- 1Laboratory of Molecular Radiology, Center for Disease Biology and Integrative Medicine, Graduate School of Medicine, The University of Tokyo, Tokyo, Japan
- 2Department of Dermatology, Graduate School of Medicine, The University of Tokyo, Tokyo, Japan
- 3Department of Clinical Cannabinoid Research, Graduate School of Medicine, The University of Tokyo, Tokyo, Japan
Hyperthermia is a promising anticancer treatment that induces heat stress, thereby stimulating various signal transduction pathways to maintain cellular homeostasis. Mitogen-activated protein kinases (MAPKs) associate various extracellular stimuli with cytoplasmic and nuclear mediators through a three-tiered cascade of kinases, including MAPKs, MAP2Ks, and MAP3Ks. In mammals, three major groups of MAPKs have been characterized: extracellular signal-regulated protein kinases (ERK1/2), p38 MAPKs (α, β, γ, and δ), and c-Jun NH2-terminal kinases (JNK1/2/3). Each group of MAPKs is activated by heat and exhibits distinct biological functions. Recent studies have indicated that in hyperthermia, MAPK signaling pathways regulate cell survival and death in unique ways. This review offers a concise overview of the MAPK signaling pathway, specifically ERK and JNK, focusing on their relevance in cancer, interplay with heat shock proteins or phosphatases, and current understanding of the MAPK signaling pathway in hyperthermia.
1 Introduction
Hyperthermia is a treatment that raises the temperature of lesions with electromagnetic waves from outside the body and often used in combination with radiotherapy or chemotherapy. In most hyperthermia treatments, the temperature is maintained over 42.5°C in order to kill cancer cells. It increases cell temperature and induces various biochemical changes, such as reactive oxygen species (ROS) generation, an increase in intracellular calcium ion concentration, and protein degradation (Roti, 2008; Hou et al., 2014). Hyperthermia-induced protein denaturation, aggregation, and degradation significantly disrupt cellular homeostasis (Ahmed et al., 2020). Cellular proteins begin to denature above 42.5°C. The mitogen-activated protein kinase (MAPK) signal transduction pathway is conserved in eukaryotic cells (Chang and Karin, 2001). MAPK signaling pathways play a crucial role in the response of cells to various extracellular stimuli, affecting cell proliferation, differentiation, survival, and death (Lavoie et al., 2020; Zeke et al., 2016; Mlakar et al., 2021). Although studies have reported heat-induced activation of the MAPK signaling pathways (Ng and Bogoyevitch, 2000; Jin et al., 2018; Liu et al., 2022), the regulatory mechanisms involved in MAPK signaling remain unclear. This review aims to explore the contribution of MAPK signal transduction to hyperthermic cell death.
2 MAPK signaling
MAPK signaling pathways mediate cellular responses to various extracellular stimuli, including growth factors and environmental stresses (Chang and Karin, 2001). The MAPK signaling pathway consists of a three-kinase cascade, which includes MAPKs (extracellular signal-regulated kinase (ERK), p38, and c-Jun NH2-terminal kinases (JNK), MAPK kinases (MAP2Ks; mitogen-activated ERK kinase (MEK) and MAP kinase kinase (MKK)), and MAPKK kinases (MAP3Ks; e.g., rapidly accelerated fibrosarcoma (RAF), mitogen-activated ERK kinase kinase (MEKK), transforming growth factor-β-activated kinase 1 (TAK1), apoptosis signal-regulating kinase 1 (ASK1), and mixed lineage kinase (MLK). MAP3Ks phosphorylate and activate MAP2Ks, thereby phosphorylating MAPKs. Biochemical and genetic analyses indicate that MAP3Ks associate various extracellular stimuli with cytoplasmic and nuclear effectors by activating downstream MAPK signaling pathways (Peterson et al., 2022). Twenty-one MAP3Ks have been identified to activate the known MAP2Ks. Upstream of the MAP3Ks, the signaling molecules involve kinases, adaptors, and receptors, which connect the pathway to specific stimuli (Chang and Karin, 2001) (Figure 1A).
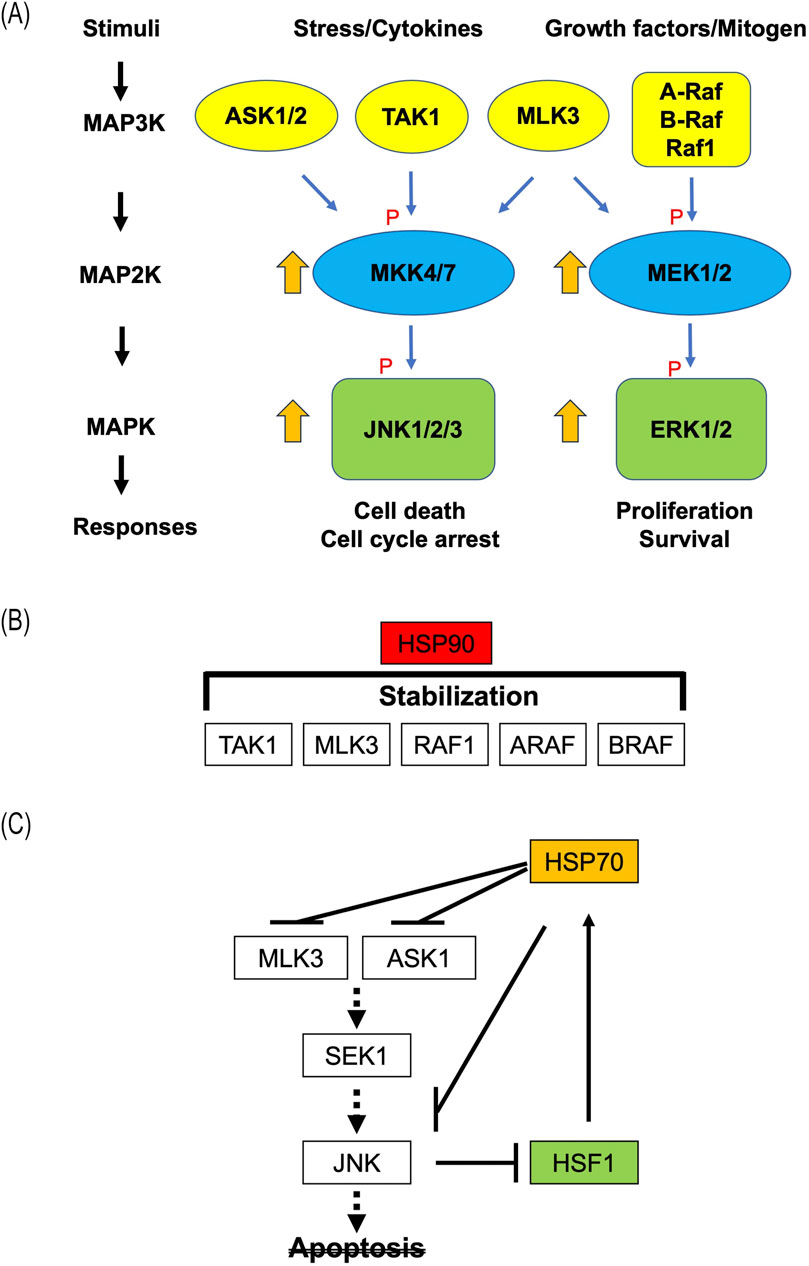
Figure 1. (A) Schematic representation of JNK and ERK pathways in MAPK signaling. Upon external stimulation, the three-tiered kinase modules comprising MAP3Ks, MAP2Ks, and MAPKs are activated through sequential protein phosphorylation. The majority of JNK and ERK signaling pathways are activated by the mitogen-activated protein-3 kinase (MAP3K) members, such as rapidly accelerated fibrosarcoma 1 (RAF1), apoptosis signal-regulating kinase 1 (ASK1), mixed lineage kinase 3 (MLK3), and transforming growth factor-β-activated kinase 1 (TAK1). The MAP3K cascades are involved in the regulation of cell growth and apoptosis. Multiple MAP3Ks can activate both JNK and ERK pathways. P, phosphorylation. (B) Roles of HSPs in regulation of MAPK signaling pathway. The stability of client proteins, including RAF1, ARAF, BRAF, TAK1 and MLK3, is influenced by HSP90 binding. (C) Modification of the JNK signaling pathways by HSP70. HSP70 prevents SEK1 activation through an interaction with ASK1. Binding of HSP70 to JNK prevents its phosphorylation by SEK1. JNK phosphorylates HSF-1 and suppresses its transcriptional activity. HSP, heat shock protein; HSF, heat shock transcription factor.
RAS is a type of small GTP-binding protein and interacts with the RAF family. At the cell surface, the activation of the RAS-RAF-MEK-ERK1/2 signaling pathway is initiated by ligand binding to receptor tyrosine kinases (Simanshu and Morrison, 2022; Bahar et al., 2023). RAF1 is associated with oncogenesis and plays a crucial role in cell growth and development (Huang et al., 2017; Wang et al., 2023; Tran et al., 2021). RAF phosphorylates and activates MEK1/2, which can then activate ERK1/2 to phosphorylate both cytoplasmic and nuclear targets. The ERK1/2 cascade is primarily activated by growth factors, cytokines, agonists of tyrosine kinase-encoded receptors, and G protein-coupled receptors that induce mitogenesis or differentiation (Lavoie et al., 2020). In contrast, the JNK and p38 cascades are predominantly activated by pro-inflammatory cytokines, endoplasmic reticulum stress, or extracellular stress (Zeke et al., 2016; Mlakar et al., 2021; Chen et al., 2021). The MAP3Ks of the JNK pathway include sterile 20 (Ste20)/Ste11/Ste7 (STE) family kinases, such as ASK1, and tyrosine kinase-like (TLK) family members, such as MLKs. The primary MAP2Ks of the JNK cascade are MKK4/stress-activated protein kinase (SAPK)/ERK kinase (SEK1) or MKK7 (Haeusgen et al., 2011; Park et al., 2019). ASK1 activates two different subgroups, the SEK1-JNK and MKK3/6-p38 pathways, and plays crucial roles in the induction of apoptosis (Hattori et al., 2009; Ryuno et al., 2017; Ogier et al., 2020). TAK1 originally associated with transforming growth factor-β (TGF-β)-mediated MAPK activation, is also activated by various cytokines, such as interleukin-1 (IL-1) and tumor necrosis factor-alpha (TNF-α) (Sakurai, 2012; Xu and Lei, 2021). Subsequently, the activated TAK1 phosphorylates inhibitors of nuclear factor-κB (IκB) kinase (IKK) and MKKs (MKK3/6, MKK4/7), resulting in the activation of nuclear factor kappa B (NF-κB) and JNK (Xu and Lei, 2021). MLK3 plays a role in cancer cell survival, migration, drug resistance, cell death, and tumor immunity (Kumar et al., 2021). Additionally, it induces the activation of the JNK and ERK signaling pathways. Oxidative stress-mediated B-Raf–MEK–ERK pathway activation results in a positive feedback loop, where ROS facilitates the ERK1/2-dependent phosphorylation of MLK3 (Schroyer et al., 2018).
3 MAPK signaling pathway in cancer
MAPK signaling pathways play crucial roles in tumor growth, metastasis, and invasion (Braicu et al., 2019). Specifically, the RAS-RAF-MEK1/2-ERK1/2 pathway has been predominant in cancer research (Tran et al., 2021; Bahar et al., 2023). Mutations in RAS and B-Raf proto-oncogene, serine/threonine kinase (BRAF) that dysregulate the MAPK signaling pathway are predominantly associated with human malignancies. RAS is extremely prevalent, with mutations detected in approximately 30% of all tumors (Fernández-Medarde and Santos, 2011). Although RAS activity varies across various cancer types, the upregulation of RAS activity results in the dysregulation of downstream protein kinase activities. RAF mutations, specifically in BRAF V600E, are frequently observed in melanoma and other cancers (Dillon et al., 2021). RAF1 alterations are observed in 2.3% of The Cancer Genome Atlas (TCGA) PanCancer Atlas samples (Cerami et al., 2012; Gao et al., 2013). Several BRAF inhibitors such as Vemurafenib were approved and showed significant benefits, but ineffectiveness was observed after long-term treatment. The MEK1/2 phosphorylation rates in colon cancer, villous adenoma, and tubular adenoma were 76, 40, and 30%, respectively, whereas in normal colonic mucosal cells, the MEK phosphorylation was merely detectable (Lee et al., 2004). Cobimetinib (GDC-0973) is an effective and highly selective allosteric MEK1/2 inhibitor and showed a good efficacy in BRAF and KRAS mutant cell lines and in BRAFV600E/K-mutant patients (Ribas et al., 2014; Amaral et al., 2016). Increased ERK1/2 activation is evident across various human cancer types, including ovarian, colon, breast, and lung cancer (Timofeev et al., 2024). In addition, the constitutive activation of the RAS/RAF/MAPK pathway contributes to tumorigenesis by inhibiting Caspase-9 through MAPK-dependent phosphorylation (Roh et al., 2014).
TAK1 plays a crucial role in regulating immune responses and cell survival through the NF-κB and JNK signaling pathways (Inokuchi et al., 2010). Because TAK1 downstream molecules (NF-κB and JNK) are involved in cancer cell survival and apoptosis, TAK1 regulates tumor initiation, proliferation, and metastasis either as a promoter or suppressor, based on specific receptors and cell types (Cai et al., 2014). Overexpression and hyperactivation of TAK1 are associated with various cancers (Lin et al., 2015; Mukhopadhyay and Lee, 2020; Teramachi et al., 2021). Inhibition of TAK1 activity resulted in reduced cell proliferation, invasion, and apoptosis induction owing to abrogation of NF-κB activation (Lin et al., 2015; Yang et al., 2017).
ASK1 facilitates ROS-induced and endoplasmic reticulum (ER)-mediated apoptosis (Ryuno et al., 2017). Reduced expression or activity of ASK1 has been observed in various cancers, including HCC and breast cancer (Jiang et al., 2016; Yin et al., 2017). However, increased ASK1 expression and activity facilitate cancer cell motility and proliferation in oral squamous cell carcinoma, ovarian, and pancreatic cancer (Luo et al., 2016; Chen et al., 2024). Recent report demonstrates that the deubiquitinase OTUD1-based aggresomes recruit ASK1 via protein-protein interactions, which in turn stabilize ASK1 and activate the downstream JNK signaling pathway for ovarian cancer stem cell maintenance (Chen et al., 2024).
MLK3 has been implicated in the regulation of tumor cell proliferation, differentiation, migration, invasion, and apoptosis (Ma et al., 2019). MLK3 expression is upregulated in hepatocellular carcinoma, and MLK3 blocking suppresses cancer progression (Ke et al., 2024). MLK3 directly binds to epidermal growth factor receptor kinase substrate 8 (EPS8) and regulates the cellular location of EPS8, suggesting that MLK3 promotes the migration and invasion by remodeling the actin cytoskeleton (Zhu et al., 2021). Knockdown of MLK3 expression reduces the breast cancer cell invasion by reducing MMP expression (MMP–1 and –9) (Rattanasinchai et al., 2017). The MLK3 kinase activity was higher in triple-negative (TNBC) than in hormone receptor-positive human breast tumors, and the knockdown of MLK3 or MLK3 inhibitors, CEP-1347 and URMC-099, attenuated tumorigenesis of TNBC cell line and Patient-Derived xenografts (Nair et al., 2023).
Previously, JNK was conceived as an apoptosis driver for cell death, implying its potential as a tumor suppressor, as demonstrated in breast and pancreatic cancers (Cellurale et al., 2012). However, emerging evidence indicates that JNK has a Janus face that regulates both cell apoptosis and survival (Yan et al., 2024). JNK1 facilitates cell survival, whereas JNK2 mediates apoptosis and death (Arbour et al., 2002; Recio-Boiles et al., 2016). Additionally, JNK facilitates cell survival under adverse conditions, including low oxygen levels, by eliminating dysfunctional cellular components (Tam et al., 2019; Wu et al., 2019). Moreover, JNK-mediated pro-survival autophagy facilitates cancer cell resistance to chemotherapy (Huang et al., 2017). The JNK protein appears to be involved in both tumor promotion and inhibition based on stimuli, cell and tissue type, and isoform.
4 Role of heat shock proteins in regulation of MAPK signaling
Cells respond to heat by induction of heat shock proteins (HSPs) (Singh and Hasday, 2013). HSPs function primarily as molecular chaperones. This chaperone activity is diverse and includes fa-cilitating the conformational maturation of its client proteins and regulating client protein location and translocation within the cell. The primary proteins within the HSP family (Hsp70 and Hsp90) have numerous sets of protein clients and play crucial roles in the regulation of cell protein physiology (Streicher, 2019). In terms of cellular response to heat, previous other reports show a correlation between HSPs expression and activation of MAPKs (Hao et al., 2018; Liu et al., 2022).
HSP90, which is evolutionarily conserved, is anti-apoptotic and plays a crucial role in the folding, stabilization, activation, function, aggregation, and proteolytic degradation of several client oncoproteins (Schopf et al., 2017). Moreover, HSP90 is of considerable interest in the search for new therapeutic cancer targets, since most HSP90 client proteins are oncogenic proteins and protein kinases that regulate cell survival, proliferation, invasion, metastasis, and angiogenesis (Birbo et al., 2021). The clientele includes various MAPK-related kinases, including BRAF, CRAF, TAK1, and MLK3 (Liu et al., 2008; Rattanasinchai and Gallo, 2016; Roberts et al., 2021; García-Alonso et al., 2022) (Figure 1B). Suppression of HSP90 expression can result in the simultaneous co-inhibition of various client proteins, thereby affecting multiple signaling pathways (Antolin et al., 2021). For example, the HSP90 inhibitor 17-AAG suppresses ERK signaling in the majority of cell lines because it targets C-RAF, active BRAF, and most of the mutant forms of BRAF (García-Alonso et al., 2022). Consequently, inhibition of HSP90 antagonizes the characteristic pathological features of cancerous cells, including self-sufficiency in growth signals, non-responsiveness to growth-suppressive signals, evasion of apoptosis, angi-ogenesis, invasiveness, and metastasis (Birbo et al., 2021).
HSP70 is involved in regulating various physiological processes, including stress responses and apoptosis. Protective HSP70 expression was associated with the activation of the MAPK signaling pathway (Zhang et al., 2020). ERK-mediated induction of HSP70 may play a crucial role in the inhibition of apoptosis (Lee et al., 2015). Inhibition of HSP70 enhanced heat-induced JNK activation and cleavage of caspase-3 (Hao et al., 2018). There is a clear correlation between the dependence of apoptosis on the activation of JNK and the suppression of apoptosis through elevated Hsp72 expression (Park et al., 2001). Additionally, Hsp70 has been demonstrated to suppress JNK activation by specifically associating with JNK and preventing SEK1-mediated phosphorylation (Gabai et al., 1998) (Figure 1C). Moreover, HSP72 inhibits ASK1 activity by directly interacting with it (Park et al., 2002). The transcriptional activation of HSP genes is regulated by heat shock factors (HSFs). Post-translational modifications are crucial for complete HSF1 activation. Phosphorylation is the most extensively studied modification, with HSF1 undergoing both stimulatory and inhibitory phosphorylation (Dai, 2018). JNK phosphorylates HSF1 at Ser363, resulting in its inhibition (Dai et al., 2000). Additionally, HSF1 reciprocally prevents JNK activation (Su et al., 2016), indicating mutual suppression of JNK and HSF1.
5 MAPK signaling pathway in hyperthermia
Hyperthermia stimulates the release of inflammatory and growth factors including interleukin (IL)-1β, IL-6, vascular endothelial growth factor (VEGF), and tumor necrosis factor alpha (TNF-α) (Katschinski et al., 1999). These cytokines and growth factors stimulates activation of MAPK activators such as RAS. Hyperthermia activates multiple MAPK signaling pathways, including ERK, p38, and JNK (Ng and Bogoyevitch, 2000; Dorion and Landry, 2002; Jin et al., 2018; Liu et al., 2022). In numerous cell types, ERK1/2 activation facilitates cell survival, including during heat shock. For example, mild heat treatment at 42 °C induced ERK1/2 activation, which was inhibited by the MEK-specific inhibitor PD98059, thereby increasing the loss of cell viability (Ng and Bogoyevitch, 2000). Intense heat stress at or above 43 °C induces the dephosphorylation of ERK1/2 and B-cell lymphoma 2 (Bcl-2) and subsequent apoptosis through superoxide production (Li et al., 2017). In contrast, the JNK and p38 MAPK signaling pathways are often associated with pro-apoptotic effects. Dominant-negative JNK prevents heat-induced cell death and Bcl-2-associated X protein (Bax) translocation to mitochondria (Stankiewicz et al., 2005). SB2035800, an inhibitor of p38, reduces heat-induced ROS accumulation and apoptosis (Li et al., 2018). Hyperthermia reduces the invasion of C6 glioma cells by stimulating TNF-α signaling to activate apoptosis, enhancing p38 MAPK expression, and inhibiting the NF-κB signaling pathway in C6 rat glioma cells (Wang et al., 2012). Under mild hyperthermia, the transient activation of JNK and p38 results in cell differentiation rather than apoptosis (Nagata and Todokoro, 1999).
Our previous reports demonstrated that hyperthermia reduces the expression and kinase activity of multiple MAP3K members, such as RAF1 and MLK3, through repression of gene expression or protein denaturation without reduction of the downstream components in the ERK cascades (Enomoto et al., 2019; Enomoto et al., 2022; Enomoto et al., 2024). Additionally, heat shock failed to stimulate the activation of A-Raf proto-oncogene, serine/threonine kinase (ARAF), BRAF, and RAF1 (Ng and Bogoyevitch, 2000). RAF1 stimulates cell proliferation and facilitates cell survival by inhibiting apoptosis (Huang et al., 2017; Wang et al., 2023). Therefore, hyperthermia-induced degradation of RAF1 and MLK3 may hinder the transmission of proliferative stimuli into activation signals and/or activate downstream targets through phosphorylation, resulting in the inhibition of cell survival and anti-apoptotic signaling, thereby contributing to thermal killing. Previous reports demonstrate that the phosphorylation of ERK1/2 was observed under milder heat shock conditions (42°C), whereas its phosphorylation was suppressed under more severe heat shock (43°C ≦), with a reduction in MAP3K expression (Ng and Bogoyevitch, 2000; Li et al., 2017). Mild hyperthermia enhances NADPH oxidase activity via the ERK pathway followed by HIF-1α activation with subsequent increased VEGF-secretion (Vaupel et al., 2023). During mild hyperthermia, ERK1/2 may be active and involved in cell survival, the inhibition of apoptosis and tumor angiogenesis.
Hyperthermia stimulates the release of cytokines. Thus, during hyperthermia, TAK1 may be active and involved in immune activation through NF-κB (Lin et al., 2015; Takaesu et al., 2003). Moreover, treatment with heat increases ROS generation, leading to activation of a ROS-responsive protein kinase ASK1. However, thermal treatment also induces an increase in intracellular calcium and protein degradation. We demonstrated that hyperthermia reduced the expression and kinase activity of TAK1 and ASK1 in the JNK pathway through calpain- or proteasome-dependent protein degradation (Enomoto et al., 2022; Enomoto et al., 2024). Hyperthermia can attenuate NF-κB activity by decreasing upstream kinase TAK1 activity, leading to the inhibition of cell survival and anti-apoptotic signaling. The degradation of ASK1 may be incapable of transducing apoptotic stimuli into activation signals and/or activating each downstream target through phosphorylation, resulting in the inhibition of JNK signaling. However, the phosphorylation of downstream JNK increased in a temperature-dependent manner, at least up to 43°C (Enomoto et al., 2024). Moreover, heat shock activates JNK independently of upstream kinases, and the exposure of cells to heat shock strongly reduces the dephosphorylation rate of JNK but not p38 (Meriin et al., 1999), indicating its specificity for JNK. A precise balance between the activation and inactivation of the JNK signaling pathway should be strongly regulated for the maintenance of cellular homeostasis. Our recent report demonstrated that dual specificity phosphatase 16 (DUSP16), a JNK phosphatase, was degraded in a proteasome-dependent manner by hyperthermia and that the knockdown of DUSP16 enhanced the heat-induced phosphorylation of ASK, SEK1, and JNK (Enomoto et al., 2024). Another previous report demonstrated that the JNK phosphatase M3/6, namely, DUSP8, is inactivated by heat shock at 45°C (Palacios et al., 2001). DUSPs dephosphorylate both threonine/serine and tyrosine residues of their substrates. A subfamily of DUSPs contains the MAP kinase-binding or kinase-interacting motifs that regulate the magnitude and duration of signal transduction of the MAPK/JNK signaling pathway by dephosphorylating their substrates (Kondoh and Nishida, 2007; Ha et al., 2019). Although DUSP16 can bind to all three MAPKs, it preferentially inactivates JNK1/2, followed by p38α/β MAPK (JNK1/2 >> p38 MAPK > ERK) (Masuda et al., 2001); this indicates that hyperthermia may prevent DUSP16 from dephosphorylating JNK but not either p38 or ERK. A recent report demonstrated that DUSP16 inhibits the activation of JNK, thereby reducing apoptosis and promoting cancer chemoresistance (Low et al., 2021). Some members of the DUSP family also regulate signaling upstream of JNK. DUSP14 directly dephosphorylates TAB1, leading to inhibition of the TAB1–TAK1 complex and sequential inactivation of TAK1 and downstream JNK activity (Yang et al., 2014). Thus, hyperthermia may reduce the ability of DUSP16 to directly dephosphorylate not only JNK but ASK1 and SEK1, leading to enhancement of ASK1–SEK1–JNK signaling and subsequent induction of apoptosis.
Additionally, for heat-induced JNK activation, there are alternatives, such as the involvement of other MAP3Ks, except for the aforementioned kinases. Because the 21 characterized MAP3Ks activate known MAP2Ks (Nguyen et al., 2022), certain heat-resistant MAP3Ks may stimulate MAP2Ks and MAPKs in JNK signaling pathway. In addition, if hyperthermia results in inactivation of the SEK1 phosphatase as protein phosphatases 2A (Avdi et al., 2002), it could lead to JNK activation. During mild hyperthermia, ERK1/2 may be active and involved in cell survival and the inhibition of apoptosis. Hyperthermia, specifically at 43 °C or higher, can disrupt the balance between ERK and JNK activation through decrease of MAP3Ks expression in ERK pathway or a reduction in JNK phosphatase function, thereby resulting in the amplification of JNK signaling and enhanced apoptosis (Figure 2). Thus, cellular homeostasis in hyperthermia may be maintained through regulation or deregulation of MAPK signaling to enhance survival, stimulate immunity, or induce cell death at different temperature.
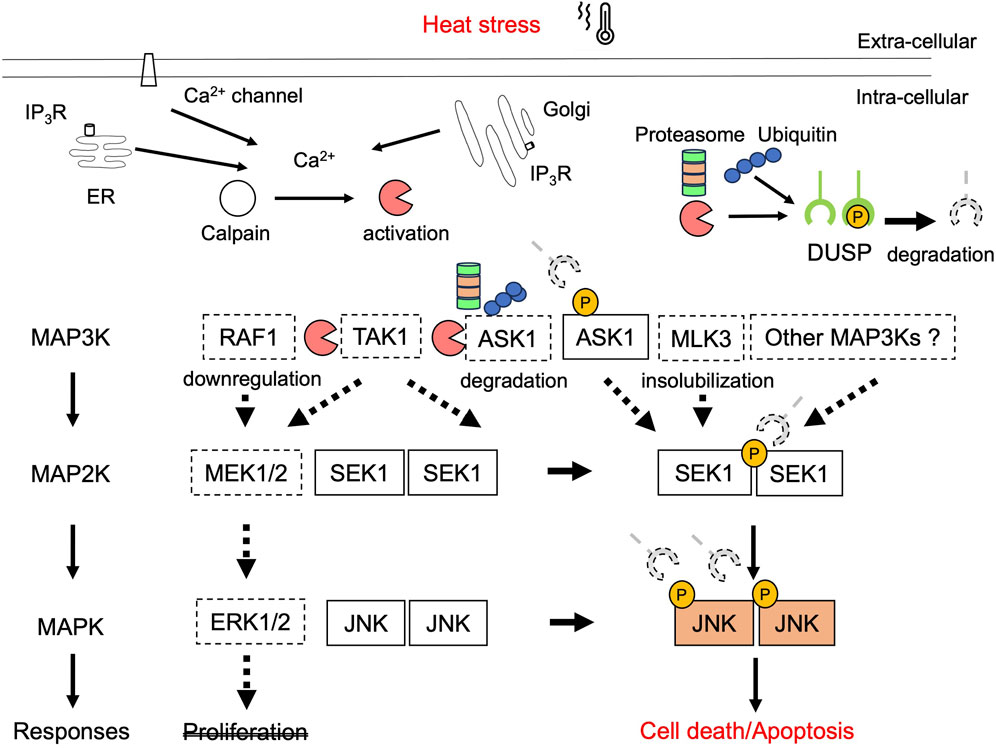
Figure 2. Schematic representation of predominance of JNK signaling in hyperthermia. Thermal treatment of cells induces an increase in intracellular Ca2+ through Ca2+ efflux from the IP3R of the ER and Ca2+ entry from outside the cell, including voltage-gated calcium channels in the plasma membrane. Hyperthermia reduces the expression levels of MAP3Ks in ERK and JNK pathways through calpain- or proteasome-mediated protein degradation, insolubilization, and downregulation of their genes. Thus, hyperthermia may inhibit MAP3Ks in the ERK signaling pathway from transducing proliferative stimuli into activation signals or activating downstream targets. Solid lines indicate the activation process, whereas dotted lines represent negative regulation. However, ASK1–SEK1–JNK signaling is activated by heat through the suppression of the JNK-specific DUSP function. This deregulated JNK activation may be one of the mechanisms underlying the heat-induced death of cancer cells. P, phosphorylation; DUSP, dual specificity phosphatase; ER, endoplasmic reticulum; IP3R, 1, 4, 5-triphosphate (IP3)-.receptor.
6 Conclusion
MAPK signaling pathways participate in various physiological processes, including cell proliferation, death, and tumorigenesis (Braicu et al., 2019; Wu et al., 2019). Hyperthermia induces the activation of ERK, p38, and JNK signaling pathways. The efficiency of hyperthermia depends on the temperature and duration of heat treatment, which affects the activity of each MAPK. The dynamic balance between the ERK and JNK signaling pathways is crucial in determining whether a cell survives or undergoes apoptosis (Xia et al., 1995). Although JNKs stimulate or inhibit cell death in a context-dependent manner, sustained activation of JNK is necessary for the induction of apoptosis (Dhanasekaran and Reddy, 2017; Yue and López, 2020). The sustained activation of JNK requires multifaceted activation of the JNK signaling pathway under appropriate conditions. For example, hyperthermia reduces JNK phosphatase function, resulting in the sustained phosphor-ylation of ASK1, SEK1, and JNK and subsequent apoptosis. Further studies should be conducted to explore the mechanisms underlying the JNK signaling pathway during hyperthermia.
Author contributions
AE: Supervision, Writing–review and editing, Writing–original draft, Investigation, Funding acquisition, Formal Analysis, Data curation. TF: Writing–review and editing, Visualization. AY: Writing–review and editing, Visualization, Validation.
Funding
The author(s) declare that financial support was received for the research, authorship, and/or publication of this article. This work was supported, in part, by grants from the Ministry of Education, Culture, Sport, Science, and Technology of Japan (20K08100) and by the Japan Health and Research Institute.
Conflict of interest
TF and AY belong to the Social Cooperation Program, Department of Clinical Cannabinoid Research, supported by the Japan Cosmetic Association and Japan Federation of Medium & Small Enterprise Organizations.
The remaining author declares that the research was conducted in the absence of any commercial or financial relationships that could be construed as a potential conflict of interest.
Publisher’s note
All claims expressed in this article are solely those of the authors and do not necessarily represent those of their affiliated organizations, or those of the publisher, the editors and the reviewers. Any product that may be evaluated in this article, or claim that may be made by its manufacturer, is not guaranteed or endorsed by the publisher.
Abbreviations
ROS, reactive oxygen species; MAPK, mitogen-activated protein kinase; ERK, extracellular signal-regulated kinase; JNK, c-Jun NH2-terminal kinases; MAP2K; MAPK kinase; MEK, mitogen-activated ERK kinase; MKK, MAP kinase kinase; MAP3K, MAPKK kinase; RAF, rapidly accelerated fibrosarcoma; ASK, apoptosis signal regulating kinase; MEKK, mitogen-activated ERK kinase kinase; MLK, mixed lineage kinase; TGF-β, transforming growth factor-β; NF-κB, nuclear factor kappa B; HSP, Heat shock protein; DUSP, dual specificity phosphatase.
References
Ahmed, K., Zaidi, S. F., Mati-Ur-Rehman, M. R., and Kondo, T. (2020). Hyperthermia and protein homeostasis: cytoprotection and cell death. J. Therm. Biol. 91, 102615. doi:10.1016/j.jtherbio.2020.102615
Amaral, T., Nouri, N., and Garbe, C. (2016). The safety and efficacy of cobimetinib for the treatment of BRAF V600E or V600K mel-anoma. Expert Rev. Anticancer Ther. 16, 705–715. doi:10.1080/14737140.2016.1192469
Antolin, A. A., Clarke, P. A., Collins, I., Workman, P., and Al-Lazikani, B. (2021). Evolution of kinase polypharmacology across HSP90 drug discovery. Cell Chem. Biol. 28, 1433–1445.e3. Epub 2021 Jun 1. doi:10.1016/j.chembiol.2021.05.004
Arbour, N., Naniche, D., Homann, D., Davis, R. J., Flavell, R. A., and Oldstone, M. B. (2002). c-Jun NH(2)-terminal kinase (JNK)1 and JNK2 signaling pathways have divergent roles in CD8(+) T cell-mediated antiviral immunity. J. Exp. Med. 195, 801–810. doi:10.1084/jem.20011481
Avdi, N. J., Malcolm, K. C., Nick, J. A., and Worthen, G. S. (2002). A role for protein phosphatase-2A in p38 mitogen-activated protein kinase-mediated regulation of the c-Jun NH2-terminal kinase pathway in human neutrophils. J. Biol. Chem. 277, 40687–40696. doi:10.1074/jbc.M204455200
Bahar, M. E., Kim, H. J., and Kim, D. R. (2023). Targeting the RAS/RAF/MAPK pathway for cancer therapy: from mechanism to clinical studies. Signal Transduct. Target Ther. 8, 455. doi:10.1038/s41392-023-01705-z
Birbo, B., Madu, E. E., Madu, C. O., Jain, A., and Lu, Y. (2021). Role of HSP90 in cancer. Int. J. Mol. Sci. 22, 10317. doi:10.3390/ijms221910317
Braicu, C., Buse, M., Busuioc, C., Drula, R., Gulei, D., Raduly, L., et al. (2019). A comprehensive review on MAPK: a promising therapeutic target in cancer. Cancers (Basel) 11, 1618. doi:10.3390/cancers11101618
Cai, P. C., Shi, L., Liu, V. W., Tang, H. W., Liu, I. J., Leung, T. H., et al. (2014). Elevated TAK1 augments tumor growth and metastatic capacities of ovarian cancer cells through activation of NF-κB signaling. Oncotarget 5, 7549–7562. doi:10.18632/oncotarget.2273
Cellurale, C., Girnius, N., Jiang, F., Cavanagh-Kyros, J., Lu, S., Garlick, D. S., et al. (2012). Role of JNK in mammary gland development and breast cancer. Cancer Res. 72, 472–481. doi:10.1158/0008-5472.CAN-11-1628
Cerami, E., Gao, J., Dogrusoz, U., Gross, B. E., Sumer, S. O., Aksoy, B. A., et al. (2012). The cBio cancer genomics portal: an open platform for ex-ploring multidimensional cancer genomics data. Cancer Discov. 2, 401–404. doi:10.1158/2159-8290.CD-12-0095
Chang, L., and Karin, M. (2001). Mammalian MAP kinase signalling cascades. Nature 410, 37–40. doi:10.1038/35065000
Chen, J., Ye, C., Wan, C., Li, G., Peng, L., Peng, Y., et al. (2021). The roles of c-jun N-terminal kinase (JNK) in infectious diseases. Int. J. Mol. Sci. 22, 9640. doi:10.3390/ijms22179640
Chen, Y., Qiang, Y., Fan, J., Zheng, Q., Yan, L., Fan, G., et al. (2024). Aggresome formation promotes ASK1/JNK signaling activation and stemness maintenance in ovarian cancer. Nat. Commun. 15, 1321. doi:10.1038/s41467-024-45698-x
Dai, C. (2018). The heat-shock, or HSF1-mediated proteotoxic stress, response in cancer: from proteomic stability to oncogenesis. Phil. Trans. R. Soc. B373, 20160525. doi:10.1098/rstb.2016.0525
Dai, R., Frejtag, W., He, B., Zhang, Y., and Mivechi, N. F. (2000). c-Jun NH2-terminal kinase targeting and phosphorylation of heat shock factor-1 suppress its transcriptional activity. J. Biol. Chem. 275, 18210–18218. doi:10.1074/jbc.M000958200
Dhanasekaran, D. N., and Reddy, E. P. (2017). JNK-signaling: a multiplexing hub in programmed cell death. Genes and Cancer 8, 682–694. doi:10.18632/genesandcancer.155
Dillon, M., Lopez, A., Lin, E., Sales, D., Perets, R., and Jain, P. (2021). Progress on ras/MAPK signaling research and targeting in blood and solid cancers. Cancers (Basel) 13, 5059. doi:10.3390/cancers13205059
Dorion, S., and Landry, J. (2002). Activation of the mitogen-activated protein kinase pathways by heat shock. Cell Stress Chaperones 7, 200–206. doi:10.1379/1466-1268(2002)007<0200:aotmap>2.0.co;2
Enomoto, A., Fukasawa, T., Terunuma, H., Nakagawa, K., Yoshizaki, A., Sato, S., et al. (2024). Deregulated JNK signaling enhances apoptosis during hyperthermia. Int. J. Hyperther. 41 (1), 2335199. doi:10.1080/02656736.2024.2335199
Enomoto, A., Fukasawa, T., Terunuma, H., Nakagawa, K., Yoshizaki, A., Sato, S., et al. (2022). Decrease in MAP3Ks expression enhances the cell death caused by hyperthermia. Int. J. Hyperther. 39, 200–208. doi:10.1080/02656736.2021.2024281
Enomoto, A., Fukasawa, T., Tsumoto, H., Karube, M., Nakagawa, K., Yoshizaki, A., et al. (2019). Prevention of calpain-dependent degradation of STK38 by MEKK2-mediated phosphorylation. Sci. Rep. 9, 16010. doi:10.1038/s41598-019-52435-8
Fernández-Medarde, A., and Santos, E. (2011). Ras in cancer and developmental diseases. Genes Cancer 2, 344–358. doi:10.1177/1947601911411084
Gabai, V. L., Meriin, A. B., Yaglom, J. A., Volloch, V. Z., and Sherman, M. Y. (1998). Role of Hsp70 in regulation of stress-kinase JNK: implications in apoptosis and aging. FEBS Lett. 1438, 1–4. doi:10.1016/s0014-5793(98)01242-3
Gao, J., Aksoy, B. A., Dogrusoz, U., Dresdner, G., Gross, B., Sumer, S. O., et al. (2013). Integrative analysis of complex cancer genomics and clinical profiles using the cBioPortal. Sci. Signal 6, pl1. doi:10.1126/scisignal.2004088
García-Alonso, S., Mesa, P., Ovejero, L. P., Aizpurua, G., Lechuga, C. G., Zarzuela, E., et al. (2022). Structure of the RAF1-HSP90-CDC37 complex reveals the basis of RAF1 regulation. Mol. Cell. 82, 3438–3452.e8. doi:10.1016/j.molcel.2022.08.012
Ha, J., Kang, E., Seo, J., and Cho, S. (2019). Phosphorylation dynamics of JNK signaling: effects of dual-specificity phosphatases (DUSPs) on the JNK pathway. Int. J. Mol. Sci. 20, 6157. doi:10.3390/ijms20246157
Haeusgen, W., Herdegen, T., and Waetzig, V. (2011). The bottleneck of JNK signaling: molecular and functional characteristics of MKK4 and MKK7. Eur. J. Cell Biol. 90, 536–544. Epub 2011 Feb 18. doi:10.1016/j.ejcb.2010.11.008
Hao, Y., Feng, Y., Li, J., and Gu, X. (2018). Role of MAPKs in HSP70's protection against heat stress-induced injury in rat small intestine. Biomed. Res. Int. 1571406, 1571406. doi:10.1155/2018/1571406
Hattori, K., Naguro, I., Runchel, C., and Ichijo, H. (2009). The roles of ASK family proteins in stress re-sponses and diseases. Cell Commun. Signal 7, 9. doi:10.1186/1478-811X-7-9
Hou, C. H., Lin, F. L., Hou, S. M., and Liu, J. F. (2014). Hyperthermia induces apoptosis through endoplasmic reticulum and reactive oxygen species in human osteosarcoma cells. Int. J. Mol. Sci. 15, 17380–17395. doi:10.3390/ijms151017380
Huang, X. L., Zhang, H., Yang, X. Y., Dong, X. Y., Xie, X. Y., Yin, H. B., et al. (2017). Activation of a c-Jun N-terminal kinase-mediated autophagy pathway attenuates the anticancer activity of gemcitabine in human bladder cancer cells. Anticancer Drugs 28, 596–602. doi:10.1097/CAD.0000000000000499
Inokuchi, S., Aoyama, T., Miura, K., Osterreicher, C. H., Kodama, Y., Miyai, K., et al. (2010). Disruption of TAK1 in hepatocytes causes hepatic injury, inflammation, fibrosis, and carcinogenesis. Proc. Natl. Acad. Sci. U. S. A. 107, 844–849. doi:10.1073/pnas.0909781107
Jiang, C. F., Wen, L. Z., Yin, C., Xu, W. P., Shi, B., Zhang, X., et al. (2016). Apoptosis signal-regulating kinase 1 mediates the inhibitory effect of hepatocyte nuclear factor-4α on hepatocellular carcinoma. Oncotarget 7, 27408–27421. doi:10.18632/oncotarget.8478
Jin, H., Zhao, Y., Yang, J., Zhang, X., and Ma, S. (2018). Hyperthermia enhances the sensitivity of pancreatic cancer SW1990 cells to gemcitabine through ROS/JNK signaling. Oncol. Lett. 16, 6742–6748. Epub 2018 Sep 18. doi:10.3892/ol.2018.9455
Katschinski, D. M., Wiedemann, G. J., Longo, W., d'Oleire, F. R., Spriggs, D., and Robins, H. I. (1999). Whole body hyperthermia cytokine induction: a review, and unifying hypothesis for myeloprotection in the setting of cytotoxic therapy. Cytokine Growth Factor Rev. 10, 93–97. doi:10.1016/s1359-6101(99)00006-4
Ke, R., Viswakarma, N., Menhart, M., Singh, S. K., Kumar, S., Srivastava, P., et al. (2024). MLK3 promotes prooncogenic signaling in hepatocel-lular carcinoma via TGFβ pathway. Oncogene 10, 2307–2324. Epub ahead of print. doi:10.1038/s41388-024-03055-8
Kondoh, K., and Nishida, E. (2007). Regulation of MAP kinases by MAP kinase phosphatases. Biochim. Biophys. Acta. 1773, 1227–1237. Epub 2006 Dec 8. doi:10.1016/j.bbamcr.2006.12.002
Kumar, S., Singh, S. K., Rana, B., and Rana, A. (2021). The regulatory function of mixed lineage kinase 3 in tumor and host immunity. Pharmacol. Ther. 219, 107704. doi:10.1016/j.pharmthera.2020.107704
Lavoie, H., Gagnon, J., and Therrien, M. (2020). ERK signalling: a master regulator of cell behaviour, life and fate. Nat. Rev. Mol. Cell Biol. 21, 607–632. doi:10.1038/s41580-020-0255-7
Lee, J. M., Lee, J. M., Kim, K. R., Im, H., and Kim, Y. H. (2015). Zinc preconditioning protects against neuronal apoptosis through the mitogen-activated protein kinase-mediated induction of heat shock protein 70. Biochem. Biophys. Res. Commun. 459, 220–226. doi:10.1016/j.bbrc.2015.02.068
Lee, S. H., Lee, J. W., Soung, Y. H., Kim, S. Y., Nam, S. W., Park, W. S., et al. (2004). Colorectal tumors frequently express phosphorylated mitogen-activated protein kinase. APMIS 112, 233–238. doi:10.1111/j.1600-0463.2004.apm11204-0502.x
Li, H., Liu, Y., Gu, Z., Li, L., Liu, Y., Wang, L., et al. (2018). p38 MAPK-MK2 pathway regulates the heat-stress-induced accumulation of reactive oxygen species that mediates apoptotic cell death in glial cells. Oncol. Lett. 15, 775–782. Epub 2017 Nov 8. doi:10.3892/ol.2017.7360
Li, L., Tan, H., Yang, H., Li, F., He, X., Gu, Z., et al. (2017). Reactive oxygen species mediate heat stress-induced apoptosis via ERK dephosphorylation and Bcl-2 ubiquitination in human umbilical vein endothelial cells. Oncotarget 8, 12902–12916. doi:10.18632/oncotarget.14186
Lin, P., Niu, W., Peng, C., Zhang, Z., and Niu, J. (2015). The role of TAK1 expression in thyroid cancer. Int. J. Clin. Exp. Pathol. 8, 14449–14456. PMID: 26823762.
Liu, J. F., Chen, P. C., Ling, T. Y., and Hou, C. H. (2022). Hyperthermia increases HSP production in human PDMCs by stimulating ROS formation, p38 MAPK and Akt signaling, and increasing HSF1 activity. Stem Cell Res. Ther. 13, 236. doi:10.1186/s13287-022-02885-1
Liu, X. Y., She, C. C., and Cheung, P. C. (2008). HSP90 is required for TAK1 stability but not for its activation in the pro-inflammatory signaling pathway. FEBS Lett. 582, 4023–4031. Epub 2008 Nov 19. doi:10.1016/j.febslet.2008.10.053
Low, H. B., Wong, Z. L., Wu, B., Kong, L. R., Png, C. W., Cho, Y. L., et al. (2021). DUSP16 promotes cancer chemoresistance through regulation of mitochondria-mediated cell death. Nat. Commun. 12, 2284. doi:10.1038/s41467-021-22638-7
Luo, Y., Gao, S., Hao, Z., Yang, Y., Xie, S., Li, D., et al. (2016). Apoptosis signal-regulating kinase 1 exhibits oncogenic activity in pancreatic cancer. Oncotarget 7, 75155–75164. doi:10.18632/oncotarget.12090
Ma, L., Cheng, Y., and Zeng, J. (2019). MLK3 silence induces cervical cancer cell apoptosis via the Notch-1/autophagy network. Clin. Exp. Pharmacol. Physiol. 46, 854–860. Epub 2019 Jul 3. doi:10.1111/1440-1681.13123
Masuda, K., Shima, H., Watanabe, M., and Kikuchi, K. (2001). MKP-7, a novel mitogen-activated protein kinase phosphatase, functions as a shuttle protein. J. Biol. Chem. 276, 39002–39011. Epub 2001 Aug 6. doi:10.1074/jbc.M104600200
Meriin, A. B., Yaglom, J. A., Gabai, V. L., Zon, L., Ganiatsas, S., Mosser, D. D., et al. (1999). Protein-damaging stresses activate c-Jun N-terminal kinase via inhibition of its dephosphorylation: a novel pathway controlled by HSP72. Mol. Cell. Biol. 19, 2547–2555. doi:10.1128/MCB.19.4.2547
Mlakar, V., Morel, E., Mlakar, S. J., Ansari, M., and Gumy-Pause, F. (2021). A review of the biological and clinical implications of RAS-MAPK pathway alterations in neuroblastoma. J. Exp. Clin. Cancer Res. 40, 189. doi:10.1186/s13046-021-01967-x
Mukhopadhyay, H., and Lee, N. Y. (2020). Multifaceted roles of TAK1 signaling in cancer. Oncogene 39, 1402–1413. Epub 2019 Nov 6. doi:10.1038/s41388-019-1088-8
Nagata, Y., and Todokoro, K. (1999). Requirement of activation of JNK and p38 for environmental stress-induced erythroid differentiation and apoptosis and of inhibition of ERK for apoptosis. Blood 94 (3), 853–863. doi:10.1182/blood.v94.3.853.415a12_853_863
Nair, R. S., Kumar, S., Das, S., Singh, S. K., Srivastava, P., Sondarva, G., et al. (2023). TrkA expression directs the anti-neoplastic activity of MLK3 inhibitors in triple-negative breast cancer. Oncogene 42, 1132–1143. doi:10.1038/s41388-023-02633-6
Ng, D. C., and Bogoyevitch, M. A. (2000). The mechanism of heat shock activation of ERK mitogen-activated protein kinases in the interleukin 3-dependent ProB cell line BaF3. J. Biol. Chem. 275, 40856–40866. doi:10.1074/jbc.M004639200
Nguyen, K., Tran, M. N., Rivera, A., Cheng, T., Windsor, G. O., Chabot, A. B., et al. (2022). MAP3K family review and correlations with patient survival outcomes in various cancer types. Types. Front. Biosci. Landmark Ed. 27, 167. doi:10.31083/j.fbl2705167
Ogier, J. M., Nayagam, B. A., and Lockhart, P. J. (2020). ASK1 inhibition: a therapeutic strategy with multi-system benefits. J. Mol. Med. 98, 335–348. doi:10.1007/s00109-020-01878-y
Palacios, C., Collins, M. K., and Perkins, G. R. (2001). The JNK phosphatase M3/6 is inhibited by protein-damaging stress. Curr. Biol. 11, 1439–1443. doi:10.1016/s0960-9822(01)00426-2
Park, H. S., Cho, S. G., Kim, C. K., Hwang, H. S., Noh, K. T., Kim, M. S., et al. (2002). Heat shock protein hsp72 is a negative regulator of apoptosis signal-regulating kinase 1. Mol. Cell Biol. 22, 7721–7730. doi:10.1128/MCB.22.22.7721-7730.2002
Park, H. S., Lee, J. S., Huh, S. H., Seo, J. S., and Choi, E. J. (2001). Hsp72 functions as a natural inhibitory protein of c-Jun N-terminal kinase. EMBO J. 20, 446–456. doi:10.1093/emboj/20.3.446
Park, J. G., Aziz, N., and Cho, J. Y. (2019). MKK7, the essential regulator of JNK signaling involved in cancer cell survival: a newly emerging anticancer therapeutic target. Ther. Adv. Med. Oncol. 11, 1758835919875574. doi:10.1177/1758835919875574
Peterson, A. F., Ingram, K., Huang, E. J., Parksong, J., McKenney, C., Bever, G. S., et al. (2022). Systematic analysis of the MAPK signaling network reveals MAP3K-driven control of cell fate. Cell Syst. 13, 885–894.e4. doi:10.1016/j.cels.2022.10.003
Rattanasinchai, C., and Gallo, K. A. (2016). MLK3 signaling in cancer invasion. Cancers 8, 51. doi:10.3390/cancers8050051
Rattanasinchai, C., Llewellyn, B. J., Conrad, S. E., and Gallo, K. A. (2017). MLK3 regulates FRA-1 and MMPs to drive invasion and transendothelial migration in triple-negative breast cancer cells. Oncogenesis 6, e345. doi:10.1038/oncsis.2017.44
Recio-Boiles, A., Ilmer, M., Rhea, P. R., Kettlun, C., Heinemann, M. L., Ruetering, J., et al. (2016). JNK pathway inhibition selectively primes pancreatic cancer stem cells to TRAIL-induced apoptosis without affecting the physiology of normal tissue resident stem cells. Oncotarget 7, 9890–9906. doi:10.18632/oncotarget.7066
Ribas, A., Gonzalez, R., Pavlick, A., Hamid, O., Gajewski, T. F., Daud, A., et al. (2014). Combination of vemurafenib and cobimetinib in patients with advanced BRAF(V600)-mutated melanoma: a phase 1b study. Lancet Oncol. 15, 954–965. doi:10.1016/S1470-2045(14)70301-8
Roberts, R., Hallee, L., and Lam, C. K. (2021). The potential of Hsp90 in targeting pathological pathways in cardiac diseases. J. Pers. Med. 11, 1373. doi:10.3390/jpm11121373
Roh, Y. S., Song, J., and Seki, E. (2014). TAK1 regulates hepatic cell survival and carcinogenesis. J. Gastroenterol. 49, 185–194. doi:10.1007/s00535-013-0931-x
Roti, J. L. (2008). Cellular responses to hyperthermia (40–46 °C): cell killing and molecular events. Int. J. Hyperther. 24, 3–15. doi:10.1080/02656730701769841
Ryuno, H., Naguro, I., and Kamiyama, M. (2017). ASK family and cancer. Adv. Biol. Regul. 66, 72–84. Epub 2017 May 20. doi:10.1016/j.jbior.2017.05.003
Sakurai, H. (2012). Targeting of TAK1 in inflammatory disorders and cancer. Trends Pharmacol. Sci. 33, 522–530. doi:10.1016/j.tips.2012.06.007
Schopf, F. H., Biebl, M. M., and Buchner, J. (2017). The HSP90 chaperone machinery. Nat. Rev. Mol. Cell Biol. 18, 345–360. Epub 2017 Apr 21. doi:10.1038/nrm.2017.20
Schroyer, A. L., Stimes, N. W. A., Saab, W. F., and Chadee, D. N. (2018). MLK3 phosphorylation by ERK1/2 is required for oxidative stress-induced invasion of colorectal cancer cells. Oncogene 37, 1031–1040. Epub 2017 Oct 30. doi:10.1038/onc.2017.396
Simanshu, D. K., and Morrison, D. K. (2022). A structure is worth a thousand words: new insights for RAS and RAF regulation. Cancer Discov. 12, 899–912. HYPERLINK. doi:10.1158/2159-8290.CD-21-1494
Singh, I. S., and Hasday, J. D. (2013). Fever, hyperthermia and the heat shock response. Int. J. Hypertherm. 29, 423–435. doi:10.3109/02656736.2013.808766
Stankiewicz, A. R., Lachapelle, G., Foo, C. P., Radicioni, S. M., and Mosser, D. D. (2005). Hsp70 inhibits heat-induced apoptosis upstream of mitochondria by preventing Bax translocation. J. Biol. Chem. 280, 38729–38739. Epub 2005 Sep 19. doi:10.1074/jbc.M509497200
Streicher, J. M. (2019). The role of heat shock proteins in regulating receptor signal transduction. Mol. Pharmacol. 95, 468–474. Epub 2019 Jan 22. doi:10.1124/mol.118.114652
Su, K. H., Cao, J., Tang, Z., Dai, S., He, Y., Sampson, S. B., et al. (2016). HSF1 critically attunes proteotoxic stress sensing by mTORC1 to combat stress and promote growth. Nat. Cell Biol. 18, 527–539. Epub 2016 Apr 4. doi:10.1038/ncb3335
Takaesu, G., Surabhi, R. M., Park, K. J., Ninomiya-Tsuji, J., Matsumoto, K., and Gaynor, R. B. (2003). TAK1 is critical for IkappaB kinase-mediated activation of the NF-kappaB pathway. J. Mol. Biol. 326, 105–115. doi:10.1016/s0022-2836(02)01404-3
Tam, S. Y., Wu, V. W. C., and Law, H. K. W. (2019). Dynamics of oxygen level-driven regulators in modulating autophagy in colorectal cancer cells. Biochem. Biophys. Res. Commun. 517, 193–200. Epub 2019 Jul 19. doi:10.1016/j.bbrc.2019.07.043
Teramachi, J., Tenshin, H., Hiasa, M., Oda, A., Bat-Erdene, A., Harada, T., et al. (2021). TAK1 is a pivotal therapeutic target for tumor progression and bone destruction in myeloma. Haematologica 106, 1401–1413. doi:10.3324/haematol.2019.234476
Timofeev, O., Giron, P., Lawo, S., Pichler, M., and Noeparast, M. (2024). ERK pathway agonism for cancer therapy: evidence, insights, and a target discovery framework. NPJ Precis. Oncol. 8, 70. doi:10.1038/s41698-024-00554-5
Tran, T. H., Chan, A. H., Young, L. C., Bindu, L., Neale, C., Messing, S., et al. (2021). KRAS interaction with RAF1 RAS-binding domain and cysteine-rich domain provides insights into RAS-mediated RAF activation. Nat. Commun. 12, 1176. doi:10.1038/s41467-021-21422-x
Vaupel, P., Piazena, H., Notter, M., Thomsen, A. R., Grosu, A. L., Scholkmann, F., et al. (2023). From local-ized mild hyperthermia to improved tumor oxygenation: physiological mechanisms critically involved in oncologic thermo-radioimmunotherapy. Cancers. (Basel). 15, 1394. doi:10.3390/cancers15051394
Wang, D. C., Zhang, Y., Chen, H. Y., Li, X. L., Qin, L. J., Li, Y. J., et al. (2012). Hyperthermia promotes apoptosis and suppresses invasion in C6 rat glioma cells. Asian pac. J. Cancer Prev. 13, 3239–3245. doi:10.7314/apjcp.2012.13.7.3239
Wang, P., Laster, K., Jia, X., Dong, Z., and Liu, K. (2023). Targeting CRAF kinase in anti-cancer therapy: progress and opportunities. Mol. Cancer. 22, 208. doi:10.1186/s12943-023-01903-x
Wu, Q., Wu, W., Fu, B., Shi, L., Wang, X., and Kuca, K. (2019). JNK signaling in cancer cell survival. Med. Res. Rev. 39, 2082–2104. Epub 2019 Mar 25. doi:10.1002/med.21574
Xia, Z., Dickens, M., Raingeaud, J., Davis, R. J., and Greenberg, M. E. (1995). Opposing effects of ERK and JNK-p38 MAP kinases on apoptosis. Science 270, 1326–1331. doi:10.1126/science.270.5240.1326
Xu, Y. R., and Lei, C. Q. (2021). TAK1-TABs complex: a central signalosome in inflammatory responses. Front. Immunol. 11, 608976. doi:10.3389/fimmu.2020.608976
Yang, C. Y., Li, J. P., Chiu, L. L., Lan, J. L., Chen, D. Y., Chuang, H. C., et al. (2014). Dual-specificity phosphatase 14 (DUSP14/MKP6) negatively regulates TCR signaling by inhibiting TAB1 activation. J. Immunol. 192, 1547–1557. doi:10.4049/jimmunol.1300989
Yang, Y., Qiu, Y., Tang, M., Wu, Z., Hu, W., and Chen, C. (2017). Expression and function of transforming growth factor‑β‑activated protein kinase 1 in gastric cancer. Mol. Med. Rep. 16, 3103–3110. Epub 2017 Jul 15. doi:10.3892/mmr.2017.6998
Yin, M., Zhou, H. J., Zhang, J., Lin, C., Li, H., Li, X., et al. (2017). ASK1-dependent endothelial cell activation is critical in ovarian cancer growth and metastasis. JCI. Insight. 2, e91828. doi:10.1172/jci.insight.91828
Yue, J., and López, J. M. (2020). Understanding MAPK signaling pathways in apoptosis. Int. J. Mol. Sci. 21, 2346. doi:10.3390/ijms21072346
Zeke, A., Misheva, M., Reményi, A., and Bogoyevitch, M. A. (2016). JNK signaling: regulation and functions based on complex pro-tein-protein partnerships. Microbiol. Mol. Biol. Rev. 80, 793–835. doi:10.1128/mmbr.00043-14
Zhang, K., Zhai, R., Xue, T., Xu, X., Ren, Y., Ma, M., et al. (2020). HSP70 regulates cell proliferation and apoptosis in actinomycin-D-treated lung cancer cells. Cancer Res. 9, 1167–1173. doi:10.21037/tcr.2019.12.100
Keywords: MAPK, signal transduction, hyperthermia, heat shock proteins, phosphatases, cell death
Citation: Enomoto A, Fukasawa T and Yoshizaki A (2024) Hyperthermia-mediated cell death via deregulation of extracellular signal-regulated kinase and c-Jun NH2-terminal kinase signaling. Front. Cell. Death 3:1465506. doi: 10.3389/fceld.2024.1465506
Received: 16 July 2024; Accepted: 24 September 2024;
Published: 07 October 2024.
Edited by:
Paola Maycotte, Instituto Mexicano del Seguro Social, MexicoReviewed by:
Martha Robles-Flores, National Autonomous University of Mexico, MexicoCopyright © 2024 Enomoto, Fukasawa and Yoshizaki. This is an open-access article distributed under the terms of the Creative Commons Attribution License (CC BY). The use, distribution or reproduction in other forums is permitted, provided the original author(s) and the copyright owner(s) are credited and that the original publication in this journal is cited, in accordance with accepted academic practice. No use, distribution or reproduction is permitted which does not comply with these terms.
*Correspondence: Atsushi Enomoto, YWVub21vdG9AbS51LXRva3lvLmFjLmpw