of molecules in and out of the nucleus occurs through the nuclear pore complex (NPC), a big protein complex of ~60 MDa inserted into the nuclear membrane (Sorokin et al., 2007). Small cargos (< 40 kDa) diffuse rapidly through the NPC. Instead, proteins larger than 40 kDa require an active transport through the NPC that involves soluble nuclear transport receptors (NTRs) that belong to the karyopherin-β protein family. NTRs that facilitate the transport of cargo proteins into the nucleus are called importins and interact with their cargo through a nuclear localization signal (NLS) rich in basic residues. NTRs facilitating the export of proteins out of the nucleus are called exportins and interact with their cargo through a nuclear export signal (NES) rich in hydrophobic residues such as leucine. The karyopherin-β importin β1 often interacts with the cargo through an adaptor of the importin α family (Sorokin et al., 2007). Importin α binds directly to the NLS of the cargo protein and to importin β1 through an IBB domain (importin β binding domain), leading to the formation of a trimeric complex.
Figure 1. The Ran system and its moonlighting functions. (A) Schematic representation of the Ran system for the spatial control of NLS and NES carrying proteins. In cells Ran is found in two forms, RanGTP (green), and RanGDP (light green). RCC1 (light blue) promotes the exchange of GDP to GTP, while RanGAP1-RanBP2 (in pink and purple) promote the hydrolysis of GTP into GDP. RanGTP binds to the importins (turquoise green) and exportins (light purple). Exportins in complex with RanGTP can associate to the NES-proteins (in brown). On the other hand, the binding of RanGTP to importins trigger their dissociation from NLS-proteins (yellow). (B) During interphase, the Ran system controls the nucleo-cytoplasmic shuttling of proteins, because RanGTP is predominant in the nucleoplasm and RanGDP is predominant in the cytoplasm (Sorokin et al., 2007). (C) During mitosis the association of RCC1, the RanGEF, with the chromosomes defines a gradient of RanGTP concentrations that promote the release of SAFs and MT nucleation around the chromatin. The Ran system is converted into a pathway for MT assembly and organization that is essential for mitotic spindle assembly. The RanGTP pathway depends on the establishment of a concentration gradient of RanGTP that peaks around the chromosomes (Kalab et al., 2002; Caudron et al., 2005). (D) At the end of mitosis, the Ran system also regulates nuclear membrane and NPC reassembly by controlling membrane fusion and releasing NPC components (Walther et al., 2003; Harel et al., 2003). (E) In ciliated cells RanGTP accumulates in the cilioplasm and promotes the transport and accumulation of Kif17 and retinis pigmentosa 2 to the cilioplasm (Dishinger et al., 2010; Fan et al., 2011; Hurd et al., 2011). (F) In neurons many SAFs have a function. Furthermore, importins localize to the dendritic synaptic space and are involved in the transport of cargos to the nucleus (Jordan and Kreutz, 2009; Panayotis et al., 2015). The Ran system is also active in the axon of the sciatic nerve, where upon injury importins promote the transport of cargos toward the neuron cell body (Hanz et al., 2003; Yudin et al., 2008).
NTRs associate with the small GTPase Ran that acts as a molecular switch. In its GTP bound form, Ran (RanGTP) interacts with karyopherin-β proteins with high affinity, while it dissociates in its GDP bound form (RanGDP). RanGTP binding to importins and exportins have very different consequences: it stabilizes the exportin-cargo interaction whereas it destabilizes the importin-cargo interaction (Figure 1A).
The RanGEF (guanine nucleotide exchange factor) RCC1 associates with the chromatin inside the nucleus, whereas RanGAP (GTPase activating protein) is cytoplasmic. As a consequence the predominant form of Ran in the nucleus is bound to GTP, while in the cytoplasm it is bound to GDP. Thereby NLS proteins transported to the nucleus by importins are released and accumulate in the nucleoplasm, whereas NES proteins in complex with exportin-RanGTP are transported out of the nucleus (Figures 1A,B).
Although the nucleo-cytoplasmic transport is no longer needed when a cell enters into mitosis, its complex molecular machinery is recycled to promote MT assembly around the chromatin and to direct the organization of the bipolar spindle (Clarke and Zhang, 2008).
As RCC1 remains associated with the chromatin after NEBD, RanGTP is highly enriched in the proximity of the chromosomes. As RanGTP diffuses away from the chromatin, RanGAP in the cytoplasm converts it into RanGDP (Figure 1C). The resulting gradient has been directly visualized in cells and Xenopus egg extracts (Kalab et al., 2002, 2006) and its properties in MT nucleation and stabilization tested and modeled (Caudron et al., 2005). Like in interphase, this system provides a spatial control over the stability of NTRs-cargo complexes. The cargos are NLS and/or NES containing proteins with specific functions related to spindle assembly and function. The NLS-proteins with a role in spindle assembly have been named SAFs (Spindle Assembly Factors).
The discovery and characterization of the RanGTP pathway prompted a re-examination of the Search and Capture model for spindle assembly proposed in 1986 (Kirschner and Mitchison, 1986). This model postulates that centrosomal MTs grow and shrink exploring the cytoplasmic space until a stochastic encounter with a kinetochore promotes their capture and attachment. However, it has been now clearly established that animal cells experimentally deprived from their centrosomes do assemble a functional mitotic spindle (Debec et al., 1995; Khodjakov et al., 2000). Moreover, mathematical simulations suggested that the Search and Capture mechanism could not account for the short division time observed in most animal cells (Wollman et al., 2005). By promoting MT nucleation and stabilization in the proximity of the chromosomes, the RanGTP pathway most certainly favors MT capture by the kinetochores increasing the efficiency of the Search and Capture mechanism. However, the role of the RanGTP pathway must go beyond MT capture by the kinetochores and kinetochore-fiber (K-fiber) formation since it also promotes MT organization in the absence of chromosomes, kinetochores, and K-fibers (Carazo-Salas et al., 1999). The identification of the direct and indirect RanGTP targets in the M-phase cytoplasm is therefore an essential step to fully understand the several roles this pathway fulfills during cell division.
A direct read out of the role of RanGTP in the M-phase cytoplasm was obtained in Xenopus egg extracts devoid of chromatin and centrosomes. Addition of RanGTP to these extracts is indeed sufficient to trigger MT nucleation, promote MT stabilization, and induce the organization of MT assemblies named mini-spindles (Carazo-Salas et al., 1999, 2001). Therefore, one or more SAFs maybe involved in these different events.
Since the identification of the first SAFs in 2001 (Gruss et al., 2001; Nachury et al., 2001; Wiese et al., 2001; Clarke and Zhang, 2008; Meunier and Vernos, 2012), the number of proteins controlled by RanGTP in mitosis has been slowly growing and several novel SAFs were identified recently (CDK11, CHD4, ISWI, Kif14, Kif2a, MCRS1, Mel28, Anillin, APC; Silverman-Gavrila et al., 2008; Yokoyama et al., 2008, 2009, 2014; Dikovskaya et al., 2010; Meunier and Vernos, 2011; Samwer et al., 2013; Wilbur and Heald, 2013). Currently, 22 proteins have been validated as SAFs (Table 1). In addition, a number of proteins with established roles in various aspects of spindle assembly are nuclear and could therefore be targets for RanGTP regulation (i.e., Kif4a/Klp1, Ino80, Reptin), but further studies should address this possibility.
Interestingly, the functional characterization of some of the SAFs is providing mechanistic insights into the RanGTP pathway functions in the dividing cell. The mechanism by which RanGTP promotes MT nucleation de novo in the M-phase cytoplasm was recently described (Scrofani et al., 2015). By releasing TPX2 from importins, RanGTP promotes its interaction with Aurora A and with a RHAMM-NEDD1-γTURC (γTubulin Ring Complex) complex. In this new complex the activated Aurora A phosphorylates NEDD1, an essential requirement for MT nucleation. Another SAF, Mel28, was shown to interact with the γTuRC and it was proposed to play a role in RanGTP dependent MT nucleation (Yokoyama et al., 2014). The potential cooperation of Mel28 with the TPX2-dependent pathway described above remains to be established.
The RanGTP pathway also contributes to centrosome maturation and its MT assembly activity (Carazo-Salas et al., 2001). In fact two SAFs, CDK11, and Mel28 were shown to favor MT assembly at the centrosome (Yokoyama et al., 2008, 2014).
The identification and characterization of another SAF, MCRS1, has revealed a novel and important mechanism for the regulation of K-fiber MT minus-end dynamics (Meunier and Vernos, 2011) and novel insights on the roles of the RanGTP pathway in spindle assembly and cell division (Meunier and Vernos, 2012). MCRS1, in complex with members of the chromatin modifier KAT8-associated nonspecific lethal (KANSL) complex (Meunier et al., 2015), is targeted to the minus-end of RanGTP-dependent MTs protecting them from depolymerisation. Within the spindle MCRS1 also associates specifically with the minus-ends of K-fiber MTs and regulates their depolymerisation rate playing an essential role in K-fiber dynamics and chromosome alignment (Meunier and Vernos, 2011; Meunier et al., 2015). The specific association of MCRS1 with the MTs nucleated by the RanGTP dependent pathway also suggests that these MTs have specific characteristics that distinguish them from the MTs nucleated by the centrosomes. If this turns out to be true, the chromosomal MTs would not be merely a local supply of MTs favoring an efficient Search and Capture mechanism, but they could provide essential unique functionalities required for the assembly and function of the bipolar spindle (Meunier et al., 2015).
Recently the MT depolymerizing kinesin Kif2a was shown to be regulated by RanGTP in mitosis, revealing an important mechanism for the scaling of the spindle to the cell size during the early development of Xenopus embryos (Wilbur and Heald, 2013). Kif2a is maintained inactive by importin α until stage 8 of embryonic development. As the soluble concentration of importin α decreases, Kif2a is released and function as a MT depolymerase promoting spindle shortening.
Although, most of the SAFs identified so far were found to play a role in the early phases of cell division, a number of recent reports indicate that the RanGTP pathway has other essential roles not directly related to spindle assembly. Indeed, the characterization of the SAF ISWI suggests functions for the RanGTP pathway during anaphase (Yokoyama et al., 2009).
Multiple lines of research also indicate that it plays a role in spindle positioning. Indeed, before entry into anaphase, the RanGTP gradient restricts the localization of the LGN-NuMa complex to cell cortex areas further away from the chromosomes, contributing to the control of spindle position and orientation (Kiyomitsu and Cheeseman, 2012).
In addition, RanGTP also regulates non-MT related targets. Indeed, it controls Anillin localization and triggers asymmetric membrane elongation during anaphase, defining spindle positioning at the center of the dividing cell (Silverman-Gavrila et al., 2008; Kiyomitsu and Cheeseman, 2012). Finally, during cytokinesis the RanGTP pathway regulates the activity of the kinesin Kif14/Nabkin in actin bundling (Carleton et al., 2006; Samwer et al., 2013) and coordinates nuclear membrane and NPC reassembly (Harel et al., 2003; Walther et al., 2003; Ciciarello et al., 2010; Roscioli et al., 2010; Forbes et al., 2015; Figure 1D).
It is therefore clear that the identification and functional characterization of the RanGTP mitotic targets is providing novel insights into the mechanism of spindle assembly and cell division. However, it is unclear whether many or only a few more RanGTP targets remain to be identified. This number could be potentially high as the number of nuclear proteins is in the order of hundreds or thousands (Dellaire et al., 2003), at least one order of magnitude above the current number of known RanGTP targets in the dividing cell (Table 1).
Most of the proteomic studies aimed at identifying novel SAFs have focused on importins α1 and β1 (Nachury et al., 2001; Wiese et al., 2001; Yokoyama et al., 2008), which are two of the most abundant importins in Xenopus egg extracts (Bernis et al., 2014; Wuhr et al., 2014). However, there are five additional α-importins and eight additional β-importins in humans (Cautain et al., 2015).
Although still scarce, some data indicate that indeed other importins also play a role during cell division. The RanGTP regulation of NuSAP was shown to depend on importin-β1 and importin-7 (Ribbeck et al., 2006) and that of Mel28, Nup107-160, and Nup98 on importin-β1 and transportin/importin-β2 (Lau et al., 2009). Transportin was also specifically shown to negatively regulate spindle assembly and nuclear membrane and NPC reassembly (Bernis et al., 2014). However, there are no described mitotic factors exclusively regulated by importin-7 or transportin.
The characterization of possible transportin specific targets and, more generally, of the other importins α and β represents an open field for exploration. This could be important to understand the regulation of the RanGTP pathway, especially considering that importins expression patterns change significantly in different developmental stages and tissues (Hosokawa et al., 2008).
Beyond the specificities of NTR-SAF interactions, several mechanisms may directly impinge upon the RanGTP pathway during cell division. Several data suggest that RCC1 itself is a key component under fine regulation. Human cells have three isoforms of RCC1, that are expressed in a tissue specific manner (Hood and Clarke, 2007). The isoforms differ at their N-terminus, a region involved in importin binding and regulated by phosphorylation, which was proposed to influence chromosome-coupled RanGTP production (Hood and Clarke, 2007; Li et al., 2007). Moreover, the level of RCC1 expression also varies in different cells and correlates with the steepness of the RanGTP gradient (Hasegawa et al., 2013). This may have important consequences as it was proposed that the steepness of the RanGTP gradient determines the length of prometaphase and metaphase which in turn may be relevant for chromosome segregation fidelity (Silkworth et al., 2012; Hasegawa et al., 2013).
Other mechanisms, such as post-translational modifications and alternative splicing are also potential strategies to control the NLS of SAFs. However, these mechanisms would rather affect a particular protein than the whole RanGTP pathway.
Recently, an alternative mechanism for the regulation of SAFs independently of RanGTP was proposed. The targeting of the Golgi protein GM130 to fragmented Golgi membranes in mitosis may compete out locally TPX2 from the importin α1 binding, thus favoring MT assembly in the vicinity of Golgi fragments (Wei et al., 2015). This competition-based mechanism could be another strategy to locally control SAFs sequestered by importins.
The binding of RanGTP to exportins stabilizes its interaction with NES-cargo proteins. The major exportin, CRM1, was shown to be involved in the targeting of NES-proteins to the kinetochore or the centrosomes. At the kinetochore, CRM1 recruits the RanBP2-RanGAP1-SUMO complex that is required for the interaction between MTs and the kinetochore (Arnaoutov et al., 2005). However, it is still mechanistically unclear how this complex favors the MT-kinetochore interaction (Forbes et al., 2015). CRM1 also promotes the recruitment of RanGAP1-RanBP2 to the spindle in a RanGTP dependent manner (Wu et al., 2013) and it is involved in tethering the Chromosome Passenger Complex to the centromere through its direct interaction with survivin (Knauer et al., 2006). CRM1 has also been shown to promote the recruitment of BRCA1 and pericentrin to the mitotic centrosomes, thus promoting the MT assembly activity of the centrosomes (Liu et al., 2009; Brodie and Henderson, 2012). Recently, the transcriptional repressor Bach1 was found to play a role in chromosome arm alignment during mitosis and to be excluded from the chromosomes during metaphase in a CRM1-dependent way (Li et al., 2012).
However, the significance of these targeting events is not entirely clear mechanistically (Yokoyama and Gruss, 2013). A major problem is that during mitosis the putative role of exportin mediated interactions may be difficult to untangle from that of importin mediated interactions, as they involve proteins having both NES and NLS [i.e., Pericentrin (Liu et al., 2010)]. Nevertheless, it seems evident that the RanGTP regulation of CRM1 has several roles during mitosis and it will be interesting to test whether other exportins are also important for mitotic events.
In the last 15 years the RanGTP pathway has been studied in several organisms and cell types. It was found to present variations on some details or in some cases to be unnecessary. Indeed, in some meiotic systems the contribution of the RanGTP pathway appears to be non-essential. For instance, Drosophila spermatocytes can assemble the meiosis I spindle in the complete absence of chromosomes (Bucciarelli et al., 2003). The assembly of the acentrosomal spindle of meiosis I in mice and frogs oocytes was also shown to be only partially dependent on the RanGTP pathway, although the pathway is strictly essential for spindle assembly during meiosis II (Dumont et al., 2007).
Even in systems relying on RanGTP for spindle assembly there are some variations at least at the level of the machinery. For instance, TPX2, which is essential in frogs and mammals, is not present in Caenorhabditis elegans and Drosophila melanogaster. Although proteins with some of the TPX2 characteristics have been identified in these systems (Ozlu et al., 2005; Goshima, 2011), they lack essential features of TPX2, like an NLS that is at the basis of the RanGTP regulation. This example indicates that the effectors of the RanGTP pathway might vary from system to system, although the main principles are probably maintained and conserved.
The RanGTP pathway is an example of a whole pathway that accomplishes essential functions in different parts of the cell cycle. In interphase, it orchestrates the nucleo-cytoplasmic transport, while in mitosis it drives spindle assembly and later nuclear membrane and NPC reassembly (Figures 1B–D). Individual proteins that have different functions at different times are defined as moonlighting proteins (Jeffery, 1999). The RanGTP pathway could therefore be an example of a moonlighting pathway.
The RanGTP pathway is particularly interesting, because it shows how the function of a protein depends on its context: most of the SAFs have nuclear functions and are kept separated from tubulins and others cytoskeleton proteins during interphase. Upon NEBD, the general context changes and the SAFs exert important functions related to the MTs.
Some data point toward a moonlighting function of the RanGTP pathway in cilia formation and in transport into the cilium. RanGTP has been shown to control the accumulation of Kif17 and retinis pigmentosa 2 to the cilioplasm (Dishinger et al., 2010; Hurd et al., 2011), where RanGTP is concentrated (Fan et al., 2011). The current working model is that the RanGTP pathway orchestrates the transport of cargos carrying a cilia localization signal through the cilia pore complex, which has been proposed to be located at the base of the cilium (Kee et al., 2012; Figure 1E). However, further studies are needed to understand how the RanGTP gradient is established in cilia and what other cargos it transports into the cilia.
Interestingly, the RanGTP pathway moonlights also in differentiated neurons, where many SAFs also have a function [TPX2, MCRS1, NuMa, Rae1, HSET (Ferhat et al., 1998; Davidovic et al., 2006; Mori et al., 2009; Tian et al., 2011; Pannu et al., 2015)]. Furthermore importins α and β accumulate at the dendritic synaptic space and have a role in the transport of cargos from the synapses to the nucleus (Jordan and Kreutz, 2009; Panayotis et al., 2015). Finally, a RanGTP regulated mechanism has been shown to be at play in response to sciatic nerve injuries (Hanz et al., 2003; Yudin et al., 2008; Figure 1F).
The identification of the role played by the RanGTP pathway during cell division occurred more than 15 years ago. We know now that the RanGTP pathway has additional functions and could be considered a moonlighting pathway controlling various important cellular processes (Figure 1). During cell division it drives essential mechanisms that we start to understand thanks to the identification and functional characterization of its direct targets. However, several open questions still need to be addressed. The total number of SAFs is difficult to anticipate and therefore we do not know how many still remain to be identified. Furthermore, most of our current knowledge is restricted to the role of only some components of the nucleo-cytoplasmic transport machinery. For instance, very little is currently known about the putative role in cell division of the different importins present in the human cell. Specific importins may regulate the activity of novel SAFs and their different expression patterns in different cell types and tissues may provide a relevant combinatorial mechanism. We also know little on the putative role of the components of the export machinery in spindle assembly and in the other novel functions of the pathway. Although, there are data suggesting various points of regulation of the pathway itself, the consequences on cell division and other processes are not clear yet, nor how it may be adapted to the requirements of different cell types or tissues. The study of the RanGTP pathway will certainly provide exciting new insights in the next few years, revealing some essential mechanisms for cell organization and function.
IV and TC wrote the manuscript, TC prepared the table and figure.
TC was supported by the Spanish Ministry of Economy and Competitiveness (MINECO) through the FPI fellowship BES-2010-031355. Work in the Vernos lab was supported by the Spanish ministry grants BFU2009-10202 and BFU2012-37163, co-financed by the European Regional Development Fund (ERDF/FEDER). We also acknowledge support of the Spanish Ministry of Economy and Competitiveness, “Centro de Excelencia Severo Ochoa 2013-2017,” SEV-2012-0208.
The authors declare that the research was conducted in the absence of any commercial or financial relationships that could be construed as a potential conflict of interest.
We want to thank the members of Vernos lab for critical discussions on the various aspects of the RanGTP pathway.
γTuRC, γTubulin Ring Complex; K-Fiber, Kinetochore-Fiber; KANLS, KAT8-associated nonspecific lethal complex; MT, Microtubule; NEBD, Nuclear Envelope Breakdown; NES, Nuclear Export Signal; NLS, Nuclear Localization Signal; NPC, Nuclear Pore Complex; NTR, Nuclear Transport Receptor; RanGAP, Ran GTPase Activating Protein; RanGEF, Ran Guanine nucleotide Exchange Factor; SAF, Spindle Assembly Factor.
Abad, P. C., Lewis, J., Mian, I. S., Knowles, D. W., Sturgis, J., Badve, S., et al. (2007). NuMA influences higher order chromatin organization in human mammary epithelium. Mol. Biol. Cell 18, 348–361. doi: 10.1091/mbc.E06-06-0551
Adam, S. A., Sengupta, K., and Goldman, R. D. (2008). Regulation of nuclear lamin polymerization by importin alpha. J. Biol. Chem. 283, 8462–8468. doi: 10.1074/jbc.M709572200
Ahmed, S. M., Theriault, B. L., Uppalapati, M., Chiu, C. W. N., Gallie, B. L., Sidhu, S. S., et al. (2012). KIF14 negatively regulates Rap1a-Radil signaling during breast cancer progression. J. Cell Biol. 199, 951–967. doi: 10.1083/jcb.201206051
Albee, A. J., Tao, W., and Wiese, C. (2006). Phosphorylation of maskin by Aurora-A is regulated by RanGTP and importin beta. J. Biol. Chem. 281, 38293–38301. doi: 10.1074/jbc.M607203200
Arnaoutov, A., Azuma, Y., Ribbeck, K., Joseph, J., Boyarchuk, Y., Karpova, T., et al. (2005). Crm1 is a mitotic effector of Ran-GTP in somatic cells. Nat. Cell Biol. 7, 626–632. doi: 10.1038/ncb1263
Beenders, B., Jones, P. L., and Bellini, M. (2007). The tripartite Motif of nuclear factor 7 is required for its association with transcriptional units. Mol. Cell. Biol. 27, 2615–2624. doi: 10.1128/MCB.01968-06
Bernis, C., Swift-Taylor, B., Nord, M., Carmona, S., Chook, Y. M., and Forbes, D. J. (2014). Transportin acts to regulate mitotic assembly events by target binding rather than Ran sequestration. Mol. Biol. Cell 25, 992–1009. doi: 10.1091/mbc.E13-08-0506
Blower, M. D., Nachury, M., Heald, R., and Weis, K. (2005). A Rae1-containing ribonucleoprotein complex is required for mitotic spindle assembly. Cell 121, 223–234. doi: 10.1016/j.cell.2005.02.016
Brodie, K. M., and Henderson, B. R. (2012). Characterization of BRCA1 protein targeting, dynamics, and function at the centrosome: a role for the nuclear export signal, CRM1, and Aurora A kinase. J. Biol. Chem. 287, 7701–7716. doi: 10.1074/jbc.M111.327296
Bucciarelli, E., Giansanti, M. G., Bonaccorsi, S., and Gatti, M. (2003). Spindle assembly and cytokinesis in the absence of chromosomes during Drosophila male meiosis. J. Cell Biol. 160, 993–999. doi: 10.1083/jcb.200211029
Carazo-Salas, R. E., Gruss, O. J., Mattaj, I. W., and Karsenti, E. (2001). Ran-GTP coordinates regulation of microtubule nucleation and dynamics during mitotic-spindle assembly. Nat. Cell Biol. 3, 228–234. doi: 10.1038/35060009
Carazo-Salas, R. E., Guarguaglini, G., Gruss, O. J., Segref, A., Karsenti, E., and Mattaj, I. W. (1999). Generation of GTP-bound Ran by RCC1 is required for chromatin-induced mitotic spindle formation. Nature 400, 178–181. doi: 10.1038/22133
Carleton, M., Mao, M., Biery, M., Warrener, P., Kim, S., Buser, C., et al. (2006). RNA interference-mediated silencing of mitotic Kinesin KIF14 disrupts cell cycle progression and induces cytokinesis failure. Mol. Cell. Biol. 26, 3853–3863. doi: 10.1128/MCB.26.10.3853-3863.2006
Casaletto, J. B. (2005). Inhibition of the anaphase-promoting complex by the Xnf7 ubiquitin ligase. J. Cell Biol. 169, 61–71. doi: 10.1083/jcb.200411056
Caudron, M., Bunt, G., Bastiaens, P., and Karsenti, E. (2005). Spatial coordination of spindle assembly by chromosome-mediated signaling gradients. Science 309, 1373–1376. doi: 10.1126/science.1115964
Cautain, B., Hill, R., de Pedro, N., and Link, W. (2015). Components and regulation of nuclear transport processes. FEBS J. 282, 445–462. doi: 10.1111/febs.13163
Ciciarello, M., Mangiacasale, R., and Lavia, P. (2007). Spatial control of mitosis by the GTPase Ran. Cell Mol. Life Sci 64, 1891–1914. doi: 10.1007/s00018-007-6568-2
Ciciarello, M., Roscioli, E., Di Fiore, B., Di Francesco, L., Sobrero, F., Bernard, D., et al. (2010). Nuclear reformation after mitosis requires downregulation of the Ran GTPase effector RanBP1 in mammalian cells. Chromosoma 119, 651–668. doi: 10.1007/s00412-010-0286-5
Clarke, P., and Zhang, R. C. (2008). Spatial and temporal coordination of mitosis by Ran GTPase. Nat. Rev. Mol. Cell Biol. 9, 464–477. doi: 10.1038/nrm2410
Compton, D. A., and Cleveland, D. W. (1993). NuMA is required for the proper completion of mitosis. J. Cell Biol. 120, 947–957. doi: 10.1083/jcb.120.4.947
Cross, M. K., and Powers, M. A. (2011). Nup98 regulates bipolar spindle assembly through association with microtubules and opposition of MCAK. Mol. Biol. Cell 22, 661–672. doi: 10.1091/mbc.E10-06-0478
Davidovic, L., Bechara, E., Gravel, M., Jaglin, X. H., Tremblay, S., Sik, A., et al. (2006). The nuclear microspherule protein 58 is a novel RNA-binding protein that interacts with fragile X mental retardation protein in polyribosomal mRNPs from neurons. Hum. Mol. Genet. 15, 1525–1538. doi: 10.1093/hmg/ddl074
Debec, A., Detraves, C., Montmory, C., Geraud, G., and Wright, M. (1995). Polar organization of gamma-tubulin in acentriolar mitotic spindles of Drosophila melanogaster cells. J. Cell Sci. 108(Pt 7), 2645–2653.
De Brabander, M., Geuens, G., De Mey, J., and Joniau, M. (1981). Nucleated assembly of mitotic microtubules in living PTK2 cells after release from nocodazole treatment. Cell Motil. 1, 469–483. doi: 10.1002/cm.970010407
Dellaire, G., Farrall, R., and Bickmore, W. A. (2003). The Nuclear Protein Database (NPD): sub-nuclear localisation and functional annotation of the nuclear proteome. Nucleic Acids Res. 31, 328–330. doi: 10.1093/nar/gkg018
Dikovskaya, D., Khoudoli, G., Newton, I. P., Chadha, G. S., Klotz, D., Visvanathan, A., et al. (2012). The adenomatous polyposis coli protein contributes to normal compaction of mitotic chromatin. PLoS ONE 7:e38102–38113. doi: 10.1371/journal.pone.0038102
Dikovskaya, D., Li, Z., Newton, I. P., Davidson, I., Hutchins, J. R. A., Kalab, P., et al. (2010). Microtubule assembly by the Apc protein is regulated by importin-ß-RanGTP. J. Cell Sci. 123, 736–746. doi: 10.1242/jcs.060806
Dikovskaya, D., Newton, I. P., and Nthke, I. S. (2004). The adenomatous polyposis coli protein is required for the formation of robust spindles formed in CSF Xenopus extracts. Mol. Biol. Cell 15, 2978–2991. doi: 10.1091/mbc.E03-08-0613
Dikovskaya, D., Zumbrunn, J., Penman, G. A., and Nathke, I. S. (2001). The adenomatous polyposis coli protein: in the limelight out at the edge. Trends Cell Biol. 11, 378–384. doi: 10.1016/S0962-8924(01)02069-4
Dishinger, J. F., Kee, H. L., Jenkins, P. M., Fan, S., Hurd, T. W., Hammond, J. W., et al. (2010). Ciliary entry of the kinesin-2 motor KIF17 is regulated by importin-beta2 and RanGTP. Nat. Cell Biol. 12, 703–710. doi: 10.1038/ncb2073
Dumont, J., Petri, S., Pellegrin, F., Terret, M. E., Bohnsack, M. T., Rassinier, P., et al. (2007). A centriole- and RanGTP-independent spindle assembly pathway in meiosis I of vertebrate oocytes. J. Cell Biol. 176, 295–305. doi: 10.1083/jcb.200605199
Eagleson, G., Pfister, K., Knowlton, A. L., Skoglund, P., Keller, R., and Stukenberg, P. T. (2015). Kif2a depletion generates chromosome segregation and pole coalescence defects in animal caps and inhibits gastrulation of the Xenopus embryo. Mol. Biol. Cell 26, 924–937. doi: 10.1091/mbc.E13-12-0721
Ems-McClung, S. C., Zheng, Y., and Walczak, C. E. (2004). Importin alpha/beta and Ran-GTP regulate XCTK2 microtubule binding through a bipartite nuclear localization signal. Mol. Biol. Cell 15, 46–57. doi: 10.1091/mbc.E03-07-0454
Etkin, L. D., el-Hodiri, H. M., Nakamura, H., Wu, C. F., Shou, W., and Gong, S. G. (1997). Characterization and function of Xnf7 during early development of Xenopus. J. Cell. Physiol. 173, 144–146.
Fan, S., Fogg, V., Wang, Q., Chen, X.-W., Liu, C.-J., and Margolis, B. (2007). A novel Crumbs3 isoform regulates cell division and ciliogenesis via importin beta interactions. J. Cell Biol.178, 387–398. doi: 10.1083/jcb.200609096
Fan, S., Whiteman, E. L., Hurd, T. W., McIntyre, J. C., Dishinger, J. F., Liu, C. J., et al. (2011). Induction of Ran GTP drives ciliogenesis. Mol. Biol. Cell 22, 4539–4548. doi: 10.1091/mbc.E11-03-0267
Farina, F., Pierobon, P., Delevoye, C., Monnet, J., Dingli, F., Loew, D., et al. (2013). Kinesin KIFC1 actively transports bare double-stranded DNA. Nucleic Acids Res. 41, 4926–4937. doi: 10.1093/nar/gkt204
Ferhat, L., Cook, C., Kuriyama, R., and Baas, P. W. (1998). The nuclear/mitotic apparatus protein NuMA is a component of the somatodendritic microtubule arrays of the neuron. J. Neurocytol. 27, 887–899. doi: 10.1023/A:1006949006728
Field, C. M., and Alberts, B. M. (1995). Anillin, a contractile ring protein that cycles from the nucleus to the cell cortex. J. Cell Biol. 131, 165–178. doi: 10.1083/jcb.131.1.165
Forbes, D. J., Travesa, A., Nord, M. S., and Bernis, C. (2015). Reprint of “Nuclear transport factors: global regulation of mitosis”. Curr. Opin. Cell Biol. 34, 122–134. doi: 10.1016/j.ceb.2015.07.005
Gaglio, T., Saredi, A., and Compton, D. A. (1995). NuMA is required for the organization of microtubules into aster-like mitotic arrays. J. Cell Biol. 131, 693–708. doi: 10.1083/jcb.131.3.693
Garriga-Canut, M., and Orkin, S. H. (2004). Transforming Acidic Coiled-coil Protein 3 (TACC3) Controls Friend of GATA-1 (FOG-1) subcellular localization and regulates the association between GATA-1 and FOG-1 during Hematopoiesis. J. Biol. Chem. 279, 23597–23605. doi: 10.1074/jbc.M313987200
Gergely, F., Kidd, D., Jeffers, K., Wakefield, J. G., and Raff, J. W. (2000). D-TACC: a novel centrosomal protein required for normal spindle function in the early Drosophila embryo. EMBO J. 19, 241–252. doi: 10.1093/emboj/19.2.241
Goshima, G. (2011). Identification of a TPX2-Like Microtubule-Associated Protein in Drosophila. PLoS ONE 6:e28120. doi: 10.1371/journal.pone.0028120
Gruss, O. J., Carazo-Salas, R. E., Schatz, C. A., Guarguaglini, G., Kast, J., Wilm, M., et al. (2001). Ran induces spindle assembly by reversing the inhibitory effect of importin alpha on TPX2 activity. Cell 104, 83–93. doi: 10.1016/S0092-8674(01)00193-3
Guo, Y., Partch, C. L., Key, J., Card, P. B., Pashkov, V., Patel, A., et al. (2013). Regulating the ARNT/TACC3 axis: multiple approaches to manipulating protein/protein interactions with small molecules. ACS Chem. Biol. 8, 626–635. doi: 10.1021/cb300604u
Hanz, S., Perlson, E., Willis, D., Zheng, J. Q., Massarwa, R., Huerta, J. J., et al. (2003). Axoplasmic importins enable retrograde injury signaling in lesioned nerve. Neuron 40, 1095–1104. doi: 10.1016/S0896-6273(03)00770-0
Harel, A., Chan, R. C., Lachish-Zalait, A., Zimmerman, E., Elbaum, M., and Forbes, D. J. (2003). Importin beta negatively regulates nuclear membrane fusion and nuclear pore complex assembly. Mol. Biol. Cell 14, 4387–4396. doi: 10.1091/mbc.E03-05-0275
Hasegawa, K., Ryu, S. J., and Kalab, P. (2013). Chromosomal gain promotes formation of a steep RanGTP gradient that drives mitosis in aneuploid cells. J. Cell Biol. 200, 151–161. doi: 10.1083/jcb.201206142
Heald, R., Tournebize, R., Blank, T., Sandaltzopoulos, R., Becker, P., Hyman, A., et al. (1996). Self-organization of microtubules into bipolar spindles around artificial chromosomes in Xenopus egg extracts. Nature 382, 420–425. doi: 10.1038/382420a0
Hood, F. E., and Clarke, P. R. (2007). RCC1 isoforms differ in their affinity for chromatin, molecular interactions and regulation by phosphorylation. J. Cell Sci. 120, 3436–3445. doi: 10.1242/jcs.009092
Hosokawa, K., Nishi, M., Sakamoto, H., Tanaka, Y., and Kawata, M. (2008). Regional distribution of importin subtype mRNA expression in the nervous system: Study of early postnatal and adult mouse. Neuroscience 157, 864–877. doi: 10.1016/j.neuroscience.2008.09.045
Hurd, T. W., Fan, S., and Margolis, B. L. (2011). Localization of retinitis pigmentosa 2 to cilia is regulated by Importin beta2. J. Cell Sci. 124, 718–726. doi: 10.1242/jcs.070839
Jeffery, C. J. (1999). Moonlighting proteins. Trends Biochem. Sci. 24, 8–11. doi: 10.1016/S0968-0004(98)01335-8
Jordan, B. A., and Kreutz, M. R. (2009). Nucleocytoplasmic protein shuttling: the direct route in synapse-to-nucleus signaling. Trends Neurosci. 32, 392–401. doi: 10.1016/j.tins.2009.04.001
Kalab, P., and Heald, R. (2008). The RanGTP gradient - a GPS for the mitotic spindle. J. Cell Sci. 121, 1577–1586. doi: 10.1242/jcs.005959
Kalab, P., Pralle, A., Isacoff, E. Y., Heald, R., and Weis, K. (2006). Analysis of a RanGTP-regulated gradient in mitotic somatic cells. Nature 440, 697–701. doi: 10.1038/nature04589
Kalab, P., Pu, R. T., and Dasso, M. (1999). The ran GTPase regulates mitotic spindle assembly. Curr. Biol. 9, 481–484. doi: 10.1016/S0960-9822(99)80213-9
Kalab, P., Weis, K., and Heald, R. (2002). Visualization of a Ran-GTP gradient in interphase and mitotic Xenopus egg extracts. Science 295, 2452–2456. doi: 10.1126/science.1068798
Karsenti, E., Newport, J., and Kirschner, M. W. (1984). Respective roles of centrosomes and chromatin in the conversion of microtubule arrays from interphase to metaphase. J. Cell Biol. 99, 47s–54s. doi: 10.1083/jcb.99.1.47s
Karsenti, E., and Vernos, I. (2001). The mitotic spindle: a self-made machine. Science 294, 543–547. doi: 10.1126/science.1063488
Kee, H. L., Dishinger, J. F., Blasius, T. L., Liu, C. J., Margolis, B., and Verhey, K. J. (2012). A size-exclusion permeability barrier and nucleoporins characterize a ciliary pore complex that regulates transport into cilia. Nat. Cell Biol. 14, 431–437. doi: 10.1038/ncb2450
Khodjakov, A. L., Cole, R. W., Oakley, B. R., and Rieder, C. L. (2000). Centrosome-independent mitotic spindle formation in vertebrates. Curr. Biol. 10, 59–67. doi: 10.1016/S0960-9822(99)00276-6
Kirschner, M. W., and Mitchison, T. J. (1986). Beyond self-assembly: from microtubules to morphogenesis. Cell 45, 329–342. doi: 10.1016/0092-8674(86)90318-1
Kiyomitsu, T., and Cheeseman, I. M. (2012). Chromosome- and spindle-pole-derived signals generate an intrinsic code for spindle position and orientation. Nat. Cell Biol. 14, 311–317. doi: 10.1038/ncb2440
Kiyomitsu, T., and Cheeseman, I. M. (2013). Cortical Dynein and Asymmetric Membrane elongation coordinately position the spindle in anaphase. Cell 154, 391–402. doi: 10.1016/j.cell.2013.06.010
Knauer, S. K., Bier, C., Habtemichael, N., and Stauber, R. H. (2006). The Survivin-Crm1 interaction is essential for chromosomal passenger complex localization and function. EMBO Rep. 7, 1259–1265. doi: 10.1038/sj.embor.7400824
Koffa, M. D., Casanova, C. M., Santarella, R., Kcher, T., Wilm, M., and Mattaj, I. W. (2006). HURP is part of a Ran-dependent complex involved in spindle formation. Curr. Biol. 16, 743–754. doi: 10.1016/j.cub.2006.03.056
Laprise, P., Viel, A., and Rivard, N. (2004). Human Homolog of Disc-large Is required for adherens junction assembly and differentiation of human intestinal epithelial cells. J. Biol. Chem. 279, 10157–10166. doi: 10.1074/jbc.M309843200
Lau, C. K., Delmar, V. A., Chan, R. C., Phung, Q., Bernis, C., Fichtman, B., et al. (2009). Transportin regulates major mitotic assembly events: from spindle to nuclear pore assembly. Mol. Biol. Cell 20, 4043–4058. doi: 10.1091/mbc.E09-02-0152
Li, H. Y., Ng, W. P., Wong, C. H., Iglesias, P. A., and Zheng, Y. (2007). Coordination of chromosome alignment and mitotic progression by the chromosome-based Ran signal. Cell Cycle 6, 1886–1895. doi: 10.4161/cc.6.15.4487
Li, J., Shiraki, T., and Igarashi, K. (2012). Transcription-independent role of Bach1 in mitosis through a nuclear exporter Crm1-dependent mechanism. FEBS Lett. 586, 448–454. doi: 10.1016/j.febslet.2012.01.028
Liu, Q., Jiang, Q., and Zhang, C. (2009). A fraction of Crm1 locates at centrosomes by its CRIME domain and regulates the centrosomal localization of pericentrin. Biochem. Biophys. Res. Commun. 384, 383–388. doi: 10.1016/j.bbrc.2009.04.154
Liu, Q., Yu, J., Zhuo, X., Jiang, Q., and Zhang, C. (2010). Pericentrin contains five NESs and an NLS essential for its nucleocytoplasmic trafficking during the cell cycle. Cell Res. 20, 948–962. doi: 10.1038/cr.2010.89
Maor-Nof, M., Homma, N., Raanan, C., Nof, A., Hirokawa, N., and Yaron, A. (2013). Axonal pruning is actively regulated by the microtubule-destabilizing protein kinesin superfamily protein 2A. Cell Rep. 3, 971–977. doi: 10.1016/j.celrep.2013.03.005
Maresca, T. J., Niederstrasser, H., Weis, K., and Heald, R. (2005). Xnf7 contributes to spindle integrity through its microtubule-bundling activity. Curr. Biol. 15, 1755–1761. doi: 10.1016/j.cub.2005.08.049
McGill, M., and Brinkley, B. R. (1975). Human chromosomes and centrioles as nucleating sites for the in vitro assembly of microtubules from bovine brain tubulin. J. Cell Biol. 67, 189–199. doi: 10.1083/jcb.67.1.189
Meunier, S., Shvedunova, M., Van Nguyen, N., Avila, L., Vernos, I., and Akhtar, A. (2015). An epigenetic regulator emerges as microtubule minus-end binding and stabilizing factor in mitosis. Nat Commun 6, 7889. doi: 10.1038/ncomms8889
Meunier, S., and Vernos, I. (2011). K-fibre minus ends are stabilized by a RanGTP-dependent mechanism essential for functional spindle assembly. Nat. Cell Biol. 13, 1406–1414. doi: 10.1038/ncb2372
Meunier, S., and Vernos, I. (2012). Microtubule assembly during mitosis - from distinct origins to distinct functions? J. Cell Sci. 125, 2805–2814. doi: 10.1242/jcs.092429
Mishra, R. K., Chakraborty, P., Arnaoutov, A., Fontoura, B. M. A., and Dasso, M. (2010). The Nup107-160 complex and gamma-TuRC regulate microtubule polymerization at kinetochores. Nat. Cell Biol. 12, 164–169. doi: 10.1038/ncb2016
Miyamoto, T., Hosoba, K., Ochiai, H., Royba, E., Izumi, H., Sakuma, T., et al. (2015). The microtubule-depolymerizing activity of a mitotic kinesin protein KIF2A drives primary cilia disassembly coupled with cell proliferation. Cell Rep. 10, 664–673. doi: 10.1016/j.celrep.2015.01.003
Mori, D., Yamada, M., Mimori-Kiyosue, Y., Shirai, Y., Suzuki, A., Ohno, S., et al. (2009). An essential role of the aPKC-Aurora A-NDEL1 pathway in neurite elongation by modulation of microtubule dynamics. Nat. Cell Biol. 11, 1057–1068. doi: 10.1038/ncb1919
Nachury, M. V., Maresca, T. J., Salmon, W. C., Waterman-Storer, C. M., Heald, R., and Weis, K. (2001). Importin beta is a mitotic target of the small GTPase Ran in spindle assembly. Cell 104, 95–106. doi: 10.1016/S0092-8674(01)00194-5
Nath, S., Bananis, E., Sarkar, S., Stockert, R. J., Sperry, A. O., Murray, J. W., et al. (2007). Kif5B and Kifc1 interact and are required for motility and fission of early endocytic vesicles in mouse liver. Mol. Biol. Cell 18, 1839–1849. doi: 10.1091/mbc.E06-06-0524
Neumayer, G., Belzil, C., Gruss, O. J., and Nguyen, M. D. (2014). TPX2: of spindle assembly, DNA damage response, and cancer. Cell. Mol. Life Sci. 71, 3027–3047. doi: 10.1007/s00018-014-1582-7
Nwagbara, B. U., Faris, A. E., Bearce, E. A., Erdogan, B., Ebbert, P. T., Evans, M. F., et al. (2014). TACC3 is a microtubule plus end-tracking protein that promotes axon elongation and also regulates microtubule plus end dynamics in multiple embryonic cell types. Mol. Biol. Cell 25, 3350–3362. doi: 10.1091/mbc.E14-06-1121
O'Connell, C. B., and Khodjakov, A. L. (2007). Cooperative mechanisms of mitotic spindle formation. J. Cell Sci. 120, 1717–1722. doi: 10.1242/jcs.03442
Ohba, T., Nakamura, M., Nishitani, H., and Nishimoto, T. (1999). Self-organization of microtubule asters induced in Xenopus egg extracts by GTP-bound Ran. Science 284, 1356–1358. doi: 10.1126/science.284.5418.1356
Orjalo, A. V., Arnaoutov, A., Shen, Z., Boyarchuk, Y., Zeitlin, S. G., Fontoura, B., et al. (2006). The Nup107-160 nucleoporin complex is required for correct bipolar spindle assembly. Mol. Biol. Cell 17, 3806–3818. doi: 10.1091/mbc.E05-11-1061
Oshaughnessy, A., and Hendrich, B. (2013). CHD4 in the DNA-damage response and cell cycle progression: not so NuRDy now. Biochem. Soc. Trans. 41, 777–782. doi: 10.1042/BST20130027
Osmanagic-Myers, S., Dechat, T., and Foisner, R. (2015). Lamins at the crossroads of mechanosignaling. Genes Dev. 29, 225–237. doi: 10.1101/gad.255968.114
Ozlu, N., Srayko, M., Kinoshita, K., Habermann, B. T., O'Toole, E., Muller-Reichert, T., et al. (2005). An essential function of the C. elegans ortholog of TPX2 is to localize activated aurora A kinase to mitotic spindles. Dev. Cell 9, 237–248. doi: 10.1016/j.devcel.2005.07.002
Panayotis, N., Karpova, A., Kreutz, M. R., and Fainzilber, M. (2015). Macromolecular transport in synapse to nucleus communication. Trends Neurosci. 38, 108–116. doi: 10.1016/j.tins.2014.12.001
Pannu, V., Rida, P. C. G., and Aneja, R. (2015). “The human Kinesin-14 motor KifC1/HSET is an attractive anti-cancer drug target,” in Kinesins and Cancer, eds F. S. Kozielski and D. A. Skoufias (Dordrecht: Springer), 101–116. Available online at: http://link.springer.com/book/10.1007%2F978-94-017-9732-0
Perchiniak, E. M., and Groden, J. (2011). Mechanisms regulating microtubule binding, DNA replication, and apoptosis are controlled by the intestinal tumor suppressor APC. Curr. Colorectal Cancer Rep. 7, 145–151. doi: 10.1007/s11888-011-0088-z
Peset, I., Seiler, J., Sardon, T., Bejarano, L. A., Rybina, S., and Vernos, I. (2005). Function and regulation of Maskin, a TACC family protein, in microtubule growth during mitosis. J. Cell Biol. 170, 1057–1066. doi: 10.1083/jcb.200504037
Petretti, C., Savoian, M., Montembault, E., Glover, D. M., Prigent, C., and Giet, R. (2006). The PITSLRE/CDK11p58 protein kinase promotes centrosome maturation and bipolar spindle formation. EMBO Rep. 7, 418–424. doi: 10.1038/sj.embor.7400639
Platani, M., Santarella-Mellwig, R., Posch, M., Walczak, R., Swedlow, J. R., and Mattaj, I. W. (2009). The Nup107-160 nucleoporin complex promotes mitotic events via control of the localization state of the chromosome passenger complex. Mol. Biol. Cell 20, 5260–5275. doi: 10.1091/mbc.E09-05-0377
Pritchard, C. E., Fornerod, M., Kasper, L. H., and van Deursen, J. M. (1999). RAE1 is a shuttling mRNA export factor that binds to a GLEBS-like NUP98 motif at the nuclear pore complex through multiple domains. J. Cell Biol. 145, 237–254. doi: 10.1083/jcb.145.2.237
Radulescu, A. E., and Cleveland, D. W. (2010). NuMA after 30 years: the matrix revisited. Trends Cell Biol. 20, 214–222. doi: 10.1016/j.tcb.2010.01.003
Raemaekers, T. (2003). NuSAP, a novel microtubule-associated protein involved in mitotic spindle organization. J. Cell Biol. 162, 1017–1029. doi: 10.1083/jcb.200302129
Raja, S. J., Charapitsa, I., Conrad, T., Vaquerizas, J. M., Gebhardt, P., Holz, H., et al. (2010). The nonspecific lethal complex is a transcriptional regulator in Drosophila. Mol. Cell 38, 827–841. doi: 10.1016/j.molcel.2010.05.021
Rasala, B. A., Orjalo, A. V., Shen, Z., Briggs, S., and Forbes, D. J. (2006). ELYS is a dual nucleoporin/kinetochore protein required for nuclear pore assembly and proper cell division. Proc. Natl. Acad. Sci. U. S. A. 103, 17801–17806. doi: 10.1073/pnas.0608484103
Ribbeck, K., Groen, A. C., Santarella, R., Bohnsack, M. T., Raemaekers, T., Kcher, T., et al. (2006). NuSAP, a mitotic RanGTP target that stabilizes and cross-links microtubules. Mol. Biol. Cell 17, 2646–2660. doi: 10.1091/mbc.E05-12-1178
Ribbeck, K., Raemaekers, T., Carmeliet, G., and Mattaj, I. W. (2007). A role for NuSAP in linking microtubules to mitotic chromosomes. Curr. Biol. 17, 230–236. doi: 10.1016/j.cub.2006.11.050
Rieder, C. L. (2005). Kinetochore fiber formation in animal somatic cells: dueling mechanisms come to a draw. Chromosoma 114, 310–318. doi: 10.1007/s00412-005-0028-2
Roscioli, E., Bolognesi, A., Guarguaglini, G., and Lavia, P. (2010). Ran control of mitosis in human cells: gradients and local signals. Biochem. Soc. Trans. 38, 1709–1714. doi: 10.1042/BST0381709
Samwer, M., Dehne, H.-J., Spira, F., Kollmar, M., Gerlich, D. W., Urlaub, H., et al. (2013). The nuclear F-actin interactome of Xenopus oocytes reveals an actin-bundling kinesin that is essential for meiotic cytokinesis. EMBO J. 32, 1886–1902. doi: 10.1038/emboj.2013.108
Scrofani, J., Sardon, T., Meunier, S., and Vernos, I. (2015). Microtubule nucleation in Mitosis by a RanGTP-dependent protein complex. Curr. Biol. 25, 131–140. doi: 10.1016/j.cub.2014.11.025
Shimono, K., Shimono, Y., Shimokata, K., Ishiguro, N., and Takahashi, M. (2005). Microspherule protein 1, Mi-2beta, and RET finger protein associate in the nucleolus and up-regulate ribosomal gene transcription. J. Biol. Chem. 280, 39436–39447. doi: 10.1074/jbc.M507356200
Silkworth, W. T., Nardi, I. K., Paul, R., Amogilner, A., and Cimini, D. (2012). Timing of centrosome separation is important for accurate chromosome segregation. Mol. Biol. Cell 23, 401–411. doi: 10.1091/mbc.E11-02-0095
Sillje, H. H., Nagel, S., Korner, R., and Nigg, E. A. (2006). HURP is a Ran-importin beta-regulated protein that stabilizes kinetochore microtubules in the vicinity of chromosomes. Curr. Biol. 16, 731–742. doi: 10.1016/j.cub.2006.02.070
Silverman-Gavrila, R. V., Hales, K. G., and Wilde, A. (2008). Anillin-mediated targeting of peanut to pseudocleavage furrows is regulated by the GTPase Ran. Mol. Biol. Cell 19, 3735–3744. doi: 10.1091/mbc.E08-01-0049
Sinnott, R., Winters, L., Larson, B., Mytsa, D., Taus, P., Cappell, K. M., et al. (2014). Mechanisms promoting escape from mitotic stress-induced tumor cell death. Cancer Res. 74, 3857–3869. doi: 10.1158/0008-5472.CAN-13-3398
Sorokin, A. V., Kim, E. R., and Ovchinnikov, L. P. (2007). Nucleocytoplasmic transport of proteins. Biochemistry 72, 1439–1457. doi: 10.1134/S0006297907130032
Stanley, F. K. T., Moore, S., and Goodarzi, A. A. (2013). CHD chromatin remodelling enzymes and the DNA damage response. Mutat. Res. 750, 31–44. doi: 10.1016/j.mrfmmm.2013.07.008
Stebbins-Boaz, B., Cao, Q., de Moor, C. H., Mendez, R., and Richter, J. D. (1999). Maskin is a CPEB-associated factor that transiently interacts with elF-4E. Mol. Cell 4, 1017–1027. doi: 10.1016/S1097-2765(00)80230-0
Tahara, K., Takagi, M., Ohsugi, M., Sone, T., Nishiumi, F., Maeshima, K., et al. (2008). Importin-β and the small guanosine triphosphatase Ran mediate chromosome loading of the human chromokinesin Kid. J. Cell Biol. 180, 493–506. doi: 10.1083/jcb.200708003
Telzer, B. R., Moses, M. J., and Rosenbaum, J. L. (1975). Assembly of microtubules onto kinetochores of isolated mitotic chromosomes of HeLa cells. Proc. Natl. Acad. Sci. U.S.A. 72, 4023–4027. doi: 10.1073/pnas.72.10.4023
Tian, X., Li, J., Valakh, V., DiAntonio, A., and Wu, C. (2011). Drosophila Rae1 controls the abundance of the ubiquitin ligase Highwire in post-mitotic neurons. Nat. Neurosci. 14, 1267–1275. doi: 10.1038/nn.2922
Tokai, N., Fujimoto-Nishiyama, A., Toyoshima, Y., Yonemura, S., Tsukita, S., Inoue, J., et al. (1996). Kid, a novel kinesin-like DNA binding protein, is localized to chromosomes and the mitotic spindle. EMBO J. 15, 457–467.
Toto, M., Dangelo, G., and Corona, D. F. V. (2014). Regulation of ISWI chromatin remodelling activity. Chromosoma 123, 91–102. doi: 10.1007/s00412-013-0447-4
Tsai, M.-Y., Wang, S., Heidinger, J. M., Shumaker, D. K., Adam, S. A., Goldman, R. D., et al. (2006). A mitotic lamin B matrix induced by RanGTP required for spindle assembly. Science 311, 1887–1893. doi: 10.1126/science.1122771
Tsou, A.-P., Yang, C.-W., Huang, C.-Y. F., Yu, R. C. T., Lee, Y.-C. G., Chang, C.-W., et al. (2003). Identification of a novel cell cycle regulated gene, HURP, overexpressed in human hepatocellular carcinoma. Oncogene 22, 298–307. doi: 10.1038/sj.onc.1206129
Vidi, P. A., Liu, J., Salles, D., Jayaraman, S., Dorfman, G., Gray, M., et al. (2014). NuMA promotes homologous recombination repair by regulating the accumulation of the ISWI ATPase SNF2h at DNA breaks. Nucleic Acids Res. 42, 6365–6379. doi: 10.1093/nar/gku296
Walczak, C. E., Verma, S., and Mitchison, T. J. (1997). XCTK2: a kinesin-related protein that promotes mitotic spindle assembly in Xenopus laevis egg extracts. J. Cell Biol. 136, 859–870. doi: 10.1083/jcb.136.4.859
Walther, T. C, Askjaer, P., Gentzel, M., Habermann, A., Griffiths, G., Wilm, M., et al. (2003). RanGTP mediates nuclear pore complex assembly. Nature 424, 689–694. doi: 10.1038/nature01898
Watanabe, S., and Peterson, C. L. (2010). The INO80 family of chromatin-remodeling enzymes: regulators of histone variant dynamics. Cold Spring Harbor Symp. Quant. Biol. 75, 35–42. doi: 10.1101/sqb.2010.75.063
Wei, J. H., Zhang, Z. C., Wynn, R. M., and Seemann, J. (2015). GM130 Regulates Golgi-derived spindle assembly by activating TPX2 and capturing microtubules. Cell 162, 287–299. doi: 10.1016/j.cell.2015.06.014
Wiese, C. (2001). Role of importin-beta in coupling Ran to downstream targets in microtubule assembly. Science 291, 653–656. doi: 10.1126/science.1057661
Wiese, C., Wilde, A., Moore, M. S., Adam, S. A., Merdes, A., and Zheng, Y. (2001). Role of importin-beta in coupling Ran to downstream targets in microtubule assembly. Science 291, 653–656. doi: 10.1126/science.1057661
Wilbur, J. D., and Heald, R. (2013). Mitotic spindle scaling during Xenopus development by kif2a and importin α. eLife 2:e00290. doi: 10.7554/eLife.00290
Wilde, A., and Zheng, Y. (1999). Stimulation of microtubule aster formation and spindle assembly by the small GTPase Ran. Science 284, 1359–1362. doi: 10.1126/science.284.5418.1359
Witt, P. L., Ris, H., and Borisy, G. G. (1980). Origin of kinetochore microtubules in Chinese hamster ovary cells. Chromosoma 81, 483–505. doi: 10.1007/BF00368158
Wittmann, T., Wilm, M., Karsenti, E., and Vernos, I. (2000). TPX2, A novel xenopus MAP involved in spindle pole organization. J. Cell Biol. 149, 1405–1418. doi: 10.1083/jcb.149.7.1405
Wollman, R., Cytrynbaum, E. N., Jones, J. T., Meyer, T., Scholey, J. M., and Amogilner, A. (2005). Efficient chromosome capture requires a bias in the 'search-and-capture' process during mitotic-spindle assembly. Curr. Biol. 15, 828–832. doi: 10.1016/j.cub.2005.03.019
Wong, R. W., Blobel, G., and Coutavas, E. (2006). Rae1 interaction with NuMA is required for bipolar spindle formation. Proc. Natl. Acad. Sci. U.S.A. 103, 19783–19787. doi: 10.1073/pnas.0609582104
Wu, Z., Jiang, Q., Clarke, P. R., and Zhang, C. (2013). Phosphorylation of Crm1 by CDK1-cyclin-B promotes Ran-dependent mitotic spindle assembly. J. Cell Sci. 126, 3417–3428. doi: 10.1242/jcs.126854
Wuhr, M., Freeman, R. M. Jr., Presler, M., Horb, M. E., Peshkin, L., Gygi, S. P., et al. (2014). Deep proteomics of the Xenopus laevis Egg using an mRNA-Derived reference database. Curr. Biol. 24, 1467–1475. doi: 10.1016/j.cub.2014.05.044
Yokoyama, H., and Gruss, O. J. (2013). New mitotic regulators released from chromatin. Front. Oncol. 3:308. doi: 10.3389/fonc.2013.00308
Yokoyama, H., Gruss, O. J., Rybina, S., Caudron, M., Schelder, M., Wilm, M., et al. (2008). Cdk11 is a RanGTP-dependent microtubule stabilization factor that regulates spindle assembly rate. J. Cell Biol. 180, 867–875. doi: 10.1083/jcb.200706189
Yokoyama, H., Koch, B., Walczak, R., Duygu, F. C., González-Sánchez, J. C., Devos, D. P., et al. (2014). The nucleoporin MEL-28 promotes RanGTP-dependent \γ-tubulin recruitment and microtubule nucleation in mitotic spindle formation. Nat. Commun. 5, 1–9. doi: 10.1038/ncomms4270
Yokoyama, H., Nakos, K., Santarella-Mellwig, R., Rybina, S., Krijgsveld, J., Koffa, M. D., et al. (2013). CHD4 Is a RanGTP-Dependent MAP that Stabilizes microtubules and regulates bipolar spindle formation. Curr. Biol. 23, 2443–2451. doi: 10.1016/j.cub.2013.09.062
Yokoyama, H., Rybina, S., Santarella-Mellwig, R., Mattaj, I. W., and Karsenti, E. (2009). ISWI is a RanGTP-dependent MAP required for chromosome segregation. J. Cell Biol. 187, 813–829. doi: 10.1083/jcb.200906020
Yudin, D., Hanz, S., Yoo, S., Iavnilovitch, E., Willis, D., Gradus, T., et al. (2008). Localized regulation of Axonal RanGTPase controls retrograde injury signaling in peripheral nerve. Neuron 59, 241–252. doi: 10.1016/j.neuron.2008.05.029
Zeng, C., He, D., Berget, S. M., and Brinkley, B. R. (1994). Nuclear-mitotic apparatus protein: a structural protein interface between the nucleoskeleton and RNA splicing. Proc. Natl. Acad. Sci. U.S.A. 91, 1505–1509. doi: 10.1073/pnas.91.4.1505
Zhang, C., Hughes, M., and Clarke, P. R. (1999). Ran-GTP stabilises microtubule asters and inhibits nuclear assembly in Xenopus egg extracts. J. Cell Sci. 112 (Pt 14), 2453–2461.
Keywords: spindle, RanGTP, microtubule, cell division, importin, SAF, nucleo-cytoplasmic transport, exportin
Citation: Cavazza T and Vernos I (2016) The RanGTP Pathway: From Nucleo-Cytoplasmic Transport to Spindle Assembly and Beyond. Front. Cell Dev. Biol. 3:82. doi: 10.3389/fcell.2015.00082
Received: 22 September 2015; Accepted: 07 December 2015;
Published: 11 January 2016.
Edited by:
Patrizia Lavia, Institute of Molecular Biology and Pathology - CNR National Research Council of Italy, ItalyReviewed by:
Daniel Ungar, The University of York, UKCopyright © 2016 Cavazza and Vernos. This is an open-access article distributed under the terms of the Creative Commons Attribution License (CC BY). The use, distribution or reproduction in other forums is permitted, provided the original author(s) or licensor are credited and that the original publication in this journal is cited, in accordance with accepted academic practice. No use, distribution or reproduction is permitted which does not comply with these terms.
*Correspondence: Isabelle Vernos, aXNhYmVsbGUudmVybm9zQGNyZy5ldQ==
Disclaimer: All claims expressed in this article are solely those of the authors and do not necessarily represent those of their affiliated organizations, or those of the publisher, the editors and the reviewers. Any product that may be evaluated in this article or claim that may be made by its manufacturer is not guaranteed or endorsed by the publisher.
Research integrity at Frontiers
Learn more about the work of our research integrity team to safeguard the quality of each article we publish.