- 1New Energy Research Group, Turku University of Applied Sciences, Turku, Finland
- 2Department of Mechanical and Materials Engineering, University of Turku, Turku, Finland
This contribution focuses on reducing the greenhouse gas (GHG) emissions of solar photovoltaic (PV) carport structures by replacing carbon-intensive steel with a wood-based material. There is a growing need for PV systems that are suitable for urban environments where the lack of roof spaces and open land limits the use of traditional PV installations. To date, PV carports have been mainly constructed with steel, which has a high carbon footprint and can be considered aesthetically unattractive. Wood structures, on the other hand, could act as carbon storage and thus reduce the GHG emissions of the whole system. Emissions and costs of supporting structures for PV systems have received very little attention, and there is virtually no literature specific to them. This study compares wood-based glued laminated timber (GLT) structures with conventional steel structures by investigating the GHG emissions and economic feasibility. The simulated 485 kWp system with wooden structures yielded base-case lifetime GHG emissions of 11.3 g CO2 eq/kWh in Turku Finland (60°N), and 8.2 g CO2 eq/kWh in Dijon France (47° N), representing a 48% lower value compared to systems with steel structures. Furthermore, wooden structures were competitive in terms of costs, being approximately 25% cheaper. Thus, wooden structures provide a very attractive way to make infrastructure integrated PV more sustainable.
1 Introduction
Solar photovoltaic (PV) is one of the key technologies used to attain a sustainable low-carbon energy system in Europe and globally. Even in Nordic countries, where the annual energy yield is lower than in areas closer to the equator and largely harnessed during the summer months, PV remains an important element of the 100% renewable energy mix. In the Nordic region, especially during winter, wind power outperforms solar power due to the presence of consistent strong winds, offering a significant contribution to the energy mix. During summertime, solar and wind power can together contribute to a resilient renewable energy portfolio in these challenging environments.
Article 15c of the European Union Renewable Energy Directive (RED III) defines renewable acceleration areas and requires member states to give “priority to artificial and built surfaces, such as rooftops and facades of buildings, transport infrastructure and their direct surroundings, (and) parking areas”. Explicit mention is made of the fact that concerns are typically not raised in these areas related to competing uses of space or environmental impact (THE EUROPEAN PARLIAMENT, 2023). PV carports avoid competition with other land use and answer well to the requirements of Article 15c.
In some European countries, there is already binding legislation regarding PV carports, as seen in France, where PV carports are mandatory for new and existing car parks (Legifrance, 2023). Similar legislation exists in parts of Germany (The Institute for Climate Protection and Energy and Mobility, 2023), where it applies to newly built parking areas. To date, carports have been mainly constructed with steel structures (Figure 1), which have a high carbon footprint and can be considered aesthetically unattractive. A study conducted in 2016 found that support structures for PV had a larger CO2 footprint than the PV panels themselves (Fahad and Gamalho Pereira, 2015). Recent studies highlight that substituting steel with wood has potential as a means of climate mitigation (Morris et al., 2021). Investigating how to reduce the cost and environmental impact of the support structures is thus a major issue for the PV system and, despite the urgent need for related research, is currently highly understudied. The environmental impact is even more significant with infrastructure-integrated PV systems, where the support structure is usually heavier than in rooftop or ground-mounted systems.
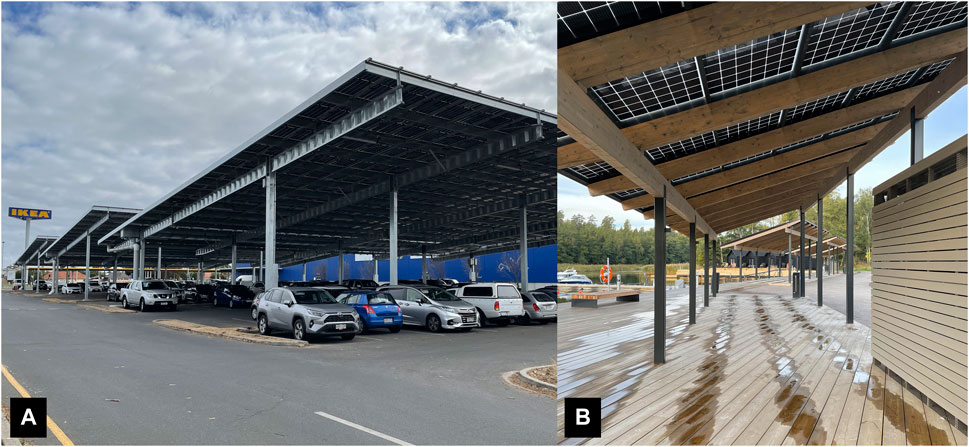
Figure 1. (A)—PV carport with similar steel structures than studied in this article, at the IKEA building in Adelaide, Australia; (B)—PV canopy with wooden GLT structures at Naantali, Finland (photographs: Samuli Ranta).
The few existing studies on PV canopies and carports primarily focus on system design, performance, and optimisation (Ranta et al., 2022; Huerta et al., 2023) rather than environmental analysis. Some other studies omit supporting structures and focuses on potential to reduce GHG emissions of PV modules and their substrate (Li et al., 2023). This study calculates lifetime electricity production and GHG emissions for a 485 kWp PV carport system in two locations: Turku, Finland (60°N) and Dijon, France (47°N). The economic and environmental benefits of using wood-based glued laminated timber (GLT) structures as replacements for steel structures in PV carport construction are analysed. GLT structures are a well-known solution in the building industry (Wood Magazine, 2021) and have been successfully used in other infrastructure constructions, such as wooden bridges (Mitterpach et al., 2023). They have proven to be robust and long-lasting solutions in these applications, making them an attractive option for PV structures as well. Finally, we consider other potential benefits of GLT structures, such as increased acceptability.
2 Methodology
2.1 Carport structure
In this study, we calculate the lifetime electricity production for a hypothetical but realistic 485 kWp PV carport that is similar to the one illustrated in Figure 1A and compare the GHG emissions and material expenses with steel- and wood-based structures. The size of the carport is 50 by 50 m, it accommodates 108 parking places, and can support a bifacial PV system of approximately 485 kWp (Figure 2). Such large carports can be located, for example, near supermarkets, shopping centres, universities, government offices, concert halls, and stadiums. The study compares hot-dip galvanised steel structures to GLT structures (untreated wood). Rainwater drainage, foundations, and paving are omitted from our calculations since these parts remain the same regardless of the structural materials used and would largely exist even without a carport. The size of the carport was chosen to be the maximum practical size for a single carport unit, considering typical maximum beam lengths, tilt angle, and height restrictions. The structure was dimensioned using commercial structural design software, with a snow load of 2.0 kN/m2, a value required in South-West Finland (Finlex, 2024).
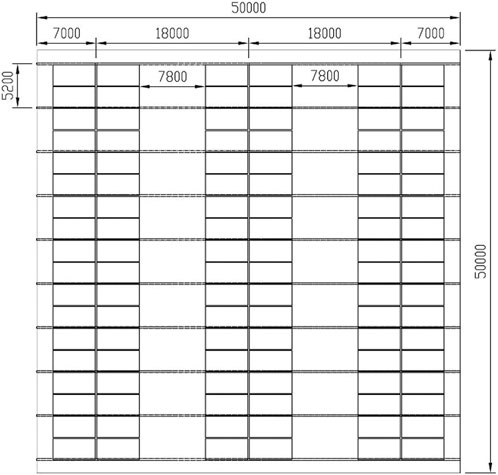
Figure 2. Studied carport measuring 50 by 50 m with 108 parking places and a bifacial PV system of approximately 485 kWp.
2.2 Weather-to-power modelling
A ray-tracing-based PV production model was used to simulate the total electricity production of the carport over a 25-years period. The typical meteorological year (TMY) data for Turku, Finland and Dijon, France were obtained from the PVGIS (Siemens, 2023) database. Geographical coordinates, GHI, DHI, DNI, air temperature, and wind speed were used as the inputs for the irradiance and power simulation. The carport’s 3D model, following the configuration shown in Figure 2, was created using Simens NX CAD software (Pelaez and Deline, 2020) for irradiance simulation. The carport is oriented towards the south and has a clearance height of 4.7 m.
The 3D model was introduced to the Bifacial Radiance toolkit (Ward, 1994) to simulate the plane-of-array (POA) irradiance on the front side of the modules using Radiance software (Súri and Hofierka, 2004). Additionally, back irradiance was simulated for the scenarios involving bifacial PV modules. The POA irradiance simulation was performed using ray-tracing technique cumulatively for each month, enabling a much faster evaluation compared to hourly simulation, while keeping the resulting annual output difference within 0.5% based on our internal comparison.
The PVsyst temperature model and single diode model, as implemented in PVlib for python (Holmgren et al., 2018), were used to simulate cell temperature and power output, respectively. The module electrical parameters of Sunpower_SPR_X22_480_COM were used for power calculation. This kind of module has a nominal power of 480Wp and a module efficiency of 22.2%, making it a suitable choice due to its good efficiency and availability in the PVlib database.
Module mismatch loss of 2%, wiring loss of 1.5%, and inverter loss of 2% were considered, using default values in PVsyst and SAM (Pvsyst 7, 2023) as references for generalised estimations. Based on the state-of-the-art technology, a moderate degradation rate of the PV modules was assumed to be 2% for the first year and 0.55% per year from the second year onwards (Longi, 2024). Possible losses due to soiling, snow coverage, and especially shading from adjacent objects which have negative impacts on power production as well as on the module’s integrity (Li et al., 2020) were omitted here, since they are highly site-specific. Carports like the one evaluated for the study are typically large and tall structures, designed for installation in expansive parking areas where shading from nearby buildings is minimal and sometimes negligible. However, carports with smaller dimensions, such as those adapted for commercial buildings within urban areas, may incur higher potential shading losses due to their proximity to surrounding structures, and in those cases, shading must be properly addressed.
2.3 Cost and GHG emission estimations
The capital expenditure (CAPEX) of both the timber- and steel-based structures was calculated based on lumber and steel futures for the end of year 2023 and considering value chain revenue expectations. The total volume or weight of each building material was taken from the structural dimensioning software and used to calculate the respective price estimation for each scenario. The purpose of these calculations was to estimate the CAPEX for each construction scenario, considering that various design decisions and local price factors can impact the final costs. In other words, the goal was to assess whether the timber-based solution is, in general, competitive against the more common steel-based one.
The GHG emissions and uptake of the timber structures were calculated based on a recent study (Linkosalmi et al., 2023). Values for cross laminated timber (CLT—Stora Enso 2022) were selected as their manufacturing and material characteristics match those of GLT. These values were then compared with another study (Ruuska, 2013) to investigate variance. Despite a 10-year difference between the studies and the use of different primary units, the results were very close. Similarly, the GHG emissions of the hot-dipped steel structures were based on another recent study (Arguillarena et al., 2021) and are presented in Table 1.
2.4 LCA analysis
The goal of this life cycle assessment (LCA) analysis is to evaluate the life cycle GHG emissions and economic feasibility of two different car port structures: GLT and steel. The functional unit is a quantified description of the function of a product that serves as the reference basis for all calculations. The functional unit for GHG impact assessment were set as 1 kg/m3, 1 kg/kg and 1 kg/PV module of materials of the hypothetical car port described in Section 2.1. The functional units for economic feasibility were set as 1 kg and 1 m3 of the materials. The system boundary of the analysis was cradle to gate.
In this study, the life cycle inventory data (GHG emissions and costs) were extracted from relevant literature (Linkosalmi et al., 2023; Rajagopalan et al., 2021). The GHG emissions of the 485 kWp PV carport systems were calculated to better understand the GHG impacts of each element in the hypothetical carport structure. Instead of using actual values for randomly selected PV module types, the base-case scenario was calculated based on the average LCA in French Ministère de la Transition Écologique tenders (367 kg CO2 eq/kWp), which represents a more realistic value of the current industry average (Ministère de la Transition Écologique, 2023). Furthermore, a worst-case scenario was considered based on the minimum requirement of the French Evaluation Carbone Simplifiée (550 kg CO2 eq/kWp) (Commission de Régulation de l’Énergie, 2023). In this scenario, the relative decrease of the CO2 emissions reaches its lowest value, providing a lower limit for emission savings when using GLT instead of steel. All LCA values focus on GHG emissions in scopes 1 and 2. Finally, the balance of system (BOS), which in this case included inverters, cables, and aluminium extrusions (excluding racking, which is calculated separately), was determined based on the literature (Rajagopalan et al., 2021).
2.5 Assumptions
Assumptions used for the GHG emissions and economical calculations:
• Uniform solar irradiance across the entire surface area of the carport throughout the year, without considering the effects of shading from nearby objects or buildings.
• The carport’s PV modules are oriented to the South to maximize solar energy capture.
• Parking area, water drainage, paving etc. are in place before carport construction and they are excluded from the analysis.
• Lifespan of the PV modules is 25 years.
• PV modules GHG emissions will reach French Ministère de la Transition Écologique tender’s requirements (Ministère de la Transition Écologique, 2023).
• Carport is installed to the location with a maximum wind or snow load requirement of 2,400 Pa.
• End-of-life GHG emissions and costs are excluded.
3 Results and discussion
3.1 Estimating GHG emissions for construction of the PV carport
If the GHG emissions of PV modules are 367 kg CO2 eq/kWp (base-case scenario), the timber structure PV carport has 53% lower total GHG emissions than the steel-structured one (Table 2). Conversely, if the GHG emissions of PV modules are more conservative, at 550 kg CO2 eq/kWp (worst-case scenario), the timber-based solution has 40% lower total GHG emissions, as shown by the values in Table 3. The main contributors to the lower GHG emissions from the timber-based solution are the significantly lower initial emissions and, equally importantly, the capability of the wood structure to act as a carbon storage. Timber products form a storage pool of wood-based carbon and can substitute environmentally problematic materials, in this case steel. The expected lifetime of timber-based structures is typically 50 years, so carbon in such structures is stored for at least that period. Since GLT is structurally a very simple product, composed only of wood, glue, and surface treatment, it is also easy to reuse or recycle after decommissioning, extending the carbon storage period beyond its first life.
The distribution of the GHG emissions is shown in Figure 3. It is notable that while the emissions from the PV modules remain the most significant part of the total emissions, the share of emissions from the steel-based construction is also significant (almost 30% for the base case, as shown in Table 2). The share of steel structures is expected to increase in the future as the efficiency of PV modules improves, leading to a reduction in their emissions. In the timber-based solution, the CO2 uptake capability of the wood can significantly offtake the emissions generated by the PV modules and make the total emissions of the system much lower (40%–53%).
3.2 Estimating the CAPEX for the construction of the system
The estimated CAPEX analysis for the timber and steel structures indicates that timber structures can also be economically competitive in the current market (Tables 4, 5). The installation costs are omitted from the calculations since they vary considerably based on local labour costs. The exact final costs for such a PV carport will vary due to market prices, local availability, and detailed design decisions. Lumber and steel futures underwent significant fluctuations (more than 100%) during the period 2020–2022, when the COVID-19 pandemic was ongoing. The futures of 2023 chosen for the current analysis are considered relatively stable, with approximately 20% cost fluctuations. Although the unit cost of timber is much higher than the unit cost of steel, the total cost of the wood-based carport is lower than that of the steel-based one (Tables 4, 5). A 10% increase in lumber futures will increase the total cost of the wood-based carport presented in Table 4 by 6%, whereas a 20% decrease in steel futures will decrease the total carport costs by 11%; thus, it would still be more expensive than the wood-based one. The main outcome of the CAPEX estimate is that even price considerations promote the use of timber-based structures for carports that reduce GHG emissions drastically.
3.3 Sensitivity analysis regarding the weight of steel
The biggest uncertainty in the calculation is the weight of the steel structures, which is influenced by two primary factors: design decisions and the selection of profile type. For example, if high-strength steel construction were used, the total weight of the system could be reduced. To study the weight impact, calculations were repeated with the weight of the steel structures reduced by 33%, which represents the maximum weight-saving potential gained by using high-strength steel. Thus, the role played by the PV modules themselves in the total CO2 emissions increased in the steel-based system. The results for the PV carport system GHG emission reduction in the base-case scenario decreased from a 53% to a 48% reduction when timber was used instead of steel (Table 6). Similarly, the results for the PV module’s GHG emissions in the worst-case scenario decreased from a 40% to a 35% reduction (Table 7).
3.4 Energy generation emissions
To calculate the total emissions of the generated electricity, the emissions of the system and its lifetime energy yield is needed. Lifetime energy production of the carport was simulated by the model described in the Methodology section. Energy yield and corresponding emissions were calculated for two locations, one for airport parking lot at Turku Finland (60° N), and another for household department store parking lot at Dijon France (47° N). Both steel and timber-based structures were considered to have a similar annual yield as differences of structural shading are minimal. The lifetime of the PV carport was assumed to be 25 years, indicating that PV panels are replaced with new ones after 25 years of operation. Energy emission calculations were also done for the 25 years, including 100% of the emissions from panels and other components. The replacement of all modules after 25 years represents a conservative scenario since the modules may have a lifetime extending beyond 30 years and substructures beyond 50 years.
The results of the energy-based emissions are presented in Table 8. When the base-case scenario PV module emissions was used, the total emissions were 8.2 g CO2 eq/kWh in Dijon and 11.3 g CO2 eq/kWh in Turku for timber structured system, and respectively, 15.9 g CO2 eq/kWh in Dijon and 21.9 g CO2 eq/kWh in Turku for steel structured system (Table 8). Results for the worst-case scenario of PV module emissions were 14.3 g CO2 eq/kWh in Dijon and 19.7 g CO2 eq/kWh in Turku for timber structured system, and respectively, 22.0 g CO2 eq/kWh in Dijon and 30.3 g CO2 eq/kWh in Turku for steel structured system (Table 9). For comparison, the emission coefficient for the electricity consumed in Finland in 2023 was 38 g CO2 eq/kWh (Fingrid, 2024). Thus, in the base-case scenario, the simulated system in Turku, Finland would produce emissions equivalent to 58% of the grid electricity with steel structures and 30% of the grid electricity with wooden structures.
3.5 Pros and cons of structure materials
The pros and cons of both construction materials are summarised in Table 9. A comparison of the emissions of the two materials has already been discussed, as have the cost aspects. Wooden structures are usually manufactured locally, so they are more readily available, including when global market disruptions occur. The usage of wooden structures reduces the EU’s dependency of the import from the countries which have a high-country risk. Local production will also have a positive impact for the local economy and thus will potentially accelerate the energy transfer. The wooden structures in a PV carport may be more acceptable to the public than steel structures for aesthetic reasons. The same phenomena are seen in construction sector in general, where it is common cover steel or concrete structures with a wood cladding to improve the aesthetic appearance and comfort. Wooden structures act as a carbon sink, which reduces the total emissions and that further improves the acceptability of the wood-based construction. On the negative side, timber-based structures require more maintenance to keep them looking good while hot-dipped galvanised steel structures are maintenance-free. Moreover, steel structures are more durable than timber ones, which can be an issue in public spaces which are more easily subject to vandalism.
Interestingly, fire safety is an area where massive wood constructions (such as GLT pillars and beams) have an advantage over steel construction: burning wood creates a carbon insulator layer on the surface of the timber element that limits the oxygen supply and reduces the temperature deeper in the wood, enabling timber beams to retain their structural integrity for longer. Unprotected steel structures, on the other hand, lose their structural integrity much faster. Fire protection for steel construction, however, would increase its GHG emissions and cost. On the other hand, if the electrical wiring shorts, untreated wood will catch fire faster than steel. However, chemical treatments to increase the fire resistance of wood also increase its GHG emissions and costs at the end-of-life stage.
4 Conclusion
The results show a significant potential for GHG emission reduction from using timber-based structures for PV carport systems, namely, 53% for total emissions in the base-case scenario. This advantage is expected to increase in the future as the efficiency of PV modules continues to increase and their GHG emissions to decrease. The third-generation organic and perovskite solar cells have a high potential of reducing the greenhouse gas emissions of the PV modules even more when become available (Li et al., 2022). Therefore, addressing GHG emissions related to mounting and supporting structures becomes increasingly important. Furthermore, this study indicates that wood-based structures can be approximately 25% cheaper. These costs were calculated based on the most recent pricing, and potential cost reductions are subject to changes due to shifts in markets. Emissions from energy production depend on the initial emissions of photovoltaic modules, BOS components and substructures, but also on the installation locations. In the two locations studied, Turku Finland (60° N), and Dijon France (47° N), energy generation emissions decreased by 48% in the base-case scenario and correspondingly by 35% in the worst-case scenario.
In addition to the clear improvements in emission reductions, timber structures offer other advantages over steel structures. For instance, GLT structures are usually produced locally and thus support local economies. In general, timber prices are less vulnerable to a global economic crisis than steel structures and pose lower supply-chain risks. Furthermore, wooden structures could be safer than steel ones in the event of fire since steel structures quickly collapse after a critical temperature is reached. The massive increase in PV energy production capacity poses a new challenge for the PV sector in terms of gaining public acceptance. Therefore, aesthetically pleasing structures that avoid compromising other land uses, such as timber-based PV carports, provide a fascinating and easily acceptable solution.
Data availability statement
The raw data supporting the conclusion of this article will be made available by the authors, without undue reservation.
Author contributions
SR: Conceptualization, Methodology, Writing–original draft, Writing–review and editing. EA: Data curation, Writing–original draft, Writing–review and editing. HH: Methodology, Software, Writing–review and editing. SW: Data curation, Software, Writing–review and editing. SJ: Data curation, Writing–review and editing. KM: Supervision, Writing–review and editing.
Funding
The author(s) declare that financial support was received for the research, authorship, and/or publication of this article. This work was carried out as part of the Resilient and Accessible Power System with Large Shares of Solar Energy (RealSolar) project. RealSolar is funded by the Strategic Research Council (SRC) established within the Research Council of Finland (decision No. 358542/359141). The work has also received partial funding from the European Union’s Horizon 2020 research and innovation program under Grant Agreements 957751 (RESPONSE).
Acknowledgments
EA thanks the Research Council of Finland (ECOSOL, decision No. 347275).
Conflict of interest
The authors declare that the research was conducted in the absence of any commercial or financial relationships that could be construed as a potential conflict of interest.
Publisher’s note
All claims expressed in this article are solely those of the authors and do not necessarily represent those of their affiliated organizations, or those of the publisher, the editors and the reviewers. Any product that may be evaluated in this article, or claim that may be made by its manufacturer, is not guaranteed or endorsed by the publisher.
Abbreviations
BOS, Balance of System; CAPEX, Capital Expenditure; CLT, Cross Laminated Timber; GHG, Greenhouse Gas; GLT, Glued Laminated Timber; LCA, Life Cycle Assessment; PV, Photovoltaic.
References
Arguillarena, A., Margallo, M., and Urtiaga, A. (2021). Carbon footprint of the hot-dip galvanisation process using a life cycle assessment approach. Clean. Eng. Technol. 2, 100041. doi:10.1016/j.clet.2021.100041
Commission de Régulation de l’Énergie (2023). 20230 Deliberation N°2023-108 de la du 19 avril 2023 portant avis sur le projet de cahier des charges de l’appel d’offres portant sur la réalisation et l’exploitation d’installations de production d’électricité à partir de l’énergie solaire et situées dans les zones non interconnectées. Available at: https://www.cre.fr/content/download/28112/file/230419_2023-108_AO_PPE2_PV_ZNI.pdf (Accessed on December 21, 2023).
Fahad, A., and Gamalho Pereira, C. (2015). Dematerializing of PV for better source of energy, sustainable built environment. University of New South Wales. doi:10.13140/RG.2.1.5043.5442
Fingrid (2024). real-time-co2-emissions-estimate. Available at: https://www.fingrid.fi/en/electricity-market-information/real-time-co2-emissions-estimate/ Accessed January 26, 2024.
Finlex (2024). Normit. Available at: https://www.finlex.fi/data/normit/42811/asetus_6-16_2016.pdf Accessed January 26, 2024.
Holmgren, W. F., Hansen, C. W., and Mikofski, M. A. (2018). Pvlib python: a python package for modeling solar energy systems. J. Open Source Softw. 3 (29), 884. doi:10.21105/joss.00884
Huerta, H., Ranta, S., Wang, S., Heinonen, A., Jouttijärvi, S., Driesse, A., et al. (2023). Bifacial PV systems at high latitude: modeling and validation with monitoring data, 2023. Lisbon Portugal: EU PVSEC. doi:10.4229/EUPVSEC2023/4BO.5.4
Legifrance (2023). LOI n° 2023-175 du 10 mars 2023 relative à l’accélération de la production d’énergies renouvelables. Available at: https://www.legifrance.gouv.fr/dossierlegislatif/JORFDOLE000046329719/ (Accessed on December 21, 2023).
Li, Q., Monticelli, C., Kutlu, A., and Zanelli, A. (2023). Feasibility of textile envelope integrated flexible photovoltaic in Europe: carbon footprint assessment and life cycle cost analysis. J. Clean. Prod. 430, 139716. 0959-6526. doi:10.1016/j.jclepro.2023.139716
Li, Q., Monticelli, C., and Zanelli, A. (2022). Life cycle assessment of organic solar cells and perovskite solar cells with graphene transparent electrodes. Renew. Energy 195, 906–917. 0960-1481. doi:10.1016/j.renene.2022.06.075
Li, Q., Zhu, L., Sun, Y., Lin, L., and Yang, Y. (2020). Performance prediction of Building Integrated Photovoltaics under no-shading, shading and masking conditions using a multi-physics model. Energy 213, 118795. 0360-5442. doi:10.1016/j.energy.2020.118795
Linkosalmi, L., Schwarzschachner, H., and Valtonen, T. (2023). “Harmonisation of the environmental product declarations for wood products,” in 13th world conference on timber engineering, WCTE 2023 (Oslo Norway: Curran Associates Inc), 4565–4570. (Accessed on December 21, 2023). doi:10.52202/069179-0594
Longi (2024). Datasheet of longi hi-MO 5m LR5-54HPH. Available at: https://static.longi.com/Hi_MO_5m_L_Gi_LE_PM_T_PMD_059_F116_LR_5_54_HPH_405_425_M_G2_and_G3_30_30_and_15_Frame_V19_0_EN_2f2d356994.pdf (Accessed on January 26, 2024).
Ministère de la Transition Écologique (2023). Les panneaux solaires bas-carbone en France: un enjeu environnemental, une opportunité industrielle? Available at: https://www.ecologie.gouv.fr/sites/default/files/document_travail_53_panneaux_solaires_octobre2021_3.pdf (Accessed on December 21, 2023).
Mitterpach, J., Fojtík, R., Machovčáková, E., and Kubíncová, L. (2023). Life cycle assessment of a road transverse prestressed wooden–concrete bridge. Forests 14, 16. doi:10.3390/f14010016
Morris, F., Allen, S., and Hawkins, W. (2021). On the embodied carbon of structural timber versus steel, and the influence of LCA methodology. Build. Environ. 206, 108285. doi:10.1016/j.buildenv.2021.108285
Pelaez, A.Deline (2020). bifacial_radiance: a python package for modeling bifacial solar photovoltaic systems. J. Open Source Softw. 5 (50), 1865. doi:10.21105/joss.01865
Rajagopalan, N., Smeets, A., Peeters, K., De Regel, S., Rommens, T., Wang, K., et al. Preliminary environmental and financial viability analysis of circular economy scenarios for satisfying PV system service lifetime (2021). Report T12-21:2021, 12. International Energy Agency (IEA) PVPS Task. 978-3-90728123-9. (Accessed on December 21, 2023) doi:10.2172/1832876
Ranta, S., Huerta, H., Jouttijärvi, S., Heinonen, A., and Driesse, A. (2022). Bifacial PV canopy system in high latitude, model development and validation with first months of monitoring data. 8th World Conf. Photovolt. Energy Convers. doi:10.4229/WCPEC-82022-4DO.5.6
A. Ruuska (2013). Carbon footprint for building products: ECO2 data for materials and products with the focus on wooden building products (Espoo: VTT Technical Research Centre of Finland).
Siemens (2023). NX CAD and CAM software. Available at: https://plm.sw.siemens.com/en-US/nx/ Accessed on December 21, 2023.
Súri, M., and Hofierka, J. (2004). A new GIS-based solar radiation model and its application to photovoltaic assessments. Trans. GIS 8 (2), 175–190. doi:10.1111/j.1467-9671.2004.00174.x
THE EUROPEAN PARLIAMENT (2023). Directive (EU) 2023/2413 of the European parliament and of the Council of 18 october 2023 amending directive (EU) 2018/2001, regulation (EU) 2018/1999 and directive 98/70/EC as regards the promotion of energy from renewable sources, and repealing Council directive (EU) 2015/652. Available at: http://data.europa.eu/eli/dir/2023/2413/oj (Accessed on December 21, 2023).
The Institute for Climate Protection, Energy and Mobility (2023). Mandatory PV installation on every car park? Position paper. Available at: https://www.ikem.de/en/mandatory-pv-installation-on-every-car-park (Accessed December 21, 2023).
Ward, G. J. (1994). “The RADIANCE lighting simulation and rendering system,” in Proceedings of the 21st annual conference on Computer graphics and interactive techniques (New York (NY, USA): ACM), 459–472. doi:10.1145/192161.192286
Wood Magazine (2021). Wood city – the flagship for Finnish wood construction. Available at: https://puuinfo.fi/2021/09/16/wood-city-the-flagship-for-finnish-wood-construction/?lang=en (Accessed on December 21, 2023).
Keywords: PV carport, life cycle assessments, GLT structures, feasability analysis, infrastructure integrated photovoltaics (IIPV)
Citation: Ranta S, Akulenko E, Huerta H, Wang S, Jouttijärvi S and Miettunen K (2024) Feasibility and greenhouse gas emissions of timber structures in solar photovoltaic carport construction. Front. Built Environ. 10:1379956. doi: 10.3389/fbuil.2024.1379956
Received: 31 January 2024; Accepted: 16 April 2024;
Published: 02 May 2024.
Edited by:
Martin Thebault, UMR5271 Laboratoire d'Optimisation de la Conception et Ingénierie de l'Environnement (LOCIE), FranceReviewed by:
Francesco Barreca, Mediterranea University of Reggio Calabria, ItalyQingxiang Li, The Chinese University of Hong Kong, China
Copyright © 2024 Ranta, Akulenko, Huerta, Wang, Jouttijärvi and Miettunen. This is an open-access article distributed under the terms of the Creative Commons Attribution License (CC BY). The use, distribution or reproduction in other forums is permitted, provided the original author(s) and the copyright owner(s) are credited and that the original publication in this journal is cited, in accordance with accepted academic practice. No use, distribution or reproduction is permitted which does not comply with these terms.
*Correspondence: Samuli Ranta, c2FtdWxpLnJhbnRhQHR1cmt1YW1rLmZp