- 1Hub for Biotechnology in the Built Environment, School of Architecture, Planning and Landscape, Newcastle University, Newcastle, United Kingdom
- 2Redhouse Studio, Ohio City Firehouse, Cleveland, OH, United States
- 3Moonprint Solutions, Dover, DE, United States
- 4Brown University, Earth, Environmental and Planetary Sciences, Providence, RI, United States
- 5Blue Marble Space Institute of Science, Moffett Field, CA, United States
- 6Mechanical Engineering Department, Stanford University, Stanford, CA, United States
- 7Aeronautics and Astronautics Department, Stanford University, Stanford, CA, United States
- 8Hub for Biotechnology in the Built Environment, School of Engineering, Newcastle University, Newcastle, United Kingdom
- 9Origins Institute, McMaster University, Hamilton, ON, Canada
- 10Hub for Biotechnology in the Built Environment, Department of Applied Sciences, Faculty of Health and Life Sciences, Northumbria University, Newcastle, United Kingdom
- 11NASA Ames Research Center, Moffett Field, CA, United States
A critical aspect of human space exploration and eventual settlement is the ability to construct habitats while minimizing payload mass launched from Earth. To respond to this challenge, we have proposed the use of fungal bio-composites for growing extra-terrestrial structures, directly at the destination, significantly lowering the mass of structural materials transported from Earth and minimizing the need for high mass robotic operations and infrastructure preparations. Throughout human history, the construction of habitats has used biologically produced materials, from bone and skins to wood and limestone. Traditionally, the materials are used only post-mortem. Currently, the idea of working with living biological organisms, and the phenomenon of growth itself, is of increasing interest in architecture and space applications. Here, we describe the use of mycelium-based composites as an alternative, biological approach for constructing regenerative and adaptive buildings in extrem environments and extraterrestrial habitats. It is a continuation of our research program initiated under the auspices of the “Myco-architecture Off Planet” NASA NIAC Team. These composites, which are fire-resistant, and insulating, do not consist of volatile organic compounds from petrochemical products and can be used independently or in conjunction with regolith, could employ the living biological growth in a controlled environment, for the process of material fabrication, assembly, maintenance, and repair, providing structures resilient to extra-terrestrial hazards. Here we outline the potential and challenges of using bio-composites for Earth and space applications. We describe how these might be addressed to make this biological approach feasible, providing new, growing materials for designing and building sustainable habitats, both on Earth and for long-duration space missions.
1 Introduction
To build on the Moon and Mars, there are energy-use, mass, and volume trade-offs associated with transporting materials from Earth. The cost of transportation of 1 kg of mass to Low Earth Orbit is somewhere between $2,720 and $10,000 (Jones et al., 2020). The mass and cost of the transportation of the materials are especially relevant when it comes to the construction of extraterrestrial habitats, which require structural materials, sufficient radiation protection, and infrastructure works. On top of the mass cost issue, storage, flexibility, and reliability are big concerns. Therefore, using locally available building materials and corresponding technologies which are both energy efficient and durable is one of the crucial aspects of space architecture. At the same time, on Earth, where the construction industry is a major resource consumer, the use of more resource-efficient materials and construction methods has become a major subject of research, and innovation, including legislation globally.
In the first part of the paper, we are introducing challenges associated with building off-Earth and introducing a potential solution; an alternative, biological approach for constructing habitats, both on Earth and in space. We are speculating on the concept of growing architecture and the use of Engineered Living Materials (ELMs) for the creation of living buildings. In the second part of the paper, we propose an approach toward growing architecture - different ways of using mycelium-based bio-composites. Bio-composites are materials composed of two or more distinct constituent materials where one is naturally derived (mycelium), which, combined, show improved performance over individual components (Rudin and Choi, 2013). The presented approaches for growing architecture focus on the efficient use of energy, water, and construction materials with minimum impact on the environment. The construction strategies are aiming to respond to both, global challenges of building on Earth, proposing solutions for extreme environments, and for constructing long-term habitats on the Moon and Mars. The work presented in this paper builds on our ongoing “Myco-architecture off planet” NASA Innovative Advanced Concepts (NIAC) Phase 1 and Phase 2 work, with input by the Stanford-Brown-RISD 2018 and DTU-Denmark 2018 iGEM Team. Initial work was made available as a NIAC report (Rothschild et al., 2018). In this paper we summarized the key outcomes from the architectural part of the Myco-architecture off planet project, putting it in a more specific, built environment context. Along with the new work, we are proposing sustainable construction solutions for Earth (The Mycohab project), Moon (inflatable structures), and Mars (regolith biocomposite). We are also proposing the next steps for technology development and presenting two directions of the research towards the creation of in situ grown, regenerative, and adaptive habitats.
Alternative construction strategies developed for space—in resource-limited environments which are far more extreme than on Earth—will find a direct application in construction on planet Earth, providing sustainable solutions for the terrestrial built environment. The research aims toward a healthy life cycle of the built environment, consisting of material production, construction planning, design, construction, operation, and maintenance processes. Additionally, for extraterrestrial habitats, the goal is to minimize the mass of structural materials transported from Earth and allow for a more flexible architecture at the destination, which will provide not only shelter but places that support human comfort and wellbeing.
2 State of the art—construction approaches for building habitats on the Moon and Mars
The lunar and Martian environments consist of a vacuum or very thin atmosphere. Due to the smaller gravitational force than on Earth (3.721 m/s2) the Martian atmosphere is less thick than the Earth’s one, with an atmospheric pressure of around 1% of Earth’s. However, it varies seasonally by around 30% as it freezes and unfreezes from the polar caps. This thin atmosphere protects the planet against large temperature fluctuations, but due to its pressure and composition [mostly carbon dioxide (95.5%)], it is not sufficient to sustain human life. To enable humans to live both on the Moon and Mars, highly pressurized structures are required. Due to the lack of a magnetic field and protective atmosphere, the surface of the Moon and Mars are exposed to harmful solar and cosmic radiation and micrometeorite impacts (Cohen, 2002). Therefore, in constructing a habitat, the occupants will need to be protected within buildings that are structurally substantial and offer sufficient radiation protection. There have been several different architectural concepts and construction approaches to the challenge of constructing on the Moon or Mars. The mass and cost of the transportation and construction process, together with the reliability and flexibility of the habitat play crucial roles in the feasibility and success of design concepts (Ruess et al., 2006) (Table 1).
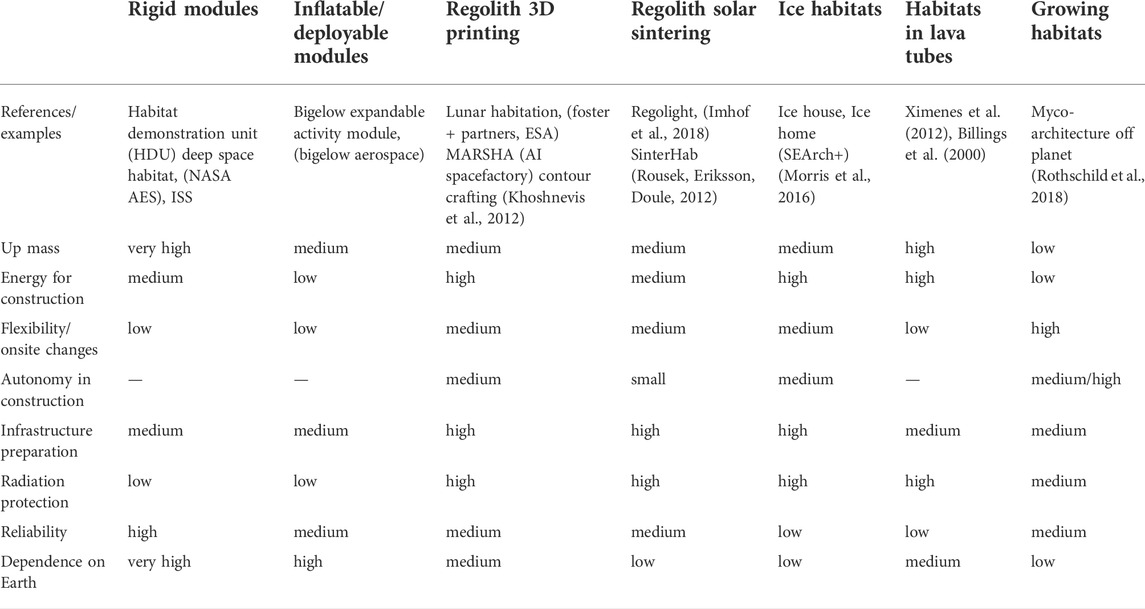
TABLE 1. Comparison of different construction strategies for lunar and Martian habitats (Billings et al., 2000; De Kastelier, 2012; Khoshnevis et al., 2012; Lyndon, 2012; Rousek et al., 2012; Ximens et al., 2012; Morris et al., 2016; Garcia, 2018; Imhof et al., 2018; Rothschild et al., 2018; AI SpaceFactory, 2021).
The first and so far the most extensively tested and lowest risk approach is to bring all habitat elements from Earth, which is in essence what the Apollo lander missions did by using the lunar module as a habitat on the surface. Similarly, the International Space Station (ISS) consists of separate modules put together, each with its own radiation protection, engineering tolerances to ensure pressurization, and installed life support systems. This method uses existing technology and could be one of the most feasible concepts for starting a Moonbase. Although this method ensures reliability, it requires bringing all of the modules from the Earth. Due to the huge energy and economic costs, the “Build it on Earth, launch it into space” approach may cause upmass and resupply problems, and minimal surface operations. Besides the energy and cost disadvantages, the reliance on Earth, in the long run, may lead to greater mission risk. The radiation and micrometeorites protection given in this example may turn out not to be enough for long-duration missions. Additionally, that approach would be even more challenging for Martian missions, where, due to the distance from Earth, the cost of transportation of materials is significantly higher (Drake, 2009a; Drake, 2009b).
The second, widely researched approach is in situ resource utilization (ISRU). For habitats, large-scale robotic operations using ice, regolith or other available or transported materials have been proposed in response to NASA’s recently concluded 3D-printed habitat challenge (Mohon, 2021). Lunar and Martian habitat concepts focusing on this approach predominately propose the utilization of the most abundant resource—regolith, by different processes such as 3D printing or solar sintering (Imhof et al., 2018). Concepts proposing regolith 3D printing suggest, for example, the use of a single multi-purpose robot for building a lunar habitat. The robot has a regolith scoop on one end which excavates the loose regolith and pours it around the dome to build the protective shell. Solar sintering is based on the use of 3D printing to build infrastructure and protective shells from regolith using the Sun as the only source of energy. The idea might be generally called Regolith Additive Construction (RAC). Contour crafting is based on robots that sinter the regolith to construct necessary infrastructure (Khoshnevis et al., 2012; Rousek et al., 2012). Solar crafting also uses robots and a balloon gantry system which sinters regolith. Another important resource that could be utilized for the construction of habitats is ice. Ice could potentially provide sufficient radiation protection (Morris et al., 2016), however, this approach uses large quantities of water which is a precious resource for other purposes including irrigation and drinking water. The consolidation process is energy intensive. Although the ISRU approach requires fewer building materials being transported from Earth, to construct habitats from in situ materials, most of the time extensive dedicated infrastructure needs to be prepared first, requiring heavy-duty robotic operations.
The aim of the presented research is to develop technology for a habitation system that would add to the palette of architectural solutions. The alternative to bringing all the materials from the Earth or using in situ resources requiring robotic operations could be the use of biomaterials - literally growing structures at destinations.
3 Life as technology—biological approach to construction
The construction of human habitats has involved the use of biologically produced materials since prehistory. We now have emerging technologies to alter living biological growth for the process of material fabrication and assembly, from tree shaping, to biologically fabricated bricks and bacteria-based self-healing concrete, to biomolecular self-assembly to create large structures from molecular building blocks (Nguyen et al., 2018). The use of advanced biomaterials and the ability to “design nature” leads to the field of material ecology, which allows for the development of hybrid materials combining multiple functions and characteristics (Gazit, 2016).
3.1 Engineered living materials for architectural applications
Biological materials found in nature are self-assembled from simple raw materials and precisely control morphology, the potential for self-healing, and the capabilities to sense and respond to the environment (Gilbert and Ellis, 2019). With the use of materials with similar capabilities—materials that continue to live and change after their final form—we can revolutionize construction approaches, both on Earth and in space. Imagine deploying a single “seed” that, similarly to a growing tree, contains all the essential information needed to grow the desired structure. The use of ELMs, which are defined by Nguyen et al. (2018) as “engineered materials composed of living cells that form or assemble the material itself or modulate the functional performance of the material in some manner”, gives potential to implement such behaviors in architectural designs. Thus, like the tree, it could be possible to grow structures capable of responding dynamically to the environment as well as maintaining and healing themselves. Replicating these beneficial characteristics of living organisms and incorporating programmed synthetic morphogenesis, may allow for the development of an autonomous construction system. Such a system could allow the fabrication, assembly, and maintenance of self-produced, functionally diverse biomaterials, for large-scale structures, in a controlled environment.
3.2 Growing architecture
One of the key features of living organisms—growth—is being used as a framework for system and policy research that will provide innovative and sustainable solutions in the field of architecture and arts. This idea of biological growth as an alternative construction method is of increasing interest in architecture and the arts and poses important questions about the role of the designer in shaping complex and emergent biological processes (Gruber and Imhof 2017; Dade-Robertson, 2021). Examples of that could be a building method where structures are created through the interaction of plant growth with technical joints called Baubotanik, vine bridges, John Krubsack’ growing chair, “Basket tree” by Axel Erlandson (Mitchell, 2006; Vallas and Courard, 2017). The approach to growing architecture is also being followed by increasing in popularity mycelium-based structures like Philip Ross’s Mycotectural Alpha concept structure composed of mycelium, on exhibit at Kunsthalle Düsseldorf in 2009, Mycotree from Block Research Group and Pascal Leboucq’s and Erik Klarenbeek’s temporary pavilion at Dutch Design Week, that is made entirely from bio-based materials (Heisel et al., 2017; Vallas and Courard, 2017). However, a phenomenon of growth at the fully architectural scale—buildings exhibiting the qualities we see in growth in nature—has been identified as a “blank spot on the landscape of biomimetic transfers” (Gruber and Imhof 2016).
In architecture and construction, the final form of a building is usually specified by a series of instructions, and most structures are only required to be functional when they are complete. The construction process is a coordinated activity with a hierarchy of management and a structured organization of information as well as utilizing many different skills and diverse knowledge. Growing architecture, in contrast, may require processes similar biological organisms. There are no blueprints representing the final structure and no simple relationship between an information source and what the final built structure will look like. A grown structure could be self-assembling in relationship to its environment and it could stay alive and be subject to constant change and modification with no fixed point of completion (Davies, 2014; Dade-Robertson, 2021).
The biological approach of growing structures directly at the destination would be less energy-intensive and leave a smaller planetary footprint than mining or melting surface material (Khoshnevis et al., 2012). The use of biomaterials could also be advantageous in enabling habitat reparations and future extensions since the material could be self-replicating. Using growth as a construction approach brings the potential for different form-making and the creation of variegated material properties.
3.3 Habitat vs. living habitation system
A usually inanimate habitat shell, could become living, participating actively in waste, recycling, oxygen production, and detoxification similar to a “living roof.” A green, or “living roof”, has a layer of soil and plants on the surface, which provides aesthetic benefits, but from a practical standpoint, this living layer decreases runoff and provides insulation. The long-term goal of creating a living habitation system is to create a system that functions beyond structure and warmth; where the organisms can be manipulated to perform tasks like self-healing, humidity regulation, energy production, nutrient production, and bioluminescence. An approach toward a living architecture was demonstrated by a five-story Bio Intelligent Quotient building in Hamburg, Germany (Perez, 2020) showing that this approach could gradually scale.
Similar to living organisms, biologically grown habitats could utilize a circulatory system to deliver nutrients to pre-seeded microbes and spores embedded within “cells” or modules. This circulatory system could be reused after the materials are fully developed to deliver nutrients and gasses for secondary processes. The habitat itself could act as an integrated bioreactor that can supply oxygen for breathing and hydrogen for fuel and radiation protection, and biomass for food or more building materials (Figure 1). The proposed bio-based concept could potentially be an all-in-one self-sustaining, living habitation system, competing with the comforts of prefabricated structures that are sent from Earth fully outfitted. Several the utilities, equipment, furnishings, and fixtures could be built directly into the expandable shell. Exploration and testing of the biocomposite’s ability to grow, together with the fabrication, processing, and tuning methods may allow for the development of a palette of materials for specific architectural uses (Haneef et al., 2017). The final goal is to be as independent as possible, to be able to stay for a long time in space without depending on supplies from Earth, and lead to permanent colonization.
4 Mycelium as a medium
Fungal Mycelium (Figure 2) represents a promising medium for growing habitation structures. Fungal mycelium is a vegetative structure of fungi consisting of branching, thread-like hyphae (Haneef et al., 2017). With the use of mycelium, the production of in situ grown biocomposites for the creation of a habitat could be possible only by sending a few fungal spores, supplemental nutrients, and a growth framework. Fabricated as a composite it can form materials that are light as a fibrous composite structure which is light and can have excellent mechanical properties, radiation protection capabilities, acting as a vapor seal, and does not outgas (Tables 2, 3). The density and material properties could be adjusted during the growth. Exploration and testing of the biocomposites’ ability to grow with combinations of organisms will allow for the development of a palette of mycelium-based composite materials for specific architectural uses (Rothschild et al., 2018). With the presence of nutrients, oxygen, and water, the mycelial building envelope could grow itself, reducing the energy required for constructing additional structures.
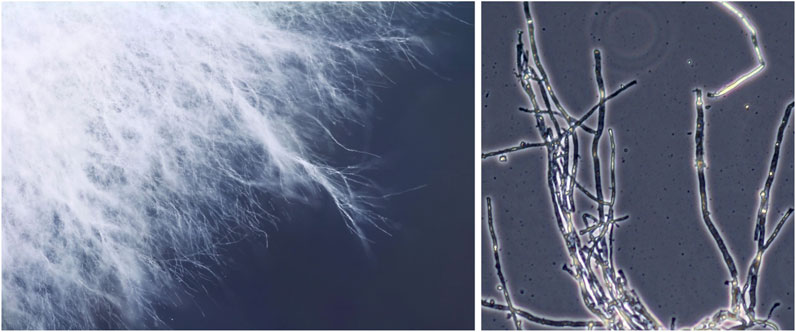
FIGURE 2. Branching mycelium structure (Brandić Lipińska) and micrograph of Ganoderma lucidum mycelia (Rothschild).
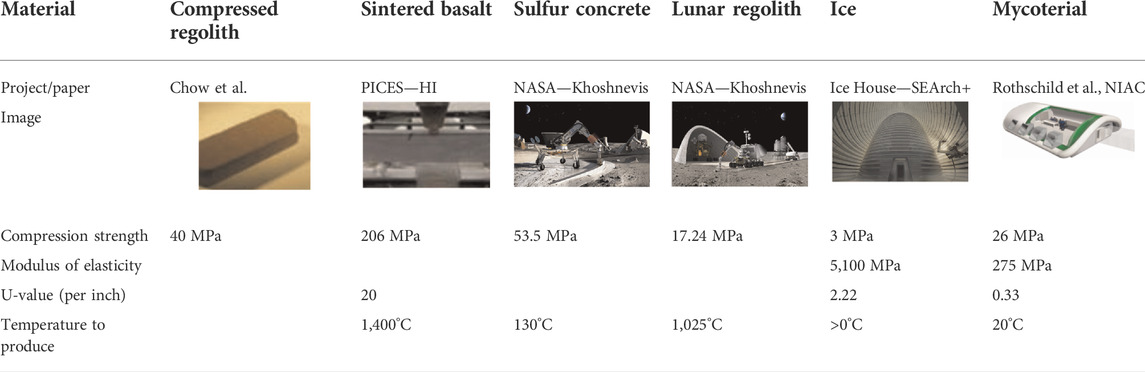
TABLE 2. Material comparison with materials under consideration for ISRU construction (Khoshnevis et al., 2012; Rothschild et al., 2018; Chow et al., 2017; Taylor and Meek, 2005; Romo et al., 2017).
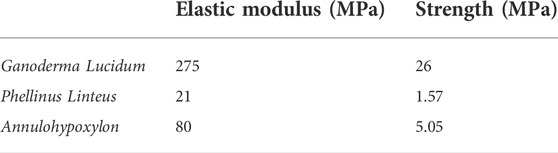
TABLE 3. Measured mechanical properties of mycelium biocomposites. The values are obtained from the curve in Figure 3. G. lucidum has the highest modulus (∼200 MPa) and highest strength (∼20 MPa), however, the results depend a lot on the size of the samples.
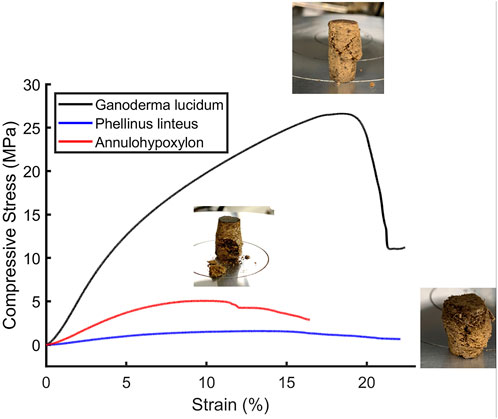
FIGURE 3. Measured mechanical properties of mycelium biocomposites. It is recommended to have all the specimens with the same dimension and at least three pieces for each kind of specimen for more accurate and reliable results in the future.
Despite the good mechanical properties of the mycelium bio-composites, due to their characteristics, it is hard to assure biocomposites’ homogeneity. Therefore, the load-bearing strength of the structure should be assessed not only through the strength of the material itself but also by the structural strength (interaction and characteristics of structural members). To ensure the structural strength of the whole structure it is important to plan, based on chosen construction strategy, the interaction between all elements (how the material is distributed, the size and shape of bio-composite components or compartments, specific pre, and post-growth conditions and treatment, etc.). Additionally, to the structural strength, the main advantages of the use of such bio-composite in extreme environments are the insulative and radiation shielding capabilities.
The material characteristics and mechanical properties presented above, together with the ability to self-multiplicate, make mycelium, and especially mycelium-based components, are great candidates for a potential solution to global challenges caused by the building industry. In the next sections, we are presenting the concepts of using mycelium for constructing in extreme environments on Earth, the Moon, and Mars.
5 Myco-architecture for extreme environments
An example of the application of mycelial structures in extreme environments is Mycohab, developed by Christopher Maurer and his architecture firm, redhouse studio, together with researchers at MIT’s Center for Bits and Atoms.
The Mycohab explores the processes of converting waste material into food and building materials using mycotecture principles. The substrate is sourced from the Acacia mellifera, or encroacher bush, that has been growing unchecked since colonial settlers fenced land for ranching and changed migration patterns of large animals. The ministry of agriculture claims there are 330 million metric tons of biomass that should be harvested every 15 years to prevent desertification of the grasslands (Smit et al., 2015). Excess encroacher bush is harvested, ground up in chippers and hammermills, soaked and bagged, pasteurized in large steamers, inoculated with mycelium spawn in labs, and placed in insulated shipping containers to grow mushrooms. Once the mushrooms are harvested and taken to market, the waste material—mycelium that has bound together with the A. mellifera dust—is put into a press that compresses the composite into a metal mold. The mold is baked in an oven, and the mycoterial (mycelium-based material) is extracted to further cure see Figure 4.
Mycoblocks made from the waste A. mellifera are 10 kg, and at 43% carbon equates to 15 kg of carbon dioxide stored. The mycoblocks have a compression strength of 5 MPa, more than the amount required for the structure of a single-story building in Namibia (Namibia Technical Services cc, 2022). They are fire-resistant and thermally insulative (internal tests).
6 Off-Earth myco-architecture
For off-Earth applications, a starting point is the creation of a composite that has structural capabilities at the same time eliminating dependence on a heavy substrate launched from Earth. In this paper, we are presenting two approaches to achieve that. The first one, initially proposed for Mars (NIAC Phase 1) and being further developed for the Moon (NIAC Phase 2), is a “standalone” approach where mycelium for the construction of habitats could be used independently, in a sealed deployable “bag” (with lightweight, deployable mesh-like, compressible scaffolding). The second approach, currently developed for Mars, is to use mycelium in conjunction with Martian regolith, reducing the amount of biomass needed to be produced (water extracted and energy used).
6.1 Myco-architecture for Moon: Standalone approach
6.1.1 Strategy: Inflatables
Inflatable structures will be used to establish the geometry of the structural elements being grown, provide the nutrient circulation system to promote the growth of the mycelium and protect the mycelium during growth. Inflatable structures provide a foundational form that has a large deployed to stowed volume ratio. This enables large structures to be packed into small volumes for launch, which in turn reduces overall launch costs. Inflatable structures also facilitate the delivery of large fully assembled structures that would not fit into a standard launch vehicle fairing as would be required with rigid structures. This is an important feature when considering long-term human habitation during space travel and the need for a habitable volume per person greater than 5 m3.
The deployable mycelium structure will have several operational stages including; 1) Stowed for Transport, 2) Deployment, 3) Growth, and 4) Structural Conversion into Operation. The inflatable support system will be a multi-layered structure with individual layers providing critical functions such as thermal protection, inflation gas containment to establish the desired geometry, and a vascular system, enabling H2O and O2 delivery, to control the growth of the mycelium. All of the materials for construction will be polymeric films, coated fabrics, and textiles similar to those used in the construction of space suits, and will be flexible in nature.
During launch and transit to the lunar or Martian surface, the inflatable mycelium structure will be constrained and vented to the ambient pressure to prevent auto-inflation from Boyle’s Law effects. During the deployment and growth phases, the system will be inflated to a low pressure (<1.0 psi) to position the mycelium structure in its desired geometry for growth. By using low-pressure inflation in comparison to inflation to operational pressure (8 psi with 100% oxygen or 14.7 psi with air), the structural mass will be conserved. This will provide considerable mass savings for the system because a lightweight structure can be used for the low-pressure growth phase. The load-carrying elements in the operational structure will be the resulting biocomposite.
The goal is to create a self-supporting structure based on the geometry of the inflatable support, with layers and channels for nutrient delivery and oxygen exchange that would facilitate the growth of mycelium and self-assembly of the biocomposite-based habitat.
6.1.2 Mycelium growth
The inflatable shell, which acts as a vapor barrier, is a multi-hulled structure that contains the inflation gas on its interior and supports cavities where the mycelium grows. After deployment, the cavities supporting the mycelium are dampened with extracted water. The water spreads within the cavities through an in-built vascular system in the inflatable structure. Next, the cavities are heated, to activate growth. The cyanobacteria or algae will start growing and releasing oxygen and sugar which would be consumed by fungal mycelia. After the period of growth, the grown mycoshell would great an insulated structure (Figure 5). Alternatively, the fungi could live off dry algae or other lightweight nutrient sources (Rothschild et al., 2018).
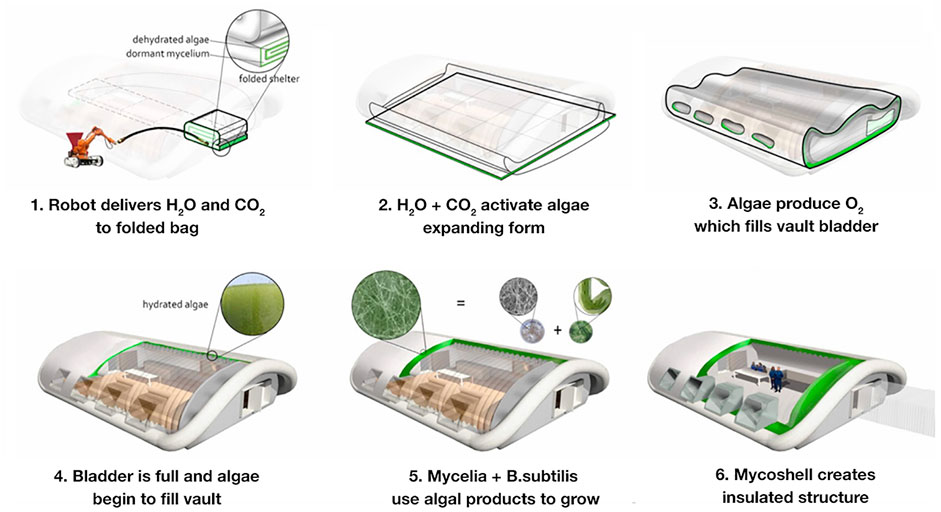
FIGURE 5. Fully encased inflatable structures self-assemble when water, CO2, and heat are added by a robot, rehouse studio.
To enable three-dimensional growth within the mycelium cavities, at the same time eliminating the dependence on a heavy substrate, commonly used in mycelium biocomposites (e.g., wood chips or sawdust), we propose to introduce a lightweight, compressible, porous scaffold that mycelium can use during growth (Figure 6). The exact design of a scaffolding structure, its form, geometry, size of the cells, and the material are yet to be defined. Initial tests were conducted on paper origami foldable structures, however, ultimately a 3D printed flexible polymeric lattice with a much finer pore size (1–5 mm) and more durable material will be tested.

FIGURE 6. Scaffoldings. Example of lattice from Solus printer available in Rothschild’s lab, mycelium growing on paper-based origami scaffold coated with nutrients and agar.
To ensure proper nutrient supply to promote fungal growth, the scaffolding structure could be coated with a nutrient-rich hydrogel. The mesh size of hydrogels can be engineered to store nutrients and deliver them in a sustained fashion through mass diffusion (Axpe et al., 2019). Growing mycelium would “climb” on, and solidify the scaffolding, binding it all together, so the whole structure becomes rigid.
Another idea for creating scaffolding for mycelium growth is the use of drop-stitch technology (Figure 7). Drop-stitch is a technology used to produce inflatable drift boats, floating docks, high-pressure rescue lifting bags, and airplane wings. The basic principle behind it is joining two pieces of any kind of fabric with thousands of fine thread lengths. Due to the utilization of fine threads along with the structure, this technology enables the forming of flat-surface inflatables with high rigidity. Similarly, a multilayered habitation system could be created utilizing drop-stitch inflatables. The bioreaction would occur in the cavity and biomass would fuse with stitches.
In the standalone approach, the growth of mycelium will be tested in the developed inflatables and drop-stitch cells. There is ongoing research on optimizing mycelium for strength and radioprotection (melanin and lipid production). We test materials for strength, UV protection, and melanin content (Raman spectroscopy). The next step is to develop “cells” that grow biomass in situ and build a scale model of the building that grows in situ.
The opportunities associated with this approach are the reduction of the mass of structural materials which must be brought from Earth, together with a reduction of infrastructure preparations and heavy-duty robotic operations. The inflatable bag protects from potential forward and backward contamination. Several utilities, equipment, furnishings, and fixtures can be built directly into the expandable shell, competing with the comforts of up-massed prefabricated structures that come fully outfitted. On the other hand, in the approach, all of the mass has to be produced in situ (biomass) or brought from Earth (scaffolding). In situ production of biomass would also require significant quantities of water for plant or algae growth and nutrients for mycelium growth. Using only an inflatable shell with the mycoshell grown inside may not be sufficient to protect humans against radiation. A layer of regolith may be needed on top of the structure to provide radiation protection and to “hold” the structure due to the pressure differences between the outside and inside of the habitat.
6.2 Myco-architecture for Mars: Regolith biocomposite
6.2.1 Strategy: Reinforcement
A second concept is an approach in alignment with ISRU. Dependence on the in situ resources on Mars is even more critical than in the case of the Moon missions. That is due to the distance from Earth, making it impossible to rely on from-Earth deliveries. In that context, everything becomes a resource—structural materials, water, O2 and CO2, sunlight, but also energy—work that needs to be done to extract water, heat the structure, or assemble the habitat. Therefore, this approach is focusing on minimizing the biomass that needs to be produced to enable mycelium growth and instead is proposing the use of regolith (loose unconsolidated rock and dust atop a layer of bedrock) as the main structural mass of the habitat. Mycelium in this approach acts as a binder enabling consolidation of the regolith. In the same way, as steel is held together by molecular bonding and concrete is held by a binder, soil strength depends on internal friction between the soil particles themselves. However, any kind of loose soil, whether it is sand, Earth, or regolith, is not strong enough to act as a construction material itself. Therefore, following the principles of geotechnical engineering, the mycelium, grown on Martian regolith (Figure 8) with minimal addition of (in situ produced) biomass and/or nutrients could act as a binder, ensuring the stability of the regolith and enabling the construction of inhabitable surface structures (Kuriakose and van Beek, 2011).
With the use of such a biological approach to reinforce the regolith mass it will be possible to build a stable structure with a smaller amount of biomass, water, and oxygen used, compared with the standalone approach. The regolith would also potentially provide sufficient radiation protection, and mass needed to hold the pressure of the habitat, caused by the pressure differences (inside and outside environment).
6.2.2 Mycelium growth
With the initial experiments of growing mycelium (Ganoderma lucidium) on the silica-based sand in Petri dishes and Petri dish-based columns, we demonstrated that mycelium could act as a binder of the inorganic soil (Figure 8). The following experiments are aiming to scale up the elements and move toward the scale of inhabitable structures. We are also planning to perform a series of experiments (compressive, tensile, and flexural strength, drilling resistance, dynamic modulus of elasticity) to understand the mechanical properties of achieved components. In further research, the grade of the soil and chemical composition of the Martian regolith will be taken under consideration, together with different sources of biomass that could potentially be grown in situ in Martian greenhouses. The goal is to explore mycelium biocomposites in resource-limited conditions and understand how mycelium grows on sand, and if it needs extra nutrients. This research aims to understand the minimum amount of biomass is to enable mycelium growth on inorganic soil (sand or Martian regolith) to bind the soil together, progressing the field of mycelium biocomposites. In the later stages of the research, it will be interesting to study the behavior of the soils in lower gravity conditions, the compaction of the regolith in the lower gravity conditions, and how that may affect the growth of mycelium.
The opportunities associated with this approach are radical reduction of the mass of structural materials which must be brought from Earth and a smaller amount of biomass (and/or nutrients) that needs to be produced, which also means that less water and energy are needed. Due to the mass or regolith, the structure would hold internal pressure and would provide sufficient radiation protection. A lower gravity environment may enable the creation of taller structures (smaller compaction on the bottom of the structure) reducing the planetary footprint.
6.3 Environmental testing
Material properties of mycelium-based bio-components under lunar or Martian environmental conditions haven’t been fully explored. To understand the biocomposites’ behavior in the space conditions (even if grown in a closed environment), it is necessary to conduct tests for UV and ionizing radiation, low temperatures, pressures, wind, abrasion, and other environmental factors different due to the terrestrial forces. It would be helpful to test how the mycelium grows at different pressures and temperatures to find the optimal growth conditions for the implementation in the growth chamber. Performing the environmental tests will be necessary to give an assessment of how the developed mycelial biocomposites will behave in the lunar and Martian conditions. This information would be crucial in order to enhance space technology readiness levels (NASA, 2012) levels. The other, more complicated to test, aspect, which could significantly affect mycelium growth is the low gravity environment.
For the environmental testing, we test samples in the Planet Simulator (Angstroem Engineering, Kitchener, Ontario, Canada), a custom-built simulation chamber in McMaster University’s Origins of Life Laboratory. The simulator is shown in Figure 9. The simulator can control temperature (−30 to 130°C), humidity (0%–100% RH), atmospheric composition, radiation (155–1,000 nm), and pressure (0.8–760 Torr). Four different gas lines can be connected and mixed to control the atmosphere inside with a composition precision of better than 0.1 vol%. UVC and UVB radiation is generated by light bulbs with an intensity of 30 W/m2 LED arrays producing radiation in the UVA, visible, infrared, and far-infrared range with intensities of up to 300 W/m2. Humid atmospheres are created by bubbling part of the gas through a water reservoir and carefully controlling the amount of “dry” and “wet” gas that is injected back into the chamber. The Planet Simulator can intake multi-step recipes controlling these parameters, which can be looped to repeat steps. The system can be cycled from the highest temperature (130°C) to the lowest temperature (30°C) in about 1 h. The combination of thermal cycling and alternating UV radiation levels (and potentially changes in humidity) can lead to significant fatigue in materials such that the simulator provides a sophisticated and important prototype testing environment.
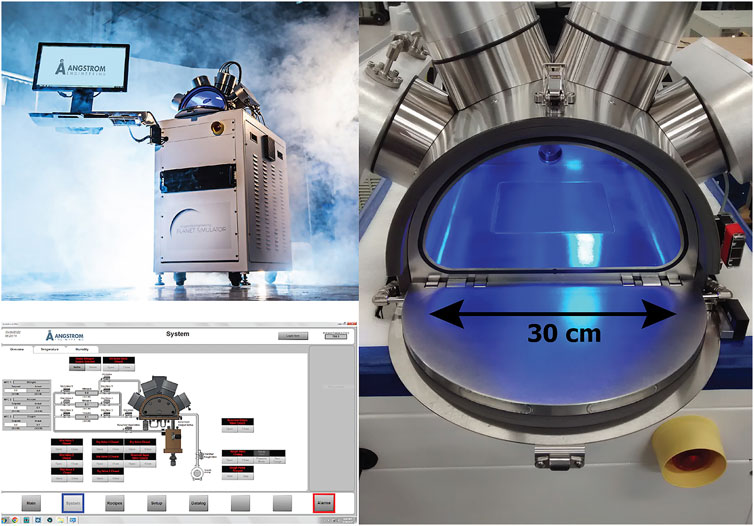
FIGURE 9. Prototypes will be tested in the Planet Simulator, an environmental chamber of about 0.5 ft3 that can control temperature, pressure, atmosphere, humidity, and radiation. Computer control allows complex simulations including cycling of parameters.
7 The future potential of mycelium use in the built environment on and off Earth
The long-term goal of the idea of utilizing mycelium for space application is to create a biocomposite that has more functions than just providing structural support and insulation for the habitat. In nature, fungal mycelia are a vital part of ecosystems for their role in decomposing organic compounds. They digest nutrients by secreting enzymes that break down materials for uptake into their cells. The same function, which is a primary role of mycelium, could be used in space habitats. Mycelia could be used to process waste from the habitat, e.g., non-edible parts of the plants from the greenhouse, or feedstock of mission-produced organic waste streams (human waste) into fertilized soil or into the structural material. However, with the use of bio-engineering, it could be possible to go way beyond the primary role of mycelium. Fungal mycelium is an extremely versatile material and may have the potential to be genetically engineered to enhance its properties or enable the primary production of other vital materials (Waters et al., 2014) Biological processes could be utilized for example to provide radiation protection (Figure 10). An example of that is the utilization of radiosynthesis. Cryptococcus neoformans can survive simulated Martian conditions (Onofri et al., 2015) and are able to shield from the radiation, twice as effective as charcoal (Dadachova et al., 2007). Melanin pigments play a crucial role in the survival of fungi when exposed to radiation. Melanin-rich fungi can absorb radioactivity (Dadachova et al., 2007). Suggesting that melanized fungal mycelia could provide radiation protection. Melanized black yeast, and some black fungi, not only survive but also benefit from exposure to ionizing radiation (Dadachova et al., 2007; Robertson et al., 2012). It could be possible to supplement the mycelium-composites with either genetically engineered mycelia that bind materials such as metals or with bacteria to enhance radiation protection capabilities.
The organisms could be bioengineered to be able to self-heal, regulate the humidity levels, produce energy, light, and nutrients (Rothschild et al., 2018) provide building ventilation and control. The biological functions that enable the growth of the materials also bestow such benefits as waste degradation, oxygen production, and heat and electricity generation (Waters et al., 2014) and could be implemented within the environmentally controlled life-support systems within the habitat (Cohen et al., 2012) or provide radiation protection (Dadachova et al., 2007) (Figure 11).
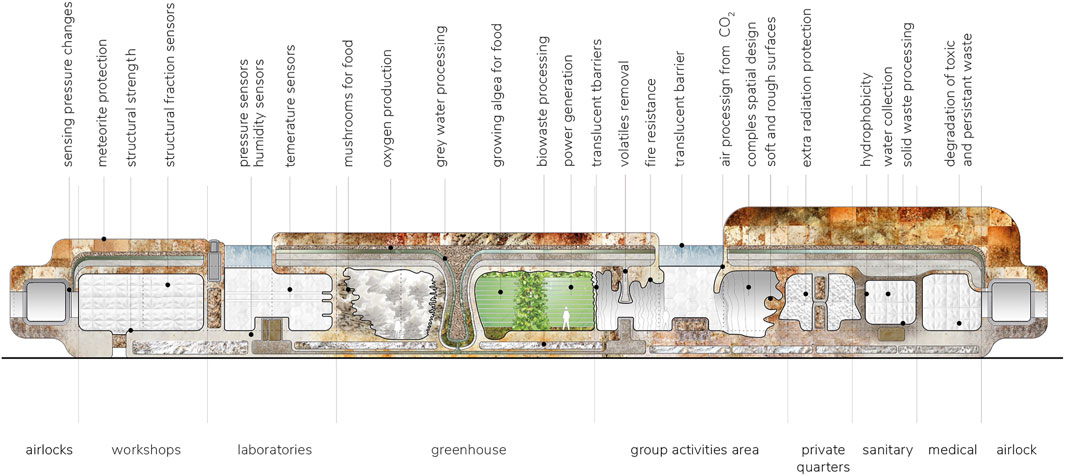
FIGURE 11. Variegated material properties and different functions of mycelium biocomposite in a space habitat.
Living mycelium within the biocomposites, with its biological capability to sporulate and regenerate, in case of any damage would start repairing micro-cracks, preventing more critical stages of damage. In theory, the self-healing of the structure would provide the ability to repair the structure without the need for any manual repair. There are limitations to the self-healing ability (e.g., the size of the crack), however, with the ongoing developments in this area (Elsacker et al., 2021; Luo et al., 2018) we can assume that this ability implemented in space habitats would significantly lower the maintenance costs and mission risks associated with habitat repairs. Self-replicating materials would also allow for any habitat repairs where more material is needed (the growth can be manually restarted) and future habitat extensions. The utilization of mycelium-based biocomposites could also lead to the creation of the living interface between architecture and digital technologies, exploring the possibilities of responsive environments. Such physical spaces, enriched by intelligent inputs, providing the ability to receive, process, and respond to information would enable varied spatial experience and interaction with the user, which is significant in space environments (Adamatzky et al., 2019; Adamatzky et al., 2021). This may mean incorporating other organisms with mycelium. For example, mycelium can live in symbiosis with bacteria such as Bacillus subtilis. This bacterium is genetically tractable and could be programmed as an integral intelligent input (biosensor). For example, mycelium can live in symbiosis with bacteria such as B. subtilis. Mycelium strain engineered with B. subtilis could sense oxygen and pressure, which will produce a color change when oxygen concentrations are low (Rothschild et al., 2018). This ability can be linked to a specific receiver, to monitor oxygen concentrations and pressure changes in the habitat. Mycelium composites produced through co-culture of fungus and B. subtilis could be also engineered to digitally report on excess stress and load in the shell, or lack of pressure and load, which could potentially relate to failures in the shell’s structural integrity (Waters et al., 2014).
The organic nature of mycelium-based materials and the ability to fine-tune the tactile environment should additionally aid in the psychological comfort of the mission participants (Arias and Otto, 2013) e.g., mycofoam made out of mycelium is similar in function to Styrofoam and it can be stained to look like wood (Rothschild et al., 2018). Through the creation of mycelium-based components, variegated not only in properties and functions but also simply in sizes and shapes, buildings would gain quality of multi-sensory experience enabling a translation of complexity and variety from nature to the built environment (Franco et al., 2017). This aspect is especially important for long-duration space missions, where haptic sense among astronauts is dampened due to a lower gravity environment, and haptic sensory inputs are crucial for astronauts’ psychological comfort.
8 Challenges of the mycelium use in the built environment on and off Earth
Mycelium, in order to grow, need oxygen, water, and a source of nutrients. Although water is a resource present, both on the Moon and Mars, its extraction is a very energy-consuming process, and it is an extremely valuable and precious resource. It is for sure, that once humans will be establishing a base on one of these celestial bodies, there will also be a water extraction system in place, however, the primary use of it will be for sustaining human life, and not for constructing habitats. The same holds for oxygen production, which is essential for human survival. Apart from sourcing biomass, one of the biggest challenges when working with biological materials for space applications is the need to respond to planetary protection requirements for robotic or human missions and comply with the current Committee on Space Research (COSPAR) human mission principles and guidelines. The level of restrictions depends on the location. The Moon is in Category II, which means that the requirements ask only for simple documentation: a short planetary protection plan, primarily to outline intended or potential impact targets, brief pre-launch and post-launch analyses detailing impact strategies, and a post-encounter and end-of-mission report providing the location of inadvertent impact, if such an event occurs. Mars, on the other hand, is in Category IV which means detailed documentation is required including a probability of contamination analysis, a bioassay to enumerate the bioburden, an inventory of the bulk constituent organics, and an increased number of implementing procedures (trajectory biasing, cleanrooms, bioburden reduction, partial sterilization of the direct contact hardware and a bioshield for that hardware) (Kminek et al., 2017).
Martian soil contains a perchlorate (ClO4−)—a chemical compound containing the perchlorate ion, at concentrations between 0.5% and 1%. At such concentrations, perchlorate could be an important source of oxygen, but it could also become a critical chemical hazard to astronauts (Davila et al., 2013). The soil toxicity could also affect the growth of any biological organisms, weather plants for a greenhouse, or mycelium for structural purposes. There are mitigation technologies originating from the mining industry, that could potentially be applied to Mars to reduce soil toxicity. One of the strategies is proposing biochemical systems that decompose ClO−4 into Cl– and O2. This way, mitigation of ClO−4 toxicity could be coupled to in situ resource utilization, providing not only oxygen but also de-toxified regolith that could be used in construction (Davila et al., 2013).
On Earth, building with mycelium is also vulnerable to contamination. In the same way, as in the case of space planetary protection, using biological materials on Earth, there is a risk of forward and backward contamination. In most cases, mycelium-based bio-components are grown in a sterile environment, baked or dried, and only assembled where they are envisioned for. However, there is a growing number of projects exploring mycelium growth, brick greeting, etc., in the non-sterile environment. The other, especially Earth-based, challenge associated with building with biological materials, and especially mycelium-based bio-components, is its life span. Mycelium-based structures are biodegradable, which adds to their sustainability aspects, however, it also means the life span of the structure is limited. When maintained in favorable and stable conditions it can have a lifespan of approximately 20 years. However, when it is in contact with the ground it may start decomposing already after around 6 weeks.
9 Discussion
The main constraint of using mycelium for the construction of extraterrestrial habitats, is to develop a construction strategy that utilizes as little brought-from-Earth materials as possible, as little energy as possible and gives maximum reliability and flexibility. The same rules may be applied to architecture and construction systems on Earth. The development of a biological material system that utilizes only in situ materials, whether on or off Earth, will contribute to future development both, in space architecture and in sustainable construction technologies on Earth.
Currently, the building industry is responsible for 40% of Earth’s carbon emissions, and with the global production of material reaching 180 billion tonnes in 2050, the manufacturing of material will be responsible for almost 60% of global CO2 emissions (Lu et al., 2020). Therefore, reducing the carbon footprint is a key aim of the construction industry. Using a low-energy, carbon-sequestering bio-fabrication method, to upcycle by-products and waste into low-cost biodegradable material could potentially reduce the use of fossil fuel-dependent materials (Jones et al., 2020).
The concepts of growing architecture and using mycelium-based materials are in alignment with UN Sustainable Development Goals (SDGs) More speculative, living buildings: rapidly deployable, growing, and self-healing structures, potentially even with embedded biosensors, respond to the UN SDG 9 (Industry, Innovation, and Infrastructure). Biodegradable, carbon-neutral mycelium-based structures respond to UN SDG 11 (Sustainable Cities and Communities) and UN SDG 12 (Responsible Consumption and Production) (United Nations, 2018). Combining ideologies of the circular economy (considering all energy and sources of impact) and human-centered design (creating spaces responsive to the users), and using biotechnology to create sustainable, adaptable construction system, resembling ecosystems (to increase resource efficiency and create cyclic resource loops) (Benyus, 2002) this research aims to respond to the need for a more holistic approach to building design.
The use of mycelium-based components has already been proposed as an alternative for synthetic construction materials such as acoustic and thermal insulation, paneling, flooring, etc., (Jones et al., 2020). However, there are still many technological gaps that will need to be identified. What makes the research in this area more difficult is that there is no standardized method of testing mycelium-based components. Therefore, it is hard to replicate outcomes and compare results. To move towards growing architecture, and mycelium-based structures at a fully building scale, we need to research different mycelium species, growth conditions, enhancements and post-processing methods, design of growth containers, shells, scaffoldings, integrity during deployment (environmental damage), systems integration for habitation (heat, power, light, etc.), end-of-lifetime (re) use.
10 Conclusion
This research explores the potential and challenges of using mycelium-based biocomposites for Earth and space applications. The presented approach of using biological growth for the off-Earth construction, similarly to other researched ISRU-based approaches aims to lower the mass of materials needed to be transported from Earth. Additionally, it focuses on lowering the energetic costs of the construction of habitat in situ (work needed to assemble the habitat). In the long-term using biological materials and growth as a construction method, bring the potential of ELMs, providing supplementary capabilities like sensing and responding to environmental stimuli, self-healing, etc., making the habits more flexible and reliable.
The further development of the research on ELMs and mycelium-biocomposites will allow for advancements in the field of biotechnology in the built environment, providing functionally graded, bio-composites for the construction of regenerative and adaptive habitats. The concepts employ living biological growth in a controlled environment for the process of material fabrication, assembly, and maintenance. Features include the modest upmass requirements of a few spores, nutrition for mycelial growth, and a growth framework, along with the potential to reproduce using in situ resources, the ability to grow to accommodate on-site terrain, and the control granted by the tunability of the materials.
There are myriad possibilities for mycotecture on and off-planet, however, the research is still in an early stage. Once the enabling technologies are identified, a technology roadmap and recommendations for further development could be generated, allowing for the feasible implementation of this approach, for growing sustainable buildings on Earth and extra-terrestrial habitats.
Data availability statement
The raw data supporting the conclusion of this article will be made available by the authors, without undue reservation.
Author contributions
MBL organized the paper structure, and has written the introduction, 2–4, 6–8, discussion and conclusions, prepared diagrams for Figures 10–12, and performed sand column experiments. LR and CM conceived the Myco-architecture Off Planet project. LR is a PI of the NASA Myco-architecutre research and contributed to the introduction, 2-8 discussion, and conclusions. CM has written 5 and contributed to 6.1 and is responsible for Mycohab and Myco-Architecture for Moon designs. MDR is supervising the project (Myco-Architecture for Mars: Regolith biocomposite), revised text, and contributed to the introduction, 2-8, discussion, and conclusions. DC has written 6.1.1 Strategy: Inflatables. MR has written the 6.3 Environmental testing part. CL and DS have conducted mechanical testing of mycelium biocomposites. JH, IL has revised the text. RM, MT, and MZ are supervising the project (Myco-Architecture for Mars: Regolith biocomposite and revised the text.
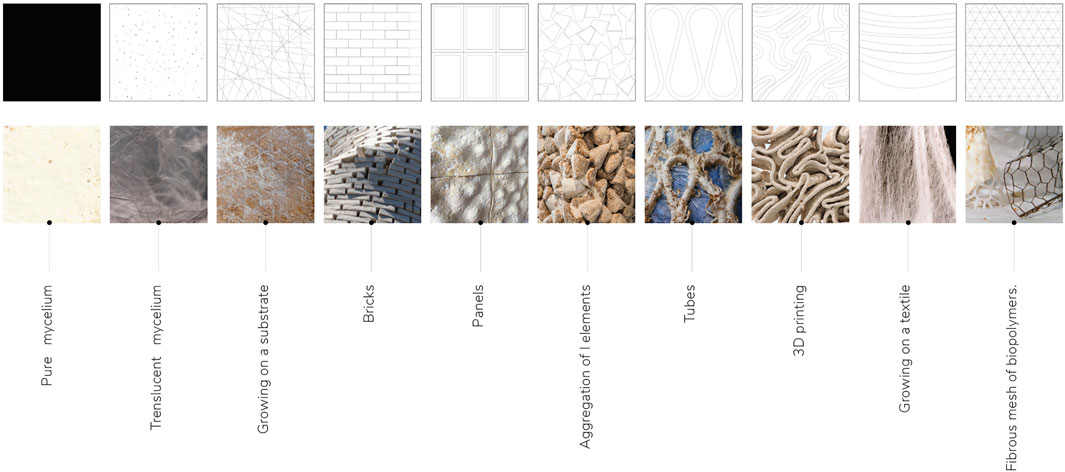
FIGURE 12. Variety of shapes and forms of mycelium-based components (growing on a substrate, panels—mogu; bricks, aggregation of elements—David Benjamin; tubes—Astudio, Aleksi Vesaluoma; 3d printing—Goidea et al., 2020, growing on textile—Jane Scott, fibrous mesh of biopolymers—cultivated).
Funding
This research is partially funded by The NASA Innovative Advanced Concepts (NIAC) Program Phase 1 and Phase 2, and partially by Northern Bridge Consortium and Research England, and the Research England fuded Hub for Biotechnology in the Built Environment (HBBE).
Acknowledgments
We are grateful to the whole “Myco-Architecture Off Planet” NIAC Phase I and Phase II teams and the NIAC program for funding, and Stanford-Brown and DTU iGEM teams. This research is supported by the research group Bio-Futures for Transplanetary Habitats.
Conflict of interest
The authors declare that the research was conducted in the absence of any commercial or financial relationships that could be construed as a potential conflict of interest.
Publisher’s note
All claims expressed in this article are solely those of the authors and do not necessarily represent those of their affiliated organizations, or those of the publisher, the editors and the reviewers. Any product that may be evaluated in this article, or claim that may be made by its manufacturer, is not guaranteed or endorsed by the publisher.
References
Adamatzky, A., Ayres, P., Belotti, G., and Wosten, H. (2019). Fungal architecture. arXiv. doi:10.48550/arXiv.1912.13262
Adamatzky, A., Gandia, A., and Chiolerio, A. (2021). Towards fungal sensing skin. Fungal Biol. Biotechnol. 8 (1), 6. doi:10.1186/s40694-021-00113-8
AI SpaceFactory (2021). MARSHA AI SPACEFACTORY'S Mars habitat. Available at: https://www.aispacefactory.com/marsha (Accessed July 7, 2021).
Arias, D., and Otto, C. (2013). Defining the scope of sensory deprivation for long duration space missions. NTRS-NASA Technical Reports.
Axpe, E., Chan, D., Offeddu, G. S., Chang, Y., Merida, D., Hernandez, H. L., et al. (2019). A multiscale model for solute diffusion in hydrogels. Macromolecules 52 (18), 6889–6897. doi:10.1021/acs.macromol.9b00753
Billings, T. L., Walden, B., and York, C. L. (2000). “Lunar lavatube base construction” in Seventh International Conference and Exposition on Engineering, Construction, Operations, and Business in Space. Albuquerque, New Mexico, US. February 27–March 2, 2000 (Reston, VA: ASCE Library).
Chow, B. J., Chen, T., Zhong, Y., and Qiao, Y. (2017). Direct formation of structural components using a martian soil simulant. Sci. Rep. 7 (1), 1151–1158. doi:10.1038/s41598-017-01157-w
Cohen, M. M., Flynn, M. T., and Matossian, R. L., (2012). Water walls architecture: Massively redundant and highly reliable life support for long duration exploration missions. Global Space Exploration Conference. Washington DC, USA. May 22–24, 2012, [online] pp.1–14.
Cohen, M. M., Selected prospects in lunar architecture, IAC-02-Q.4.3.08, 53rd International Astronautical Congress. Houston, Texas, 2002, 10-19 October.
Dadachova, E., Bryan, R. A., Huang, X., Moadel, T., Schweitzer, A., Aisen, P., et al. (2007). Ionizing radiation changes the electronic properties of melanin and enhances the growth of melanized fungi. PLoS ONE 2, e457. doi:10.1371/journal.pone.0000457
Davies, J. A. (2014). Life Unfolding: How the human body creates itself. Oxford, United Kingdom: Oxford University Press.
Davila, A. F., Willson, D., Coates, J. D., and McKay, C. P. (2013). Perchlorate on Mars: A chemical hazard and a resource for humans. Int. J. Astrobiol. 12 (4), 321–325. doi:10.1017/s1473550413000189
De Kestelier, X. (2012). Lunar habitation. Available at: https://www.fosterandpartners.com/projects/lunar-habitation/(Accessed July 7, 2021).
Drake, B. G. (2009a). Human exploration of Mars design reference architecture 5.0. Washington D.C., US: NASA.
Drake, B. G. (2009b). Human exploration of Mars design reference architecture 5.0. Washington D.C., US: NASA.
DTU-Denmark (2018). Fungal building materials for extreme environments. Available at: http://2018.igem.org/Team:DTU-Denmark (Accessed April 8, 2021).
Elsacker, E., Søndergaard, A., Van Wylick, A., Peeters, E., and De Laet, L. (2021). Growing living and multifunctional mycelium composites for large-scale formwork applications using robotic abrasive wire-cutting. Constr. Build. Mater. 283, 122732. doi:10.1016/j.conbuildmat.2021.122732
Franco, L. S., Shanahan, D. F., and Fuller, R. A. (2017). A review of the benefits of nature experiences: More than meets the eye. Int. J. Environ. Res. Public Health 14 (8), 864–927. doi:10.3390/ijerph14080864
Garcia, M. (2018). International space station, bigelow expandable activity module (BEAM). Available at: https://www.nasa.gov/mission_pages/station/structure/elements/bigelow-expandable-activity-module.html (Accessed July 7, 2021).
Gazit, M. (2016). Living matter: Biomaterials for design and architecture. Cambridge, Massachusetts, United States: MIT. Master’s Thesis.
Gilbert, C., and Ellis, T. (2019). Biological engineered living materials: Growing functional materials with genetically programmable properties. ACS Synth. Biol. 8 (1), 1–15. online. doi:10.1021/acssynbio.8b00423
Goidea, A., Andreen, D., and Dimitrios, F. (2020). “Pulp faction: 3d printed material assemblies through microbial biotransformation” in Fabricate (conference). London.
Gruber, P., and Imhof, B. (2016). Built to grow: Blending architecture and biology. Vienna: Walter de Gruyter.
Gruber, P., and Imhof, B. (2017). Patterns of growth-biomimetics and architectural design. Buildings 7, 32. doi:10.3390/buildings7020032
Haneef, M., Ceseracciu, L., Canale, C., Bayer, I. S., Heredia-Guerrero, J. A., and Athanassiou, A. (2017). Advanced materials from fungal mycelium: Fabrication and tuning of physical properties. Scientific Reports, 7, 41292. doi:10.1038/srep41292
Heisel, F., Schlesier, K., Lee, J., Rippmann, M., Saeidi, N., Javadian, A., et al. Design of a load-bearing mycelium structure through informed structural engineering. Proceeding of the World Congress on Sustainable Technologies (WCST), Cambridge, UK, 2017, pp.1–5.
Imhof, B., Sperl, M., Urbina, D. A., Weiss, P., Preisinger, C., Waclavicek, R., et al. Using solar sintering to build infrastructure on the moon latest advancements in the regolight project, 2018, IAC-18.E5.1. X47746, 69th International Astronautical Congress, Bremen, Germany, 2018 1-5 October.
Jones, M., Mautner, A., Luenco, S., Bismarck, A., and John, S. (2020). Engineered mycelium composite construction materials from fungal biorefineries: A critical review. Mat. Des. 187, 108397. doi:10.1016/j.matdes.2019.108397
Khoshnevis, B., Carlson, N., and Thangavelu, M., (2012). Contour crafting simulation plan for lunar settlement infrastructure build-up, Thirteenth ASCE Aerospace Division Conference on Engineering, Science, Construction, and Operations in Challenging Environments, and the 5th NASA/ASCE Workshop On Granular Materials in Space Exploration. Pasadena, United States. April 15–18, 2012.
Kminek, G., Conley, C., Hipkin, V., and Yano, H. (2017). COSPAR’s planetary protection policy. Paris, France: Committee on Space Research.
Kuriakose, S. L., and van Beek, L. P. H. (2011). “Plant root strength and slope stability,” in Encyclopedia of agrophysics. Encyclopedia of earth sciences series. Editors J. Gliński, J. Horabik, and J. Lipiec (Dordrecht: Springer).
Lu, W., Tam, V. W. Y., Chen, H., and Du, L. (2020). A holistic review of research on carbon emissions of green building construction industry. Eng. Constr. Archit. Manag. 27 (5), 1065–1092. doi:10.1108/ecam-06-2019-0283
Luo, J., Chen, X., Crump, J., Zhou, H., Davies, D. G., Zhou, G., et al. (2018). Interactions of fungi with concrete: Significant importance for bio-based self-healing concrete. Constr. Build. Mater. 164, 275–285. doi:10.1016/j.conbuildmat.2017.12.233
Lyndon, B. (2012). NASA facts: Habitat demonstration unit - deep space habitat. Houston, Texas: August Johnson Space Center. Available at: https://www.nasa.gov/pdf/468441main_HDU_FactSheet_508.pdf (Accessed July 7, 2021).
Mitchell, J. W. (2006). Ecotransology integrated design for urban mobililt (PhD). Cambridge, U.S.A: Massachusetts Institute of Technology.
Mohon, L. (2021). Nasa stmd: Centennial challenges. Available at: https://www.nasa.gov/directorates/spacetech/centennial_challenges/3DPHab/index.html (Accessed June 10, 2021).
Morris, M., Ciardullo, C., Lets, K., Yashar, M., Montes, J., Rudakevych, O., et al. Martian ice habitats: Approaches to additive manufacturing with H20 beyond Mars ice house, AIAA 2016-5528, AIAA SPACE, Long Beach, California, 2016, 13-16 September.
Namibia Technical Services cc (2022). Summary of tests on cement bricks. Available at: https://www.ntscc.com/testing.html Accessed June 6, 2022).
NASA (2021). Technology readiness level definitions. Available at: https://www.nasa.gov/pdf/458490main_TRL_Definitions.pdf (Accessed September 13, 2021).
Nguyen, P. Q., Courchesne, N. M. D., Duraj-Thatte, A., Praveschotinunt, P., and Joshi, N. S. (2018). Engineered living materials: Prospects and challenges for using biological systems to direct the assembly of smart materials. Adv. Mat. 30 (19), 1704847–1704934. doi:10.1002/adma.201704847
Onofri, S., De Vera, J. P., Zucconi, L., Selbmann, L., Scalzi, G., Venkateswaran, K. J., et al. (2015). Survival of antarctic cryptoendolithic fungi in simulated martian conditions on board the international space station. Astrobiology 15 (12), 1052–1059. doi:10.1089/ast.2015.1324
Perez, D. (2020). The first algae-powered building presents unique renewable energy solution. Available at: https://www.engineering.com/story/the-first-algae-powered-building-presents-unique-renewable-energy-solution (Accessed July 7, 2021).
Robertson, K. L., Mostaghim, A., Cuomo, C. A., Soto, C. M., Lebedev, N., Bailey, R. F., et al. (2012). Adaptation of the black yeast wangiella dermatitidis to ionizing radiation: Molecular and cellular mechanisms. Plos one 7, e48674. doi:10.1371/journal.pone.0048674
Romo, R., Andersen, C., Hamilton, J., and Mueller, R. P., Basalt derived feedstock for ISRU manufacturing, CIP 2017 convention, Planetary & Terrestrial Mining Sciences, Montreal, Quebec, 2017, 1-3 May.
Rothschild, L. J., Maurer, C., Lima, I. G. P., Senesky, D., Wipat, A., Head, J., et al. (2018). Myco-architecture off planet: Growing surface structures at destination. NTRS-NASA Technical Reports Server.
Rousek, T., Eriksson, K., and Doule, O. (2012). SinterHab. Acta Astronaut. 74, 98–111. doi:10.1016/j.actaastro.2011.10.009
Rudin, A., and Choi, P. (2013). “Chapter 13 - biopolymers,” in The elements of polymer science & engineering. Editors A. Rudin, and P. Choi. Third Edition (Cambridge, Massachusetts, United States: Academic Press), 521–535.
Ruess, F., Schaenzlin, J., and Benaroya, H. (2006). Structural design of a lunar habitat. J. Aerosp. Eng. 19 (3), 133–157. doi:10.1061/(asce)0893-1321(2006)19:3(133)
Smit, G. N., de Klerk, J. N., Schneider, M. B., and van Eck, J. (2015). Quantifying harvestable encroacher bush. Bonn, Germany: Deutsche Gesellschaft fur Internationale Zisammenarbeit (GIZ) GmbH.
Stanford-Brown-RISD (2018). Myco for Mars. Available at: http://2018.igem.org/Team:Stanford-Brown-RISD (Accessed April 8, 2021).
Taylor, L. A., and Meek, T. T. (2005). Microwave sintering of lunar soil: Properties, theory, and practice. J. Aerosp. Eng. 18 (3), 188–196. doi:10.1061/(asce)0893-1321(2005)18:3(188)
United Nations (2018). The 2030 agenda and the sustainable development goals: An opportunity for Latin America and the caribbean (LC/G. 2681-P/Rev. 3). Santiago: United Nations Digital Repository. https://repositorio.cepal.org/handle/11362/40156.4
Vallas, T., and Courard, L. (2017). Using nature in architecture: Building a living house with mycelium and trees. Front. Archit. Res. 6 (3), 318–328. doi:10.1016/j.foar.2017.05.003
Waters, S. M., Robles-Martinez, J. A., and Nicholson, W. L. (2014). Exposure of Bacillus subtilis to low pressure (5 kilopascals) induces several global regulons, including those involved in the SigB- mediated general stress response. Appl. Environ. Microbiol. 80, 4788–4794. doi:10.1128/aem.00885-14
Ximenes, S. W., Elliott, J. O., and Bannova, O., (2012). Defining a mission architecture and technologies for lunar lava tube reconnaissance. Earth and Space 2012 - Proceedings of the 13th ASCE Aerospace Division Conference and the 5th NASA/ASCE Workshop on Granular Materials in Space Exploration. Pasadena, United. April 15–18, 2012, pp.344–354.
Keywords: construction materials, sustainability, biodesign, mycelium, space architecture, biocomposites, in-situ resource utilization
Citation: Brandić Lipińska M, Maurer C, Cadogan D, Head J, Dade-Robertson M, Paulino-Lima IG, Liu C, Morrow R, Senesky DG, Theodoridou M, Rheinstädter MC, Zhang M and Rothschild LJ (2022) Biological growth as an alternative approach to on and off-Earth construction. Front. Built Environ. 8:965145. doi: 10.3389/fbuil.2022.965145
Received: 09 June 2022; Accepted: 25 August 2022;
Published: 19 September 2022.
Edited by:
Saurabh Rawat, Jaypee University of Information Technology, IndiaReviewed by:
Gabriele Masera, Politecnico di Milano, ItalyGeorgios Kampas, University of Greenwich, United Kingdom
Copyright © 2022 Brandić Lipińska, Maurer, Cadogan, Head, Dade-Robertson, Paulino-Lima, Liu, Morrow, Senesky, Theodoridou, Rheinstädter, Zhang and Rothschild. This is an open-access article distributed under the terms of the Creative Commons Attribution License (CC BY). The use, distribution or reproduction in other forums is permitted, provided the original author(s) and the copyright owner(s) are credited and that the original publication in this journal is cited, in accordance with accepted academic practice. No use, distribution or reproduction is permitted which does not comply with these terms.
*Correspondence: Monika Brandić Lipińska, bS5oLmxpcGluc2thMkBuZXdjYXN0bGUuYWMudWs=; Lynn J. Rothschild, bHlubi5qLnJvdGhzY2hpbGRAbmFzYS5nb3Y=