- 1Division of Sustainable Development, College of Science and Engineering, Hamad Bin Khalifa University, Doha, Qatar
- 2Qatar Environment and Energy Research Institute, Hamad Bin Khalifa University, Doha, Qatar
Heat recovery from roads is a promising technology to address the urban heat island effect. This review paper aims to provide a comprehensive analysis of the current state and future directions of heat recovery from roads to address the urban heat island effect while generating renewable energy. The study covers various aspects such as theoretical background, economic feasibility, environmental impact, and materials design techniques. A systematic search of relevant literature was conducted to analyze and synthesize different heat recovery systems’ efficiency, performance, and potential. The paper also discusses the economic feasibility and environmental impact of these systems, including installation and maintenance costs, revenue generation, and local ecosystem impact. The review explores the role of different materials, such as PEX pipe, asphalt mix, and carbon nanotubes, in improving heat harvesting systems’ efficiency. The paper concludes with a discussion of research gaps and future directions in the field of heat recovery from roads. This study provides a valuable resource for researchers, practitioners, and policymakers interested in sustainable and efficient energy system development.
Highlights
- Comprehensive analysis of the potential of heat recovery from roads to address urban heat island effect and generate renewable energy.
- Coverage of various aspects, including theoretical background, economic feasibility, environmental impact, and materials design techniques.
- Exploration of different materials, such as PEX pipe, asphalt mix, and carbon nanotubes, in improving heat harvesting systems’ efficiency.
- Discussion of research gaps and future directions in the field of heat recovery from roads.
1 Introduction and background
As the world becomes increasingly urbanized, the negative effects of urbanization on the environment and public health have become more apparent. One of the most pressing issues facing urban areas is the urban heat island (UHI) effect, which results in higher temperatures in urban areas compared to surrounding rural areas. UHI is a phenomenon where urban areas experience higher temperatures than surrounding rural areas due to the absorption and retention of heat by urban materials such as concrete and asphalt. The increased temperature in urban areas can have significant impacts on energy consumption, air quality, and public health (Marando et al., 2022).
In the unique climate of arid and desert regions like the Middle East, the Urban Heat Island (UHI) effect presents a distinctive challenge and opportunity. Here, road surfaces frequently reach extreme temperatures, often exceeding 80°C, exacerbating not only the discomfort of UHI but also hastening the degradation of asphalt infrastructure (Abotaleb et al., 2022). Intriguingly, these elevated temperatures align with the ranges observed in geothermal sources (Gude, 2018), making them ideal for innovative applications such as low-temperature multieffect desalination. This phenomenon transforms from a mere environmental challenge into a valuable resource in landscapes plagued by freshwater scarcity. This review delves into the potential of heat recovery systems in these settings, emphasizing their dual benefits: enhancing urban livability and extending infrastructure longevity, while also offering a sustainable avenue for harnessing excess heat.
In such arid regions, there is a need for effective strategies to mitigate the effects of UHI, and the use of road-based heat recovery systems is a promising approach (Debbage and Shepherd, 2015). UHI is caused by alterations of surface area, improper urban planning, air pollution, and anthropic activities. The UHI effect can cause discomfort to urban dwellers, human casualties, and a decline in climate. There are various mitigation measures to encounter the UHI effect, including using high albedo materials and pavements, green vegetation and green roofs, urban planning, pervious pavements, shade trees, and the existence of water bodies in city areas. Green vegetation seems to be the most effective measure, and other strategies can also play a major role under proper conditions. The spatial contiguity of urban development, regardless of its density or degree of sprawl, is a critical factor that influences the magnitude of the UHI effect (Vargo et al., 2016; Shaker et al., 2019; Zulian et al., 2021). UHI phenomenon can lead to increased energy consumption, reduced air quality, and negative impacts on public health. To mitigate the effects of UHI, there has been a growing interest in the use of heat recovery technologies, and one promising approach is the use of roads as a source of energy. Roads absorb a significant amount of solar radiation during the day, leading to high surface temperatures that can be used for various applications, such as heating buildings, producing hot water, or generating electricity (Nuruzzaman, 2015; Zheng et al., 2022a). Despite the potential benefits of road-based heat recovery systems, there is still limited implementation of such technologies. This is largely due to the lack of knowledge on the effectiveness, feasibility, and limitations of these systems. Therefore, there is a need for a comprehensive review of the existing literature on heat recovery from roads for mitigating UHI (Debbage and Shepherd, 2015). Heat recovery from roads for mitigating UHI involves the application of various engineering principles, including heat transfer mechanisms, types of heat recovery systems, factors affecting the efficiency of heat recovery, and applications of heat recovered from roads (Nuruzzaman, 2015; Pratiwi, 2018).
Heat transfer mechanisms play a critical role in the design and operation of road-based heat recovery systems (Marshall et al., 2017). The three fundamental modes of heat transfer are conduction, convection, and radiation. Conduction is the transfer of heat through a solid material, while convection is the transfer of heat through a fluid medium. Radiation is the transfer of heat through electromagnetic waves. The efficiency of heat transfer mechanisms depends on various factors (Gui et al., 2007), such as the material properties of the road surface, air temperature, wind speed, and humidity (Pratiwi, 2018; C. F; Wang et al., 2010). There are several types of heat recovery systems used for extracting heat from roads, including air-based systems, liquid-based systems, and thermoelectric systems. Air-based systems involve using a fan to blow air over the heated road surface (Q. Xu et al., 2019), while liquid-based systems use a fluid medium, such as water or a glycol solution, to extract heat from the road surface (Cheng et al., 2021). Thermoelectric systems use the Seebeck effect to generate electricity from the temperature difference between the road surface and the ambient air (Hasebe et al., 2006).
The efficiency of heat recovery systems from roads depends on various factors, such as the thermal conductivity of the road surface material, the temperature difference between the road surface and the ambient air, the type of heat recovery system used, and the wind speed. In addition, factors such as the time of day, season, and location of the road surface also affect the efficiency of heat recovery systems. The efficiency of heat transfer in heat recovery systems is influenced by several factors, as revealed by various studies (Yang, 2005). reported that the choice of heat transfer technology is a critical determinant of efficiency. Additionally (Quoilin et al., 2011), identified the design and operation of the heat recovery system as a key factor, including the heat transfer surface area, heat transfer coefficient, fluid flow rate, and temperature difference between the hot and cold fluids. The quality and characteristics of the waste heat source, such as temperature and flow rate, were noted as essential factors affecting heat transfer efficiency. The maintenance and cleaning of the heat recovery system also play a crucial role in determining its efficiency (Qihao et al., 2007).
Wasted heat is dumped directly into the environment even though it could still be reused for economic purposes. Waste heat recovery enhances the system’s overall efficiency and reduces emissions, a clean and environmentally friendly solution. The applications of heat recovered from roads and the potential practical applications of this energy. In general, a significant amount of heat energy can be recovered from roads, particularly in urban areas where roads cover a large proportion of the land surface. The use of thermoelectric generators, heat exchangers, and heat pumps are some of the technologies that can be utilized to recover this energy (Hasebe et al., 2006). Other diverse range of applications for the recovered energy are pavement de-icing, space heating, and hot water production, the use of heat energy recovered from roads could be a promising solution to address the hazards of driving on icy roads in cold climates. This could be achieved by circulating heated fluid through pipes embedded in the pavement surface, a method that has been successfully piloted in a few projects. This approach has the potential to reduce the use of salt and other chemicals for de-icing, thereby benefiting the environment (Pei et al., 2019). Regarding space heating, the use of heat pumps to extract heat from the fluid circulating through the pavement-embedded pipes and transfer it to the heating systems of buildings located near roads. This approach could be particularly effective in urban areas with dense building clusters and significant road coverage (Pallua, 2021). The potential benefits of this approach include reducing reliance on fossil fuels for heating and consequent environmental and economic benefits. Nonetheless, further research is required to ascertain the economic feasibility of this approach and the potential impact on pavement durability (Quoilin et al., 2011; Pei et al., 2019).
Figure 1 shows a graphic representation of the Urban Heat Island (UHI) phenomenon and its mitigation strategies in an urban setting. The image features a Residential/Commercial Building and an Urban Green Space on either side of an Asphalt Road Surface, illustrating the sources and solutions for UHI. Red arrows indicate heat absorption by the asphalt road, which can reach temperatures up to 80°C, significantly contributing to UHI. Another red arrow, rising from the building, shows heat emissions from urban structures, exacerbating UHI. Blue arrows represent cooling strategies. One arrow from the Urban Green Space shows the cooling effect of vegetation in reducing air temperatures and countering UHI. Another blue arrow depicts the cooling impact of a Heat Recovery System, which reduces heat by repurposing energy. A green circular arrow in the bottom right corner highlights the innovative use of clean energy for Desalination, proposing a dual-purpose approach for UHI mitigation and clean water supply, symbolizing sustainable urban development. A legend clarifies that red arrows symbolize heat flow and blue arrows indicate cooling effects, providing a comprehensive view of the relationship between urban infrastructure and the environment, emphasizing integrated solutions for UHI challenges.
1.1 Importance and relevance of the topic
The effects of UHI can be felt in various ways, including increased energy consumption, reduced air quality, and negative impacts on public health. The potential of heat recovery systems in mitigating the urban heat island effect is particularly pronounced in specific climates, such as those of arid and desert regions like the Middle East. In these environments, road surfaces frequently reach extreme temperatures, sometimes soaring to 80°C (Abotaleb et al., 2022), contributing not only to the formation of uncomfortable urban heat islands but also to the accelerated deterioration of asphalt. This phenomenon aligns closely with the temperature ranges of most geothermal sources, which typically range from 70 to 90°C (Gude, 2018), rendering them ideal for applications like low temperature multieffect desalination, but not for power generation.
In such arid landscapes, where freshwater scarcity is a pressing issue, desalination emerges as a key method for obtaining essential freshwater. The excessive heat accumulated in asphalt surfaces, thus, transcends from being a mere environmental challenge to an untapped resource. Research by Abotaleb et al. (2022) underscores the dual benefits of implementing cooling measures on these roads: enhancing urban livability and extending the durability of the roads, while concurrently providing a sustainable method to utilize the excess heat. This review underscores the geographical variability in the effectiveness and applicability of heat recovery systems, particularly highlighting the transformative potential they hold for urban sustainability in arid regions like the Middle East.
Heat recovery from roads presents a promising solution to mitigate the effects of UHI by harnessing the thermal energy stored in roads (Jain et al., 2022; Sharma et al., 2009). The importance of road-based heat recovery systems lies in their potential to reduce the energy consumption and carbon emissions associated with traditional heating and cooling systems. Additionally, they offer the potential for cost savings, as the heat recovered from roads can be used to supplement or replace conventional energy sources (Boyd et al., 2022; Ibrahim et al., 2018a; O’Malley et al., 2015). Furthermore, road-based heat recovery systems can also help to mitigate the negative effects of UHI by reducing the temperature of urban surfaces, which can lead to improved air quality, reduced heat-related illnesses, and increased comfort for urban residents. Given the increasing interest in sustainable urban development and the need to reduce carbon emissions, there is a growing need to explore innovative solutions such as heat recovery from roads (Solecki et al., 2005).
1.2 Purpose and scope of the review
This systematic review aims to provide a comprehensive analysis of the current state of research on this topic, identify knowledge gaps, and suggest future directions for research and development of road-based heat recovery systems. The review will synthesize the existing literature on the theoretical background, types of heat recovery systems, factors affecting the efficiency of heat recovery, and applications of heat recovered from roads The review will focus on research studies that investigate the potential for heat recovery from roads in urban areas, including laboratory and field studies, as well as case studies and pilot projects. The review will also highlight knowledge gaps and research needs and suggest future directions for the development of road-based heat recovery systems for mitigating the UHI effect.
This work will provide a useful resource for researchers, practitioners, and policymakers interested in the potential for heat recovery from roads as a sustainable and effective solution for mitigating the effects of UHI in urban areas by addressing the following research questions:
• What is the current state of research on heat recovery from roads as a method for mitigating UHI, and what are the main findings and trends in this area?
• What are the different types of heat recovery systems that have been investigated for recovering heat from roads, and what are the factors that affect their efficiency and performance?
• What are the main applications of the heat recovered from roads, and what are the economic, environmental, and social benefits and challenges associated with these applications?
• What are the key knowledge gaps and research needs in the field of road-based heat recovery systems, and what are the future directions for research and development in this area?
1.3 Objective of review:
The objective of the review paper research can be summarized as follows.
• To identify knowledge gaps, lay the groundwork for future research, assess global and Middle Eastern significance, and aid regional research and feasibility studies in the Middle East.
• To develop and refine efficient heat recovery systems for urban heat management.
• Provide scientific research driven insights to shape policies and urban planning strategies for mitigating UHI effects.
• Advocate for sustainable and environmentally friendly practices in urban development to combat the UHI phenomenon.
2 Methods and procedures
This systematic review followed the Preferred Reporting Items for Systematic Reviews and Meta-Analyses (PRISMA) guidelines to analyze the current state and future directions of heat recovery from roads as a solution to mitigate the UHI effect and generate renewable energy. The study defined the research question and inclusion and exclusion criteria, including articles that discuss the efficiency, performance, and potential of different heat recovery systems, their economic feasibility, and environmental impact, while excluding articles that do not discuss heat recovery systems or are not related to the research question.
2.1 Search strategy and selection criteria
The full text of the selected articles was assessed for eligibility based on the inclusion criteria, and relevant data was extracted from the eligible articles, such as study design, sample size, data analysis, and results. The data from the selected articles was synthesized and presented in a systematic review format, following the PRISMA guidelines (Page et al., 2021). Data was reviewed, sorted, and analyzed and the results are presented in different visual formats such as tables and graphs. Finally, the implications of the findings were discussed, research gaps were identified, and future directions for research were suggested by comparing the results of the selected articles, identifying common themes, and discussing their relevance to the research question. This systematic review will aid in providing critical information and will act as a valuable resource for researchers, practitioners, and policymakers interested in the potential for heat recovery from roads as a sustainable and effective solution for mitigating the effects of UHI in urban areas. Figure 2 illustrates the scheme and selection criteria used in screening the articles for the review, where the total number of articles after the final screening was 90, while initially, 1892 articles were found from three databases that had relevance with the research topic.
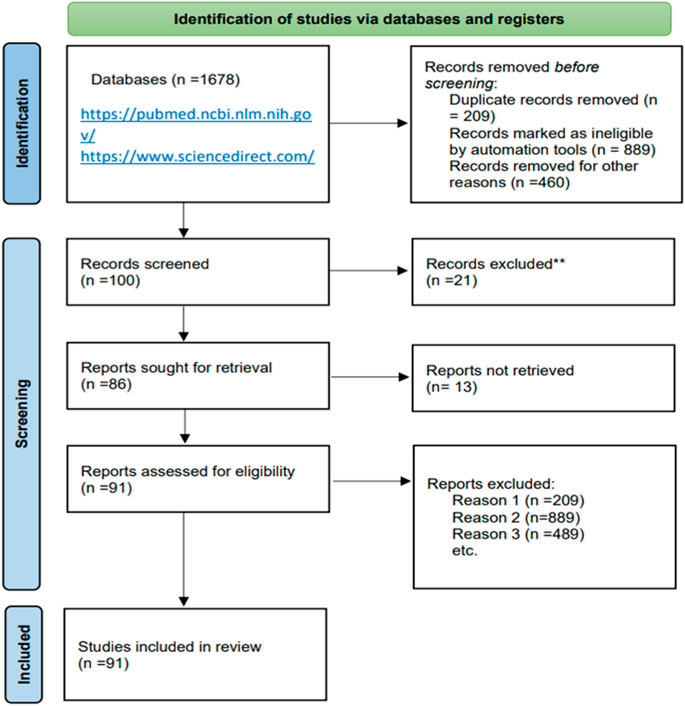
FIGURE 2. Flow diagram illustrating the literature review process for heat recovery from roads, detailing the stages of identification, screening, and final selection of papers.
2.2 Data sources and databases
A systematic search of relevant literature was conducted using appropriate databases and search engines, such as Science Direct, PubMed, Google Scholar, and Scopus, based on the defined inclusion and exclusion criteria. The articles were screened independently by two or more reviewers based on their titles and abstracts to identify the ones that met the inclusion criteria. While conducting the literature review, the Mendeley reference management software was employed to facilitate the systematic organization and effective management of the research articles that were collected. This tool, designed for ease of use, optimized the workflow by simplifying the importation, storage, and arrangement of relevant sources, while also aiding in the generation of citations and bibliographies.
3 Materials design techniques
Materials design techniques are vital for enhancing the performance of heat recovery systems from roads, which can help mitigate the UHI effect (Xu et al., 2021). Various materials have been used in the development of these systems, such as polyethylene (PEX) pipes (Yoshitake et al., 2011), asphalt mixes (Piao et al., 2021), and. carbon nanotubes (Han and Fina, 2011).
3.1 Overview of the importance of materials in heat harvesting systems
Studies prove that many materials design techniques are essential for improving the performance of heat recovery systems from roads to mitigate the UHI effect. Various materials are used for this purpose, including PEX pipes, asphalt mixes, and carbon nanotubes (Han and Fina, 2011; Yoshitake et al., 2011). PEX pipes have high thermal conductivity and flexibility, while asphalt mixes can be modified with additives to improve thermal properties. Carbon nanotubes are being explored for their potential to enhance thermal conductivity significantly. Other materials such as phase change and thermoelectric materials are also promising for thermal energy storage and electricity conversion, respectively. Researchers are also exploring new materials design techniques, including 3D printing and machine learning, to optimize the performance of these systems. These innovative approaches allow for tailored material properties and the efficient design and optimization of materials for heat recovery applications.
3.2 Comparison of different materials for heat recovery
The comparison of different materials used for heat recovery from various studies proved that PEX pipes have the highest thermal conductivity and are also flexible and durable, making them a popular material choice in heat recovery systems. Carbon nanotubes have high thermal conductivity but are expensive to produce. Asphalt mixes can be modified to enhance their thermal properties, but their thermal conductivity is only moderate. Phase Change Materials (PCMs) have the potential to store large amounts of thermal energy (Zhang et al., 2006), but they are expensive. Thermoelectric materials have the added benefit of generating electricity from waste heat, but they are not yet widely available (Esteves and Lazard, 2020). A comparison of different materials used for heat recovery from roads was performed through, comparative research findings.
3.3 Advanced design techniques
Advanced design techniques for improving heat transfer efficiency are crucial for developing effective heat recovery systems aimed at mitigating UHI effects. Various advanced design techniques have been explored in the literature, and some of the most promising ones are discussed. Microfluidic channels (Davies et al., 2014), Nanofluids, advanced heat exchanger designs, phase change materials, and coatings made of nanomaterials can significantly improve the performance of heat recovery systems. By increasing the surface area-to-volume ratio, microfluidic channels enhance heat transfer efficiency (Marshall et al., 2017). Nanofluids have high thermal conductivity and can be used to enhance the thermal properties of base fluids (Hajatzadeh Pordanjani et al., 2019). Phase change materials can improve the thermal storage capacity of heat recovery systems. Coatings made of nanomaterials can improve heat exchanger surfaces and increase heat transfer efficiency. To identify the most effective advanced design techniques, a graphical representation of their heat transfer coefficients can be used. One such technique is the use of porous asphalt pavement, which has been found to mitigate the effects of urban heat islands.
4 Environmental impact
In evaluating the ecological ramifications of heat harvesting systems that recover heat from roads, it is imperative to contemplate their potential impact on the ambient milieu, including the quality of air and water, as well as the level of noise emission. The implementation and functioning of said systems may result in amplified emissions from construction machinery and augmented vehicular movement, thereby potentially exacerbating the issue of air pollution (Aneesa and Reshma, 2022).
The literature proposes that heat harvesting systems utilizing heat recovery from roads may prove effective in the urban heat island phenomenon. The potential impact of heat harvesting systems on wildlife and ecosystems must also be considered. In certain instances, there may be a need to eliminate plant life or disturb the natural habitats of animals, resulting in adverse effects on biodiversity (Harlan and Ruddell, 2011a). It is crucial to take into account not solely the ecological ramifications, but also the financial and societal implications of the technology. For instance, it is imperative to consider the cost-effectiveness, employment generation potential, and community acceptance while evaluating the feasibility of the proposal. Heat harvesting systems have the potential to generate substantial economic advantages through the mitigation of energy expenses and the establishment of employment opportunities within the renewable energy industry. Conversely, individuals may encounter societal obstacles, such as adverse visual stimuli and auditory contamination that may be deemed unfavorable by the general populace (Ando Junior et al., 2018). In order to guarantee a thorough and precise evaluation, it is imperative to engage stakeholders from diverse sectors, including governmental bodies, industries, and community organizations. Through this approach, it is possible to take into account multiple perspectives and guarantee that the evaluation aligns with the requirements and preferences of the society (Yang, 2005).
The Table 1 presents a concise overview of the key environmental aspects associated with heat harvesting systems. These systems, primarily designed to recover heat from roadways, have far-reaching impacts on the urban environment and ecological balance. This section categorizes these impacts into three primary areas.
4.1 Heat island effect and cooling impact
As per the scholarly literature, the deployment of heat harvesting mechanisms that retrieve thermal energy from roadways has the potential to serve as a feasible remedy for mitigating the urban heat island phenomenon. A detail review of the literature findings can are tabulated in Table 2.
The research papers collectively suggest that road pavement can have a significant impact on the UHI effect (Ibrahim et al., 2018a). Conducted a study that suggests that the UHI phenomenon may be intensified by the presence of asphalt pavement. Conversely, the use of StormPav, a type of pavement composed of concrete, permeable materials, and industrialized building systems (IBS), may serve to mitigate the UHI effect.
The employment of heat harvesting mechanisms has been recognized as a feasible strategy to tackle the urban heat island phenomenon. It is imperative to consider the impact of road pavement and other pertinent variables when deploying such systems. The proper selection of pavement type and design, in conjunction with the incorporation of supplementary measures to mitigate the UHI effect, can enhance the effectiveness of heat harvesting systems and promote the advancement of a more environmentally sustainable and adaptable urban environment.
4.2 Carbon footprint
The term carbon footprint refers to the total amount of greenhouse gas emissions resulting from human activities like deforestation, industrial processes, and burning of fossil fuels. The emission of greenhouse gases contributes to climate change, which poses a significant threat to the planet and its inhabitants. According to existing literature, the utilization of thermal energy from asphalt pavements has the capability to alleviate the UHI. Nonetheless, a scarcity of information exists concerning the carbon emissions linked to these technologies. The findings on the literature review for heat harvesting system’s impact on carbon footprint are summarized in Table 3.
To mitigate the impacts of climate change, there is a need to prioritize the reduction of carbon footprint in all human activities. Energy-efficient technologies like heat harvesting systems, combined with sustainable practices, can help us achieve this goal while ensuring a more sustainable future for ourselves and future generations (Balany et al., 2020).
4.3 Impact on local ecosystems
The literature posits that the recuperation of heat from roadways can yield both favorable and unfavorable consequences for nearby ecosystems and the environment with respect to the UHI phenomenon. The key findings on impact on local ecosystems are presented in Table 4.
The effect of heat recovery from roads on local ecosystems is a complex matter, greatly influenced by the specific type and design of pavement employed. Some types of pavement materials can exacerbate the UHI effect and have detrimental effects on local ecosystems. However, there are also pavement materials that are specifically engineered to prioritize environmental sustainability, which can effectively reduce these negative impacts. The research highlights the importance of careful planning and the use of environmentally friendly pavement solutions as part of a larger approach to harmonizing urban development and ecological preservation. When considering the topic at hand, the thoughtful utilization of heat recovery from roads can be seen as a sustainable and environmentally aware approach to address the issue of urban heat island effect and safeguarding local ecosystems.
5 Economic feasibility
Economic feasibility is an important consideration in the development and implementation of heat harvesting systems, as it helps determine whether the system is financially viable and sustainable in the long term. The assessment of economic feasibility is crucial for ensuring the successful adoption and implementation of heat harvesting systems as a sustainable solution for mitigating the UHI effect (Quoilin et al., 2011). The economic viability of heat harvesting systems is influenced by various factors, including the cost and availability of materials, their thermal conductivity and durability, and the efficiency of the design techniques used. PEX pipes are the most economical choice due to their high thermal conductivity, durability, and widespread availability. However, carbon nanotubes, while more expensive and less durable, offer even greater thermal conductivity. Asphalt mix, commonly used for pavement construction, provides a moderate level of thermal conductivity and is widely available at a low cost (Nwakaire et al., 2020a; Kumar et al., 2022). Phase change materials, although they have moderate thermal conductivity, offer efficient heat transfer and high latent heat storage capacity. Thermoelectric materials, on the other hand, have moderate thermal conductivity but are expensive and less available. Design techniques such as microfluidic channels and Nanofluids offer high heat transfer coefficients and efficiency but can be costly and limited in scalability. The use of composite and porous materials can increase thermal conductivity and surface area (Stempihar et al., 2012), respectively, but may be limited by their availability and fragility (Atinafu et al., 2020).
5.1 Cost of installation and maintenance
Associated with maintaining a heat harvesting system, regular maintenance and potential repairs will be necessary to ensure the system runs efficiently over time, which should be budgeted for. However, it is important to consider the potential cost savings in the long run, as investing in a heat harvesting system can significantly reduce energy costs and provide a sustainable and environmentally friendly way of heating and cooling a building. Therefore, when considering the cost and maintenance of a heat harvesting system, it is important to take into account the materials and design techniques used, as well as their thermal conductivity, durability, and availability. Table 5 shows that the cost of materials will vary depending on the specific components chosen for the system. Table 6 presents various design techniques that can improve heat transfer but also come with their own limitations and cost considerations. The initial cost of installation will depend on the size of the system, the materials used, and the complexity of the design, with material and labor costs being the two main cost components. It is also important to factor in the long-term costs of maintaining and potentially repairing the system. Ultimately, considering these factors can help ensure the system is efficient, effective, sustainable, and cost-effective in the long run (S. Al-Humairi et al., 2021; Zabihi and Saafi, 2020a).
PEX pipe is a low-cost material that is readily available, while carbon nanotubes are more expensive and less readily available. The use of microfluidic channels or porous materials may increase the cost of materials but could reduce energy requirements and increase heat transfer efficiency. On the other hand, design techniques like microfluidic channels and nanofluids can have high heat transfer coefficients and increased thermal conductivity, respectively, but also come with their own limitations and cost considerations.
Table 5 compares five different materials based on their thermal conductivity, durability, availability, and cost. The materials included in the comparison are PEX Pipe, Carbon Nanotube, Asphalt Mix, PCM, and Thermoelectric Material. PEX Pipe is ranked as the most suitable material for thermal management applications due to its high thermal conductivity, good durability, high availability, and low cost. Carbon Nanotubes are ranked second, followed by Asphalt Mix, Phase Change Material, and Thermoelectric Material, respectively.
Table 6 compares various design techniques and materials used for heat transfer applications based on their advantages and limitations. The design techniques include microfluidic channels, nanofluids, phase change materials (PCM), composite materials, and porous materials. Each material and design technique is evaluated based on its advantages and limitations. This comparison can assist researchers and professionals in selecting the appropriate material and design technique for their specific heat transfer application based on their requirements. The references cited in the table provide further information on the advantages and limitations of each material and design technique.
5.2 Potential revenue from generated electricity and water treatment
As per the literature, the deployment of cool pavements, water-retentive pavements, and photovoltaic pavements exhibits the capability to mitigate the urban heat island phenomenon and generate economic benefits via energy generation. Santamouris (2013) conducted research that suggests that the utilization of cool pavements can lead to a significant decrease in temperature in urban regions. (Yamagata et al. (2008) conducted a study that found that the utilization of reclaimed wastewater on water-retentive pavement is a viable approach to mitigate the heat island effect (Sangiorgi et al., 2017). As per the findings of Takebayashi and Moriyama (2012) research conducted in 2012, the utilization of water-retentive pavement exhibits the capability to reduce the sensible heat flux up to a maximum of 150 W/m2. Xie and Wang, 2021a conducted a study that found that the utilization of photovoltaic pavement has the capacity to decrease surface temperature by 3°C–5°C in the summer season and generate a reduction in heat output of 11%–12% across various climate conditions. The papers exhibit a deficiency in providing specific information pertaining to the prospective earnings that may arise from the electricity and water treatment procedures.
5.3 Health impact via enhancing air quality
The preservation of clean and healthy air is of utmost importance, especially in metropolitan regions where elevated temperatures can intensify the levels of pollution. The attainment of this goal can be facilitated by employing green spaces, such as parks and trees, to assimilate pollutants and provide shade and cooling, which can be an efficacious strategy (Zulian et al., 2021). The empirical research suggests that the implementation of this intervention can significantly improve the quality of air and reduce the likelihood of respiratory illnesses resulting from exposure to contaminated air. An alternative approach to improving air quality entails the utilization of reflective surfaces, such as roofs and roads that reflect solar radiation rather than absorbing it. The execution of this measure has the potential to alleviate the urban heat island phenomenon and improve air quality, ultimately decreasing the probability of respiratory and cardiovascular illnesses. The deployment of heat recovery systems has the potential to alleviate energy consumption in edifices, consequently leading to a decrease in power plant emissions (Piracha and Chaudhary, 2022). This is especially significant in metropolitan regions where a variety of edifices necessitate significant quantities of energy. The incorporation of heat recovery mechanisms has the potential to enhance air quality and promote its safety for inhalation. As per the literature, mitigating the UHI effect has the potential to result in both positive and negative outcomes for air quality and human wellbeing. Henao et al. (2020) conducted a study that suggests that the execution of UHI alleviation strategies could potentially result in adverse effects on air quality within urban valleys. Harlan and Ruddell (2011a) argue that the incorporation of both mitigation and adaptation strategies in urban climate risk management plans has the potential to generate health co-benefits. This can be achieved by reducing emissions and decreasing temperatures through modifications in the built environment. Shahmohamadi et al. (2011) conducted research that suggests the utilization of appropriate transportation, landscape, and building materials can effectively alleviate the negative impacts of UHI on human health. Dave et al. (2022) study reveals a noteworthy and affirmative association between UHI and the levels of particulate matter. According to his proposal, the adoption of urban greening and white roofs could potentially mitigate the negative impacts of UHI on air quality. The literature suggests that appropriately mitigating the UHI phenomenon can result in positive impacts on air quality and human health (Vujovic et al., 2021), contingent upon the implementation being tailored to the specific regional context.
6 Critical discussion
6.1 Summary of the key findings from the literature review
As the challenges posed by UHI effects become increasingly prominent, the literature reveals a burgeoning interest in Heat Recovery from Roads (HRR) as a promising avenue for mitigation and energy optimization. The literature review offers valuable insights into the potential advantages of HRR technology in addressing the UHI phenomenon in urban settings (Debbage and Shepherd, 2015). The utilization of waste heat generated by traffic and its conversion into useful energy through the implementation of HRR has the potential to mitigate both the UHI effects and greenhouse gas emissions (Henao et al., 2020). This paper elucidates the prospective advantages of HRR, including the capacity to diminish air temperature by a maximum of 5°C and surface temperature by up to 15°C. Additionally, HRR has the potential to facilitate renewable energy production, mitigate greenhouse gas emissions, and diminish reliance on non-renewable energy sources. The answer to the first research question can be concluded that the idea of harnessing heat from roads as a means of mitigating UHI effects represents a nascent field of inquiry, and the present state of scholarship remains in its preliminary phases. Nevertheless, there exist encouraging discoveries so far. Research findings indicate that the implementation of heat recovery systems on roads can result in a decrease in the surface temperature of roads. This reduction in temperature can subsequently lead to a decrease in the overall air temperature in urban regions. The implementation of this measure can potentially alleviate the impacts of UHI effects, thereby enhancing the livability of urban areas, curbing the energy demand for cooling, and enhancing the quality of ambient air (Nakayama and Fujita, 2010a; Wang J. et al., 2018).
Despite progress in implementing HRR, there remain a number of obstacles to its widespread adoption, including technical hurdles, concerns over cost-effectiveness, and questions surrounding public acceptance. This paper conducts a comprehensive review of the current literature on the effects of High-Reflectivity Roofing on UHI, encompassing empirical studies, modeling techniques, and technological advancements. The study identifies areas where research is lacking and offers suggestions for future research and development in the field of HRR as a means of mitigating UHI effects. Prior research has demonstrated that Heat Recovery and Recycling technology, encompassing thermoelectric generators, heat exchangers, and phase-change materials, can be effectively employed to recuperate heat for a range of applications, such as domestic hot water, electricity generation, and the mitigation of energy consumption in streetlights and traffic signals. According to (Zhu et al., 2019), asphalt pavements have the ability to capture as much as 50% of the solar energy that is incident upon them, thereby rendering them a viable and economical option for heat recovery. An innovative approach by Kalnæs and Jelle (2015) utilizes phase-change materials integrated within the asphalt pavement to accumulate thermal energy, which can be subsequently discharged during the night to provide warmth to buildings. This method was found to effectively mitigate the UHI phenomenon, resulting in a reduction of up to 2.5°C during the daytime and 5.5°C during the nighttime. The study conducted by Hasebe et al. (2006) examined the efficacy of thermoelectric generators integrated into roads for the purposes of energy harvesting and UHI mitigation. The results of the investigation indicated that this technology has the potential to generate electricity while simultaneously decreasing the energy consumption of streetlights and traffic signals (Wang H. et al., 2018). The paper’s conclusion posits that the utilization of HRR technology holds promise in addressing the UHI phenomenon and promoting urban development that is sustainable. Additional investigation is required to tackle the obstacles and restrictions of these technologies and investigate their prospective implementations in diverse urban settings. According to Dogeanu et al. (2022a), there exists a temperature disparity of up to 10°C between urban and rural regions, which can have unfavorable consequences on human health and overall welfare. These consequences include heat-related ailments and amplified energy usage for cooling purposes.
6.2 Strengths and limitations of the studies
There is a need for additional research in the field of mitigating urban heat islands because a review of the relevant literature reveals that while the implementation of advanced design techniques can improve heat transfer efficiency, there is no technique that stands out in terms of its ability to effectively mitigate the effects of urban heat islands. Carbon-based 1-dimensional nanomaterials, such as carbon nanotubes, exhibit exceptional thermal conductivity and are frequently utilized to enhance the thermal conductivity of polymer composites. Additionally, nanotubes demonstrate a thermoelectric performance that is comparable to other materials in the field (Badenhorst, 2019; Han and Fina, 2011; Zhang et al., 2006) discovered that the implementation of phase change material (PCM) and expanded graphite in composite building materials results in a high thermal conductivity and significant heat storage capacity, rendering them a viable option for thermal storage applications.
The literature review indicates that the implementation of advanced design techniques can enhance heat transfer efficiency with no definitive technique that delivers in terms of mitigating the effects of urban heat islands. This leads to the conclusion that further research is required in this area. The potential of carbon nanotubes as a material to enhance heat transfer efficiency has been identified. Nonetheless, additional investigation is required to assess and contrast the various design methodologies for mitigating UHI effects. Although these techniques have demonstrated efficacy, there is a lack of consensus regarding the optimal design technique. The papers under review do not provide a direct comparison of the relative benefits and drawbacks of these methodologies; here, we tried to compare different materials based on different factors to be used to harness heat from roads.
The utilization of waste heat from transportation can potentially mitigate UHI effects and decrease greenhouse gas emissions through the implementation of a technology known as the Heat Recovery and Recycling (HRR) system. According to the report, the implementation of HRR has the potential to decrease air and surface temperatures by 5°C and 15°C, produce renewable energy, diminish greenhouse gas emissions, and decrease reliance on non-renewable energy sources. The deployment of HRR (Highly Automated Road Vehicles) presents a number of challenges, including technological obstacles, considerations of cost-effectiveness, and the need to secure public acceptance. The present report conducts an analysis of empirical studies, modeling methodologies, and technical advancements pertaining to the impacts of Heat-Related Mortality (HRR) and UHI.
Previous studies have indicated that HRR technology, including thermoelectric generators, heat exchangers, and phase-change materials, can be utilized to recuperate heat for various purposes, such as residential hot water, electricity production, and energy savings for streetlights and traffic signals. According to Luca & Mrawira, (2005), it has been shown that asphalt pavements have the potential to capture as much as 50% of solar energy, thereby rendering heat recovery a viable and economically feasible option (Nasir et al., 2020). In their study, Jia et al. (2023) proposed an innovative approach that involves the utilization of phase-change materials integrated within asphalt pavement to store thermal energy. The stored energy is subsequently released during the night to provide warmth to buildings, thereby mitigating the UHI effect (Nakayama and Fujita, 2010a; Almeida and Picado-Santos, 2022). The results of the study by Yuan et al. (2023) indicate that this technology can reduce the UHI effect by 2.5°C during the day and 5.5°C at night. The incorporation of thermoelectric generators into roads can serve the dual purpose of energy collection and mitigation of UHI effects. This approach has the potential to generate power while also reducing the energy consumption of streetlights and traffic signals (Ando Junior et al., 2018; Tahami et al., 2019). Utilization of HRR technology has the potential to mitigate the effects of UHI and facilitate the advancement of sustainable urban development (Xu et al., 2021). Further research is required to investigate the issues and applications of these technologies in diverse urban settings. The temperature variance between urban and rural areas can reach up to 10°C, leading to elevated energy consumption for cooling and heat-related ailments (Wang and Xue, 2012). In recent studies, a variety of materials and techniques have been explored for their potential to enhance heat transfer efficiency. This includes innovations like microfluidic channels, Nanofluids, phase change materials, composite materials, and porous materials (Zhang et al., 2006; Stempihar et al., 2012; Delavar and Azimi, 2013a; Davies et al., 2014; Atinafu et al., 2020).
The literature suggests that the implementation of advanced design techniques can enhance the effectiveness of heat transfer; however, it is noteworthy that no solitary approach can effectively alleviate the consequences of the urban heat island phenomenon. The potential enhancement of heat transfer efficiency through the utilization of carbon nanotubes warrants additional investigation. However, a comprehensive evaluation of the advantages and disadvantages of urban heat island mitigation design strategies is necessary. Although design techniques have demonstrated effectiveness, there remains a lack of agreement regarding the optimal approach. The evaluated literature does not provide a comparative analysis of the advantages and disadvantages of these methodologies.
6.3 Comparison of the different approaches and systems in heat recovery from roads
As the urgency to harness waste heat from urban roadways intensifies, a multitude of heat recovery systems and methodologies have emerged, each with its unique strengths and limitations. Several systems have been devised, such as thermoelectric generators (TEGs), heat exchangers, and phase change materials (PCMs) (Marshall et al., 2017; Hajatzadeh Pordanjani et al., 2019). Thermoelectric generators (TEGs) utilize thermal gradients to produce electrical energy and are characterized by their dependability and resilience. However, their efficacy is limited, and their production costs are high. Heat exchangers are utilized to extract heat from roads by means of fluids and subsequently transfer it to a heat sink. They are characterized by their affordability and ease of installation, however, their efficacy is restricted, and they are susceptible to fouling. Phase Change Materials (PCMs) are capable of storing thermal energy by undergoing a phase transition (Kalnæs and Jelle, 2015). Despite their compact and efficient nature, they exhibit low thermal conductivity and may not be able to rapidly absorb heat. The selection of a particular methodology is contingent upon various factors, including but not limited to financial considerations, efficacy, and alignment with pre-existing infrastructure. Further investigation is required to enhance the efficacy of heat recovery systems in addressing the impacts of the urban heat island phenomenon.
The primary utilization of the heat recuperated from roadways encompasses the provision of thermal energy for space conditioning and temperature regulation, domestic sanitary hot water, and the generation of electrical power. The advantages and drawbacks of these applications in terms of economy, environment, and society are contingent upon the particular application and its circumstances. Possible advantages encompass decreased energy expenditures, diminished emissions of greenhouse gases, and enhanced air quality. Nevertheless, there are certain challenges that need to be considered, such as the significant initial expenses, the upkeep and management of the system, and the probable effects on road safety and maintenance (Henao et al., 2020; Dave et al., 2022).
6.4 Interpretation of the results
The results indicate that the implementation of advanced design techniques can effectively enhance heat transfer efficiency and alleviate the adverse impacts of the urban heat island phenomenon. Nonetheless, it is noteworthy that no singular methodology emerges as the preeminent means of achieving optimal results. Consequently, additional investigation is warranted to evaluate and contrast the respective benefits and drawbacks of various strategies. Carbon nanotubes have shown potential as a material that can enhance the efficiency of heat transfer. Further investigation is necessary to comprehensively comprehend the prospective advantages and constraints of employing them in the alleviation of urban heat islands.
PEX pipes are a favored option for heat recovery systems due to their high thermal conductivity, durability, and flexibility. Although possessing high thermal conductivity, carbon nanotubes are associated with significant production costs. The thermal properties of asphalt mixes can be improved through modification; however, their thermal conductivity remains moderate. Phase change materials (PCMs) possess the capacity to accumulate substantial quantities of thermal energy; however, their acquisition cost is high. Thermoelectric materials possess the advantageous characteristic of producing electrical energy from residual heat; however, their accessibility is not yet ubiquitous. One potential strategy for managing thermal energy in urban environments involves the integration of phase change materials (PCMs) into infrastructure. This approach would enable the storage of surplus heat energy during daylight hours, which could then be gradually released during the night to provide space heating. The task at hand may encompass the creation of novel materials and technologies aimed at integrating phase change materials (PCMs) into various urban infrastructure components such as building materials and pavement. The research aims to examine the environmental and social implications of various techniques for enhancing heat transfer. The task at hand may encompass the evaluation of environmental impacts throughout the life cycle of various materials and technologies, alongside the examination of potential social and economic implications of distinct mitigation approaches.
It is imperative to acknowledge that this ranking is not definitive and must be evaluated in the context of particular applications and prerequisites. In instances where cost is the primary consideration, asphalt mixes may present a more feasible alternative. On the contrary, in situations where high thermal conductivity holds paramount importance, carbon nanotubes might emerge as the most suitable option. The choice of materials for heat recovery systems is contingent upon a multitude of factors, and a comprehensive evaluation of the characteristics of each material is imperative in order to ascertain the optimal selection for a given application.
A concise overview of the benefits and drawbacks associated with different design methodologies aimed at alleviating the impacts of urban heat island phenomena is presented in Table 2. As per the tabulated data, microfluidic channels exhibit a notable heat transfer coefficient and proficient heat transfer capabilities (Davies et al., 2014). However, their cost is high, and their scalability is limited. Nanofluids exhibit enhanced thermal conductivity and improved heat transfer characteristics, albeit with potential drawbacks such as pipe blockage and long-term stability concerns (Hajatzadeh Pordanjani et al., 2019). Phase change materials exhibit a notable latent heat storage capacity and effective heat transfer, albeit with a concomitant reduction in thermal conductivity and heat transfer rates (Atinafu et al., 2020). Composite materials exhibit superior thermal conductivity and efficient heat transfer characteristics; however, their availability is restricted, and the production cost is relatively high. Porous materials exhibit low thermal conductivity, high porosity, and augmented surface area, albeit they are susceptible to fragility and possess diminished mechanical strength (Stempihar et al., 2012). The tabular representation does not offer a direct comparison of the techniques; however, it presents a valuable overview of their respective benefits and drawbacks. Additional research is required to compare the relative effectiveness and limitations of these methods in addressing urban heat island impacts and to devise novel approaches that are more efficacious and streamlined.
The limitations of this work can be listed as follows:
• The examined research does not directly compare the pros and downsides of each design technique; therefore, there is no consensus on which is best.
• Some research’ empirical evidence may not apply to all contexts and applications because they were done under specified conditions and may not reflect real-world conditions.
• Some studies focus on specific materials or design processes; therefore, the results may not apply to others.
• More research is needed to analyze the pros and cons of urban heat island mitigation design methods and optimize them for efficiency and environmental benefit.
This ranking is relative to certain applications and needs. For instance, asphalt mixes may be cheaper. Carbon nanotubes are better for high heat conductivity. A thorough evaluation of each material’s qualities is needed to choose the appropriate material for a heat recovery system. The absence of research on the enduring efficacy and resilience of these methodologies, particularly in authentic scenarios, is a notable concern. Further investigation is required to ascertain the robustness of these materials and design methodologies, as well as their viability for enduring integration within urban settings.
Further investigation is required to assess the environmental ramifications of these design methodologies, specifically their capacity to mitigate greenhouse gas emissions and other harmful substances. Subsequent research endeavors ought to assess the economic viability of said methodologies and their capacity for broad implementation within metropolitan regions. Additional investigation is required to tackle the aforementioned research deficiencies and furnish more substantial substantiation regarding the efficacy, longevity, and ecological repercussions of diverse design methodologies aimed at enhancing heat transfer efficiency in metropolitan settings.
6.5 Research gaps and future directions
The evaluated papers provide useful insights into the effectiveness of various design strategies for improving heat transfer efficiency in urban environments, but several research gaps remain:
• Most investigations are laboratory trials or simulations, and there are few field studies to evaluate these methodologies in urban areas. These methods need more investigation to determine their efficacy and suitability for urban environments.
• Comparative studies are needed to assess the pros and cons of urban heat island reduction design methods. The examined studies provide some insights into the usefulness of different strategies, but further research is needed to compare and assess them.
• Lack of long-term performance and durability tests of these approaches, especially in real-world settings. These materials and design methods must be tested for durability and long-term use in urban contexts.
• These design methods may reduce greenhouse gas emissions and other pollutants, but more research is needed. Future studies should assess these methods’ economic viability and urban adoption potential.
• More studies are needed to fill these gaps and demonstrate the efficacy, durability, and environmental impact of various urban heat transfer efficiency design strategies.
Some future directions based on the research gaps can listed as follows:
• Comparing urban heat island mitigation design methods to find pros and cons. This could involve testing and assessing different materials, materials combinations, design techniques, and technologies.
• Creating and testing urban-specific materials and procedures. This could involve studying innovative composites or nanomaterials or establishing multi-technique design methodologies.
• Using phase change materials (PCMs) in urban infrastructure to store daytime heat and release it at night for space heating. New materials and technologies for embedding PCMs into building materials, pavement, and other urban infrastructure may be needed.
• Assessing heat transfer enhancement strategies’ environmental and social implications. This could involve examining the life cycle environmental implications of different materials and technologies and the potential social and economic impacts of mitigating methods.
• Promoting urban heat transfer augmentation through laws and regulations. This could involve requiring certain materials or technology in building codes or incentivizing property owners to invest in heat transfer enhancement technologies.
• This topic has many potential study pathways, and continuing research and innovation will be crucial to tackling urban heat islands and encouraging sustainable urban development.
7 Conclusions and outlook
The study reviewed the use of heat recovery from roads (HRR) technology to mitigate UHI effects. Various design methodologies, such as microfluidic channels, Nanofluids, phase change materials, composite materials, and porous materials, were discussed. Carbon nanotubes were identified as a potential material to enhance heat transfer efficiency. Further research is needed to evaluate the practical effectiveness, compare design techniques, and establish long-term performance, durability, viability, and environmental impacts of HRR technology in urban settings.
7.1 Summary of the main findings
The present study is a review paper that examines the current status of heat recovery from roads (HRR) technology as a means of mitigating the impacts of the UHI phenomenon. The article delineates a range of sophisticated design methodologies, such as microfluidic channels, Nanofluids, phase change materials, composite materials, and porous materials, that have the potential to enhance the efficacy of heat transfer. The potential of carbon nanotubes as a material to enhance heat transfer efficiency is a subject of interest. However, additional investigation is required to assess and contrast the various design approaches for mitigating UHI. Drawing from the extant literature, a viable amalgamation of materials and design methodologies that may yield favorable heat transfer outcomes and environmentally beneficial consequences involves the integration of microfluidic channels within asphalt mixtures. The amalgamation of these substances has the potential to augment the thermal conductivity of the system and curtail energy demands, thereby resulting in favorable ecological consequences.
The proposed model plan seeks to leverage solar energy and offer eco-friendly energy solutions via a pavement-based infrastructure. The process entails the assimilation of solar radiation by the pavement’s exterior, the conduction of thermal energy to a system of pipes or tubes that are integrated into the pavement’s composition, and the utilization of a heat transfer medium and an operational medium to produce electrical power. The optimization of the proposed model plan could be enhanced through the integration of phase change materials (PCMs) and the implementation of monitoring and optimization strategies aimed at improving energy efficiency and minimizing environmental impact.
The field of road-based heat recovery systems presents several knowledge gaps and research needs. These include the need for a more comprehensive understanding of the long-term performance and durability of such systems, as well as the development of improved models and simulations to optimize their design and performance. Additionally, there is a need to better understand the potential impacts of these systems on road maintenance and safety, as well as the social and economic factors that may influence their adoption and scalability. Prospective avenues for research and advancement in this domain may encompass the creation of novel materials and technologies to enhance the efficacy of the system, investigating fresh utilities for recuperated heat, and formulating tactics to promote extensive implementation of these systems in metropolitan regions.
The strength and limitation of the current heat recovery studies are summaries in Table 7.
7.2 Implications for practical applications
The significance of the practical applications of the reviewed studies is noteworthy. The phenomenon of urban heat islands has emerged as a significant challenge in urban settings. A comprehensive review of relevant studies has identified various techniques and strategies that hold promise in addressing the adverse effects of urban heat islands on human wellbeing, energy usage, and ecological balance. The utilization of sophisticated materials and design methodologies, such as Nanofluids, phase change materials, and porous materials, can considerably enhance the efficacy of heat transfer in building materials and pavements. This, in turn, can lead to a reduction in the energy consumption required for cooling and heating buildings and can help alleviate the urban heat island phenomenon.
The pragmatic ramifications of these methodologies surpass the realm of energy efficiency and ecological advantages. The implementation of energy-saving measures and optimization of thermal conditions in buildings and urban areas can potentially ameliorate the wellbeing of city dwellers and mitigate the incidence of heat-related ailments. The implementation of advanced design techniques aimed at enhancing heat transfer efficiency in urban settings can yield favorable environmental outcomes that extend beyond the amelioration of the urban heat island phenomenon. The utilization of porous materials and phase change materials has the potential to diminish energy consumption and greenhouse gas emissions linked with the cooling and heating of buildings. This can aid in decreasing the carbon footprint of urban regions and fostering sustainable urban development. Nevertheless, additional investigation is required to assess the ecological ramifications of diverse materials and design methodologies, their capacity to diminish other contaminants, their comprehensive environmental impacts throughout their life cycle, and their economic viability and potential for extensive implementation in metropolitan regions. Moreover, the possible economic advantages of these methodologies are noteworthy. Through the implementation of energy conservation measures and enhancements to urban infrastructure efficacy, these methodologies have the potential to curtail the aggregate expenses associated with the establishment and upkeep of urban infrastructure. Additionally, they can foster novel prospects for innovation and economic advancement.
7.3 Recommendations for future research
The key recommendations are summarized in Table 8. Which are further elaborated in this section.
Drawing from the identified research gaps and potential avenues for future inquiry in the reviewed literature, It is recommended to increase the number of field studies conducted. The utilization of laboratory experiments and simulations is beneficial in assessing the efficiency of various design techniques. However, there exists a necessity for additional field studies to evaluate the practical effectiveness of these techniques in authentic urban settings. Field studies have the potential to yield more comprehensive and reliable data regarding the efficacy, longevity, and ecological ramifications of diverse design methodologies aimed at enhancing heat transfer efficiency within urban settings.
The comparative analysis of various design techniques for mitigating urban heat island effects is crucial to ascertain their respective merits and demerits. Further research is required to establish a comprehensive understanding of these techniques. Prospective research endeavors ought to undertake a comparative and evaluative analysis of diverse methodologies, encompassing the examination and assessment of various materials and their amalgamations alongside distinct design approaches and technologies.
Further investigation is required to ascertain the long-term performance and durability of the aforementioned materials and design techniques, as well as their viability for sustained use in urban settings. Subsequent research endeavors ought to examine the enduring efficacy and robustness of said methodologies, particularly in authentic settings.
The evaluation of environmental impacts is crucial. Therefore, it is recommended that future studies undertake an assessment of the environmental impact of various heat transfer enhancement methods. This should include an analysis of the life cycle environmental impacts of different materials and technologies. This will aid in the identification of the most sustainable design methodologies and materials.
To enhance the suitability of materials and design techniques for urban settings, it is recommended that researchers delve into novel materials and design strategies that are tailored to such environments. This could involve the utilization of advanced composites, nanomaterials, and innovative design approaches that incorporate multiple techniques. This endeavor aims to identify novel and inventive approaches to enhance heat transfer efficiency within urban localities.
It is imperative to formulate and execute policies and regulations that incentivize the uptake of heat transfer augmentation techniques within urban locales. One potential solution is to implement building codes and standards mandating the utilization of specific materials or technologies or offering incentives to encourage property owners to adopt heat transfer enhancement technologies. In order to tackle the escalating issue of urban heat islands and foster sustainable urban development, it is imperative to prioritize ongoing research and innovation.
Author contributions
MA: Formal Analysis, Investigation, Methodology, Writing–original draft. YB: Conceptualization, Supervision, Writing–review and editing. TA-A: Conceptualization, Supervision, Writing–review and editing. AA: Project administration, Supervision, Writing–review and editing.
Funding
The author(s) declare financial support was received for the research, authorship, and/or publication of this article. This research was supported by a scholarship (210009366) from Hamad Bin Khalifa University (HBKU), a member of Qatar Foundation (QF), and Lean Construction Institute—Qatar (LCI-Q). Any opinions, findings, conclusions, or recommendations expressed in this material are those of the author(s) and do not necessarily reflect the views of HBKU or QF. The use of AI tool-ChatGPT to summarize and to improve the language and readability of the paper is acknowledged.
Conflict of interest
The authors declare that the research was conducted in the absence of any commercial or financial relationships that could be construed as a potential conflict of interest.
The author(s) declared that they were an editorial board member of Frontiers, at the time of submission. This had no impact on the peer review process and the final decision.
Publisher’s note
All claims expressed in this article are solely those of the authors and do not necessarily represent those of their affiliated organizations, or those of the publisher, the editors and the reviewers. Any product that may be evaluated in this article, or claim that may be made by its manufacturer, is not guaranteed or endorsed by the publisher.
References
Abotaleb, A., Almasri, D., Elrayyah, A., Al-Kuwari, M., and Amhamed, A. (2022). Sustainable energy harvesting system for roads in desert climate. Adv. Sci. Technol. Innovation, 369–379. doi:10.1007/978-3-030-76081-6_45
Al-Humairi, S., Alias, A., Haron, N., Hassim, S., and Mohd Jakarni, F. (2021). Sustainable pavement: a review on the usage of pavement as a mitigation strategy for UHI. IOP Conf. Ser. Mater. Sci. Eng. 1075 (1), 012010. doi:10.1088/1757-899X/1075/1/012010
Almeida, A., and Picado-Santos, L. (2022). Asphalt road pavements to address climate change challenges—an overview. Appl. Sci. Switz. 12 (24), 12515. doi:10.3390/APP122412515
Ando Junior, O. H., Maran, A. L. O., and Henao, N. C. (2018). A review of the development and applications of thermoelectric microgenerators for energy harvesting. Renew. Sustain. Energy Rev. 91, 376–393. doi:10.1016/j.rser.2018.03.052
Aneesa, S., and Reshma, J. K. (2022). Various causes of urban heat islands, effects and their mitigation measures for urban ecology – a review. Ecol. Environ. Conservation, 1489–1493. doi:10.53550/EEC.2022.v28i03.055
Atinafu, D. G., Ok, Y. S., Kua, H. W., and Kim, S. (2020). Thermal properties of composite organic phase change materials (PCMs): a critical review on their engineering chemistry. Appl. Therm. Eng. 181, 115960. doi:10.1016/j.applthermaleng.2020.115960
Badenhorst, H. (2019). A review of the application of carbon materials in solar thermal energy storage. Sol. Energy 192, 35–68. doi:10.1016/j.solener.2018.01.062
Balany, F., Ng, A. W. M., Muttil, N., Muthukumaran, S., and Wong, M. S. (2020). Green infrastructure as an urban heat island mitigation strategy—a review. WaterSwitzerl. 12 (12), 3577. doi:10.3390/W12123577
Boyd, D., Pathak, M., van Diemen, R., and Skea, J. (2022). Mitigation co-benefits of climate change adaptation: a case-study analysis of eight cities. Sustain. Cities Soc, 77. doi:10.1016/j.scs.2021.103563
Cheela, V. R. S., John, M., Biswas, W., and Sarker, P. (2021a). Combating urban heat island effect—a review of reflective pavements and tree shading strategies. Buildings 11 (3), 93. doi:10.3390/buildings11030093
Cheng, C., Dai, Y., Yu, J., Liu, C., Wang, S., Feng, S. P., et al. (2021). Review of liquid-based systems to recover low-grade waste heat for electrical energy generation. Energy and Fuels 35 (1), 161–175. doi:10.1021/acs.energyfuels.0c03733
Datta, U., Dessouky, S., and Papagiannakis, A. T. (2017a). Harvesting thermoelectric energy from asphalt pavements. Transp. Res. Rec. J. Transp. Res. Board 2628 (1), 12–22. doi:10.3141/2628-02
Dave, N. M., Vasani, R. P., and Chhasiya, P. (2022). Modelling the impact of urban heat island mitigation strategies on urban air quality. Curr. World Environ. 17 (2), 393–409. doi:10.12944/CWE.17.2.11
Davies, E., Christodoulides, P., Florides, G., and Kalli, K. (2014). Microfluidic flows and heat transfer and their influence on optical modes in microstructure fibers. Materials 7 (11), 7566–7582. doi:10.3390/MA7117566
Debbage, N., and Shepherd, J. M. (2015). The urban heat island effect and city contiguity. Comput. Environ. Urban Syst. 54, 181–194. doi:10.1016/j.compenvurbsys.2015.08.002
Delavar, M. A., and Azimi, M. (2013a). I using porous material for heat transfer enhancement in heat exchangers: review. J. Eng. Sci. Technol. Rev. 6 (1), 14–16. doi:10.25103/jestr.061.03
Dogeanu, A., Tacutu, L., Iatan, E., Nicolae, A.-M., and Lungu, C. I. (2022a). Asphalt heat recovery application for sustainable green energy. Appl. Sci. 12 (3), 1196. doi:10.3390/app12031196
Esteves, R. B., and Lazard, M. (2020). Thermoelectric modules applied in buildings. Tecnica Italiana-Italian J. Eng. Sci. 64 (2–4), 312–316. doi:10.18280/ti-ijes.642-428
Golden, J. S., and Kaloush, K. E. (2006a). Mesoscale and microscale evaluation of surface pavement impacts on the urban heat island effects. Int. J. Pavement Eng. 7 (1), 37–52. doi:10.1080/10298430500505325
Gude, V. G. (2018). Geothermal source for water desalination—challenges and opportunities. Renew. Energy Powered Desalination Handb. Appl. Thermodyn., 141–176. doi:10.1016/B978-0-12-815244-7.00004-0
Gui, J. G., Carlson, J., Phelan, P. E., Kaloush, K. E., and Golden, J. S. (2007). Impact of pavement thickness on surface diurnal temperatures. J. Green Build. 2 (2), 121–130. doi:10.3992/JGB.2.2.121
Hajatzadeh Pordanjani, A., Aghakhani, S., Afrand, M., Mahmoudi, B., Mahian, O., and Wongwises, S. (2019). An updated review on application of nanofluids in heat exchangers for saving energy. Energy Convers. Manag. 198, 111886. doi:10.1016/j.enconman.2019.111886
Han, Z., and Fina, A. (2011). Thermal conductivity of carbon nanotubes and their polymer nanocomposites: a review. Prog. Polym. Sci. 36 (7), 914–944. doi:10.1016/j.progpolymsci.2010.11.004
Harlan, S. L., and Ruddell, D. M. (2011a). Climate change and health in cities: impacts of heat and air pollution and potential co-benefits from mitigation and adaptation. Curr. Opin. Environ. Sustain. 3 (3), 126–134. doi:10.1016/j.cosust.2011.01.001
Hasebe, M., Kamikawa, Y., and Meiarashi, S. (2006). “Thermoelectric generators using solar thermal energy in heated road pavement,” in 2006 25th International Conference on Thermoelectrics, 697–700. doi:10.1109/ICT.2006.331237
Henao, J. J., Rendón, A. M., and Salazar, J. F. (2020). Trade-off between urban heat island mitigation and air quality in urban valleys. Urban Clim. 31, 100542. doi:10.1016/j.uclim.2019.100542
Ibrahim, S. H., Ibrahim, N. I. A., Wahid, J., Goh, N. A., Koesmeri, D. R. A., and Nawi, M. N. M. (2018a). The impact of road pavement on urban heat island (UHI) phenomenon. Int. J. Technol. 9(8), 1597. doi:10.14716/ijtech.v9i8.2755
Jain, R., Brar, T. S., and Kamal, M. A. (2022). Environmental impact and mitigation benefits of urban heat island effect: a systematic review. Archit. Eng. Sci. 3 (4), 230. doi:10.32629/aes.v3i3.1008
Jia, M., Sha, A., Jiang, W., Li, X., and Jiao, W. (2023). Developing a solid–solid phase change heat storage asphalt pavement material and its application as functional filler for cooling asphalt pavement. Energy Build. 285, 112935. doi:10.1016/j.enbuild.2023.112935
Kaggwa, A., and Carson, J. K. (2019). Developments and future insights of using nanofluids for heat transfer enhancements in thermal systems: a review of recent literature. Int. Nano Lett. 9 (4), 277–288. doi:10.1007/s40089-019-00281-x
Kalnæs, S. E., and Jelle, B. P. (2015). Phase change materials and products for building applications: a state-of-the-art review and future research opportunities. Energy Build. 94, 150–176. doi:10.1016/j.enbuild.2015.02.023
Kumar, V., Coleri, E., Obaid, I., Belc, A. L., and Sutherland, A. J. (2022). Selection of durable, environmentally friendly, and cost-effective asphalt mixtures. Materials 15 (14), 4873. doi:10.3390/ma15144873
Kyratsi, T., Angelopoulos, A., and Fildisis, T. (2010a). Thermoelectric materials and applications on the recovery of waste heat energy. AIP Conf. Proc., 700–705. doi:10.1063/1.3322538
Lee, J. S., Kim, J. T., and Lee, M. G. (2014a). Mitigation of urban heat island effect and greenroofs. Indoor Built Environ. 23 (1), 62–69. doi:10.1177/1420326X12474483
Luca, J., and Mrawira, D. (2005). New measurement of thermal properties of superpave asphalt concrete. J. Mater. Civ. Eng. 17 (1), 72–79. doi:10.1061/(asce)0899-1561(2005)17:1(72)
Mallick, R. B., Chen, B. L., and Bhowmick, S. (2009a). Harvesting energy from asphalt pavements and reducing the heat island effect. Int. J. Sustain. Eng. 2 (3), 214–228. doi:10.1080/19397030903121950
Manteghi, G., and Mostofa, T. M. (2019). Evaporative pavements as an urban heat island (uhi) mitigation strategy: a review.
Marando, F., Heris, M. P., Zulian, G., Udías, A., Mentaschi, L., Chrysoulakis, N., et al. (2022). Urban heat island mitigation by green infrastructure in European Functional Urban Areas. Sustain. Cities Soc. 77, 103564. doi:10.1016/J.SCS.2021.103564
Marshall, S. D., Arayanarakool, R., Balasubramaniam, L., Li, B., Lee, P. S., and Chen, P. C. Y. (2017). “Heat exchanger improvement via curved, angular and wavy microfluidic channels: a comparison of numerical and experimental results”in 2017 16th IEEE Intersociety Conference on Thermal and Thermomechanical Phenomena in Electronic Systems (ITherm), 585–592. doi:10.1109/ITHERM.2017.7992539
Mohajerani, A., Bakaric, J., and Jeffrey-Bailey, T. (2017a). The urban heat island effect, its causes, and mitigation, with reference to the thermal properties of asphalt concrete. J. Environ. Manag. 197, 522–538. doi:10.1016/J.JENVMAN.2017.03.095
Mohajerani, A., Bakaric, J., and Jeffrey-Bailey, T. (2017b). The urban heat island effect, its causes, and mitigation, with reference to the thermal properties of asphalt concrete. J. Environ. Manag. 197, 522–538. doi:10.1016/J.JENVMAN.2017.03.095
Nakayama, T., and Fujita, T. (2010a). Cooling effect of water-holding pavements made of new materials on water and heat budgets in urban areas. Landsc. Urban Plan. 96 (2), 57–67. doi:10.1016/J.LANDURBPLAN.2010.02.003
Nasir, S. D., Xu, W., Vital, B., Pantua, C., Zhou, B., Calautit, J., et al. (2020). Urban road and pavement solar collector system for heat island mitigation: assessing the beneficial impact on outdoor temperature. IOP Conf. Ser. Earth Environ. Sci. 463 (1), 012038. doi:10.1088/1755-1315/463/1/012038
Nuruzzaman, Md. (2015). Urban heat island: causes, effects and mitigation measures - a review. Int. J. Environ. Monit. Analysis 3 (2), 67. doi:10.11648/j.ijema.20150302.15
Nwakaire, C. M., Onn, C. C., Yap, S. P., Yuen, C. W., and Onodagu, P. D. (2020a). Urban Heat Island Studies with emphasis on urban pavements: a review. Sustain. Cities Soc. 63, 102476. doi:10.1016/j.scs.2020.102476
O’Malley, C., Piroozfar, P., Farr, E. R. P., and Pomponi, F. (2015). Urban Heat Island (UHI) mitigating strategies: a case-based comparative analysis. Sustain. Cities Soc. 19, 222–235. doi:10.1016/J.SCS.2015.05.009
Page, M. J., McKenzie, J. E., Bossuyt, P. M., Boutron, I., Hoffmann, T. C., Mulrow, C. D., et al. (2021). The PRISMA 2020 statement: an updated guideline for reporting systematic reviews. BMJ n71, n71. doi:10.1136/bmj.n71
Pallua, I. (2021). The materiality of space heating: heat pumps and heating transitions in Twentieth-century Switzerland. Hist. Technol. 37 (4), 505–526. doi:10.1080/07341512.2022.2033385
Pei, J., Zhou, B., and Lyu, L. (2019). e-Road: the largest energy supply of the future? Appl. Energy 241, 174–183. doi:10.1016/J.APENERGY.2019.03.033
Peluso, P., Persichetti, G., and Moretti, L. (2022a). Effectiveness of road cool pavements, greenery, and canopies to reduce the urban heat island effects. Sustain. Switz. 14 (23), 16027. doi:10.3390/SU142316027
Piao, Z., Mikhailenko, P., Kakar, M. R., Bueno, M., Hellweg, S., and Poulikakos, L. D. (2021). Urban mining for asphalt pavements: a review. J. Clean. Prod. 280, 124916. doi:10.1016/j.jclepro.2020.124916
Piracha, A., and Chaudhary, M. T. (2022). Urban air pollution, urban heat island and human health: a review of the literature. Sustainability 14 (15), 9234. doi:10.3390/su14159234
Pratiwi, S. N. (2018). A review of material cover features for mitigating urban heat island. Int. J. Livable Space 3 (2), 71–80. doi:10.25105/livas.v3i2.3196
Qihao, Y., Xicai, P., Guodong, C., and Yang, B. (2007). Heat transfer process of roadway embankments with different type and width of road surface in permafrost regions. Prog. Nat. Sci. 17 (3), 314–319. doi:10.1080/10020070612331343263
Qin, Y. (2015a). A review on the development of cool pavements to mitigate urban heat island effect. Renew. Sustain. Energy Rev. 52, 445–459. doi:10.1016/j.rser.2015.07.177
Quoilin, S., Declaye, S., Tchanche, B. F., and Lemort, V. (2011). Thermo-economic optimization of waste heat recovery Organic Rankine Cycles. Appl. Therm. Eng. 31 (14–15), 2885–2893. doi:10.1016/j.applthermaleng.2011.05.014
Rizwan, A. M., Dennis, L. Y. C., and Liu, C. (2008a). A review on the generation, determination and mitigation of Urban Heat Island. J. Environ. Sci. 20 (1), 120–128. doi:10.1016/S1001-0742(08)60019-4
Sangiorgi, C., Tataranni, P., Simone, A., Vignali, V., Lantieri, C., and Dondi, G. (2017). A laboratory and filed evaluation of Cold Recycled Mixture for base layer entirely made with Reclaimed Asphalt Pavement. Constr. Build. Mater. 138, 232–239. doi:10.1016/j.conbuildmat.2017.02.004
Santamouris, M. (2013). Using cool pavements as a mitigation strategy to fight urban heat island—a review of the actual developments. Renew. Sustain. Energy Rev. 26, 224–240. doi:10.1016/j.rser.2013.05.047
Shahmohamadi, P., Che-Ani, A. I., Etessam, I., Maulud, K. N. A., and Tawil, N. M. (2011). Healthy environment: the need to mitigate urban heat island effects on human health. Procedia Eng. 20, 61–70. doi:10.1016/j.proeng.2011.11.139
Shaker, R. R., Altman, Y., Deng, C., Vaz, E., and Forsythe, K. W. (2019). Investigating urban heat island through spatial analysis of New York City streetscapes. J. Clean. Prod. 233, 972–992. doi:10.1016/j.jclepro.2019.05.389
Sharma, A., Tyagi, V. V., Chen, C. R., and Buddhi, D. (2009). Review on thermal energy storage with phase change materials and applications. Renew. Sustain. Energy Rev. 13 (2), 318–345. doi:10.1016/j.rser.2007.10.005
Solecki, W. D., Rosenzweig, C., Parshall, L., Pope, G., Clark, M., Cox, J., et al. (2005). Mitigation of the heat island effect in urban New Jersey. Environ. Hazards 6 (1), 39–49. doi:10.1016/j.hazards.2004.12.002
Stempihar, J. J., Pourshams-Manzouri, T., Kaloush, K. E., and Rodezno, M. C. (2012). Porous asphalt pavement temperature effects for urban heat island analysis. Transp. Res. Rec. J. Transp. Res. Board 2293 (1), 123–130. doi:10.3141/2293-15
Tahami, S. A., Gholikhani, M., Nasouri, R., Dessouky, S., and Papagiannakis, A. T. (2019). Developing a new thermoelectric approach for energy harvesting from asphalt pavements. Appl. Energy 238, 786–795. doi:10.1016/j.apenergy.2019.01.152
Takebayashi, H., and Moriyama, M. (2012). Study on surface heat budget of various pavements for urban heat island mitigation. Adv. Mater. Sci. Eng. 2012, 1–11. doi:10.1155/2012/523051
Vargo, J., Stone, B., Habeeb, D., Liu, P., and Russell, A. (2016). The social and spatial distribution of temperature-related health impacts from urban heat island reduction policies. Environ. Sci. Policy 66, 366–374. doi:10.1016/j.envsci.2016.08.012
Vujovic, S., Haddad, B., Karaky, H., Sebaibi, N., and Boutouil, M. (2021). Urban heat island: causes, consequences, and mitigation measures with emphasis on reflective and permeable pavements. CivilEng 2 (2), 459–484. doi:10.3390/CIVILENG2020026
Wang, C. F., Ji, D. B., Yi, Z. Q., and Liu, L. (2010). Analysis of high-permeable concrete pavement heat transfer mechanism. Adv. Mater. Res. 168–170, 859–863. doi:10.4028/www.scientific.net/AMR.168-170.859
Wang, H., Jasim, A., and Chen, X. (2018). Energy harvesting technologies in roadway and bridge for different applications – a comprehensive review. Appl. Energy 212, 1083–1094. doi:10.1016/j.apenergy.2017.12.125
Wang, J., Zhang, Z., Guo, D., Xu, C., and Zhang, K. (2018). Study on cooling effect and pavement performance of thermal-resistant asphalt mixture. Adv. Mater. Sci. Eng. 2018, 1–11. doi:10.1155/2018/6107656
Wang, J. P., and Xue, X. Z. (2012). Discussion of the mitigation on urban heat island effect based on sustainable development. Adv. Mater. Res. 616–618, 1465–1469. doi:10.4028/www.scientific.net/AMR.616-618.1465
Xie, P., and Wang, H. (2021a). Potential benefit of photovoltaic pavement for mitigation of urban heat island effect. Appl. Therm. Eng. 191, 116883. doi:10.1016/j.applthermaleng.2021.116883
Xu, L., Wang, J., Xiao, F., Ei-Badawy, S., and Awed, A. (2021). Potential strategies to mitigate the heat island impacts of highway pavement on megacities with considerations of energy uses. Appl. Energy 281, 116077. doi:10.1016/j.apenergy.2020.116077
Xu, Q., Riffat, S., and Zhang, S. (2019). Review of heat recovery technologies for building applications. Energies 12 (7), 1285. doi:10.3390/en12071285
Yamagata, H., Nasu, M., Yoshizawa, M., Miyamoto, A., and Minamiyama, M. (2008). Heat island mitigation using water retentive pavement sprinkled with reclaimed wastewater. Water Sci. Technol. 57 (5), 763–771. doi:10.2166/wst.2008.187
Yang, J. (2005). “Potential applications of thermoelectric waste heat recovery in the automotive industry,” in ICT 2005. 24th International Conference on Thermoelectrics, 170–174. doi:10.1109/ICT.2005.1519911
Yoshitake, I., Yasumura, N., Syobuzako, M., and Scanlon, A. (2011). Pipe heating system with underground water tank for snow thawing and ice prevention on roads and bridge decks. J. Cold Regions Eng. 25 (2), 71–86. doi:10.1061/(ASCE)CR.1943-5495.0000023
Yuan, D., Jiang, W., Xiao, J., Ling, X., Zhang, Y., and Lu, R. (2023). Experimental study on the temperature-regulating function of road thermoelectric generator system. J. Clean. Prod. 384, 135586. doi:10.1016/j.jclepro.2022.135586
Zabihi, N., and Saafi, M. (2020a). Recent developments in the energy harvesting systems from road infrastructures. Sustainability 12 (17), 6738. doi:10.3390/su12176738
Zhang, Y. P., Lin, K. P., Yang, R., Di, H. F., and Jiang, Y. (2006). Preparation, thermal performance and application of shape-stabilized PCM in energy efficient buildings. Energy Build. 38 (10), 1262–1269. doi:10.1016/j.enbuild.2006.02.009
Zheng, T., Qu, K., Darkwa, J., and Calautit, J. K. (2022a). Evaluating urban heat island mitigation strategies for a subtropical city centre (a case study in Osaka, Japan). Energy 250, 123721. doi:10.1016/J.ENERGY.2022.123721
Zhu, X., Yu, Y., and Li, F. (2019). A review on thermoelectric energy harvesting from asphalt pavement: configuration, performance and future. Constr. Build. Mater. 228, 116818. doi:10.1016/j.conbuildmat.2019.116818
Keywords: road based heat energy, economic fesibility, heat recovery, carbon footprint, urban sustainability, waste heat
Citation: Ali M, Bicer Y, Al-Ansari T and Amhamed AI (2023) A systematic review of heat recovery from roads for mitigating urban heat island effects: current state and future directions. Front. Built Environ. 9:1292913. doi: 10.3389/fbuil.2023.1292913
Received: 13 September 2023; Accepted: 23 November 2023;
Published: 18 December 2023.
Edited by:
Bao-Jie He, Chongqing University, ChinaReviewed by:
Yan Liu, Xi’an University of Architecture and Technology, ChinaLiseane Padilha Thives, Federal University of Santa Catarina, Brazil
Copyright © 2023 Ali, Bicer, Al-Ansari and Amhamed. This is an open-access article distributed under the terms of the Creative Commons Attribution License (CC BY). The use, distribution or reproduction in other forums is permitted, provided the original author(s) and the copyright owner(s) are credited and that the original publication in this journal is cited, in accordance with accepted academic practice. No use, distribution or reproduction is permitted which does not comply with these terms.
*Correspondence: Moiz Ali, bW9tYWxpQGhia3UuZWR1LnFh