- Department of Biochemistry, Stellenbosch University, Stellenbosch, South Africa
Plasmodium falciparum, the predominant cause of severe malaria, thrives within both poikilotherm mosquitoes and homeotherm humans, navigating challenging temperature shifts during its life cycle. Survival in such varying environments necessitate the development of robust mechanisms, including a sophisticated protein folding system to mitigate proteopathy. The parasite needs to control the survival of its host cells which affects its chances of development and propagation. Central to this system are heat shock proteins (Hsps), among which small Hsps (sHsps) play pivotal roles in maintaining proteostasis (protein homeostasis). In both humans and P. falciparum, numerous sHsps have been identified, making them attractive candidates as biomarkers for diagnostic and drug development strategies. Evidence is accumulating suggesting that these sHsps participate in cell death processes, potentially influencing disease pathogenesis. Despite their significance, the precise functions of sHsps in P. falciparum’s adaptation to stress conditions remains largely unknown. Comparative structural analysis of sHsps between human and P. falciparum reveals species-specific variations. Despite conserved tertiary structures, unique motifs are found in parasite sHsps which may modulate specialised chaperone functions. This review discusses the conserved and distinctive motifs of sHsps from the human host and the parasite, offering insights into shared and unique attributes. These findings illuminate the potential for species-specific targeting of sHsps, as players in cell death processes that may foster innovative biomarker identification approaches. As malaria continues to ravage Sub-Saharan Africa, understanding the molecular intricacies guiding parasite survival are essential in the development of interventions with heightened efficacy against this global health crisis.
1 Introduction
The main malaria agent, Plasmodium falciparum, survives between two physiologically distinct environments, in the female Anopheles mosquito and the human hosts. Throughout its complicated life cycle, the parasite encounters stressful conditions as it transitions the diverse environmental conditions, including fluctuations in temperature. Despite, these unfavourable conditions, the parasite has developed a highly efficient protein folding system for survival within the host (Shonhai and Blatch, 2014; Proellocks et al., 2016). Heat shock proteins (Hsps), a conserved and ubiquitous group of molecular chaperones that facilitates protein folding, have been implicated in cellular stress responses in both prokaryotic and eukaryotic organisms (Lindquist and Craig, 2003). Within P. falciparum, Hsps play vital roles in the parasite’s ability to adapt and survive through efficient protein folding, translocation (Pérez-Morales and Espinoza, 2015) and the formation of protein complexes (Botha et al., 2011). The formation of protein complexes by Hsps is significant for facilitating interactions between the host and the parasite (Saha et al., 2018; Zininga and Shonhai, 2019) which are part of the determinants of the pathophysiology of malaria.
In some eukaryotic organisms, Hsps account for approximately 2% of the cell proteome (Blatch, 2022). Among these proteins there are small Hsps (sHsps) which are low molecular weight 15–30 kDa members (Buchner et al., 1998). The sHsps are involved in stress response mechanisms and survival strategies that regulate cell death processes. This positions them as promising biomarker candidates for diagnosis and drug development across a wide array of organisms (Nakamoto and Vígh, 2007; Treweek et al., 2014).
The sHsps are molecular chaperones that function as “holdases” to inhibit the aggregation of denatured proteins in a nucleotide independent manner, thus, without refolding capability. They do this by binding to exposed hydrophobic patches on substrate proteins to suppress unproductive binding to other exposed hydrophobic patches on unfolded protein forming toxic aggregates that result in proteopathy (Gusev et al., 2002; Haslbeck and Vierling, 2015). In response to cellular stress such as heat and oxidative stress, the expression of sHsp is upregulated serving to maintain cellular homeostasis. This phenomenon has been linked to the suppression of cell death in cervical cancer cells (Arrigo and Welch, 1987). It is intriguing to note that the P. falciparum resides within human hepatocytes during the pre-erythrocytic stages, and in the red blood cells during the erythrocytic stages which are cells prone to oxidative stress. It has been reported that cells infected with the parasite have upregulated levels of Hsps (Pires et al., 2023), suggesting that Hsps are involved in the host’s response to the presence of the parasite. Consequently, the modulation of autophagy and apoptosis by sHsps in human cells may also affect the fate of P. falciparum. However, our understanding of the roles of P. falciparum sHsps is currently limited, as they have not been fully characterized. Therefore, comparing the structure and function of these parasite sHsps to their human homologs can provide insights into their functions in the parasite. Additionally, other Hsps have been implicated in parasite survival, drug resistance, and pathogenicity, making them potential targets for drug development (Shonhai and Blatch, 2014; Stofberg et al., 2021). Thus, we utilized bioinformatic tools to examine the structural and functional attributes of P. falciparum sHsps and their human homologues. This knowledge will facilitate the subsequent characterization of P. falciparum sHsps and their potential as biomarkers in cell death.
2 Subcellular localization of P. falciparum small Hsps and their human homolog
In humans, ten isoforms of sHsp, also referred to as HspB1 - 10 have been identified. On the other hand, P. falciparum expresses six isoforms of sHsp which we here refer to as PfHsp20-a (PlasmoDB accession number: PF3D_1304500), PfHsp20-b (PF3D7_0816500), PfHsp20-c (PF3D7_1211100), PfHsp20-d (PF3D7_1448700), PfHsp20-e (PF3D7_1472800), and PfHsp20-f (PF3D7_0314000) (Table 1). As in humans, multiple members of the sHsp exist within the same cellular compartment such as HspB1-10 in cytoplasm, implying functional redundancy. However, they also exhibit tissue-specific expression, such as HspB4 and HspB5 being abundant in the eye lens, HspB9 and HspB10 in the testis and HspB2 in cardiac and skeletal muscles (Tedesco et al., 2022), Additionally, during stress, these cytosolic sHsps translocate to the nucleus where they reside in sub-nuclear structures (Mackay et al., 2003). The P. falciparum sHsps, are also widely distributed in different parasite cell compartments as PfHsp20-a, PfHsp20-b, and PfHsp20-c are predominantly localized within the mitochondria, whilst PfHsp20-d and PfHsp20-f are localized within the nucleus, and PfHsp20-e is resident within the apicoplast, suggesting functional specialisation (Hall et al., 2002). This suggest specialised roles for the sHsps in different organisms.
3 The general structure of sHsps
The sHsp family members are found across all organisms and have a general structure characterized by a tripartite domain architecture. There are two main groups of sHsps with the first group protomers comprised of a conserved protein-binding domain, resembling the alpha crystallins called the α-crystallin domain (ACD), flanked by a variable N-terminal domain (NTD) and a C-terminal domain (CTD) (Figure 1). The other isoforms have a CHORD and Sgt1 (CS) domain in place of the ACD domain. As a result, there is low sequence conservation between the different sHsp family members. This suggest that the sHsps can be selectively targeted as biomarkers unique in parasites when compared to the human Homologs.
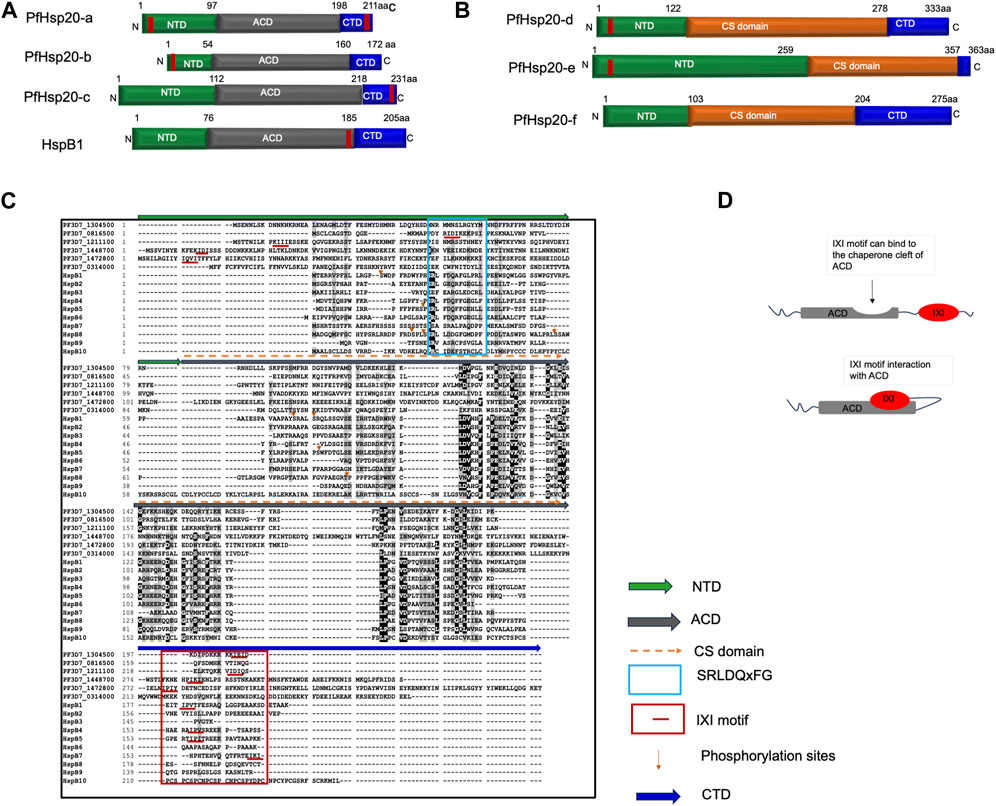
FIGURE 1. General structure of sHsp. (A) Schematic model of the domain organization of parasite ACD containing sHsp isoforms and homo sapiens HspB1. (B) Domain organization of CS domain containing parasite sHsp isoforms. (C) Multiple sequence alignment of human and P. falciparum sHsps. (D) Model interaction model of the C-terminal localized IXI motif with ACD in regulating substrate chaperone binding. In MSA, green line above the alignment indicates the N-terminal domain, grey line shows the ACD, and blue bar shows CTD. Cyan box shows conserved SRLDQxFG sequence on NTD of Human HspBs, Red lines/box indicates IXI/V motif in the NTD/CTD. Orange arrows show known phosphorylation sites. Identical residues are shown in white text on black background and similar residues are shown in black text on grey background, and non-conserved residues are shown in black text on white background. MSA was performed using ClustalW (https://www.ebi.ac.uk/Tools/msa/clustalw2/).
3.1 The sHsp alpha crystallin domain
Generally, the ACD is the most conserved domain among the main members of sHsp family, consisting of approximately 80–100 amino acid residues (Hilton et al., 2013). The ACD is characterized by a significant presence of immunoglobulin G (IgG) like β-pleated sheets (Janowska et al., 2019). This domain forms a compact β-sandwich structure comprising two anti-parallel β-sheets (Figure 1). The ACD of humans forms an antiparallel dimer along the β6–β7 strand. The chaperone functionality of sHsps is attributed to the ACD, which consists of two β-sheets flanked by α-helices.
3.2 The sHsp N-terminal domain
Despite the conserved ACD, sHsps have low amino acid sequence conservation levels on the NTD (Figure 1). The N-terminal domain of sHsps is rich in proline and aromatic residues (Kriehuber et al., 2010). This influences the structural variability which exhibits short α-helices, β-strands, and disordered regions (Van Montfort et al., 2001; Wintrode et al., 2003) (Figure 2). However, this flexibility makes the NTD to play an important role in substrate binding and oligomer assembly, and its truncation leads to a loss of chaperone function (Chaves et al., 2017). In addition, the NTD has higher presence of multiple phosphorylable serine/threonine residues (Strózecka et al., 2012; Heirbaut et al., 2014). The multiple phosphorylation sites identified in the mammalian sHsp NTD facilitate the protein functional roles in cellular protection and stress response. Conversely, a conserved stretch of N-terminal residues from 31 to 35 (residues SRLDQxFG) in HspB1, HspB6, and HspB8 has been identified as a negative regulator of activity (Tedesco et al., 2022). Deletion of this stretch enhanced the chaperoning capability (Heirbaut et al., 2014). In contrast, the SRLFDQxFG was shown to be important in the structure-function regulation of HspB4 and HspB5, where this motif plays a role in the quaternary structure and dynamics of subunit assembly of α-crystallins (Pasta et al., 2003). Both the NTD and the ACD of sHsps have been found to be involved in direct binding to client proteins and functioning as chaperones (Janowska et al., 2019).
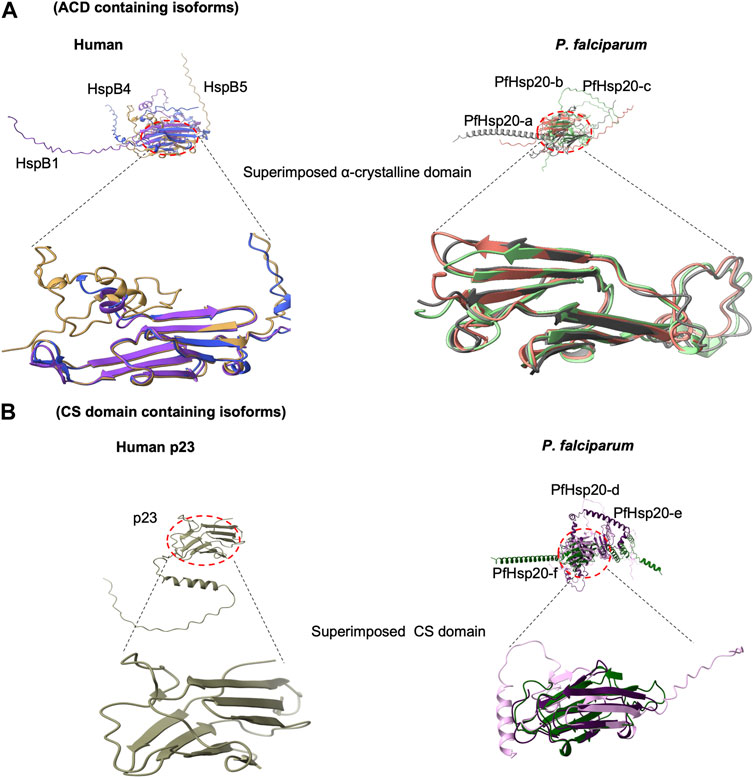
FIGURE 2. Comparison of 3-dimensional structure of ACD with CS domain. Superimposed structures of Human and P. falciparum sHsp. (A) shows structural arrangement in ACD, (B) The CS domain fold into similar antiparallel beta sandwich structure that corresponds to the fold of ACD. All structures were retrieved from Alphafold (https://alphafold.ebi.ac.uk/; accessed on 25 September 2023) and visualized using Chimera X (https://www.rbvi.ucsf.edu/chimerax).
3.3 The sHsp C-terminal domain
The CTD of sHsps exhibits significant variation in length and is characterized by a high content of polar and charged residues, rendering it highly disordered (Figure 1). In HspB1, HspB2, HspB4, HspB5, and HspB6, the CTD contains a flexible extension that remains disordered and mobile in solution, which acts as a solubilizing agent during chaperone-mediated interactions with client proteins (Strózecka et al., 2012; Boelens, 2020). Moreover, the CTD regions of HspB1, HspB2, HspB4, and HspB5 feature a conserved motif known as IXI/V (Janoskwa et al., 2019; Mogk et al., 2019). This motif facilitates binding to the hydrophobic groove of a neighbouring ACD, acting as a bridge between dimers and influencing the plasticity and overall structure of sHsp oligomers (Janowska et al., 2019; Boelens, 2020). Interestingly, the flexibility of the CTD enables interactions with various binding partners, regardless of their positioning. This is possibly due to their self-association of the ACD module and IXI/V motif. This contact between the ACD cleft and the IXI motif has the potential to hinder interactions between the sHsp and its client proteins (Figure 1D). For instance, in the HspB1 oligomer and HspB2/HspB3 tetramer, the IXI of one monomer extends to engage with the ACD cleft of an adjacent monomer (Clark et al., 2018; Nappi et al., 2020). Consequently, it is suggested that the dynamic control of this interaction plays an important role in regulating the activity of these sHsps (Balana et al., 2021). In HspB3, HspB6, and HspB8 the absence of the IXI/V motif in the CTD is thought to contribute to their reduced tendency to form higher order oligomers (Boelens, 2020). This is possibly due to their self-association of the ACD module and IXI/V motif. Therefore, absence of the IXI motif in some of the parasite sHsp might as well lead to reduced tendency to form higher order oligomers and the loss of interaction with the ACD. This could impact the protein’s ability to regulate or participate in certain protein-protein interactions.
In, P. falciparum sHsp, the domain architecture comprises of the tripartite, NTD, ACD and CTD as in the human equivalences. Despite possessing these three domains, there is low sequence conservation among the members of the parasite sHsp family (Figure 1). For example, like HspB3, HspB4, HspB5, and HspB6, the parasite PfHsp20-b, PfHsp20-d and PfHsp20-e also possesses the IXI motif in their NTD but lack the conserved SRLDQxFG sequence. Also, PfHsp20-a, PfHsp20-c and PfHsp20-d possesses the IXI motif in their CTD similar to the human HspB1, B2, B4. This implies that this motif may impact the function of PfsHsp in the same way as the human ones.
3.4 The CS domain of P. falciparum and their human homologs
Although sHsp are typically characterized by the presence of a conserved ACD, the three P. falciparum sHsp isoforms PfHsp20-d, PfHsp20-e, and PfHsp20-f possess a conserved CS domain (Figure 2). The CS domain generally share 25% sequence similarities with known human p23 as well as the SGT1protein and Rar1 (Kim et al., 1998; Biebl et al., 2021). The p23 is an established co-chaperone of Hsp90 chaperone family, where it plays an integral role to the chaperone machinery function (Grad et al., 2006). On the other hand, the SGT1 proteins are involved in kinetochore assembly and Rar1 protein implicated in development in animals (Garcia-Ranea et al., 2002). This suggests the CS domain implicates a role for sHsps in developmental processes in animals. It is interesting that structurally, the CS domains fold into a similar antiparallel beta sandwich structural fold of ACD (Figure 2). Thus, there is structural conservation between the ACD and the CS domain suggestive of similarities in function to the human p23 and SGT1 proteins (Singh et al., 2015). Therefore, these CS containing P. falciparum sHsp may have chaperone roles that are specialised for parasite co-chaperone p23 in modulating Hsp90 functional efficiency and in parasite development.
The sHsps chaperone function is regulated by the formation of either large hetero or homo—oligomeric structures of about 150–800 kDa (Mymrikov et al., 2020). The formation of higher order oligomers is a result of ionic and hydrophobic interactions. These interactions are influenced by environmental stressors like temperature fluctuations, pH and protein concentration, which subsequently impact the oligomer size and function with smaller oligomers having enhanced functional efficiency (Janowska et al., 2019).
3.5 Expression patterns of small Hsps
Most Hsps are upregulated in response to stress such as heat shock and oxidative stress due to increased levels of ROS. The sHsps family, known for its heterogeneity, displays temporal and tissue-specific expression patterns, suggesting distinct functions and regulatory mechanisms (Morrow and Tanguay, 2012). For example, increased expression patterns of HspB1 were observed in craniofacial bones during development. It was involved in the balance between differentiation and apoptosis, through modulating the viability of osteoblasts and chondrocytes, which are important in fetal skeletal maturation (Petryszak et al., 2014). In contrast, decreased expression of HspB2 were reported during skeletal muscle cell differentiation which limited the cell’s response to heat stress (Thakur et al., 2019). This resulted in the HspB2 primarily localizing to the cytoplasm of myoblasts and consequently myotubes formed distinct aggregates in the perinuclear spaces of myoblasts after heat shock (Thakur et al., 2019). Thus, sHsps play important roles in limiting cellular stress and maintaining proteostasis during different stages of development.
In P. falciparum, chaperone expression profiles correspond to different physiological states of the malaria parasite (Pallavi et al., 2010). A study by Acharya et al. (2009) observed that organellar chaperones that were targeted to mitochondria or apicoplast were highly upregulated in different physiological states of the parasite. Also, the expression of Hsps in P. falciparum has been implicated in modulating parasite developmental stage and response to oxidative stress (Akide-Ndunge et al., 2009). However, the effect of specific sHsps in parasite survival, and responses when subjected to antimalarials that induce oxidative stress in parasitized RBCs still need to be elucidated. A genome-wide expression profiling shows differential expression of Hsps between trophozoite and schizont stages in a Honduran chloroquine-sensitive HB3 P. falciparum parasite cell line (Bozdech et al., 2003). Other previous studies also reported that the expression profiles for parasite Hsps follows a coordinated and stage-dependent pattern (Rawat et al., 2021). Recently, Hsp20-b was observed to be upregulated in dihydroartemisinin treated parasite cells (Pires et al., 2023). These findings imply that parasite’s Hsp system responds to changes in redox conditions and exhibits significantly increased expression in oxidatively stressed parasites. The simultaneous disruption of the parasite’s antioxidant enzymes and Hsp system could impede parasite growth. Taken together, this suggest that parasite sHsp maybe important players in regulating cell survival under stressful conditions such as drug pressure thereby enhancing drug resistance development.
4 Perspectives of small Hsps in cell death
The existence of three primary types of cell death processes, namely, apoptosis, necrosis, and autophagic cell death, has been well-established in many eukaryotes including humans (Figure 3). Apoptosis functions to eliminate damaged or abnormal cells. Cells depend on checkpoints in the cell cycle to hinder the build-up and propagation of genetic mistakes. These control points in the cell cycle rely on signalling pathways that oversee issues such as DNA damage between cell divisions, the breakdown of DNA replication structures in the synthesis phase, and the inadequate assembly of spindle fibres during the mitosis phase (Beere, 2004). Some of the cell cycle regulatory proteins are controlled by the stress protein responses which influence the progression through the cell cycle influencing survival or death (Tokunaga et al., 2022). As such, several heat shock proteins such as Hsp70, Hsp90 and sHsps have been implicated in cell death processes in humans. For instance, Hsp70 inhibits apoptosis by binding to Apaf-1 and decreasing procaspase-9 recruitment, it also sustains the ability to inhibit apoptosis via other channels other than the cytochrome c/Apaf-1/caspase pathway (Albakova et al., 2020). By binding to anionic phospholipids and maintaining the lysosomal membrane, Hsp70 inhibits stress-induced membrane permeabilization and thus apoptosis (Calvaresi et al., 2021). By extension, this suggest that the role of PfHsp70-1 during intraerythrocytic replication may be involved with inhibition of apoptosis. PfHsp70-1 depletion decreases parasite development, showing its relevance in parasite survival (Lu et al., 2020). Similarly, Hsp90 interacts with proteins such as Bcl-2 to reduce Cytochrome C (Cyt C) release and to regulate the formation of apoptotic bodies. It also has an effect on the cleavage and function of caspases such as caspase 3, 6, and 9 (Peng et al., 2022). The detailed discussion on role of these Hsp90 and Hsp70 family members in cell death processes is beyond the scope of this study. However, it should be mentioned that some sHsps directly interact with various components of the tightly regulated cell death machinery, both upstream and downstream of mitochondrial events (Arrigo, 2005; Cristofani et al., 2019). The protective effects of sHsps are attributed to various biochemical mechanisms, including their role as molecular chaperones for misfolded proteins, regulation of enzyme activities, assistance in cellular detoxification against oxidative stress, and interaction with cell death pathways (Bellyei et al., 2007).
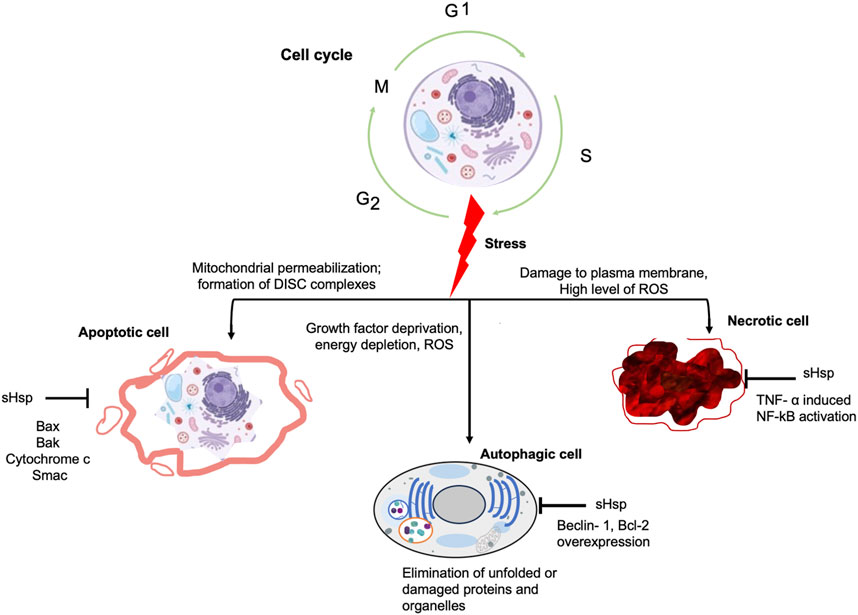
FIGURE 3. Schematic representation of the effect of stress on cell cycle. The cell undergoes the synthesis (S), gap (G1, G2) and mitosis phases during its cycle. However, constant and unmanageable stress events in the mitochondrial or plasma membrane affect this normal cycle leading to cell senescence through either apoptosis, autophagy or necrotic cell death.
4.1 Small heat shock proteins in apoptotic pathways
Apoptosis is the most common type of programmed cell death (PCD) in which plasma membrane and membrane organelles play a pivotal role (Galluzzi et al., 2012). Apoptosis has two main signalling pathways that later converge for its effector mechanism. These pathways include the intrinsic pathway which is a mitochondrial mediated pathway where there is release of apoptogenic factors (Figure 4). The second is the extrinsic pathway which involves signalling through the “death” receptors on the cell surface that function with the specific ligands to effect cell death (Lossi, 2022). Additionally, there is another important mechanism known as perforin/granzyme-mediated apoptosis. This pathway is primarily employed by immune cells to eliminate infected or aberrant cells (Rousalova and Krepela, 2010). It is an essential part of the immune system’s defence against pathogens and cancerous cells. Granzymes are cytotoxic proteases released by cytotoxic T lymphocytes (CD8 T cells) to induce cell death in target cells (Zininga et al., 2018; Hay and Slansky, 2022). They are contained within granules along with perforin. Apart from these, there are also mechanisms that inhibit apoptosis which include the pro-survival pathways that activate the PI3K/Akt (phosphoinositide 3-kinase/protein kinase B pathway (Rane et al., 2001; Song et al., 2005) and the cytoskeletal modulation of cell cycle events. The regulation of the pro- and anti-apoptotic pathways is essential in dictating the fate of the cell. Despite the lack of some members of these apoptotic signalling pathways in P. falciparum, all three forms of cell death have been suggested to occur in liver-stage parasites (Eickel et al., 2013).
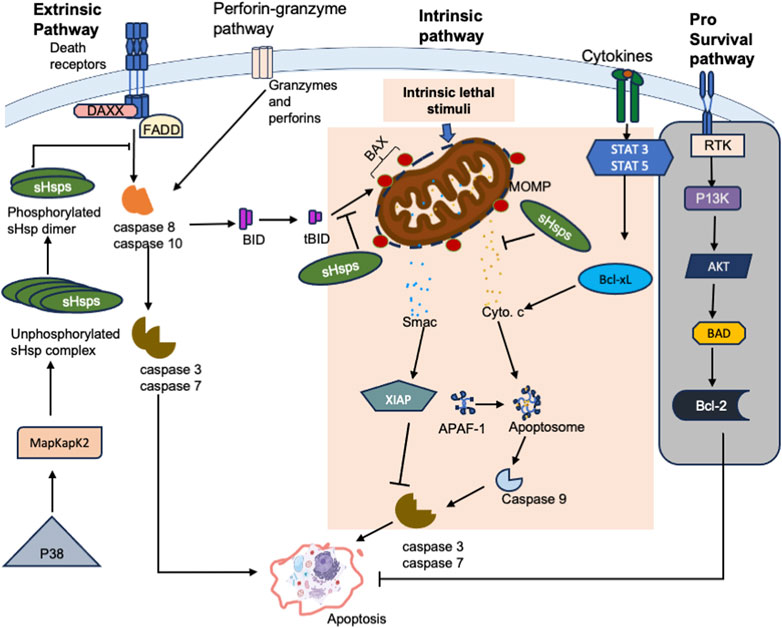
FIGURE 4. The schematic representation of the interactions of sHsps with factors on the apoptotic pathways. Interaction of Fas with Daxx triggers apoptosis. Phosphorylated dimers of sHsp interact with Daxx preventing its interaction with ASK1 and Fas, thus inhibiting Daxx mediated apoptosis. sHsp also interacts with the pro-apoptotic molecule, Bax and inhibit its translocation into the mitochondria and subsequent release of cytochrome c. sHsp interacts with cytochrome c and prevent cytochrome c mediated interaction of Apaf-1 with caspase-9 from apoptosome. sHsp inhibits the release of the second mitochondria -derived activator of caspases, Smac, which binds to the inhibitor of apoptosis proteins (IAPs) and promote apoptosis via activation of caspases. Receptor Tyrosine Kinases (RTKs) promote cell survival by activating downstream signalling pathways that inhibit apoptosis. However, suppression of this pathway shifts the balance towards pro-apoptotic signals, leading to cell death (grey background).
4.1.1 Extrinsic and intrinsic apoptotic pathways
Apoptotic signalling through the extrinsic pathway is triggered when specific extracellular ligands bind to transmembrane death receptors on the cell. Some of the receptors involved in signalling include Tumor necrosis factor (TNF) receptor 1 (TNFR1), Fas (also known as CD95/Apo-1), and TNF-related apoptosis-inducing ligand (TRAIL) receptors reviewed in Jan and Chaudhry (2019). The binding of the respective ligands on these death receptors leads to the formation of a complex called the death-inducing signalling complex (DISC) (Ghobrial et al., 2005). This DISC is composed of the Fas-associated death domain (FADD), which acts as an adaptor molecule, along with procaspase-8, procaspase-10, and cellular FLICE inhibitory proteins (c-FLIPs) to activate caspase-8. Caspase-8 is a cysteine—aspartate specific protease that dimerises when activated and dissociates from the DISC to initiate a cascade of caspase activations, which marks the execution phase of apoptosis (Mandal et al., 2020; Orning and Lien, 2021). Several caspases play crucial roles as both initiators and executioners of apoptosis, with their functions tightly linked to their structural characteristics and specific substrate preferences.
The intrinsic apoptotic pathway primarily involves mitochondrial mediation and is activated in response to various cellular stresses like oxidative stress, radiation, and cytotoxic drugs. This pathway includes activation of key apoptotic effector proteins Bax and Bak that form pores onto the mitochondrial membranes (Heimer et al., 2019). These pores lead to the release of cytochrome c to facilitate the formation of the apoptosome formation together with Apaf-1 and procaspase-9. The apoptosome complex then triggers caspase-9 activation, which activates a caspase cascade involving caspase-3, leading to cell breakdown and apoptosis (Unnisa et al., 2023).
The extrinsic and intrinsic pathways converge in the execution phase. The execution phase involves further caspase activation, cytoplasmic endonuclease activity, chromatin condensation, and formation of apoptotic bodies. Caspase-3 is a key player, activated by initiator caspases and activating the caspase-activated DNase (CAD) for DNA breakdown. Executioner caspases also drive cytoskeletal reorganization and apoptotic body formation (Wu and Bratton, 2011; Dorstyn et al., 2018).
4.1.2 Small Hsps and apoptosis
The mechanism by which sHsps regulate apoptosis involves their association with key stress signalling and apoptotic molecules, thereby blocking cell death and promoting cell survival, proliferation, or differentiation (Mehlen et al., 1996; Ikwegbue et al., 2018). Hsps post-translational modifications and/or their presence in a given cellular compartment are important variables in determining the interaction of an sHsp with a given apoptotic partner which influences the outcomes on signalling pathway activation or repression. The role of sHsps in various diseases and their involvement in apoptotic events are summarised in Table 2.
4.1.2.1 Inhibition of pro-apoptotic proteins
The sHsps play a regulatory role in apoptosis by suppressing the activation of pro-apoptotic proteins through three different strategies. The first strategy is used by HspB5, HspB1, and HspB2 which exert their potent anti-apoptotic effects by directly interacting with pro-apoptotic signalling proteins. sHsp interact with Bcl-2 family of proteins and interfere with their functions thereby preventing permeabilization of the mitochondrial membrane (Bruey et al., 2000) and apoptosome formation (Beere, 2004; Breckenridge and Xue, 2004). By activating survival pathways such as Akt and ERK (Palmieri et al., 2017; Sun et al., 2017), sHsps promote the phosphorylation and inactivation of pro-apoptotic proteins like Bad, effectively neutralizing their apoptotic potential. Furthermore, some sHsps such as HspB5 can enhance the activity of NF-κB, a transcription factor that governs the expression of anti-apoptotic genes (Llambi et al., 2011; Pécot et al., 2016). This leads to an increase in the levels of anti-apoptotic proteins like Bcl-2, further increasing the cell’s resistance to apoptosis.
The second strategy is where some sHsps inhibit both pro-inflammatory and pro-apoptotic caspases by binding directly to them and suppressing caspase activation (Kamradt et al., 2005; Ikwegbue et al., 2018). This regulatory mechanism occurs at multiple levels, each contributing to the modified control of cell survival (Figure 4). HspB1, for instance, interacts with pro-caspase 3, inhibiting its maturation into the active caspase. This inhibition curtails the downstream effector apoptotic events. Similarly, HspB5 has been identified as a negative regulator of the extrinsic apoptotic pathway, specifically inhibiting caspase 3 activation (Kamradt et al., 2002). By disrupting the recruitment of these initiator caspases to death receptor complexes, HspB5 impedes their activation and the subsequent activation of downstream apoptotic effectors.
Another key strategy employed by sHsps is the direct sequestration of cytochrome c, a crucial factor in the initiation of caspase activation (Bruey et al., 2000). Upon release from mitochondria into the cytosol, cytochrome c is inhibited by HspB1, preventing its engagement with caspases. The interaction between HspB1 and cytochrome c is intricate and necessitates the dimerization of HspB1 (Paul et al., 2002). This engagement not only inhibits the direct activation of caspases by cytochrome c but also shields cytochrome c from promoting downstream apoptotic signals. Moreover, HspB1 extends its influence on actively obstructing caspase 3, a key executioner caspase, in its active state. This binding prevents the detrimental impact of active caspase 3 on cellular components and processes crucial for apoptosis execution (Bruey et al., 2000; Paul et al., 2002). For instance, in monocytes undergoing apoptosis, HspB1 binds to and effectively suppresses the activity of caspase 3, providing an additional layer of protection against premature cell death (Paul et al., 2002).
4.1.2.2 Regulation of protein folding and repair
sHsps play a role in protein folding, repair, and refolding of misfolded peptides, which can prevent the accumulation of damaged proteins that may trigger apoptosis. HspB1 as a notable example, it plays a pivotal role in regulating apoptosis by controlling protein folding and repair processes (Shan et al., 2021). HspB1’s interactions with clients guide apoptotic proteins toward correct folding, preventing misfolded proteins from triggering erroneous signals that could lead to cell death (Webster et al., 2019). Additionally, sHsps act as safeguards during cellular stress, forming complexes with malfunctioning proteins to prevent aggregation and disruption of cellular functions (Treweek et al., 2014), thereby averting inadvertent activation of apoptotic pathways. HspB1’s involvement extends to enveloping misfolded proteins, facilitating their restoration to functional states and curbing their accumulation. By facilitating substrate transfer to the main foldases, Hsp70 and Hsp90, sHsps ensure timely repair of misfolded or damaged proteins, particularly crucial in thwarting signals that could initiate cell death (Wegele et al., 2004; van Ommeren et al., 2016). This intricate regulation underscores sHsps’ role in preserving cellular equilibrium and offers potential avenues for modulating apoptotic pathways therapeutically.
4.1.2.3 sHsp in oxidative stress regulation
In reducing oxidative damage, sHsps scavenge reactive oxygen species (ROS) generated during oxidative stress, thereby suppressing apoptosis [reviewed in Ikwegbue et al. (2018)]. sHsps, particularly HspB1 and HspB5, exhibit direct antioxidant activity by scavenging ROS and reactive nitrogen species (RNS) (Arrigo et al., 2005). These harmful molecules, generated as by-products of cellular metabolism or due to environmental factors, can damage lipids, proteins, and DNA, ultimately leading to apoptosis. The main mechanism through which sHsps combat oxidative stress, is by binding to ROS molecules, such as hydrogen peroxide (H2O2), hydroxyl radicals (OH), and superoxide radicals (O2) (Huot et al., 1996; Wu et al., 2015). This interaction helps neutralize the reactivity of these species, reducing their potential to cause cellular damage. By scavenging ROS, sHsps maintain the cellular redox balance and protect cells from oxidative stress-induced apoptosis.
Furthermore, sHsps play a crucial role in regulating apoptosis by modulating the intracellular redox state (Christians et al., 2012). Oxidative stress, characterized by an imbalance between ROS production and the antioxidant defence system, can trigger apoptotic pathways. HspB1 and HspB5 stabilize glutathione levels preventing excessive ROS accumulation, in this way, sHsps help mitigate oxidative damage to cellular components (Préville et al., 1999; Xiong et al., 2020). Additionally, sHsps' ability to interact with and stabilize mitochondrial proteins, such as those involved in the electron transport chain, contributes to reducing ROS generation (Arrigo, 2007). By maintaining a balanced redox environment, sHsps inhibit the activation of pro-apoptotic signalling pathways that often arise from oxidative stress, thereby promoting cell survival and suppressing apoptosis.
Given that the intrinsic pathway of apoptosis is mitochondrial dependent and most of the P. falciparum sHsp are localized within the mitochondria, it is plausible to suggest that these proteins may serve a physiological role similar to their human counterparts in regulating apoptosis. In addition, some of the antimalarial drugs, such as chloroquine, target parasite redox homeostasis generating ROS that ultimately cause parasite death (Akide-Ndunge et al., 2009; Nepveu and Turrini, 2013; Coertzen et al., 2018; Siddiqui et al., 2022). The capability of P. falciparum to regulate the oxidative stress, herald the development of drug resistance development (Lehane et al., 2012; Rosenthal and Ng, 2020). It is tempting to speculate that sHsps could play an important role in antimalarial drug resistance.
4.1.2.4 Phosphorylation of sHsps
The post translational modification of sHsps modulates their function. For instance, phosphorylation of sHsps has been implicated in their regulation of apoptosis and other cellular functions (Janowska et al., 2019). Rapid phosphorylation of sHsps, particularly on serine residues after stress, affect their chaperone function prior to increased protein expression over time (Kostenko and Moens, 2009). Phosphorylation can impact the oligomerization state of sHsps, influencing whether they form large oligomers or exist as smaller oligomers or monomers. The oligomerization state is also a functional regulatory mechanism modulating the chaperone activities of sHsps [reviewed in Haslbeck et al. (2019)]. Phosphorylation-induced changes in oligomerization might alter their affinity for client proteins and their ability to prevent protein aggregation (Janowska et al., 2019; Liu et al., 2020).
In their phosphorylated state, sHsps, exhibit differential capability to interact with other proteins involved in apoptosis regulation. For example, the phosphorylated form of HspB1 directly interacts with Daxx, a protein that connects Fas signalling to apoptosis, inhibiting its interaction with Ask1 and Fas proteins, thus blocking apoptosis triggered by their co-expression (Charette and Landry, 2000). This inhibition is influenced by the phosphorylation and oligomerization status as only dimeric phosphorylated HspB1 is capable of binding Daxx and preventing apoptosis. Interestingly, different triggers like heat, oxidative stress, growth factors, and cytokines causes HspB1 to become phosphorylated by MAPKAP kinase 2, which is activated by MAP kinase SAKP2/p38 (Guay et al., 1997; Kostenko et al., 2009). This phosphorylation changes its structure from large homo-oligomers to dimers and monomers. Stress induced phosphorylation of sHsp provides protection against diverse harmful agents and results in their translocation into the nucleus (Liu et al., 2020). In this state, HspB5 and Hsp25 interact with nuclear components, such as splicing factors and the intracellular lamin A/C (Beere, 2004). This implicates the sHsps’ involvement in maintaining nuclear stability and potentially influencing apoptotic signalling pathways originating from the nucleus.
In addition, phosphorylation of sHsps can also impact signalling pathways involved in apoptosis regulation (Figure 4). For example, phosphorylated HspB1 activates the pro-survival Akt pathway, which in turn affects apoptotic responses by inhibiting caspase activity and phosphorylating pro-apoptotic proteins such as Bad (Song et al., 2005). Moreover, the phosphorylation of HspB1 enhances its interactions with kinases like MK2 and p38-MAPK (Kostenko et al., 2009), leading to activation of these pathways and downstream protective effects. This intricate crosstalk demonstrates how phosphorylated sHsps actively participate in orchestrating key events in cell survival and apoptotic signalling cascades. Furthermore, the chaperone activity of sHsps, which is important for their ability to prevent protein misfolding and aggregation is dependent on their regulation state (Rogalla et al., 1999; Ecroyd et al., 2007). Phosphorylation can either enhance or inhibit the chaperone function of sHsps, depending on the specific phosphorylation sites. Phosphorylated HspB1, for instance, binds to and stabilizes actin, preventing its depolymerization under thermal stress (Ammendola et al., 2021). This preservation of the cytoskeletal structure is crucial for preventing cytochrome c release from mitochondria and subsequent caspase activation. The phosphorylation-induced chaperone activity of sHsps is thus an essential component of their anti-apoptotic function, contributing to the overall cellular response to stress-induced apoptotic stimuli (Mounier and Arrigo, 2002; Povea-Cabello et al., 2017).
Apoptosis has been observed in both multicellular organisms and in unicellular eukaryotes, including P. falciparum. Like mammalian apoptosis, the apoptosis in P. falciparum appears to be an active and protein synthesis-dependent mechanism (Meslin et al., 2011; Sena-Dos-santos et al., 2021) and shows typical apoptotic features such as chromatin condensation, DNA fragmentation, and the formation of apoptotic bodies (Mohapatra et al., 2022). These apoptotic-like features are displayed by the malaria parasite during its lifecycle, particularly during the differentiation of gametocytes into gametes within the mosquito midgut (Kumari et al., 2022). A global proteomic analysis study revealed that P. falciparum increases the production of stress-related proteins, including Hsp70, as well as components of the translation machinery (Rathore et al., 2015). This suggests that the parasite is actively trying to combat the stress, demonstrating its mechanisms to adapt and overcome the challenges posed by proteasome inhibition. However, unlike humans, Plasmodium parasites lack the caspase genes responsible for cell death regulation. Nevertheless, several studies have identified metacaspases (MCA) in P. falciparum parasites, which possess a catalytic domain that bears resemblance to caspases (Meslin et al., 2011; Kumar et al., 2019; Vandana et al., 2020). This similarity has been confirmed that metacaspases are key players involved in cell death through apoptotic-like pathways (Vercammen et al., 2007; Jiang and Wang, 2004; Kumar et al., 2019). Similar to caspases, metacaspases’ activity depends on a functional catalytic domain generated through auto processing, mimicking caspase activation and also function as effectors of cell death (Coll et al., 2010; Zalila et al., 2010). In these organisms, metacaspases have been shown to undergo autoprocessing, leading to the removal of the prodomain, which is likely essential for their cell death function (Meslin et al., 2011). P. falciparum genome contains three metacaspase genes: PfMCA1, PfMCA2, and PfMCA3. PfMCA1 is the only one with the necessary histidine and atypical catalytic dyad with serine in place of cysteine (Meslin et al., 2011; Kumar et al., 2019). When PfMCA1 is expressed in vitro, it undergoes auto-processing, leading to the removal of the pro-domain (Meslin et al., 2011). This auto-processing and release of the catalytic domain of PfMCA1 likely play a vital role in its cell death function in the parasite. However, the PfMCA3 was shown to exhibit a unique activity similar to trypsin and not caspases like activity (Kumar et al., 2019). On the other hand, PfMCA2 was shown that its inhibition promotes apoptosis (Shankar et al., 2020). These findings suggest that parasite metacaspases may modulate cell death processes through unique pathways that still needs to be elucidated.
Interestingly, like other intracellular obligate protozoan, P. falciparum have evolved strategies to avoid direct destruction by the host’s immune system. However, the infected red blood cells that initiate apoptosis as a defence mechanism, express surface receptors such as P. falciparum glutamic-acid-rich protein (PfGARP) that are recognised by macrophages (Mohapatra et al., 2022). These apoptotic red cells are then engulfed by macrophages, potentially eliminating both the parasite and the infected red blood cell (Al-Olayan et al., 2002; Raj et al., 2020). This powerful defence mechanism exerts selective pressure on parasites leading them to develop strategies to manipulate the host red cell’s apoptotic signalling in their favour. This comes at the backdrop that, several protozoan parasites including P. falciparum have been reported to inhibit the apoptotic signalling pathway of the host cell to ensure their survival (Al-Olayan et al., 2002; Viriyavejakul et al., 2014). It still remains to be validated if parasite sHsps modulate host cell death processes such as apoptosis to ensure their survival.
4.2 Small heat shock proteins and autophagy
Autophagy is a fundamental cellular process responsible for the degradation and recycling of cellular constituents. This cellular process known as "self-eating," plays a pivotal role in maintaining cellular homeostasis and supplying energy during periods of nutrient scarcity or stress. Autophagy encompasses three distinct types in eukaryotic cells: macroautophagy, microautophagy, and chaperone-mediated autophagy (CMA) (Locatelli and Cenci, 2022). While both macroautophagy and microautophagy involve membrane rearrangement for sequestering cytoplasmic material, they differ in the site of sequestration (Figure 5). Macroautophagy forms a double-membrane vesicle, the autophagosome, which likely involves the endoplasmic reticulum (ER), Golgi complex, and/or mitochondria. This autophagosome then fuses with the lysosome or vacuole, delivering its contents (Yorimitsu and Klionsky, 2005). On the other hand, microautophagy directly engulfs cytosolic components into the lysosome or vacuole through invagination and scission of the organelle’s limiting membrane. In contrast, CMA does not entail significant membrane rearrangement, but rather relies on the lipid composition of the lysosome membrane (Yang et al., 2019), Hsps, both in the cytosol and within the lysosome lumen, along with the lysosome-associated membrane protein (LAMP)-2a (Cuervo et al., 2004; Massey et al., 2006). CMA involves the translocation of unfolded proteins across the lysosome’s limiting membrane and requires the involvement of the molecular chaperones such as Hsc70. These Hsc70 identifies misfolded substrates and directs them to the lysosome, where they are unfolded and translocated across the lysosomal membrane for degradation (Baaklini et al., 2020).
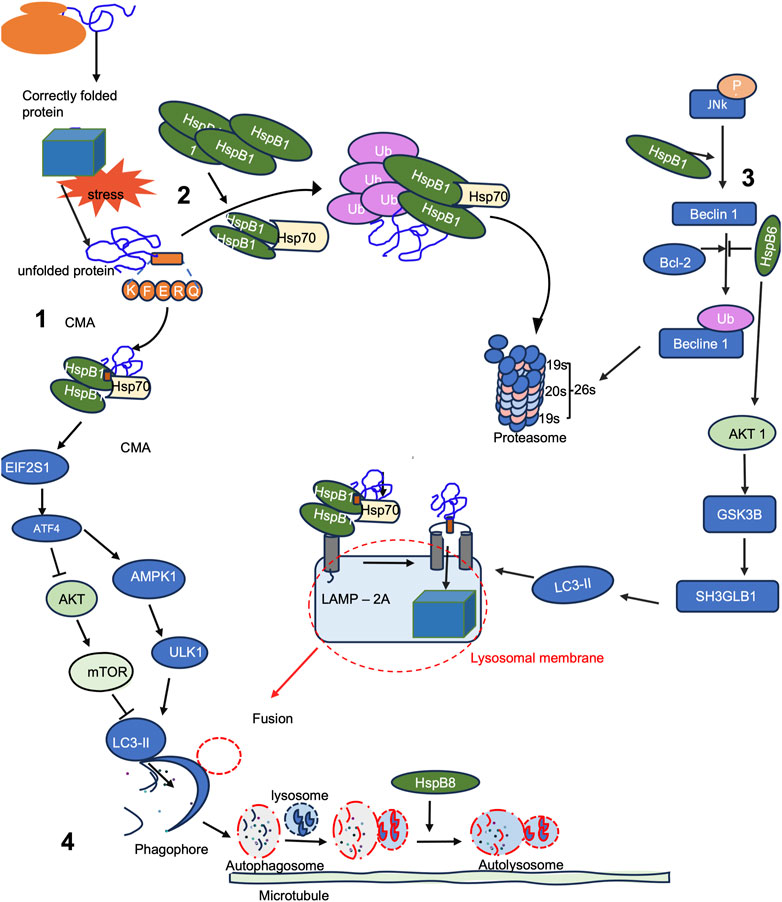
FIGURE 5. sHsps are involved in the activation and progression of autophagy. (1) Chaperone-mediated autophagy (CMA), and (2) the ubiquitin/proteasome system are key pathways for oxidized protein degradation. (3) Opposing effects of sHsp in the JNK pathways leads to beclin ubiquitylation and degradation, whilst sHsps activates AKT1 pathway that results in the formation of lysosomal membrane complexes with Hsp70 and LC3-II. (4) Both CMA and JNK pathways converge with effector signalling by fusion to form autophagosome from the fusion of phagophore and lysosome. The formation of the autolysosome is mediated by sHsps.
Increasing evidence has illuminated the multifaceted roles of autophagy in different aspects of human health. The core components of the mammalian autophagic machinery are homologous to the autophagy-related genes (Atg) initially discovered in yeast (Mizushima et al., 2008; Li et al., 2020). Starvation, a potent inducer of autophagy, inhibits the mammalian target of rapamycin (mTOR), a component of the macromolecular complex mTORC1 (Figure 5). mTORC1 negatively regulates another complex involving Unc-51 like autophagy activating kinase 1 (ULK1), ATG13, ATG101, and FAK Family Kinase-Interacting Protein Of 200 kDa (FIP200), which is activated upon nutrient depletion or energy exhaustion, initiating autophagy (Kim et al., 1998; Zhang et al., 2019). The Beclin 1-interacting complex acts as a regulatory platform for autophagy, comprising Beclin 1, BCL-2 family proteins (which inhibit autophagy), class III phosphatidylinositol 3-kinase (VPS34), and ATG14L (He and Levine, 2010). Activation of this complex leads to the production of phosphatidylinositol-3-phosphate, promoting the nucleation of autophagosomal membranes. Autophagosomal elongation requires two ubiquitin-like conjugation systems: ATG5-ATG12 and LC3-ATG8. The conversion of LC3-I to its autophagosomal membrane-associated form, LC3-II, serves as a marker for autophagosome formation (Ravikumar et al., 2010).
Small Hsps have emerged as key regulators of autophagy and protein quality control. Some sHsps in humans, such as HspB1, HspB5, and HspB2, are implicated as significant regulators of autophagic processes in cells by interacting with key autophagy-related proteins (De Maio et al., 2019). For instance, it was reported that over expression of HspB1 induced autophagy and autophagic flux in rat renal (kidney) proximal tubular NRK-LC3 cells (Matsumoto et al., 2015). Hamouda et al. (2014) demonstrated that HspB8 confers resistance to bortezomib, a proteasome inhibitor, by promoting autophagy-mediated clearance of misfolded proteins (Figure 5). Moreover, the enhanced clearance of aggregates through the autophagy pathway was found to re-sensitize bortezomib-resistant myeloma cells to proteasome inhibitors, thereby improving cell viability. The expression levels of sHsps have an effect on the role they play in autophagy (Li et al., 2018). For example, in cardiac myocytes, low HspB8 levels lead to enlarged cell size by activating phosphoglucomutase and inhibiting casein kinase 2, an anti-apoptotic protein (Hamouda et al., 2014). Conversely, elevated HspB8 levels promote apoptosis. HspB8’s interaction with Bag3 forms a complex involving Hsc70 and CHIP, triggering autophagy to clear misfolded proteins (Cheng et al., 2023). This complex also prompts eIF2α phosphorylation, inhibiting protein synthesis and enhancing macroautophagy (Humeau et al., 2020). Depending on the cellular context, autophagy either safeguards against death by clearing damaged organelles and providing energy substrates or leads to self-destructive cell outcomes via excessive autophagic activity. Additionally, autophagy can activate apoptotic or necrotic cell death pathways through shared regulators, like Bcl-2 family proteins.
In P. falciparum, the autophagic pathway has been identified as a process similar to the parasite’s apoptosis under drug pressure. The erythrocytic stages of the parasite were observed to undergo cell death through autophagy-like mechanism (Gaviria et al., 2013). Immunofluorescence and electron microscopic analysis of P. falciparum and P. vivax parasites treated with either chloroquine or artemisinin (Gaviria et al., 2013; Singh and Chakraborty, 2016) showed the presence of vacuoles with single or double membranes, resembling autophagosomes (Ray et al., 2022). However, the canonical autophagy pathway in P. falciparum uses different upstream signalling molecules unlike other organisms, certain upstream kinases responsible for detecting nutrient limitation and inducing autophagy are thought to be absent in the parasite (Cervantes et al., 2014; Usman et al., 2023). Consistent with this, mild nutrient starvation or inhibitors of key upstream kinases did not induce autophagy in P. falciparum. However, downstream proteins involved in autophagy were detected and appear to be functional in the parasite. Several PfATG proteins involved in autophagosome formation, retrieval, and vesicle breakdown were identified (Cervantes et al., 2014). Notably, PfATG8 and PfATG18, critical proteins in the autophagy process, were found to be associated with vesicles throughout the asexual and sexual erythrocytic cycle (Agrawal et al., 2019). These vesicles were also observed within the host red blood cells, suggesting the involvement of a complex export system in transporting parasite proteins to the host cell membrane (Brennand et al., 2011; Duszenko et al., 2011). Despite the accumulating evidence of autophagic processes in P. falciparum, the role of sHsps remains unexplored. Understanding the molecular mechanisms by which sHsps regulate autophagy in the parasite holds the potential for the development of novel malaria therapies.
4.3 Role of small heat shock proteins in necrosis
Necrosis is a form of cell death occurring as a result of prolonged strong cell stress which is associated with the loss of plasma membrane integrity and subsequent leakage of the cytoplasmic material. In some cases, necrosis is secondary to other cell death processes such as late stages of apoptosis and autophagy [reviewed in Green and Llambi (2015)]. Due to these passive processes, necrosis is generally considered as accidental cell death. However, there are some cases where signalling pathways play a role in active necrotic cell death “necroptosis” (Degterev et al., 2008). Necroptosis is not fully understood but is thought to be a receptor-interacting protein (RIP)-kinase dependent cell death process that is triggered by Toll-like receptor (TLR)-3, TRL-4 and/or TNFRI (Llambi et al., 2011; Green and Llambi, 2015; Yu et al., 2021). These death cell receptors activate the kinase activity of RIP3 to recruit mixed lineage kinase domain-like (complex II) facilitated by RIP1 (de Almagro and Vucic, 2015). Complex II when phosphorylated by RIP3 facilitate the plasma membrane disruption and subsequent cell lysis. The increased levels of ROS from mitochondrial damage, NADPH oxidase 1, increase in calcium influx and ATP depletion are thought to be initiating signals for necroptosis. Necroptosis is also thought to initiate inflammatory responses from the released intracellular material and damage associated molecular patterns (DAMP). In addition, RIP3 was shown to induce the cleavage and maturation of highly inflammatory cytokines interleukin –(IL)-1β and IL-18 mediated by NOD–LRR- and pyrin domain containing protein 3 (NLRP3) inflammasome (Vince et al., 2012; Weinlich et al., 2017).
The complications of malaria in the liver, lung, and brain are due to neutrophil infiltration and activation contributing to tissue necrosis (Rocha et al., 2015; Feintuch et al., 2016; de Menezes et al., 2019). In another study, it was observed that during acute P. chabaudi malaria, the liver recruits IL-1α-producing neutrophils as part of the immune response to infected red blood cells in the liver vasculature (de Menezes et al., 2019). This causes the neutrophils in the infected liver to upregulate IL-1α production at both mRNA and protein levels. The recruitment of IL-1α-producing neutrophils to the liver amplifies the inflammatory response through IL-1R signalling, leading to the local secretion of pro-inflammatory cytokines (Gross et al., 2012; de Menezes et al., 2019). The local release of IL-1α promotes TNF-α production, leading to hepatocyte death and the development of necrotic lesions (Basu et al., 2010). This excessive production of pro-inflammatory cytokines, including TNF-α, contributes to the immunopathology of malaria and is associated with disease severity (Nussler et al., 1993). Additionally, TNF-α and NO are involved in the loss of infectivity of circulating gametocytes. TNF-α, together with IFN-γ, optimizes nitric oxide (NO) production for parasite clearance and resolution of fever (Artavanis-Tsakonas and Riley, 2002). Some Hsps play a role in modulating necrotic cell death. For example, HspB1 is involved in regulating necrosis. TNF-α treatment leads to the rapid phosphorylation of HspB2, a homolog of HspB1, independent of C kinase (Rogalla et al., 1999). This phosphorylation is important in mediating HspB1’s interaction with IKK-γ and suppressing TNF-α-induced NF-κB activation (Saklatvala et al., 1991). Also, Hsp90 exerts influence over key proteins within the RIP1/RIP3/MLKL pathway by modulating their stability, phosphorylation, and expression. Hsp90 is involved in the regulation of both RIP3 and MLKL, shaping their roles in necroptosis. The exact effects of Hsp90 inhibitors may differ, and prolonged inhibition can result in the degradation of these proteins (Jacobsen et al., 2016).
When comparing necrosis in mammals to that in Plasmodium infection, it becomes evident that in mammals, necrosis arises under pathological conditions with stronger or prolonged stimuli. However, in Plasmodium infection, tissue necrosis is an unfortunate complication that is primarily driven by the inflammatory response against parasite infection (Hui et al., 2023). This results in the release of pro-inflammatory cytokines such as TNF-α and IL-1α (Porter et al., 2008). These cytokines contribute to cell death. Similarly, P. vivax infection has been reported to cause renal cortical (Nair et al., 2019), intestinal (Pawelka et al., 2021), necrosis. Despite the lack of data on the role of endogenous parasite sHsps in parasite death, the potential role of sHsps in host tissue necrosis highlights the complex interplay between the host’s immune response and tissue damage.
5 Future perspectives
5.1 Small Hsp as immunomodulants
Hsps exhibit immunomodulatory functions, making them attractive candidates for vaccines (Miroshnichenko et al., 2005; Zininga et al., 2018) specifically, sHsps plays a role in immunomodulation through their participation in antigen presentation (AP), a critical step in activating adaptive immune responses (Li et al., 2002; Miroshnichenko et al., 2005). AP facilitates peptide binding and delivery to major histocompatibility complex (MHC) molecules (Li et al., 2002). This interaction enhances antigen processing and presentation, leading to the activation of T cells and subsequent immune responses. Furthermore, sHsps have been found to modulate inflammatory responses by regulating the activity of pro-inflammatory cytokines and chemokines (Van Noort et al., 2012; Powell et al., 2021). For instance, sHsps have been implicated in enhancing the functions of immune cells. There have been reports that Hsp70 enhances the cytotoxic activity of natural killer (NK) cells against tumor cells and parasite infected RBCs through perforin and granzyme B dependent cell lysis (Lv et al., 2012; Zininga and Shonhai, 2019). This highlights the potential of sHsps, although not shown to be surface exposed on RBCs to enhance innate immune responses and contribute to antiparasitic immunity. Due to their immunomodulatory properties, sHsps also have the potential to serve as adjuvants in malaria vaccine formulations to boost vaccine efficacy.
5.2 Small Hsps as diagnostic markers
sHsps have also shown promise as diagnostic markers for various diseases. The unique expression patterns and alterations of sHsps in diseased conditions make them attractive candidates for diagnostic purposes (Yu et al., 2010; Morrow and Tanguay, 2012; Wyciszkiewicz et al., 2019). Aberrant expression of sHsps has been observed in different types of cancer, and their levels can serve as indicators of disease progression and prognosis. High HspB1 expression levels are associated with advanced tumour stages, metastasis, and poor patient outcomes. Moreover, sHsps have been explored as diagnostic markers in cardiovascular diseases (Bleijerveld et al., 2013) and neurodegenerative disorders [reviewed in Navarro-zaragoza et al. (2021)]. Differential life-stage specific expression levels and localization of sHsps in malaria could potentially aid in the early diagnosis and monitoring of malaria. In addition, the low level of conservation between the human and malarial sHsp, offers promise to selective targeting of parasite Hsps as diagnostic markers. There is need for further validation studies to fully establish the clinical utility of sHsps as diagnostic markers in malaria.
5.3 Small Hsps as drug target
Small Hsps also serve as attractive drug targets due to their involvement in various disease processes. The unique structural and functional characteristics of sHsps among the human host and the parasite make them valuable targets for therapeutic intervention. The sHsp potential as drug targets in cancer and other diseases heighten the need for exploration as targets for novel antimalarial drugs. The overexpression of certain Hsps, such as sHsp and Hsp70, has been associated with cancer progression, chemotherapy resistance, and poor patient outcomes (Acunzo et al., 2012). By targeting sHsps, it is possible to disrupt their pro-survival and anti-apoptotic functions, thereby sensitizing cancer cells to chemotherapy or radiation therapy. Several approaches have been investigated, to repurpose some of the anti-Hsp cancer drugs into malaria treatment options including the development of small molecule inhibitors that specifically bind to Hsps and disrupt their interactions with client proteins (Zininga and Shonhai, 2019; Stofberg et al., 2021). Beyond general inhibitors of the heat shock response, there is a need to develop inhibitors that target specific sHsps, particularly HspB1 and HspB5. Peptide aptamers that interact specifically with HspB1 have been proposed as potential inhibitors (Gibert et al., 2013). In cell cultures, these aptamers disrupted the phosphorylation and oligomerization of HspB1, weakening its anti-apoptotic and cytoprotective property (Gibert et al., 2013; Yoon and Rossi, 2018). In addition, the small molecule inhibitor (E)-5-(2-Bromovinyl)-2-Deoxyuridine (RP101) that targets HspB1 in cancer therapy (Seul-Ki et al., 2019), offers promise for repurposing to malaria. RP101 is a compound that counteracts HspB1’s functions, hindering gene amplification and enhancing cell death (Fahrig et al., 2006; Heinrich et al., 2011). Clinical studies involving RP101 have shown promise in extending the survival of pancreatic cancer patients (Heinrich et al., 2011; de Gooijer et al., 2021). RP101 acts as a chemo-sensitizing agent, effectively countering resistance and amplifying the impact of several chemotherapeutic medications such as mitomycin, gemcitabine, cisplatin, and cyclophosphamide (Xiong et al., 2020; Yang et al., 2021). Similarly, another drug, quercetin, a naturally occurring bioflavonoid derived from plants, exhibit chemo sensitising capabilities through inhibition of the expression of heat shock factor-1 (HSF-1) and its binding to the heat shock element in DNA (Wang et al., 2018; Vafadar et al., 2020). Quercetin augments the efficacy of primary chemotherapeutic agents like doxorubicin, gemcitabine, 5-fluorouracil, and cisplatin. Interestingly, beyond inhibiting HspB1 expression, quercetin also inhibits HspB1’s function by impeding its phosphorylation within cancer stem cells (CSCs) (Chen et al., 2012). Considering the WHO guidelines on malaria treatment that combination therapeutics are the first line options in several countries, the chemo sensitization of RP101 and quercetin has the potential to be the “silver bullet” in reversing drug resistance to common antimalarial therapeutics.
6 Conclusion
In conclusion, sHsps play crucial roles in the stress response and survival strategies of P. falciparum, the causative agent of malaria. These proteins are involved in protein folding, translocation, and the formation of protein complexes, facilitating the parasite’s adaptation and interactions with the host. Comparing the structure and function of P. falciparum sHsps to their human counterparts provides valuable insights into their potential as druggable targets. Additionally, the ability of sHsps to modulate antigen presentation, regulate inflammatory responses, and enhance immune cell functions highlights their potential in designing effective vaccines. Furthermore, sHsps show promise as diagnostic markers for malaria due to their altered stage specific expression patterns and unique structure that can be targeted by antibodies used in diagnostic kit development. Lastly, targeting sHsps opens new possibilities for drug development, in antimalarial treatments from repurposed and further improved cancer therapeutics. Disrupting the functions of sHsps can sensitize P. falciparum to current antimalarials in efforts to roll back malaria.
Author contributions
FT: Investigation, Visualization, Writing–original draft. TZ: Conceptualization, Funding acquisition, Investigation, Project administration, Resources, Supervision, Visualization, Writing–review and editing.
Funding
The authors declare financial support was received for the research, authorship, and/or publication of this article. This work is based on the research supported in part by the Department of Science and Technology/National Research Foundation (NRF) of South Africa (Grant Numbers 129401 and 145,405) awarded to TZ; Mandela-Rhodes scholarship awarded to FT.
Acknowledgments
The authors would like to acknowledge Stellenbosch University Sub-Committee B and the ECAD and the Department of Higher Education and Training (DHET) Future Professors Programme fellowship for support to TZ. We thank Karli Bothma for critical reading of this manuscript. We are grateful to Frontiers for waiver of the Article processing charges for this publication.
Conflict of interest
The authors declare that the research was conducted in the absence of any commercial or financial relationships that could be construed as a potential conflict of interest.
Publisher’s note
All claims expressed in this article are solely those of the authors and do not necessarily represent those of their affiliated organizations, or those of the publisher, the editors and the reviewers. Any product that may be evaluated in this article, or claim that may be made by its manufacturer, is not guaranteed or endorsed by the publisher.
References
Acharya, P., Chandran, S., Chakravarti, H., Middha, S., Acharya, J., Kochar, S., et al. (2009). A glimpse into the clinical proteome of human malaria parasites Plasmodium falciparum and Plasmodium vivax. Wiley Online Libr. 3 (11), 1314–1325. doi:10.1002/prca.200900090
Acunzo, J., Katsogiannou, M., and Rocchi, P. (2012). Small heat shock proteins HSP27 (HspB1), αB-crystallin (HspB5) and HSP22 (HspB8) as regulators of cell death. Int. J. Biochem. Cell. Biol. 44 (10), 1622–1631. doi:10.1016/J.BIOCEL.2012.04.002
Agrawal, P., Manjithaya, R., and Surolia, N. (2020). Autophagy-related protein PfATG18 participates in food vacuole dynamics and autophagy-like pathway in Plasmodium falciparum. Mol. Microbiol. 113, 766–782. doi:10.1111/mmi.14441
Akide-Ndunge, O. B., Tambini, E., Giribaldi, G., McMillan, P. J., Müller, S., Arese, P., et al. (2009). Co-ordinated stage-dependent enhancement of Plasmodium falciparum antioxidant enzymes and heat shock protein expression in parasites growing in oxidatively stressed or G6PD-deficient red blood cells. Malar. J. 8 (1), 113. doi:10.1186/1475-2875-8-113
Albakova, Z., Armeev, G. A., Kanevskiy, L. M., Kovalenko, E. I., and Sapozhnikov, A. M. (2020). HSP70 multi-functionality in cancer. Cells 9 (3), 587. doi:10.3390/CELLS9030587
Al-Olayan, E. M., Williams, G. T., and Hurd, H. (2002). Apoptosis in the malaria protozoan, Plasmodium berghei: a possible mechanism for limiting intensity of infection in the mosquito. Int. J. Parasitol. 32 (9), 1133–1143. doi:10.1016/S0020-7519(02)00087-5
Ammendola, R., Parisi, M., Esposito, G., and Cattaneo, F. (2021). Pro-resolving FPR2 agonists regulate NADPH oxidase-dependent phosphorylation of HSP27, OSR1, and MARCKS and ctivation of the respective upstream kinases. Antioxidants (Basel, Switz. 10 (1), 134–222. doi:10.3390/ANTIOX10010134
Arrigo, A. P. (2005). In search of the molecular mechanism by which small stress proteins counteract apoptosis during cellular differentiation. J. Cell. Biochem. 94 (2), 241–246. doi:10.1002/JCB.20349
Arrigo, A. P. (2007). The cellular “networking” of mammalian Hsp27 and its functions in the control of protein folding, redox state and apoptosis. Adv. Exp. Med. Biol. 594, 14–26. doi:10.1007/978-0-387-39975-1_2
Arrigo, A. P., Virot, S., Chaufour, S., Firdaus, W., Kretz-Remy, C., and Diaz-Latoud, C. (2005). Hsp27 consolidates intracellular redox homeostasis by upholding glutathione in its reduced form and by decreasing iron intracellular levels. Antioxidants Redox Signal. 7 (3–4), 414–422. doi:10.1089/ARS.2005.7.414
Arrigo, A. P., and Welch, W. J. (1987). Characterization and purification of the small 28,000-dalton mammalian heat shock protein. J. Biol. Chem. 262 (32), 15359–15369. doi:10.1016/S0021-9258(18)47733-2
Artavanis-Tsakonas, K., and Riley, E. M. (2002). Innate immune response to malaria: rapid induction of IFN-gamma from human NK cells by live Plasmodium falciparum-infected erythrocytes. J. Immunol. Baltim. Md, 1950) 169 (6), 2956–2963. doi:10.4049/JIMMUNOL.169.6.2956
Baaklini, I., Gonçalves, C. de C., Lukacs, G. L., and Young, J. C. (2020). Selective binding of HSC70 and its Co-chaperones to structural hotspots on CFTR. Sci. Rep. 10 (1), 1–12. doi:10.1038/s41598-020-61107-x
Balana, A. T., Levine, P. M., Craven, T. W., Mukherjee, S., Pedowitz, N. J., Moon, S. P., et al. (2021). O-GlcNAc modification of small heat shock proteins enhances their anti-amyloid chaperone activity. Nat. Chem. 13 (5), 441–450. doi:10.1038/s41557-021-00648-8
Basu, M., Maji, A. K., Chakraborty, A., Banerjee, R., Mullick, S., Saha, P., et al. (2010). Genetic association of Toll-like-receptor 4 and tumor necrosis factor-alpha polymorphisms with Plasmodium falciparum blood infection levels. Infect. Genet. Evol. 10 (5), 686–696. doi:10.1016/J.MEEGID.2010.03.008
Beere, H. M. (2004). “The stress of dying”: the role of heat shock proteins in the regulation of apoptosis. J. Cell. Sci. 117 (Pt 13), 2641–2651. doi:10.1242/JCS.01284
Bellyei, S., Szigeti, A., Pozsgai, E., Boronkai, A., Gomori, E., Hocsak, E., et al. (2007). Preventing apoptotic cell death by a novel small heat shock protein. Eur. J. Cell. Biol. 86 (3), 161–171. doi:10.1016/J.EJCB.2006.12.004
Biebl, M. M., Lopez, A., Rehn, A., Freiburger, L., Lawatscheck, J., Blank, B., et al. (2021). Structural elements in the flexible tail of the co-chaperone p23 coordinate client binding and progression of the Hsp90 chaperone cycle. Nat. Commun. 12 (1), 828. doi:10.1038/S41467-021-21063-0
Blatch, G. L. (2022). Plasmodium falciparum molecular chaperones: guardians of the malaria parasite proteome and renovators of the host proteome. Front. Cell. Dev. Biol. 10, 921739. doi:10.3389/fcell.2022.921739
Bleijerveld, O. B., Zhang, Y. N., Beldar, S., Hoefer, I. E., Sze, S. K., Pasterkamp, G., et al. (2013). Proteomics of plaques and novel sources of potential biomarkers for atherosclerosis. PROTEOMICS – Clin. Appl. 7 (7–8), 490–503. doi:10.1002/PRCA.201200119
Boelens, W. C. (2020). Structural aspects of the human small heat shock proteins related to their functional activities. Cell. Stress & Chaperones 25 (4), 581–591. doi:10.1007/S12192-020-01093-1
Botha, M., Chiang, A. N., Needham, P. B., Stephens, L. L., Hoppe, H. C., Külzer, S., et al. (2011). Plasmodium falciparum encodes a single cytosolic type I Hsp40 that functionally interacts with Hsp70 and is upregulated by heat shock. Cell. Stress & Chaperones 16 (4), 389–401. doi:10.1007/S12192-010-0250-6
Bozdech, Z., Llinás, M., Pulliam, B. L., Wong, E. D., Zhu, J., and DeRisi, J. L. (2003). The transcriptome of the intraerythrocytic developmental cycle of Plasmodium falciparum. PLoS Biol. 1 (1), E5. doi:10.1371/journal.pbio.0000005
Breckenridge, D. G., and Xue, D. (2004). Regulation of mitochondrial membrane permeabilization by BCL-2 family proteins and caspases. Curr. Opin. Cell. Biol. 16 (6), 647–652. doi:10.1016/J.CEB.2004.09.009
Brennand, A., Gualdrón-López, M., Coppens, I., Rigden, D. J., Ginger, M. L., and Michels, P. A. M. (2011). Autophagy in parasitic protists: unique features and drug targets. Mol. Biochem. Parasitol. 177 (2), 83–99. doi:10.1016/J.MOLBIOPARA.2011.02.003
Bruey, J. M., Ducasse, C., Bonniaud, P., Ravagnan, L., Susin, S. A., Diaz-Latoud, C., et al. (2000). Hsp27 negatively regulates cell death by interacting with cytochrome c. Nat. Cell Biol. 2 (9), 645–652. doi:10.1038/35023595
Buchner, J., Ehrnsperger, M., Gaestel, M., and Walke, S. (1998). Purification and characterization of small heat shock proteins. Methods Enzym. 290, 339–349. doi:10.1016/S0076-6879(98)90030-1
Bukach, O. V., Seit-Nebi, A. S., Marston, S. B., and Gusev, N. B. (2004). Some properties of human small heat shock protein Hsp20 (HspB6). Eur. J. Biochem. 271 (2), 291–302. doi:10.1046/J.1432-1033.2003.03928.X
Calvaresi, V., Truelsen, L. T., Larsen, S. B., Petersen, N. H. T., Kirkegaard, T., and Rand, K. D. (2021). Conformational dynamics of free and membrane-bound human Hsp70 in model cytosolic and endo-lysosomal environments. Commun. Biol. 4 (1), 1369–1411. doi:10.1038/s42003-021-02892-7
Carra, S., Seguin, S. J., Lambert, H., and Landry, J. (2008). HspB8 chaperone activity toward poly(Q)-containing proteins depends on its association with Bag3, a stimulator of macroautophagy. J. Biol. Chem. 283 (3), 1437–1444. doi:10.1074/JBC.M706304200
Cervantes, S., Bunnik, E. M., Saraf, A., Conner, C. M., Escalante, A., Sardiu, M. E., et al. (2014). The multifunctional autophagy pathway in the human malaria parasite, Plasmodium falciparum. Autophagy 10 (1), 80–92. doi:10.4161/AUTO.26743
Charette, S. J., and Landry, J. (2000). The interaction of HSP27 with Daxx identifies a potential regulatory role of HSP27 in Fas-induced apoptosis. Ann. N. Y. Acad. Sci. 926, 126–131. doi:10.1111/J.1749-6632.2000.TB05606.X
Chaves, J. M., Gupta, R., Srivastava, K., and Srivastava, O. (2017). Human alpha A-crystallin missing N-terminal domain poorly complexes with filensin and phakinin. Biochem. Biophysical Res. Commun. 494 (1–2), 402–408. doi:10.1016/J.BBRC.2017.09.088
Chen, S. F., Nieh, S., Jao, S. W., Liu, C. L., Wu, C. H., Chang, Y. C., et al. (2012). Quercetin suppresses drug-resistant spheres via the p38 MAPK-hsp27 apoptotic pathway in oral cancer cells. PLoS ONE 7 (11), e49275. doi:10.1371/JOURNAL.PONE.0049275
Cheng, J., Ji, M., Jing, H., and Lin, H. (2023). DUSP12 ameliorates myocardial ischemia-reperfusion injury through HSPB8-induced mitophagy. J. Biochem. Mol. Toxicol. 37 (5), e23310. doi:10.1002/JBT.23310
Christians, E. S., Ishiwata, T., and Benjamin, I. J. (2012). Small heat shock proteins in redox metabolism: implications for cardiovascular diseases. Int. J. Biochem. Cell. Biol. 44 (10), 1632–1645. doi:10.1016/J.BIOCEL.2012.06.006
Clark, A. R., Vree Egberts, W., Kondrat, F. D. L., Hilton, G. R., Ray, N. J., Cole, A. R., et al. (2018). Terminal regions confer plasticity to the tetrameric assembly of human HspB2 and HspB3. J. Mol. Biol. 430 (18 Pt B), 3297–3310. doi:10.1016/J.JMB.2018.06.047
Coertzen, D., Reader, J., Van Der Watt, M., Nondaba, S. H., Gibhard, L., Wiesner, L., et al. (2018). Artemisone and artemiside are potent panreactive antimalarial agents that also synergize redox imbalance in Plasmodium falciparum transmissible gametocyte stages. Antimicrob. Agents Chemother. 62 (8), e02214–17. doi:10.1128/AAC.02214-17
Coll, N. S., Vercammen, D., Smidler, A., Clover, C., Van Breusegem, F., Dangl, J. L., et al. (2010). Arabidopsis type I metacaspases control cell death. SCIENCE 330, 1393–1397. doi:10.1126/science.1194980
Cristofani, R., Rusmini, P., Galbiati, M., Cicardi, M. E., Ferrari, V., Tedesco, B., et al. (2019). The regulation of the small heat shock protein B8 in misfolding protein diseases causing motoneuronal and muscle cell death. Front. Neurosci. 13, 796. doi:10.3389/fnins.2019.00796
Cuervo, A. M., Stafanis, L., Fredenburg, R., Lansbury, P. T., and Sulzer, D. (2004). Impaired degradation of mutant alpha-synuclein by chaperone-mediated autophagy. Sci. (New York, N.Y.) 305 (5688), 1292–1295. doi:10.1126/SCIENCE.1101738
Cui, L., Fan, Q., Cui, L., and Miao, J. (2008). Histone lysine methyltransferases and demethylases in Plasmodium falciparum. Int. J. Parasitol. 38 (10), 1083–1097. doi:10.1016/J.IJPARA.2008.01.002
Cui, X., Feng, R., Wang, J., Du, C., Pi, X., Chen, D., et al. (2020). Heat shock factor 4 regulates lysosome activity by modulating the αB-crystallin-ATP6V1A-mTOR complex in ocular lens. Biochimica Biophysica Acta. General Subj. 1864 (3), 129496. doi:10.1016/J.BBAGEN.2019.129496
de Almagro, M. C., and Vucic, D. (2015). Necroptosis: pathway diversity and characteristics. Seminars Cell. & Dev. Biol. 39, 56–62. doi:10.1016/J.SEMCDB.2015.02.002
de Gooijer, C. J., van der Noort, V., Stigt, J. A., Baas, P., Biesma, B., Cornelissen, R., et al. (2021). Switch-maintenance gemcitabine after first-line chemotherapy in patients with malignant mesothelioma (NVALT19): an investigator-initiated, randomised, open-label, phase 2 trial. Lancet Respir. Med. 9 (6), 585–592. doi:10.1016/S2213-2600(20)30362-3
Degterev, A., Hitomi, J., Germscheid, M., Ch’en, I. L., Korkina, O., Teng, X., et al. (2008). Identification of RIP1 kinase as a specific cellular target of necrostatins. Nat. Chem. Biol. 4 (5), 313–321. doi:10.1038/NCHEMBIO.83
De Maio, A., Cauvi, D. M., Capone, R., Bello, I., Egberts, W. V., Arispe, N., et al. (2019). The small heat shock proteins, HSPB1 and HSPB5, interact differently with lipid membranes. Cell. Stress & Chaperones 24 (5), 947–956. doi:10.1007/S12192-019-01021-Y
de Menezes, M. N., Salles, É. M., Vieira, F., Amaral, E. P., Zuzarte-Luís, V., Cassado, A., et al. (2019). IL-1α promotes liver inflammation and necrosis during blood-stage Plasmodium chabaudi malaria. Sci. Rep. 9 (1), 7575. doi:10.1038/S41598-019-44125-2
Dorstyn, L., Akey, C. W., and Kumar, S. (2018). New insights into apoptosome structure and function. Cell. Death Differ. 25 (7), 1194–1208. doi:10.1038/S41418-017-0025-Z
Duszenko, M., Ginger, M. L., Brennand, A., Gualdrón-López, M., Colombo, M. I., Coombs, G. H., et al. (2011). Autophagy in protists. Autophagy 7 (2), 127–158. doi:10.4161/AUTO.7.2.13310
Ecroyd, H., Meehan, S., Horwitz, J., Aquilina, J. A., Benesch, J. L. P., Robinson, C. V., et al. (2007). Mimicking phosphorylation of alphaB-crystallin affects its chaperone activity. Biochem. J. 401 (1), 129–141. doi:10.1042/BJ20060981
Eickel, N., Kaiser, G., Prado, M., Burda, P. C., Roelli, M., Stanway, R. R., et al. (2013). Features of autophagic cell death in Plasmodium liver-stage parasites. Autophagy 9 (4), 568–580. doi:10.4161/AUTO.23689
Fahrig, R., Quietzsch, D., Heinrich, J. C., Heinemann, V., Boeck, S., Schmid, R. M., et al. (2006). RP101 improves the efficacy of chemotherapy in pancreas carcinoma cell lines and pancreatic cancer patients. Anti-Cancer Drugs 17 (9), 1045–1056. doi:10.1097/01.CAD.0000231472.92406.D2
Fan, G. C., Chu, G., Mitton, B., Song, Q., Yuan, Q., and Kranias, E. G. (2004). Small heat-shock protein Hsp20 phosphorylation inhibits β-agonist-induced cardiac apoptosis. Circulation Res. 94 (11), 1474–1482. doi:10.1161/01.RES.0000129179.66631.00
Feintuch, C. M., Saidi, A., Seydel, K., Chen, G., Goldman-Yassen, A., Mita-Mendoza, N. K., et al. (2016). Activated neutrophils are associated with pediatric cerebral malaria vasculopathy in Malawian children. MBio 7 (1), e01300–e01315. doi:10.1128/MBIO.01300-15
Galluzzi, L., Vitale, I., Abrams, J. M., Alnemri, E. S., Baehrecke, E. H., Blagosklonny, M. V., et al. (2012). Molecular definitions of cell death subroutines: recommendations of the Nomenclature Committee on Cell Death 2012. Cell. Death Differ. 19 (1), 107–120. doi:10.1038/CDD.2011.96
Garcia-Ranea, J. A., Mirey, G., Camonis, J., and Valencia, A. (2002). p23 and HSP20/alpha-crystallin proteins define a conserved sequence domain present in other eukaryotic protein families. FEBS Lett. 529 (2–3), 162–167. doi:10.1016/S0014-5793(02)03321-5
Gardner, M. J., Hall, N., Fung, E., White, O., Berriman, M., Hyman, R. W., et al. (2002). Genome sequence of the human malaria parasite Plasmodium falciparum. Nature 419 (6906), 498–511. doi:10.1038/NATURE01097
Gaviria, D., Paguio, M. F., Turnbull, L. B., Tan, A., Siriwardana, A., Ghosh, D., et al. (2013). A process similar to autophagy is associated with cytocidal chloroquine resistance in Plasmodium falciparum. PLoS ONE 8 (11), 79059. doi:10.1371/JOURNAL.PONE.0079059
Ghobrial, I. M., Witzig, T. E., and Adjei, A. A. (2005). Targeting apoptosis pathways in cancer therapy. CA A Cancer J. Clin. 55 (3), 178–194. doi:10.3322/CANJCLIN.55.3.178
Gibert, B., Simon, S., Dimitrova, V., Diaz-Latoud, C., and Arrigo, A. P. (2013). Peptide aptamers: tools to negatively or positively modulate HSPB1(27) function. Philosophical Trans. R. Soc. Lond. Ser. B, Biol. Sci. 368 (1617), 20120075–20120078. doi:10.1098/RSTB.2012.0075
Grad, I., McKee, T. A., Ludwig, S. M., Hoyle, G. W., Ruiz, P., Wurst, W., et al. (2006). The Hsp90 cochaperone p23 is essential for perinatal survival. Mol. Cell. Biol. 26 (23), 8976–8983. doi:10.1128/MCB.00734-06
Green, D. R., and Llambi, F. (2015). Cell death signaling. Cold Spring Harb. Perspect. Biol. 7 (12), a006080. doi:10.1101/CSHPERSPECT.A006080
Gross, O., Yazdi, A. S., Thomas, C. J., Masin, M., Heinz, L. X., Guarda, G., et al. (2012). Inflammasome activators induce interleukin-1α secretion via distinct pathways with differential requirement for the protease function of caspase-1. Immunity 36 (3), 388–400. doi:10.1016/J.IMMUNI.2012.01.018
Guay, J., Lambert, H., Gingras-Breton, G., Lavoie, J. N., Huot, J., and Landry, J. (1997). Regulation of actin filament dynamics by p38 map kinase-mediated phosphorylation of heat shock protein 27. J. Cell. Sci. 110 (Pt 3), 357–368. doi:10.1242/JCS.110.3.357
Gusev, N. B., Bogatcheva, N. V., and Marston, S. B. (2002). Structure and properties of small heat shock proteins (sHsp) and their interaction with cytoskeleton proteins. Biochem. Mosc. 67 (5), 511–519. doi:10.1023/a:1015549725819
Hall, N., Pain, A., Berriman, M., Churcher, C., Harris, B., Harris, D., et al. (2002). Sequence of Plasmodium falciparum chromosomes 1, 3–9 and 13. Nature 419 (6906), 527–531. doi:10.1038/nature01095
Haslbeck, M., and Vierling, E. (2015). A first line of stress defense: small heat shock proteins and their function in protein homeostasis. J. Mol. Biol. 427 (7), 1537–1548. doi:10.1016/J.JMB.2015.02.002
Haslbeck, M., Weinkauf, S., and Buchner, J. (2019). Small heat shock proteins: simplicity meets complexity. J. Biol. Chem. 294 (6), 2121–2132. doi:10.1074/JBC.REV118.002809
Havasi, A., Li, Z., Wang, Z., Martin, J. L., Botla, V., Ruchalski, K., et al. (2008). Hsp27 inhibits Bax activation and apoptosis via a phosphatidylinositol 3-kinase-dependent mechanism. J. Biol. Chem. 283 (18), 12305–12313. doi:10.1074/jbc.M801291200
Hay, Z. L. Z., and Slansky, J. E. (2022). Granzymes: the molecular executors of immune-mediated cytotoxicity. Int. J. Mol. Sci. 23 (3), 1833. doi:10.3390/IJMS23031833
He, C., and Levine, B. (2010). The Beclin 1 interactome. Curr. Opin. Cell. Biol. 22 (2), 140–149. doi:10.1016/J.CEB.2010.01.001
Heimer, S., Knoll, G., Schulze-Osthoff, K., and Ehrenschwender, M. (2019). Raptinal bypasses BAX, BAK, and BOK for mitochondrial outer membrane permeabilization and intrinsic apoptosis. Cell. Death Dis. 10 (8), 556. doi:10.1038/S41419-019-1790-Z
Heinrich, J. C., Tuukkanen, A., Schroeder, M., Fahrig, T., and Fahrig, R. (2011). RP101 (brivudine) binds to heat shock protein HSP27 (HSPB1) and enhances survival in animals and pancreatic cancer patients. J. Cancer Res. Clin. Oncol. 137 (9), 1349–1361. doi:10.1007/S00432-011-1005-1
Heirbaut, M., Beelen, S., Strelkov, S. V., and Weeks, S. D. (2014). Dissecting the functional role of the N-terminal domain of the human small heat shock protein HSPB6. PLOS ONE 9 (8), e105892. doi:10.1371/JOURNAL.PONE.0105892
Hilton, G. R., Hochberg, G. K. A., Laganowsky, A., McGinnigle, S. I., Baldwin, A. J., and Benesch, J. L. P. (2013). C-terminal interactions mediate the quaternary dynamics of αB-crystallin. Philos. Trans. R. Soc. B Biol. Sci. 368, 20110405. doi:10.1098/rstb.2011.0405
Hui, C., Bosch, A., Mwizerwa, O., McColl, J., Corbeil, A., Malcolmson, C., et al. (2023). Case report: a case of bone marrow necrosis and hyperinflammation in a 10-year-old boy after Plasmodium falciparum infection. Am. J. Trop. Med. Hyg. 109 (3), 611–615. doi:10.4269/AJTMH.22-0550
Humeau, J., Bezu, L., Kepp, O., and Kroemer, G. (2020). EIF2α phosphorylation: a hallmark of both autophagy and immunogenic cell death. Mol. Cell. Oncol. 7 (5), 1776570. doi:10.1080/23723556.2020.1776570
Huot, J., Houle, F., Spitz, D. R., and Landry, J. (1996). HSP27 phosphorylation-mediated resistance against actin fragmentation and cell death induced by oxidative stress. Cancer Res. 56, 273–279.
Ikwegbue, P. C., Masamba, P., Oyinloye, B. E., and Kappo, A. P. (2018). Roles of heat shock proteins in apoptosis, oxidative stress, human inflammatory diseases, and cancer. Pharmaceuticals 11 (1), 2. doi:10.3390/PH11010002
Jacobsen, A. V., Lowes, K. N., Tanzer, M. C., Lucet, I. S., Hildebrand, J. M., Petrie, E. J., et al. (2016). HSP90 activity is required for MLKL oligomerisation and membrane translocation and the induction of necroptotic cell death. Cell. Death Dis. 7 (1), e2051. doi:10.1038/CDDIS.2015.386
Jan, R., and Chaudhry, G.-E.-S. (2019). Understanding apoptosis and apoptotic pathways targeted cancer therapeutics. Adv. Pharm. Bull. 9, 205–218. doi:10.15171/apb.2019.024
Janowska, M. K., Baughman, H. E. R., Woods, C. N., and Klevit, R. E. (2019). Mechanisms of small heat shock proteins. Cold Spring Harb. Perspect. Biol. 11 (10), a034025. doi:10.1101/CSHPERSPECT.A034025
Jiang, X., and Wang, X. (2004). Cytochrome C-mediated apoptosis. Annu. Rev. Biochem. 73, 87–106. doi:10.1146/annurev.biochem.73.011303.073706
Jie, X. Y., Ling, H. M., Hui, Z. L., Qi, W., Quan, Z. X., Bin, L. Q., et al. (2019). Effect of HSPB9 on apoptosis of DF-1 cells. Biomed. Environ. Sci. 32 (2), 107–120. doi:10.3967/BES2019.015
Kalioraki, M. A., Artemaki, P. I., Sklirou, A. D., Kontos, C. K., Adamopoulos, P. G., Papadopoulos, I. N., et al. (2020). Heat shock protein beta 3 (HSPB3) is an unfavorable molecular biomarker in colorectal adenocarcinoma. Mol. Carcinog. 59 (1), 116–125. doi:10.1002/MC.23133
Kamradt, M. C., Chen, F., and Cryns, V. L. (2001). The small heat shock protein alpha B-crystallin negatively regulates cytochrome c- and caspase-8-dependent activation of caspase-3 by inhibiting its autoproteolytic maturation. J. Biol. Chem. 276 (19), 16059–16063. doi:10.1074/JBC.C100107200
Kamradt, M. C., Chen, F., Sam, S., and Cryns, V. L. (2002). The small heat shock protein alpha B-crystallin negatively regulates apoptosis during myogenic differentiation by inhibiting caspase-3 activation. J. Biol. Chem. 277 (41), 38731–38736. doi:10.1074/JBC.M201770200
Kamradt, M. C., Lu, M., Werner, M. E., Kwan, T., Chen, F., Strohecker, A., et al. (2005). The small heat shock protein alpha B-crystallin is a novel inhibitor of TRAIL-induced apoptosis that suppresses the activation of caspase-3. J. Biol. Chem. 280 (12), 11059–11066. doi:10.1074/jbc.M413382200
Kaushansky, A., Ye, A. S., Austin, L. S., Mikolajczak, S. A., Vaughan, A. M., Camargo, N., et al. (2013). Suppression of host p53 is critical for Plasmodium liver-stage infection. Cell. Rep. 3 (3), 630–637. doi:10.1016/J.CELREP.2013.02.010
Kim, K. K., Kim, R., and Kim, S. H. (1998). Crystal structure of a small heat-shock protein. Nature 394 (6693), 595–599. doi:10.1038/29106
Kostenko, S., Johannessen, M., and Moens, U. (2009). PKA-induced F-actin rearrangement requires phosphorylation of Hsp27 by the MAPKAP kinase MK5. Cell. Signal. 21 (5), 712–718. doi:10.1016/J.CELLSIG.2009.01.009
Kostenko, S., and Moens, U. (2009). Heat shock protein 27 phosphorylation: kinases, phosphatases, functions and pathology. Cell. Mol. Life Sci. 66 (20), 3289–3307. doi:10.1007/S00018-009-0086-3
Krief, S., Faivre, J. F., Robert, P., Le Douarin, B., Brument-Larignon, N., Lefrère, I., et al. (1999). Identification and characterization of cvHsp. A novel human small stress protein selectively expressed in cardiovascular and insulin-sensitive tissues. J. Biol. Chem. 274 (51), 36592–36600. doi:10.1074/JBC.274.51.36592
Kriehuber, T., Rattei, T., Weinmaier, T., Bepperling, A., Haslbeck, M., and Buchner, J. (2010). Independent evolution of the core domain and its flanking sequences in small heat shock proteins. FASEB J. Official Publ. Fed. Am. Soc. Exp. Biol. 24 (10), 3633–3642. doi:10.1096/FJ.10-156992
Kumar, B., Verma, S., Kashif, M., Sharma, R., Atul, R., Singh, A. P., et al. (2019). Metacaspase-3 of Plasmodium falciparum: an atypical trypsin-like serine protease. Int. J. Biol. Macromol. 138, 309–320. doi:10.1016/J.IJBIOMAC.2019.07.067
Kumari, V., Prasad, K. M., Kalia, I., Sindhu, G., Dixit, R., Rawat, D. S., et al. (2022). Dissecting the role of Plasmodium metacaspase-2 in malaria gametogenesis and sporogony. Emerg. Microbes Infect. 11 (1), 938–955. doi:10.1080/22221751.2022.2052357
LaCount, D. J., Vignali, M., Chettier, R., Phansalkar, A., Bell, R., Hesselberth, J. R., et al. (2005). A protein interaction network of the malaria parasite Plasmodium falciparum. Nature 438 (7064), 103–107. doi:10.1038/nature04104
Laure, L., Long, R., Lizano, P., Zini, R., Berdeaux, A., Depre, C., et al. (2012). Cardiac H11 kinase/Hsp22 stimulates oxidative phosphorylation and modulates mitochondrial reactive oxygen species production: involvement of a nitric oxide-dependent mechanism. Free Radic. Biol. Med. 52 (11–12), 2168–2176. doi:10.1016/J.FREERADBIOMED.2012.03.001
Lehane, A. M., McDevitt, C. A., Kirk, K., and Fidock, D. A. (2012). Degrees of chloroquine resistance in Plasmodium - is the redox system involved? Int. J. Parasitol. Drugs Drug Resist. 2, 47–57. doi:10.1016/J.IJPDDR.2011.11.001
Li, F., Xiao, H., Hu, Z., Zhou, F., and Yang, B. (2018). Exploring the multifaceted roles of heat shock protein B8 (HSPB8) in diseases. Eur. J. Cell. Biol. 97 (3), 216–229. doi:10.1016/J.EJCB.2018.03.003
Li, X., He, S., and Ma, B. (2020). Autophagy and autophagy-related proteins in cancer. Mol. Cancer 19 (1), 12. doi:10.1186/S12943-020-1138-4
Li, Z., Menoret, A., and Srivastava, P. (2002). Roles of heat-shock proteins in antigen presentation and cross-presentation. Curr. Opin. Immunol. 14 (1), 45–51. doi:10.1016/S0952-7915(01)00297-7
Lindquist, S., and Craig, E. A. (2003). The heat-shock proteins. Annu. Ge 22, 631–677. doi:10.1146/ANNUREV.GE.22.120188.003215
Liu, S., Li, J., Tao, Y., and Xiao, X. (2006). Small heat shock protein alphaB-crystallin binds to p53 to sequester its translocation to mitochondria during hydrogen peroxide-induced apoptosis. Biochem. Biophysical Res. Commun. 354 (1), 109–114. doi:10.1016/j.bbrc.2006.12.152
Liu, Z., Zhang, S., Gu, J., Tong, Y., Li, Y., Gui, X., et al. (2020). Hsp27 chaperones FUS phase separation under the modulation of stress-induced phosphorylation. Nat. Struct. Mol. Biol. 27 (4), 363–372. doi:10.1038/s41594-020-0399-3
Llambi, F., Moldoveanu, T., Tait, S. W. G., Bouchier-Hayes, L., Temirov, J., McCormick, L. L., et al. (2011). A unified model of mammalian BCL-2 protein family interactions at the mitochondria. Mol. Cell. 44 (4), 517–531. doi:10.1016/J.MOLCEL.2011.10.001
Locatelli, A. G., and Cenci, S. (2022). Autophagy and longevity: evolutionary hints from hyper-longevous mammals. Front. Endocrinol. 13, 1085522. doi:10.3389/FENDO.2022.1085522
Lossi, L. (2022). The concept of intrinsic versus extrinsic apoptosis. Biochem. J. 479 (3), 357–384. doi:10.1042/BCJ20210854
Lu, K. Y., Pasaje, C. F. A., Srivastava, T., Loiselle, D. R., Niles, J. C., and Derbyshire, E. (2020). Phosphatidylinositol 3-phosphate and hsp70 protect Plasmodium falciparum from heat-induced cell death. ELife 9, 567733–e56827. doi:10.7554/ELIFE.56773
Lv, L. H., Wan, Y., Zhang, W., Yang, M., Li, G. N., Lin, H. M., et al. (2012). Anticancer drugs cause release of exosomes with heat shock proteins from human hepatocellular carcinoma cells that elicit effective natural killer cell antitumor responses in vitro. J. Biol. Chem. 287 (19), 15874–15885. doi:10.1074/JBC.M112.340588
Ma, X., Nan, Y., Huang, C., Li, X., Yang, Y., Jiang, W., et al. (2023). Expression of αA-crystallin (CRYAA) in vivo and in vitro models of age-related cataract and the effect of its silencing on HLEB3 cells. Aging (Albany NY) 15 (10), 4498–4509. doi:10.18632/AGING.204754
Mackay, D. S., Andley, U. P., and Shiels, A. (2003). Cell death triggered by a novel mutation in the alphaA-crystallin gene underlies autosomal dominant cataract linked to chromosome 21q. Eur. J. Hum. Genet. 11 (10), 784–793. doi:10.1038/SJ.EJHG.5201046
Mandal, R., Barrón, J. C., Kostova, I., Becker, S., and Strebhardt, K. (2020). Caspase-8: the double-edged sword. Biochimica Biophysica Acta Rev. Cancer 1873 (2), 188357. doi:10.1016/J.BBCAN.2020.188357
Massey, A. C., Zhang, C., and Cuervo, A. M. (2006). Chaperone-mediated autophagy in aging and disease. Curr. Top. Dev. Biol. 73, 205–235. doi:10.1016/S0070-2153(05)73007-6
Matsumoto, T., Urushido, M., Ide, H., Ishihara, M., Hamada-Ode, K., Shimamura, Y., et al. (2015). Small heat shock protein beta-1 (HSPB1) is upregulated and regulates autophagy and apoptosis of renal tubular cells in acute kidney injury. PLoS ONE 10 (5), e0126229. doi:10.1371/JOURNAL.PONE.0126229
Mehlen, P., Schulze-Osthoff, K., and Arrigo, A. P. (1996). Small Stress Proteins as Novel Regulators of Apoptosis: heat shock protein 27 blocks fas/apo-1- and staurosporine-induced cell death. J. Biol. Chem. 271 (28), 16510–16514. doi:10.1074/JBC.271.28.16510
Meslin, B., Beavogui, A. H., Fasel, N., and Picot, S. (2011). Plasmodium falciparum metacaspase PfMCA-1 triggers a z-VAD-fmk inhibitable protease to promote cell death. PLoS ONE 6 (8), e23867. doi:10.1371/JOURNAL.PONE.0023867
Miroshnichenko, S., Tripp, J., Zur Nieden, U., Neumann, D., Conrad, U., and Manteuffel, R. (2005). Immunomodulation of function of small heat shock proteins prevents their assembly into heat stress granules and results in cell death at sublethal temperatures. Plant J. 41 (2), 269–281. doi:10.1111/J.1365-313X.2004.02290.X
Mizushima, N., Levine, B., Cuervo, A. M., and Klionsky, D. J. (2008). Autophagy fights disease through cellular self-digestion. Nature 451 (7182), 1069–1075. doi:10.1038/NATURE06639
Mogk, A., Ruger-Herreros, C., and Bukau, B. (2019). Cellular functions and mechanisms of action of small heat shock proteins. Annu. Rev. Microbiol. 73, 89–110. doi:10.1146/annurev-micro-020518-115515
Mohapatra, A. D., Zuromski, J., and Kurtis, J. (2022). Assessing PfGARP-mediated apoptosis of blood-stage Plasmodium falciparum parasites. Methods Mol. Biol. Clift. N.J.) 2470, 659–672. doi:10.1007/978-1-0716-2189-9_49
Morrow, G., and Tanguay, R. M. (2012). Small heat shock protein expression and functions during development. Int. J. Biochem. Cell. Biol. 44 (10), 1613–1621. doi:10.1016/J.BIOCEL.2012.03.009
Mounier, N., and Arrigo, A. P. (2002). Actin cytoskeleton and small heat shock proteins: how do they interact? Cell. Stress & Chaperones 7 (2), 167–176. doi:10.1379/1466-1268(2002)007<0167:acashs>2.0.co;2
Mymrikov, E. V., Riedl, M., Peters, C., Weinkauf, S., Haslbeck, M., and Buchner, J. (2020). Regulation of small heat-shock proteins by hetero-oligomer formation. J. Biol. Chem. 295 (1), 158–169. doi:10.1074/JBC.RA119.011143
Nagasawa, T., Matsushima-Nishiwaki, R., Toyoda, H., Matsuura, J., Kumada, T., and Kozawa, O. (2014). Heat shock protein 20 (HSPB6) regulates apoptosis in human hepatocellular carcinoma cells: direct association with Bax. Oncol. Rep. 32 (3), 1291–1295. doi:10.3892/or.2014.3278
Nair, R. K., Rao, K. A., Mukherjee, D., Datt, B., Sharma, S., and Prakash, S. (2019). Acute kidney injury due to acute cortical necrosis following vivax malaria. Saudi J. Kidney Dis. Transplant. 30 (4), 960–963. doi:10.4103/1319-2442.265474
Nakamoto, H., and Vígh, L. (2007). The small heat shock proteins and their clients. Cell. Mol. Life Sci. 64 (3), 294–306. doi:10.1007/S00018-006-6321-2
Nappi, L., Aguda, A. H., Al Nakouzi, N., Lelj-Garolla, B., Beraldi, E., Lallous, N., et al. (2020). Ivermectin inhibits HSP27 and potentiates efficacy of oncogene targeting in tumor models. J. Clin. Investigation 130 (2), 699–714. doi:10.1172/JCI130819
Navarro-zaragoza, J., Cuenca-bermejo, L., Almela, P., Laorden, M. L., and Herrero, M. T. (2021). Could small heat shock protein HSP27 Be a first-line target for preventing protein aggregation in Parkinson’s disease? Int. J. Mol. Sci. 22 (6), 3038–3113. doi:10.3390/IJMS22063038
Nepveu, F., and Turrini, F. (2013). Targeting the redox metabolism of Plasmodium falciparum. Future Med. Chem. 5 (16), 1993–2006. doi:10.4155/FMC.13.159
Nussler, A. K., Rénia, L., Pasquetto, V., Miltgen, F., Matile, H., and Mazier, D. (1993). In vivo induction of the nitric oxide pathway in hepatocytes after injection with irradiated malaria sporozoites, malaria blood parasites or adjuvants. Eur. J. Immunol. 23 (4), 882–887. doi:10.1002/EJI.1830230417
Orning, P., and Lien, E. (2021). Multiple roles of caspase-8 in cell death, inflammation, and innate immunity. J. Leukoc. Biol. 109 (1), 121–141. doi:10.1002/JLB.3MR0420-305R
Oshita, S. E., Chen, F., Kwan, T., Yehiely, F., and Cryns, V. L. (2010). The small heat shock protein HspB2 is a novel anti-apoptotic protein that inhibits apical caspase activation in the extrinsic apoptotic pathway. Breast Cancer Res. Treat. 124 (2), 307–315. doi:10.1007/S10549-010-0735-0
Pallavi, R., Acharya, P., Chandran, S., Daily, J. P., and Tatu, U. (2010). Chaperone expression profiles correlate with distinct physiological states of Plasmodium falciparum in malaria patients. Malar. J. 9 (1), 236. doi:10.1186/1475-2875-9-236
Palmieri, M., Pal, R., Nelvagal, H. R., Lotfi, P., Stinnett, G. R., Seymour, M. L., et al. (2017). Corrigendum: mTORC1-independent TFEB activation via Akt inhibition promotes cellular clearance in neurodegenerative storage diseases. Nat. Commun. 8, 15793. doi:10.1038/ncomms15793
Pasta, S. Y., Raman, B., Ramakrishna, T., and Rao, Ch. M. (2003). Role of the conserved SRLFDQFFG region of alpha-crystallin, a small heat shock protein. Effect on oligomeric size, subunit exchange, and chaperone-like activity. J. Biol. Chem. 278 (51), 51159–51166. doi:10.1074/jbc.m307523200
Paul, C., Manero, F., Gonin, S., Kretz-Remy, C., Virot, S., and Arrigo, A. P. (2002). Hsp27 as a negative regulator of cytochrome c release. Mol. Cell. Biol. 22 (3), 816–834. doi:10.1128/MCB.22.3.816-834.2002
Paul, C., Simon, S., Gibert, B., Virot, S., Manero, F., and Arrigo, A. P. (2010). Dynamic processes that reflect anti-apoptotic strategies set up by HspB1 (Hsp27). Exp. Cell. Res. 316 (9), 1535–1552. doi:10.1016/J.YEXCR.2010.03.006
Pawelka, E., Seitz, T., Hoepler, W., Karolyi, M., Laferl, H., Neuhold, S., et al. (2021). Intestinal necrosis as an uncommon complication of Plasmodium falciparum malaria with a parasite count of 50. J. Travel Med. 28 (5), taaa203. doi:10.1093/JTM/TAAA203
Pécot, J., Maillet, L., Le Pen, J., Vuillier, C., Trécesson, S. de C., Fétiveau, A., et al. (2016). Tight sequestration of BH3 proteins by BCL-xL at subcellular membranes contributes to apoptotic resistance. Cell. Rep. 17 (12), 3347–3358. doi:10.1016/J.CELREP.2016.11.064
Peng, C., Zhao, F., Li, H., Li, L., Yang, Y., and Liu, F. (2022). HSP90 mediates the connection of multiple programmed cell death in diseases. Cell. Death Dis. 13 (11), 1–12. doi:10.1038/s41419-022-05373-9
Pérez-Morales, D., and Espinoza, B. (2015). The role of small heat shock proteins in parasites. Cell. Stress Chaperones 20 (5), 767–780. doi:10.1007/s12192-015-0607-y
Petryszak, R., Burdett, T., Fiorelli, B., Fonseca, N. A., Gonzalez-Porta, M., Hastings, E., et al. (2014). Expression Atlas update--a database of gene and transcript expression from microarray- and sequencing-based functional genomics experiments. Nucleic Acids Res. 42, D926–D932. doi:10.1093/NAR/GKT1270
Pires, C. V., Oberstaller, J., Wang, C., Casandra, D., Zhang, M., Chawla, J., et al. (2023). Chemogenomic profiling of a Plasmodium falciparum transposon mutant library reveals shared Effects of Dihydroartemisinin and Bortezomib on lipid metabolism and exported proteins. Microbiol. Spectr. 11 (3), e0501422–22. doi:10.1128/spectrum.05014-22
Porter, H., Gamette, M. J., Cortes-Hernandez, D. G., and Jensen, J. B. (2008). Asexual blood stages of Plasmodium falciparum exhibit signs of secondary necrosis, but not classical apoptosis after exposure to febrile temperature (40 C). J. Parasitol. 94 (2), 473–480. doi:10.1645/GE-1343.1
Povea-Cabello, S., Oropesa-Ávila, M., de la Cruz-Ojeda, P., Villanueva-Paz, M., De La Mata, M., Suárez-Rivero, J. M., et al. (2017). Dynamic reorganization of the cytoskeleton during apoptosis: the two coffins hypothesis. Int. J. Mol. Sci. 18 (11), 2393. doi:10.3390/IJMS18112393
Powell, I. J., Chinni, S. R., Reddy, S. S., Zaslavsky, A., and Gavande, N. (2021). Pro-inflammatory cytokines and chemokines initiate multiple prostate cancer biologic pathways of cellular proliferation, heterogeneity and metastasis in a racially diverse population and underlie the genetic/biologic mechanism of racial disparity: update. Urol. Oncol. 39 (1), 34–40. doi:10.1016/J.UROLONC.2020.08.019
Préville, X., Salvemini, F., Giraud, S., Chaufour, S., Paul, C., Stepien, G., et al. (1999). Mammalian small stress proteins protect against oxidative stress through their ability to increase glucose-6-phosphate dehydrogenase activity and by maintaining optimal cellular detoxifying machinery. Exp. Cell. Res. 247 (1), 61–78. doi:10.1006/EXCR.1998.4347
Proellocks, N. I., Coppel, R. L., Mohandas, N., and Cooke, B. M. (2016). Malaria parasite proteins and their role in alteration of the structure and function of red blood cells. Adv. Parasitol. 91, 1–86. doi:10.1016/BS.APAR.2015.09.002
Raj, D. K., Das Mohapatra, A., Jnawali, A., Zuromski, J., Jha, A., Cham-Kpu, G., et al. (2020). Anti-PfGARP activates programmed cell death of parasites and reduces severe malaria. Nature 582 (7810), 104–108. doi:10.1038/S41586-020-2220-1
Rane, M. J., Coxon, P. Y., Powell, D. W., Webster, R., Klein, J. B., Pierce, W., et al. (2001). p38 Kinase-dependent MAPKAPK-2 activation functions as 3-phosphoinositide-dependent kinase-2 for Akt in human neutrophils. J. Biol. Chem. 276 (5), 3517–3523. doi:10.1074/JBC.M005953200
Rathore, S., Datta, G., Kaur, I., Malhotra, P., and Mohmmed, A. (2015). Disruption of cellular homeostasis induces organelle stress and triggers apoptosis like cell-death pathways in malaria parasite. Cell. Death Dis. 6 (7), e1803. doi:10.1038/cddis.2015.142
Ravikumar, B., Sarkar, S., Davies, J. E., Futter, M., Garcia-Arencibia, M., Green-Thompson, Z. W., et al. (2010). Regulation of mammalian autophagy in physiology and pathophysiology. Physiol. Rev. 90 (4), 1383–1435. doi:10.1152/PHYSREV.00030.2009
Rawat, M., Srivastava, A., Johri, S., Gupta, I., and Karmodiya, K. (2021). Single-cell RNA sequencing reveals cellular heterogeneity and stage transition under temperature stress in synchronized Plasmodium falciparum cells. Microbiol. Spectr. 9 (1), e0000821. doi:10.1128/SPECTRUM.00008-21
Ray, A., Mathur, M., Choubey, D., Karmodiya, K., and Surolia, N. (2022). Autophagy underlies the proteostasis mechanisms of artemisinin resistance in P. falciparum malaria. MBio 13 (3), e0063022. doi:10.1128/MBIO.00630-22
Richter, L., Flodman, P., Von-Bischhoffshausen, F. B., Burch, D., Brown, S., Nguyen, L., et al. (2008). Clinical variability of autosomal dominant cataract, microcornea and corneal opacity and novel mutation in the alpha A crystallin gene (CRYAA). Am. J. Med. Genet. Part A 146A (7), 833–842. doi:10.1002/AJMG.A.32236
Rocha, B. C., Marques, P. E., Leoratti, F. M. D. S., Junqueira, C., Pereira, D. B., Antonelli, L. R. D. V., et al. (2015). Type I interferon transcriptional signature in neutrophils and low-density granulocytes are associated with tissue damage in malaria. Cell. Rep. 13 (12), 2829–2841. doi:10.1016/J.CELREP.2015.11.055
Rogalla, T., Ehrnsperger, M., Preville, X., Kotlyarov, A., Lutsch, G., Ducasse, C., et al. (1999). Regulation of Hsp27 oligomerization, chaperone function, and protective activity against oxidative stress/tumor necrosis factor alpha by phosphorylation. J. Biol. Chem. 274 (27), 18947–18956. doi:10.1074/JBC.274.27.18947
Rosenthal, M. R., and Ng, C. L. (2020). Plasmodium falciparum artemisinin resistance: the effect of heme, protein damage, and parasite cell stress response. ACS Infect. Dis. 6 (7), 1599–1614. doi:10.1021/ACSINFECDIS.9B00527
Rousalova, I., and Krepela, E. (2010). Granzyme B-induced apoptosis in cancer cells and its regulation (review). Int. J. Oncol. 37, 1361–1378. doi:10.3892/ijo_00000788
Saha, S., Sengupta, K., Chatterjee, P., Basu, S., and Nasipuri, M. (2018). Analysis of protein targets in pathogen–host interaction in infectious diseases: a case study on Plasmodium falciparum and Homo sapiens interaction network. Briefings Funct. Genomics 17 (6), 441–450. doi:10.1093/bfgp/elx024
Saklatvala, J., Kaur, P., and Guesdon, F. (1991). Phosphorylation of the small heat-shock protein is regulated by interleukin 1, tumour necrosis factor, growth factors, bradykinin and ATP. Biochem. J. 277 (3), 635–642. doi:10.1042/BJ2770635
Sena-Dos-santos, C., Braga-Da-silva, C., Marques, D., Dos Santos Pinheiro, J. A., Ribeiro-Dos-santos, Â., and Cavalcante, G. C. (2021). Unraveling cell death pathways during malaria infection: what do we know so far? Cells 10 (2), 479–529. doi:10.3390/CELLS10020479
Seul-Ki, C., Kam, H., Kye-Young, K., Park, S., and Yun-Sil, L. (2019). Targeting heat shock protein 27 in cancer: a druggable target for cancer treatment? Cancers 11 (8), 1195. doi:10.3390/CANCERS11081195
Shan, R., Liu, N., Yan, Y., and Liu, B. (2021). Apoptosis, autophagy and atherosclerosis: relationships and the role of Hsp27. Pharmacol. Res. 166, 105169. doi:10.1016/J.PHRS.2020.105169
Shonhai, A., and Blatch, G. L. (2014). Heat shock proteins of malaria: what do we not know, and what should the future focus be? Heat Shock Proteins Malar. 9789400774384, 207–211. doi:10.1007/978-94-007-7438-4_11
Shankar, S., Prasad, K. M., Kashif, M., Kalia, I., Rai, R., Singh, A. P., et al. (2020). A nonpeptidyl molecule modulates apoptosis-like cell death by inhibiting P. falciparum metacaspase-2. Biochem. J. 477, 1323–1344. doi:10.1042/BCJ20200050
Siddiqui, G., Giannangelo, C., De Paoli, A., Schuh, A. K., Heimsch, K. C., Anderson, D., et al. (2022). Peroxide antimalarial drugs target redox homeostasis in Plasmodium falciparum infected red blood cells. ACS Infect. Dis. 8 (1), 210–226. doi:10.1021/ACSINFECDIS.1C00550
Singh, M., Wang, Z., Cascio, D., and Feigon, J. (2015). Structure and interactions of the CS domain of human H/ACA RNP assembly protein Shq1. J. Mol. Biol. 427 (4), 807–823. doi:10.1016/J.JMB.2014.12.012
Singh, P. P., and Chakraborty, P. (2016). Malaria: autophagy as a potential therapeutic target. J. Pharm. Pharmacol. 4, 298–306. doi:10.17265/2328-2150/2016.07.003
Song, G., Ouyang, G., and Bao, S. (2005). The activation of Akt/PKB signaling pathway and cell survival. J. Cell. Mol. Med. 9 (1), 59–71. doi:10.1111/J.1582-4934.2005.TB00337.X
Stofberg, M. L., Caillet, C., de Villiers, M., and Zininga, T. (2021). Inhibitors of the Plasmodium falciparum Hsp90 towards selective antimalarial drug design: the past, present and future. Cells 10 (11), 2849. doi:10.3390/CELLS10112849
Strózecka, J., Chruściel, E., Górna, E., Szymańska, A., Ziȩtkiewicz, S., and Liberek, K. (2012). Importance of N- and C-terminal regions of IbpA, Escherichia coli small heat shock protein, for chaperone function and oligomerization. J. Biol. Chem. 287 (4), 2843–2853. doi:10.1074/JBC.M111.273847
Sun, Y., Zhang, J. R., and Chen, S. (2017). Suppression of alzheimer’s disease-related phenotypes by the heat shock protein 70 inducer, geranylgeranylacetone, in APP/PS1 transgenic mice via the ERK/p38 MAPK signaling pathway. Exp. Ther. Med. 14 (6), 5267–5274. doi:10.3892/etm.2017.5253
Suzuki, A., Sugiyama, Y., Hayashi, Y., Yoshida, M., Nonaka, I., Ishiura, S. I., et al. (1998). MKBP, a novel member of the small heat shock protein family, binds and activates the myotonic dystrophy protein kinase. J. Cell. Biol. 140 (5), 1113–1124. doi:10.1083/JCB.140.5.1113
Tedesco, B., Cristofani, R., Ferrari, V., Cozzi, M., Rusmini, P., Casarotto, E., et al. (2022). Insights on human small heat shock proteins and their alterations in diseases. Front. Mol. Biosci. 9, 842149. doi:10.3389/FMOLB.2022.842149
Thakur, S. S., James, J. L., Cranna, N. J., Chhen, V. L., Swiderski, K., Ryall, J. G., et al. (2019). Expression and localization of heat-shock proteins during skeletal muscle cell proliferation and differentiation and the impact of heat stress. Cell. Stress & Chaperones 24 (4), 749–761. doi:10.1007/S12192-019-01001-2
Tokunaga, Y., Otsuyama, K. I., and Hayashida, N. (2022). Cell cycle regulation by heat shock transcription factors. Cells 11 (2), 203. doi:10.3390/CELLS11020203
Treweek, T. M., Meehan, S., Ecroyd, H., and Carver, J. A. (2014). Small heat-shock proteins: important players in regulating cellular proteostasis. Cell. Mol. Life Sci. 72 (3), 429–451. doi:10.1007/S00018-014-1754-5
Unnisa, A., Greig, N. H., and Kamal, M. A. (2023). Inhibition of caspase 3 and caspase 9 mediated apoptosis: a multimodal therapeutic target in traumatic brain injury. Curr. Neuropharmacol. 21 (4), 1001–1012. doi:10.2174/1570159X20666220327222921
Usman, M. A., Salman, A. A., Ibrahim, M. A., Furukawa, K., and Yamasaki, K. (2023). Biological functions and structural biology of Plasmodium falciparum autophagy-related proteins: the under-explored options for novel antimalarial drug design. Chem. Biol. Drug Des. 101 (6), 1241–1251. doi:10.1111/CBDD.14225
Vafadar, A., Shabaninejad, Z., Movahedpour, A., Fallahi, F., Taghavipour, M., Ghasemi, Y., et al. (2020). Quercetin and cancer: new insights into its therapeutic effects on ovarian cancer cells. Cell. Biosci. 10 (1), 32. doi:10.1186/S13578-020-00397-0
Vandana, S., Shankar, S., Prasad, K. M., Kashif, M., Kalia, I., Rai, R., et al. (2020). A nonpeptidyl molecule modulates apoptosis-like cell death by inhibiting P. falciparum metacaspase-2. Biochem. J. 477 (7), 1323–1344. doi:10.1042/BCJ20200050
Van Montfort, R., Slingsby, C., and Vierling, E. (2001). Structure and function of the small heat shock protein/alpha-crystallin family of molecular chaperones. Adv. Protein Chem. 59, 105–156. doi:10.1016/S0065-3233(01)59004-X
Van Noort, J. M., Bsibsi, M., Nacken, P., Gerritsen, W. H., and Amor, S. (2012). The link between small heat shock proteins and the immune system. Int. J. Biochem. Cell. Biol. 44 (10), 1670–1679. doi:10.1016/J.BIOCEL.2011.12.010
van Ommeren, R., Staudt, M. D., Xu, H., and Hebb, M. O. (2016). Advances in HSP27 and HSP90-targeting strategies for glioblastoma. J. Neuro-Oncology 127 (2), 209–219. doi:10.1007/s11060-016-2070-8
Vercammen, D., Declercq, W., Vandenabeele, P., and Van Breusegem, F. (2007). Are metacaspases caspases? J. Cell Biol. 179, 375–380. doi:10.1083/jcb.200705193
Vince, J. E., Wong, W. W. L., Gentle, I., Lawlor, K. E., Allam, R., O’Reilly, L., et al. (2012). Inhibitor of apoptosis proteins limit RIP3 kinase-dependent interleukin-1 activation. Immunity 36 (2), 215–227. doi:10.1016/J.IMMUNI.2012.01.012
Viriyavejakul, P., Khachonsaksumet, V., and Punsawad, C. (2014). Liver changes in severe Plasmodium falciparum malaria: histopathology, apoptosis and nuclear factor kappa B expression. Malar. J. 13, 106. doi:10.1186/1475-2875-13-106
Vos, M. J., Kanon, B., and Kampinga, H. H. (2009). HSPB7 is a SC35 speckle resident small heat shock protein. Biochimica Biophysica Acta 1793 (8), 1343–1353. doi:10.1016/J.BBAMCR.2009.05.005
Wang, R., Yang, L., Li, S., Ye, D., Yang, L., Liu, Q., et al. (2018). Quercetin inhibits breast cancer stem cells via downregulation of aldehyde dehydrogenase 1A1 (ALDH1A1), chemokine receptor type 4 (CXCR4), mucin 1 (MUC1), and epithelial cell adhesion molecule (EpCAM). Med. Sci. Monit. Int. Med. J. Exp. Clin. Res. 24, 412–420. doi:10.12659/MSM.908022
Webster, J. M., Darling, A. L., Uversky, V. N., and Blair, L. J. (2019). Small heat shock proteins, big impact on protein aggregation in neurodegenerative disease. Front. Pharmacol. 10, 1047. doi:10.3389/FPHAR.2019.01047
Wegele, H., Müller, L., and Buchner, J. (2004). Hsp70 and Hsp90 a relay team for protein folding. Rev. Physiology, Biochem. Pharmacol. 151, 1–44. doi:10.1007/S10254-003-0021-1
Weinlich, R., Oberst, A., Beere, H. M., and Green, D. R. (2017). Necroptosis in development, inflammation and disease. Nat. Rev. Mol. Cell. Biol. 18 (2), 127–136. doi:10.1038/NRM.2016.149
Wintrode, P. L., Friedrich, K. L., Vierling, E., Smith, J. B., and Smith, D. L. (2003). Solution structure and dynamics of a heat shock protein assembly probed by hydrogen exchange and mass spectrometry. Biochemistry 42 (36), 10667–10673. doi:10.1021/BI034117M
Wu, C. C., and Bratton, S. B. (2011). DARK apoptosome secrets come to light. Structure 19 (1), 4–6. doi:10.1016/J.STR.2010.12.009
Wu, C. W., Biggar, K. K., Zhang, J., Tessier, S. N., Pifferi, F., Perret, M., et al. (2015). Induction of antioxidant and heat shock protein responses during torpor in the gray mouse Lemur, Microcebus murinus. Genomics, Proteomics Bioinforma. 13 (2), 119–126. doi:10.1016/J.GPB.2015.03.004
Wu, T., Mu, Y., Bogomolovas, J., Fang, X., Veevers, J., Nowak, R. B., et al. (2017). HSPB7 is indispensable for heart development by modulating actin filament assembly. Proc. Natl. Acad. Sci. U. S. A. 114 (45), 11956–11961. doi:10.1073/pnas.1713763114
Wyciszkiewicz, A., Kalinowska-Łyszczarz, A., Nowakowski, B., Kaźmierczak, K., Osztynowicz, K., and Michalak, S. (2019). Expression of small heat shock proteins in exosomes from patients with gynecologic cancers. Sci. Rep. 9 (1), 9817. doi:10.1038/S41598-019-46221-9
Xiong, J., Li, Y., Tan, X., and Fu, L. (2020). Small heat shock proteins in cancers: functions and therapeutic potential for cancer therapy. Int. J. Mol. Sci. 21 (18), 6611–6622. doi:10.3390/IJMS21186611
Yang, K., Meinhardt, A., Zhang, B., Grzmil, P., Adham, I. M., and Hoyer-Fender, S. (2012). The small heat shock protein ODF1/HSPB10 is essential for tight linkage of sperm head to tail and male fertility in mice. Mol. Cell. Biol. 32 (1), 216–225. doi:10.1128/MCB.06158-11
Yang, Q., Wang, R., and Zhu, L. (2019). Chaperone-mediated autophagy. Adv. Exp. Med. Biol. 1206, 435–452. doi:10.1007/978-981-15-0602-4_20
Yang, S., Xiao, H., and Cao, L. (2021). Recent advances in heat shock proteins in cancer diagnosis, prognosis, metabolism and treatment. Biomed. Pharmacother. 142, 112074. doi:10.1016/J.BIOPHA.2021.112074
Yin, B., Tang, S., Xu, J., Sun, J., Zhang, X., Li, Y., et al. (2019). CRYAB protects cardiomyocytes against heat stress by preventing caspase-mediated apoptosis and reducing F-actin aggregation. Cell. Stress Chaperones 24 (1), 59–68. doi:10.1007/S12192-018-0941-Y/
Yoon, S., and Rossi, J. J. (2018). Aptamers: uptake mechanisms and intracellular applications. Adv. Drug Deliv. Rev. 134, 22–35. doi:10.1016/J.ADDR.2018.07.003
Yorimitsu, T., and Klionsky, D. J. (2005). Autophagy: molecular machinery for self-eating. Cell. Death Differ. 12 (Suppl. 2), 1542–1552. doi:10.1038/SJ.CDD.4401765
Yu, Z., Jiang, N., Su, W., and Zhuo, Y. (2021). Necroptosis: a novel pathway in neuroinflammation. Front. Pharmacol. 12, 701564. doi:10.3389/FPHAR.2021.701564
Yu, Z., Zhi, J., Peng, X., Zhong, X., and Xu, A. (2010). Clinical significance of HSP27 expression in colorectal cancer. Mol. Med. Rep. 3 (6), 953–958. doi:10.3892/mmr.2010.372
Zalila, H., González, I. J., El-Fadili, A. K., Delgado, M. B., Desponds, C., Schaff, C., et al. (2010). Processing of metacaspase into a cytoplasmic catalytic domain mediating cell death in Leishmania major. Mol. Microbiol. 79 (1), 222–239. doi:10.1111/j.1365-2958.2010.07443.x
Zhang, X., Chen, W., Gao, Q., Yang, J., Yan, X., Zhao, H., et al. (2019). Rapamycin directly activates lysosomal mucolipin TRP channels independent of mTOR. PLOS Biol. 17 (5), e3000252. doi:10.1371/JOURNAL.PBIO.3000252
Zhang, X., Wang, X., Zhu, H., Kranias, E. G., Tang, Y., Peng, T., et al. (2012). Hsp20 functions as a novel cardiokine in promoting angiogenesis via activation of VEGFR2. PloS One 7 (3), e32765. doi:10.1371/JOURNAL.PONE.0032765
Zhao, K. K., Cui, Y. G., Jiang, Y. Q., Wang, J., Li, M., Zhang, Y., et al. (2013). Effect of HSP10 on apoptosis induced by testosterone in cultured mouse ovarian granulosa cells. Eur. J. Obstetrics Gynecol. Reproductive Biol. 171 (2), 301–306. doi:10.1016/J.EJOGRB.2013.09.026
Zininga, T., Ramatsui, L., and Shonhai, A. (2018). Heat shock proteins as immunomodulants. Mol. (Basel, Switz. 23 (11), 2846. doi:10.3390/MOLECULES23112846
Keywords: small heat shock proteins, molecular chaperones, biomarker candidates, Plasmodium falciparum, malaria
Citation: Timothy FM and Zininga T (2023) Small heat shock proteins as modulators of cell death in Plasmodium falciparum parasites and its human host. Front. Cell. Death 2:1322780. doi: 10.3389/fceld.2023.1322780
Received: 16 October 2023; Accepted: 14 November 2023;
Published: 29 November 2023.
Edited by:
Farhang Alem, George Mason University, United StatesReviewed by:
Yuyao Tian, Boston Children’s Hospital and Harvard Medical School, United StatesMahendra Seervi, All India Institute of Medical Sciences, India
Copyright © 2023 Timothy and Zininga. This is an open-access article distributed under the terms of the Creative Commons Attribution License (CC BY). The use, distribution or reproduction in other forums is permitted, provided the original author(s) and the copyright owner(s) are credited and that the original publication in this journal is cited, in accordance with accepted academic practice. No use, distribution or reproduction is permitted which does not comply with these terms.
*Correspondence: Tawanda Zininga, dHppbmluZ2FAc3VuLmFjLnph