- 1Department of Engineering Technology, Faculty of Technology, University of Jaffna, Kilinochchi, Sri Lanka
- 2Division of Sustainable Resources Engineering, Graduate School of Engineering, Hokkaido University, Sapporo, Japan
- 3Division of Sustainable Resources Engineering, Faculty of Engineering, Hokkaido University, Sapporo, Japan
Microbial Induced Carbonate Precipitation (MICP) has recently become a new technology for stabilizing the slope surface. The applicability of MICP, however, is limited in fine-grained soils due to the restrictions regarding the transportation of bacteria cells. The purpose of this study was to assess the feasibility of an alternative called Bacterial-Enzyme Induced Carbonate Precipitation (B-EICP) for stabilizing the fine-grained slope soils. Unlike the MICP strategy (involving whole-cells of bacteria), the proposed B-EICP utilizes bacterial urease to induce the bio-cement formation within soil. The whole-cell culture of Lysinibacillus xylanilyticus was subjected to cyclic sonication to extract the free urease suspension. The B-EICP treatment was performed to the columns prepared using two different soils obtained from representative expressway slopes. The cementation responses of the proposed B-EICP were compared with that of typical MICP method, especially from the following viewpoints, (i) adaptability to soil with high fine-grained content, (ii) conditions under which B-EICP can be effectively applied and (iii) cementation under low temperature. The results revealed that the extract solution had higher urease activity compared to original bacteria culture, and the activity remained more stable at low temperature conditions (15°C). The results further confirmed that B-EICP method is more suitable for stabilizing soils with large amount of fine particles. Comparing with MICP, the B-EICP resulted higher unconfined compressive strength (over 1200 kPa) and deeper cementation in the silty sand. Microscale analysis suggested that the B-EICP could induce smaller calcium carbonate crystals than that by MICP, but the number of crystals in B-EICP were significantly more, thus contributed to increased particle-particle cementation.
1 Introduction
Due to the increasing awareness of energy crisis and environmental protection in the field of Geotechnical Engineering, the demand for sustainable technologies for soil stabilization continues to increase. Bio-cementation is an emerging soil stabilization technology that relies on environmentally friendly biogeochemical processes. Microbial induced carbonate precipitation (MICP) and Enzyme induced carbonate precipitation (EICP) are the two most common and widely investigated bio-cementation techniques. It should be noted that there are already several hundred articles published on the application of MICP and EICP for various geotechnical purposes (Ivanov and Chu, 2008; Liu et al., 2020; Omoregie et al., 2020; Sharma et al., 2022). The MICP technology utilizes living bacteria cells containing intra-cellular urease to mineralize the calcium carbonate (Dejong et al., 2013; Sun et al., 2020). On the other hand, in the EICP technology, free urease enzymes are used in the place of ureolytic bacteria (Hamdan and Kavazanjian, 2016; Almajed et al., 2018). In both the technologies, bacteria or enzymes that are introduced to a target soil catalyse the hydrolysis of supplied urea and produce ammonium and carbonate ions. In the supply of media containing calcium ions (e.g., CaCl2), the calcium carbonate mineralizes inside the pores of the soil, binds the soil particles together at particle-particle contact points and enhances the engineering properties of soil (Montoya and de Jong, 2015; Lin et al., 2016). The chemical reactions involved in the technologies are listed below,
After the recognition of bio-cementation methods in the fields of Geotechnical and Environmental Engineering, several researchers attempted to implement the methods for stabilizing the slopes and surfaces, provided beneficial outcomes to the scientific community. It should be noted that the MICP has been their major focus, thus far. For instance, Salifu et al. (2016) applied the MICP treatment to small-scale sandy slopes and demonstrated the surface stability against simulated tidal waves. Jiang and Soga (2017) quantitatively studied the responses of gravel-soil mixtures under seepage-induced internal erosion, demonstrated the influence of precipitation content on sand erosion. Using a set of bench-scale models, the surface erosion of sandy slopes was investigated under simulated rainfall by Jiang et al. (2019). In another study, Gowthaman et al. (2019) proposed the use of native bacteria for the preservation of an expressway slope surface, rather using specialized bacterial strains such as Sporoscarcina pasteuri. The researchers emphasized that introducing non-native bacteria into natural soil ecosystems would possibly result in economic challenges and uncertainty regarding ecological consequences bacteria. Few researchers evaluated the feasibility of implementing the MICP treatment for the mitigation of coastal sand dunes (Liu et al., 2020; Imran et al., 2022). Moreover, the longevity of MICP treated slope surface under possible environmental deteriorations such as cyclic freeze-thaw actions (Gowthaman et al., 2020), cyclic wet-dry actions (Sharma and Satyam, 2021; Gowthaman et al., 2022) and acid-rain induced losses (Gowthaman et al., 2021b) was also comprehensively studied in the recent past.
In spite of the considerable interest in MICP, there are no studies found on the deployment of MICP in stabilizing fine-grained slope soils. Almajed et al. (2018) once reported that the MICP generally cannot be applied in soils with pore throats smaller than the ureolytic bacteria, limiting its applicability in fine-grained soils. The prime factor that governs the microbial transport within the soil is the size of pore throats through which the cells should pass (Mitchell and Santamarina, 2005). Narrow pore throats formed by fine soil grains prevent their transport from one pore to another, filtering the cells at near-surface. In recent years, the EICP has been suggested as a means to eliminate the limitations associated with the use of bacteria cells (Putra et al., 2016; Almajed et al., 2018; Hoang et al., 2019). The small size (12 nm per subunit) of solubilized urease enzyme provides distinct advantage over ureolytic bacteria during the transport through narrow pore spaces of fine-grained soils. Moreover, unlike the MICP, there is no need in EICP to pay serious attention on nutrients provision, competing effects, bio-safety and oxygen availability (Hoang et al., 2019; Putra et al., 2020).
The literature survey suggests that the free urease enzymes that were used in the EICP research works are agriculturally-derived, i.e., from the sources such as soybeans, jack beans, watermelon seeds, etc., either in the purified form or crude form. Jack bean is the most commonly used urease source in EICP studies; however, the activity of the extract might vary depending on the state and conditions that the extraction was carried out (Almajed et al., 2020, 2018). The outcomes of the study performed by Imran et al. (2021) disclosed that the urease derived from germinated watermelon seeds consists of higher activity compared to that from normal seeds. In fact, the commercially available enzymes are very expensive, produced at high purity levels for the use of specific purposes such as food industry, drug industry and bio-medical applications. Their cost was once reported as 10 million JPY per kilogram (Gowthaman et al., 2021c). It is therefore manifest that the use of commercial enzymes is not affordable in real-scale applications in terms of the cost.
Keeping the limitations of both MICP and EICP methods in mind, this study was performed with the purpose of evaluating the feasibility of Bacterial-Enzyme Induced Carbonate Precipitation (B-EICP) for stabilizing the fine-grained soils obtained from representative expressway slope. Worth mentioning that the proposed B-EICP offers an alternative pathway for typical EICP, involving bacterial urease extracted through sonication process, rather the use of expensive-commercial enzymes and limitedly-available plant-based enzyme sources. For that, the culture of Lysinibacillus xylanilyticus was subjected to cyclic sonication to extract urease suspension. As recommended in many previous feasibility studies (Almajed et al., 2018; Gowthaman et al., 2019), soil columns were subjected to B-EICP treatment by anticipating that the tested columns represent the surface zone of the slope in 1-Dimension. The responses of the proposed method were compared with the typical MICP from the following viewpoints, (i) adaptability to soil with high fine-grained content, (ii) conditions under which B-EICP can be effectively applied and (iii) cementation under low-temperature. The evaluation program was based on the needle penetration tests, measurements of cement content, scanning electron microscopy (SEM), energy dispersive spectroscopy (EDS) and X-ray diffraction (XRD) analyses, and the outcomes are discussed in subsequent sections.
2 Materials and methods
2.1 Bacteria culturing, sonication, and enzyme extraction
The ureolytic bacterium used in this research work was Lysinibacillus xylanilyticus. The bacterium was earlier isolated by Gowthaman et al. (2019) from Hokkaido expressway slope, Japan. Pre-sterilized Beer Yeast (BY) was used as the culture medium for cultivating Lysinibacillus xylanilyticus. For the preparation of BY medium, 4 g of NICHIGA beer yeast powder was subjected to stirring in 100 ml distilled water at 600 rpm for 24 h, followed by the solution was centrifuged at 8000 rpm to eliminate the undissolved solid particles. The solution was then autoclaved to reduce the risk of culture contamination. The bacteria were precultured in 5 ml medium under shaking incubation at 25°C and 160 rpm for 24 h. 1 ml of the preculture was inoculated into 100 ml of fresh BY medium and incubated at 25°C and 160 rpm. Sufficient growth of the bacteria was ensured by measuring the intensity of the culture under the wave length of 600 nm (OD600). It should be noted that the optimal growth of Lysinibacillus xylanilyticus is typically attained after 48 h of incubation.
Prior to the sonication, the bacteria culture was centrifuged at the conditions of 25°C and 160 rpm for 5 min, and the supernatant solution was replaced with lysis buffer of pH 8.0. Through a series of cyclic sonication steps (i.e., 1 min “on” followed by 1 min “off” - for a 5-min period), ultrasonic crushing was performed to the bacteria culture by placing it directly into the sonication chamber. Previous studies indicated that continuous sonication leads to the temperature buildup in the culture medium, hence resulting in enzyme denaturation (Mawson et al., 2011). Therefore, to curtail the temperature buildup and to impose a minimal damage to free enzymes, the cyclic sonication was preferably adopted.
The anticipated changes in the bacteria culture during the sonication is graphically illustrated in Figure 1. Following the sonication process, to separate residual cellular debris (i.e., solids) from the extracted urease, the solution was subjected to a centrifugal force of 11,900 rpm for 10 min. The extracted enzyme solution was then diluted using the lysis buffer equivalent to the initial volume of the bacteria culture. The activity of the extracted urease was determined using the method described in the subsequent section.
2.2 Measuring the activity of extracted bacterial enzyme
The activity of extracted urease was measured using spectrophotometric determination of ammonia as indophenol (Bolleter et al., 1961). 1 ml of the solution was subjected to the reaction with 0.1 mol/L urea prepared in phosphate buffer solution. The phenol, in the presence of hypochlorite, reacted with ammonium ions produced as the result of urea hydrolysis (refer Eq. 1) and produced a blue color dye (also referred to as indophenol dye). The dye with different intensity indicated the concentration of ammonium ions produced during the reaction. At every 5 min interval, the intensity of the dye was measured using spectrophotometry at the wave length of 630 nm, and the rate of urea hydrolysis was estimated using the calibration curve developed between the concentration of ammonium ions and intensity. The activity of extracted urease is expressed in terms of urea hydrolysis rate.
2.3 Soil materials and treatment procedures
The soils obtained from two erosion-prone expressway slopes of Hokkaido were essentially involved in this research work. Hokkaido is the northmost island and the coldest region of Japan. Both the sampling locations are indicated in Figure 2A. One was sampled from Watsu area of Kitahiroshima (42°57′00.0″N, 141°30′49.0″E), which is hereinafter referred to as Watsu soil. The other one was from the expressway slope located in Onuma area (42°23′18.7″N, 140°17′05.1″E), which is hereinafter referred to as Onuma soil. Sieve analysis was conducted to both soils for determining the grain size distributions. The grain size distributions of the soils are depicted in Figure 2B. The results showed that the fine content (<0.075 mm, passed through the Sieve No. 200) in Watsu soil was quite high - around 17%, while the fine content was only 3% in Onuma soil. It should be noted, despite different gradation, the mean particle diameters of both the soils were nearly the same, around 0.22 mm. As per the American Association of State Highway and Transport Officials (AASHTO) soil classification system, the Watsu and Onuma soils are categorized as silty sand (A-2-5) and fine sand (A-3), respectively.
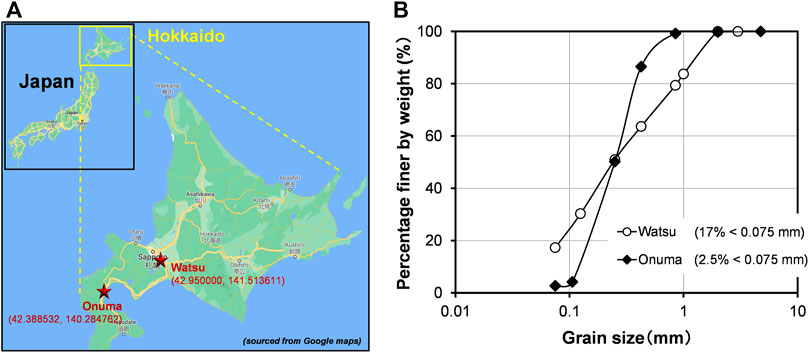
FIGURE 2. (A) Map indicating the sampling locations and coordinates of the expressway slopes and (B) the grain size distribution curves of the slope soils.
The soils were initially washed with deionized distilled water to remove soluble chemicals, followed by the oven-drying at 100°C for 24 h before being treated. Number of different soil columns were prepared using both soils; for that, syringe columns with 30 mm diameter and 60 mm height dimensions were used. Prior to the preparation, the filter layer was placed at the lower end (outlet) to prevent the loss of soil particles during the treatment. The soils were packed into the columns in three successive layers with equal thickness of nearly 20 mm per layer. Dry-tamping was then applied, i.e., each layer was gently and consistently tamped by using a steel rod.
The prepared soil columns were subjected to two different types of treatments: (i) proposed B-EICP and (ii) conventional MICP. In both the treatments, a two-phase injection strategy was adopted: an initial injection of biological solution, followed by multiple injections of cementation solution. For B-EICP, the biological solution was extracted urease, whereas the bacteria culture (whole cells) was injected for the case of MICP. Subsequently, the cementation solution which consisted of calcium chloride (1 mol/L), urea (1 mol/L) and nutrient broth (3 g/L) was injected. The outlets were remained opened throughout the treatment. All the solutions were simply injected at the top of the soil columns at the rate of 4–5 ml/min and allowed to percolate under gravity and capillary effects. As recommended in many previous works (Feng and Montoya, 2016; Hoang et al., 2019), a 2-h gap was permitted between biological and cementation injections, which allowed the biological substances (either bacteria for MICP or extracted urease for B-EICP) to attach or sorb onto the surface of soil particles. Different test cases were adopted to investigate different treatment phenomena, and the detail of all the test cases are listed in Table 1. The triplicate specimens were tested in each case. C-0-1 (W) and C-0-2 (O) are the control cases of Watsu and Onuma soils, where the distilled water was injected in the place of biological solution.
By the end of the treatment, the soils columns were percolated by tap water (with the volume equivalent to around 1 L) to eliminate the unreacted and soluble chemicals and salts. The syringe molds were then cut, and the soil columns were carefully taken out. The specimens were ready for further testing which are explicated in subsequent sections.
2.4 Needle penetration test
Needle penetration is a reliable test to indirectly determine the unconfined compressive strength (UCS) of cemented soil specimens (Ulusay and Erguler, 2012). SH-70 needle penetrometer (Manufacturer - Maruto Testing Machine Company, Tokyo) was used in accordance with the standards recommended by the Japanese Geotechnical Society (JGS, 2012). The cemented specimen was positioned laterally, followed by the needle fixed onto the device was subjected to the penetration into cylindrical surface of the specimen. For every specimen, three number of penetrations were performed—top, middle and bottom (respectively, at 1 cm, 3 cm and 5 cm depths measured from the injection surface). During each test, readings such as penetration resistance (measured in N) and penetration depth (measured in mm) were obtained, and the UCS was determined using the developed regression relationship between UCS and needle penetration ratio.
2.5 Determination of CaCO3 content
The precipitated CaCO3 content was measured by acid reaction method (Fukue et al., 1999). The oven-dried (60°C for 48 h) representative sample of known weight was placed into a closed chamber and reacted with concentrated HCl under constant volume and temperature conditions. When the CaCO3 gets into contact with HCl, the dissolution occurs, which results in the formation of CO2 gas. The internal pressure variations during the reaction were monitored using the digital manometer fabricated with the system. From the pressure reading obtained during the reaction, mass of the existed CaCO3 was determined using the developed calibration curve, hence the content of CaCO3 was estimated using the Eq. 3.
2.6 Scanning electron microscopy, energy dispersive spectroscopy and X-ray diffraction
The representative samples were dried at 60° for 48 h and preserved for SEM, EDS and XRD analyses. Using energy dispersive X-ray fluorescence spectrometer equipped with SEM (JSM-IT200(JEOL), Tokyo, Japan), the SEM-EDS was performed at an accelerating voltage of 15 kV. The preserved segments were coated using carbon coater (EC-32010CC(JEOL), Tokyo, Japan) prior to the analysis. XRD analysis was carried out to the powdered samples using the diffractometer (MultiFlex-Rigaku, Tokyo, Japan) equipped with CuKα X-ray source (operating at 40kV and 40 mA). The testing was performed at a scan rate of 6.5°/min and at angles from 5° to 70° (2θ).
2.7 Statistical analysis
To determine the significance of differences between the means of obtained experimental sets, the experimental results were analyzed using R Statistical Package (version 4.0.3). The p value less than 0.05 (i.e., p < 0.05) was considered as the significance range.
3 Results and interpretations
3.1 Activity of the urease extract
Figure 3 presents the comparison between activities of the bacteria culture (whole cell) and extracted enzyme, i.e., measured immediately before and after sonication (at room temperature conditions). The activity of the bacteria culture was 2.86 ± 0.63 U/mL, and the activity of the urease extract (3.40 ± 0.17 U/mL) revealed an increase around 18% compared to that of original bacteria culture (p = 0.2251). The above increase in activity could possibly be attributed to the reduced transport constraints of urea molecules (substrate) through cell membrane. During the sonication, the rupture of the bacteria cell membrane occurs, resulting in the release of free urease in the solution. This surges the exposure of substrate to urease enzymes that are suspended freely in solution, rather than those localized inside the cell membrane. Hoang et al. (2019) earlier pointed out that the sonication (cyclic run-cool process) typically causes around 20% evaporation of the solution, resulting in a considerable increase in activity. It should be noted that to avoid the impact of the evaporation, the sonicated solution was diluted herein to be equivalent to the initial volume of the bacteria culture.
Following the sonication, the urease extracts were stored at different temperatures (15–35°C), and the activity of the urease extract with the storage time are presented in Figure 4. The observation indicates that there is a general decrease in the activity of urease extract with the time, which might be attributed to the enzyme denaturation. As well known, the urease is composed of protein subunits and could naturally be broken down into amino acids (Mobley et al., 1995). The interesting finding here is that with the decrease in storage temperature, the rate of drop in the activity is observed to decrease. For instance, a sharp drop could be seen at the beginning for the temperatures 35 and 30°C, while it is inconspicuous for the temperature of 15°C. The initial activity measured at 35°C was 3.5 U/ml, and at the end of the storage time of 7 days, the extract almost (>95%) loses its activity (refer to Figure 4). On the other hand, the loss in the activity was only around 35% for the extract stored at 15°C, indicating high stability of the urease at low temperature.
3.2 Cementation responses of bacterial-enzyme induced carbonate precipitation and microbial induced carbonate precipitation on fine sand
The cases X-1 (O) and X-3 (O) were respectively subjected to MICP and B-EICP treatments to evaluate the cementation responses on fine sand. All the testing conditions are summarized in Table 1. It should be noted that all the conditions are identical in both the cases except the form of biological solution, i.e., the whole-cell bacteria culture and extracted urease were used for the biological injection in MICP and B-EICP, respectively. Figure 5 presents the comparison of the MICP and B-EICP methods on fine sand in terms of UCS. It could be seen that the MICP produced apparently a higher UCS compared to the B-EICP treatment for the identical treatment cycles (p = 0.0001, 0.0069 and 0.0069 for top, middle and bottom, respectively). Another notable observation is that the UCS value in the MICP case was higher at the injection point (i.e., specimen top), and that decreases with the depth of the specimen (the physical appearance of the MICP treated sand is shown in Figure 6A). The tendency observed here is consistent with many prior MICP studies (Whiffin et al., 2007; Feng and Montoya, 2016; Gowthaman et al., 2020). This can be explained by the following two reasons: (i) filtration of the bacteria and (ii) utilization of the resources. During the biological injection, the bacteria cells that transported through pore spaces tend to position themselves at particle-particle contact points via filtration (DeJong et al., 2010). During the percolation of bacterial culture, greater concentrations of the microbes were reported to retain near the injection port compared to those at distances (Harkes et al., 2010; Martinez et al., 2013). At the same time, the zone closest to the injection port is exposed to significantly more urea and calcium chloride (van Paassen et al., 2010). As the result of the exposure of more reactants to greater concentration of bacteria, high content of carbonate apparently gets deposited at the specimen top. The spatial distribution of calcium carbonate in different cases are listed in Table 2, indicating around 9.7-folds higher precipitation at the top compared to the bottom.
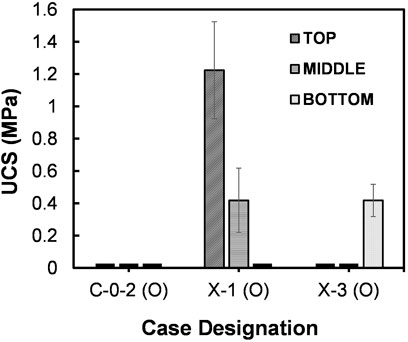
FIGURE 5. Comparison of UCS between MICP and B-EICP samples of fine sand–Onuma soil (where the case X-1 (O) is MICP, X-3 (O) is B-EICP and C-0-2 (O) is the control).
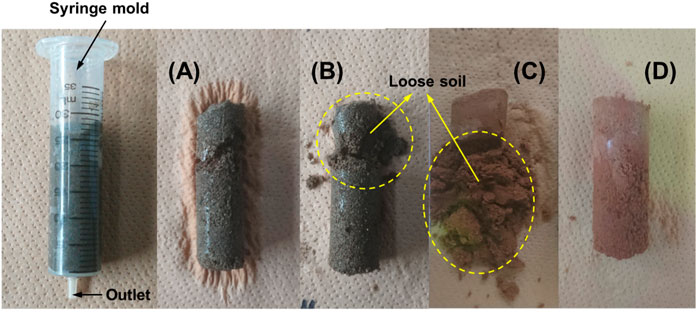
FIGURE 6. Physical appearance of the (A) MICP treated fine sand (Onuma), (B) B-EICP treated fine sand (Onuma), (C) MICP treated silty sand (Watsu) and (D) B-EICP treated silty sand (Watsu).
In the case of B-EICP, only the specimen bottom revealed the measurable UCS of around 400 kPa (refer Figure 5), while the top half of the specimen was found to be very lightly cemented. Even the loose uncemented state of the sand was evident near the specimen top (as indicated in Figure 6B). A possible explanation can be that high proportion of the urease might be flushed out through the larger void spaces of the fine sand during the injection of cementation solution. Thus, the UCS and amount of precipitated CaCO3 within the sand columns were pretty much lower compared to those observed in the case of MICP. The calcium carbonate measurements further reveal that the precipitated content in B-EICP treated fine sand was about half that of MICP under the same number of treatments. Similar observation has also been reported by Hoang et al. (2019).
3.3 Cementation responses of bacterial-enzyme induced carbonate precipitation and microbial induced carbonate precipitation on silty sand
Cemented column specimens of silty sand treated by MICP and B-EICP methods are shown in Figures 6C,D, respectively. The successful capability of B-EICP method to stabilize the full-column specimen of silty sand is physically seen (Figure 6D). The MICP, on the other hand, resulted the cementation only at the specimen top, for a depth of less than 2 cm (out of 6 cm full-column). The UCS results (presented in Figure 7) reveal that the highest UCS in the case of B-EICP (1.2 MPa) was achieved at the top, and the values dropped with the increasing depth of the specimen. Statistical analysis confirmed that the comparison yielded a significant difference between the UCS achieved in MICP and B-EICP cases (p = 0.0696).
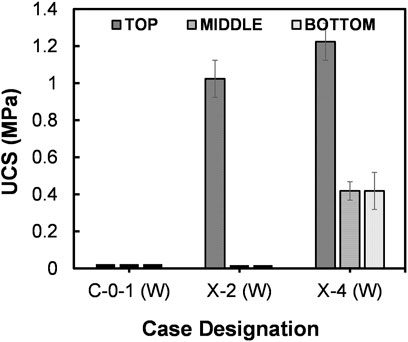
FIGURE 7. Comparison of UCS between MICP and B-EICP samples of silty sand–Watsu soil (where the case X-2 (W) is MICP, X-4 (W) is EB-EICP and C-0-1 (W) is the control).
In fact, there are two major factors that determine the retention of bacteria cells or urease, and which are (i) gradation of the soil and (ii) adsorption capacity of the bacteria or urease. The typical size of the bacteria cells ranges between 1 and 8 μm (Mitchell and Santamarina, 2005), while the size of an enzyme is reported to be nearly 12 nm (Blakeley and Zerner, 1984). In the case of silty sand or well graded soil, the pore throat sizes are pretty much smaller, having a high filtration capacity. Therefore, the larger bacteria cells are more likely to get trapped on the surface zone rather penetrating onto such soils. As the result, the MICP case showed the improvement only at the surface zone.
When the extracted urease is used in the place of bacteria cells, the UCS and the depth of cementation were considerably higher (Figure 7). Worth mentioning that since the free enzymes are in the scale of nanometers, they could possibly be transported even through smaller pore throats with minimal seepage forces. In the meantime, the enzymes are either filtered at the particle-particle contacts or adsorbed at the soil surface, enabling their possible distribution throughout the column depth. It should be stated that the adsorption capacity of the bacteria cells or urease rely on their biological nature such as the charge of the substance, shape and surface roughness (Zita and Hermansson, 1994).
3.4 Cementation responses under various injection volumes and frequencies
Many previous studies confirmed that the biological treatments are strategy-dependent (Cheng et al., 2019; Tang et al., 2020; Sharma et al., 2021). The UCS of the specimens treated under various strategies are compared in Figure 8. Figure 8A presents the comparison between two cases treated with different frequencies of biological injection. In the case of X-4 (W), the enzyme extract was injected once in 7 days, and the injection was once in 4 days for X-8 (W). The outcome indicated that a 2.8-fold increase in UCS was achieved at the top when the injection frequency was increased (p = 0.0001); however, the uniformity of cementation appears to decrease. This could possibly be attributed to the influence by the previously formed calcite crystals. As reported by Whiffin et al. (2007), the calcium carbonate starts to form immediately after the injection of cementation media, and the formation within pore spaces tends to narrow the pore throats while increasing the roughness of soil surface. This results in diffusion barrier to the urease injected in subsequent rounds. When the urease extract was injected frequently [in the case of X-8 (W)], high concentration of urease got adsorbed at or near surface, which led to the rapid formation of calcium carbonate there. This was evident by the clear improvement in UCS at the specimen top. Amarakoon and Kawasaki (2018) also observed a higher UCS value at the specimen surface when the biological injection was performed on everyday-basis compared to that performed on once in a two days-basis.
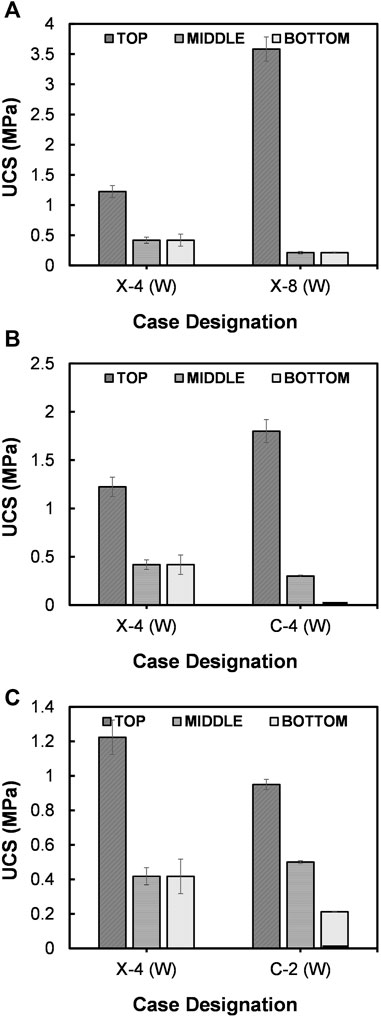
FIGURE 8. Comparison of UCS between the cases treated under (A) different injection frequencies of biological solution, (B) different injection frequencies of cementation media and (C) different injection volumes of cementation media.
The increase in injection frequency of cementation media also resulted the increase in UCS values in B-EICP treatment (refer Figure 8B). However, the profile of UCS appears to become less homogeneous over the column length when the cementation media is injected every 12-h [Case C-4 (W)]. It was earlier reported that shorter injection intervals lead to the formation of smaller crystals in bluk, which are perhaps irregularly shaped and combined of vaterite and amorphous precipitates (Wang et al., 2019). This might possibly be the reason of observed UCS buildup at the surface of C-4 (W) case (Figure 8B). Long injection intervals (23–25 h) are typically recommended owing to the potential formation of large carbonate crystals which provide effective bonding (Qabany et al., 2011).
In another case [C-2 (W)], the volume of injected cementation media was increased to 20 ml, and the UCS results are compared in Figure 8C. A slight decrease in the UCS could be seen for the case treated by 20 ml cementation media [C-2 (W)] when compared with that treated by 10 ml [X-4 (W)]. A possible explanation is that a large proportion of urease were flushed out during the increased injection of cementation media. Nevertheless, a similar gradient was evident in both the profiles, with relatively high UCS values at the injection top that decreased over the depth.
3.5 Cementation responses under low temperature treatment
As discussed earlier, the temperature is one of the key factors that govern the response of urease. Following the observation in Figure 4, a set of B-EICP cases (refer Table 1) were treated at 15°C, and the UCS responses are compared in Figure 9 together with that of the B-EICP case treated at 25°C [X-4 (W)]. Under same injection phenomena, the case treated at 15°C [C-3 (W)] showed a better surficial strength, i.e., with 25% increment than that at 25°C [X-4 (W)] (p = 0.4279), and which could be attributed to the increased stability of the urease under low temperature conditions. Furthermore, when the injection frequency of biological solution and cementation solution were increased [the cases C-5 (W) and X-10 (W)], the surficial UCS promoted further, suggesting an increase of around 3.3-fold and 8-fold, respectively (p = 0.0005 and 0.0001, respectively).
4 Discussion
From the results presented above, it is perceived that the B-EICP method has a potential to offer a reliable pathway for EICP method while eliminating the challenges associated with typical MICP method. The activity of the extract solution was measured to be 3.4 ± 0.17 U/mL (Figure 3), which indicated two important information, (i) the sonication was successful in deriving the intra-cellular enzymes in free suspension form without destructing their functionality and (ii) the extract solution was found to have a higher urease activity compared to the original whole-cell culture. The turbidity of the bacteria culture (OD600) prior to the sonication was 3.0 ± 0.2, while the turbidity of extract solution was 0.3 ± 0.1. This suggest that almost all the ruptured cells were removed during the centrifuging process.
Another notable finding is that the derived urease enzymes were more likely to be stable under low temperature condition (i.e., 15°C) (Figure 4). Our previous study, on the other hand, suggested that the activity of the bacteria used in this study (Lysinibacillus xylanilyticus) was more stable under the room temperature (25°C), and at 15°C, the activity of the species was found to be trivial. Similarly, many previous studies unveiled that the activity of the bacteria (such as Sporosarcina pasteurrii, Pararhodobacter sp., etc.) is higher at moderate to high temperatures, and which rapidly drops with the decrease in temperature, particularly below 20°C (Whiffin, 2004; Fujita et al., 2017). The proposal to use the extracted bacterial urease is therefore highly compatible and beneficial for stabilizing the slopes located in cold regions like Hokkaido, Japan.
The observation verified that the effectiveness of B-EICP and MICP would vary depending on the soil to be treated. From the UCS results (Figures 5, 6), it is perceived that the B-EICP treatment is less compatible for stabilizing sandy soils (i.e., uniformly graded), while it appears to suit very well for silty sand (i.e., the slope soil with higher fine-grained content). Figure 10 compares the SEM images for the MICP and B-EICP treated fine sand. In both cases, the formation of calcium carbonate crystals was evident. By closely observing the crystals, the shape of the crystals in both the cases can be said to be the same - smaller cubic or prismatic shaped. However, two major differences have been observed between the cases. Firstly, the size of the crystals is found to be different in both cases. In the case of MICP (Figure 10A), the calcium carbonate was found to mineralize as larger clusters, while it was formed as many smaller crystals in B-EICP treatment (Figure 10B). The observations are consistent with the report by Almajed et al. (2019). The difference in the crystal size can be explained by two reasons: (i) size of the bacteria cells or enzyme and (ii) the rate of crystallization. The size of the bacteria cell (in the scale of μm) is over 200-fold higher than the enzyme (in the scale of nm); thereby, more or less of the size of the substance (that provides nucleation site) would in turn result in the formation of larger or smaller crystals, respectively. Since the rate of urea hydrolysis in the case of B-EICP is shorter than that of MICP case (as per Figure 3), the rate of CaCO3 crystallization in B-EICP would be faster. The faster the crystallization rate results in smaller the crystals (Tang et al., 2020). Secondly, the location of the crystals is also different. The crystals were formed at the particle-particle contact points and on the grain surface, respectively in the cases of MICP and B-EICP. This is due to the fact that the larger bacteria cells are filtered at particle contact points, while a proportion of smaller enzymes got attached all over the grain surface. It must be mentioned that the crystals formed between the adjacent soil particles is the one that contributes to the enhancement of strength (Lin et al., 2016). The crystals formed in B-EICP exhibit no connections to develop force chain between adjacent particles, thus seeming to be ineffective to produce UCS.
Figure 11 compares the micrographs for the MICP and B-EICP treated silty sand. In the case of MICP (Figure 11A), the crystals are not clearly recognizable, suggesting that the formed crystals are below the detectable threshold amount in the representative sample obtained from the middle of the column specimen. This is due to the reason that the transport of bacterial cells was restricted through narrow pores of the soil. On the other hand, in the case of B-EICP (Figure 11B), the smaller crystals were found in bulk all over the soil matrix, localized almost everywhere including grain contact points as well as grain surface. Cui et al. (2020) once reported that formation of more cementation points of calcium carbonate would produce more strength in EICP treatment, which immensely pronounced in the measured UCS values (refer Figure 7).
The SEM images with EDS analysis depicted in Figure 12 show the distribution of calcium (Ca) with different testing cases. The Figures 12A–C corresponds to the control soil, the soil treated by EICP under 25°C and the soil treated by B-EICP under 15°C, respectively. It should be noted that there is no difference in the crystal morphology between the B-EICP cases treated under 25°C and 15°C. However, the spectrum highlights elucidate that the quantity of precipitated calcium carbonate more likely to increase with the decrease in treatment temperature, corroborating the propensity observed in both the UCS and measured cement content. Other than the temperature, the treatment phenomena of both the cases were identical. Therefore, the possible reason behind the observation is the persistence of higher enzymatic activity for longer periods at 15°C than that of at 25°C. In most regions of the world, the peak value of the soil temperature is not greater than 30°C, and the soil temperature (exclusive of winter season) in Hokkaido also ranges between 5°C and 25°C with considerable day-night fluctuations (Farukh and Yamada, 2018). It was earlier anticipated that the neither MICP or EICP would be applicable for the slopes located in cold regions (like Hokkaido, Japan). The finding is, therefore, in significant and opens up a potential pathway to implement the bio-cementation.
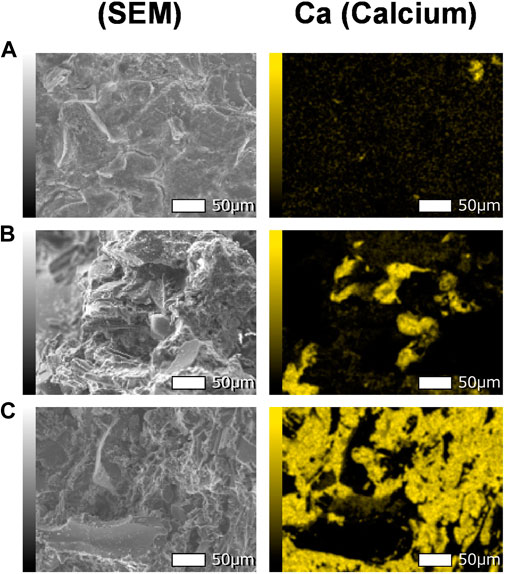
FIGURE 12. The SEM-EDS spectrum highlights (for Ca) for (A) untreated silty sand (Watsu), (B) B-EICP treated silty sand (Watsu) at 25°C and (C) B-EICP treated silty sand (Watsu) at 15°C.
The XRD spectra shown in Figure 13 demonstrate that the calcium carbonate that precipitated during the treatment is calcite, irrespective of the cases. Figure 13A presents the XRD results of untreated silty sand (Watsu). The major component of the soil was found to be quartz. Figures 13B,C are respectively the MICP and B-EICP treated silty sand. Even under low temperature conditions, calcite was formed alone. Wang et al. (2019) reported that the calcite (typically forms in rhombohedral shape) is the most stable form of calcium carbonate, having higher binding strength than vaterite or aragonite. The formation of calcite and vaterite crystals were evidenced in the findings of Cui et al. (2020) for the case of MICP. In this study, however, the spherical vaterites have not been encountered. Overall, the analysis suggests that the precipitation content, crystal shape, size and the location where they formed would determine the strength characteristics of the treated fine-grained slope soil.
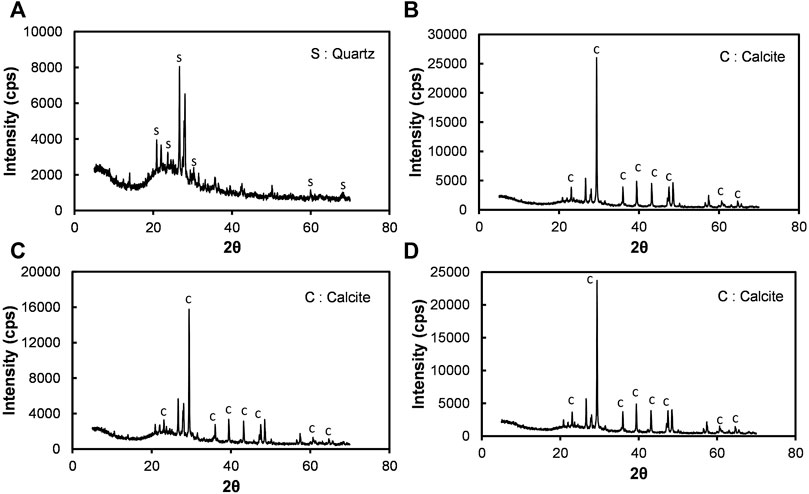
FIGURE 13. XRD analysis results of (A) untreated, (B) MICP treated, (C) B-EICP treated (25°C)and (D) B-EICP treated (15°C) silty sand (Watsu).
5 Applicability and limitations
Slope surfaces often requires appropriate treatment to withstand against erosion/aggregate transport during rainfall and snowmelt. The biological treatments aim to develop a calcite armor along the surface, which greatly prevents the surfaces from aggregate loss and excess infiltration. Adequate strength must be achieved by producing calcium carbonate bonds between soil particles to a sufficient depth. As discussed earlier, stabilizing fine-grained soils to the required depth had been a great challenge, remained a major hurdle in implementing typical biological methods for stabilizing slopes with high content of fines. Through the B-EICP method, the issues associated with the microbial transport through the smaller pores have been eliminated, hence the applicability of biological method has been expanded to fine sand and silty soils. The UCS achieved in proposed B-EICP method was comparable with those of conventional biocementation methods (MICP/EICP), satisfying threshold requirements for slope surface stabilization. The observed formation of fine calcite crystals is more likely to help in retarding the rapid clogging of pore voids, favoring the treatment of fine-grained soils. It should be noted, however, the applicability of the B-EICP method for the case of clay soils might be still a challenge due to their extremely low permeability characteristics, which may require mechanical mixing or pressurized supply of the treatment solutions, as reported in previous works (Islam et al., 2020; Gowthaman et al., 2021a). Moreover, fine-grained soils are often reported to consist of certain percentage of organic matter (Chen et al., 2022); therefore, the impact of humus on enzyme-induced cementation needs to be addressed in future works to make the technology fully feasible.
During the application, the B-EICP treatment solutions can be simply introduced at the surface with minimal equipment requirement. The spraying can be a better recommendation, which is convenient at large-scale and time-saving (Dagliya et al., 2022). The production of harmful ammonium ions is another a matter to deal carefully here; the lasting solution containing ammonium ions must be extracted from subsoil before contaminating ground water and subjected to posttreatment. Cation exchange with zeolite, precipitating ammonium as struvite and biological nitrification-denitrification are some posttreatment methods proposed for ammonium removal (Lee et al., 2019; Mohsenzadeh et al., 2021).
6 Conclusion
The paper presented an initial work performed to evaluate the feasibility of Bacterial-Enzyme Induced Carbonate Precipitation (B-EICP) for stabilizing the fine-grained slope soils obtained from representative expressway slopes. The proposed B-EICP offers an alternative pathway for typical EICP, involving bacterial urease extracted through sonication process. The following key conclusions are drawn from this study.
i) Intracellular urease enzymes could be extracted by cyclic sonication of Lysinibacillus xylanilyticus bacteria culture without disrupting the functionality. The activity of the urease extract was 3.4 ± 0.17 U/mL, which was found to be (18%) higher than that of original bacteria culture. The derived urease enzymes were more stable under low temperature conditions, i.e., 15°C.
ii) The effectiveness of B-EICP and MICP would vary depending on the gradation of slope soil to be treated. The UCS results suggested that the B-EICP treatment is less compatible for stabilizing sandy soils (uniformly graded), while it appears to suit very well for silty sand (the soil with higher fine-grained content). The highest UCS in the case of B-EICP treated silty sand was 1.2 MPa, achieved at the top; but, as expected, the UCS values dropped with the depth of the specimen. At the same time, the MICP treatment on silty sand showed an improvement only at the surface zone, and the specimen bottom remained untreated due to the restriction related to the transport of the bacteria.
iii) With the increase in injection frequencies of enzyme solution, the formation of calcium carbonate appears to increase. SEM-EDS analysis suggested that the treatment at 15°C produced more calcium carbonate compared to that at typical room temperature (25°C), indicating that the proposed B-EICP can be potentially implemented in slopes located in cold regions.
iv) Microscopy analysis showed that the B-EICP treatment in sand resulted the formation of numerous prismatic crystals on the grain surface. The MICP, on the other hand, resulted the formation of larger crystals at the particle-particle connections. The B-EICP treatment on silty sand resulted the formation of smaller crystals in bulk at both particle contacts and grain surface. X-ray spectra results suggested that regardless of the treatment cases, all the treatments could produce calcite which is the most stable form of calcium carbonate.
Data availability statement
The raw data supporting the conclusions of this article will be made available by the authors, without undue reservation.
Author contributions
All the authors made direct and intellectual contribution to the reported research work. SG planned the study, analyzed the testing results, and prepared the manuscript; TI and AI carried out the laboratory tests and analysis; KN supported in data interpretations and critically reviewed the manuscript; SK contributed primary supervision, critically reviewed, and approved the final version of the manuscript. SG, TI, and SK involved in site investigation at the early stage of the project.
Acknowledgments
The authors would like to appreciate Hokkaido Regional Head Office, East Nippon Expressway Company Limited, Sapporo, Hokkaido, Japan, for the collaboration and supports provided during the site visits and soil sampling.
Conflict of interest
The authors declare that the research was conducted in the absence of any commercial or financial relationships that could be construed as a potential conflict of interest.
Publisher’s note
All claims expressed in this article are solely those of the authors and do not necessarily represent those of their affiliated organizations, or those of the publisher, the editors and the reviewers. Any product that may be evaluated in this article, or claim that may be made by its manufacturer, is not guaranteed or endorsed by the publisher.
Supplementary material
The Supplementary Material for this article can be found online at: https://www.frontiersin.org/articles/10.3389/fbuil.2022.1044598/full#supplementary-material
References
Almajed, A., Abbas, H., Arab, M., Alsabhan, A., Hamid, W., and Al-Salloum, Y. (2020). Enzyme-Induced Carbonate Precipitation (EICP)-Based methods for ecofriendly stabilization of different types of natural sands. J. Clean. Prod. 274, 122627. doi:10.1016/j.jclepro.2020.122627
Almajed, A., Khodadadi Tirkolaei, H., and Kavazanjian, E. (2018). Baseline investigation on enzyme-induced calcium carbonate precipitation. J. Geotech. Geoenviron. Eng. 144, 04018081. doi:10.1061/(asce)gt.1943-5606.0001973
Almajed, A., Tirkolaei, H. K., Kavazanjian, E., and Hamdan, N. (2019). Enzyme induced biocementated sand with high strength at low carbonate content. Sci. Rep. 9, 1135. doi:10.1038/s41598-018-38361-1
Amarakoon, G. G. N. N., and Kawasaki, S. (2018). Factors affecting sand solidification using MICP with pararhodobacter sp. Mat. Trans. 59, 72–81. doi:10.2320/matertrans.M-M2017849
Blakeley, R. L., and Zerner, B. (1984). Jack bean urease: The first nickel enzyme. J. Mol. Catal. 23, 263–292. doi:10.1016/0304-5102(84)80014-0
Bolleter, W. T., Bushman, C. J., and Tidwell, P. W. (1961). Spectrophotometric determination of ammonia as indophenol. Anal. Chem. 33, 592–594. doi:10.1021/ac60172a034
Chen, M., Gowthaman, S., Nakashima, K., and Kawasaki, S., 2022. Influence of humic acid on microbial induced carbonate precipitation for organic soil improvement. Environ. Sci. Pollut. Res. (accepted article). doi:10.1007/s11356-022-23157-8
Cheng, L., Shahin, M. A., and Chu, J. (2019). Soil bio-cementation using a new one-phase low-pH injection method. Acta Geotech. 14, 615–626. doi:10.1007/s11440-018-0738-2
Cui, M.-J., Lai, H.-J., Hoang, T., and Chu, J. (2020). One-phase-low-pH enzyme induced carbonate precipitation (EICP) method for soil improvement. Acta Geotech. 16, 481–489. doi:10.1007/s11440-020-01043-2
Dagliya, M., Satyam, N., Sharma, M., and Garg, A. (2022). Experimental study on mitigating wind erosion of calcareous desert sand using spray method for microbially induced calcium carbonate precipitation. J. Rock Mech. Geotechnical Eng. doi:10.1016/j.jrmge.2021.12.008
DeJong, J. T., Mortensen, B. M., Martinez, B. C., and Nelson, D. C. (2010). Bio-mediated soil improvement. Ecol. Eng. 36, 197–210. doi:10.1016/j.ecoleng.2008.12.029
Dejong, J. T., Soga, K., Kavazanjian, E., Burns, S., van Paassen, L. A., al Qabany, A., et al. (2013). Biogeochemical processes and geotechnical applications: Progress, opportunities and challenges. Geotechnique 63, 287–301. doi:10.1680/geot.SIP13.P.017
Farukh, M. A., and Yamada, T. J. (2018). Synoptic climatology of winter daily temperature extremes in Sapporo, northern Japan. Int. J. Climatol. 38, 2230–2238. doi:10.1002/joc.5329
Feng, K., and Montoya, B. M. (2016). Influence of confinement and cementation level on the behavior of microbial-induced calcite precipitated sands under monotonic drained loading. J. Geotech. Geoenviron. Eng. 142, 04015057. doi:10.1061/(ASCE)GT.1943-5606.0001379
Fujita, M., Nakashima, K., Achal, V., and Kawasaki, S. (2017). Whole-cell evaluation of urease activity of Pararhodobacter sp. isolated from peripheral beachrock. Biochem. Eng. J. 124, 1–5. doi:10.1016/j.bej.2017.04.004
Fukue, M., Nakamura, T., and Kato, Y. (1999). Cementation of soils due to calcium carbonate. Soils Found. 39, 55–64. doi:10.3208/sandf.39.6_55
Gowthaman, S., Chen, M., Nakashima, K., and Kawasaki, S. (2021a). Effect of scallop powder addition on MICP treatment of amorphous peat. Front. Environ. Sci. 9, 1–13. doi:10.3389/fenvs.2021.690376
Gowthaman, S., Mitsuyama, S., Nakashima, K., Komatsu, M., and Kawasaki, S. (2019). Biogeotechnical approach for slope soil stabilization using locally isolated bacteria and inexpensive low-grade chemicals: A feasibility study on Hokkaido expressway soil, Japan. Soils Found. 59, 484–499. doi:10.1016/j.sandf.2018.12.010
Gowthaman, S., Nakashima, K., and Kawasaki, S. (2020). Freeze-thaw durability and shear responses of cemented slope soil treated by microbial induced carbonate precipitation. Soils Found. 60, 840–855. doi:10.1016/j.sandf.2020.05.012
Gowthaman, S., Nakashima, K., and Kawasaki, S. (2021b). Durability analysis of bio-cemented slope soil under the exposure of acid rain. J. Soils Sediments 21, 2831–2844. doi:10.1007/s11368-021-02997-w
Gowthaman, S., Nakashima, K., and Kawasaki, S. (2022). Effect of wetting and drying cycles on the durability of bio-cemented soil of expressway slope. Int. J. Environ. Sci. Technol. (Tehran). 19, 2309–2322. doi:10.1007/s13762-021-03306-1
Gowthaman, S., Yamamoto, M., Nakashima, K., Ivanov, V., and Kawasaki, S. (2021c). Calcium phosphate biocement using bone meal and acid urease: An eco-friendly approach for soil improvement. J. Clean. Prod. 319, 128782. doi:10.1016/j.jclepro.2021.128782
Hamdan, N., and Kavazanjian, E. (2016). Enzyme-induced carbonate mineral precipitation for fugitive dust control. Geotechnique 66, 546–555. doi:10.1680/jgeot.15.P.168
Harkes, M. P., van Paassen, L. A., Booster, J. L., Whiffin, V. S., and van Loosdrecht, M. C. M. (2010). Fixation and distribution of bacterial activity in sand to induce carbonate precipitation for ground reinforcement. Ecol. Eng. 36, 112–117. doi:10.1016/j.ecoleng.2009.01.004
Hoang, T., Alleman, J., Cetin, B., Ikuma, K., and Choi, S. G. (2019). Sand and silty-sand soil stabilization using bacterial enzyme-induced calcite precipitation (BEICP). Can. Geotech. J. 56, 808–822. doi:10.1139/cgj-2018-0191
Imran, M. A., Nakashima, K., Evelpidou, N., and Kawasaki, S. (2022). Durability improvement of biocemented sand by fiber-reinforced MICP for coastal erosion protection. Materials 15, 2389. doi:10.3390/ma15072389
Imran, M. A., Nakashima, K., and Kawasaki, S. (2021). Bio-mediated soil improvement using plant derived enzyme in addition to magnesium ion. Cryst. (Basel) 11, 516. doi:10.3390/cryst11050516
Islam, M. T., Chittoori, B. C. S., and Burbank, M. (2020). Evaluating the applicability of biostimulated calcium carbonate precipitation to stabilize clayey soils. J. Mat. Civ. Eng. 32, 1–11. doi:10.1061/(ASCE)MT.1943-5533.0003036
Ivanov, V., and Chu, J. (2008). Applications of microorganisms to geotechnical engineering for bioclogging and biocementation of soil in situ. Rev. Environ. Sci. Biotechnol. 7, 139–153. doi:10.1007/s11157-007-9126-3
JGS (2012). Japanese standards and explanations of geotechnical and geoenvironmental investigation methods (3431-2012). Tokyo: Japanese Geotechnical Society, 426–432.
Jiang, N.-J., Tang, C.-S., Yin, L.-Y., Xie, Y.-H., and Shi, B. (2019). Applicability of microbial calcification method for sandy-slope surface erosion control. J. Mat. Civ. Eng. 31, 04019250. doi:10.1061/(ASCE)MT.1943-5533.0002897
Jiang, N. J., and Soga, K. (2017). The applicability of microbially induced calcite precipitation (MICP) for internal erosion control in gravel–sand mixtures. Géotechnique 67, 42–55. doi:10.1680/jgeot.15.P.182
Lee, M., Gomez, M. G., San Pablo, A. C. M., Kolbus, C. M., Graddy, C. M. R., DeJong, J. T., et al. (2019). Investigating ammonium by-product removal for ureolytic bio-cementation using meter-scale experiments. Sci. Rep. 9, 18313. doi:10.1038/s41598-019-54666-1
Lin, H., Suleiman, M. T., Brown, D. G., and Kavazanjian, E. (2016). Mechanical behavior of sands treated by microbially induced carbonate precipitation. J. Geotech. Geoenviron. Eng. 142, 04015066. doi:10.1061/(ASCE)GT.1943-5606.0001383
Liu, K.-W., Jiang, N.-J., Qin, J.-D., Wang, Y.-J., Tang, C.-S., and Han, X.-L. (2020). An experimental study of mitigating coastal sand dune erosion by microbial- and enzymatic-induced carbonate precipitation. Acta Geotech. 16, 467–480. doi:10.1007/s11440-020-01046-z
Martinez, B. C., DeJong, J. T., Ginn, T. R., Montoya, B. M., Barkouki, T. H., Hunt, C., et al. (2013). Experimental optimization of microbial-induced carbonate precipitation for soil improvement. J. Geotech. Geoenviron. Eng. 139, 587–598. doi:10.1061/(ASCE)GT.1943-5606.0000787
Mawson, R., Gamage, M., Terefe, N. S., and Knoerzer, K. (2011). Ultrasound in enzyme activation and inactivation. Food Eng. Ser., 369–404. doi:10.1007/978-1-4419-7472-3_14
Mitchell, J. K., and Santamarina, J. C. (2005). Biological considerations in geotechnical engineering. J. Geotech. Geoenviron. Eng. 131, 1222–1233. doi:10.1061/(asce)1090-0241(2005)131:10(1222)
Mobley, H. L., Island, M. D., and Hausinger, R. P. (1995). Molecular biology of microbial ureases. Microbiol. Rev. 59, 451–480. doi:10.1128/mr.59.3.451-480.1995
Mohsenzadeh, A., Aflaki, E., Gowthaman, S., Nakashima, K., Kawasaki, S., and Ebadi, T. (2021). A two-stage treatment process for the management of produced ammonium by-products in ureolytic bio-cementation process. Int. J. Environ. Sci. Technol. (Tehran). 19, 449–462. doi:10.1007/s13762-021-03138-z
Montoya, B. M., and de Jong, J. T. (2015). Stress-strain behavior of sands cemented by microbially induced calcite precipitation. J. Geotech. Geoenviron. Eng. 141. doi:10.1061/(ASCE)GT.1943-5606.0001302
Omoregie, A. I., Palombo, E. A., and Nissom, P. M. (2020). Bioprecipitation of calcium carbonate mediated by ureolysis: A review. Environ. Eng. Res. 26, 1–16. doi:10.4491/eer.2020.379
Putra, H., Yasuhara, H., Erizal, S., and Fauzan, M. (2020). Review of enzyme-induced calcite precipitation as a ground-improvement technique. Infrastructures (Basel) 5, 66. doi:10.3390/infrastructures5080066
Putra, H., Yasuhara, H., Kinoshita, N., Neupane, D., and Lu, C.-W. (2016). Effect of magnesium as substitute material in enzyme-mediated calcite precipitation for soil-improvement technique. Front. Bioeng. Biotechnol. 4, 37. doi:10.3389/fbioe.2016.00037
Qabany, A., Mortensen, B., Martinez, B., Soga, K., and Dejong, J. (2011). Microbial carbonate precipitation: Correlation of S-wave velocity with calcite precipitation. Reston, VA: Geotechnical Special Publication. American Society of Civil Engineers, 3993–4001. doi:10.1061/41165(397)408
Salifu, E., MacLachlan, E., Iyer, K. R., Knapp, C. W., and Tarantino, A. (2016). Application of microbially induced calcite precipitation in erosion mitigation and stabilisation of sandy soil foreshore slopes: A preliminary investigation. Eng. Geol. 201, 96–105. doi:10.1016/j.enggeo.2015.12.027
Sharma, M., Satyam, N., and Reddy, K. R. (2022). Large-scale spatial characterization and liquefaction resistance of sand by hybrid bacteria induced biocementation. Eng. Geol. 302, 106635. doi:10.1016/j.enggeo.2022.106635
Sharma, M., Satyam, N., and Reddy, K. R. (2021). Effect of freeze-thaw cycles on engineering properties of biocemented sand under different treatment conditions. Eng. Geol. 284, 106022. doi:10.1016/j.enggeo.2021.106022
Sharma, M., and Satyam, N. (2021). Strength and durability of biocemented sands: Wetting-drying cycles, ageing effects, and liquefaction resistance. Geoderma 402, 115359. doi:10.1016/j.geoderma.2021.115359
Sun, X., Miao, L., and Chen, R. (2020). The application of bio-cementation for improvement in collapsibility of loess. Int. J. Environ. Sci. Technol. (Tehran). 18, 2607–2618. doi:10.1007/s13762-020-02974-9
Tang, C. S., Yin, L. Y., Jiang, N. J., Zhu, C., Zeng, H., Li, H., et al. (2020). Factors affecting the performance of microbial-induced carbonate precipitation (MICP) treated soil: A review. Environ. Earth Sci. 79, 94. doi:10.1007/s12665-020-8840-9
Ulusay, R., and Erguler, Z. A. (2012). Needle penetration test: Evaluation of its performance and possible uses in predicting strength of weak and soft rocks. Eng. Geol. 149–150, 47–56. doi:10.1016/j.enggeo.2012.08.007
van Paassen, L. A., Ghose, R., van der Linden, T. J. M., van der Star, W. R. L., and van Loosdrecht, M. C. M. (2010). Quantifying biomediated ground improvement by ureolysis: Large-scale biogrout experiment. J. Geotech. Geoenviron. Eng. 136, 1721–1728. doi:10.1061/(ASCE)GT.1943-5606.0000382
Wang, Y., Soga, K., Dejong, J. T., and Kabla, A. J. (2019). Microscale visualization of microbial-induced calcium carbonate precipitation processes. J. Geotech. Geoenviron. Eng. 145, 1–13. doi:10.1061/(ASCE)GT.1943-5606.0002079
Whiffin, V. S. (2004). Doctoral dissertation. Australia: Murdoch University.Microbial CaCO3 precipitation for the production of biocement
Whiffin, V. S., van Paassen, L. A., and Harkes, M. P. (2007). Microbial carbonate precipitation as a soil improvement technique. Geomicrobiol. J. 24, 417–423. doi:10.1080/01490450701436505
Keywords: microbial induced carbonate precipitation, bacterial-enzyme induced carbonate precipitation, free urease, fine-grained soil, slope surface, cementation
Citation: Gowthaman S, Iki T, Ichinohe A, Nakashima K and Kawasaki S (2022) Feasibility of bacterial-enzyme induced carbonate precipitation technology for stabilizing fine-grained slope soils. Front. Built Environ. 8:1044598. doi: 10.3389/fbuil.2022.1044598
Received: 14 September 2022; Accepted: 26 September 2022;
Published: 12 October 2022.
Edited by:
Kennedy Chibuzor Onyelowe, Michael Okpara University of Agriculture, NigeriaReviewed by:
Michael Onyia, University of Nigeria, Nsukka, NigeriaMeghna Sharma, Indian Institute of Technology Bombay, India
Copyright © 2022 Gowthaman, Iki, Ichinohe, Nakashima and Kawasaki. This is an open-access article distributed under the terms of the Creative Commons Attribution License (CC BY). The use, distribution or reproduction in other forums is permitted, provided the original author(s) and the copyright owner(s) are credited and that the original publication in this journal is cited, in accordance with accepted academic practice. No use, distribution or reproduction is permitted which does not comply with these terms.
*Correspondence: Sivakumar Gowthaman, Z293dGhhbTEwMTJAb3V0bG9vay5jb20=, Z293dGhhbUB0ZWNoLmpmbi5hYy5saw==