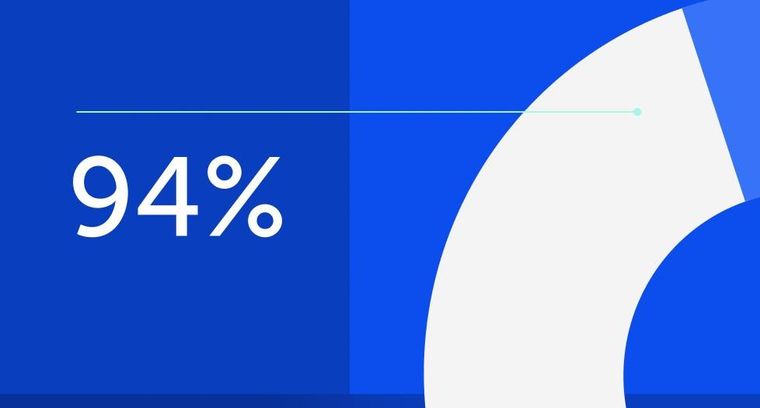
94% of researchers rate our articles as excellent or good
Learn more about the work of our research integrity team to safeguard the quality of each article we publish.
Find out more
REVIEW article
Front. Built Environ., 10 March 2022
Sec. Wind Engineering and Science
Volume 8 - 2022 | https://doi.org/10.3389/fbuil.2022.830207
This article is part of the Research TopicHorizons in Built EnvironmentView all 9 articles
This paper surveys the rapidly growing field of performance-based wind engineering (PBWE) of engineered systems, with focus on not only how PBWE has evolved since its early incarnations inspired by performance-based seismic engineering, but also the unique challenges of PBWE and the research that continues to emerge to tackle them. The limitations of traditional prescriptive wind design approaches are discussed with the aim of illustrating how such approaches are inadequate for providing acceptable building performance during extreme wind events, thus motivating why performance-based strategies for wind engineering are gaining traction and are poised to complement, if not replace, current approaches to wind design. In this respect, the current state of knowledge on the factors that affect building performance via extreme structural response, damage to the envelope system, and nonstructural components, is reviewed and challenges are identified. Lastly, the potential benefit of integrating optimization methods is identified while acknowledging the computational difficulty associated with such approaches.
With the burgeoning growth of high-rise building construction around the globe and an increased awareness for the creation of sustainable urban habitats, solutions for performance-oriented efficient and economical building systems are in great need. To address this, extensive research has been carried out over the past four decades in the area of performance-based engineering (PBE). While initial focus was on developing methods for achieving buildings systems with greater earthquake resistance (e.g., Moehle and Deierlein (2004)), the concepts of PBE have extended to other hazards, including wind, fire and tsunamis (e.g., Ciampoli et al. (2011); Wang et al. (2012); Attary et al. (2017). Furthermore, the successful development of performance-based seismic engineering (PBSE) and its adoption in codes and practice over the past two decades has provided strong evidence for, not only the application of similar approaches for other natural and man-made hazards, but also risk-consistent multi-hazard design approaches (Gardoni and LaFave (2016); Suksuwan and Spence (2018); Kwag et al. (2021). To successfully transfer this knowledge to wind engineering, the fundamental differences between seismic and wind effects for both structural and non-structural components, especially the envelope system, must be embraced while respecting the unique characteristics of wind loading and concurrent hazards (e.g., rainfall and debris impact).
Wind-excited structures have been historically designed to respond elastically under strength-level loads. In transitioning to a PBE setting, there is growing interest in allowing controlled inelastic deformation in specifically designed members under extreme winds (ASCE/SEI, 2019). The advantages of such a design approach are two fold, first it provides a means to engineer more economic systems through enabling the exploration of the full resistance of materials and components, secondly, it provides a means to design innovative systems for resisting both wind and seismic actions when they are comparable. These advantages come at the price of requiring careful assessment of the response of both the deformation-controlled components as well as the system as a whole. This implies the need for development of design guidelines that are informed by research on the hysteretic response and damage accumulation until collapse of structures designed with controlled inelasticity, as well as the consequences of such a design philosophy on the performance metrics and reliabilities of such systems. In the same vein, performance assessment frameworks for the building envelope (i.e. cladding system) and nonstructural components/systems need to be capable of quantifying potential damage arising from dynamic wind pressures, structural response, wind-driven rain, and wind-borne debris. Fundamental to such an assessment is the proper capture of the dependence between structural response and the net pressure demands of the envelope system as this will dictate the capacity of the cladding system to resist the hazards (Ouyang and Spence, 2019).
This paper is written and organized to serve as a review of the origins of PBWE. Through reflecting on the beginnings of PBSE, the major considerations enabling the leap from the current state-of-practice to a PBWE setting are discussed. The unique challenges and the latest developments in this transition are outlined. The potential benefits and challenges to integrating PBWE with optimization are also discussed.
Performance-based engineering may be defined as the practice of thinking and working in terms of ends rather than means (Gibson, 1982; Ellingwood, 1998). Considering a building system as an example, performance-based design (PBD) centers on what the building system is required to do rather than explicitly prescribing how it is to be constructed. While there is a strong interest in moving towards such an approach when it comes to designing buildings to resist natural hazards, most building codes are still prescriptive in nature (Meacham, 2010). In a typical building design process, design professionals select, proportion, and detail components to satisfy prescriptive criteria contained within a building code. Many of these criteria were developed with the intent to provide some level of performance; however, the intended performance levels are often fuzzy, and the actual ability of the resulting designs to provide the intended reliability is seldom evaluated or understood (Federal Emergency Management Agency (FEMA), 2012a; Ellingwood, 2001). An area of structural engineering that has been particularly active in attempting to apply the principles of PBD is that concerning the design of buildings to resist earthquakes.
Traditional prescriptive provisions for seismic design were developed commencing from the late 1920s (Applied Technology Council (ATC), 1995a) and can be viewed as implicitly performance-oriented in that they were developed with the intent of achieving specific performance, that is avoidance of collapse and assurance of life safety. However, damage assessments made on buildings following minor, moderate and intense ground shaking over the past 80+ years have shown that these implicit performance targets cannot be reliably realized following such an approach (Whittaker et al., 2003). The significant economic losses, as well as the loss of function of critical facilities, during the 1989 Loma Prieta and 1994 Northridge earthquakes may be seen as the events that spurred the initial development of modern performance-based seismic design with the aim of developing resilient, loss-resistant communities (Whittaker et al., 2003; Ghobarah, 2001). Indeed, in the early to mid 1990s, FEMA funded the Applied Technology Council (ATC) and the Building Seismic Safety Council (BSSC) with the aim of developing procedures for the implementation of performance-based seismic design (PBSD). This led to the publication of the NEHRP Guidelines and Commentary for Seismic Rehabilitation of Buildings (Federal Emergency Management Agency (FEMA), 1997). The concepts and procedures proposed in this work are generally considered to constitute the foundation of the first generation of PBSD methods. In particular, several important earthquake-related concepts that may now be considered not only as a baseline for understanding the underlying philosophy of PBSD, but also the starting point for applying the principles of PBD to resist other natural and man-made hazards, were conceptualized (Whittaker et al., 2003; Moehle and Deierlein, 2004). Other important pioneering PBSD efforts that significantly contributed to this end include the SEAOC’s Vision 2000 (Structural Engineers Association of California (SEAOC), 1995), ATC-32 (Applied Technology Council (ATC), 1996a) and ATC-40 (Applied Technology Council (ATC), 1996b) reports as well as the FEMA 356 (Federal Emergency Management Agency (FEMA), 2000a) report. The key concept introduced by the aforementioned works was the idea of performance objective, consisting of a design event of specified intensity (earthquake hazard), which the building is to be designed to resist, and a permissible level of damage (performance level) given that the design event occurs. In particular, standard performance levels with performance-oriented descriptions (Fully Operational, Functional - referred to as Immediate Occupancy in Applied Technology Council (ATC) (1995b) - Life Safety, and Near Collapse - referred to as Collapse Prevention in Applied Technology Council (ATC) (1995b)) - were introduced for quantifying both structural and non-structural damage in terms of typical response parameters (inter-story drifts, inelastic member deformations, member forces etc.) therefore defining a number of standard performance objectives as illustrated in Figure 1 for three different occupancy categories. For this first generation of PBSD procedures, a building was said to satisfy its global objectives if structural analyses indicated that the member forces or deformations imposed on each element did not exceed predefined limits (Porter, 2003).
FIGURE 1. Standard performance objectives, SEAOC’s Vision 2000 (Structural Engineers Association of California (SEAOC), 1995).
While the first generation of PBSD methodologies represented an important milestone in the practical application of PBD principles to earthquake resilient design, several shortcomings were identified (Porter, 2003; Whittaker et al., 2003; Moehle and Deierlein, 2004). Among these were: 1) the performance of the system is identified on the basis of damage sustained at a component-level; 2) the inherent uncertainty that affects all aspects of the structural response prediction was not explicitly modeled (Ellingwood, 2008); and 3) the standard discrete performance levels did not directly address some primary stakeholders’ concerns, such as probable repair costs and time of occupancy loss in the building, due to earthquake induced damage. To address these and other limitations, FEMA published an action plan (Federal Emergency Management Agency (FEMA), 2000b; Federal Emergency Management Agency (FEMA), 2006) for the development of the next generation of PBSD procedures. This resulted in the publication by FEMA of the P-58 volumes (Federal Emergency Management Agency (FEMA), 2012a; 2012b; 2012c). These volumes outline a general methodology for the seismic performance assessment of individual buildings that explicitly accounts for the inevitable uncertainty in the ability to accurately predict response while communicating performance through system-level measures that are easily understood by decision-makers and/or stakeholders, i.e. probable consequences, in terms of human losses (deaths and serious injuries), direct economic losses (building repair or replacement costs), and indirect losses. The recent completion of phase 2 of the FEMA P-58 project, in which, among other products, the performance of a suite of archetype code conforming buildings were evaluated in terms of the P-58 performance metrics (Federal Emergency Management Agency (FEMA), 2018), promise to continue the evolution of seismic standards and codes towards the principles of PBE. The technical backbone of the procedure is based on the well-known analytical framework developed by the researchers at the Pacific Earthquake Engineering Research Center (PEER) during the period between 1997 and 2010 (Cornell and Krawinkler, 2000; Moehle and Deierlein, 2004; Yang et al., 2009; Günay and Mosalam, 2013). Unlike the first generation of PBD methodologies, in order to provide results that can be used by a multitude of decision models, performance can be assessed for a particular earthquake scenario or intensity, or considering all earthquakes that could occur, and the likelihood of each, over a specified period of time. While the framework was developed for PBSD, it is relatively general and can be considered as a convenient analytical language with which to implement the principles of PBD for obtaining resilient and risk-consistent structures to mitigate the effects of other natural hazards.
The current state-of-the-practice in wind engineering involves the selection of hazard intensities, derived from an appropriate code or standard, with which to carry out performance assessments and therefore design the structural elements of a building or facility. Taking for example the ASCE 7-16 (ASCE 7-16 (2016)), the hazard intensity is given by the maximum 3-s gust wind speed with prescribed mean recurrence interval (MRI). Which MRI to consider is generally governed by the level of resilience that the designer/stakeholder wishes to give the structure, e.g., in the ASCE 7-16 a risk category I, II, III, or IV is selected. Based on the wind speed with prescribed MRI, wind loads are derived that account for aspects such as wind exposure, topography, wind directionality as well as the external geometry of the building under consideration. The loads so obtained are in general to be used for strength level design, i.e., the ultimate limit state that has the purpose of ensuring life safety. Serviceability design is generally left to the purview of the engineer and stakeholder. Once the loads are defined for a given building/facility, an appropriate material code (e.g., ACI 318-11 (2012) for reinforced concrete buildings and AISC 360-16 (2016) for structural steel buildings) is generally adopted for designing the structural elements. These provide detailed prescriptive requirements that the design engineer should comply with in order to ensure life safety. While the procedure outlined above is relatively effective in ensuring the adequacy of the main wind force resisting system (MWFRS), some observations can be made: 1) the process is prescriptive, therefore the actual performance of the system is not known, it is only implicitly assumed to be achieved through following the prescriptions (Griffis et al., 2013; Ghosn et al., 2016b,a); 2) the process is largely deterministic, even though it is known that modeling assumptions greatly affect the results of the procedure (Griffis et al., 2013; Ghosn et al., 2016b,a); 3) the damage sustained by non-structural elements due to excessive drift of the MWFRS is not explicitly contemplated even though it often plays an important economic role in defining the overall building performance (Griffis, 1993; Aswegan et al., 2015; Ghosn et al., 2016b,a); 4) only prescriptive measures, instructions to use impact resistant glazing or storm shutters in wind prone regions etc., are considered for mitigating the risk of debris impact to the building envelope, i.e., no explicit assessment of the risk associated with this important loss mechanism (ASTM, 2007a,b; Vickery, 1970; Wyatt and May 1971; Tsujita et al., 1998; Ohkuma et al., 1998; Chen and Davenport, 2000; Tamura et al., 2001; Hong, 2004; Gani and Légeron, 2011; Vamvatsikos and Cornell, 2002; Maier, 1979; König and Maier, 1981; König, 1987; Maier and Munro, 1982; Maier and Lloyd-Smith, 1986) is contemplated; and 5) losses associated with water ingress due to wind-driven rain, and therefore damage to interior non-structural elements such as partitions, fixed furniture, ceilings, doors etc., are not considered even though they can account for a significant portion of the total losses associated with extreme wind events (Maier et al., 2000).
The limitations outlined above of current wind engineering practice can only be rectified through the definition of a full PBD philosophy similar to that outlined in Section 3 concerning the earthquake resistant design of structures. However, the direct transfer of these concepts to the field of wind engineering is not possible due to: 1) the unique excitation mechanism associated with complex phenomena such as turbulence, detached flow and vortex shedding, that are the driving forces behind pressure induced damage to the building envelope; 2) the difference in the ultimate performance of wind excited structures compared to earthquake excited structures (e.g., wind excited structural components generally experience less damage than non-structural components); 3) the considerably longer duration of wind excitation that makes progressive and interdependent damage mechanisms the norm; and 4) the important role played by performance objectives, such as envelope penetration due to debris or water ingress, that are not contemplated in earthquake resistant design. Notwithstanding these differences, the framework proposed by FEMA in Federal Emergency Management Agency (FEMA) (2012a,b,c) represents a useful and established language with which the principles of PBD can be applied to other natural hazards, including severe windstorms. Additionally, it is important to recognize that while performance objectives, such as fully operational and immediate occupancy, originate in PBSE (as discussed in Section 3.1), they represent statements of desired building functionality at specified load intensities. Therefore, in defining target performance objectives for wind excited structures, the qualitative goals of the aforementioned performance objectives can be retained. Having said this, it should be recognized that additional performance objectives, such as those associated with evacuation prior to severe hurricanes, may be required in developing frameworks for the effective implementation of PBWE.
The devastation and significant economic losses caused by hurricanes Andrew [$27.3 billion (1992 USD)], Iniki [$3.1 billion (1992 USD)] and Opal [$4.7 billion (1995 USD)] during the 1990s, together with the growing acceptance of PBSE, can be seen as events that spurred initial interest in applying the principles of PBE in the assessment and design of wind excited structures (Ellingwood et al., 2004). One of the first frameworks to be proposed for PBWE focused on the performance assessment of residential wood structures (Rosowsky and Ellingwood, 2002). Important contributions of this work included the conceptualization of a suite of performance objectives (from serviceability to ultimate load levels) for wind excited residential buildings, the identification of the need for system-level analysis (as opposed to traditional component-level analysis) if greater confidence in performance predictions were to be achieved, as well as the need to consider uncertainty. Subsequent to this work, the possibility of modeling the performance of wind excited engineered structures within a PBWE setting began to take root (Paulotto et al., 2004; Bashor and Kareem, 2007; Augusti and Ciampoli, 2008; Ciampoli et al., 2011). The initial focus of these works was primarily on establishing the applicability of the PEER framework (Cornell and Krawinkler, 2000; Yang et al., 2009; Günay and Mosalam, 2013), or similar (i.e. reliability integral), to the performance assessment of wind excited tall buildings and long span bridges. Since these initial research efforts, PBWE has seen an explosion of interest with numerous frameworks being proposed for both residential buildings (Rosowsky and Ellingwood, 2002; Barbato et al., 2013; Baheru et al., 2015; Peng et al., 2016; Unnikrishnan and Barbato, 2017) as well as engineered systems (Ciampoli et al., 2011; Griffis et al., 2013; Spence and Kareem, 2014; Bernardini et al., 2015; Judd and Charney, 2015; Chuang and Spence, 2017; Cui and Caracoglia, 2018; Judd, 2018; Chuang and Spence, 2019; Ierimonti et al., 2019; Micheli et al., 2019; Mohammadi et al., 2019; Cui and Caracoglia, 2020; Ouyang and Spence, 2020).
Over the past decade, significant progress has been made towards the development of general PBWE frameworks for the probabilistic assessment and optimal design of engineered systems subject to severe winds. Major breakthroughs have been achieved in modeling structural and non-structural damage and loss due to both synoptic and hurricane winds through probabilistic system-level metrics associated with repair costs, downtime, life cycle costs, as well as occupant comfort (Ciampoli et al., 2011; Petrini and Ciampoli, 2012; Griffis et al., 2013; Spence and Kareem, 2014; Bernardini et al., 2015; Judd and Charney, 2015; Chuang and Spence, 2017; Cui and Caracoglia, 2018; Judd, 2018; Ierimonti et al., 2019; Mohammadi et al., 2019; Chuang and Spence, 2019; Micheli et al., 2019; Cui and Caracoglia, 2020; Ouyang and Spence, 2020). Progress has also been made to extend PBWE for non-synoptic wind events characterized by intricate vortical flows, such as those found in tornadoes and thunderstorm downbursts (Le and Caracoglia, 2018, 2020; Masoomi and van de Lindt, 2016). Notwithstanding these efforts, there is still a lack of consensus on the most appropriate wind field models for capturing the complexities of tornado and thunderstorm downburst flows within a PBWE setting, as well as a need for more general models for simulating the non-stationary and non-straight fluctuating load component while retaining computational efficiency. Interestingly, the closer relationship of non-synoptic winds (as compared to synoptic) to seismic loading may indicate the possibility of translating some of the approaches used in seismic engineering for dissipating energy through material nonlinearity to PBWE. The adoption of such an approach, however, would require careful validation, since non-synoptic winds are not necessarily zero-mean. As will be discussed in more detail in Section 6, approaches have also been proposed for the single-/multi-objective design optimization within the space of the aforementioned probabilistic system-level metrics (Spence and Kareem, 2014; Spence, 2018; Suksuwan and Spence, 2019b,a; Venanzi et al., 2020; Petrini et al., 2020). While many of these frameworks were initially inspired by the fragility/consequence function-based damage/loss modeling approaches introduced by the PEER framework (and subsequently refined in the P-58 methodologies), they have since evolved to include additional metrics, e.g., life cycle costs, as well as wind specific performance criteria associated with, for example, occupant comfort (Bernardini et al., 2015). Importantly, during this evolution, they have generally preserved the fundamental idea underpinning the PEER framework of explicit evaluation of probabilistic system-level metrics that can be understood by a wide range of technical and non-technical decision makers.
Two important limitations of many of the aforementioned frameworks include: 1) the neglect of damage to the envelope system due to direct action of local net wind pressures; and 2) the assumption that the MWFRS can be modeled as elastic (structural damage is only implicitly modeled through fragility functions evaluated from demands estimated from elastic models of the MWFRS).
With respect to the first point, recent extensions of the Florida Public Hurricane Loss Model (FPHLM) to mid-rise residential buildings (e.g. Pita et al. (2016)) have considered these aspects. Nevertheless, the intent of the FPHLM is the performance assessment of portfolios containing hundreds of buildings. The detail with which each building is modeled is not therefore at the level of PBWE where the focus is on the performance assessment of individual buildings. With an explicit focus on individual buildings and PBWE, a fragility-based progressive damage model was recently introduced in Ouyang and Spence (2019). Within the framework, each component of the envelope system is modeled as susceptible to multiple coupled damage states characterized through suites of fragility functions. Demands are modeled through dynamic drift and net pressure characterized through non-Gaussian stochastic models calibrated to specific wind tunnel tests. To model the wind driven rain on the envelope due to the rain event that inevitably accompanies severe windstorms, Eulerian multiphase models based on computational fluid dynamics were adopted. The approach was subsequently embedded with a conditional stochastic simulation scheme, therefore defining a PBWE framework capable of estimating system-level loss and consequences related to decision variables such as repair costs and ingressed water due to envelope damage (Ouyang and Spence, 2020). This approach has recently been extended to consider nonlinearity in the MWFRS (Ouyang and Spence, 2021b) as well as more complex representations of the wind hazard, i.e., the non-stationary/-straight/Gaussian wind pressures that are characteristic of hurricanes before idealization (Ouyang and Spence, 2021a).
With respect to the second point, the neglect of potential nonlinearity in the MWFRS can be traced back to the following difficulties: 1) the long duration (in the order of hours) of typical dynamic wind loads, therefore creating a significant computational barrier to propagating uncertainty through nonlinear models of the MWFRS in determining the probabilistic performance metrics; and 2) the complexity of modeling the nonlinear response of the MWFRS where the presence of a substantial mean wind load component (for certain wind directions) creates theoretical difficulties in applying state-of-the-art nonlinear modeling approaches that have been calibrated to zero mean seismic loads. The long duration and substantial mean wind load for certain directions also make the exploitation of nonlinear material behavior for energy dissipation less straightforward than in seismic engineering, since potential issues can arise due to low-cycle fatigue failure and lack of complete internal force reversal in the structural elements. Notwithstanding these challenges, the neglect of potential damage to the MWFRS is fundamentally contrary to the concept of PBE that is based on the explicit modeling of performance of the system over a full range of hazard intensities. This has inspired interest in developing methods that can explicitly treat damage through nonlinear modeling of the MWFRS. In addition to studies that have looked at understanding specific aspects of inelasticity from a fundamental standpoint, e.g. (Hong, 2004; Gani and Légeron, 2011; Feng and Chen, 2017, 2018; Bezabeh et al., 2021a,b), two approaches have essentially been investigated within the setting of PBWE. The first is based on application of the theory of plasticity through defining the state of dynamic shakedown as a collapse prevention performance objective (Tabbuso et al., 2016; Chuang and Spence, 2017, 2019; Chuang and Spence, 2020; Chuang and Spence, 2022), while the second is based on directly applying nonlinear modeling approaches developed in seismic engineering for the nonlinear analysis of the MWFRS (Judd and Charney, 2015; Mohammadi et al., 2019; Nikellis et al., 2019; Ouyang and Spence, 2021b; Ghaffary and Moustafa, 2021; Huang and Chen, 2022). The intent of the first approach is to rapidly provide a means for identifying a region in which inelasticity can occur safely, i.e. without potential failure due to low-cycle fatigue (acrosswind failure), ratcheting (alongwind failure), instantaneous plastic collapse, or excessive plastic deformation. The computational efficacy of the approach enables evaluation of reliability through direct stochastic simulation (Chuang and Spence, 2022). While the second approach provides greater modeling flexibility, a major challenge lies in the huge computational effort necessary to propagate uncertainty through the nonlinear finite element models (due to the long duration of wind events as compared to earthquakes) and therefore estimate general system-level damage/loss metrics that are consistent with current PBWE frameworks.
The need to bring low-rise buildings under the umbrella of PBWE is strongly recognized as they represent the majority of the building stock in the United States. Better damage assessment through frameworks that are based on the principles of PBWE would support improved residential building practices, and limit economic losses and social disruption (Ellingwood et al., 2008). Interestingly, as mentioned in Section 5.1, one of the earliest works in conceptualizing PBWE concerned the performance assessment of residential wood structures (Rosowsky and Ellingwood, 2002). Although research in the area of PBWE of low-rise buildings has lagged that of engineered buildings, some notable recent research efforts include the development of initial PBWE frameworks for non-engineered buildings with multi-hazard considerations (Unnikrishnan and Barbato, 2017, 2016), introduction of scales for classifying post-disaster structural functionality within the setting of PBWE (Nevill and Lombardo, 2020), wind-induced damage assessment of low-rise building envelopes with potential openings (Ji et al., 2020), and the experimental investigation of the propagation of wind-driven rain into the building interior of low-rise buildings (Raji et al., 2020).
The important research developments outlined in Section 5.2, coupled with the significant interest from industry to implement PBWE in practice, has culminated in the recent publication by the American Society of Civil Engineers (ASCE) of the Prestandard on PBWD (ASCE/SEI, 2019). Major innovations of this document are the introduction of limit states that explicitly allow (for the first time) nonlinearity in the MWFRS, the explicit integration of acceptance criteria related to the performance of the envelope system, and the definition of performance objectives over a full range of hazard intensities.
The performance objectives span occupant comfort through serviceability to ultimate strength where additional capacity arising from controlled inelasticity is permitted. To demonstrate building functionality across the range of objectives, linear elastic analysis is permitted for evaluating occupant comfort and operational performance targets since the system itself is required to remain elastic, whereas advanced analysis procedures can be employed to evaluate the continuous occupancy performance objective. To this end, three methods have been proposed, with two of them requiring nonlinear response history analysis at collapse or reliability-based dynamic shakedown to evaluate the intended performance of the deformation-controlled elements. The Prestandard has also explicitly included performance objectives and acceptance criteria for the evaluation of the building envelope and non-structural components.
As a relevant example of adoption in building design/construction practices of performance-based engineering, PBSE took around 25–30 years to advance from conception to widespread acceptance in practice. As highlighted in Section 2, first-generation of PBSE began in the early 1990s with second-generation PBSE starting in the early 2000s and achieving a certain maturity by the mid-2010s with widespread acceptance and adoption by industry thereafter. Similarly, since the beginning of focused research on PBWE in the late 2000s, significant progress has been made over the past decade. The release of the Prestandard on PBWD is a major milestone and, if current research and standards development efforts continue, a similar trend as seen for PBSE can be expected, leading to the widespread implementation in practice of PBWE in the next 10–15 years.
As has been outlined in the previous sections, the practical implementation of modern PBD requires the rigorous use of reliability/probabilistic models for the performance evaluation of the system. Compared to traditional deterministic design, this approach therefore entails the use of more complex and computationally cumbersome models. This makes the traditional trial-and-error approach to finding designs that satisfy the multiple performance objectives both time-consuming and non-intuitive. This is further compounded if systems that are economically optimum in meeting the performance goals are also desired. To overcome these difficulties, PBD procedures must be coupled with optimization algorithms, as shown in Figure 2, that are capable of rigorously handling the reliability/probabilistic performance assessment models of current PBWE frameworks. A class of optimization methodologies that respond to this need is constituted by the reliability-based design optimization (RBDO) algorithms (Schuëller and Jensen, 2008; Valdebenito and Schuëller, 2010). Indeed, in RBDO the aim is the resolution of problems that are characterized by generally deterministic cost/objective functions subject to a number of probabilistic constraints (e.g., Valdebenito and Schuëller (2010)). The recent boom in computational power has spawn intense research in this area as it has opened the door to the possibility of solving problems that were previously deemed intractable. Notwithstanding these research efforts, there is still need for the development of specific RBDO algorithms that efficiently yield optimum solutions to practical probabilistic PBD problems that are often posed in terms of multiple performance constraints, high-dimensional random variable vectors as well as discrete high-dimensional design variable vectors. As outlined in Federal Emergency Management Agency (FEMA) (2006), each of these characteristics makes the RBDO problem non-trivial due to the implicit nature, in terms of the design variable vector, of the probabilistic constraints and the inherently nested nature of the reliability analysis within the optimization loop (Aoues and Chateauneuf, 2010; Valdebenito and Schuëller, 2010).
The main difficulty in solving the optimization loop outlined in Figure 2 is presented by the probabilistic nature of the performance assessment that essentially requires the resolution of a reliability integral similar to that of the classic PEER framework (Ouyang and Spence, 2020; 2021b). Indeed, the treatment of this type of integral within an optimization problem is characterized by the following difficulties: 1) it is implicit in the design variable vector therefore hindering sensitivity analyses; and 2) its evaluation requires probabilistic analyses which will in general be computationally cumbersome. These difficulties are further compounded if the design variable vector is of high dimensions (hundreds of components), as is the case for many practical applications, and if the number of constraints to be considered is also elevated. Further complication is added if the uncertain vector has more than a handful of components as this will practically eliminate the possibility of using approximate reliability analysis (first or second order reliability methods) due to the increasing difficulty this produces in finding the design point (Schuëller et al., 2003). In addition, the various methods that have been developed over the years for optimizing stochastic systems modeled through large and complex finite element models (e.g., metamodeling approaches (Zhu et al., 2011; Chen et al., 2015; Moustapha et al., 2016), subset simulation optimization (SSO) (Taflanidis and Beck, 2009; Jia and Taflanidis, 2013; Jia et al., 2015), and sequential optimization methods (Du and Chen, 2004; Zou and Mahadevan, 2006; Jensen et al., 2008; Valdebenito and Schuëller, 2011; Jensen et al., 2012)), are not generally applicable to systems with more than a dozen or so free design parameters. The main reason for this can be traced back to how the focus of the aforementioned approaches is mainly on treating problems with complex and generally nonlinear response behaviors. In the case of the large-scale structures often found in practice, this can represent a significant limitation as these systems are generally designed in terms of hundreds of free parameters. A philosophical approach that can in theory efficiently treat problems with high-dimensional design spaces is that based on decoupling the probabilistic analysis from the optimization loop through approximations that are constructed from information pertaining to a limited number of probabilistic analyses (Spence and Gioffrè, 2012; Royset et al., 2001; Du and Chen, 2004; Zou and Mahadevan, 2006; Ching and Hsieh, 2007; Jensen et al., 2008; Valdebenito and Schuëller, 2011). This approach has been explored within the context of PBWE with the introduction of schemes for both single and multi-objective optimization while considering performance metrics ranging from accelerations at the performance objective of occupant comfort (Spence, 2018), through drifts and component responses at the performance objectives of serviceability and continuous occupancy (Spence and Kareem, 2014), to explicit evaluation of system-level loss metrics (Suksuwan and Spence, 2019b,a; Subgranon and Spence, 2021). This approach has also been extended to topology optimization formulated explicitly in the space of PBWE metrics (Kareem et al., 2013; Bobby et al., 2014; Bobby et al., 2016). The difficulty associated with optimizing in high-dimensional spaces of design variables can be avoided by choosing small subsets of parameters that are most influential to the performance metrics. For example, recent works have looked at optimally choosing the parameters of auxiliary damping devices for minimizing a variety of performance metrics (Petrini et al., 2020; Venanzi et al., 2020).
This paper reviewed the origins and current state-of-the-art of PBWE that is poised to inform the next generation of load and design codes for wind. A historical account is presented and pioneering works are briefly summarized with key emphasis on the differences between PBSE and PBWE. The current state of practice is reviewed, and its limitations are highlighted. The broad areas of active research within PBWE are identified as the inelastic modeling/design of wind excited structures and the modeling of the envelope performance that includes consideration of the risk from wind driven rain and debris impact. The role of optimization was discussed within the context of optimally satisfying the performance objectives associated with occupant comfort, serviceability and ultimate capacity. Additional areas of future research include the experimental validation of the state-of-the-art numerical frameworks associated with, but not limited to, wind load modeling and nonlinear structural analysis, with particular attention on assessing the validity of models/tools borrowed from seismic engineering. In a similar vein, the applicability of the R-factor (force reduction factor), ductile detailing concepts and innovative damping devices in PBWE, and more in general, in mixed hazard environments, requires investigation. Additional research developments that would be of relevance concern the assimilation in the models of field data on cladding performance during hurricane events. This would enable the establishment of better semi-empirical fragility functions as well as damage states and consequence functions for describing building envelope performance. More research on PBWE of low-rise structures as well as PBWE for non-synoptic winds is also needed. In conclusion, the significant advances in PBWE of the past decade are changing the way buildings are assessed and designed against wind. Although there is still much to be done, the continued development of PBWE promises to enhance the resilience of future communities to extreme wind events while increasing sustainability through enabling greater design innovation.
The original contributions presented in the study are included in the article/Supplementary Material, further inquiries can be directed to the corresponding author.
All authors listed have made a substantial, direct, and intellectual contribution to the work and approved it for publication.
The research effort was supported in part by the United States National Science Foundation (NSF) under Grants No. CMMI-1462084, CMMI-1562388, CMMI-1750339, and CMMI-2118488.
The authors declare that the research was conducted in the absence of any commercial or financial relationships that could be construed as a potential conflict of interest.
All claims expressed in this article are solely those of the authors and do not necessarily represent those of their affiliated organizations, or those of the publisher, the editors, and the reviewers. Any product that may be evaluated in this article, or claim that may be made by its manufacturer, is not guaranteed or endorsed by the publisher.
ACI 318-11 (2012). Building Code Requirements for Structural concrete and Commentary. Michigan, USA: American Concrete Institute.
AISC 360-16 (2016). Specification for Structural Steel Buildings. Chicago, IL: American Institute of Steel Construction.
Aoues, Y., and Chateauneuf, A. (2010). Benchmark Study of Numerical Methods for Reliability-Based Design Optimization. Struct. Multidisc Optim 41, 277–294. doi:10.1007/s00158-009-0412-2
Applied Technology Council (ATC) (1995a). A Critical Review of Current Approaches to Earthquake-Resistant Design. Redwood City, CA: Tech. rep., Report no. ATC-34.
Applied Technology Council (ATC) (1995b). Guidelines and Commentary for Seismic Rehabilitation of Buildings. Redwood City, CA: Tech. rep., Report no. ATC-33.
Applied Technology Council (ATC) (1996a). Seismic Design Criteria for California Bridges: Provisional Recommendations. Redwood City, CA: Tech. rep., Report no. ATC-32.
Applied Technology Council (ATC) (1996b). Seismic Evaluation and Retrofit of concrete Buildings. Redwood City, CA: Tech. rep., Report no. ATC-40.
ASCE 7-16 (2016). Minimum Design Loads and Associated Criteria for Buildings and Other Structures. Reston, VA: American Society of Civil Engineers.
ASCE/SEI (2019). Prestandard for Performance-Based Wind Design (Prepared by Structural Engieering Institute (SEI). American Society of Civil Engineers.
ASTM (2007a). “Standard Guide for Seismic Risk Assessment of Buildings,” in ASTM E2026-07 (West Conshohocken, PA: ASTM International).
ASTM (2007b). “Standard Practice for Probable Maximum Loss (Pml) Evaluations for Earthquake Due-Diligence Assessments,” in ASTM E2557-07 (West Conshohocken, PA: ASTM International).
Aswegan, K., Charney, F. A., and Jarrett, J. (2015). Recommended Procedures for Damage Based Serviceability Design of Steel Buildings under Wind Loads. AISC Eng. J. 52, 1–25.
Attary, N., Unnikrishnan, V. U., van de Lindt, J. W., Cox, D. T., and Barbosa, A. R. (2017). Performance-based Tsunami Engineering Methodology for Risk Assessment of Structures. Eng. Structures 141, 676–686. doi:10.1016/j.engstruct.2017.03.071
Augusti, G., and Ciampoli, M. (2008). Performance-based Design in Risk Assessment and Reduction. Probabilistic Eng. Mech. 23, 496–508. doi:10.1016/j.probengmech.2008.01.007
Baheru, T., Chowdhury, A. G., and Pinelli, J.-P. (2015). Estimation of Wind-Driven Rain Intrusion through Building Envelope Defects and Breaches during Tropical Cyclones. Nat. Hazards Rev. 16, 04014023. doi:10.1061/(asce)nh.1527-6996.0000158
Barbato, M., Petrini, F., Unnikrishnan, V. U., and Ciampoli, M. (2013). Performance-based hurricane Engineering (PBHE) Framework. Struct. Saf. 45, 24–35. doi:10.1016/j.strusafe.2013.07.002
Bashor, R., and Kareem, A. (2007). “Probabilistic Performance Evaluation of Buildings: an Occupant comfort Perspective.” in Proceedings of the 12th International Conference on Wind Engineering (12-ICWE). 1335–1342.
Bernardini, E., Spence, S. M. J., Kwon, D.-K., and Kareem, A. (2015). Performance-based Design of High-Rise Buildings for Occupant comfort. J. Struct. Eng. 141, 04014244. doi:10.1061/(asce)st.1943-541x.0001223
Bezabeh, M. A., Bitsuamlak, G. T., and Tesfamariam, S. (2021a). Nonlinear Dynamic Response of Single-Degree-Of-freedom Systems Subjected to Along-Wind Loads. I: Parametric Study. J. Struct. Eng. 147, 04021177. doi:10.1061/(asce)st.1943-541x.0003125
Bezabeh, M. A., Bitsuamlak, G. T., and Tesfamariam, S. (2021b). Nonlinear Dynamic Response of Single-Degree-Of-freedom Systems Subjected to Along-Wind Loads. II: Implications for Structural Reliability. J. Struct. Eng. 147, 04021178. doi:10.1061/(asce)st.1943-541x.0003124
Bobby, S., Spence, S. M. J., Bernardini, E., and Kareem, A. (2014). Performance-based Topology Optimization for Wind-Excited Tall Buildings: A Framework. Eng. Structures 74, 242–255. doi:10.1016/j.engstruct.2014.05.043
Bobby, S., Spence, S. M. J., and Kareem, A. (2016). Data-driven Performance-Based Topology Optimization of Uncertain Wind-Excited Tall Buildings. Struct. Multidisc Optim 54, 1379–1402. doi:10.1007/s00158-016-1474-6
Chen, D., and Davenport, A. G. (2000). Vulnerability of Tall Buildings in Typhoons. Adv. Struct. Dyn. 2, 1455–1462.
Chen, Z., Peng, S., Li, X., Qiu, H., Xiong, H., Gao, L., et al. (2015). An Important Boundary Sampling Method for Reliability-Based Design Optimization Using Kriging Model. Struct. Multidisc Optim 52, 55–70. doi:10.1007/s00158-014-1173-0
Ching, J., and Hsieh, Y.-H. (2007). Approximate Reliability-Based Optimization Using a Three-step Approach Based on Subset Simulation. J. Eng. Mech. 133, 481–493. doi:10.1061/(asce)0733-9399(2007)133:4(481)
Chuang, W.-C., and Spence, S. M. J. (2017). A Performance-Based Design Framework for the Integrated Collapse and Non-collapse Assessment of Wind Excited Buildings. Eng. Structures 150, 746–758. doi:10.1016/j.engstruct.2017.07.030
Chuang, W.-C., and Spence, S. M. J. (2019). An Efficient Framework for the Inelastic Performance Assessment of Structural Systems Subject to Stochastic Wind Loads. Eng. Structures 179, 92–105. doi:10.1016/j.engstruct.2018.10.039
Chuang, W.-C., and Spence, S. M. J. (2020). Probabilistic Performance Assessment of Inelastic Wind Excited Structures within the Setting of Distributed Plasticity. Struct. Saf. 84, 101923. doi:10.1016/j.strusafe.2020.101923
Chuang, W. C., and Spence, S. M. J. (2022). A Framework for the Efficient Reliability Assessment of Inelastic Wind Excited Structures at Dynamic Shakedown. J. Wind Eng. Ind. Aerodynamics. In Press. doi:10.1016/j.jweia.2021.104834
Ciampoli, M., Petrini, F., and Augusti, G. (2011). Performance-based Wind Engineering: towards a General Procedure. Struct. Saf. 33, 367–378. doi:10.1016/j.strusafe.2011.07.001
Cornell, C. A., and Krawinkler, H. (2000). Progress and Challenges in Seismic Performance Assessment. PEER Cent. News 3, 1–4.
Cui, W., and Caracoglia, L. (2018). A Unified Framework for Performance-Based Wind Engineering of Tall Buildings in hurricane-prone Regions Based on Lifetime Intervention-Cost Estimation. Struct. Saf. 73, 75–86. doi:10.1016/j.strusafe.2018.02.003
Cui, W., and Caracoglia, L. (2020). Performance-based Wind Engineering of Tall Buildings Examining Life-Cycle Downtime and Multisource Wind Damage. J. Struct. Eng. 146, 04019179. doi:10.1061/(asce)st.1943-541x.0002479
Du, X., and Chen, W. (2004). Sequential Optimization and Reliability Assessment Method for Efficient Probabilistic Design. J. Mech. Des. 126, 225–233. doi:10.1115/1.1649968
Ellingwood, B. R. (2001). Acceptable Risk Bases for Design of Structures. Prog. Struct. Engng Mater. 3, 170–179. doi:10.1002/pse.78
Ellingwood, B. R. (1998). “Reliability-based Performance Concept for Building Construction,” in Proceedings of Structural Engineering World Wide. T178-4, CD-ROM.
Ellingwood, B. R., Rosowsky, D. V., Li, Y., and Kim, J. H. (2004). Fragility Assessment of Light-Frame wood Construction Subjected to Wind and Earthquake Hazards. J. Struct. Eng. 130, 1921–1930. doi:10.1061/(asce)0733-9445(2004)130:12(1921)
Ellingwood, B. R., Rosowsky, D. V., and Pang, W. (2008). Performance of Light-Frame wood Residential Construction Subjected to Earthquakes in Regions of Moderate Seismicity. J. Struct. Eng. 134, 1353–1363. doi:10.1061/(asce)0733-9445(2008)134:8(1353)
Ellingwood, B. R. (2008). Structural Reliability and Performance-Based Engineering. Structures and Buildings 161, 199–207. doi:10.1680/stbu.2008.161.4.199
Federal Emergency Management Agency (FEMA) (2000b). Action Plan for Performance Based Seismic Design. Washington, DC: Tech. rep., Report no. FEMA-349.
Federal Emergency Management Agency (FEMA) (1997). NEHRP Guidelines for the Seismic Rehabilitation of Buildings. Washington, DC: Tech. rep., Report no. FEMA-273.
Federal Emergency Management Agency (FEMA) (2006). Next-generation Performance-Based Seismic Design Guidelines - Program Plan for New and Existing Buildings. Washington, DC: Tech. rep., Report no. FEMA-445.
Federal Emergency Management Agency (FEMA) (2000a). Prestandard and Commentary for Seismic Rehabilitation of Buildings. Washington, DC: Tech. rep., Report no. FEMA-356.
Federal Emergency Management Agency (FEMA) (2012a). Seismic Performance Assessment of Buildings, Volume 1 - Methodology (FEMA Publication P-58-1). Washington, D.C.: Tech. rep.
Federal Emergency Management Agency (FEMA) (2012b). Seismic Performance Assessment of Buildings, Volume 2 - Implementation (FEMA Publication P-58-2). Washington, D.C.: Tech. rep.
Federal Emergency Management Agency (FEMA) (2012c). Seismic Performance Assessment of Buildings, Volume 3 - Supporting Electronic Materials and Background Documentation (FEMA Publication P-58-3). Washington, D.C.: Tech. rep.
Federal Emergency Management Agency (FEMA) (2018). Seismic Performance Assessment of Buildings, Volume 5 - Expected Seismic Performance of Code-Conforming Buildings (FEMA Publication P-58-5). Washington, D.C.: Tech. rep.
Feng, C., and Chen, X. (2017). Crosswind Response of Tall Buildings with Nonlinear Aerodynamic Damping and Hysteretic Restoring Force Character. J. Wind Eng. Ind. Aerodynamics 167, 62–74. doi:10.1016/j.jweia.2017.04.012
Feng, C., and Chen, X. (2018). Inelastic Responses of Wind-Excited Tall Buildings: Improved Estimation and Understanding by Statistical Linearization Approaches. Eng. structures 159, 141–154. doi:10.1016/j.engstruct.2017.12.041
Gani, F., and Légeron, F. (2011). Relationship between Specified Ductility and Strength Demand Reduction for Single Degree-Of-freedom Systems under Extreme Wind Events. J. Wind Eng. Ind. Aerodynamics 109, 31–45.
Gardoni, P., and LaFave, J. M. (2016). “Multi-hazard Approaches to Civil Infrastructure Engineering: Mitigating Risks and Promoting Resilence,” in Multi-hazard Approaches to Civil Infrastructure Engineering (Berlin, Germany: Springer), 3–12. doi:10.1007/978-3-319-29713-2_1
Ghaffary, A., and Moustafa, M. A. (2021). Performance-based Assessment and Structural Response of 20-story SAC Building under Wind Hazards through Collapse. J. Struct. Eng. 147, 04020346. doi:10.1061/(asce)st.1943-541x.0002911
Ghobarah, A. (2001). Performance-based Design in Earthquake Engineering: State of Development. Eng. structures 23, 878–884. doi:10.1016/s0141-0296(01)00036-0
Ghosn, M., Dueñas-Osorio, L., Frangopol, D. M., McAllister, T., Bocchini, P., Manuel, L., et al. (2016a). Performance Indicators for Structural Systems and Infrastructure Networks. J. Struct. Eng. 142, F4016003. doi:10.1061/(asce)st.1943-541x.0001542
Ghosn, M., Frangopol, D. M., McAllister, T. P., Shah, M., Diniz, S. M. C., Ellingwood, B. R., et al. (2016b). Reliability-based Performance Indicators for Structural Members. J. Struct. Eng. 142, F4016002. doi:10.1061/(asce)st.1943-541x.0001546
Gibson, E. J. (1982). Working with the Performance Approach in Building. Rotterdam, Netherlands: Tech. rep., CIB.
Griffis, L. G., Patel, V., Muthukumar, S., and Baldava, S. (2013). “A Framework for Performance-Based Wind Engineering,” in Proceedings of the 2012 ATC & SEI Conference on Advances in Hurricane Engineering, Miami, FL. doi:10.1061/9780784412626.105
Günay, S., and Mosalam, K. M. (2013). Peer Performance-Based Earthquake Engineering Methodology, Revisited. J. Earthquake Eng. 17, 829–858.
Hong, H. P. (2004). Accumulation of Wind Induced Damage on Bilinear Sdof Systems. Wind and Structures 7, 145–158. doi:10.12989/was.2004.7.3.145
Huang, J., and Chen, X. (2022). Inelastic Performance of High-Rise Buildings to Simultaneous Actions of Alongwind and Crosswind Loads. J. Struct. Eng. 148, 04021258. doi:10.1061/(asce)st.1943-541x.0003236
Ierimonti, L., Venanzi, I., Caracoglia, L., and Materazzi, A. L. (2019). Cost-based Design of Nonstructural Elements for Tall Buildings under Extreme Wind Environments. J. Aerosp. Eng. 32, 04019020. doi:10.1061/(asce)as.1943-5525.0001008
Jensen, H. A., Kusanovic, D. S., Valdebenito, M. A., and Schuëller, G. I. (2012). Reliability-based Design Optimization of Uncertain Stochastic Systems: Gradient-Based Scheme. J. Eng. Mech. 138, 60–70. doi:10.1061/(asce)em.1943-7889.0000304
Jensen, H. A., Valdebenito, M. A., and Schuëller, G. I. (2008). An Efficient Reliability-Based Optimization Scheme for Uncertain Linear Systems Subject to General Gaussian Excitation. Comput. Methods Appl. Mech. Eng. 198, 72–87. doi:10.1016/j.cma.2008.01.003
Ji, X., Huang, G., Wu, F., and Lu, Z.-H. (2020). Wind-induced hazard Assessment for Low-Rise Building Envelope Considering Potential Openings. J. Struct. Eng. 146, 04020039. doi:10.1061/(asce)st.1943-541x.0002553
Jia, G., Taflanidis, A. A., and Beck, J. L. (2015). Non-parametric Stochastic Subset Optimization for Design Problems with Reliability Constraints. Struct. Multidisc Optim 52, 1185–1204. doi:10.1007/s00158-015-1300-6
Jia, G., and Taflanidis, A. A. (2013). Non-parametric Stochastic Subset Optimization for Optimal-Reliability Design Problems. Comput. Structures 126, 86–99. doi:10.1016/j.compstruc.2012.12.009
Judd, J. P., and Charney, F. A. (2015). Inelastic Behavior and Collapse Risk for Buildings Subjected to Wind Loads. Structures Congress 2015, 2483–2496. doi:10.1061/9780784479117.215
Judd, J. P. (2018). Windstorm Resilience of a 10-story Steel Frame Office Building. Asce-asme J. Risk Uncertainty Eng. Syst. Part. A: Civ. Eng. 4, 04018020. doi:10.1061/ajrua6.0000971
Kareem, A., Spence, S. M. J., Bernardini, E., Bobby, S., and Wei, D. (2013). Wind Engineering: Using Computational Fluid Dynamics to Optimize Tall Building Design. CTBUH J. 2013, 38–43.
König, A., and Maier, G. (1981). Shakedown Analysis of Elastoplastic Structures: a Review of Recent Developments. Nucl. Eng. Des. 66, 81–95.
Kwag, S., Gupta, A., Baugh, J., and Kim, H.-S. (2021). Significance of Multi-hazard Risk in Design of Buildings under Earthquake and Wind Loads. Eng. Structures 243, 112623. doi:10.1016/j.engstruct.2021.112623
Le, V., and Caracoglia, L. (2020). A Neural Network Surrogate Model for the Performance Assessment of a Vertical Structure Subjected to Non-stationary, Tornadic Wind Loads. Comput. Structures 231, 106208. doi:10.1016/j.compstruc.2020.106208
Le, V., and Caracoglia, L. (2018). Computationally Efficient Stochastic Approach for the Fragility Analysis of Vertical Structures Subjected to Thunderstorm Downburst Winds. Eng. structures 165, 152–169. doi:10.1016/j.engstruct.2018.03.007
Maier, G., Carvelli, V., and Cocchetti, G. (2000). On Direct Methods for Shakedown and Limit Analysis. Eur. J. Mech. - A/Solids 19, 79–100.
Maier, G., and Lloyd-Smith, D. (1986). “Mathematical Programming Applications to Engineering Plastic Analysis: Upyear to November 1985,” in Applied Mechanics Upyear 1986. Editors C. R. Steele, and G. S. Springer (New York: ASME), 377–383.
Maier, G., and Munro, J. (1982). Mathematical Programming Methods in Engineering Plastic Analysis. Appl. Mech. Rev. - ASME 35, 1631–1643.
Maier, G. (1979). “Shakedown Analysis,” in Engineering Plasticity by Mathematical Programming. Editors M. Z. Cohn, and G. Maier (Oxford, UK: Pergamon Press), 107–134. chap. 6.
Masoomi, H., and van de Lindt, J. W. (2016). Tornado Fragility and Risk Assessment of an Archetype Masonry School Building. Eng. Structures 128, 26–43. doi:10.1016/j.engstruct.2016.09.030
Meacham, B. J. (2010). Risk‐informed Performance‐based Approach to Building Regulation. J. Risk Res. 13, 877–893. doi:10.1080/13669871003703260
Micheli, L., Alipour, A., Laflamme, S., and Sarkar, P. (2019). Performance-based Design with Life-Cycle Cost Assessment for Damping Systems Integrated in Wind Excited Tall Buildings. Eng. Structures 195, 438–451. doi:10.1016/j.engstruct.2019.04.009
Moehle, J., and Deierlein, G. G. (2004). “A Framework Methodology for Performance-Based Earthquake Engineering,” in Proceedings of the 13th World Conference on Earthquake Engineering.
Mohammadi, A., Azizinamini, A., Griffis, L., and Irwin, P. (2019). Performance Assessment of an Existing 47-story High-Rise Building under Extreme Wind Loads. J. Struct. Eng. 145, 04018232. doi:10.1061/(asce)st.1943-541x.0002239
Moustapha, M., Sudret, B., Bourinet, J.-M., and Guillaume, B. (2016). Quantile-based Optimization under Uncertainties Using Adaptive Kriging Surrogate Models. Struct. Multidisc Optim 54, 1403–1421. doi:10.1007/s00158-016-1504-4
Nevill, J. B., and Lombardo, F. T. (2020). Structural Functionality Scale for Light-Framed wood Buildings with Indicators for Windstorm Damage. J. Struct. Eng. 146, 04020033. doi:10.1061/(asce)st.1943-541x.0002551
Nikellis, A., Sett, K., and Whittaker, A. S. (2019). Multihazard Design and Cost-Benefit Analysis of Buildings with Special Moment-Resisting Steel Frames. J. Struct. Eng. 145, 04019031. doi:10.1061/(asce)st.1943-541x.0002298
Ohkuma, T., Kurita, T., and Ninomiya, M. (1998). “Response Estimation Based on Energy Balance for Elasto-Plastic Vibration of Tall Building in Across-Wind Direction,” in ICOSSAR 97: Proceedings of the 7th International Conference on Structural Safety and Reliability, Kyoto, Japan, 1379–1386.
Ouyang, Z., and Spence, S. M. J. (2019). A Performance-Based Damage Estimation Framework for the Building Envelope of Wind-Excited Engineered Structures. J. Wind Eng. Ind. Aerodynamics 186, 139–154. doi:10.1016/j.jweia.2019.01.001
Ouyang, Z., and Spence, S. M. J. (2021a). A Performance-Based Wind Engineering Framework for Engineered Building Systems Subject to Hurricanes. Front. Built Environ. 7, 133. doi:10.3389/fbuil.2021.720764
Ouyang, Z., and Spence, S. M. J. (2020). A Performance-Based Wind Engineering Framework for Envelope Systems of Engineered Buildings Subject to Directional Wind and Rain Hazards. J. Struct. Eng. 146, 04020049. doi:10.1061/(asce)st.1943-541x.0002568
Ouyang, Z., and Spence, S. M. J. (2021b). Performance-based Wind-Induced Structural and Envelope Damage Assessment of Engineered Buildings through Nonlinear Dynamic Analysis. J. Wind Eng. Ind. Aerodynamics 208, 104452. doi:10.1016/j.jweia.2020.104452
Paulotto, C., Ciampoli, M., and Augusti, G. (2004). “Some Proposals for a First Step towards a Performance Based Wind Engineering,” in Forum in Engineering Decision Making (IFED), Stoos, Switzerland.
Peng, X., Roueche, D. B., Prevatt, D. O., and Gurley, K. R. (2016). “An Engineering-Based Approach to Predict Tornado-Induced Damage,” in Multi-hazard Approaches to Civil Infrastructure Engineering (Berlin, Germany: Springer), 311–335. doi:10.1007/978-3-319-29713-2_15
Petrini, F., and Ciampoli, M. (2012). Performance-based Wind Design of Tall Buildings. Struct. Infrastructure Eng. 8, 954–966.
Petrini, F., Giaralis, A., and Wang, Z. (2020). Optimal Tuned Mass-Damper-Inerter (TMDI) Design in Wind-Excited Tall Buildings for Occupants' comfort Serviceability Performance and Energy Harvesting. Eng. Structures 204, 109904. doi:10.1016/j.engstruct.2019.109904
Pita, G. L., Pinelli, J.-P., Gurley, K., Weekes, J., Cocke, S., and Hamid, S. (2016). Hurricane Vulnerability Model for Mid/high-Rise Residential Buildings. Wind and Structures 23, 449–464. doi:10.12989/was.2016.23.5.449
Porter, K. A. (2003). “An Overview of Peer’s Performance-Based Earthquake Engineering Methodology,” in Proceedings of the 9th International Conference on Applications of Statistics and Probability in Civil Engineering (ICASP9), San Francisco, CA, 973–980. Vol. 2.
Raji, F., Zisis, I., and Pinelli, J. P. (2020). Experimental Investigation of Wind-Driven Rain Propagation in a Building interior. J. Struct. Eng. 146, 04020114. doi:10.1061/(asce)st.1943-541x.0002670
Rosowsky, D. V., and Ellingwood, B. R. (2002). Performance-based Engineering of wood Frame Housing: Fragility Analysis Methodology. J. Struct. Eng. 128, 32–38. doi:10.1061/(asce)0733-9445(2002)128:1(32)
Royset, J. O., Der Kiureghian, A., and Polak, E. (2001). Reliability-based Optimal Structural Design by the Decoupling Approach. Reliability Eng. Syst. Saf. 73, 213–221. doi:10.1016/s0951-8320(01)00048-5
Schuëller, G. I., and Jensen, H. A. (2008). Computational Methods in Optimization Considering Uncertainties - an Overview. Comput. Methods Appl. Mech. Eng. 198, 2–13.
Schuëller, G. I., Pradlwarter, H. J., and Koutsourelakis, P. S. (2003). “A Comparative Study of Reliability Estimation Procedures for High Dimensions,” in Proceedings of the 16th ASCE Engineering Mechanics Conference, Seattle, WA.
Spence, S. M. J., and Gioffrè, M. (2012). Large Scale Reliability-Based Design Optimization of Wind Excited Tall Buildings. Probabilistic Eng. Mech. 28, 206–215. doi:10.1016/j.probengmech.2011.08.001
Spence, S. M. J., and Kareem, A. (2014). Performance-based Design and Optimization of Uncertain Wind-Excited Dynamic Building Systems. Eng. Structures 78, 133–144. doi:10.1016/j.engstruct.2014.07.026
Spence, S. M. J. (2018). Optimization of Uncertain and Dynamic High-Rise Structures for Occupant comfort: An Adaptive Kriging Approach. Struct. Saf. 75, 57–66. doi:10.1016/j.strusafe.2018.05.008
Structural Engineers Association of California (SEAOC) (1995). Vision 2000 - A Framework for Performance Based Design. Sacramento, CA: Tech. rep., vol. I, II, III.
Subgranon, A., and Spence, S. M. J. (2021). Performance-based Bi-objective Optimization of Structural Systems Subject to Stochastic Wind Excitation. Mech. Syst. Signal Process. 160, 107893. doi:10.1016/j.ymssp.2021.107893
Suksuwan, A., and Spence, S. M. J. (2019a). Performance-based Bi-objective Design Optimization of Wind-Excited Building Systems. J. Wind Eng. Ind. Aerodynamics 190, 40–52. doi:10.1016/j.jweia.2019.03.028
Suksuwan, A., and Spence, S. M. J. (2019b). Performance-based Design Optimization of Uncertain Wind Excited Systems under System-Level Loss Constraints. Struct. Saf. 80, 13–31. doi:10.1016/j.strusafe.2019.03.004
Suksuwan, A., and Spence, S. M. J. (2018). Performance-based Multi-hazard Topology Optimization of Wind and Seismically Excited Structural Systems. Eng. Structures 172, 573–588. doi:10.1016/j.engstruct.2018.06.039
Tabbuso, P., Spence, S. M. J., Palizzolo, L., Pirrotta, A., and Kareem, A. (2016). An Efficient Framework for the Elasto-Plastic Reliability Assessment of Uncertain Wind Excited Systems. Struct. Saf. 58, 69–78. doi:10.1016/j.strusafe.2015.09.001
Taflanidis, A. A., and Beck, J. L. (2009). Stochastic Subset Optimization for Reliability Optimization and Sensitivity Analysis in System Design. Comput. Structures 87, 318–331. doi:10.1016/j.compstruc.2008.12.015
Tamura, Y., Yasui, H., and Marukawa, H. (2001). Non-elastic Responses of Tall Steel Buildings Subjected to Across-Wind Forces. Wind and Structures 4, 147–162. doi:10.12989/was.2001.4.2.147
Tsujita, O., Hayabe, Y., and Ohkuma, T. (1998). “A Study on Wind-Induced Response for Inelastic Structure,” in ICOSSAR 97: Proceedings of the 7th International Conference on Structural Safety and Reliability, Kyoto, Japan, 1359–1366.
Unnikrishnan, V. U., and Barbato, M. (2017). Multihazard Interaction Effects on the Performance of Low-Rise wood-frame Housing in hurricane-prone Regions. J. Struct. Eng. 143, 04017076. doi:10.1061/(asce)st.1943-541x.0001797
Unnikrishnan, V. U., and Barbato, M. (2016). Performance-based Comparison of Different Storm Mitigation Techniques for Residential Buildings. J. Struct. Eng. 142, 04016011. doi:10.1061/(asce)st.1943-541x.0001469
Valdebenito, M. A., and Schuëller, G. I. (2010). A Survey on Approaches for Reliability-Based Optimization. Struct. Multidisc Optim 42, 645–663. doi:10.1007/s00158-010-0518-6
Valdebenito, M. A., and Schuëller, G. I. (2011). Efficient Strategies for Reliability-Based Optimization Involving Non-linear, Dynamical Structures. Comput. Structures 89, 1797–1811. doi:10.1016/j.compstruc.2010.10.014
Vamvatsikos, D., and Cornell, C. A. (2002). Incremental Dynamic Analysis. Earthquake Engng. Struct. Dyn. 31, 491–514. doi:10.1002/eqe.141
Venanzi, I., Ierimonti, L., and Caracoglia, L. (2020). Life-cycle-cost Optimization for the Wind Load Design of Tall Buildings Equipped with Tmds. Wind & Structures 30, 379–392.
Vickery, B. J. (1970). Wind Action on Simple Yielding Structures. J. Engrg. Mech. Div. 96, 107–120. doi:10.1061/jmcea3.0001221
Wang, Y., Burgess, I., Wald, F., and Gillie, M. (2012). Performance-based Fire Engineering of Structures. Boca Raton, Florida, USA: CRC Press.
Whittaker, A., Hamburger, R., and Mahoney, M. (2003). “Performance-based Engineering of Buildings for Extreme Events,” in Proceedings of the AISC-SINY Symposium on Resisting Blast and Progressive Collapse (New York, NY: American Institute of Steel Construction), 55–66.
Wyatt, T. A., and May, H. I. (1971). “The Ultimate Load Behavior of Structures under Wind Loading,” in Proceedings of the 3rd International Conference on Wind Effects on Buildings and Structures, Tokyo, Japan, 501–510.
Yang, T. Y., Moehle, J., Stojadinovic, B., and Der Kiureghian, A. (2009). Seismic Performance Evaluation of Facilities: Methodology and Implementation. J. Struct. Eng. 135, 1146–1154. doi:10.1061/(asce)0733-9445(2009)135:10(1146)
Zhu, P., Zhang, Y., and Chen, G. (2011). Metamodeling Development for Reliability-Based Design Optimization of Automotive Body Structure. Comput. Industry 62, 729–741. doi:10.1016/j.compind.2011.05.008
Keywords: performance-based wind engineering, hurricanes, building envelopes, probabilistic damage and loss modeling, extreme winds, performance-based design optimization
Citation: Spence SMJ and Arunachalam S (2022) Performance-Based Wind Engineering: Background and State of the Art. Front. Built Environ. 8:830207. doi: 10.3389/fbuil.2022.830207
Received: 06 December 2021; Accepted: 09 February 2022;
Published: 10 March 2022.
Edited by:
Gregory A Kopp, Western University, CanadaReviewed by:
Luca Caracoglia, Northeastern University, United StatesCopyright © 2022 Spence and Arunachalam. This is an open-access article distributed under the terms of the Creative Commons Attribution License (CC BY). The use, distribution or reproduction in other forums is permitted, provided the original author(s) and the copyright owner(s) are credited and that the original publication in this journal is cited, in accordance with accepted academic practice. No use, distribution or reproduction is permitted which does not comply with these terms.
*Correspondence: Seymour M. J. Spence, c21qc0B1bWljaC5lZHU=
Disclaimer: All claims expressed in this article are solely those of the authors and do not necessarily represent those of their affiliated organizations, or those of the publisher, the editors and the reviewers. Any product that may be evaluated in this article or claim that may be made by its manufacturer is not guaranteed or endorsed by the publisher.
Research integrity at Frontiers
Learn more about the work of our research integrity team to safeguard the quality of each article we publish.