- Department of Neurology, Paul and Sheila Wellstone Muscular Dystrophy Center, Stem Cell Institute, University of Minnesota Medical School, Minneapolis, MN, USA
Adult skeletal muscle possesses extraordinary regeneration capacities. After muscle injury or exercise, large numbers of newly formed muscle fibers are generated within a week as a result of expansion and differentiation of a self-renewing pool of muscle stem cells termed muscle satellite cells. Normally, satellite cells are mitotically quiescent and reside beneath the basal lamina of muscle fibers. Upon regeneration, satellite cells are activated, and give rise to daughter myogenic precursor cells. After several rounds of proliferation, these myogenic precursor cells contribute to the formation of new muscle fibers. During cell division, a minor population of myogenic precursor cells returns to quiescent satellite cells as a self-renewal process. Currently, accumulating evidence has revealed the essential roles of satellite cells in muscle regeneration and the regulatory mechanisms, while it still remains to be elucidated how satellite cell self-renewal is molecularly regulated and how satellite cells are important in aging and diseased muscle. The number of satellite cells is decreased due to the changing niche during ageing, resulting in attenuation of muscle regeneration capacity. Additionally, in Duchenne muscular dystrophy (DMD) patients, the loss of satellite cell regenerative capacity and decreased satellite cell number due to continuous needs for satellite cells lead to progressive muscle weakness with chronic degeneration. Thus, it is necessary to replenish muscle satellite cells continuously. This review outlines recent findings regarding satellite cell heterogeneity, asymmetric division and molecular mechanisms in satellite cell self-renewal which is crucial for maintenance of satellite cells as a muscle stem cell pool throughout life. In addition, we discuss roles in the stem cell niche for satellite cell maintenance, as well as related cell therapies for approaching treatment of DMD.
Introduction
Skeletal muscle is the most abundant tissue in the mammalian body accounting for approximately 40% of body weight, and is composed of multinucleated fibers that contract to generate force and movement. In addition, skeletal muscle possesses a remarkable ability to regenerate, and can go through rapid repair following severe damage caused by exercise, toxins or diseases. Muscle satellite cells were discovered by Alexander Mauro in 1961 (Mauro, 1961), and the research about satellite cells has been stimulated to clarify the regulatory mechanisms of muscle regeneration. This has contributed to the development of therapeutic approaches for several muscular diseases including Duchenne muscular dystrophy (DMD).
Satellite cells comprise 30–35% of all muscle fiber nuclei in postnatal mouse muscle, whereas the number decreases to 2.5–6% in adult muscles (Schultz, 1974; Hawke and Garry, 2001). Based on electron microscopic analysis, satellite cells are located beneath the basal lamina and adjacent to the plasma membrane of muscle fibers, and are in a mitotically quiescent state (Mauro, 1961). Upon muscle injury, satellite cells are activated, driven out of their quiescent states, and start to proliferate. Proliferating satellite cells, termed myogenic precursor cells or myoblasts, then stop their proliferation, undergo differentiation into myocytes, and fuse either with each other or existing myofibers in order to repair injured muscle (Charge and Rudnicki, 2004). While forming myotubes, a minor fraction of satellite cells generate themselves or self-renew, and eventually return to a quiescent state as satellite cells under normal physical conditions (Collins et al., 2005). This capacity, that is the ability to maintain the number of satellite cells ready to participate in repetitive muscle regeneration, is an essential characteristic of these cells. Discovery of satellite cells, together with recent advances in techniques of molecular biology, cell biology, and genetics, have significantly contributed to clarification of the molecular and cellular mechanisms of skeletal muscle regeneration. Here we present an overview of current knowledge of muscle regeneration with a focus on satellite cells, along with a summary of the molecular processes governing satellite cell self-renewal.
Muscle Satellite Cells
Satellite cells are characterized by the expression of the paired type homeobox transcription factor, Pax7, which was identified as the first quantifiable marker for satellite cells in both the quiescent and activated states and essential for satellite cell development and survival (Seale et al., 2000; Oustanina et al., 2004; Kuang et al., 2006; Lepper et al., 2009). Pax7 and the closely related Pax3, which is also expressed in quiescent satellite cells in some muscles including diaphragm (Montarras et al., 2005; Relaix et al., 2006; Hirai et al., 2010), play key roles in maintaining the proliferation of progenitors and preventing early myogenic differentiation and apoptotic cell death. The myogenic basic helix-loop-helix (bHLH) transcription factor, myogenic factors consisting of MyoD, Myf5, MRF4 and myogenin, play essential roles in myogenic specification, differentiation, and maintenance during muscle development and regeneration (Tapscott, 2005). MyoD specifically serves as a potent myogenic master transcription factor that can reprogram many non-muscle cell types to a myogenic lineage when expressed in those cells (Weintraub et al., 1989). In adult skeletal muscle, Myf5 is detected in the majority of quiescent satellite cells (Cornelison and Wold, 1997). However, Myf5 mRNA, together with microRNA-31 which suppresses Myf5 translation, is sequestered in mRNP granules present in the quiescent satellite cells, and thus protein translation of Myf5 is suspended (Crist et al., 2012). In quiescent satellite cells, both MyoD mRNA and protein are not detected (Cornelison et al., 2000). Upon muscle injury, satellite cells initiate the myogenic program by the expression of MyoD, withdraw from their quiescent state, and enter into the cell cycle as activated satellite cells. These proliferating activated satellite cells are termed myogenic precursor cells or myoblasts. During satellite cell activation, mRNP granules are dissociated, relative levels of miR-31 are reduced, and Myf5 protein accumulates, which causes the initiation of the myogenic program in satellite cells (Crist et al., 2012). Additionally, during muscle regeneration, satellite cells can be distinguished based on Pax7 and MyoD protein expression. Pax7(+)MyoD(−) cells are in a quiescent state, Pax7(+)MyoD(+) cells are in a proliferating state, and Pax7(−)MyoD(+) cells are undergoing myogenic differentiation followed by cell fusion to generated multinucleated myofibers (Olguin and Olwin, 2004; Zammit et al., 2004). In addition to cell proliferation and myofiber differentiation, a minor fraction of activated satellite cells give rise to Pax7(+)MyoD(−) non-dividing mononucleated cells, which revert to a quiescent state as a quiescent satellite cell (referred to as reserve cells for in vitro) (Baroffio et al., 1996; Yoshida et al., 1998). This is an important function in maintaining their own satellite cell reserve pool through self-renewal, which is essential for continuous muscle regeneration throughout the life of an animal. Collins et al. have first identified that satellite cells can generate a large number of satellite cells and myonuclei after transplantation of single myofibers into mouse regenerating muscle, and that satellite cells are self-sufficient as a source of myogenic cells (Collins et al., 2005). These observations clearly demonstrated that satellite cells function as true muscle stem cells.
Satellite Cell Heterogeneity
Since most satellite cells are expressing Pax7, these cells were likely considered to be a homogeneous population as muscle progenitor cells. However, the accumulating evidence through gene expression profiling and cell surface marker analysis has revealed that satellite cells are a heterogeneous population (Figure 1). In addition to Pax7, large numbers of satellite cells also express Myf5 (Cornelison and Wold, 1997), M-cadherin (Irintchev et al., 1994), α7-integrin (Gnocchi et al., 2009), CD34 (Beauchamp et al., 2000), Syndecan-3/4 (Cornelison et al., 2001) or calcitonin receptor (Fukada et al., 2007). According to a recent report from Beaucamp et al. a subpopulation of satellite cells do not express Myf5, CD34 or M-cadherin (Beauchamp et al., 2000). Additionally, recent studies using Myf5-nLacZ mice, in which the nLacZ gene is inserted into the Myf5 locus, and thus expression of nLacZ recapitulates endogenous Myf5 mRNA expression, revealed that approximately 10% of quiescent satellite cells are LacZ(−), indicating the heterogeneity of quiescent satellite cells (Kuang et al., 2007). To support this, RT-PCR based gene expression studies in single satellite cells demonstrated that a part of Pax7(+) satellite cells express Pax3 and/or MyoD (Sacco et al., 2008).
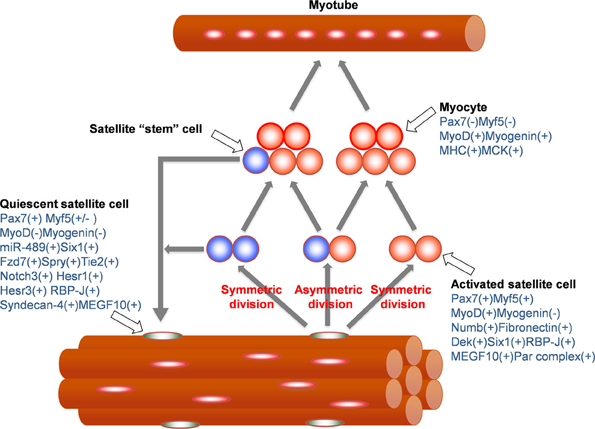
Figure 1. Molecular markers for quiescent satellite cells, activated satellite cells, and myocytes. Quiescent satellite cells are activated by signals from muscle injury and start cell division which include symmetric and asymmetric divisions to produce activated satellite cells and self-renewing satellite cell-stem cells. After several round of cell division, activated satellite cells (myogenic precursor cells or myoblasts) exit their cell cycles and give rise to myocytes which fuse each other to form multinucleated myotubes. Markers expressed in each cell types are summarized (blue letters).
The characteristics of satellite cells are also distinguished depending on muscle types with the distinct differences of gene expression and cell behavior in vitro and in vivo: gene expression profiling experiments have revealed the different characteristics between satellite cells from head muscles and other muscles (Harel et al., 2009), or extraocular muscle and pharyngeal muscle (Sambasivan et al., 2009). Ono et al. have also reported the gene expression differences as well as the distinct stem cell abilities in satellite cells from extensor digitorum longus (EDL) and masseter muscle (Ono et al., 2010). These observations clearly indicated that satellite cells from different muscle types are not only genetically but also functionally heterogeneous populations, and that their different characteristics of satellite cells from different muscle types might be governed by their developmental origins, environments, and niches.
In order to evaluate functional heterogeneity of satellite cells, several recent studies have demonstrated multiple transplantation experiments. Collins et al. elegantly performed an experiment wherein single myofibers with satellite cells were isolated from mouse muscle, and then transplanted into radiation-ablated muscles of mdx/nude mice (Collins et al., 2005). A single myofiber with a few satellite cells gave rise to a large number of myofibers as well as self-renewed satellite cells. In addition, the number of myofibers generated by tibialis anterior (TA) muscle was significantly less than those formed from EDL or soleus (SOL) muscle (Collins et al., 2005). These observations strongly suggest that satellite cells possess intrinsically different properties depending on the origin of muscle. Sacco et al. conducted the experiments that quiescent satellite cells [CD45(−) CD11b(−) CD31(−) Sca1(−) α7-integrin(+) CD34(+) cells] were isolated from adult muscle by FACS, and single quiescent satellite cells were transplanted into irradiated muscle. As a result, they found that a single satellite cell has a remarkable ability of proliferation and differentiation, and further revealed that some transplanted satellite cells generated Pax7-expressing satellite cells after engraftment (Sacco et al., 2008). These findings are strong evidence that satellite cell populations are heterogeneous and exhibit high potency of self-renewal in vivo.
Increasing evidence has revealed the exhibition of “stemness” potential in satellite cells described above, while several studies have proposed that satellite cells are composed of two different populations: one population consists of myogenic satellite cells, which possess myogenic differentiation potential. The other population is an undifferentiated subgroup, which retains the satellite stem cell profile (Collins et al., 2005; Kuang et al., 2007; Ono et al., 2010). Previous studies proposed that a small subset of satellite cells maintain an undifferentiated subpopulation and remain in a quiescent state during myogenic progression (Yoshida et al., 1998). Additionally, the majority of satellite cells are a highly proliferative, whereas the remaining number of satellite cells divide slowly (Schultz, 1996; Shinin et al., 2006). These observations suggest that the slow-dividing minor population exhibits the high potency to self-renew whereas the fast-proliferating cell population tends to undergo myogenic differentiation. This hypothesis was supported by a recent study in which satellite cells could be distinguished as fast and slow-dividing cells through labeling with fluorescent lipophilic dye (PKH26) (Ono et al., 2012). Fast dividing cells gave rise to a higher number of myogenic differentiated cells while slow dividing cells represented quiescent-like self-renewing cells (Ono et al., 2012). In addition, transplantation experiments demonstrate that slow dividing cells possess stem cell like potential, and contribute to continuous muscle regeneration in vivo, suggesting that slow dividing myogenic cells are able to produce myogenic stem cell progeny (Ono et al., 2012). In this study, gene expression analysis in slow dividing cells demonstrated some important clues in distinguishing the “stem cell” population from the myogenic population. As a next step, the identification and isolation of slow-dividing “stem cell” like populations will be required.
Muscle satellite cells mainly contribute to muscle regeneration while several studies have revealed that non-satellite cells, such as bone marrow derived stem cells (Dreyfus et al., 2004; Dezawa et al., 2005), side population (SP) cells (Gussoni et al., 1999; Asakura et al., 2002), mesoangioblasts/pericytes (Dellavalle et al., 2007) and CD133+ cells (Torrente et al., 2004), are able to contribute in myotube formation as well as satellite cell reconstitution during muscle regeneration. These cells do not initially express Pax7. However, once they enter in the satellite cell niche, they can fuse with other myogenic cells to form muscle fibers. In addition, these cells are also able to differentiate into Pax7-expressing cells, indicating that non-satellite cells also form muscle satellite cells. These phenomena also may attribute to muscle satellite cell heterogeneity.
Satellite Cell Self-Renewal and the Molecular Mechanisms
Satellite cell differentiation is essential to provide newly formed myofibers while satellite cell self-renewal is also essential to replenish the satellite cell pool. Maintenance of this balance between satellite cell differentiation and self-renewal is required for muscle homeostasis. A defect in self-renewal ability leads to a decrease in satellite cell number, resulting in depletion of the satellite cell pool as well as in reduced muscle regeneration capacity. The mechanisms regulating satellite cell self-renewal are exhibited in two different manners of cell division; one is by asymmetric division, in which a major population of cells generates daughter cells committed to myogenic differentiation whereas small population cells give rise to self-renewing daughter cells. The other is through symmetric division, in which one stem cell population generates two identical functional “stem” daughter cells. Current studies have carefully demonstrated the self-renewal processes for satellite cells by asymmetric and/or symmetric cell division, and proposed the mechanism of self-renewal that is governed by gene expression, niche, and microenvironment.
It is well described that the myogenic determination factors Myf5, MyoD, MRF4, and Myogenin, play essential roles in myogenic specification, differentiation and maintenance during muscle development and regeneration, and their expression largely contributes to the determination of myogenic cell fate (Weintraub et al., 1991). In addition, Pax3 and Pax7 also play essential roles in myogenic specification, differentiation, and maintenance during muscle development and regeneration as an upstream transcription factor of myogenic determination factors (Lagha et al., 2008). Genetic ablation experiments demonstrated that Pax7 gene knockout (KO) mice display reduced significant reduction in satellite cell number, resulting in the failure of muscle growth and neonatal lethality of most Pax7 KO mice (Seale et al., 2000; Oustanina et al., 2004; Kuang et al., 2006). Following a cardiotoxin-induced skeletal muscle injury, the Pax7 KO mutant displayed significantly reduced muscle regeneration capacity. These results strongly indicate that Pax7 is essential for normal skeletal muscle growth and regeneration through the maintenance and regulation of muscle satellite cells (Oustanina et al., 2004; Kuang et al., 2006). Spontaneous Pax3-mutant (Splotch) mice display neural tube and cardiac chamber malformations, missing limb muscles which originate from migratory myogenic cells from somites, and die embryonically by day E14 (Borycki et al., 1999). In order to elucidate the functional role of Pax3 and Pax7 during muscle regeneration, inducible transgenic mouse systems to conditionally remove Pax3 and Pax7 in development and muscle regeneration have been generated (Lepper et al., 2009). Interestingly, Pax3/Pax7 conditional double-mutant mice have demonstrated that Pax7 is only essential for satellite cell maintenance in juvenile mice, while adult satellite cells do not require either Pax3 or Pax7 for muscle regeneration (Lepper et al., 2009). However, a more recent publications from several groups demonstrated that continuous inactivation of Pax7 induces cell cycle arrest, myogenic differentiation, and impairment of muscle regeneration in vivo, concluding that Pax7 is an essential factor for satellite cell maintenance in adult muscle (Murphy et al., 2011; Sambasivan et al., 2011; von Maltzahn et al., 2013).
Current studies have elucidated which genes essentially regulate muscle satellite cell self-renewal. Interestingly, heterozygous Myf5 gene KO (Myf5+/−) satellite cells demonstrated the equivalent regenerative potency compared to wild-type satellite cells (Gayraud-Morel et al., 2012). However, Myf5+/−satellite cells exhibited high potency of self-renewal capacity compared to wild-type cells, while Pax7 heterozygous mutant satellite cells did not show any differences for self-renewal capacity compared to wild-type cells (Gayraud-Morel et al., 2012). Taken together, these data suggest that Myf5 is one of the key modulators of satellite cell self-renewal (Gayraud-Morel et al., 2012). As previously described, quiescent satellite cells [Pax7(+) MyoD(−)] rapidly initiate to express MyoD soon after satellite cell activation in vitro (Zammit et al., 2004). Most Pax7(+)MyoD(+) activated satellite cells or myoblasts undergo Pax7(−)MyoD(+)Myogenin(+) myocyte differentiation, whereas a subset of Pax7(+)MyoD(+) myoblasts down-regulate MyoD expression and return into Pax7(+)MyoD(−) reserve cells, which are in a quiescent state and are considered to be an equivalent cell population to quiescent satellite cells (Yoshida et al., 1998; Zammit et al., 2004). These cells have the potential to re-enter the cell cycle under growth conditions and can eventually give rise to differentiating myocytes and self-renewing reserve cells (Kitzmann et al., 1998). Therefore, the subset's reserve cells and quiescent satellite cells are currently considered satellite stem cells, and down-regulation of MyoD is essential for the self-renewal process. However, it has not been fully elucidated how reserve cells are generated from myoblast cultures. Recently, we have reported that engraftment and survival efficiency of myoblasts isolated from adult mice lacking the MyoD gene (MyoD−/−) was significantly increased compared to wild-type myoblasts following intramuscular injection into regenerating mouse muscle (Asakura et al., 2007). In addition, MyoD−/− muscle contains an increased number of satellite cells (Megeney et al., 1996; Cornelison et al., 2000; Hirai et al., 2010), suggesting that transplanted MyoD−/− myoblasts, but not wild-type myoblasts, could give rise to the satellite cell compartment in muscle. Importantly, the MyoD−/− myoblasts were much more resistant to apoptosis compared to wild-type myoblasts (Hirai et al., 2010). Therefore, MyoD−/− wild-type myoblasts preserve stem cell characteristics including resistance to apoptosis, efficient engraftment and contribution to the satellite cell compartment following transplantation. Taken together, these observations strongly suggested that down-regulation of MyoD expression is a key event for the satellite cell self-renewal process, and identification of genes and factors that repress MyoD expression may be important for the clarification of satellite cell pool maintenance mechanisms. Importantly, this anti-apoptotic property of MyoD−/− myoblasts is mediated by up-regulation of Pax3. Pax3 works as an anti-apoptotic factor in myoblasts in stress-induced environments and during satellite cell activation and myogenic differentiation by transcriptional activation of anti-apoptotic genes, Bcl-2 and Bcl-xl. During satellite cell activation, expression of MyoD and MyoD-induced microRNA-1 (miR-1) and -206 (miR-206) are up-regulated, while Pax3 is down-regulated by miR-1 and miR-206 via direct binding of these miRNAs to the 3' untranslated region (UTR) of Pax3. This Pax3 down-regulation in activated satellite cells is also mediated by miR-27b (Crist et al., 2009). Interestingly, Pax3 transcripts are subject to alternative polyadenylation, resulting in multiple transcripts. One transcript contains a shorter 3'UTR that has a miR-27b binding site but lacks miR-1 and miR-206 binding sites. Another transcript contains a longer 3'UTR that contains miR-1 and miR-206 binding sites but lacks miR-27b binding site (Crist et al., 2009). These data may reflect a general role of alternative polyadenylation in miRNA-mediated gene regulation in stem cell function.
Recent evidence has revealed that satellite cells initiate to self-renew after an initial cell division during muscle regeneration, and that the mechanism of asymmetric cell division is regulated by the p38 α/β MAP Kinase (MAPK) pathway (Troy et al., 2012). This pathway is, as previously reported, activated in response to muscle injury to induce MyoD expression, resulting in the activation of satellite cells that then undergo myogenic differentiation (Jones et al., 2005). During myoblast proliferation, one daughter cell asymmetrically expressing Par complex, including Par-3 and Protein Kinase-Cλ (PKCλ), activated the p38 α/β MAPK signaling leading to MyoD expression, and promoted myoblast proliferation. While other daughter cells absent for Par complex did not express MyoD and generated self-renewed quiescent satellite cells (Jones et al., 2005). Moreover, it was revealed that PKCλ is required for MyoD-dependent myogenic progression, and that Par-3 knockdown (KD) promoted the generation of satellite cell self-renewal, suggesting that regulation of p38 α/β MAPK mediated by Par complex is deeply-involved in asymmetric cell division and choice between self-renewal and myogenic differentiation (Jones et al., 2005).
Additionally, recent studies have presented the several mechanisms of myoblast self-renewal. MiR-489, which is highly expressed in quiescent satellite cells and decreased in activated satellite cells, functions as the regulator of the satellite cell fate that leads back to the quiescent state by suppressing the expression of the oncogene Dek (Cheung et al., 2012). In quiescent satellite cells, miR-489 is high but Dek is absent. By contrast, down-regulation of miR-489 induces the activation of satellite cells through the up-regulation of Dek expression. These data suggest that the miR-489-Dek pathway is important for maintenance of quiescent satellite cells. Sprouty-1 (Spry1), a receptor tyrosine kinase signaling inhibitor, is essential for maintenance of the quiescent satellite cell pool (Shea et al., 2010). Spry1 is expressed in quiescent satellite cells in uninjured muscle that results in inhibition of FGF signaling to protect satellite cells from activation. Spry1 expression decreased in proliferating myoblasts after injury. Intriguingly, this expression was restored in self-renewed quiescent satellite cells. In addition, the absence of the Spry1 gene has shown a reduction in satellite cell number after muscle regeneration, suggesting that Spoutry-1 is required for the return of myogenic precursor cells to a quiescent state through inhibition of FGF signaling and replenishment of the satellite cell pool during muscle regeneration (Shea et al., 2010). Six1, which belongs to the Six homeoprotein family, is a principal regulator of embryonic myogenesis as an upstream regulator of myogenic factors including Pax3, Myf5, and Myogenin (Giordani et al., 2007; Grifone et al., 2007). Six1 was shown as a regulator of myogenic differentiation as well as satellite cell self-renewal through Dusp6 and ERK1/2 signaling (Le Grand et al., 2009). Six1 is expressed in both quiescent and activated satellite cells (Le Grand et al., 2009). Cell transplantation experiments demonstrated that Six1 regulates myogenic differentiation through up-regulation of MyoD and Myogenin expression. Importantly, the absence of Six1 in satellite cells interfered with muscle regeneration, but led to an increase in the number of satellite cells, indicating that Six1 is required to replenish the satellite cell pool during muscle regeneration. Interestingly, Six1 inhibits extracellular signal-regulated kinase 1/2 (ERK1/2) signaling by up-regulation of Dusp6, an inhibitor of ERK1/2 signaling, which is important for the satellite cell activation process (Le Grand et al., 2009). A previous study presented that angiopoietin-1 (Ang1) and its receptor Tie-2, mediated by ERK1/2 signaling, promotes satellite cell self-renewal (Abou-Khalil et al., 2009), suggesting that the activation of ERK1/2 signaling is essential for satellite cell self-renewal, and that Six1-mediated ERK1/2 signaling inhibition is essential for satellite cell activation. Taken together, the factors that down-regulate MyoD and up-regulate Pax7 could be crucial to guiding satellite cells from an active to a quiescent state. However, it still remains to be elucidated what factors selectively induce asymmetric or symmetric division of myogenic precursor cells.
Satellite Cell Asymmetric Division
Kuang and colleagues have revealed the heterogeneity and the “stem cell” of satellite cells using Myf5-Cre:Rosa26-YFP mice, which can trace Myf5 expression (Kuang et al., 2007). After single muscle fiber isolation from Myf5-Cre:Rosa26-YFP mice, approximately 10% of Pax7(+) quiescent satellite cells were YFP(−) cells, indicating that these YFP(−) quiescent satellite cells have never expressed Myf5. Since Myf5 is one of the myogenic regulatory factors and known to regulate embryonic myogenesis, YFP(−) satellite cells may be less committed cells compared to the YFP(+) population. In addition, the transplantation experiments clearly revealed that Pax7(+)YFP(+) cells preferentially underwent myogenic differentiation while Pax7(+)YFP(−) cells were able to extensively contribute to generate satellite “stem cell” in regenerating muscle (Kuang et al., 2007). Interestingly, Pax7(+)YFP(−) cells were able to asymmetrically divide into both Pax7(+)YFP(−) satellite “stem cells” and Pax7(+)YFP(+) muscle committed progenitor cells (Kuang et al., 2007), while Pax7(+)YFP(+) cells never generated Pax7(+)YFP(−) satellite “stem cells.” Based on this study, Pax7(+)YFP(−) satellite “stem” cells have never expressed the Cre gene inserted in the Myf5 locus, whereas recent studies have revealed that Myf5 or MyoD-expressing myogenic cells also have the potential to develop self-renewable satellite stem cells (Kuang et al., 2007). Inactivation of Pax7 in Myf-5(+) lineage using Myf5Cre/+/Pax7loxP/loxP mice revealed that the majority of adult satellite cells originate from Myf5-expressing myogenic cells, and that the majority of satellite stem cells are replenished from Myf5-expressing cells (Gunther et al., 2013). In addition, Myf5Cre/+/Pax7loxP/loxP/MyoD−/− mice revealed that MyoD(+)/Myf5(−) myogenic progenitor cells also give rise to Pax7-positive satellite cells during development (Gunther et al., 2013). Furthermore, Kanisicak and colleagues have shown that, using MyoDiCre/+/R26R-EYFP lineage tracing system, almost all satellite cells in adult muscle had activated MyoD once during satellite cell development, while MyoD expression is shut down in adult satellite cells (Kanisicak et al., 2009). These results strongly indicate that Myf5 and/or MyoD-expressing myogenic cells are the origin of satellite cells and satellite stem cells. Thus, it is still an open question as to how expression of Myf5 and MyoD are down-regulated in satellite stem cells during satellite cell development and self-renewal.
The idea that a portion of satellite cells are asymmetrically dividing during mitosis has been proven by multiple studies. Numb, an antagonist of the Notch signaling pathway, is asymmetrically localized during satellite cell mitosis; one daughter cell inherited a high level of Numb, and the other daughter cells had little or no Numb. Selectively Numb-expressed cells are capable of self-renewal (Conboy and Rando, 2002; Shinin et al., 2006). In addition, Shinin and colleagues have proposed by pulse chase labeling cells that template “immortal” chromosomes cosegregate into the stem-like daughter cells, while newly synthesized chromosomes are inherited by the differentiated daughter cells during asymmetric divisions of satellite cells (Shinin et al., 2006). This is based on the idea proposed by John Cairn that the oldest DNA strands of each chromosome in some stem cell populations were effectively immortal during mitosis to prevent genome mutation from continuous DNA replication (Cairns, 1975), suggesting that stem cells perform consecutive asymmetric divisions. A recent study has demonstrated that, using Pax7-nGFP mice, Pax7-nGFPHigh muscle stem cells have a low metabolic rate and that only proliferating Pax7-nGFPHigh cells asymmetrically segregate old DNA strands to the renewing stem cells or committed cells, indicating that Pax7-nGFPHigh cells possess a high capacity to self-renew by inheriting old DNA strands (Rocheteau et al., 2012).
In summary, as described here, the accumulating evidence has revealed that satellite cells are a heterogeneous population, while it is still not clear how to separately isolate functional satellite “stem cell” populations and muscle committed satellite cells without using a transgene, and it remains totally unknown whether these “satellite stem cells” are homogeneous or still heterogenous populations. Further studies will be required to progress toward more specific means of identifying and isolating “stem cell” populations within satellite cells such as through the use of FACS with endogenous cell surface markers. Moreover, satellite cells asymmetrically or symmetrically are divided to generate myogenic committed progenitor cells as well as to replenish satellite stem cell pools that contribute to extensive muscle growth and regeneration. Currently, many groups attempt to elucidate the molecular and biological mechanisms of satellite cell self-renewal, and what factors selectively induce asymmetric or symmetric division of satellite cells.
Niches and Satellite Cell Self-Renewal: Satellite Cell-to-Matrix Interactions
The concept of a stem cell niche entails the idea that cell behavior and fate is determined by the microenvironment in which stem cells exist. For example, special niches may maintain stem cells in a quiescent state while, upon injury, the microenvironment surrounding the quiescent stem cells changes, and activates stem cells to induce cell proliferation and differentiation or to promote self-renewal (Ohlstein et al., 2004; Scadden, 2006). Accumulating evidence demonstrates that multiple factors within these specific niches, which include cell-to-cell or cell-to-matrix interactions, autocrine/paracrine signaling as well as biochemical environment, are required to regulate stem cell specification (Ohlstein et al., 2004; Scadden, 2006). Recently several studies have emerged to define the satellite cell niche as well as the underlying mechanisms of how the niche regulates satellite cell self-renewal (Table 1).
Wnt signaling pathways play key roles in the regulation of multiple developmental processes, from embryonic myotome induction, to postnatal myogenic differentiation (Rochat et al., 2004; Gros et al., 2009). In addition, Wnt signaling acts as a protagonist for myogenic specification in muscle resident stem cells (Polesskaya et al., 2003; Brack et al., 2007). Activation of Wnt signaling is normally initiated through binding with the G protein-coupled receptor proteins of the Frizzled (Fzd) family. Activated Wnt signaling branches into two distinct pathways, the canonical Wnt/β-catenin pathway, and the non-canonical Wnt/planar cell polarity (PCP) pathway. The canonical Wnt pathway induces satellite cell proliferation during muscle regeneration (Otto et al., 2008), whereas the non-canonical Wnt pathway induces myocyte growth in the developing myotome (Gros et al., 2009). Recent studies have presented that one of the Wnt receptors, Fzd7, is highly expressed in Pax7(+)Myf5(−) quiescent satellite stem cells, and that Wnt7a is a ligand for Fzd7. Intriguingly, Wnt7a/Fzd7 promoted the symmetric division of a Pax7(+)Myf5(−) satellite stem cell population through the activation of the non-canonical Wnt pathway (Le Grand et al., 2009). Additionally, overexpression of Wnt7 enhanced muscle regeneration with an expansion of satellite stem cell number, while overexpression of Wnt3, which stimulated the canonical Wnt pathway, impaired muscle regeneration. In addition, recent studies have found that Syndecan-4 and Fzd7 form a co-receptor complex for Wnt7a in activated satellite cells, and that binding of the ECM glycoprotein, Fibronectin, to Syndecan-4 promoted Wnt signaling to induce the symmetric expansion of satellite stem cells (Bentzinger et al., 2013). These observations suggest that the activation of the non-canonical Wnt pathway through fibronectin controls the symmetric division of satellite cells and replenishes the satellite cell pool during muscle regeneration.
The Notch signaling pathway is also known to be important for various cell functions, including cell-cell interaction and cell fate determination during tissue development, homeostasis, and regeneration (Lai, 2004). Activation of Notch signaling prevents myoblast differentiation and promote satellite cell self-renewal by repressing MyoD activity through up-regulation of HES proteins, an inhibitor of MyoD (Kuroda et al., 1999). By contrast, attenuation of Notch signaling by Numb, a Notch inhibitor, results in the progression of myogenesis from myoblast proliferation to differentiation (Conboy and Rando, 2002). Additionally, a recent paper has demonstrated that absence of Numb in Pax7-CreER/Numbfl/fl mice results in a satellite cell proliferation defect due to up-regulation of cell cycle inhibitor p21 and Myostatin and display the severe impairment of muscle regeneration, indicating a unique role for Numb in regulating the activation and proliferation of satellite cells (George et al., 2013). In fact, Numb expression was asymmetrically localized in satellite stem cells during satellite cell activation. Thus, the balance between Notch1 and Numb regulates satellite cell proliferation, differentiation, and self-renewal (Conboy and Rando, 2002). In regenerating aged muscle, the disruption of Notch activity extensively impairs muscle regeneration, whereas activation of Notch signaling promotes muscle regeneration (Conboy et al., 2003). Microarray analysis identified that Notch3 is highly expressed in quiescent satellite cells (Fukada et al., 2007), and Notch3-deficient mice presented an increased number of Pax7(+) self-renewing cells (Kitamoto and Hanaoka, 2010). In addition, the absence of Notch signaling in satellite cells of adult mice induces spontaneous activation from the quiescent state to rapid differentiation, resulting in depletion of the satellite cell pool and the failure of muscle regeneration (Bjornson et al., 2012; Mourikis et al., 2012). Moreover, Hesr1 and Hesr3, which are downstream target genes of Notch signaling, are expressed in quiescent satellite cells. The absence of both Hesr1 and Hesr3 genes also leads to the reduction of satellite cell self-renewal and the depletion of the satellite cell pool, leading to the consequent impairment of muscle regeneration (Fukada et al., 2011). A recent paper demonstrated that mice lacking the RBP-J gene, an effector transcription factor of Notch signaling, display the depletion of the satellite cell pool, and this satellite cell depletion is recovered in MyoD−/− background (coRbpj;MyoD−/−) (Brohl et al., 2012). However, in coRbpj;MyoD−/− mice, satellite cells are not located in the normal position, and are instead positioned in the interstitial space of muscle fibers, and do not contribute to myofiber growth (Brohl et al., 2012). Furthermore, microarray analysis revealed that Notch signaling is required to produce sufficient numbers of basement membrane proteins and adhesion molecules including α7-Integrin, Collagen XVIIIa1, Megf10, and M-CAM (Table 1). One of them, MEGF10 was found to be expressed in quiescent and activated satellite cells, and overexpression of MEGF10 stimulates the generation of quiescent satellite cells (Holterman et al., 2007). These observations strongly suggest that Notch signaling contributes to maintenance of the satellite cell pool by repressing MyoD as well as by promoting the homing of satellite cells along with stimulation of basal lamina production.
In normal skeletal muscle, the distributions of satellite cells are anatomically observed close to capillaries, and the paracrine signaling of endothelial cells stimulates muscle satellite cell growth (Christov et al., 2007), suggesting that the maintenance and proliferation of satellite cells may be influenced by the interaction between endothelial cells and satellite cells. A recent study revealed that Ang1/Tie2 signaling, which are the regulatory factors of vascular homeostasis, are involved in the regulation of satellite cell self-renewal (Abou-Khalil et al., 2009). Ang1 and Tie2 expression is high in quiescent satellite cells as well as in reserve cells. In addition, inhibition of Tie2 attenuated the number of quiescent satellite cells, whereas activation of Ang1/Tie2 signaling through the ERK1/2 pathway increased the generation of quiescent satellite cells. In addition, secreted Ang1 from vascular smooth muscle cells or fibroblasts located around satellite cells promotes Pax7 expression (Abou-Khalil et al., 2009). These observations suggest that both autocrine and paracrine Ang-1 mediated Tie2 signaling is required to regulate satellite cell self-renewal. Although it is still not clear whether the direct connection between endothelial cells and satellite cells is critical for niches to maintain the satellite cell pool, it is an attractive hypothesis that stem cell niches are generated by endothelial cells surrounding satellite cells, similar to the other stem cell systems including hematopoietic stem cells and neural stem cells (Gomez-Gaviro et al., 2012).
Since satellite cells are normally located in contact with the plasma membrane and the basal lamina of muscle fibers, the association of satellite cells with muscle fibers is also considered to be an important niche for maintaining the satellite cell pool (Bischoff, 1990). In ageing muscle, the number of satellite cells and the muscle regeneration ability were decreased (Shefer et al., 2006; Collins et al., 2007) due to the changing niches in aging muscles fibers (Conboy et al., 2003; Brack et al., 2007; Carlson et al., 2008), suggesting that the stem cell niche is responsible for the maintenance of quiescent satellite cells. Therefore, the essential factors to maintaining quiescent satellite cells and thus muscle homeostasis may exist in muscle fibers. Recent studies have revealed that increased levels of FGF2, a member of the fibroblasts growth factor family, induced the loss of satellite cell number in aged muscle fibers (Chakkalakal et al., 2012). The FGF family is a well-characterized growth factor, and considered to be a stimulator of satellite cell activation and proliferation (Sheehan and Allen, 1999). This increase in FGF signaling prevents the withdrawal of satellite cells from the cell cycle, resulting in reduced satellite cell numbers. Furthermore, as described above, continuous FGF signaling through the absence of Spry-1, an inhibitor of FGF signaling, has induced the loss of satellite cells, suggesting that FGF signaling through the stem cell niche is crucial to the maintenance of the satellite cell pool during aging (Sheehan and Allen, 1999).
The extracellular matrix (ECM) surrounding muscle cells is also known to undergo extensive remodeling during muscle regeneration. Consequently, excessive composition of interstitial ECM promotes the appearance of connective tissue or fibrosis in muscle under certain conditions such as those seen in DMD or aging muscles. Urciuolo and colleagues have recently demonstrated that an ECM protein, collagen VI, is critical as an extracellular niche protein to maintain the satellite cell pool (Urciuolo et al., 2013). Mice having an absence of collagen VI (Col6a1−/−) showed delayed muscle regeneration and reduced satellite cell self-renewal (Urciuolo et al., 2013). Importantly, transplantation of fibroblasts, isolated from wild-type mice into Col6a1−/− mice, could rescue muscle satellite cell self-renewal; this indicates that a muscle environment consisting of ECM components, including Collagen VI, can modulate satellite cell behavior and that ECMs are critical factors in maintaining the muscle satellite cell pool.
As described above, accumulating evidence has elucidated the signaling network for self-renewal in satellite cells while Gilbert and colleagues provide us new insight that the skeletal muscle microenvironment potentially regulates stem cell fate (Gilbert et al., 2010). They have engineered a hydrogel platform, which can recapitulate muscle physiological elasticity in vitro. Satellite cells cultured on this substrate have shown higher potency of self-renewal than those cultured on traditional plastic dishes. Additionally, myoblasts cultured on hydrogel could promote the efficacy of cell engraftment and the reconstitution of satellite stem cells in vivo, indicating that substrate elasticity is also an important factor of satellite cell self-renewal (Gilbert et al., 2010).
In addition to cell commitment, recent reports indicate that physiological conditions in satellite cells may influence the regulation of self-renewal. Liu and colleagues have proposed that hypoxia does not affect myoblast proliferation but instead promotes satellite cell self-renewal through up-regulating Pax7 (Liu et al., 2012). In fact, hypoxia promotes the activation of Notch signaling, leading to the down-regulation of miRNA-1/206 expression. Since miRNA-1/206 can bind to its target site on the Pax7-3'UTR, decreased levels of both miRNAs through hypoxic conditions can induce Pax7 expression (Liu et al., 2012). In addition, a previous study demonstrated that hypoxic conditions strongly reduce MyoD and myogenin expression during myoblast differentiation without inducing apoptotic cell death (Majmundar et al., 2012), suggesting that the hypoxic condition mediating Notch signaling and microRNAs could be an important factor in regulation of MyoD and Pax7 for the maintenance of the satellite cell pool.
Conclusions
It is widely accepted that satellite cells are essential for postnatal muscle growth and muscle regeneration. Despite high potency of myogenic differentiation in satellite cells, these cells are currently not applicable for cell transplantation therapy against DMD due to severe limitations, such as low cellular survival, incomplete myogenic differentiation, and especially poor satellite cell formation (Tedesco and Cossu, 2012). Alternatively, mesenchymal stem cells including bone marrow derived stem cells were expected to be a new cell source for cell therapy. However, since the transplantation efficacy is quite low, stem cell therapy in muscular dystrophy is still an elusive goal. To accomplish effective cell therapy, injected cells must build and maintain the satellite cell pool with continuous self-renewal. In order to solve these issues, it is essential to understand the mechanisms of muscle regeneration through satellite cells and non-satellite cells.
Recently, accumulating evidence has revealed the satellite cell heterogeneity and the molecular mechanisms of cell fate determination, specifically whether satellite cells differentiate into myocytes or self-renew as stem cells. These studies demonstrated that maintenance of the satellite cell pool is intrinsically and extrinsically regulated by many regulators. Novel knowledge about muscle regeneration through satellite cells may provide us new therapeutic approaches for DMD patients. Additionally, there are multiple unexplained muscular diseases, such as sarcopenia or muscle atrophy. In order to discover ideal therapies for muscular diseases, it is essential to explore fundamental molecular mechanisms of muscle satellite cells using new methodological technologies such as sequencing-mediated global gene regulation.
Author Contributions
Norio Motohashi and Atsushi Asakura wrote the manuscript. Both authors read and approved the final manuscript.
Conflict of Interest Statement
The authors declare that the research was conducted in the absence of any commercial or financial relationships that could be construed as a potential conflict of interest.
Acknowledgments
We thank Alex Hron and Michael Baumrucker for critical reading of the manuscript. This work was supported by grants from National Institute of Arthritis and Musculoskeletal and Skin Diseases (NIAMS) and the Muscular Dystrophy Association (MDA).
Abbreviations
DMD, Duchenne muscular dystrophy; bHLH, Basic-helix-loop-helix; mRNP, Messenger ribonucleoprotein; FACS, Fluorescence-activated cell sorting; UTR, Untranslated region; MAPK, Mitogen-activated Protein Kinase; PKC, Protein kinase C; Spry1, Sprouty homolog 1; FGF, Fibroblast growth factors; Dusp6, Dual specificity phosphatase 6; ERK, Extracellular signal-regulated kinase; Ang1, Angiopoietin-1; Fzd7, Frizzled 7; PCP pathway, planar cell polarity pathway; ECM, Extracellular matrix; M-CAM, Melanoma cell adhesion molecule.
References
Abou-Khalil, R., Le Grand, F., Pallafacchina, G., Valable, S., Authier, F. J., Rudnicki, M. A., et al. (2009). Autocrine and paracrine angiopoietin 1/Tie-2 signaling promotes muscle satellite cell self-renewal. Cell Stem Cell 5, 298–309. doi: 10.1016/j.stem.2009.06.001
Asakura, A., Hirai, H., Kablar, B., Morita, S., Ishibashi, J., Piras, B. A., et al. (2007). Increased survival of muscle stem cells lacking the MyoD gene after transplantation into regenerating skeletal muscle. Proc. Natl. Acad. Sci. U.S.A. 104, 16552–16557. doi: 10.1073/pnas.0708145104
Asakura, A., Seale, P., Girgis-Gabardo, A., and Rudnicki, M. A. (2002). Myogenic specification of side population cells in skeletal muscle. J. Cell Biol. 159, 123–134. doi: 10.1083/jcb.200202092
Baroffio, A., Hamann, M., Bernheim, L., Bochaton-Piallat, M. L., Gabbiani, G., and Bader, C. R. (1996). Identification of self-renewing myoblasts in the progeny of single human muscle satellite cells. Differentiation 60, 47–57. doi: 10.1046/j.1432-0436.1996.6010047.x
Beauchamp, J. R., Heslop, L., Yu, D. S., Tajbakhsh, S., Kelly, R. G., Wernig, A., et al. (2000). Expression of CD34 and Myf5 defines the majority of quiescent adult skeletal muscle satellite cells. J. Cell Biol. 151, 1221–1234. doi: 10.1083/jcb.151.6.1221
Bentzinger, C. F., Wang, Y. X., von Maltzahn, J., Soleimani, V. D., Yin, H., and Rudnicki, M. A. (2013). Fibronectin regulates Wnt7a signaling and satellite cell expansion. Cell Stem Cell 12, 75–87. doi: 10.1016/j.stem.2012.09.015
Bischoff, R. (1990). Interaction between satellite cells and skeletal muscle fibers. Development 109, 943–952.
Bjornson, C. R., Cheung, T. H., Liu, L., Tripathi, P. V., Steeper, K. M., and Rando, T. A. (2012). Notch signaling is necessary to maintain quiescence in adult muscle stem cells. Stem Cells 30, 232–242. doi: 10.1002/stem.773
Borycki, A. G., Li, J., Jin, F., Emerson, C. P., and Epstein, J. A. (1999). Pax3 functions in cell survival and in pax7 regulation. Development 126, 1665–1674.
Brack, A. S., Conboy, M. J., Roy, S., Lee, M., Kuo, C. J., Keller, C., et al. (2007). Increased Wnt signaling during aging alters muscle stem cell fate and increases fibrosis. Science 317, 807–810. doi: 10.1126/science.1144090
Brohl, D., Vasyutina, E., Czajkowski, M. T., Griger, J., Rassek, C., Rahn, H. P., et al. (2012). Colonization of the satellite cell niche by skeletal muscle progenitor cells depends on Notch signals. Dev. Cell 23, 469–481. doi: 10.1016/j.devcel.2012.07.014
Cairns, J. (1975). Mutation selection and the natural history of cancer. Nature 255, 197–200. doi: 10.1038/255197a0
Carlson, M. E., Hsu, M., and Conboy, I. M. (2008). Imbalance between pSmad3 and Notch induces CDK inhibitors in old muscle stem cells. Nature 454, 528–532. doi: 10.1038/nature07034
Chakkalakal, J. V., Jones, K. M., Basson, M. A., and Brack, A. S. (2012). The aged niche disrupts muscle stem cell quiescence. Nature 490, 355–360. doi: 10.1038/nature11438
Charge, S. B., and Rudnicki, M. A. (2004). Cellular and molecular regulation of muscle regeneration. Physiol. Rev. 84, 209–238. doi: 10.1152/physrev.00019.2003
Cheung, T. H., Quach, N. L., Charville, G. W., Liu, L., Park, L., Edalati, A., et al. (2012). Maintenance of muscle stem-cell quiescence by microRNA-489. Nature 482, 524–528. doi: 10.1038/nature10834
Christov, C., Chretien, F., Abou-Khalil, R., Bassez, G., Vallet, G., Authier, F. J., et al. (2007). Muscle satellite cells and endothelial cells: close neighbors and privileged partners. Mol. Biol. Cell 18, 1397–1409. doi: 10.1091/mbc.E06-08-0693
Collins, C. A., Olsen, I., Zammit, P. S., Heslop, L., Petrie, A., Partridge, T. A., et al. (2005). Stem cell function, self-renewal, and behavioral heterogeneity of cells from the adult muscle satellite cell niche. Cell 122, 289–301. doi: 10.1016/j.cell.2005.05.010
Collins, C. A., Zammit, P. S., Ruiz, A. P., Morgan, J. E., and Partridge, T. A. (2007). A population of myogenic stem cells that survives skeletal muscle aging. Stem Cells 25, 885–894. doi: 10.1634/stemcells.2006-0372
Conboy, I. M., Conboy, M. J., Smythe, G. M., and Rando, T. A. (2003). Notch-mediated restoration of regenerative potential to aged muscle. Science 302, 1575–1577. doi: 10.1126/science.1087573
Conboy, I. M., and Rando, T. A. (2002). The regulation of Notch signaling controls satellite cell activation and cell fate determination in postnatal myogenesis. Dev. Cell 3, 397–409. doi: 10.1016/S1534-5807(02)00254-X
Cornelison, D. D., Filla, M. S., Stanley, H. M., Rapraeger, A. C., and Olwin, B. B. (2001). Syndecan-3 and syndecan-4 specifically mark skeletal muscle satellite cells and are implicated in satellite cell maintenance and muscle regeneration. Dev. Biol. 239, 79–94. doi: 10.1006/dbio.2001.0416
Cornelison, D. D., Olwin, B. B., Rudnicki, M. A., and Wold, B. J. (2000). MyoD(-/-) satellite cells in single-fiber culture are differentiation defective and MRF4 deficient. Dev. Biol. 224, 122–137. doi: 10.1006/dbio.2000.9682
Cornelison, D. D., and Wold, B. J. (1997). Single-cell analysis of regulatory gene expression in quiescent and activated mouse skeletal muscle satellite cells. Dev. Biol. 191, 270–283. doi: 10.1006/dbio.1997.8721
Crist, C. G., Montarras, D., and Buckingham, M. (2012). Muscle satellite cells are primed for myogenesis but maintain quiescence with sequestration of Myf5 mRNA targeted by microRNA-31 in mRNP granules. Cell Stem Cell 11, 118–126. doi: 10.1016/j.stem.2012.03.011
Crist, C. G., Montarras, D., Pallafacchina, G., Rocancourt, D., Cumano, A., Conway, S. J., et al. (2009). Muscle stem cell behavior is modified by microRNA-27 regulation of Pax3 expression. Proc. Natl. Acad. Sci. U.S.A. 106, 13383–13387. doi: 10.1073/pnas.0900210106
Dellavalle, A., Sampaolesi, M., Tonlorenzi, R., Tagliafico, E., Sacchetti, B., Perani, L., et al. (2007). Pericytes of human skeletal muscle are myogenic precursors distinct from satellite cells. Nat. Cell Biol. 9, 255–267. doi: 10.1038/ncb1542
Dezawa, M., Ishikawa, H., Itokazu, Y., Yoshihara, T., Hoshino, M., Takeda, S., et al. (2005). Bone marrow stromal cells generate muscle cells and repair muscle degeneration. Science 309, 314–317. doi: 10.1126/science.1110364
Dreyfus, P. A., Chretien, F., Chazaud, B., Kirova, Y., Caramelle, P., Garcia, L., et al. (2004). Adult bone marrow-derived stem cells in muscle connective tissue and satellite cell niches. Am. J. Pathol. 164, 773–779. doi: 10.1016/S0002-9440(10)63165-3
Fukada, S., Uezumi, A., Ikemoto, M., Masuda, S., Segawa, M., Tanimura, N., et al. (2007). Molecular signature of quiescent satellite cells in adult skeletal muscle. Stem Cells 25, 2448–2459. doi: 10.1634/stemcells.2007-0019
Fukada, S., Yamaguchi, M., Kokubo, H., Ogawa, R., Uezumi, A., Yoneda, T., et al. (2011). Hesr1 and Hesr3 are essential to generate undifferentiated quiescent satellite cells and to maintain satellite cell numbers. Development 138, 4609–4619. doi: 10.1242/dev.067165
Gayraud-Morel, B., Chretien, F., Jory, A., Sambasivan, R., Negroni, E., Flamant, P., et al. (2012). Myf5 haploinsufficiency reveals distinct cell fate potentials for adult skeletal muscle stem cells. J. Cell Sci. 125, 1738–1749. doi: 10.1242/jcs.097006
George, R. M., Biressi, S., Beres, B. J., Rogers, E., Mulia, A. K., Allen, R. E., et al. (2013). Numb-deficient satellite cells have regeneration and proliferation defects. Proc. Natl. Acad. Sci. U.S.A. 110, 18549–18554. doi: 10.1073/pnas.1311628110
Gilbert, P. M., Havenstrite, K. L., Magnusson, K. E., Sacco, A., Leonardi, N. A., Kraft, P., et al. (2010). Substrate elasticity regulates skeletal muscle stem cell self-renewal in culture. Science 329, 1078–1081. doi: 10.1126/science.1191035
Giordani, J., Bajard, L., Demignon, J., Daubas, P., Buckingham, M., and Maire, P. (2007). Six proteins regulate the activation of Myf5 expression in embryonic mouse limbs. Proc. Natl. Acad. Sci. U.S.A. 104, 11310–11315. doi: 10.1073/pnas.0611299104
Gnocchi, V. F., White, R. B., Ono, Y., Ellis, J. A., and Zammit, P. S. (2009). Further characterisation of the molecular signature of quiescent and activated mouse muscle satellite cells. PLoS ONE 4:e5205. doi: 10.1371/journal.pone.0005205
Gomez-Gaviro, M. V., Lovell-Badge, R., Fernandez-Aviles, F., and Lara-Pezzi, E. (2012). The vascular stem cell niche. J. Cardiovasc. Transl. Res. 5, 618–630. doi: 10.1007/s12265-012-9371-x
Grifone, R., Demignon, J., Giordani, J., Niro, C., Souil, E., Bertin, F., et al. (2007). Eya1 and Eya2 proteins are required for hypaxial somitic myogenesis in the mouse embryo. Dev. Biol. 302, 602–616. doi: 10.1016/j.ydbio.2006.08.059
Gros, J., Serralbo, O., and Marcelle, C. (2009). WNT11 acts as a directional cue to organize the elongation of early muscle fibres. Nature 457, 589–593. doi: 10.1038/nature07564
Gunther, S., Kim, J., Kostin, S., Lepper, C., Fan, C. M., and Braun, T. (2013). Myf5-Positive Satellite Cells Contribute to Pax7-Dependent Long-Term Maintenance of Adult Muscle Stem Cells. Cell Stem Cell. 13, 590–601. doi: 10.1016/j.stem.2013.07.016
Gussoni, E., Soneoka, Y., Strickland, C. D., Buzney, E. A., Khan, M. K., Flint, A. F., et al. (1999). Dystrophin expression in the mdx mouse restored by stem cell transplantation. Nature 401, 390–394. doi: 10.1038/43919
Harel, I., Nathan, E., Tirosh-Finkel, L., Zigdon, H., Guimaraes-Camboa, N., Evans, S. M., et al. (2009). Distinct origins and genetic programs of head muscle satellite cells. Dev. Cell 16, 822–832. doi: 10.1016/j.devcel.2009.05.007
Hawke, T. J., and Garry, D. J. (2001). Myogenic satellite cells: physiology to molecular biology. J. Appl. Physiol. 91, 534–551.
Hirai, H., Verma, M., Watanabe, S., Tastad, C., Asakura, Y., and Asakura, A. (2010). MyoD regulates apoptosis of myoblasts through microRNA-mediated down-regulation of Pax3. J. Cell Biol. 191, 347–365. doi: 10.1083/jcb.201006025
Holterman, C. E., Le Grand, F., Kuang, S., Seale, P., and Rudnicki, M. A. (2007). Megf10 regulates the progression of the satellite cell myogenic program. J. Cell Biol. 179, 911–922. doi: 10.1083/jcb.200709083
Irintchev, A., Zeschnigk, M., Starzinski-Powitz, A., and Wernig, A. (1994). Expression pattern of M-cadherin in normal, denervated, and regenerating mouse muscles. Dev. Dyn. 199, 326–337. doi: 10.1002/aja.1001990407
Jones, N. C., Tyner, K. J., Nibarger, L., Stanley, H. M., Cornelison, D. D., Fedorov, Y. V., et al. (2005). The p38alpha/beta MAPK functions as a molecular switch to activate the quiescent satellite cell. J. Cell Biol. 169, 105–116. doi: 10.1083/jcb.200408066
Kanisicak, O., Mendez, J. J., Yamamoto, S., Yamamoto, M., and Goldhamer, D. J. (2009). Progenitors of skeletal muscle satellite cells express the muscle determination gene, MyoD. Dev. Biol. 332, 131–141. doi: 10.1016/j.ydbio.2009.05.554
Kitamoto, T., and Hanaoka, K. (2010). Notch3 null mutation in mice causes muscle hyperplasia by repetitive muscle regeneration. Stem Cells 28, 2205–2216. doi: 10.1002/stem.547
Kitzmann, M., Carnac, G., Vandromme, M., Primig, M., Lamb, N. J., and Fernandez, A. (1998). The muscle regulatory factors MyoD and myf-5 undergo distinct cell cycle-specific expression in muscle cells. J. Cell Biol. 142, 1447–1459. doi: 10.1083/jcb.142.6.1447
Kuang, S., Charge, S. B., Seale, P., Huh, M., and Rudnicki, M. A. (2006). Distinct roles for Pax7 and Pax3 in adult regenerative myogenesis. J. Cell Biol. 172, 103–113. doi: 10.1083/jcb.200508001
Kuang, S., Kuroda, K., Le Grand, F., and Rudnicki, M. A. (2007). Asymmetric self-renewal and commitment of satellite stem cells in muscle. Cell 129, 999–1010. doi: 10.1016/j.cell.2007.03.044
Kuroda, K., Tani, S., Tamura, K., Minoguchi, S., Kurooka, H., and Honjo, T. (1999). Delta-induced Notch signaling mediated by RBP-J inhibits MyoD expression and myogenesis. J. Biol. Chem. 274, 7238–7244. doi: 10.1074/jbc.274.11.7238
Lagha, M., Sato, T., Bajard, L., Daubas, P., Esner, M., Montarras, D., et al. (2008). Regulation of skeletal muscle stem cell behavior by Pax3 and Pax7. Cold Spring Harb. Symp. Quant. Biol. 73, 307–315. doi: 10.1101/sqb.2008.73.006
Lai, E. C. (2004). Notch signaling: control of cell communication and cell fate. Development 131, 965–973. doi: 10.1242/dev.01074
Le Grand, F., Jones, A. E., Seale, V., Scimè, A., and Rudnicki, M. A. (2009). Wnt7a activates the planar cell polarity pathway to drive the symmetric expansion of satellite stem cells. Cell Stem Cell 4, 535–547. doi: 10.1016/j.stem.2009.03.013
Lepper, C., Conway, S. J., and Fan, C. M. (2009). Adult satellite cells and embryonic muscle progenitors have distinct genetic requirements. Nature 460, 627–631. doi: 10.1038/nature08209
Liu, W., Wen, Y., Bi, P., Lai, X., Liu, X. S., Liu, X., et al. (2012). Hypoxia promotes satellite cell self-renewal and enhances the efficiency of myoblast transplantation. Development 139, 2857–2865. doi: 10.1242/dev.079665
Majmundar, A. J., Skuli, N., Mesquita, R. C., Kim, M. N., Yodh, A. G., Nguyen-McCarty, M., et al. (2012). O(2) regulates skeletal muscle progenitor differentiation through phosphatidylinositol 3-kinase/AKT signaling. Mol. Cell. Biol. 32, 36–49. doi: 10.1128/MCB.05857-11
Mauro, A. (1961). Satellite cell of skeletal muscle fibers. J. Biophys. Biochem. Cytol. 9, 493–495. doi: 10.1083/jcb.9.2.493
Megeney, L. A., Kablar, B., Garrett, K., Anderson, J. E., and Rudnicki, M. A. (1996). MyoD is required for myogenic stem cell function in adult skeletal muscle. Genes Dev. 10, 1173–1183. doi: 10.1101/gad.10.10.1173
Montarras, D., Morgan, J., Collins, C., Relaix, F., Zaffran, S., Cumano, A., et al. (2005). Direct isolation of satellite cells for skeletal muscle regeneration. Science 309, 2064–2067. doi: 10.1126/science.1114758
Mourikis, P., Sambasivan, R., Castel, D., Rocheteau, P., Bizzarro, V., and Tajbakhsh, S. (2012). A critical requirement for notch signaling in maintenance of the quiescent skeletal muscle stem cell state. Stem Cells 30, 243–252. doi: 10.1002/stem.775
Murphy, M. M., Lawson, J. A., Mathew, S. J., Hutcheson, D. A., and Kardon, G. (2011). Satellite cells, connective tissue fibroblasts and their interactions are crucial for muscle regeneration. Development 138, 3625–3637. doi: 10.1242/dev.064162
Ohlstein, B., Kai, T., Decotto, E., and Spradling, A. (2004). The stem cell niche: theme and variations. Curr. Opin. Cell Biol. 16, 693–699. doi: 10.1016/j.ceb.2004.09.003
Olguin, H. C., and Olwin, B. B. (2004). Pax-7 up-regulation inhibits myogenesis and cell cycle progression in satellite cells: a potential mechanism for self-renewal. Dev. Biol. 275, 375–388. doi: 10.1016/j.ydbio.2004.08.015
Ono, Y., Boldrin, L., Knopp, P., Morgan, J. E., and Zammit, P. S. (2010). Muscle satellite cells are a functionally heterogeneous population in both somite-derived and branchiomeric muscles. Dev. Biol. 337, 29–41. doi: 10.1016/j.ydbio.2009.10.005
Ono, Y., Masuda, S., Nam, H. S., Benezra, R., Miyagoe-Suzuki, Y., and Takeda, S. (2012). Slow-dividing satellite cells retain long-term self-renewal ability in adult muscle. J. Cell Sci. 125, 1309–1317. doi: 10.1242/jcs.096198
Otto, A., Schmidt, C., Luke, G., Allen, S., Valasek, P., Muntoni, F., et al. (2008). Canonical Wnt signalling induces satellite-cell proliferation during adult skeletal muscle regeneration. J. Cell Sci. 121, 2939–2950. doi: 10.1242/jcs.026534
Oustanina, S., Hause, G., and Braun, T. (2004). Pax7 directs postnatal renewal and propagation of myogenic satellite cells but not their specification. EMBO J. 23, 3430–3439. doi: 10.1038/sj.emboj.7600346
Polesskaya, A., Seale, P., and Rudnicki, M. A. (2003). Wnt signaling induces the myogenic specification of resident CD45+ adult stem cells during muscle regeneration. Cell 113, 841–852. doi: 10.1016/S0092-8674(03)00437-9
Relaix, F., Montarras, D., Zaffran, S., Gayraud-Morel, B., Rocancourt, D., Tajbakhsh, S., et al. (2006). Pax3 and Pax7 have distinct and overlapping functions in adult muscle progenitor cells. J. Cell Biol. 172, 91–102. doi: 10.1083/jcb.200508044
Rochat, A., Fernandez, A., Vandromme, M., Moles, J. P., Bouschet, T., Carnac, G., et al. (2004). Insulin and wnt1 pathways cooperate to induce reserve cell activation in differentiation and myotube hypertrophy. Mol. Biol. Cell 15, 4544–4555. doi: 10.1091/mbc.E03-11-0816
Rocheteau, P., Gayraud-Morel, B., Siegl-Cachedenier, I., Blasco, M. A., and Tajbakhsh, S. (2012). A subpopulation of adult skeletal muscle stem cells retains all template DNA strands after cell division. Cell 148, 112–125. doi: 10.1016/j.cell.2011.11.049
Sacco, A., Doyonnas, R., Kraft, P., Vitorovic, S., and Blau, H. M. (2008). Self-renewal and expansion of single transplanted muscle stem cells. Nature 456, 502–506. doi: 10.1038/nature07384
Sambasivan, R., Gayraud-Morel, B., Dumas, G., Cimper, C., Paisant, S., Kelly, R. G., et al. (2009). Distinct regulatory cascades govern extraocular and pharyngeal arch muscle progenitor cell fates. Dev. Cell 16, 810–821. doi: 10.1016/j.devcel.2009.05.008
Sambasivan, R., Yao, R., Kissenpfennig, A., Van Wittenberghe, L., Paldi, A., Gayraud-Morel, B., et al. (2011). Pax7-expressing satellite cells are indispensable for adult skeletal muscle regeneration. Development 138, 3647–3656. doi: 10.1242/dev.067587
Scadden, D. T. (2006). The stem-cell niche as an entity of action. Nature 441, 1075–1079. doi: 10.1038/nature04957
Schultz, E. (1974). A quantitative study of the satellite cell population in postnatal mouse lumbrical muscle. Anat. Rec. 180, 589–595. doi: 10.1002/ar.1091800405
Schultz, E. (1996). Satellite cell proliferative compartments in growing skeletal muscles. Dev. Biol. 175, 84–94. doi: 10.1006/dbio.1996.0097
Seale, P., Sabourin, L. A., Girgis-Gabardo, A., Mansouri, A., Gruss, P., and Rudnicki, M. A. (2000). Pax7 is required for the specification of myogenic satellite cells. Cell 102, 777–786. doi: 10.1016/S0092-8674(00)00066-0
Shea, K. L., Xiang, W., LaPorta, V. S., Licht, J. D., Keller, C., Basson, M. A., et al. (2010). Sprouty1 regulates reversible quiescence of a self-renewing adult muscle stem cell pool during regeneration. Cell Stem Cell 6, 117–129. doi: 10.1016/j.stem.2009.12.015
Sheehan, S. M., and Allen, R. E. (1999). Skeletal muscle satellite cell proliferation in response to members of the fibroblast growth factor family and hepatocyte growth factor. J. Cell. Physiol. 181, 499–506. doi: 10.1002/(SICI)1097-4652(199912)181:3<499::AID-JCP14>3.0.CO;2-1
Shefer, G., Van de Mark, D. P., Richardson, J. B., and Yablonka-Reuveni, Z. (2006). Satellite-cell pool size does matter: defining the myogenic potency of aging skeletal muscle. Dev. Biol. 294, 50–66. doi: 10.1016/j.ydbio.2006.02.022
Shinin, V., Gayraud-Morel, B., Gomes, D., and Tajbakhsh, S. (2006). Asymmetric division and cosegregation of template DNA strands in adult muscle satellite cells. Nat. Cell Biol. 8, 677–687. doi: 10.1038/ncb1425
Tapscott, S. J. (2005). The circuitry of a master switch: mMyod and the regulation of skeletal muscle gene transcription. Development 132, 2685–2695. doi: 10.1242/dev.01874
Tedesco, F. S., and Cossu, G. (2012). Stem cell therapies for muscle disorders. Curr. Opin. Neurol. 25, 597–603. doi: 10.1097/WCO.0b013e328357f288
Torrente, Y., Belicchi, M., Sampaolesi, M., Pisati, F., Meregalli, M., D'Antona, G., et al. (2004). Human circulating AC133(+) stem cells restore dystrophin expression and ameliorate function in dystrophic skeletal muscle. J. Clin. Invest. 114, 182–195. doi: 10.1172/JCI20325
Troy, A., Cadwallader, A. B., Fedorov, Y., Tyner, K., Tanaka, K. K., and Olwin, B. B. (2012). Coordination of satellite cell activation and self-renewal by Par-complex-dependent asymmetric activation of p38alpha/beta MAPK. Cell Stem Cell 11, 541–553. doi: 10.1016/j.stem.2012.05.025
Urciuolo, A., Quarta, M., Morbidoni, V., Gattazzo, F., Molon, S., Grumati, P., et al. (2013). Collagen VI regulates satellite cell self-renewal and muscle regeneration. Nat. Commun. 4, 1964. doi: 10.1038/ncomms2964
von Maltzahn, J., Jones, A. E., Parks, R. J., and Rudnicki, M. A. (2013). Pax7 is critical for the normal function of satellite cells in adult skeletal muscle. Proc. Natl. Acad. Sci. U.S.A. 110, 16474–16479. doi: 10.1073/pnas.1307680110
Weintraub, H., Davis, R., Tapscott, S., Thayer, M., Krause, M., Benezra, R., et al. (1991). The myoD. gene family: nodal point during specification of the muscle cell lineage. Science 251, 761–766. doi: 10.1126/science.1846704
Weintraub, H., Tapscott, S. J., Davis, R. L., Thayer, M. J., Adam, M. A., Lassar, A. B., et al. (1989). Activation of muscle-specific genes in pigment, nerve, fat, liver, and fibroblast cell lines by forced expression of MyoD. Proc. Natl. Acad. Sci. U.S.A. 86, 5434–5438. doi: 10.1073/pnas.86.14.5434
Yoshida, N., Yoshida, S., Koishi, K., Masuda, K., and Nabeshima, Y. (1998). Cell heterogeneity upon myogenic differentiation: down-regulation of MyoD and Myf-5 generates “reserve cells.” J. Cell Sci. 111(Pt 6), 769–779.
Keywords: skeletal muscle, myogenesis, satellite cells, self-renewal, muscle regeneration, MyoD, Pax7, Myf5
Citation: Motohashi N and Asakura A (2014) Muscle satellite cell heterogeneity and self-renewal. Front. Cell Dev. Biol. 2:1. doi: 10.3389/fcell.2014.00001
Received: 18 December 2013; Accepted: 14 January 2014;
Published online: 30 January 2014.
Edited by:
Motohiro Komaki, Tokyo Medical and Dental University Graduate School, JapanReviewed by:
Motohiro Komaki, Tokyo Medical and Dental University Graduate School, JapanGuillaume Grenier, Université de Sherbrooke, Canada
Copyright © 2014 Motohashi and Asakura. This is an open-access article distributed under the terms of the Creative Commons Attribution License (CC BY). The use, distribution or reproduction in other forums is permitted, provided the original author(s) or licensor are credited and that the original publication in this journal is cited, in accordance with accepted academic practice. No use, distribution or reproduction is permitted which does not comply with these terms.
*Correspondence: Atsushi Asakura, Stem Cell Institute, University of Minnesota, McGuire Translational Research Facility, Room 4-220, 2001 6th Street SE, Minneapolis, MN 55455, USA e-mail:YXNha3VyYUB1bW4uZWR1