- 1Faculty of Agricultural and Food Sciences and Environmental Management, Institute of Water and Environmental Management, University of Debrecen, Debrecen, Hungary
- 2Faculty of Mechanical Engineering and Informatics, Institute of Energy Engineering and Chemical Machinery, University of Miskolc, Miskolc, Hungary
One of the challenges in livestock production is the significant volume of manure generated, which must be appropriately managed to mitigate its environmental impacts. Untreated manure poses a potential hazard to soil, surface water, groundwater, and human and animal health. Based on the life cycle assessment (LCA) method, the research aims to evaluate the ecological load of composted-pelletized poultry litter (CPPL) in maize and winter wheat production. Furthermore, the environmental loads of CPPL applications are compared with those of other N, P, and K fertilizers. The research study utilized the openLCA software with the Agribalyse 3.1 database to calculate eleven impact categories. In the case of maize, only ozone depletion has higher emissions. For winter wheat production, scenarios where the P fertilizer was MAP had lower impacts for NPK combinations. While for the CPPL, fuel was the main contributor to loads, for the NPK fertilizer scenarios, energy use for fertilizer production contributed more. The results can be relevant to the burdens of using different nutrient replacement products and creating diverse feed mixtures. The application of CPPL promises to reduce the burden of crop production and, consequently, feed production. Additionally, it allows for the recovery of manure not useable by the livestock industry.
1 Introduction
One of the objectives of the Green Deal for agriculture (European Union, 2023) is to reduce fertilizer usage and promote the use of organic fertilizers. Although chemical fertilizers provide nutrients to plants quickly and easily, their use can negatively affect soil health. Chemical fertilizers contribute to soil erosion, acidification, soil structure degradation, and loss of organic matter (EEA, 2004). The change in the nitrogen cycle is the most significant environmental problem affecting the soil. Intensive food production has significantly reduced the natural nitrogen content of soils. In contrast, nitrogen from artificial sources has increased (Sainju et al., 2018). Inappropriate fertilization practices can significantly impact surface and groundwater, leading to pollution from phosphates and nitrates (Savci, 2012; Khan et al., 2018; Tamás et al., 2022). Nitrate in groundwater also risks human health, as it can harm health if drinking water is extracted (Ward et al., 2018; Rahman et al., 2021). Regarding air pollution, CO2 and N2O emissions are primarily associated with crop production processes. This is primarily attributed to electricity consumption, fuel usage by agricultural machinery, and land use change (Aguilera et al., 2016; Ahmed et al., 2020). The production of nitrogen fertilizers and their raw materials also emits CO2, N2O, and NOx, as does their use, resulting in NH3 and N2O emissions (Mbonimpa et al., 2014; Nyamadzawo et al., 2014; Dhadli et al., 2016). Reducing and replacing chemical fertilizers is becoming increasingly important from an environmental perspective. The by-products of livestock production, such as manure and other organic materials (e.g., compost, meat, bone, and feather meal, etc.), can play a significant role in replenishing soil resources and can even serve as a suitable alternative to chemical fertilizers (Tamás, 2010; Mézes et al., 2015; He, 2020; Gorliczay et al., 2021). It also makes livestock production a significant source of soil fertility (Moyo and Swanepoel, 2010; Magnusson, 2016). Recently, the livestock sector, particularly broiler chicken production (Chia et al., 2019), has gained increasing importance in the food industry (Kasule et al., 2014; Enahoro et al., 2018; Van Harn et al., 2019). As a result, the issue of effectively utilizing growing quantities of manure has become more pressing. Poultry manure can be used directly as an organic fertilizer. However, it is recommended to treat it before application due to its high nitrogen, phosphorus, moisture, and fibre content. Due to its high nitrogen, phosphorus, moisture, and fiber content, it is recommended to treat it before application. Composting effectively treats and utilizes solid organic wastes (and by-products under aerobic conditions) and various manures (Masters, 1997; Wang and Dalal, 2015). The Hosoya composting plant is a three-phase system consisting of two-phase aerobic fermentation and one-phase final drying (Georgakakis and Krintas, 2000; Hosoya and Co. Ltd, 2020), where the product is CPPL. Considering the impact of composting plants, it is essential to analyse their environmental impacts. LCA is one of the helpful methods for estimating potential environmental burdens and is mainly used for the construction industry (Buyle et al., 2013; Bahramian and Yetilmezsoy, 2020), grinding processes (Kruszelnicka, 2020; Mannheim and Kruszelnicka, 2022; Mannheim and Kruszelnicka, 2023); plastic manufacturing (Civancik-Uslu et al., 2018; Baldowska-Witos et al., 2019; Alhazmi et al., 2021; Mannheim, 2021), and waste management (Brancoli and Bolton, 2019; Alwaeli and Mannheim, 2022; Cano-Londoño et al., 2022; Rimantho et al., 2022; Avató and Mannheim, 2022; Mannheim, 2022). In the last decade, the environmental impacts associated with livestock and crop production have become increasingly significant. Previous research (Kiss et al., 2021) shows that the environmental impact of CPPL (53% broiler manure and litter, 27% manure layer and litter, and 20% chicken and bone meal) production is more favourable than the most used fertilizer combinations. This study aimed to evaluate the environmental impact of CPPL as a potential alternative to chemical N, P and K fertilisers in maize and winter wheat production. Environmental impacts were compared with those of common chemical fertiliser combinations: ammonium nitrate (AN), calcium ammonium nitrate (CAN), urea, triple superphosphate (TSP), monoammonium phosphate (MAP), and potassium chloride (KCl). Possible results may also help identify critical points in the cultivation technology for harvesting 1 ton of maize (Zea mays L.) and winter wheat (Triticum aestivum L.).
2 Materials and methods
2.1 Life cycle assessment and life cycle inventory
The LCA structure and its four main phases are based on the ISO 14040:2006 standard (International Organization for Standardization, 2006a; International Organization for Standardization, 2006b), which include goal and scope, life cycle inventory, life cycle impact assessment, and interpretation of the results (Gabathuler, 2006). LCA was conducted using the openLCA software (OpenLCA Nexus, 2022). The two most essential field crops grown in Europe and Hungary are, maize (Zea mays L.) and winter wheat (Triticum aestivum L.), which were used as the basis for the LCA of crop production, where the material and energy flows necessary for producing one tonne of each crop were determined. The CPPL was supplemented with different N, P, and K fertilizers combinations. The Agribalyse 3.1 French database contained a substantial amount of data for all the necessary analyses (Colomb et al., 2015; Koch and Salou, 2020; Asselin-Balençon et al., 2020; OpenLCA Nexus, 2022). The LCI includes field operations such as tilling, nutrient replenishment, basic tillage, soil smoothing, seedbed preparation, sowing, crop protection, and harvesting. It also encompasses the machinery required for these operations and all inputs like seeds, CPPL, and pesticides. In the provided database, the selected ‘Process’ displays the duration of processes in hours and calculates the material and energy inputs required for the process and the necessary machinery. The process also considers emissions from fuel combustion. The system boundary is “from harvest to harvest”, but it does not consider post-harvest processes such as drying or storage, even though these operations are conducted on the farm. Irrigated production has been considered for maize cultivation since the sample farm and another farm, which also provides fodder crops to the sample farm, cultivate maize under irrigated conditions.
2.2 Life cycle impact assessment method
In Europe, the EcoIndicator, ReCiPe, ILCD, and CML approaches are commonly used as impact assessment methods (Guinée et al., 2002; Gabathuler, 2006; Kabakian et al., 2015; Lamnatou and Chemisana, 2015). This research uses the CML 2001 method, which assumes that emissions with similar effects can be summarized across different media. It also employs an impact-oriented classification of material and energy flows for impact assessments. The impact of emissions and consumption on the environment is illustrated through eleven categories (Gaidajis and Kakanis, 2021; Baldini et al., 2018). The calculated potentials include the abiotic depletion potential for elements (ADPe), abiotic depletion potential for fossil fuels (ADPf), acidification potential (AP), eutrophication potential (EP), global warming potential (GWP), ozone layer depletion potential (ODP), photochemical oxidation potential (POP), freshwater aquatic ecotoxicity potential (FAETP), human toxicity potential (HTP), marine aquatic ecotoxicity potential (MAETP), and terrestrial ecotoxicity potential (TETP). Kiss et al. (2021) describe the impact categories in more detail.
2.3 Interpretation methods
Since CPPL is a complex nutrient supplement containing all the macronutrients in one product, the crop production scenario assumed the combined application of NPK fertilizers. The application rate of CPPL was determined at 1.5 t/ha, as suggested by the manufacturer and researchers (Szabó et al., 2019) (Supplementary Material S1). This amount corresponds to 82.5 kg N/ha, which aligns with the recommendation of Kátai (2011) that 80 kg N/ha is the minimum nitrogen requirement for soils with a low to medium N supply. In addition to the environmental impact of crop production processes, the production of CPPL and chemical fertilizers has also been considered. Based on dividing the difference between the maximum and minimum impact category values into three equal intervals, three categories (low, medium, and high burden) were established. Finally, normalization and weighting methods were used to compare the categories: CML-IA baseline, EU25 + 3, and 2000.
3 Results
This work estimates eleven environmental impacts of the CPPL, N-, P-, and K fertilizers. Table 1 summarizes the calculated LCA values for maize and winter wheat productions. It shows that ozone layer depletion and acidification (in the case of S2) are slightly higher for CPPL than for NPK combinations. However, there were very slight differences in the ODP of maize; it was only 1.9%–1.3% between CPPL and chemical fertilizers. The differences in wheat ODP were slightly more significant, ranging from 6% to 12.5%, between CPPL and NPK combinations. For the AP, differences ranging from 2.6% to 6.8% were observed in wheat cultivation when comparing the use of CPPL with NPK combinations. In the case of ADPe, applying CPPL and NPK5 resulted in the lowest impacts in both scenarios. NPK5 was the only NPK combination that could have a medium environmental load, while the other NPK combinations had a significant ecological impact. Maize production with CPPL has an 11%–14% lower impact, and winter wheat production with CPPL has at least a 30%–40% lower impact. In the case of ADPf, fuel consumption and heavy machinery were the main contributors to emissions. This value is 14%–56% lower when using CPPL. The main contributors to acidification were winter wheat production using CPPL and maize production using NPK1 fertilizer. In the case of maize production, acidification was 40% lower when using CPPL. The value of eutrophication is 2–3 times lower for S2 than for S1. In the EP values concerning NPKs, there are no significant differences. In the case of EP, the two most important contributors were field operations (N2O and CO2 emissions) and fuel consumption. Using CPPL, global warming is almost three times higher for S1 and, on average, 66%–87% lower for the CPPL scenario compared to NPK combinations. In the case of S2, although the production of CPPL itself represents only 1.5% of winter wheat production with CPPL, in production systems where NPK fertilizers were used, this means, on average, 9.7%. For the POPs, combinations 2, 4, and 6 of NPK can have a medium environmental impact, while combinations 1, 3, and 5 can be classified as having a high environmental impact in both cases. POP values are the lowest for CPPL’s application. For toxicity potentials, the emissions were 3%–5% lower for FAETP, 11%–15% lower for MAETP, 2%–3% lower for TETP, and 4%–5% lower for HTP when CPPL was applied. The highest values were observed for the MAETP, followed by the FAETP as the second highest and the TETP as the third most significant impact category. While higher MAETP and FAETP values were more related to CPPL and NPK fertilizer production, the TETP values were linked to cultivation technology. Figure 1 shows the main phases of the LCA with the system boundaries, the detailed LCI data and the results of the environmental impact assessment.
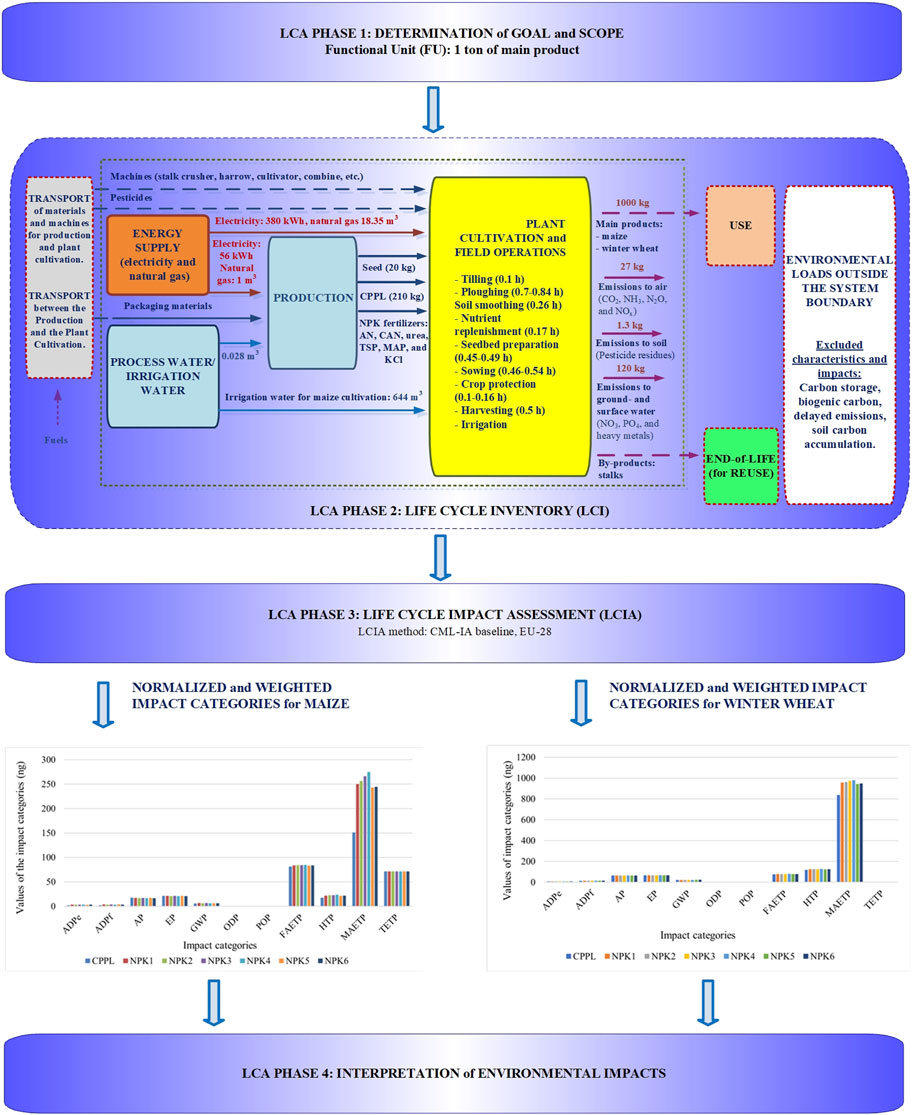
FIGURE 1. The examined phases of the Life Cycle Assessment with the system boundaries, the detailed Life Cycle Inventory data and the results of the environmental impact assessment.
4 Discussion
4.1 Discussion about maize production
According to previous research studies (Holka et al., 2017; Taki et al., 2018), the environmental impacts depend mainly on the heavy machinery used for each field operation and the types of nutrient supplements. According to this research, the ADPe primarily relates to the processes preceding crop production, such as the extraction and production of raw materials. It explains why emissions were higher in those crop cultivation systems due to the production of various fertilizers. In addition, transport also contributes to ADPe, as demonstrated by Holka and his co-authors (Holka et al., 2017) determined during an analysis of maize production on two Polish farms. Their results showed no difference between the two farms, and the estimated value of 0.001 kg Sb-Eq was similar to this study. In the case of AP, they estimated 6.6 and 7.9 kg SO2-Eq. For POP values, fuel consumption and heavy machinery are the primary contributors to emissions, along with the use of pesticides, both in this study and in Holka et al. (2017) research. Some scientific literature (Whitman et al., 2011; Holka et al., 2017) is available on GWP, where the values are highly variable and lower than those measured in the present study. However, most studies have considered non-irrigated conditions. In their studies, Whitman et al. (2011) found values of 320 and 488 kg CO2-Eq/t for maize, respectively. Their research concluded that the primary sources of greenhouse gas emissions were losses in soil organic carbon (40%–61%), followed by NO2 emissions (10%–31%) and finally, field operations, with harvesting processes being the main contributor (14%–22%). Holka and co-authors (Holka et al., 2017) measured 297 and 331 kg CO2-Eq when comparing two maize production systems in Poland. Another study (Holka and Bieńkowski, 2020) compared the CO2-Eq emissions of reduced tillage and no-tillage systems. Their results showed no significant differences between the systems (values ranged from 178 to 190 kg CO2-Eq/t). Jayasundara and colleagues (Jayasundara et al., 2014) measured 243–353 kg CO2-Eq, while Supasri and co-researchers (Supasri et al., 2020) estimated 351 kg CO2-Eq. Comparing irrigated and non-irrigated maize production, Wettstein and his colleagues (Wettstein et al., 2017) found that non-irrigated systems emit 490 kg CO2-Eq. In contrast, the emissions were higher in irrigated systems, ranging between 530 and 800 kg CO2-Eq. Ghasempour and Ahmadi (2018) estimated the ODP at 2.05 × 10−5 kg CFC-11. According to their research, nitrogen fertilizers and pesticides were the primary contributors to ozone depletion. No literature on maize production regarding impact categories expressed in kg 1,4-DB equivalent, such as FAETP, MAETP, TETP, and HTP, is available.
4.2 Discussion about winter wheat production
There is more literature on life cycle assessment for winter wheat than for maize. Williams and his colleagues (Williams et al., 2010) estimated the AP at 3.3 kg SO2-Eq per 1 tonne of wheat, while Holka et al. (Holka et al., 2016) estimated it at 4.6–6.6 kg SO2-Eq. Taki et al. (Taki et al., 2018), comparing irrigated and non-irrigated cropping technologies, noted 8.99 kg SO2-Eq. for the former and 11.9 kg SO2-Eq. for the latter. According to their study, microbial oxidation of fertilizers is the primary acid-forming reaction. According to the results of Holka and Bienkowski (Holka and Bieńkowski, 2020), the AP of conventional, reduced, and no-tillage systems were 2.7, 3.5, and 5.1 kg SO2-Eq., respectively. In the present research, the EP values are 2.9 kg PO4-Eq. These values are close to those estimated by Williams et al. (Williams et al., 2010) and Taki et al. (Taki et al., 2018), who recorded 3.1 kg and 2.2 kg PO4-Eq for irrigated areas, and 3.2 kg PO4-Eq for non-irrigated areas. For EP, regardless of the nutrient amendment, field operations were the main contributors to the leaching (NO3 to groundwater, NH3 to air, PO4 to surface water, N2O and NOx to air). Hoshyar and Grundman (Hoshyar and Grundmann, 2017) also reported that the main parameters influencing EP were field operations, seed production, and nitrogen fertilizer application were the main parameters influencing EP. They found that eutrophication was significantly impacted by NOx and NH3 deposition (Potting et al., 2001). As with maize, most of the literature on winter wheat is based on GWP. Biswas and his co-authors (Biswas et al., 2008) estimated GHG emissions for wheat cultivation to be 308–487 kg CO2-Eq. Based on their research, fertilizer production represents 35% of total emissions, 27% of field operations, and 12% of transport processes. Similar results were recorded by Holka et al. (Holka et al., 2016), with 324–404 kg CO2-Eq per tonne of winter wheat. Taki et al. (Taki et al., 2018) estimated 318 kg CO2-Eq for irrigated areas and 380 kg CO2-Eq for non-irrigated areas.
5 Conclusion
Whether it is the cultivation of maize or winter wheat, the primary environmental impact is caused by field operations (including the use of pesticides), electricity (mainly the release of Cr(VI) into the air and the toxicity due to the release of copper), and fuel consumption (resulting in emissions of CO2, N2O, SO2, CH4, and NOx into the air, primarily contributing to the formation of POP, GWP, ODP, EP, and AP) from both CPPL and NPK fertilizers. There are negligible amounts of CPPL and NPK fertilizers. However, when considering acidification, eutrophication, and global warming, the main contributors to the environmental burden are the environmental impacts caused by cultivation technology. However, for GWP, we observed lower emissions of 11.1%–14% in maize cultivation and 30.1%–33.9% in winter wheat cultivation when nutrient replenishment was managed with CPPL. For the acidification in CPPL wheat production, field operations had the highest environmental impact due to NH3 and NOx emissions, followed by CPPL production and heavy machinery fuel. In the case of NPK1-6 combinations, field operations are the main contributors to acidification, fuel usage, and the extraction and production of raw materials for chemical fertilizer manufacturing. The environmental burden was lower for the toxicity categories when nutrient replenishment was applied using CPPL. Marine aquatic ecotoxicity was the most significant impact on winter wheat production, followed by human toxicity as the second most significant, and terrestrial ecotoxicity as the third most significant. Based on the results, implementing CPPL can reduce the environmental burden associated with meat production. Furthermore, CPPL could be a potential alternative to fertilizers, provided that complex fertilization is considered. Thus, substituting fertilizers also fulfils the ambition of the European Green Deal.
Data availability statement
The raw data supporting the conclusion of this article will be made available by the authors, without undue reservation.
Author contributions
All authors listed have made a substantial, direct, and intellectual contribution to the work and approved it for publication.
Funding
This research was supported by EU grant to Hungary GINOP 2.2.1.-15-2017-00043. The publication was supported and funded by the European Union’s Horizon 2020 “WATERAGRI Water retention and nutrient recycling in soils and steams for improved agricultural production” research and innovation programme under Grant Agreement No. 858375.
Conflict of interest
The authors declare that the research was conducted in the absence of any commercial or financial relationships that could be construed as a potential conflict of interest.
Publisher’s note
All claims expressed in this article are solely those of the authors and do not necessarily represent those of their affiliated organizations, or those of the publisher, the editors and the reviewers. Any product that may be evaluated in this article, or claim that may be made by its manufacturer, is not guaranteed or endorsed by the publisher.
Supplementary material
The Supplementary Material for this article can be found online at: https://www.frontiersin.org/articles/10.3389/fbuil.2023.1237476/full#supplementary-material
Abbreviations
ADPe, Abiotic Depletion Potential for elements; ADPf, Abiotic Depletion Potential for fossil fuels; AN, Ammonium Nitrate; AP, Acidification Potential; CAN, Calcium Ammonium Nitrate; CPPL, Composted-pelletized Poultry Litter; EP, Eutrophication Potential; FAETP, Freshwater Aquatic Ecotoxicity Potential; GWP, Global Warming Potential; HTP, Human Toxicity Potential; KCl, Potassium Chloride; LCA, Life Cycle Assessment; MAETP, Marine Aquatic Ecotoxicity Potential; MAP, Monoammonium Phosphate; POP, Photochemical Oxidation Potential; TETP, Terrestrial Ecotoxicity Potential; TSP, Triple Superphosphate.
References
Aguilera, E., Guzmán, G., and Alonso, A. (2016). Greenhouse gas emissions from conventional and organic cropping systems in Spain. I. Herbaceous crops. Agron. Sustain. Dev. 35, 713–724. doi:10.1007/s13593-014-0267-9
Ahmed, J., Almeida, E., Aminetzah, D., Denis, N., Henderson, K., Katz, J., et al. (2020). Agriculture and climate change. Reducing emissions through improved farming practices in McKinsey and Company. Available at: https://www.mckinsey.com/∼/media/mckinsey/industries/agriculture/our%20insights/reducing%20agriculture%20emissions%20through%20improved%20farming%20practices/agriculture-and-climate-change.pdf.
Alhazmi, H., Almansour, F. H., and Aldhafeeri, Z. (2021). Plastic waste management: A review of existing life cycle assessment studies. Sustainability 13, 5340. doi:10.3390/su13105340
Alwaeli, M., and Mannheim, V. (2022). Investigation into the current state of nuclear energy and nuclear waste management—a state-of-the-art review. Energies 15 (12), 4275. doi:10.3390/en15124275
Asselin-Balençon, A., Broekema, R., Teulon, H., Gastaldi, G., Houssier, J., Moutia, A., et al. (2020). Agribalyse 3.0: the French agricultural and food LCI database. Methodology for the food products. Ed. ADEME. Available at:https://nexus.openlca.org/database/Agribalyse.
Avató, J. L., and Mannheim, V. (2022). Life cycle assessment model of a catering product: comparing environmental impacts for different end-of-life scenarios. Energies 15, 5423. doi:10.3390/en15155423
Bahramian, M., and Yetilmezsoy, K. (2020). Life cycle assessment of the building industry: an overview of two decades of research (1995-2018). Energy Build. 219, 109917. doi:10.1016/j.enbuild.2020.109917
Baldini, C., Bava, L., Zucali, M., and Guarino, M. (2018). Milk production life cycle assessment: A comparison between estimated and measured emission inventory for manure handling. Sci. Total Environ. 625, 209–219. doi:10.1016/j.scitotenv.2017.12.261
Baldowska-Witos, P., Kruszelnicka, W., Kasner, R., Tomporowski, A., Flizikowski, J., and Mrozinski, A. (2019). Impact of the plastic bottle production on the natural environment. Part 2. Analysis of data uncertainty in the assessment of the life cycle of plastic beverage bottles using the Monte Carlo technique. Przem. Chem. 98, 1668–1672. doi:10.15199/62.2019.10.28
Biswas, W. K., Barton, L., and Carter, D. (2008). Global warming potential of wheat production in western Australia: a life cycle assessment. Water Environ. J. 22, 206–216. doi:10.1111/j.1747-6593.2008.00127.x
Brancoli, P., and Bolton, K. (2019). “Life cycle assessment of waste management systems,” in Sustainable resource recovery and zero waste approaches. Editors M. Taherzadeh, K. Bolton, J. Wong, and A. Pandey (Amsterdam: Elsevier), 23–33. doi:10.1016/B978-0-444-64200-4.00002-5
Buyle, M., Braet, J., and Audenaert, A. (2013). Life cycle assessment in the construction sector: A review. Renew. Sustain. Energy Rev. 26, 379–388. doi:10.1016/j.rser.2013.05.001
Cano-Londoño, N., Heriberto, C., and Baracza, K. (2022). Integrated sustainability assessment: exergy, emergy, life cycle assessment. Front. Sustain. 3, 921874. doi:10.3389/frsus.2022.921874
Chia, S. Y., Tanga, C. M., Van Loon, J. J., and Dicke, M. (2019). Insects for sustainable animal feed: inclusive business models involving smallholder farmers. Curr. Opin. Env. Sus. 41, 23–30. doi:10.1016/j.cosust.2019.09.003
Civancik-Uslu, D., Ferrer, L., Puig, R., and Fullana-i-Palmer, P. (2018). Are functional fillers improving environmental behavior of plastics? A review on LCA studies. Sci. Total Environ. 626, 927–940. doi:10.1016/j.scitotenv.2018.01.149
Colomb, V., Amar, S. A., Mens, C. B., Gac, A., Gaillard, G., Koch, P., et al. (2015). Agribalyse®, the French LCI database for agricultural products: high-quality data for producers and environmental labelling. OCL 22, D104. doi:10.1051/ocl/20140047
Dhadli, H. S., Brar, B. S., and Kingra, P. K. (2016). Temporal variations in N2O emissions in maize and wheat crop seasons: impact of N-fertilization, crop growth, and weather variables. J. Crop Improv. 30, 17–31. doi:10.1080/15427528.2015.1095264
Enahoro, D., Lannerstad, M., Pfeifer, C., and Dominguez-Salas, P. (2018). Contributions of livestock-derived foods to nutrient supply under changing demand in low- and middle-income countries. Glob. Food Sec. 19, 1–10. doi:10.1016/j.gfs.2018.08.002
European Environment Agency (2004). Agriculture and the environment in the EU accession countries. Implications of applying the EU common agricultural policy. Environmental issue report No 37. EEA, Copenhagen. Available at: https://www.eea.europa.eu/publications/environmental_issue_report_2004_37 (Accessed April 27, 2004).
European Union (2023). Waste and recycling. Available at: https://environment.ec.europa.eu/topics/waste-and-recycling_en (Accessed January 18, 2023).
Gabathuler, H. (2006). The CML story: how environmental sciences entered the debate on LCA. Int. J. Life Cycle Assess. 11, 127–132. doi:10.1065/lca2006.04.021
Gaidajis, G., and Kakanis, I. (2021). Life cycle assessment of nitrate and compound fertilizers production—a case study. Sustainability 13, 148. doi:10.3390/su13010148
Georgakakis, D., and Krintas, T. (2000). Optimal use of the Hosoya system in composting poultry manure. Bioresour. Technol. 72, 227–233. doi:10.1016/S0960-8524(99)00122-4
Ghasempour, A., and Ahmadi, E. (2018). Evaluation of environmental effects in producing three main crops (corn, wheat and soybean) using life cycle assessment. CIGR 20 (2), 126–137.
Gorliczay, E., Boczonádi, I., Kiss, N. É., Tóth, F. A., Pabar, S. A., Biró, B., et al. (2021). Microbiological effectivity evaluation of new poultry farming organic waste recycling. Agriculture 11, 683. doi:10.3390/agriculture11070683
Guinée, J. B., Gorrée, M., Heijungs, R., Huppes, G., Kleijn, R., de Koning, A., et al. (2002). Handbook on life cycle assessment: Operational guide to the ISO standards’, series: Eco-efficiency in industry and science. Dordrecht, Netherlands: Kluwer Academic Publisher.
He, Z. (2020). “Organic animal farming and comparative studies of conventional and organic manures,” in Animal manure: Production, characteristics, environmental concerns, and management. Editors H. M Waldrip, P. H Pagliari, and Z He. 1st ed (Madison, WI, USA: ASA), 165–182. doi:10.2134/asaspecpub67.c9
Holka, M., Bieńkowski, J. F., Jankoxiak, J., and Dąbrowicz, R. (2017). Life cycle assessment of grain maize in intensive conventional crop production system. Rom. Agric. Res. 34, 301–310. Available at:http://www.incda-fundulea.ro/rar.htm.
Holka, M., and Bieńkowski, J. F. (2020). “Life cycle assessment of grain maize production in different soil tillage systems,” in Proceedings of International Academic Conferences 9211579 IISES, Prague, 14-15 November 2019. doi:10.20472/IAC.2019.047.006
Holka, M., Jankowiak, J., Bieńkowski, J. F., and Dąbrowicz, R. (2016). Life cycle assessment (LCA) of winter wheat in an intensive crop production system in wielkopolska region (Poland). Appl. Ecol. Environ. Res. 14, 535–545. doi:10.15666/aeer/1403_535545
Hoshyar, E., and Grundmann, P. (2017). Environmental impacts of energy use in wheat tillage systems: A comparative life cycle assessment (LCA) study in Iran. Energy 122, 11–24. doi:10.1016/j.energy.2017.01.069
Hosoya and Co., Ltd (2020). HOSOYA poultry manure fermentation system. Kanagawa, Japan. Available at: http://www.k-hosoya.co.jp/en/product/(Accessed February, 2020).
International Organisation for Standardization (2006a). ISO 14040:2006, environmental management—life cycle assessment—principles and framework. Geneva, Switzerland: ISO. Available at: https://www.iso.org/standard/37456.html (Accessed June 6, 2019).
International Organisation for Standardization (2006b). ISO 14044:2006, environmental management—life cycle assessment—requerements and guidelines. Geneva, Switzerland: ISO. Available at: https://www.iso.org/standard/38498.html (Accessed June 6, 2019).
Jayasundara, S., Wagner-Riddle, C., Dias, G., and Kariyapperuma, K. A. (2014). Energy and greenhouse gas intensity of corn (Zea mays L.) production in ontario: A regional assessment. Can. J. Soil Sci. 94, 77–95. doi:10.4141/cjss2013-044
Kabakian, V., McManus, M., and Harajli, H. (2015). Attributional life cycle assessment of mounted 1.8 kWp monocrystalline photovoltaic system with batteries and comparison with fossil energy production system. Appl. Energy 154, 428–437. doi:10.1016/j.apenergy.2015.04.125
Kasule, L., Katongole, C., Nambi-Kasozi, J., Lumu, R., Bareeba, F., Presto, M., et al. (2014). Low nutritive quality of own-mixed chicken rations in Kampala City, Uganda. Agron. Sustain. Dev. 34, 921–926. doi:10.1007/s13593-013-0205-2
Kátai, J. (2011). “Determination of nitrogen doses (In Hungarian: nitrogén dózisok megállapítása),” in Soil ecology (in Hungarian: Talajökológia). Editor J Kátai. 1st ed (Debrecen, Hungary: University Press: University of Debrecen), 59–61. University of West Hungary: Sopron, Hungary; University of Pannonia: Veszprém, Hungary.
Khan, M. N., Mobin, M., Abbas, Z. K., and Alamri, S. A. (2018). “Fertilizers and their contaminants in soils, surface and groundwater,” in The encyclopedia of the anthropocene vol 5. Editors D. A. DellaSala, and M. I. Goldstein (Elsevier), 225–240. doi:10.1016/B978-0-12-809665-9.09888
Kiss, N. É., Tamás, J., Szőllősi, N., Gorliczay, E., and Nagy, A. (2021). Assessment of composted pelletized poultry litter as an alternative to chemical fertilizers based on the environmental impact of their production. Agriculture 11 (11), 1130. doi:10.3390/agriculture11111130
Koch, P., and Salou, T. (2020). Agribalyse®: Methodology, agricultural stage; version 3.0. Angers, France: ADAME.
Kruszelnicka, W. (2020). A new model for environmental assessment of the comminution process in the chain of biomass energy processing. Energies 13, 330. doi:10.3390/en13020330
Lamnatou, C., and Chemisana, D. (2015). Evaluation of photovoltaic-green and other roofing systems by means of ReCiPe and multiple life cycle–based environmental indicators. Build. Environ. 93, 376–384. doi:10.1016/j.buildenv.2015.06.031
Magnusson, U. (2016). Sustainable global livestock development for food security and nutrition including roles for Sweden. Stockholm: Ministry of Enterprise and Innovation, Swedish FAO Committee. ISSN: 1652-9316.
Mannheim, V., and Kruszelnicka, W. (2022). Energy-Model and life cycle-model for grinding processes of limestone products. Energies 15 (11), 3816. doi:10.3390/en15103816
Mannheim, V., and Kruszelnicka, W. (2023). Relation between scale-up and life cycle assessment for wet grinding process of pumice. Energies 16, 4470. doi:10.3390/en16114470
Mannheim, V. (2021). Life cycle assessment model of plastic products: comparing environmental impacts for different scenarios in the production stage. Polymers 13 (5), 777. doi:10.3390/polym13050777
Mannheim, V. (2022). Perspective: comparison of end-of-life scenarios of municipal solid waste from viewpoint of life cycle assessment. Front. Built Environ. 8, 991589. doi:10.3389/fbuil.2022.991589
Masters, G. M. (1997). Introduction to environmental engineering and science. USA: Prentice Hall, Inc.
Mbonimpa, E. G., Hong, C. O., Owens, V. N., Lehman, M. R., Osborne, S. L., Schumacher, T. E., et al. (2014). Nitrogen fertilizer and landscape position impacts on CO2 and CH4 fluxes from a landscape seeded to switchgrass. Glob. Change Biol. Bioenergy 7, 836–849. doi:10.1111/gcbb.12187
Mézes, L., Nagy, A., Gálya, B., and Tamás, J. (2015). Poultry feather wastes recycling possibility as soil nutrient. Eurasian Soil Sci. 4, 244–252. doi:10.18393/ejss.2015.4.244-252
Moyo, S., and Swanepoel, F. J. C. (2010). “Multifunctionality of livestock in developing communities,” in The role of livestock in developing communities: Enhancing multifunctionality. Editors F. J. C Swanepoel, A Stroebel, and S Moyo. 1st ed (South Africa and Netherlands: University of Free State and the technical Centre for Agricultural and Rural Cooperation, 1–11.
Nyamadzawo, G., Wuta, M., Nyamangara, J., Smith, J. L., and Rees, R. M. (2014). Nitrous oxide and methane emissions from cultivated seasonal wetland (dambo) soils with inorganic, organic and integrated nutrient management. Nutrient Cycl. Agroecosyst. 100, 161–175. doi:10.1007/s10705-014-9634-9
OpenLCA Nexus (2022). Databases. AGRIBALYSE 3.1. Available at: https://nexus.openlca.org/database/Agribalyse (Accessed April 23, 2022).
Potting, J., Klöpffer, W., Seppälä, J., Risbey, J., Meilinguer, S., Norris, G., et al. (2001). Best available practice in life cycle assessment of climate change, stratospheric ozone depletion, photo-oxidant formation, acidification and eutrophication. Backgrounds and general issues. RIVM report 550015002. Bilthoven, Netherlands: National Institute of Public Health.
Rahman, A., Mondal, N. C., and Tiwari, K. K. (2021). Anthropogenic nitrate in groundwater and its health risks in the view of background concentration in a semi arid area of Rajasthan, India. Sci. Rep. 11, 9279. doi:10.1038/s41598-021-88600-1
Rimantho, D., Syaiful, S., Nurfaida, , and Sulandari, U. (2022). Electronic waste bank model as a solution for implementing circular economy: case study DKI Jakarta-Indonesia. Front. Built Environ. 8, 1030196. doi:10.3389/fbuil.2022.1030196
Sainju, U. M., Ghimire, R., and Pradhan, G. P. (2018). “Nitrogen fertilization I: impact on crop, soil, and environment,” in Nitrogen fixation. Editors E. C. Rigobelo, and A. P. Serra (Intech). doi:10.5772/intechopen.86028
Savci, S. (2012). Investigation of effect of chemical fertilizers on environment. APCBEE Procedia 1, 287–292. doi:10.1016/j.apcbee.2012.03.047
Supasri, T., Itsubo, N., Gheewala, S. H., and Sampattagul, S. (2020). Life cycle assessment of maize cultivation and biomass utilization in northern Thailand. Sci. Rep. 10, 3516. doi:10.1038/s41598-020-60532-2
Szabó, A., Tamás, J., and Nagy, A. (2019). Spectral evaluation of the effect of poultry manure pellets on pigment content of maize (Zea mays L.) and wheat (Triticum aestivum L.) seedlings. Nat. Resour. Sustain. Dev. 9, 70–79. doi:10.31924/nrsd.v9i1.025
Taki, M., Soheili-Fard, F., Rohani, A., Chen, G., and Yildizhan, H. (2018). Life cycle assessment to compare the environmental impacts of different wheat production systems. J. Clean. Prod. 197, 195–207. doi:10.1016/j.jclepro.2018.06.173
Tamás, J. (2010). “Integration of agricultural biogas production and GPS/GIS technology based on LCA,” in 7th international conference (ORBIT 2010): Organic resources in the carbon economy. Editor K. Lasaridi (Thessaloniki: Grafima Publ), 91–98.
Tamás, J., Nagy, A., and Németh, T. (2022). Risks of agricultural water management and opportunities to reduce them in V4 countries. Sci. Secur. 2 (4), 459–467. doi:10.1556/112.2021.00064
Van Harn, J., Dijkslag, M. A., and Van Krimpen, M. M. (2019). Effect of low protein diets supplemented with free amino acids on growth performance, slaughter yield, litter quality, and footpad lesions of male broilers. Poult. Sci. 98, 4868–4877. doi:10.3382/ps/pez229
Wang, W., and Dalal, R. C. (2015). Nitrogen management is the key for low-emission wheat production in Australia: A life cycle perspective. Eur. J. Agron. 66, 74–82. doi:10.1016/j.eja.2015.02.007
Ward, M. H., Jones, R. R., Brender, J. D., De Kok, T. M., Weyer, P. J., Nolan, B. T., et al. (2018). Drinking water nitrate and human health: an updated review. IJERPH 15, 1557. doi:10.3390/ijerph15071557
Wettstein, S., Muir, K., Scharfy, D., and Stucki, M. (2017). The environmental mitigation potential of photovoltaic-powered irrigation in the production of South African maize. Sustainability 9, 1772. doi:10.3390/su9101772
Whitman, T., Yanni, S. F., and Whalen, J. K. (2011). Life cycle assessment of corn stover production for cellulosic ethanol in Quebec. Can. J. Soil Sci. 91, 997–1012. doi:10.4141/CJSS2011-011
Keywords: life cycle assessment, environmental impacts, composted-pelletized poultry litter, chemical fertilizers, maize, winter wheat
Citation: Kiss NÉ, Tamás J, Mannheim V and Nagy A (2023) Comparing the environmental impact of poultry manure and chemical fertilizers. Front. Built Environ. 9:1237476. doi: 10.3389/fbuil.2023.1237476
Received: 09 June 2023; Accepted: 17 August 2023;
Published: 31 August 2023.
Edited by:
Georgios Bartzas, National Technical University of Athens, GreeceReviewed by:
Elio Romano, Centro di ricerca per l'Ingegneria e le Trasformazioni agroalimentari (CREA-IT), ItalyCopyright © 2023 Kiss, Tamás, Mannheim and Nagy. This is an open-access article distributed under the terms of the Creative Commons Attribution License (CC BY). The use, distribution or reproduction in other forums is permitted, provided the original author(s) and the copyright owner(s) are credited and that the original publication in this journal is cited, in accordance with accepted academic practice. No use, distribution or reproduction is permitted which does not comply with these terms.
*Correspondence: Viktoria Mannheim, dmlrdG9yaWEubWFubmhlaW1AdW5pLW1pc2tvbGMuaHU=