- 1Environmental Laboratory, U.S. Army Engineer Research and Development Center, Vicksburg, MS, United States
- 2Warnell School of Forestry and Natural Resources, University of Georgia, Athens, GA, United States
- 3Coastal and Hydraulics Laboratory, U.S. Army Engineer Research and Development Center, Vicksburg, MS, United States
Design alternatives for traditional infrastructure are often compared in terms of expected–and often narrowly defined–costs and benefits to justify the selected plan. Taking a broader life cycle perspective in the benefit-cost evaluation process helps account for potentially rare, indirect, or accruing project benefits. Natural infrastructure design alternatives are generally difficult to compare to conventional alternatives due to their distinctly different costs and benefits. Natural infrastructure differs from conventional infrastructure in terms of performance and benefit development over time, lifespan, materials, intensity of intervention needs, and social and environmental benefits. This paper presents a life cycle framework that expands conventional life cycle analysis to capture other important and relevant aspects of natural and conventional infrastructure, enabling a more complete and equitable comparison of project costs and benefits. The framework consists of four dimensions: risk mitigation performance (e.g., traditional benefit of flood risk management), co-benefits, financial costs (life cycle cost analysis), and environmental costs (life cycle assessment). The framework takes current benefit cost analysis practice for both infrastructure types into account, is informed by existing life cycle evaluation methods and tools and is responsive to the unique needs and characteristics of natural infrastructure. Components of this framework have been advanced elsewhere, including in business product management, asset management, building code development, environmental certifications, ecosystem goods and services accounting, and others, but are generally not developed for natural infrastructure. Our proposed framework provides a roadmap for development of supporting resources to conduct life cycle evaluation for natural infrastructure. Systematically grasping the temporal flow of costs and benefits of natural infrastructure, in comparison to conventional flood risk management projects, will be important as societies address vast infrastructure needs in the face of climate change.
1 Introduction
Natural infrastructure (NI), a type of nature-based solution (NBS), is natural or nature-based elements of the landscape that are built or managed to provide engineering, ecological, and social services utilizing natural ecological processes (Infrastructure Investment and Jobs Act, 2021). NBS including NI are “fundamental pillar(s) of fighting the climate crisis” in the 2022 Report to the National Climate Task Force. While NI can be utilized for a variety of purposes, flood risk management (FRM), including sea level rise mitigation and stormwater management, are the most common applications. Flooding is a frequent and costly natural hazard with a large cumulative economic impact to the US. Since the 1980’s, flooding not associated with tropical cyclones was approximately $150 billion USD; fluvial and pluvial flooding combined with damages from tropical cyclones led to approximately $1.1 trillion USD in damages (Smith, 2020). Interest in integrating NI approaches with conventional flood risk management approaches is increasing amongst many Federal, state, and local agencies and municipalities, most notably due to their potential to produce environmental, social, and/or economic co-benefits in addition to their primary flood risk or stormwater management functions. A life cycle view of prospective infrastructure projects can help to better understand the value of NI as a complement or alternative to conventional infrastructure by accounting for the flows of costs and benefits over time.
Life cycle approaches to planning and managing infrastructure are becoming more common for many types of conventional infrastructure (e.g., roads, bridges, power generation) (Biondini and Frangopol, 2018) but have not been widely developed, accepted, or adopted for NI, particularly in the United States, with the exception of a few published examples mostly examining stormwater measures (Ruangpan et al., 2020). Life cycle methods and tools commonly used for conventional flood and stormwater infrastructure, such as levees and detention ponds, logically should be adapted to include other types of NI, and new tools to plan, design, implement, and manage NI projects are needed to facilitate such adaptation. The objective of this paper is to present a life cycle framework that expands conventional life cycle analysis to capture other important and relevant aspects of natural and conventional infrastructure, enabling a more complete and equitable comparison of project costs and benefits. We provide an overview of aspects of life cycle management relevant to NI, how life cycle methods and tools are applied to conventional flood and stormwater infrastructure and assess the applicability of current life cycle methods and tools to NI. We present a methodological framework that consists of four dimensions to support improved FRM alternative analysis: risk mitigation performance (e.g., traditional benefit of flood risk management), co-benefits, financial costs (life cycle cost analysis), and environmental costs (life cycle assessment). Such a framework, along with supporting tools, is necessary to assess and plan natural infrastructure projects, particularly those dealing with the uncertainties associated with climate change adaptation.
This framework was developed jointly by U.S. Army Corps of Engineers and University of Georgia researchers collaborating through the Network for Engineering With Nature (NEWN). NEWN seeks to improve our understanding of NI costs and benefits, develop tools for implementation, and identify data gaps regarding NI performance over project life cycles. The research reported here is motivated by the need to understand the specific differences and favorability of NI compared to conventional FRM.
1.1 Life cycle management and its application to flood risk management
Life cycle management is a broad conceptual approach to account for the temporal dimension of various categories of costs and benefits; the concept advocates expanding the temporal boundaries of an analysis to capture potentially important but overlooked events. Common motivations are to account for embedded greenhouse gas emissions or understand the true affordability of purchasing or constructing an asset. Life cycle management is broad in that it can be applied to a wide range of products, processes, and systems. Infrastructure life cycle management utilizes methods from business product life cycle management for the design, construction and management of infrastructure elements or systems (Wallbaum and Ebrahimi, 2021). Assessment of infrastructure life cycle takes place during planning and requires tools and data to estimate anticipated asset costs and benefits for all parts of a project life cycle including 1) acquisition and/or construction, 2) performance, 3) operation and/or management, 4) maintenance, 5) rehabilitation, and 6) decommissioning and disposal (The Institute of Asset Management, 2015). This process is closely aligned with asset management. Calls for more widespread use of asset management and life cycle approaches exist for all infrastructure including extension to nontraditional infrastructure such as NI (ASCE, 2020). Increasingly planners are recognizing NI projects - implemented alone and in concert with conventional infrastructure–as an alternative tool for FRM, and thus life cycle management must be adapted to include NI and mixed NI projects.
Aspects of life cycle management are discussed for a subset of NI types referred to as natural and nature-based features in the International Guideline for the Use of Natural and Nature-Based Features (Bridges et al., 2021). However, US Army Corps of Engineers practitioners identified uncertainty and discomfort in planning life cycle management activities as the most significant barrier to implementation of them in coastal storm risk management studies (Piercy et al., 2021). While life cycle management of NI should not be significantly different from life cycle management of conventional flood and stormwater infrastructure, tools and methods used for conventional flood and stormwater infrastructure are neither comprehensive nor mature and were not developed with NI in mind. In particular, the analyses that inform life cycle management rely on data richness for the system of interest, which does not necessarily exist for NI. Databases will need to be expanded and tools adapted for application to NI. The White House Council on Environmental Quality, Office of Science and Technology Policy, and Domestic Climate Policy Office (White House Council on Environmental Quality, 2022) have identified the need for tools to assess benefits and costs of NI projects and has implemented a technical working group, Frontiers of Benefit-Cost Analysis, of federal agency staff to collaborate on benefit-cost analysis of nature-based solutions including NI. Similar efforts are underway in Europe under the Regenerating Ecosystems with Nature-based solutions for hydro-meteorological risk rEduCTion (RECONECT) project, which seeks to develop a framework to evaluate the efficacy of large-scale nature-based solutions (and fall under the larger umbrella of NI), for flood risk management to include pluvial, fluvial, and coastal flooding (Ruangpan and Vojinovic, 2022).
In FRM planning studies, life cycle management usually is limited to a life cycle cost analysis (LCCA) where capital costs and life cycle costs are compared to the monetary benefits produced by the system performance to obtain a defensible cost-benefit ratio or return-on-investment. The top panel of Figure 1 conceptualizes how LCCA is applied during the planning phases of a project and how annual damages, costs, and benefits associated with coastal FRM projects are estimated using a variety of modeling tools. Under this framework, benefits are accrued in association with storm and flood events where damages, avoided damages, or changes in features caused by flood events are the main driver.
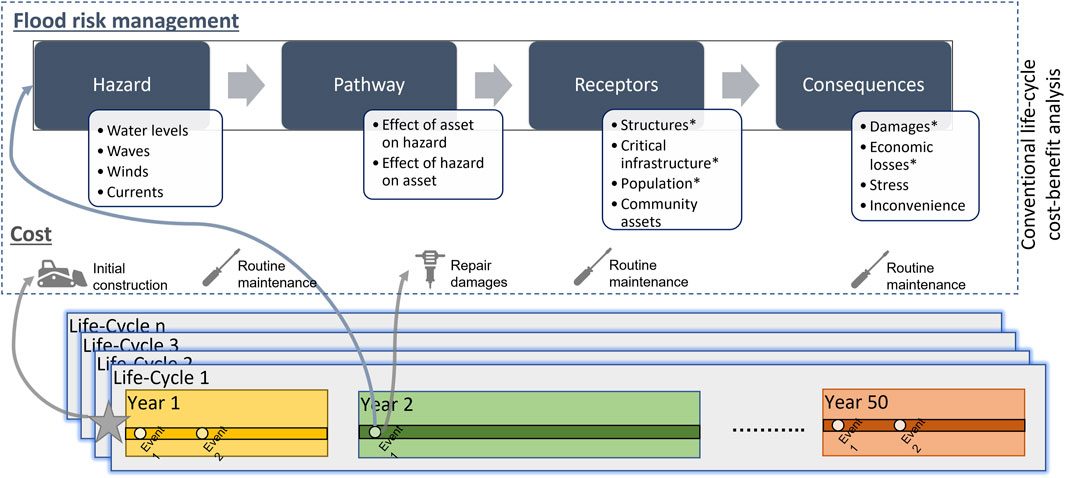
FIGURE 1. Flow diagram of stochastic life cycle cost-benefit analysis for flood risk management assessment. Conventionally, flood events drive the benefits associated with a measure through avoided damages or increase in life safety. Construction, maintenance, and repair drive costs.
Co-benefits (i.e., the additional benefits that are produced by a project beyond its intended purpose) of the project are typically ignored except in some limited cases, such as recreation benefits from beach nourishment. However, co-benefits are fundamental to NI projects. Co-benefits of NI vary by feature but given the multi-purpose nature of most NI projects, co-benefits should be included as part of life cycle management considerations. Hoang et al. (2018) demonstrate how multi-benefit analysis of urban flood management practices can be practically applied to an urban floodplain restoration project on eight different co-benefits. While FRM benefits of NI are still accrued during flood and storm events, co-benefits are typically accrued in the periods between floods and storms (Figure 2). For some projects, these co-benefits may represent a significant proportion of the total project benefits (Stroud et al., 2023).
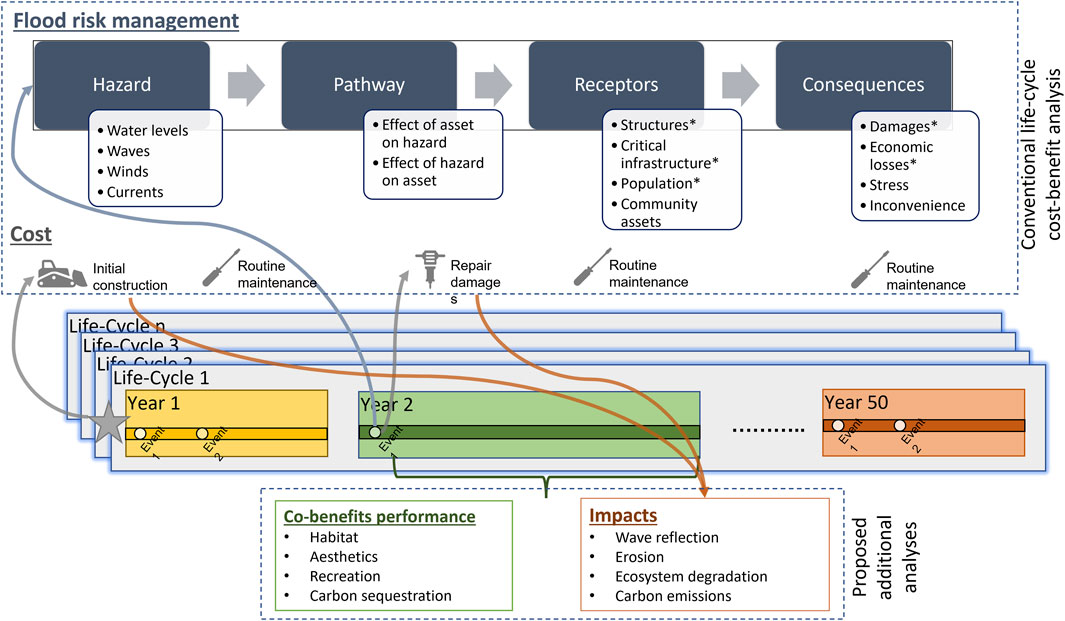
FIGURE 2. Flow diagram of stochastic life cycle cost-benefit analysis for flood risk management assessment including proposed aspects related to explicit accounting for co-benefits and impacts. Note that co-benefits and impacts accrue largely during the intervals between flood events as opposed to the conventional cost-benefit approach that primarily considers benefits associated with flood events.
Impacts (and mitigation for impacts) of projects are considered as part of the cost and regulatory process, typically through environmental impact assessment requirements, such as the process required in the U.S. under the National Environmental Policy Act (NEPA), (Department of Energy, 1970) for many federal activities. Environmental impact assessments conducted for federal projects in the U.S. characterize local impacts to environmental resources such as wetlands, wildlife including threatened and endangered species, water and air quality, and cultural resources. Criticisms of environmental impact assessment have highlighted its shortcomings in demonstrably influencing decision processes (Cashmore et al., 2004; Jay et al., 2007) or for considering societal impacts associated with project decisions (Dendena and Corsi, 2015). Başoğlu et al. (2018) proposed a life cycle assessment methodology to conduct a dynamic environmental impact assessment of urban nature-based solutions designed to address a variety of urban challenges related to climate-risks, which include various hazards such as urban heat islands, pollution, and flooding. Larrey-Lassalle et al. (2022) reviewed 130 studies that use life cycle assessment methodologies to assess nature-based solutions implemented for a variety of reasons, demonstrating the applicability of these methods to NI systems. LCA offers a new dimension for considering environmental impact, which is distinct from that which is currently conducted for FRM projects. Notably, LCA accounts for material and energy flows associated with projects, which enables projects to be compared according to the necessary materials, equipment, and types of interventions at different life cycle stages.
A variety of frameworks have been proposed that integrate these disparate analyses to better inform project decisions. De Risi et al. (2018) proposes life cycle cost and return on investment as complementary decision variables for selecting measures to protect flood-prone buildings. They note that life cycle cost analysis should be complemented by an environmental and social impact assessment (De Risi et al., 2018). To address the issue of comparability of conventional and natural FRM, Lallemant et al. (2021) used hydrodynamic modeling and probabilistic flood risk analysis to capture the flood mitigation ecosystem services of natural infrastructure. Alves et al. (2019) present a method to monetize co-benefits of urban stormwater management measures so they can be included directly in a benefit cost analysis. Petit-Boix et al. (2017) address environmental impacts associated with damage and replacement of assets and the impacts avoided by urban stormwater management measures.
FRM alternative analysis has been framed as a multi-objective or multi-criteria problem. However, findings from Larrey-Lassalle et al. (2022) found many nature-based solutions projects, a subset of which were implemented to address FRM, often mentioned multi-benefits but did not explicitly analyze the project using multi-objective or multi-criteria methodologies. Alves et al. (2018) presents a multi-criteria decision analysis framing to evaluate alternatives based on their performance with respect to risk reduction at different recurrence intervals, cost, and co-benefits and enables inclusion of preferences. Zhu et al. (2023) optimize the placement of urban stormwater management measures to control runoff, reduce life cycle cost, and maximize social, environmental, and economic benefits. Life cycle cost, life cycle assessment, and primary and co-benefit accounting have been applied to urban stormwater management [Xu et al. (2021), and related tools have been developed by the Water Research Foundation (Water Research Foundation, 2023)].
1.2 Differences between NI and traditional infrastructure affecting life cycle management
NI are dynamic due to landscape-level interactions among different features and with environmental processes, which can afford NI the benefit of having some degree of natural resilience (Kurth et al., 2020). They often evolve over time in form and function whereas conventional infrastructure is largely expected to remain static outside of predicted deterioration. For example, renourished beaches and dunes are reshaped and sculpted by waves, tides, and storms, transforming over time to a profile that is different from at the time of nourishment. Aeolian transport processes (i.e., wind) can also passively build dunes, increasing the performance potential of the system in advance of future storm events. Understanding NI performance throughout the life cycle requires understanding the processes that occur between events as well.
Dynamism may present a challenge for life cycle management of NI because it introduces temporal variation and uncertainty into performance, which can result in engineers eliminating NI options from consideration or application of increased factors of safety and possible overdesign of NI solutions. Although conventional infrastructure is faced with environmental processes that deteriorate it, hardened structures are assumed to react more predictably, and engineers are more comfortable with the level of uncertainty in performance, largely due to their familiarity with degradation to conventional infrastructure, whereas assumptions about the evolution of NI do not exist. Conventional structures are not necessarily more robust than NI simply because they are static (robustness may be considered as retaining an acceptable level of performance over a designated range of uncertainty; Spence et al., 2015). Conventional assessments assume that environmental conditions (i.e., hydrologic, climactic) are stationary, but this assumption has been debated for over a decade (Milly et al., 2008; Brown, 2010; Montanari and Koutsoyiannis, 2014). In rivers, it appears that data on a primary physical driver (flow) fall within the range of observed variability (stationary) in regions with little human disturbance, but the majority of catchments with human alternations displayed non-stationary flows over the same time periods (Yang et al., 2021). Similarly, climate change and/or human development is introducing nonstationarity to coastal systems.
NI engineering and management for FRM is relatively new therefore there is little data on the time series of performance and conditions of these projects with respect to FRM performance. Many existing NI projects were not expressly engineered to provide FRM functions and consequently data on the long-term performance of NI with respect to flood risk are sparse. NI evolve in response to episodic phenomenon like flooding as well as everyday phenomenon like tidal inundation or seasonal temperature fluctuations. Coupled with limited data on the long-term performance of NI, it may be necessary to monitor NI more frequently to understand its form and effectiveness over time. Additionally, NI projects may have longer or shorter lifespans than conventional infrastructure depending on how they evolve, which challenges conventional analysis that require a set lifespan. However, monitoring will improve the databased from which projects can draw such estimates.
Instructive examples of NI do exist and these provide evidence of both the long-term performance of NI-based solutions and the ongoing efforts to manage them in the face of changing environmental conditions (See Figure 3). Beach and dune nourishment is an example of a well-established NI practice and is a standard tool for coastal FRM. Beach and dune features naturally build and recover provided sediment supply is sufficient. However, as sediment availability and coastal conditions widely vary, the life span of beach nourishments similarly varies. Some nourishments, especially those that utilize large sediment volumes and include placement in the nearshore, may be largely self-maintaining for relatively long periods (on the order of 10–15 years) even in erosive environments (Brand et al., 2022). Conversely, beaches suffering from high rates of erosion must typically be renourished at intervals from a few years (Weathers and Voulgaris, 2013; Qiu et al., 2020). NI projects such as beach nourishment and dune restoration that utilize sediment should be considered long-term programs, not capital investments requiring only routine maintenance to sustain their function over standardized lifespans (typical for conventional infrastructure; see Figure 1).
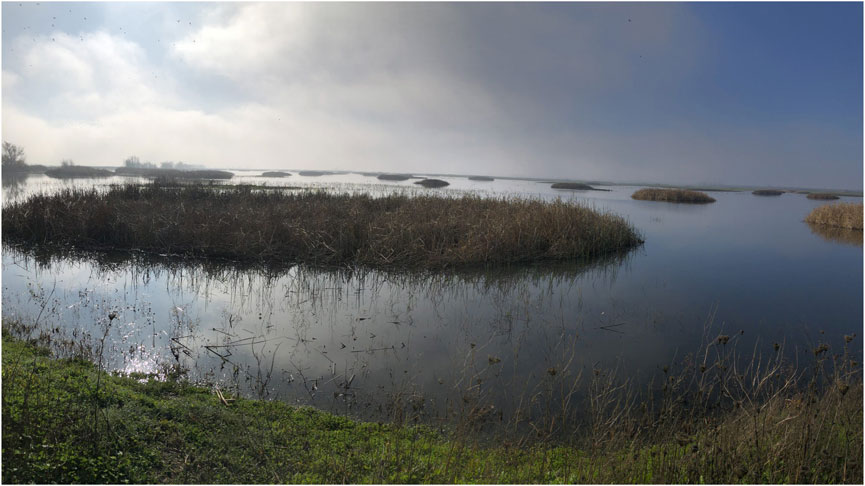
FIGURE 3. Example of Yolo Bypass, CA, an engineered floodplain constructed in the 1930s, demonstrating the longevity and efficacy of NI measures in practice.
1.3 Proposed life cycle management framework for NI
Predicting asset performance is foundational to the analyses that are used for project planning and asset management (Figure 4). Traditional economic analysis associated with flood infrastructure takes a narrow focus on benefits and costs: the benefits of flood risk mitigation are reductions in property damages, injuries, loss-of-lives, and the like and costs are capital costs associated with known construction and operations. However, the cost-benefit concept as applied to NI should be expanded to broadly understand the implications of selecting a project, for example considering the value of the NI itself through the production of co-benefits to fully assess the value of these measures. Previous work on stormwater management has indicated the need to couple both typical “material” flood damages with ecological damages utilizing a risk-informed framework that accounts for ancillary benefits of stormwater best management practices (Petit-Boix et al., 2017). While the environmental impacts of projects are considered as part of project planning through compliance with NEPA, some environmental impacts have not historically been considered as part of life cycle management and consequently many coastal, estuarine, and riverine shorelines have become hardened due to FRM measures, resulting in degradation of the local ecological system (hardened shorelines impacts), compounding flood risks to adjacent areas in some cases (Gittman et al., 2015), and increasing anthropogenically-derived materials in waterways (Elhacham et al., 2020).
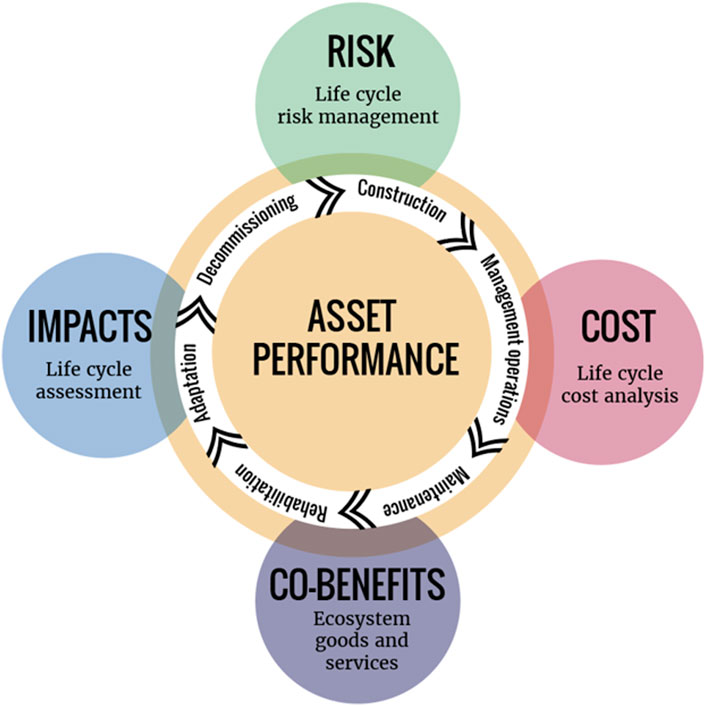
FIGURE 4. Proposed life cycle management approach designed to better capture the benefits, costs, and impacts associated with natural infrastructure.
A broader view of life cycle management also provides opportunity for greater stakeholder engagement. Identifying, organizing, and unifying stakeholders is widely recognized as a primary component to promote the success of any hazard mitigation plan [e.g., FEMA’s Hazard Mitigation Planning Process (FEMA, 2011)] and naturally translates to NI solutions to mitigate flood risks (Kumar et al., 2020). Community integration provides the means to identify conflicts of interest early in the development process, and can provide the opportunity to reconcile opposing attitudes, promote trust, and foster long-term stewardship (Jahn et al., 2012; Santoro et al., 2019; Kumar et al., 2020). Europe’s Open-Air Laboratories for nature-based solutions to manage hydro-meteo risks (OPERANDUM) is a program currently applying multi-level stakeholder engagement to manage multinational NI projects. While community involvement is often a precursor to initiating any hazard mitigation project, it is also a living process that touches every component of a project’s life cycle.
2 Materials and methods
U.S. Army Corps of Engineers and University of Georgia researchers began by reviewing documented and experiential shortcomings of existing FRM project planning and alternatives analysis. Both practitioners and affected parties have noted that overly narrow definitions and boundaries of project costs and benefits have skewed project comparisons (Government Accountability Office, 2019). There is also acknowledgement that the current paradigm is not well suited to NI alternatives, coupled with barriers posed by uncertainty (Nelson et al., 2020).
The methodology employed to support the development of a life cycle management framework is threefold. First, the researchers sought a more complete understanding of FRM planning, both from the perspective of policy as well as in practice. Much was derived from first-hand experience of being involved with USACE studies and in the community of practice with others that have experience. The researchers consulted policy documents and sought clarification from subject matter experts.
Secondly, researchers investigated the applicability of current practice to NI. NI planning analysis needs were well known to researchers and consultant experts from experience. Many of the needs are described in the International Guidelines on Natural And Nature-Based Features For Flood Risk Management (Bridges et al., 2021). An element that was generally missing from practice, but that is crucial for NI is the ability to consider dynamism of features over time and the implications for performance, costs, and benefits. Further, costs and benefits are traditionally not defined broadly enough to capture those associated with NI, omitting considerations of outcomes that may be important to stakeholders. These include benefits of NI over conventional FRM such as inconvenience costs averted, emissions avoided, transportation costs for construction material avoided, as well as many a collection of other ancillary benefits that are cited for NI (e.g., ten Veldhuis, 2011; Bates et al., 2015; Adamowicz et al., 2019). These missed costs and benefits are further detailed in the description of the framework, within their respective life cycle management dimensions. Dimensions that emerged from this process comprise the proposed framework.
Lastly, researchers conducted a review of existing life cycle management studies and tools. This effort searched for research on life cycle management for FRM, NI, and infrastructure more broadly that addresses one or more dimensions of the envisioned framework. Tools were also reviewed, resulting in many options to recommend for inclusion in FRM planning practice. Overall, it was concluded that a single framework and tool set for FRM planning does not already exist. Related research that was found is cited within the most relevant life cycle management dimensions. Tools that were discovered that may be useful to satisfying the proposed life cycle management framework are documented in the appendix to this paper. FRM projects including NI projects are diverse, therefore a single tool kit is not suitable; practitioners will need to select the most appropriate tools for their needs. Later in the paper, there is a discussion about gaps in tools and data that should be remedied to more fully support the life cycle management framework proposed.
3 Results–newly proposed NI lifecycle management framework
We propose an expanded view of life cycle management that can capture the additional co-benefits associated with NI measures as well as potentially overlooked aspects of infrastructure planning such as carbon emissions associated with construction, maintenance, and eventual decommissioning of FRM assets (Figure 4). These aspects are assessed independently or dependently, as the four concepts (Risk management, Cost, Co-benefits, and Impacts) are ultimately linked, and are guided by stakeholder needs and values, standards and best practices and supported by software tools and various methodologies. Project requirements for FRM infrastructure are defined using life cycle risk assessment approaches to determine how the asset manages risk from flood hazards. In the case of NI measures, assets may have additional requirements to produce co-benefits such as recreation, habitat, aesthetics, air and water quality, and others, requiring the addition of approaches such as ecosystem goods and services. Performance with respect to flood risk management and co-benefit production is offset by economic costs and environmental impacts which are quantified using life cycle cost analysis to determine the economic cost of life cycle activities and life cycle assessment to determine the environmental impacts of the asset including impacts associated with the construction and decommissioning of the asset. These principles are similar to those espoused in International Standards Organization (ISO) 15686 on service life planning, which focuses on life cycle planning concepts for buildings and constructed assets with added specificity for NI (International Organization for Standardization, 2011). In practice, additional consideration of co-benefits and environmental impacts associated with FRM projects requires analysis not only of individual flood events as is demonstrated in Figure 1, but also the periods between flood events (see Figure 2) during which long-term co-benefits largely accrue. Environmental impacts due to construction, repair, and maintenance of FRM assets are largely accounted for indirectly via regulatory compliance. However, not all environmental impacts associated with FRM projects are explicitly considered in the conventional benefit-cost approach. The proposed framework provides a way these impacts such as carbon emissions or erosion adjacent to projects can be explicitly considered and not simply mitigated for.
3.1 Flood risk management performance
While NI approaches can be used to address many risks, we limit our discussion here to flood risk. FRM is commonly examined using a source-pathway-receptor-consequence model (CIRIA, 2013). In this context, performance typically refers to the ability of the NI measure (alone or combined with a conventional measure) to transform the pathway of the source hazard, reducing its impact on the receptors such as structures or people and lessening consequences. As such, FRM benefits are often quantified as damages avoided. While this approach is designed to be broadly applied to examine the efficacy of all FRM systems, the analysis requires quantification of the performance of individual FRM assets under different scenarios or probabilistically (Figure 1).
The quantification of flood hazards for NI is the same as for conventional infrastructure and requires an assessment of all forms of flood hazard, which may include fluvial, pluvial, and/or coastal sources for current and future conditions, in the intended context. Typically some combination of low- and high-resolution meteorological and regional and local hydrodynamic models are used to define the flood hazard (Afshari et al., 2018; Wing et al., 2018; Diehl et al., 2021) as well as the flood pathway. While high-resolution models are not always required to make FRM planning decisions, for most NI (and some types of conventional measures), performance is time-dependent (e.g., the duration of the flood event affects the performance), requiring temporally-explicit analysis that considers flood event dynamics. Forecasting how performance will change under alternative climate scenarios is also important (FEMA, 2011; Beechie et al., 2012; Wohl et al., 2015; Daigneault et al., 2016), and can entail modeling how key indicators (e.g., flood peaks, duration, and volumes) vary across different climate scenarios, such as by using general circulation models and regional climate models (Spyrou et al., 2021). Life cycle analysis of the performance of any measure, conventional and/or NI, require site specific considerations and application of tools and methods relevant to the location, as demonstrated in the life cycle performance analysis of Chesapeake Bay islands (Melby et al., 2005).
As with many planning and design processes, the fidelity of modeling efforts increases as more detail is merited. Simplified models and screening processes dominate early stages while higher fidelity ones are used during design. In general, there are modeling gaps at both the screening and high-fidelity modeling study phases for NI. Although some NI measures such as wetlands or levee setbacks can be considered in the same hydrodynamic models as conventional measures by modifying parameters, dedicated NI tools in existing hydrodynamic models are limited outside of a few implementations (see Supplementary Material). Risk-informed design approaches more explicitly consider the uncertainties in all aspects of FRM assessments including the performance of all FRM measures under all limit states (USACE, 2019) but design standards, which do not currently exist for NI, are still used within the process.
Modeling and engineering design can be more challenging for NI because it is naturally more dynamic than conventional infrastructure owing to its reliance on natural processes such as sediment transport and biological components such as vegetation to help provide its benefits (Piercy et al., 2021). (For an example of how dynamic coastal headlands and barrier islands are modeled please see the Supplementary Material). While the natural dynamism of NI does not affect the efficacy, NI asset performance can be more variable than conventional assets since as-built conditions do not necessarily indicate full functionality. NI performance quantification necessitates risk-informed approaches that require explicit consideration of performance variability. As FRM policies shift from standards-based to risk-informed design approaches, more complex tools are required to develop full designs of FRM measures including NI (Johnson et al., 2022).
Conventionally, FRM measures are designed to minimize impacts to receptors, e.g., dwellings, other structures, and critical infrastructure such as bridges and hospitals, and ultimately reduce damages. Since damages tend to occur under low-frequency, major flood events, applicable FRM measures tend to favor conventional measures such as floodwalls and storm surge barriers that provide greater relative FRM performance for the same project footprint. However, quantifying only tangible consequences such as damages may only provide a partial accounting for economic and social flood impacts. Neglecting intangible or indirect impacts to infrastructure function, such as road flooding, (regardless of whether the road is damaged) can omit important economic and life safety consequences (Pregnolato et al., 2017). Broadening the definition of consequences to include intangible consequences as those that cause disruptions to daily life including flooding of roads, sidewalks, parking lots, etc., can shift FRM projects towards addressing higher frequency events (ten Veldhuis, 2011). Consequently, the set of applicable FRM measures broadens and NI, which typically require larger project footprints to deliver similar performance as conventional measures, are not immediately screened from consideration.
3.2 Co-benefit production
Existing NI features, such as living shorelines (Bilkovic et al., 2016) or the Yolo Bypass (Figure 3), demonstrate the ability of NI to provide other appreciable benefits in addition to FRM such as habitat, nutrient cycling, and carbon sequestration, among others. As such, life cycle management methods should capture these in addition to economic and FRM benefits, such as ecological and social co-benefits. Ecosystem services (ES) and the natural capital that sustain them provide a framework to quantify and link ecological processes to our socio-economic needs (Costanza et al., 1997; Carpenter et al., 2009; Ruckelshaus et al., 2013; Adamowicz et al., 2019) and have been steadily becoming more impactful in linking science with decision-makers and policy experts (Guerry et al., 2015) However, salient indicators are often context-specific and variability in physical and biological processes can complicate our ability to predict how a given system will respond to intervention. A brief review of restoration efforts and their use of ES metrics can provide insights into some of these challenges.
Restoration projects often begin with forecasting changes to physical properties, e.g., flow, sediment distribution, and water quality. Redistribution of sediments is generally the primary physical characteristic that is considered with regards to dam removal (Foley et al., 2017), along with mobilization of sediment contaminants (Evans, 2015). Improved connectivity between a river and its adjoining floodplain can be quantified using changes in hyporheic exchanges (Singh et al., 2018) that promote more opportunities for biogeochemical cycling and can improve water quality. A variety of hydrologic and hydraulic models are available to inform project alternatives for riverine efforts (Foley et al., 2017; Juan et al., 2020; Kumar et al., 2020; Spyrou et al., 2021), yet there remains a great deal of uncertainty. Consider dam removals, which have increased exponentially since the 1970’s (Hart et al., 2002; O’Connor et al., 2015) and are well documented (American Rivers data base). While the redistribution of sediments that have accumulated behind the dam varies by location and strategy, sediment distributions have tended to return to a steady state much sooner than expected based on numerical models (Foley et al., 2017).
Biological processes tend to manifest at slower rates and with greater variability than physical ones (Foley et al., 2017). This additional uncertainty can be reduced by coupling physical models with ecologically-oriented ones to forecast habitat suitability for a target species (Tomsic et al., 2007). Life history characteristics and regional population dynamics, however, play crucial roles in tuning response rates. Consider the Pacific Northwest, where a central ecological goal is the re-establishment of regional anadromous salmonid populations. Historical habitats can be opened up by removing a dam, but this can lead to short-term recruitment of multiple species that may stray into the newly available habitats (Quinn et al., 2021). Such species-specific biological goals assume that reviving natural physical conditions will preferentially aid native populations recover across generations–processes that can occur quickly (Beheshti et al., 2021) or take decades (Wohl et al., 2015; Foley et al., 2017). Restoration efforts at a local (e.g., reach) scale can also be offset by regional forces or stressors that can dominate any local potential, such as the sensitivity of local communities to agricultural stressors or dam operations (Palmer et al., 2014; Wohl et al., 2015; Markovic et al., 2019).
Despite the challenges of predicting how an intervention will impact a region’s ecosystem, guiding principles and frameworks have surfaced. In riverine systems, efforts focused on reconnecting rivers to their floodplains and enabling seasonal variability based on natural cycles tend to be more productive than projects that focus on structural changes, e.g., channelization or adding woody debris (Palmer et al., 2014; Wohl et al., 2015). Adopting reference sites facilitates the task of identifying tangible goals and measuring the effect of an intervention (Beheshti et al., 2021), whether that be moving towards or away from a given condition (Palmer et al., 2014). The use of ES to evaluate projects has also grown since their promotion by the Millennium Ecosystem Assessment (Millennium Ecosystem Assessment, 2005). Deciding how to value non-market goods, like biodiversity, has long been problematic (Costanza et al., 1997; Wilson and Carpenter, 1999; Ruckelshaus et al., 2015) and the range of potential ES metrics available has resulted in diverse methodologies and applications that can be difficult to synthesize (Polasky et al., 2015; Hanna et al., 2017). Yet, focusing on the monetary value of a social or ecological metric is not always necessary for effective decision-making (Ruckelshaus et al., 2015) or can be avoided by treating non-market metrics as constraints or trade-offs in multi-objective optimization models (Poff et al., 2015; Grimm and Lund, 2016) or other multi-criteria methods. The diversity of metrics available to quantify ES is not necessarily detrimental either, as context-dependencies and regional constraints will certainly call for different metrics to measure success in different regions. It is far more important to engage local stakeholders early and often during projects to ensure that any physical interventions will map onto perceived value to the local communities (Palmer et al., 2014; Ruckelshaus et al., 2015; Kumar et al., 2020).
3.3 Financial costs–Life cycle cost analysis
Life cycle cost analysis (LCCA) is an economic project evaluation method that considers all costs including costs associated with owning, operating, maintaining, and rehabilitating or disposing of the project at the end of its service life (Kneifel and Webb, 2022). LCCA is formalized in ISO 15686-5 for asset service life planning (ISO, 2006) and adopted by into other standards for sustainability (ASTM, 2022). The ISO provides guidance on scoping, selecting costs to include, decision-relevance, and more (ISO, 2006). ASCE recommends LCCA methods be employed as part of the planning process for all significant infrastructure investments to avoid placing too much importance on up-front capital costs (ASCE, 2018). However, LCCA methods have not been widely adopted at the Federal level (beyond Federal facilities) and guidance is generally piecemeal or narrowly defined for infrastructure components (Eno Center for Transportation and ASCE, 2014; USACE, 2020; Kneifel and Webb, 2022). In reviewing the current state of practice of LCCA in infrastructure development, methods found were overwhelmingly designed for and applied to buildings, roads, and bridges, and generally not to infrastructure categories such as flood infrastructure. These tools are not universally applied to other infrastructure categories such as flood infrastructure. Even when LCCA methods are required, issues arise in application that may especially impact the estimated costs of NI measures. USACE planning guidance explicitly requires estimation of project implementation costs including costs for planning and design, construction, construction contingency, and operations, maintenance, repair, rehabilitation, and replacement (OMRR&R) costs as well as any costs associated with the project such as land costs or environmental compliance (USACE, 2000; USACE, 2016). However, in practice, the detail and specificity of each cost component estimate varies. For instance, the Interstate Council on Water Policy has issued a principal statement requesting USACE provide more specificity in OMRR&R costs (ICWP, 2020).
Even when LCCA methods are utilized, unintended consequences can emerge. Examples of application of LCCA methods often include only costs borne by the entity responsible for planning, design, and construction, sometimes referred to as agency costs, often neglecting costs borne by users as well as externalities such as environmental and social costs and costs of failure (Jawad et al., 2018). For instance, many USACE FRM projects are cost-shared between the Federal government and a local sponsor such as a state or municipal government. After construction and a defined post-construction period-of-performance, the FRM infrastructure becomes the responsibility of the local sponsor for all OMRR&R costs. Since OMRR&R costs depend heavily on future conditions as well specific procedures used to implement required OMRR&R actions, the associated costs are subject to a greater degree of uncertainty than planning, design, and construction costs. The dynamism of NI features can lead to an additional source of uncertainty related to OMRR&R cost, e.g., because it is uncertain how often and to what degree a project will need maintenance or repair.
Cost analysis relies heavily on data from past projects to estimate costs for future projects. In early planning stages, average costs per applicable measurement unit (e.g., project area, shoreline length, quantity of necessary material) can be calculated from datasets of similar projects and directly applied to the size of a proposed project in a method called parametric cost estimation. A discount rate is then applied to the capital cost and lifespan of maintenance costs to generate a present-day dollar value for comparing multiple potential projects. The accuracy of this method is inherently dependent on the size and biases of the dataset, and a greater number of projects contributing to the estimated parametric cost reduces uncertainty in costs. Since there are relatively fewer examples of NI combined with general unfamiliarity with NI measures, cost engineers may tend to overestimate NI costs, especially maintenance costs, to counteract the uncertainty in NI performance into the future (van der Jagt et al., 2017). Additionally, monitoring of NI costs and performance is not widely conducted in a manner that provides useful data for potential future projects (Nelson et al., 2020; van Rees et al., 2022). Finally, those projects that do monitor and share cost data are still young, meaning maintenance costs are difficult to accurately assess for projects expected to last several decades. US-based NI cost tools like The Water Research Foundation’s CLASIC (2021) and Water environment Research Foundation BMP and LID Whole Life Cost Models (Water Research Foundation, 2023) do exist but focus primarily on stormwater management. On the other hand, the United Kingdom Environment Agency has a long-term costing tool for coastal risk management that is limited for US use by UK-specific costs (UK Environment Agency, 2015). Currently, NI cost data can be gathered from the documentation of previously mentioned tools and the projects and implementation guides that exist (USACE, 2015; USDOT, 2019; Taylor Engineering, 2020).
3.4 Environmental impacts–Life cycle assessment
Life cycle assessment (LCA) focuses on environmental impacts of a product at stages of its life cycle (ASTM, 2022). LCA is an established tool in the ISO 14000 series standards for environmental management and sustainability, specifically 14040/44 (ISO, 2006) and serves an important role in managing environmental impacts and sustainability of the built environment (ASTM, 2022). The primary steps of LCA are to define the boundaries of the system in question, create an inventory of the flows of materials and energy into and out of the system, and then translate those flows into impact, usually with databases intended for that purpose. The inventory phase is an exercise of accounting for the quantity of outputs of concern, e.g., greenhouse gases emitted or sequestered, and the subsequent impact analysis estimates the implications of those quantities. Some studies stop at the inventory stage. Sometimes LCA and LCCA are conducted in conjunction, or one as a component of the others. Parrish and Chester, 2013 consider life cycle costing within LCA to be one of three components of the larger analysis and when used in this way, takes a broader view than is used in the architecture, engineering, and construction (AEC) industry.
ISO 14040 lays out the guidelines and requirements for conducting an LCA. It provides notable guidance on scoping, analysis, and reporting to create comparable results. It notes that LCA is one of many environmental management techniques (ISO, 2006). Other techniques many be appropriate for specific FRM studies however to meet the objectives of the framework proposed here, assessment over the life cycle of features is integral.
During our review of LCA tools and applications, buildings were identified as a potentially instructive analog for FRM infrastructure type. LCA is used for buildings in pursuit of designs that are “greener” than conventional ones by considering building materials and illuminating the performance implications of design decisions (e.g., energy consumption). Lower impact or higher performance building materials and designs can lead to higher capital costs but are intended to have economic, environmental, and/or social payoffs over a building’s life cycle. LCA outputs are important to understanding whether an alternative design is worth pursuing; the environmental impacts and benefits can be considered alongside and traded-off with cost, performance, and other metrics. The building industry has at least 30 years of experience in mainstreaming nonconventional material and designs to improve environmental performance and occupant health, as evidenced by the US Green Building Council and their Leadership Energy and Environmental Design certification program.
There are several off-the-shelf tools that are available for conducting LCA for building applications and otherwise, some of which have built-in databases, such as SimaPro (PRé Sustainability, 2023), Product Sustainability Solutions Software (formerly known as GaBi) (Sphera, 2023), and Building for Environmental and Economic Sustainability (BEES) (NIST, 2023). However, there are some characteristics of NI that make existing tools unsuitable. Since many NI options primarily utilize sediments and organic materials such as vegetation, material manufacturing and disposal costs may be greatly diminished compared with conventional infrastructure options that rely on materials such as steel and concrete, for example, which may have additional costs. To the knowledge of this project, the materials and flows associated with NI project life cycles are not generally featured in existing LCA tools and databases.
Another challenge of LCA for NI, as previously mentioned, is life cycle uncertainty. The life cycle needs of NI are highly dependent on uncertain external factors. Given that one of the primary expected benefits of many NI features is to attenuate environmental stressors (e.g., wave attack or high river flows), it is reasonable to consider that they will degrade and need maintenance and repair over time. On the other hand, some features may be partially or fully self-sustaining (e.g., accrete sediment or reestablish vegetation). Understanding the range of possible maintenance and repair intensities is important in the context of characterizing NI feature life cycles because they constitute material and energy flows but must be linked to expected stressors and performance. Probabilistic methods for life cycle considerations do exist for natural hazard prone buildings, e.g., to evaluate damage and then associate environmental impact with repair and retrofit measures (Di Bari et al., 2020). Performance uncertainty impacts all the aspects of a life cycle approach, as envisioned here, and therefore should likely be handled outside of specific analytical tools.
LCA for NI should potentially be extended to beneficial impacts as well. NI benefits are both in the form of positive environmental outputs as well as environmental impact avoidance. The broader community of LCA tools may introduce additional categories of benefits associated with NI. Some benefit categories of NI are closely related to negative impact categories but there may not be an existing approach to account for offset (e.g., LCA can be used to account for water quality impacts of a product or process but not the water quality improvements).
4 Example applications
While there are many examples of NI in practice and in the peer-reviewed literature of projects that address some aspects of the proposed life cycle management framework for, no examples were found demonstrating all four aspects of the framework presented here. Below we highlight three NI projects that address some of the dimensions of the proposed framework and can serve as initial demonstrations of the life cycle management concept in practice. We expect that different projects will need to take different approaches to satisfy each dimension based on available data and models as well as resource constraints. Therefore, the results will take different forms but generally will be structured like the results of economic or multi-objective alternative analyses.
4.1 Louisiana coastal master planning: application of life cycle management concepts
To combat the U.S.’s most severe coastal land loss problems, Louisiana’s Coastal Protection and Restoration Authority (CPRA) has developed and maintains a living Coastal Master Plan that lays out a comprehensive set of structural (levees, floodgates, barrier islands, etc.) and nonstructural (e.g., elevation, acquisition, floodproofing) land development and flood risk reduction projects and goals for the state (CPRA, 2017a). As of 2017, CPRA has completed or funded 135 projects that have resulted in nearly 15,000 ha of land benefits, 450 km of levees improved, and 97 km of barrier islands and berms constructed (CPRA 2017b). The planning framework of The Coastal Master Plan is an iterative process between integrated coastal system models and an optimized planning tool (Brown et al., 2017; Groves et al., 2017) designed to consider both FRM metrics as well as co-benefits. A suite of models is employed to inform the Planning Tool, and these can undergo extensive revision and updating based on reviews from outside advisory panels (Cobell et al., 2017; Rose and Sable, 2013; Callaway et al., 2017). An Integrated Compartment Model (ICM) is used to forecast landscape change (White et al., 2016) and an Ecopath with Ecosim (EwE) model (Cobell et al., 2017) determines fish and shellfish responses to simulate the coastal ecosystem response, which describe the co-benefits of the coastal Louisiana system.
Additionally, statistical flood risk metrics are determined using a combination of Advanced Circulation-Simulated Wave Nearshore models (ADCIRC-SWAN) to calculate storm surge induced water depths and the Coastal Louisiana Risk Assessment Model (CLARA) to determine the equivalent risk (CPRA 2017b; CPRA 2017a; Brown et al., 2017; Groves et al., 2017; Hijuelos and Reed, 2017). Outputs from these models are combined with planning constraints (i.e., sediment availability, funding, stakeholder preference) to inform the Planning Tool, a computer-based decision support software system. Project cost is integrated into the Planning Tool and combined with sediment availability to select cost-effective restoration projects that are informed by the availability of sediment. This tool is comprised of Multi-Criterion Decision Analysis (MCDA) and Robust Decision Making (RDM) within an overarching deliberation-with-analysis process which ultimately identifies design alternatives to construct the most land or reduce the flood risk while meeting the specific criteria (Groves et al., 2017; Hijuelos and Reed, 2017). Simulations are focused on a 50-year horizon to capture long-term trends while trying to limit environmental uncertainties (e.g., sea level rise, project costs, etc.) which are deemed too great beyond this timeline (Brown et al., 2017; Groves et al., 2017).
4.2 South corridor flow restoration, Tillamook River, Oregon
In 2017, tidal flow was restored to 690 acres of leveed publicly and privately owned pasture and farmland at the confluence of the Wilson and Trask Rivers as part of a habitat restoration project focused on salmon habitat creation while reducing flooding to adjacent communities (Shaw and Dundas, 2021). The resulting project restored 443 acres of tidal wetland and subsequent hydrodynamic modeling indicated the project resulted in reductions in flood duration and magnitude in the area for storms with water levels up to an annual exceedance probability of 0.2 (i.e., floods that occur approximately 1 year in five) (Collins, 2019). While the hydrodynamic analysis indicated the project achieved the desired FRM performance, other co-benefits of the project were not initially quantified or monitored. A subsequent analysis (Shaw and Dundas, 2021) of the ecosystem goods and services produced by the project indicated the following potential socio-economic benefits of the project:
• savings of $1,500-$8,000 per year from reduced dredging requirements,
• reduced travel disruption costs of $7,200 per flood event,
• $530,000-$736,000 in carbon storage,
• an increase of 10% in home values within 0.75 mi of the project,
• 108 jobs and total economic output of $14.6M, and
• unquantified but potential large benefits to the salmon fishery as well as quality of life benefits to the community.
The cost of the project through 2021 was estimated at $11.2 M. While the ecosystem goods and services were not pooled and analyzed, the initial evaluation indicates the co-benefits of the project may exceed those related to FRM. Benefits to the salmon fishery alone could reach the millions of dollars as the habitat matures, indicating the need to understand the dynamics of NI projects as many co-benefits may mature years after project implementation.
4.3 Vegetated foreshore life cycle
Estuarine shorelines across the Netherlands commonly utilize a NI method in which a vegetated foreshore is maintained seaward of the dike system. The vegetated foreshore component of the system is a salt marsh-mudflat feature of varying width that serves to dampen waves before they impact the earthen dike while the dike serves as a barrier to higher surge water levels. The two components combined provide a greater degree of FRM performance as compared to earthen dike alone (Vuik et al., 2017). The vegetated foreshore also reduces wave overtopping and erosion of the dike, increasing its efficacy during surge events. Analysis of historical levee breaches also found that the presence of vegetated foreshores reduced the size of breaches and limited flooding into the hinterlands (Zhu et al., 2020). These multiple modes of performance highlight how analysis of NI measures may require additional analyses to assess all aspects of their function, especially when considered in combination with conventional measures such as dikes or floodwalls.
Decadal-scale analysis of vegetated foreshores found them to be relatively stable over time throughout the Western Scheldt Estuary with spatial variability between sites greater than the temporal variability at any given site (Willemsen et al., 2020). While the long-term stability of vegetated foreshores in other locations will vary with sediment availability and wave energy, modeling studies of the vegetated foreshore-dike system indicate the long-term dynamics and FRM performance are predictable using existing modeling tools (Willemsen et al., 2022). The relative stability of vegetated foreshores indicates maintenance and management requirements of these features is limited. Since vegetated foreshores have been demonstrated to reduce maintenance and management requirements for adjacent dikes as well (Marin-Diaz et al., 2023), logic follows that environmental impacts associated with dike maintenance would also be reduced.
An analysis of various adaptive measures designed to increase the safety level of existing earthen dikes in the Netherlands found vegetated foreshores, especially those that utilized detached breakwaters or high elevation transitional zones adjacent to the dike, were more cost-effective than the conventional option of dike heightening (Vuik et al., 2019). Vegetated foreshores that were actively managed to maintain high elevation transition zones adjacent to the dike provided a greater safety level under future sea level rise conditions than the conventional dike heightening option, demonstrating the cost-effectiveness, efficacy, and adaptive capacity of NI combined with conventional measures. While most work on vegetated foreshores has been done in the Netherlands, a global scale analysis that includes both marsh and mangrove vegetation indicated implementing vegetated foreshores can reduce wave heights by 25% for 11.5% of the world’s coastline and the required reduction in dike height for a 0.01 annual exceedance probability event would save $320.2B if all flood-prone populations were protected (van Zelst et al., 2021).
While co-benefits of vegetated foreshores as a practice have not been fully quantified, the various ES of coastal wetlands have been documented including primary productivity, carbon sequestration, habitat, tourism, and support of wild and commercial fisheries, among others (Whittaker and Likens, 1975; Boesch and Turner, 1984; Costanza et al., 2014; Barbier, 2019). The exact monetary or non-monetary value of the co-benefits produced at a particular vegetated foreshore site depends on the site characteristics and history, but regional data can be used to provide an estimate of the co-benefit of interest at a site.
5 Discussion
The life cycle framework developed in this paper addresses multiple accounting needs related to planning and selecting NI alternatives. It is intended to reflect planning procedures that are used for other infrastructure types and prescribed by ISO, while also aspiring to improve on the status quo in FRM planning procedures by bringing inter-related project outcomes under one framework (e.g., costs and benefits depend on performance). Government agencies involved in FRM typically conduct alternative analysis with narrowly defined economic performance however there are policy developments that will require more comprehensive accounting. A life cycle perspective is particularly important for NI because lack of experience with it as a formal infrastructure type challenges both intuition and common engineering judgement applied to conventional infrastructure (van der Jagt et al., 2017). NI costs and benefits can accrue differently over time than conventional infrastructure; whereas conventional infrastructure has the greatest benefits when construction is complete and generally degrades as time passes, NI can have the opposite trajectory.
The framework advocates for inclusion of project co-benefits, which can have a similar unconventional temporal trajectory and, the accumulation of which can be non-trivial to stakeholders and decision-makers that seek to balance multiple objectives in alternative selection. There is general increasing demand for nature-based solutions to manage flooding and other environmental risks, likely driven by a number of factors including public distaste for large concrete barriers to manage infrequency weather events as well as the promise of natural infrastructure to deliver numerous benefits regardless of extreme events materializing. Co-benefits and non-monetary costs have historically been left out of benefit cost analysis, rendering a decision rubric that does not necessarily reflect what people value. Ultimately, the framework should support project cost and benefit accounting that will help decision-makers to select a project design that matches stakeholder preferences and meets objectives.
In the review of existing frameworks, we found that NI researchers and practitioners are recommending various elements of the framework proposed here. Frameworks for FRM-related projects tend to focus on hydrometeorological risk and specify the importance of probabilistic analysis to account for project lifespans. Frameworks found almost exclusively are designed for urban stormwater management, or green infrastructure, which is similar to natural infrastructure for FRM and is more mature in terms of resources to support decision-making. Tools and data from green infrastructure were not found to be suitable for NI because green infrastructure tends to be small scale whereas NI will need to be landscape scale. Perhaps more importantly is that green infrastructure is designed to hold and filter overland flow, which is different kind of load than wave attack, wind, and riverine flows. Furthermore, green infrastructure tends to be less dynamic than NI. Co-benefits are noted in most research on natural or green infrastructure for FRM. The life cycle emphasis is implicit in many projects that use a net present value analysis but explicit integration of the framework dimensions with a life cycle emphasis, in which costs and benefits are dependent on performance and evolution, has not been found in the literature.
Although the framework developed in this paper appears simple, in practical application, there are many barriers that exist to its implementation or barriers to the antecedents to using the framework. This paper acknowledges that a) there are some tools and datasets that already exist that can support the implementation of the framework but also that b) new tools and data are needed in order to apply the framework to a diversity of NI feature types, improve the accuracy of forecasting project costs and benefits, and account for a wide variety of costs and benefits that stakeholders may be interested in. Challenges also exist related to the need to generate results that are comparable - across NI feature types, between conventional and natural alternatives, and with mixed units for costs and benefits (e.g., monetary and non-monetary results). Study boundaries also influence whether results are comparable but consistent bounding may be complicated by peculiarities in FRM in the US.
As risk-informed design and life cycle accounting for projects becomes the status quo for all FRM projects, some of the perceived uncertainties associated with NI (i.e., lack of understanding of evolution of NI features over time) will manifest for conventional infrastructure as well. For instance, nonstationary conditions threaten to render some conventional FRM measures unable to provide the designed FRM performance. NI combined with the conventional measures, as in the case of vegetated foreshores, may provide an adaptive mechanism for existing FRM systems. Ultimately advancing life cycle management for all FRM projects may facilitate the utilization of NI measures as complementary measures that can provide additional value and design life to conventional measures.
Monitoring of NI projects is often intended to ensure that projects establish as intended (e.g., new plantings grow and propagate) and inform any necessary corrective action. Monitoring for broader knowledge generation to improve life cycle evaluation is not an established practice and there are barriers to establish that as the new norm. One barrier is lack of consensus on which metrics should be monitored and relatedly, which co-benefits should be accounted for in planning analysis. NI comprises diverse feature types and therefore, there is not a single set of broadly applicable metrics that can be monitored. Data collection and management face challenges posed by funding constraints, typical change in ownership of natural infrastructure during its life cycle complicates whose monitoring responsibility, and currently there is no data reporting and management process.
Implementation of NI should not be delayed by lack of data, instead, monitoring should be regarded as central in order to build the new knowledge base that is needed to support the life cycle framework for NI. In general, many knowledge barriers can be addressed through NI project implementation. Careful project documentation and monitoring will help build the knowledge based. Careful project documentation and monitoring during the execution of NI projects can catalyse a virtuous cycle to reduce uncertainty in all of the dimensions of the proposed life cycle framework, which in turn improves the data available to plan new projects. This would essentially build the database of project costs and benefits that is currently lacking for NI, and greatly reduce the uncertainty associated with all components of the framework. Conventional infrastructure has this essential historical record, formalized into tools and design codes, and on which practitioners can draw when they plan and design projects, e.g., material and labor costs, forecasted and actual performance record, minimum specifications, and economic value of benefiting assets. These do not necessarily exist for NI, especially as a resource that is broadly applicable to different types of features. To some extent, existing NI projects can be leveraged to collect life cycle costs and benefits however, the framework developed here can help inform systematic knowledge generation through the implementation of new projects.
6 Conclusion
The need for such holistic life cycle frameworks for assessing NI projects may be intuitive, but there are many barriers to their implementation. New tools and data are needed in order to apply the framework to a diversity of NI feature types, account for a wide variety of costs and benefits, include social preferences and equity considerations, and improve forecasting accuracy of future costs and benefits. One barrier to life analysis of NI is lack of consensus on which co-benefits should be included and what performance measures can and should be quantified, modelled, and monitored.
Co-benefit production is integral to NI projects, and the time series of benefits of NI projects may be complicated, often increasing over time. Traditional benefit/cost analysis has historically neglected co-benefits, including social and ecological benefits, as well as non-monetary costs, rendering decision rubrics that often neglect social values and preferences. Consequently, NI life cycle analysis fundamentally differs from conventional infrastructure and requires alternate analysis frameworks. More explicit integration of co-benefits and non-monetary costs and incorporation of evolving and possibly stochastic benefit/cost time series are necessary for NI projects.
The uncertain evolution of project co-benefits, particularly the effects of unusually extreme forcing functions produced by climate change, may be perceived as drawbacks of NI projects. However, many of the perceived uncertainties associated with NI will also manifest for conventional infrastructure. Risk-informed design and life cycle accounting will become the status quo for all FRM project, NI and traditional. Engineering and social adaptation to the changing climate will become the norm.
Data availability statement
The original contributions presented in the study are included in the article/Supplementary Material, further inquiries can be directed to the corresponding author.
Author contributions
CJ, MK, and CP contributed to conception and design of the study. CJ, MK, and CP wrote the first draft of the manuscript. BL and BH wrote sections of the manuscript. All authors contributed to the article and approved the submitted version.
Funding
Funding for University of Georgia was provided via a Cooperative Ecosystem Studies Unit agreement with the U.S. Army Engineer Research and Development Center via the U.S. Army Corps of Engineers Engineering With Nature™ research program. Funding for Federal employees was provided directly through the U.S. Army Corps of Engineers Engineering With Nature™ research program.
Acknowledgments
Thank you to Caleb Sytsma and Oscar Villegas for help with editing and formatting. A big thanks to all of the Network for Engineering With Nature™ Life Cycle team members including Mark Risse, Clark Alexander, Ellis Kalaidjian, Emily Russ, Dave Smith, Jose Lopes, and Summer Wright. To Sarah Buckleitner, thanks for the help with graphics. Thank you also to Liya Abera for her guidance on life cycle cost analysis of green stormwater infrastructure. And finally, much appreciation to John Winkelman and Elizabeth Godsey for helping us understand the current USACE planning paradigm for flood risk management projects.
Conflict of interest
The authors declare that the research was conducted in the absence of any commercial or financial relationships that could be construed as a potential conflict of interest.
Publisher’s note
All claims expressed in this article are solely those of the authors and do not necessarily represent those of their affiliated organizations, or those of the publisher, the editors and the reviewers. Any product that may be evaluated in this article, or claim that may be made by its manufacturer, is not guaranteed or endorsed by the publisher.
Supplementary material
The Supplementary Material for this article can be found online at: https://www.frontiersin.org/articles/10.3389/fbuil.2023.1181835/full#supplementary-material
References
Adamowicz, W., Calderon-Etter, L., Entem, A., Fenichel, E. P., Hall, J. S., Lloyd-Smith, P., et al. (2019). Assessing ecological infrastructure investments. Proc. Natl. Acad. Sci. 116 (12), 5254–5261. doi:10.1073/pnas.1802883116
Afshari, S., Tavakoly, A. A., Rajib, M. A., Zheng, X., Follum, M. L., Omranian, E., et al. (2018). Comparison of new generation low-complexity flood inundation mapping tools with a hydrodynamic model. J. Hydrol. 556, 539–556. doi:10.1016/j.jhydrol.2017.11.036
Alves, A., Gersonius, B., Kapelan, Z., Vojinovic, Z., and Sanchez, A. (2019). Assessing the Co-Benefits of green-blue-grey infrastructure for sustainable urban flood risk management. J. Environ. Manag. 239 (1), 244–254. doi:10.1016/j.jenvman.2019.03.036
Alves, A., Gersonius, B., Sanchez, A., Vojinovic, Z., and Kapelan, Z. (2018). Multi-criteria approach for selection of green and grey infrastructure to reduce flood risk and increase co-benefits. Water Resour. Manag. 32, 2505–2522. doi:10.1007/s11269-018-1943-3
American Rivers (2017). American Rivers dam removal database. Available at: https://www.americanrivers.org/media-item/american-rivers-dam-removal-database-now-available-public/ (Accessed March 3, 2023).
American Society of Civil Engineers (2018). Policy statement 451. Life-cycle cost analysis. Reston, VA: American Society of Civil Engineers.
American Society of Civil Engineers (2020). Changing the infrastructure equation: using asset management to optimize investments. Reston, VA: American Society of Civil Engineers.
ASTM (2022). ASTM Standard E2921-22, 2022, “Standard Practice for Minimum Criteria for Comparing Whole Building Life Cycle Assessments for Use with Building Codes, Standards, and Rating Systems,”. West Conshohocken, PA: ASTM International, Available at: www.astm.org. doi:10.1520/E2921-22
Barbier, E. B. (2019). “The value of coastal wetland ecosystem services,” in Coastal wetlands (Netherlands: Elsevier), 947–964.
Başoğlu, D., Yöntem, E., Yöntem, S., Şenyurt, B., and Yılmaz, Ö. (2018). Dynamic assessment of nature based solutions through urban level LCA. From designing sustainable technologies, products and policies: from science to innovation. Berlin, Germany: Springer, 293–305.
Bates, M. E., Fox-Lent, C., Seymour, L., Wender, B. A., and Linkov, I. (2015). Life cycle assessment for dredged sediment placement strategies. Sci. Total Environ. 511, 309–318. doi:10.1016/j.scitotenv.2014.11.003
Beechie, T., Imaki, H., Greene, J., Wade, A., Wu, H., Pess, G., et al. (2012). Restoring salmon habitat for a changing climate. River Res. Appl. 29 (8), 939–960. doi:10.1002/rra.2590
Beheshti, K. M., Williams, S. L., Boyer, K. E., Endris, C., Clemons, A., Grimes, T., et al. (2021). Rapid enhancement of multiple ecosystem services following the restoration of a coastal foundation species. Ecol. Appl. 32 (1), e02466. doi:10.1002/eap.2466
Bilkovic, D. M., Mitchell, M., Mason, P., and Duhring, K. (2016). The role of living shorelines as estuarine habitat conservation strategies. Coast. Manage 44 (3), 161–174. doi:10.1080/08920753.2016.1160201
Biondini, F., and Frangopol, D. M. (2018). Life-cycle performance of civil structure and infrastructure systems: survey. J. Struct. Eng. 144 (1), 06017008. doi:10.1061/(ASCE)ST.1943-541X.0001923
Boesch, D. F., and Turner, R. E. (1984). Dependence of fishery species on salt marshes: the role of food and refuge. Estuaries 7, 460–468. doi:10.2307/1351627
Brand, E., Ramaekers, G., and Lodder, Q. (2022). Dutch experience with sand nourishments for dynamic coastline conservation–An operational overview. Ocean. Coast. Manage. 217, 106008. doi:10.1016/j.ocecoaman.2021.106008
Bridges, T. S., King, J. K., Simm, J. D., Beck, M. W., Collins, G., Lodder, Q., et al. (2021). International guidelines on natural and nature-based features for flood risk management.
Brown, C. (2010). The end of reliability. J. Water Res. Plan. Man. 136 (2), 143–145. doi:10.1061/(asce)wr.1943-5452.65
Brown, S., Couvillion, B., Conzelmann, C., de Mutsert, K., Fischbach, J., Hunnicutt, C., et al. (2017). 2017 coastal master plan: appendix C: modeling chapter 3 - modeling components and overview. Version final. Baton Rouge, Louisiana: Coastal Protection and Restoration Authority, 72.
California Department of Fish and Game (2008). Final land management plan for the yolo bypass wildlife area. Available at: https://wildlife.ca.gov/Lands/Planning/Yolo-Bypass-WA (Accessed February 23, 2023).
Carpenter, S. R., Mooney, H. A., Agard, J., Capistrano, D., DeFries, R. S., Daz, S., et al. (2009). Science for managing ecosystem services: beyond the Millennium ecosystem assessment. Proc. Natl. Acad. Sci. U.S.A. 106 (5), 1305–1312. doi:10.1073/pnas.0808772106
Cashmore, M., Gwilliam, R., Morgan, R., Cobb, D., and Bond, A. (2004). The interminable issue of effectiveness: substantive purposes, outcomes and research challenges in the advancement of environmental impact assessment theory. Impact Assess. Proj. Apprais. 22 (4), 295–310. doi:10.3152/147154604781765860
Callaway, J., Hagen, S., Harris, C., Kimmerer, W., and Waldon, W. (2017). “2017 Coastal Master Plan: Attachment C5-1: Predictive Models Technical Advisory Committee Report,” in Version Final. (Baton Rouge, Louisiana: Coastal Protection and Restoration Authority).
Christian-Smith, J. (2010). “Managing for multiple benefits: farming, flood protection, and habitat restoration in the yolo bypass wildlife area,” in California farm water success stories (Oakland: Pacific Institute), 17–24.
Cobell, Z., de Mutsert, K., Duke-Sylvester, S., Fischbach, J., McCorquodale, J. A., Meselhe, E., et al. (2017). 2017 Coastal Master Plan: Appendix C: Chapter 5−Modeling Conclusions and Looking Forward. Version Final. Baton Rouge, Louisiana: Coastal Protection and Restoration Authority, 1–24.
Collins, V. (2019). As-built project validation of peak water level reduction during the october 2017 flood. Tillamook Oregon solutions. Available at: https://tillamookoregonsolutions.com/2019/02/21/as-built-project-validation-of-Peck-water-level-reduction-during-the-october-2017-flood/.
Construction Industry Research and Information Association (2013). The international levee handbook. Ministére de l’Écologie, du Développement durable et de l’Énergie, and the U.S. Army Corps of Engineers. London: CIRIA report C731.
Costanza, R., d’Arge, R., de Groot, R., Farber, S., Grasso, M., Hannon, B., et al. (1997). The value of the worlds ecosystem services and natural capital. Nature 387 (6630), 253–260. doi:10.1038/387253a0
Costanza, R., de Groot, R., Sutton, P., van der Ploeg, S., Anderson, S. J., Kubiszewski, I., et al. (2014). Changes in the global value of ecosystem services. Glob. Environ. Chang. 26, 152–158. doi:10.1016/j.gloenvcha.2014.04.002
CPRA (2017a). 2017 coastal master plan: appendix E: flood risk and resilience program framework. Version final. Baton Rouge, Louisiana: Coastal Protection and Restoration Authority, 1–66.
Croft, J. (2022). Flood control partnerships aim for a safer Sacramento. Stormwater Solutions. Available at: https://www.estormwater.com/sewers-drainage-systems/flood-control/article/10983682/flood-control-partnerships-aim-for-a-safer-sacramento (Accessed on March 6, 2023).
Daigneault, A., Brown, P., and Gawith, D. (2016). Dredging versus hedging: comparing hard infrastructure to ecosystem-based adaptation to flooding. Ecol. Econ. 122, 25–35. doi:10.1016/j.ecolecon.2015.11.023
Dendena, B., and Corsi, S. (2015). The Environmental and Social Impact Assessment: a further step towards an integrated assessment process. J. Clean. Prod. 108, 965–977. doi:10.1016/j.jclepro.2015.07.110
De Risi, R., De Paloa, F., Turpie, J., and Kroeger, T. (2018). Life Cycle Cost and Return on Investment as complementary decision variables for urban flood risk management in developing countries. Int. J. Disaster Risk Reduct. 28, 88–106. doi:10.1016/j.ijdrr.2018.02.026
Di Bari, R., Belleri, A., Marini, A., Horn, R., and Gantner, J. (2020). Probabilistic life-cycle assessment of service life extension on renovated buildings under seismic hazard. Buildings 10 (3), 48. doi:10.3390/buildings10030048
Diehl, R. M., Gourevitch, J. D., Drago, S., and Wemple, B. C. (2021). Improving flood hazard datasets using a low-complexity, probabilistic floodplain mapping approach. PLoS One 16 (3), e0248683. doi:10.1371/journal.pone.0248683
Elhacham, E., Ben-Uri, L., Grozovski, J., Bar-On, Y. M., and Milo, R. (2020). Global human-made mass exceeds all living biomass. Nature 588, 442–444. doi:10.1038/s41586-020-3010-5
Eno Center for Transportation and American Society of Civil Engineers (2014). Maximizing the value of investments using life cycle cost analysis. Reston, VA: Eno Center for Transportation.
Evans, J. (2015). Contaminated sediment and dam removals: problem or opportunity? Eos 96. doi:10.1029/2015eo036385
Federal Emergency Management Agency (2005). National flood insurance program floodplain management requirements – a study guide and desk reference for local officials. FEMA 480. Available at: https://www.fema.gov/sites/default/files/documents/fema-480_floodplain-management-study-guide_local-officials.pdf (Accessed on March 6, 2023).
Federal Emergency Management Agency (2009). BCA reference guide. Gaithersburg, MD: Prepared by URS Group, Inc. https://www.fema.gov/sites/default/files/2020-04/fema_bca_reference-guide.pdf.
Federal Emergency Management Agency (2011). FEMA Climate change adaptation policy statement. U.S. Dept. Homeland Security. 2011-OPPA-01. Available at: https://www.fema.gov/sites/default/files/2020-07/fema_climate-change-policy-statement_2013.pdf (Accessed March 6, 2023).
Federal Emergency Management Agency (2017). National flood insurance program community rating system coordinator’s manual. FIA-15/2017.
Federal Emergency Management Agency (FEMA) (2022). FEMA Ecosystem Services Value Updates. https://www.fema.gov/sites/default/files/documents/fema_ecosystem-service-value-updates_2022.pdf
Foley, M. M., Bellmore, J. R., O’Connor, J. E., Duda, J. J., East, A. E., Grant, G. E., et al. (2017). Dam removal: listening in. Water Resour. Res. 53 (7), 5229–5246. doi:10.1002/2017wr020457
Garnche, C., and Howitt, R. E. (2011). Species conservation on a working landscape: the joint production of wildlife and crops in the Yolo Bypass. AERE. Available at: https://ageconsearch.umn.edu/record/103973/files/AAEAv4.pdf (Accessed February 23, 2023).
Gittman, R. K., Fodrie, F. J., Popowich, A. M., Keller, D. A., Bruno, J. F., Currin, C. A., et al. (2015). Engineering away our natural defenses: an analysis of shoreline hardening in the US. Frontiers in Ecology and the Environment 13 (6), 301–307. doi:10.1890/150065
Government Accountability Office (2019). Army Corps of engineers, consideration of project costs and benefits in using natural coastal infrastructure and associated challenges.
Grecco, S. E., and Larsen, E. W. (2014). Ecological design of multifunctional open channels for flood control and conservation planning. Landsc. Urban Plan. 131, 14–26. doi:10.1016/j.landurbplan.2014.07.002
Grimm, R. S., and Lund, J. R. (2016). Multi-purpose optimization for reconciliation ecology on an engineered floodplain–yolo bypass, California, USA. San Franc. Estuary Watershed Sci. 14 (1). doi:10.15447/sfews.2016v14iss1art5
Groves, D. G., Panis, T., and Sanchez, R. (2017). 2017 coastal master plan: appendix D: planning tool. Version final. Baton Rouge, Louisiana: Coastal Protection and Restoration Authority, 119.
Guerry, A. D., Polasky, S., Lubchenco, J., Chaplin-Kramer, R., Daily, G. C., Griffin, R., et al. (2015). Natural capital and ecosystem services informing decisions: from promise to practice. Proc. Natl. Acad. Sci. U.S.A. 112 (24), 7348–7355. doi:10.1073/pnas.1503751112
Hanna, D. E. L., Tomscha, S. A., Dallaire, C. O., and Bennett, E. M. (2017). A review of riverine ecosystem service quantification: research gaps and recommendations. J. Appl. Ecol. 55 (3), 1299–1311. doi:10.1111/1365-2664.13045
Hart, D. D., Johnson, T. E., Bushaw-Newton, K. L., Horwitz, R. J., Bednarek, A. T., Charles, D. F., et al. (2002). Dam removal: challenges and opportunities for ecological research and river restoration. BioScience 52 (8), 669. doi:10.1641/0006-3568(2002)052[0669:drcaof]2.0.co;2
Hijuelos, A. C., and Reed, D. J. (2017). 2017 coastal master plan: appendix F: adaptive management. Version final. Baton Rouge, Louisiana: Coastal Protection and Restoration Authority, 1–43.
Hoang, L., Fenner, R. A., and Skenderian, M. (2018). A conceptual approach for evaluating the multiple benefits of urban flood management practices. J. Flood Risk Manag. 11, S943–S959. doi:10.1111/jfr3.12267
Infrastructure Investment and Jobs Act (2021). Public law 117-58 117th congress. Washington, D.C. USA. https://www.govinfo.gov/content/pkg/PLAW-117publ58/pdf/PLAW-117publ58.pdf.
International Organization for Standardization (2011). Buildings and constructed assets — service life planning. ISO 15686.
International Standards Organization (2006). Environmental management — life cycle assessment — requirements and guidelines. ISO 14044.
Interstate Council on Water Policy (2020). ICWP principle statement on U.S. Army Corps’ of engineers project partnership agreements. Available at: https://icwp.org/wp-content/uploads/2022/06/202001USACE_PPA.pdf (Accessed on March 6, 2023).
Jahn, T., Bergmann, M., and Keil, F. (2012). Transdisciplinarity: between mainstreaming and marginalization. Ecol. Econ. 79, 1–10. doi:10.1016/j.ecolecon.2012.04.017
Jawad, D., Medawar, Y., and Ghanimeh, S. (2018). “Framework for conducting life cycle cost analysis for infrastructure facilities,” in Urbanization challenges in emerging economies: energy and water infrastructure; transportation infrastructure; and planning and financing (Reston, VA: American Society of Civil Engineers), 450–461.
Jay, S., Jones, C., Slinn, P., and Wood, C. (2007). Environmental impact assessment: retrospect and prospect. Environ. impact Assess. Rev. 27 (4), 287–300. doi:10.1016/j.eiar.2006.12.001
Johnson, D. R., Wang, J., Geldner, N. B., and Zehr, A. B. (2022). Rapid, risk-based levee design framework for greater risk reduction at lower cost than standards-based design. J. Flood Risk Manag. 15 (2), e12786. doi:10.1111/jfr3.12786
Juan, A., Gori, A., and Sebastian, A. (2020). Comparing floodplain evolution in channelized and unchannelized urban watersheds in Houston, Texas. J. Flood Risk Manag. 13 (2). doi:10.1111/jfr3.12604
Kamada, M., Nishihiro, J., and Nakamura, F. (2022). “Governance for realizing multifunctional floodplain: flood control, agriculture, and biodiversity in yolo bypass wildlife area, California, USA,” in Green infrastructure and climate change adaptation. Ecological research monographs. Editor F. Nakamura(Singapore: Springer). doi:10.1007/978-981-16-6791-6_28
Klerk, W., and Den Heijer, F. (2016). “A framework for life-cycle management of public infrastructure,” in Life-cycle of engineering systems: emphasis on sustainable civil infrastructure, proceedings of the fifth international symposium on life-cycle civil engineering (IALCCE 2016) (Delft, Netherlands: CRC Press), 16–19.
Kneifel, J., and Webb, D. (2022). “LIFE CYCLE COSTING MANUAL for the Federal Energy Management Program,” in Handbook (NIST HB). (Gaithersburg, MD: National Institute of Standards and Technology). doi:10.6028/NIST.HB.135e2022-upd1
Kumar, P., Debele, S. E., Sahani, J., Aragão, L., Barisani, F., Basu, B., et al. (2020). Towards an operationalisation of nature-based solutions for natural hazards. Sci. Total Environ. 731, 138855. doi:10.1016/j.scitotenv.2020.138855
Kurth, M. H., Ali, R., Bridges, T. S., Suedel, B. C., and Linkov, I. (2020). Evaluating resilience Co-benefits of engineering with nature projects. Front. Ecol. Evol. 8, 149. doi:10.3389/fevo.2020.00149
Lallemant, D., Hamel, P., Balbi, M., Lim, T. N., Schmitt, R., and Win, S. (2021). Nature-based solutions for flood risk reduction: a probabilistic modeling framework. One Earth 4 (9), 1310–1321. doi:10.1016/j.oneear.2021.08.010
Larrey-Lassalle, P., Armand Decker, S., Perfido, D., Naneci, S., and Rugani, B. (2022). Life cycle assessment applied to nature-based solutions: learnings, methodological challenges, and perspectives from a critical analysis of the literature. Land 11 (5), 649. doi:10.3390/land11050649
Marin-Diaz, B., van der Wal, D., Kaptein, L., Martinez-Garcia, P., Lashley, C. H., de Jong, K., et al. (2023). Using salt marshes for coastal protection: effective but hard to get where needed most. J. Appl. Ecol. 60 (7), 1286–1301. doi:10.1111/1365-2664.14413
Markovic, D., Walz, A., and Kärcher, O. (2019). Scale effects on the performance of niche-based models of freshwater fish distributions: local vs. upstream area influences. Ecol. Model. 411, 108818. doi:10.1016/j.ecolmodel.2019.108818
Melby, J. A., Thompson, E. F., Cialone, M. A., Smith, J. M., Borgman, L. E., Demirbilek, Z., et al. (2005). Life-cycle analysis of mid Bay and polar island projects. Maryland: Chesapeake Bay.
Millennium Ecosystem Assessment (2005). Ecosystem and human well-being: synthesis. Washington, D.C: Island Press. https://www.millenniumassessment.org/en/index.html (Accessed March 6, 2023).
Milly, P. C. D., Betancourt, J., Falkenmark, M., Hirsch, R. M., Kundzewicz, Z. W., Lettenmaier, D. P., et al. (2008). Stationarity is dead: whither water management? Science 319 (5863), 573–574. doi:10.1126/science.1151915
Montanari, A., and Koutsoyiannis, D. (2014). Modeling and mitigating natural hazards: stationarity is immortal. Water Resour. Res. 50 (12), 9748–9756. doi:10.1002/2014wr016092
National Institute of Standards and Technology (NIST) (2023). Building for environmental and economic sustainability (BEES) online 2.1. Available at: https://simapro.com/meet-the-developer/https://sphera.com/product-sustainability-software/https://ws680.nist.gov/bees2.
Nelson, D. R., Bledsoe, B. P., Ferreira, S., and Nibbelink, N. P. (2020). Challenges to realizing the potential of nature-based solutions. Curr. Opin. Environ. Sustain. 45, 49–55. doi:10.1016/j.cosust.2020.09.001
O’Connor, J. E., Duda, J. J., and Grant, G. E. (2015). 1000 dams down and counting. Science 348 (6234), 496–497. doi:10.1126/science.aaa9204
Parrish, K., and Chester, M. (2013). Life-cycle assessment for construction of sustainable infrastructure. Practice Periodical on Structural Design and Construction. 19 (1). doi:10.1061/(ASCE)SC.1943-5576.0000187
Palmer, M. A., Hondula, K. L., and Koch, B. J. (2014). Ecological restoration of streams and rivers: shifting strategies and shifting goals. Annu. Rev. Ecol. Evol. Sys. 45 (1), 247–269. doi:10.1146/annurev-ecolsys-120213-091935
Petit-Boix, A., Sevigné-Itoiz, E., Rojas-Gutierrez, L. A., Barbassa, A. P., Josa, A., Rieradevall, J., et al. (2017). Floods and consequential life cycle assessment: integrating flood damage into the environmental assessment of stormwater Best Management Practices. J. Clean. Prod. 162, 601–608. doi:10.1016/j.jclepro.2017.06.047
Piercy, C. D., Simm, J. D., Bridges, T. S., Hettiarachchi, M., and Lodder, Q. (2021). Chapter 5: NNBF performance. International guidelines on natural and nature-based features for flood risk management.
Poff, N. L., Brown, C. M., Grantham, T. E., Matthews, J. H., Palmer, M. A., Spence, C. M., et al. (2015). Sustainable water management under future uncertainty with eco-engineering decision scaling. Nat. Clim. Change 6 (1), 25–34. doi:10.1038/nclimate2765
Polasky, S., Tallis, H., and Reyers, B. (2015). Setting the bar: standards for ecosystem services. Proc. Natl. Acad. Sci. U.S.A. 112 (24), 7356–7361. doi:10.1073/pnas.1406490112
Pregnolato, M., Ford, A., Wilkinson, S. M., and Dawson, R. J. (2017). The impact of flooding on road transport: a depth-disruption function. Transp. Res. part D Transp. Environ. 55, 67–81. doi:10.1016/j.trd.2017.06.020
PRé Sustainability (2023). SimaPro. Available at: https://simapro.com/.
Qiu, Y., Gopalakrishnan, S., Klaiber, H. A., and Li, X. (2020). Dredging the sand commons: the economic and geophysical drivers of beach nourishment. Clim. Change 162, 363–383. doi:10.1007/s10584-020-02757-8
Quinn, T. P., Pess, G. R., Sutherland, B. J. G., Brenkman, S. J., Withler, R. E., Flynn, K., et al. (2021). Resumption of anadromy or straying? Origins of sockeye salmon in the elwha river. Tran. Am. Fish. Soc. 150 (4), 452–464. doi:10.1002/tafs.10294
Rose, K. A., and Sable, S. (2013). 2017 Coastal master plan: strategy for selecting fish modeling approaches (p. 122) (Baton Rouge, LA: Coastal Protection and Restoration Authority).
Ruangpan, L., and Vojinovic, Z. (2022). “A framework for evaluating performance of large-scale nature-based solutions to reduce hydro-meteorological risks and enhance Co-benefits,” in Advances in hydroinformatics. Editors P. Gourbesville, and G. Caignaert (Singapore: Springer Water). doi:10.1007/978-981-19-1600-7_33
Ruangpan, L., Vojinovic, Z., Di Sabatino, S., Leo, L. S., Capobianco, V., Oen, A. M., et al. (2020). Nature-based solutions for hydro-meteorological risk reduction: a state-of-the-art review of the research area. Nat. Hazards Earth Syst. Sci. 20 (1), 243–270. doi:10.5194/nhess-20-243-2020
Ruckelshaus, M., Doney, S. C., Galindo, H. M., Barry, J. P., Chan, F., Duffy, J. E., et al. (2013). Securing ocean benefits for society in the face of climate change. Mar. Policy 40, 154–159. doi:10.1016/j.marpol.2013.01.009
Ruckelshaus, M., McKenzie, E., Tallis, H., Guerry, A., Daily, G., Kareiva, P., et al. (2015). Notes from the field: lessons learned from using ecosystem service approaches to inform real-world decisions. Ecol. Econ. 115, 11–21. doi:10.1016/j.ecolecon.2013.07.009
Santoro, S., Pluchinotta, I., Pagano, A., Pengal, P., Cokan, B., and Giordano, R. (2019). Assessing stakeholders risk perception to promote Nature Based Solutions as flood protection strategies: the case of the Glinscica river (Slovenia). Sci. Total Environ. 655, 188–201. doi:10.1016/j.scitotenv.2018.11.116
Shaw, G. R., and Dundas, S. J. (2021). Socio-economic impacts of the southern flow corridor restoration project: tillamook Bay, Oregon. Garibaldi, OR: Tillamook Estuaries Partnership, 47.
Singh, H. V., Faulkner, B. R., Keeley, A. A., Freudenthal, J., and Forshay, K. J. (2018). Floodplain restoration increases hyporheic flow in the Yakima River Watershed, Washington. Ecol. Eng. 116, 110–120. doi:10.1016/j.ecoleng.2018.02.001
Smith, A. B. (2020). 2010–2019: a landmark decade of US. billion-dollar weather and climate disasters. National Oceanic and Atmospheric Administration. https://www.climate.gov/news-features/blogs/beyond-data/2010-2019-landmark-decade-us-billion-dollar-weather-and-climate.
Sommer, T. R., Harrell, W. C., and Nobriga, M. L. (2005). Habitat use and stranding risk of juvenile chinook salmon on a seasonal floodplain. N. Am. J. Fish. Manage. 25, 1493–1504. doi:10.1577/M04-208.1
Sommer, T. R., Nobriga, M. L., Harrell, W. C., Batham, W., and Kimmerer, W. J. (2001). Floodplain rearing of juvenile chinook salmon: evidence of enhanced growth and survival. Can. J. Fish. Aquat. Sci. 58 (2), 325–333. doi:10.1139/f00-245
Spence, C. M., Grantham, T., Poff, N. L., and Brown, C. M. (2015). Maximizing joint economic and ecological robustness in floodplain planning. In World Environmental and Water Resources Congress 2015. 2386–2394. doi:10.1061/9780784479162.234
Sphera (2023). Product sustainability solutions software. Available at: https://sphera.com/product-sustainability-software/.
Spyrou, C., Loupis, M., Charizopoulos, N., Apostolidou, I., Mentzafou, A., Varlas, G., et al. (2021). Evaluating nature-based solution for flood reduction in spercheios river basin under current and future climate conditions. Sustainability 13 (7), 3885. doi:10.3390/su13073885
Stroud, H. M., Kirshen, P. H., and Timmons, D. (2023). Monetary evaluation of co-benefits of nature-based flood risk reduction infrastructure to promote climate justice. Mitig. Adapt. Strategies Glob. Change 28 (1), 5. doi:10.1007/s11027-022-10037-2
ten Veldhuis, J. A. E. (2011). How the choice of flood damage metrics influences urban flood risk assessment. J. Flood Risk Manage 4 (4), 281–287. doi:10.1111/j.1753-318X.2011.01112.x
Tomsic, C. A., Granata, T. C., Murphy, R. P., and Livchak, C. J. (2007). Using a coupled eco-hydrodynamic model to predict habitat for target species following dam removal. Ecol. Eng. 30 (3), 215–230. doi:10.1016/j.ecoleng.2006.11.006
UK Environment Agency (2015). Long term costing tool for flood and coastal risk management. Available at: https://www.gov.uk/flood-and-coastal-erosion-risk-management-research-reports/long-term-costing-tool-for-flood-and-coastal-risk-management (Accessed March 6, 2023).
USACE (2015). North atlantic coast comprehensive study: resilient adaptation to increasing risk. Available at: https://www.nad.usace.army.mil/Portals/40/docs/NACCS/NACCS_main_report.pdf.
USACE (2019). Risk assessment for flood risk management studies. ER 1105-2-101. Washington, D.C: USACE Publications.
USACE (2020). Execution and documentation requirements for life cycle cost analysis. ECB 2020-8. Washington, D.C: USACE Publications.
USDOT (2019). Nature-based solutions for coastal highway resilience: an implementation guide. Available at: https://www.fhwa.dot.gov/environment/sustainability/resilience/ongoing_and_current_research/green_infrastructure/implementation_guide/fhwahep19042.pdf (Accessed March 6, 2023).
van der Jagt, A., Dorst, H., Raven, R., and Hens, R. (2017). The nature of innovation for urban sustainability. Naturvation report. Netherlands: Copernicus Institute for Sustainable Development Utrecht University.
van Rees, C. B., Naslund, L., Hernandez-Abrams, D. D., McKay, S. K., Woodson, C. B., Rosemond, A., et al. (2022). A strategic monitoring approach for learning to improve natural infrastructure. Sci. Total Environ. 832, 155078. doi:10.1016/j.scitotenv.2022.155078
van Zelst, V. T., Dijkstra, J. T., van Wesenbeeck, B. K., Eilander, D., Morris, E. P., Winsemius, H. C., et al. (2021). Cutting the costs of coastal protection by integrating vegetation in flood defences. Nat. Commun. 12 (1), 6533. doi:10.1038/s41467-021-26887-4
Vuik, V., Borsje, B. W., Willemsen, P. W., and Jonkman, S. N. (2019). Salt marshes for flood risk reduction: quantifying long-term effectiveness and life-cycle costs. Ocean Coast. Manag. 171, 96–110. doi:10.1016/j.ocecoaman.2019.01.010
Vuik, V., Van Balen, W., and van Vuren, S. (2017). Fully probabilistic assessment of safety against flooding along the Dutch coast. J. Flood risk Manag. 10 (3), 349–360. doi:10.1111/jfr3.12153
Wallbaum, H., and Ebrahimi, B. (2021). “Life cycle management of infrastructures,” in Industry, innovation and infrastructure. Encyclopedia of the UN sustainable development goals. Editors W. Leal Filho, A. M. Azul, L. Brandli, A. Lange Salvia, and T. Wall (Cham: Springer). doi:10.1007/978-3-319-95873-6_22
Weathers, H. D., and Voulgaris, G. (2013). Evaluation of beach nourishment evolution models using data from two South Carolina, USA beaches: folly beach and hunting island. J. Coast. Res. 69 (1), 84–98. doi:10.2112/SI_69_7
White, E. D., Meselhe, E., McCorquodale, A., Couvillion, B., Dong, Z., Duke-Sylvester, S. M., et al. (2016). 2017 coastal master plan: attachment C2-22 – integrated compartment model (ICM) development. Version I. Baton Rouge, Louisiana: Coastal Protection and Restoration Authority, 1–51.
White House Council on Environmental Quality (2022). Opportunities for accelerating nature-based solutions: a roadmap for climate progress, thriving nature, equity, and prosperity. Report to the national climate task force. Washington, D.C: The White House.
Whittaker, R. H., and Likens, G. E. (1975). “The Biosphere and Man,” in Primary Productivity of the Biosphere. Editors H. Lieth, and R. H. Whittaker (Heidelberg, Berlin: Ecological Studies, Springer), 14. doi:10.1007/978-3-642-80913-2_15
Willemsen, P. W., Borsje, B. W., Vuik, V., Bouma, T. J., and Hulscher, S. J. (2020). Field-based decadal wave attenuating capacity of combined tidal flats and salt marshes. Coast. Eng. 156, 103628. doi:10.1016/j.coastaleng.2019.103628
Willemsen, P. W. J. M., Smits, B. P., Borsje, B. W., Herman, P. M. J., Dijkstra, J. T., Bouma, T. J., et al. (2022). Modeling decadal salt marsh development: variability of the salt marsh edge under influence of waves and sediment availability. Water Resour. Res. 58 (1), e2020WR028962. doi:10.1029/2020WR028962
Wilson, M. A., and Carpenter, S. R. (1999). Economic valuation of freshwater ecosystem services in the United States: 1971-1997. Ecol. Appl. 9 (3), 772–783. doi:10.1890/1051-0761(1999)009[0772:evofes]2.0.co;2
Wing, O. E. J., Bates, P. D., Smith, A. M., Sampson, C. C., Johnson, K. A., Fargione, J., et al. (2018). Estimates of present and future flood risk in the conterminous United States. Environ. Res. Lett. 13 (3), 034023. doi:10.1088/1748-9326/aaac65
Wohl, E., Lane, S. N., and Wilcox, A. C. (2015). The science and practice of river restoration. Water Resour. Res. 51 (8), 5974–5997. doi:10.1002/2014wr016874
Water Research Foundation (WRF) (2023). BMP and LID Whole Life Cost Models: Version 2.0. Available at: https://www.waterrf.org/research/projects/bmp-and-lid-whole-life-cost-models-version-20.
Xu, C., Liu, Z., Chen, Z., Zhu, Y., Yin, D., Leng, L., et al. (2021). Environmental and economic benefit comparison between coupled grey-green infrastructure system and traditional grey one through a life cycle perspective. Resour. Conservation Recycl. 174, 105804. doi:10.1016/j.resconrec.2021.105804
Yang, Y., Roderick, M. L., Yang, D., Wang, Z., Ruan, F., McVicar, T., et al. (2021). Streamflow stationarity in a changing world. Environ. Res. Lett. 16 (6), 064096. doi:10.1088/1748-9326/ac08c1
Zhu, Y., Xu, C., Liu, Z., Yin, D., Jia, H., and Guan, Y. (2023). Spatial layout optimization of green infrastructure based on life-cycle multi-objective optimization algorithm and SWMM model. Resour. Conservation, Recycl. 191, 106906. doi:10.1016/j.resconrec.2023.106906
Keywords: nature-based solutions, life cycle, natural infrastructure, flood risk management, ecosystem goods and services
Citation: Kurth MH, Piercy CD, Jackson CR, Lemasson BH and Harris BD (2024) Life cycle management of natural infrastructure: assessment of state of practice and current tools. Front. Built Environ. 9:1181835. doi: 10.3389/fbuil.2023.1181835
Received: 07 March 2023; Accepted: 08 December 2023;
Published: 07 February 2024.
Edited by:
Petra Schneider, Magdeburg-Stendal University of Applied Sciences, GermanyReviewed by:
Debora Lithgow, Instituto de Ecología (INECOL), MexicoPaul Kirshen, University of Massachusetts Boston, United States
Copyright © 2024 Kurth, Piercy, Jackson, Lemasson and Harris. This is an open-access article distributed under the terms of the Creative Commons Attribution License (CC BY). The use, distribution or reproduction in other forums is permitted, provided the original author(s) and the copyright owner(s) are credited and that the original publication in this journal is cited, in accordance with accepted academic practice. No use, distribution or reproduction is permitted which does not comply with these terms.
*Correspondence: Margaret H. Kurth, bWFyZ2FyZXQuaC5rdXJ0aEB1c2FjZS5hcm15Lm1pbA==
†These authors have contributed equally to this work