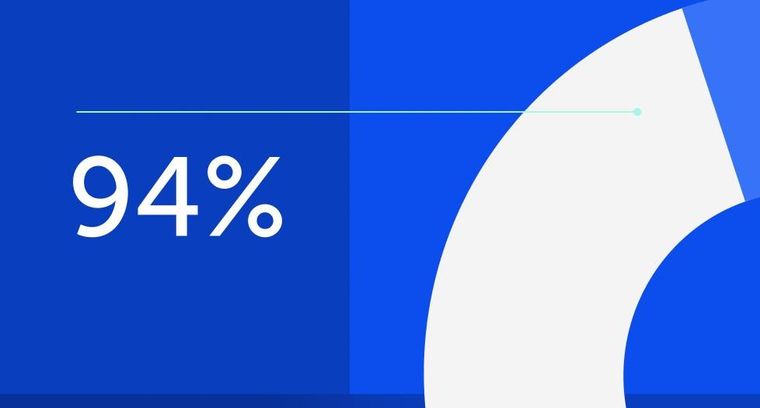
94% of researchers rate our articles as excellent or good
Learn more about the work of our research integrity team to safeguard the quality of each article we publish.
Find out more
REVIEW article
Front. Built Environ., 04 January 2023
Sec. Structural Sensing, Control and Asset Management
Volume 8 - 2022 | https://doi.org/10.3389/fbuil.2022.1034020
This article is part of the Research TopicHorizons in Built Environment 2022View all 6 articles
Concrete 3D Printing (3DP) is a potential technology for increasing automation and introducing digital fabrication in the construction industry. Concrete 3D Printing provides a significant advantage over conventional or precast methods, such as the prospects of topologically optimized designs and integrating functional components within the structural volume of the building components. Many previous studies have compiled state-of-art studies in design parameters, mix properties, robotic technologies, and reinforcement strategies in 3D printed elements. However, there is no literature review on using concrete 3D Printing technology to fabricate structural load-carrying elements and systems. As concrete 3DP is shifting towards a large-scale construction technology paradigm, it is essential to understand the current studies on structural members and focus on future studies to improve further. A systematic literature review process is adopted in this study, where relevant publications are searched and analyzed to answer a set of well-defined research questions. The review is structured by categorizing the publications based on issues/problems associated with structural members and the recent technology solutions developed. It gives an overall view of the studies, which is still in its nascent stage, and the areas which require future focus on 3D printing technology in large-scale construction projects.
Concrete 3D printing (3DP) is an emerging digital construction technique that can realize geometrically complex designs. It was initially developed by (Khoshnevis et al., 2012) and is continuously evolving to be a prominent technology in the construction industry. It is proven to bring many benefits like increased customization, reduced construction time, labor, and material requirement. D-shape, contour crafting, and extrusion-based concrete printing are the common techniques in concrete 3DP (Perkins and Skitmore, 2015). Out of them, extrusion-based concrete printing is the most commonly used technology, and the number of research studies is increasing exponentially worldwide (Buswell et al., 2018).
Improvements were brought in terms of mix design, digital implementation, and printing parameters to concrete 3DP through many research studies. Multiple literature review studies are available focusing on the different aspects of 3DP technology. There have been review studies in terms of general implementation in the construction industry that brought out the required overall improvements in terms of large-scale implementation (Wu et al., 2016; Shakor et al., 2019b; Siddika et al., 2020). Other review studies were done to understand the digital planning methods in 3DP building parts and the process involved (Paolini et al., 2019). A review study was made to illustrate the commonly used raw materials, critical factors that need to be controlled, and the measuring methods required to assess the fresh and hardened properties of concrete 3DP (Ma and Wang, 2018). Review studies were done to emphasize the 3D printable material requirement at different developments, material fresh and hardened properties (Lu et al., 2019; Paul et al., 2018; Li et al., 2020b). A review study also brought out overall progress in workability, mechanical properties, and building plan design (Zhang et al., 2019). Further, there are comprehensive studies on the different state-of-the-art technologies used to fabricate 3D-printed formwork (Jipa and Dillenburger, 2022).
Even though concrete 3D printing (3DP) has been demonstrated to be beneficial in several aspects, constructing structural load-carrying elements using this technology still involves many challenges. In many implementations, only architectural elements or predominantly compression members are made with concrete 3D printing. The future of 3D printing technology is to take up its freeform buildability advantages into large-scale implementations. But large-scale implementation of this technology will require the development of methodologies for printing structural elements that take tension and flexure. Structural elements need reinforcement techniques to increase their tensile and flexural load-carrying capacity, which cannot be easily introduced into the conventional printing process. There have been recent studies on the technologies for reinforcement introduction and other designs for concrete 3D-printed structural elements.
But, a comprehensive review with a special emphasis on all the issues associated with printing structural elements and the methods developed till date is currently missing. This study will help identify the research gaps/problems that need to be addressed for the large-scale implementation of structural 3D printed elements. This paper reviews the research performed in printing structural elements and systems and highlights the problems related to the execution of structural elements using the concrete 3DP methodology. Also, extrusion-based concrete 3DP is given predominant focus in this study as it is the most used technique worldwide.
A systematic literature review methodology is adopted in this study involving three stages, a. planning, b. compilation and c. analysis. In the planning stage, the purpose of the review is established by formulating the research questions precisely. The compilation stage involves identifying, screening, shortlisting, and including research papers. The analysis stage involves critically examining the selected papers to answer the research questions.
In this study, in-depth significance is given to the research studies involving 3D-printed structural load-carrying members. Also, among the different 3DP technologies available in concrete, most studies focus on extrusion-based concrete 3DP techniques. Hence the predominant focus is given to extrusion-based concrete 3DP studies. Further, the planning and compilation stage is narrowed to two research questions.
The research questions guiding this study are the following:
RQ1: What are the challenges in constructing load-carrying structures using 3D printing technology
RQ2: What technologies have been developed and tested for concrete 3D printing of structural elements or improving their tensile and flexural strength
The compilation of research papers is done through the library database Scopus, which is universally adopted as a source of scientific information. The systematic search procedure involves the identification of keywords, performing keyword searches and selecting relevant papers, examining cited papers in each selected paper, and compiling the publications that have cited the selected papers.
Analysis of the compiled papers involves reading either the abstract or the entire paper, identifying the key issues discussed, and classifying the content. The following classification tree has been developed through a systematic literature search (Figure 1).
Further, the search for publications using Scopus is summarized in the form of a bibliometric network using VOSviewer software, as shown in Figure 2. From this figure, important topics/fields that need to be discussed in depth to review 3D-printed structural members can be brought out. The network also proves that the topics of reinforcement method, strength requirements, topology optimization, modeling, and bond strength are some critical topics/keywords that need to be addressed for this review.
The rest of the paper is structured based on the issues and technologies depicted in Figure 1. Section 3 summarizes the major issues/problems associated with structural members. A summary of the issues/problems and their impacts on the load-carrying capabilities of 3DP elements are discussed in different headings, as shown in Figure 1. Then the overall advancements in technology pertaining to 3DP structural members are discussed in detail in Section 4. It gives a comprehensive overview of all the technologies developed in this area. Finally, a critical review of major problems that need to be addressed in future studies is given in Section 5.
The main challenge in constructing structures using concrete 3D Printing is imparting tensile and flexural strength in the printed members. Various studies have focused on developing the tensile and flexural strength members by incorporating reinforcement techniques into 3DP technology. But apart from tensile strength requirements, there are other problems associated with the large-scale 3DCP structural members. The major problems are discussed in the following sub-sections.
Insertion of rebars or other reinforcement techniques inside the concrete is the most conventional way of producing structural load-carrying elements. Bos et al. (2017a) studied the direct entrainment of steel cables as reinforcement for 3DCP elements. The failure behavior for high-strength cable specimens was found to be due to the bond loss between the concrete and the cables. The failure due to bond loss is highly unpredictable, hence, a proper understanding of the concept is required.
In the case of reinforcement bar penetration, understanding the bond profile between the steel bar and the concrete is important (Marchment and Sanjayan (2020a). Similar bonding issues are found where reinforcement is inserted into the hollow sections of the printed elements at the hardened state (Aramburu et al., 2022). Here, bonding is brought out by mortar between the steel bars and the concrete printed elements. Loss of bond strength between steel and concrete at any location may also trigger failure in those areas, ultimately affecting the printed elements’ strength-carrying capacities.
The system developed by (Marchment and Sanjayan, 2020b) for 3DP provided a novel way of simultaneously printing and reinforcing (using steel mesh) the element. Still, there is a need for testing elements printed using the technique on a larger scale and curved elements. There is also a need to develop a system for minimizing the lap length by exploring ways to stitch the reinforcement meshes Hojati et al. (2022) found that the usage of barbed wires enhanced the bond strength, flexural strength, and moment carrying capacity compared with plain concrete elements, but maintaining the straightness of the barbed wire is challenging and it hinders the automation level of this method of reinforcement. Wang et al. (2021) studied the usage of U-shaped nails as reinforcement for concrete 3DP elements yielding favorable results. But, the increase in the mechanical properties matched only a material-level replacement for reinforcement, not the structural level. In most of these unconventional reinforcement techniques, reinforcement is embedded into the printed layers after the completion of each layer increasing the printing time and reducing the automation level.
Gebhard et al. (2020) proved that placing and aligning the fibers in between the layers increased the tensile strength. The fibers are kept in a particular orientation after every layer which is difficult to automate as the placement of fibers needed to be synchronized with the printing process to reach the same level of automation and reliability. The selection for fiber reinforcement is subjective to a particular case and may change from case to case.
The carbon fibers improve the shape and stability of the reinforced elements, but their use is limited due to their performance under alkaline and varying temperature conditions (Schneider et al., 2018). Similarly, Mineral Impregnated Carbon Fiber has challenges during mixing and more studies are required to investigate its durability and robustness (Mechtcherine et al., 2020a).
Glass fibers of different sizes are used as reinforcement techniques in 3DCP (Hambach and Volkmer, 2017; Panda et al., 2017a; Shakor et al., 2020; Yang et al., 2022b). But the strength enhancement was insufficient to eliminate steel reinforcement. The orientation of the fibers against the loading direction during mechanical testing has a significant impact. Similarly, the impact of textile reinforcement added in the layer interfaces on the strength requirements proved that they do not impart much strength enhancement to the printed elements (Gries et al., 2016).
Lower interlayer bond strength creates a weak plane in the absence of reinforcement and gives rise to anisotropic behavior as the direction of the load changes (Baz et al., 2021). Interlayer bond strength also affects the shear, flexural and compressive strength of 3DP elements. The printing cycle time significantly affects the layers’ bond strength in 3DCP, reducing the flexural strength (Al-Qutaifi et al., 2018). Buswell et al. (2018) states that the main problem with interlayer bond strength is the formation of cold joints due to longer cycle times in case of larger geometry of the element. Though interlayer bonding improved by using nails and screws (Baz et al., 2021; Perrot et al., 2020), there is still an issue regarding the corrosion of nails and screws. Steps like maintaining low permeability, sufficient cover, and using materials like fly ash must be taken to prevent this. Also, if the roughness of nails or screws goes above a specific limit, they may destroy the surrounding layers and cause additional voids.
Liu et al. (2022) studied the interlayer bonding in 3DCP using recycled coarse aggregates (RAC). With an increase in the proportion of the RAC led to a significant decrease in the interlayer bond strength among the samples but it was still greater than the bond strength achieved using 3D printing mortar of the same cementitious material system mix ratio due to the increase in the bonding area.
Panda et al. (2019a) stated that a higher yield strength resulted in weaker interlayer bond strength due to the formation of the pores at the interlayer interface and the printer head standoff distance greater than the nozzle diameter also reduced the bond strength. The interlayer bond strength can be enhanced with the addition of the superplasticizer providing better bonding surfaces and reducing the pores at the interface zone, besides reducing the effect of the printer head standoff distance. The addition of the superplasticizer reduces the thixotropy and allows higher printing speed for better interlayer bond strength (Weng et al., 2021). But adding superplasticizers impacts the rheology of the concrete and requires active monitoring.
Shakor et al. (2020) have investigated the influence of the glass fibers incorporated in the mortar on the mechanical properties of the casted elements. The results showed an enhancement in the compressive strength and flexure strength to the extent of 108% and 68%, respectively. The extruder speed also has a significant impact on the printing quality. The glass fibers also act as an interlayer reinforcement, thus contributing to the inter-bond strength of the elements. Shakor et al. (2017) studied the influence of the properties of the concrete mix designs on the printing quality using a six DOF robotic system and found out that the mix properties and printer extruder speed are crucial in determining the printing quality. 12 different mixes were tested, and only three out of them performed well in compressive strength and squeeze flow tests which are used as the basis for flow quality and buildability.
Due to the differences in the material property, the layer interfaces in 3DCP elements tend to act as failure planes reducing the structural performance of the concrete. Kim et al. (2017) studied the fracture energy of the layer interfaces of 3DCP elements and suggested a suitable bridging material to increase the fracture energy and thus increasing the structural integrity of the printed elements. Using the model following Modified Compression Fied Theory (MCFT) theory, it was found that fracture energy diminishes during the initial setting time of concrete, affecting the overall behavior of the elements. Markin et al. (2019) developed printable concrete with mechanical strengths varying from 8 MPa to 100 MPa, but 3DPC still faces issues with plastic and dry shrinkages due to the high amount of cement content and low amount of coarse aggregate. Accelerators added in the mix to improve buildability also create durability issues due to the crystallization of salts (Lloret-Fritschi et al., 2019).
Falliano et al. (2020) compared fresh state dimensional stability and mechanical properties of “3D-printable lightweight foamed concrete” (3DP-LWFC) with conventional lightweight foamed concrete (C-LWFC). 3DP-LWFC showed better dimensional stability, no instantaneous and delayed settlement, and better compressive strength at the same density compared to C-LWFC with mixing intensity as major influencer, however, there is a need to study other properties like interlayer bonding, plastic and drying shrinkage crack. Le et al. (2012) considered the external environment’s effect on the shrinkage in 3DCP mix. Dry shrinkage was found dependent on the curing conditions in different environments. Amba et al. (2010), Nerella et al. (2019) found that due to the differential moisture distribution attributed to the deposition of the layer at different time intervals, shrinkage, creep, and strain relaxation effects are observed, leading to reduced bond strength between the layers.
Wang et al. (2020) observed that the increase in ceramsite content to an optimum level in 3DP lightweight hollow concrete elements reduced slump value and increased penetration resistance and enhanced compressive strength. Further studies are required on other mechanical properties and problems like environmental degradation and integration of tension reinforcement for the elements. Yang Y. et al. (2022) found that the mechanical strength properties of 3DP ultra-high-performance fibre-reinforced concrete (3DP-UHPFRC) are better than the Mould Casted ultra-high-performance fiber-reinforced concrete (MC-UHPFRC) due to the directional distribution of steel fibers in 3DP specimens. Though the compressive elastic modulus was anisotropic, the variation in tensile elastic modulus was insignificant. Studies are required in terms of buildability of 3D-UHPFRC and the external environment’s effect on 3DCP elements.
A theoretical framework is developed and validated by simulating layer loads corresponding to the yield strength of the mix in a series of laboratory tests (Perrot et al., 2016). While designing a mix for 3DP, the two critical factors are its pumpability and buildability (Tay et al., 2019b). The inter-layer bond strength of the printed layers decreases with an increase in the time gap between the layers. The decrease in strength is logarithmic for up to the first 5 min of gap time, and then the loss in strength is minimal (Tay et al., 2019b). The addition of flyash to a hybrid mix of 95% concrete and 5% geopolymer based on fly ash or metakaolin was found to increase the compressive strength of the mix by 20% (Ziejewska et al., 2022). But the cast concrete elements showed 40% higher compressive strength than printed elements.
Alkali-activated materials (AAM) are used in 3DCP as substitute cementitious binders, to reduce overall CO2 emissions (Amran et al., 2022). But various areas need to be studied to enhance the reach of AAM-based 3D printing to a greater extent. Mechtcherine et al. (2019) tried to leverage the advantages of 3DP using coarse aggregates. The materials easily available at construction sites were used to improve the adaptability. Rahul and Santhanam (2020) worked on analyzing the printability of light-expanded clay aggregates (LECA). Being expensive, LECA will impact the cost of 3DP elements and long-term durability, and the external environment’s effect on the 3DCP elements needs to be studied. Rushing et al. (2017) studied the possible inclusion of coarse limestone aggregate in a 3DP mix using a fine-aggregate: coarse-aggregate ratio of about 1:2 and type I/II portland cement. It showed that coarse aggregates can be included in a 3DP mix, but a delivery system and optimum combination of additives for such a mix need to be devised.
Şahin and Mardani-Aghabaglou (2022) highlighted the importance of the mix design on the printability performance and the rheological properties in 3DCP. Sulfoaluminate cement is found to induce rapid hardening, high strength, and low shrinkage. The addition of nano-clay and viscosity modifying agents (VMA) increased the buildability properties of the mix. Adding recycled wastes, waste materials, and geo-polymers significantly impacts sustainability.
Izadgoshasb et al. (2021) developed a model combining a multi-objective grasshopper optimization algorithm (MOGOA) and artificial neural network (ANN), ANNMOGOA, to predict the compressive strength of 3DP concrete. Mix proportions and fresh-state properties of concrete are used as input for the ANNMOGOA. A single-layered model was found to predict compressive strength. Though the study is novel, there are a few deficiencies like the need to include the type of materials, climatic conditions, temperature, and humidity, to get a more realistic prediction model.
Strano et al. (2013) tried optimizing the support structures by optimizing the built orientation and the cellular structure to determine critical places where more robust support is required. The algorithm uses implicit mathematical 3D functions for optimization and resulted in 45% saving on the materials on a truss part. The elements with overhangs are also printed sideways and then rotated to serve their intended use (Vanek et al., 2014). Some studies suggest that the inclination of the overhanging part should be 45° (Kuo et al., 2018; Strano et al., 2013), and some suggest that it is dependent on the properties of the features to be printed (Gaynor and Guest, 2016). Pre-fabricated optimized support structures are used to optimize the material usage and express the optimized complex geometry of the elements (Anton et al., 2020). Topology optimization for support design and its requirement is developed by (Vanek et al., 2014) to optimize the material usage while effectively supporting the overhangs. Bi et al. (2022) and Langelaar (2016) presented a topology optimization formulation with layer-wise filtering to remove the unprintable geometries to generate fully self-supported optimized designs helpful in reducing the need for support structures but limiting the design freedom.
3DCP of curved surfaces and overhangs presents a challenge because of the geometric constraints and the surface quality. Lao et al. (2021) developed a nozzle-geometry dynamic controller setup to counter the irregular surface finish observed in normal 3DP fixed-geometry nozzles. Extra details of layer geometry are derived from the thicker slicing of the 3D model and used to adjust the nozzle diameter as required, reducing the surface irregularities like the staircase effect.
Shakor et al. (2019a) pointed out that printing rectangular layers tend to sustain more layer load than the spherical or circular-shaped layers and enhances the printed layers’ outer surface quality. McGee et al. (2020) used a nozzle equipped with adjustable side plates to do the finishing for the printed layers. However, it is not feasible to print elements having self-intersecting paths.
McGee et al. (2020) used an optimized mix with cement and fly ash and was able to print overhangs up to an angle of 17.5° only due to the tension stresses in the upper border of the printed specimen. Tay et al. (2019a) found that the maximum gap length of 10 mm can be allowed in the bottom layer without causing a slump in the subsequent layer. The support structure layers are printed with intermittent gaps of 10 mm to support the main structure and these could be carved out easily. This method is suitable for small openings only as it would generate huge waste in case of large openings, thus, more work needs to be done in this direction to make it a feasible solution.
Mengesha et al. (2023) implemented a numerical simulation to understand the relationship between process parameters and the 3DP products to avoid the trial-and-error approach. The numerical model developed by (Wolfs et al., 2018) to determine the early-age mechanical performance of the 3DCP samples showed a promising approach by matching the experimental results qualitatively. The lack of quantitative reliability can be explained by the overestimation of properties of concrete that has been compacted after printing (better properties than the concrete which has not been printed yet) and also due to the inability to incorporate the geometric defects in the numerical model, which can cause eccentricity and early failure in an axial load test.
Chandra et al. (2020) developed an analytical model using finite element software to estimate the mechanical behavior of the 3DP RC beams. The final analytical model accurately predicted the maximum flexural strength of the 3DP RC beams. But it was not accurate in finding the initial stiffness and the several local failures, like a shear failure at nodal points.
A numerical model of Steel Reinforced Fiber concrete (SRFC) developed by (Nguyen et al., 2022) showed compliance with Eurocodes, but still, there is a need for developing a model that captures the layering pattern of 3D printing to make structural analysis using numerical models more reliable.
Vantyghem et al. (2020) developed structurally efficient 3DP structural elements with minimum material wastage, but the complex design shapes made it challenging to introduce reinforcement. Also, it may require sufficient support structures during printing and installation.
Brackett et al. (2013) attempted to exploit the design freedom of AM through Bi-directional Evolutionary Structural Optimization (BESO). Latifi Rostami et al. (2022) carried out the BESO topology optimization method for hybrid additive-subtractive manufacturing (HASM) with additive and subtractive manufacturing constraints. To enable AM of self-supporting topologically optimized structures (Bi et al., 2020) adopted the BESO framework with a layer-wise geometric self-supporting constraint. The first algorithm, which considered the orthotropic nature of printed concrete and the practical limitations posed by AM process, was developed by (Martens et al., 2018). This algorithm provides an initial step towards structurally stable, printable, and optimized structures using the modified Solid Isotropic Material with Penalisation (SIMP) method. Langelaar (2017) presented a filter that can be used with density-based topology optimization techniques to allow only printable designs in AM. Yang et al. (2018), after an experimental study of topologically optimized 3DP polymer materials, concluded that behaviors of 3DP materials must be considered in optimization for better results. Safonov (2019) and Schmidt et al. (2020) discussed the topological optimization of 3DP fiber composite structures. Carstensen (2020) considers nozzle size of extrusion type AM process as a constraint in topology optimization. The nozzle size must be included in the design stage or post-processing stage. Fiuk and Mrzygłód (2020) presented an effective topology optimization technique called Constant Surface Criterium Algorithm (CSSA), with printing direction and stress as constraints.
Wu et al. (2016) suggests that 3DP is limited by the lack of large-scale implementation, mass customization, LCA, and the development of building information modeling. The authors signified the importance of the size of the printer in large-scale implementation as it limits the maximum size of the element that can be printed. If the printer is small, smaller segments must be printed, transported to the site, and assembled to complete the large-scale structure. Though large-scale structures have been constructed using the assembling of segments methodology (Winsun constructing a two-story villa and a five-story apartment in China), some limitations have been found. The first of such limitations is the requirement of special joining methods to ensure the tightness of the segments. Although glass fibers were used, brittle failure was observed when printed elements were used in load-bearing structures. Another limitation stated was the manual installation of plumbing and electrical fixtures.
An external reinforcement system was introduced by (Asprone et al., 2018a), where they printed individual small segments and joined them using an external reinforcement system. Upon flexural testing, issues like the extent of overall ductility of the fabricated beam, degradation of the external reinforcement system due to environmental factors, steel bar embedment, and the fire resistance capacity were seen.
Ding et al. (2014) pointed out that material rheology impacting the bonding and setting of the printed layers needs to be designed and optimized to achieve impeccable results in large-scale printing. BIM model processing efficiency and printer parameters such as nozzle size and axial movement precision are important factors in the upscaling of the printing system. Developing lightweight printer components with progressive attachment and detachment, optimizing filler layer algorithms to reduce the printing time, and considering multi-nozzle and multi-material printing could add to the versatility of large-scale printing systems.
Heineman et al. (2019) developed a cable-driven 3DCP system where the extruder is held at the top of the structure with the help of a crane and is controlled in axial directions by the cable tension stations on the ground. Two designs of this superstructure–an angled tripod and a vertical tripod achieved the target deflection values. The cost and installation time of the angled one is more than the vertical one. There are many things to be improved, such as economy, site constraints, logistics, etc., to attain an efficient system.
Tiryaki et al. (2019) presented a novel printing method while moving a 3DCP system consisting of a robotic arm mounted on a mobile base as an improvement over a previous system where the mobile robot could print only in stationary conditions (Zhang et al., 2018). The development of the system required improvements in the system’s movement, localization, and motion control for proper deposition. Meisel et al. (2022) demonstrated a system for printing fully enclosed structures without much external support. The potential to use such a system in the intra-planetary civilization is explored, but it needed improvements in printing complex geometries.
The previous section focused on the issues involved in the 3D printing structural elements. This section will focus on the technologies developed in 3D printing with respect to structural elements.
One of the challenges 3D Concrete Printing (3DCP) faces is integrating reinforcement while printing the element. Numerous studies have incorporated reinforcement in 3DCP, but a robust and efficient solution is yet to be proposed (Spangenberg et al., 2022). This section will discuss the studies and technologies developed for integrating the reinforcements in 3DCP.
Though digital fabrication with concrete (Concrete 3DP) offers many advantages, there are no reinforcement strategies to ensure structural properties. The study by (Gebhard et al., 2021) focuses on this problem by conducting an experimental investigation on the 3D printed elements with different reinforcement strategies. The different reinforcement strategies studied here are interlayer shear reinforcement, aligned end hook fibers, and steel cables which are kept between the layers. Then, unbonded post-tensioning and conventional bonded passive reinforcement are explored for longitudinal reinforcement. This study developed post-tensioned beams consisting of interlayer shear reinforcement inserted during the printing process and longitudinal reinforcement post-installed in the printed voids. The structure is evaluated for the situation with and without inter-layer shear reinforcement. The structure was assessed using four-point bending tests to place fibers for interlayer shear reinforcement. A robotic fiber placement hopper places the fibers directly after printing each layer. The results of the tests show that the unbonded post-tensioned beam failed in a most brittle manner. Also, it was found that adding fibers in between the layers increased the ultimate load to a certain extent due to better control of micro-cracking. However, bonded post-tensioned reinforcement showed multiple bending and shear cracks activating the interlayer shear reinforcement. Cable reinforcement is also explored in this study and was found to be more efficient at failure than fiber reinforcement.
The review study by (Cao et al., 2022) found four major techniques for reinforcing 3D printed elements. They are mixing reinforcing materials inside the mix, inserting reinforcing techniques across the layers, placing reinforcing materials between the layers, and embedding reinforcing materials inside the filaments between the layers. These major techniques of reinforcement can be achieved by using different materials, like the usage of fibers in the mix and inserting reinforcement bars between the layers. Some of the reinforcement strategies are discussed in the following sections.
The conventional reinforcement approaches have been optimized to fit digital fabrication technologies. However, it still presents a stern challenge to establish a suitable reinforcement strategy for 3D printing technology. Asprone et al. (2018b) pointed out that using the existing reinforcement strategies in new technologies like 3D printing will restrict the new technology’s performance and economy. The study has suggested emphases on the sustainability analysis of the old reinforcement techniques with respect to new construction technologies like 3D Printing and the development of new reinforcement approaches based on the structural requirement and the digital technology used. Some studies that tried to integrate conventional reinforcement in 3DCP are mentioned in this section.
In 2018, in Champaign, Illinois, United States, a demonstration was performed by the Engineer Research and Development Center Construction Engineering Research Laboratory (ERDC-CERL) (Kreiger et al., 2019). 512ft2 reinforced additively constructed concrete printing of a building was done continuously. This methodology adopted the advantages of both 3D printing (freeform construction and reduced labor) and traditional reinforcement (tensile strength, increased load-bearing capacity). But there was no mention of the structural performance of the building in the study. An experimental study was conducted by (Baz et al., 2020) to characterize the quality of the bond between 3DPC and steel reinforcement bars using pull-out tests under different printing conditions. Also, the printing direction (parallel or perpendicular) with respect to the reinforcement bars has been considered, which helped prove that a highly thixotropic material doesn’t affect the bond between the reinforcement and the concrete. The result of the pull-out tests on conventional casted elements, parallel to bar printed element and perpendicular to bar printed element, showed that the bond strength is less in printed elements compared to the conventionally casted elements due to the external vibrations given to the latter. Still, the bond developed in printed elements is satisfactory. The decrease can be counter-balanced using proper safety factors.
Cohen and Carlson (2020) worked on a unique way of printing with conventional reinforcement bars instead of using a conventional linear layering approach for printing, using pointillistic time-based deposition (PTBD), discovered by (Cohen, 2019). A system was developed under this study in which the concrete was extruded at a point between the reinforcement cage standing vertically, and then after some time, it was pressed to flow out through the reinforcement cage. This demonstrates how a steel cage can be used as formwork and reinforcement. As the study is quite recent, it needs development in many areas: a) covering the corners of the reinforcement cage, b) after developing (a), there is a need for tests for the structural performance of the column. c) Aesthetic appearance, d) connection with other building elements like beams, slabs, and Earth, e) Scaling of the pump to make it capable of printing structures with large aggregates.
Other than conventional reinforcement with rebar cages, steel or micro-cables can be used in 3D-printed elements. During the 3D printing process, the steel cables are embedded into the printing layers simultaneously, along with the printing of the layers using a reinforcement entrainment device (RED) (Bos et al., 2017a). A study on the direct entrainment of cable reinforcement into concrete 3D printed elements is done by researchers (Bos et al., 2017b). A device is developed consisting of a spool with a servo-driven motor that inserts cable reinforcement directly before the mix leaves the print nozzle. A four-point bending test is done to validate the increase in ductility. Three different cable types were used to create specimens with tensile strengths of 0.5 kN, 1.0 kN, and 2.0 kN. The test results showed that all the samples show a linear elastic behavior until the first crack in the concrete happens. Two types of failure patterns are seen. In the case of lower-strength cables, the failure of the cable was the main reason for specimen failure. In the case of higher-strength cables, the failure was attributed to the loss of bond between the cables and the concrete mix. This study proves the importance of bond strength between the steel cables and the printing mix.
Hack and Lauer (2014) proposed a digital fabrication method of building reinforced meshes and formwork simultaneously with printing. This consist of a robot printing the mesh, which was used initially as formwork, and then once the concrete was poured on the mesh, it will flow and cover the whole mesh, and then the mesh will start acting as a reinforcement. However, this approach is yet to be tested on a larger scale. An overlapping mesh reinforcement technique was explored by (Marchment and Sanjayan, 2020b). In the study, galvanized steel reinforcement mesh was used to create a continuous mesh and nozzle design, which helped in the progressive laying of the reinforcement in single extruded layers. A wall section was printed using the setup and was tested using a 3-point bending test. The results showed that the failure first occurred by steel yielding rather than the failure of the bond between reinforcement and cement matrix. Hence, the nozzle helps create good bond strength between the reinforcement and print material. Also, calculations observed a 170%–290% increase at the moment in flexure.
Different layers of mesh reinforcement are used in the study by (Liu et al., 2021). The various layers of steel wire meshes (SWM) are added to the concrete 3D printed elements, and different destructive and non-destructive methods are employed to enhance the mechanical properties. It was found that the peak loads increased by 59.2%–173.3%, and the deflection capacity increased by more than 11 times more than the non-reinforced ones.
Hojati et al. (2022) explored the usage of barbed wires and fencing wires with thorn-like projections as a reinforcement for 3D-printed concrete elements to increase their tensile and flexural capacity. The barbed wires are manually placed along the 3D-printed filaments, and the specimens are tested to get the required results. Then the specimens are subjected to mechanical tests like a four-point bending test, flexural strength test, and pull-out test to understand that the mechanical properties of the elements increased significantly. The moment capacity and the bond strength of the barbed wire-placed concrete elements are increased by 363% and 71%, respectively, compared with plain 3D-printed concrete. The pull-out strength tests showed a considerable increase in bond strength with barbed wires due to protrusions proving it to be strong enough to prevent slip. The moment capacity and maximum deflection are also found to be 4.63 and 13.01 times larger than the plain concrete element.
Fiber reinforcement has been used in the construction industry mainly to reduce cracking problems but has recently been investigated for applications like reinforcements for 3D-printed elements. Fiber reinforcement for concrete 3DP is tested with different material fibers, and some of the studies are discussed in this section.
An experimental study concluded that basalt and glass do not have any significant effect on compressive strength and modulus of Elasticity (Kizilkanat et al., 2015). In contrast, the tensile and flexural strength were increased until an optimum dosage, and no change was observed. While comparing both fibers in case of crack resistance and ductility, Basalt fiber performed much better than Glass Fiber. Though some amount of tensile strength was increased, using these fibers for reinforcement may not be sufficient for the 3D concrete Printing Process.
Adding glass, basalt, or carbon fibers to concrete 3DP increases the flexural strength to 30 MPa (Hambach and Volkmer, 2017). It was also found that the fibers align along the direction of printing; hence, the build path was optimized to have maximum flexural strength. But the addition of steel reinforcement is important to increase the flexural strength.
Glass fibers (GF) of different lengths (3 mm, 6 mm, and 8 mm) with varying percentages from 0.25% to 1% in the concrete printing mix are used as reinforcement by (Panda et al., 2017a). The experimental results showed improved properties of the printed specimen with glass fiber by up to 1%. The properties were found to be dependent on the direction and orientation of the glass fibers.
Similarly, the impact of adding chopped glass fibers of length 6 mm on the strength of concrete 3DP elements is investigated by (Shakor et al., 2020). Studies are conducted to assess the deposition velocity changes on the developed mix mortar. But the study’s primary outcome is that the 1% (by weight of the cementitious material) short glass fibers added to the mix increase the compressive strength by 108% and the flexural strength by 68%.
A combination involving 3DP with lightweight engineered cementitious composites (LWECCs) is studied to improve various aspects of concrete 3DP (Sun et al., 2021; Yang R. et al., 2022). This study introduces hollow glass microspheres (HGMs) and polyvinyl alcohol (PVA) fibers to improve the strength-carrying capacities. When the orientation of the fibers was parallel to the loading direction during testing, the unconfined compression strength was found to be high. But interestingly, if the orientation of the fibers is perpendicular to the direction of loading, it results in an increase in the flexural strength and a 40% increase in the toughness behavior. The concept of glass fibers aligning parallel to the printing direction is reinstated by the study under X-ray computed tomography (XCT) (Yang R. et al., 2022). The XCT studies track the movement of cement and glass fiber microparticles in extrusion molded cementitious composites. It was found that the glass fibers in the matrix of filaments were mostly aligned parallel to the printing direction.
The carbon fibers have high tensile strength and low specific weight and do not corrode like conventional steel reinforcements. It allows the construction of thinner structures without compromising load-bearing capacity and durability, reducing the ecological footprint and leading to sustainable and cost-effective construction (Brameshuber, 2006; Curbach et al., 2007; Scholzen et al., 2012; Curbach, 2013).
Polymer-based carbon fiber is one of the reinforcing techniques that can operate over a wide range of temperatures and has proven to provide good mechanical performance (Schneider et al., 2018). It doesn’t have corrosion issues and has excellent bonding properties, providing a great alternative to conventional steel and polymer-based reinforcements. Mechtcherine et al. (2020b)worked on automating the process of manufacturing reinforcement using MCF composites. This study provided a direction toward fully automating the 3DCP by automating the reinforcing process, which, combined with 3DCP, can help to automate construction activities fully.
The Mineral—impregnated Carbon-Fiber (MCF), due to its advantages of high mechanical properties, durability, and ability to be processed and shaped during its fresh state, is used as reinforcement in the study by (Mechtcherine et al., 2020a). The three-point test results show that the reinforced printed element showed higher impact enhancement and deformability in the case of 3D-printed beams. Continuous Carbon fibers (CCF) are used as a mesh reinforcement after impregnating them with grained minerals (Mechtcherine et al., 2019). As pointed out by (Scheurer et al., 2020), the drawback of this technique is that the reinforcement can be placed in the direction of the printing only.
Reinforcing textiles usually provides higher specific strength and corrosion resistance, which allows for a reduction in reinforcement cover and is thus helpful in creating thin architectural elements (Gries et al., 2016). However, the increase in mechanical strength is not substantially high compared with other fiber reinforcement techniques.
3D-printed polymer and metallic fiber reinforcement with different surface morphology and roughness are used to strengthen the cement mortars (Farina et al., 2016). It was found that the mortar specimens reinforced with titanium alloy fibers exhibited a load-carrying capacity twice as high as specimens reinforced with photo-polymeric fibers. The use of Ultra-High-Performance Concrete (UHPC) with steel fibers possesses a promising potential to enhance the flexural strength of the concrete (Larsen and Thorstensen, 2020). UHPC is used for 3D printing a slab prototype to analyze the explosion resistance of UHPC 3DP concrete (Ma et al., 2022). The 3DP UHPC with steel fibers showed blast resistance as good as a steel bar reinforced UHPC with decreased steel requirement by weight.
Gebhard et al. (2020) explored the entrainment of fibers technique in the concrete 3D printing process. Unlike conventional construction, fibers in 3D printing need to be re-engineered with short and expensive fibers to pump through the nozzles for the printing process. This study explored the changes in mechanical properties of the construction elements when the fibers are placed on interlayer surfaces. The fibers are placed in a controlled amount and orientation, and then post-tensioning reinforcement is given in the perpendicular direction as the main reinforcement. The results showed that aligning the fibers between the layers results in higher tensile strength. In the case of main reinforcement, unbonded post-tensioning reinforcement proved promising, but brittle failure cannot be avoided due to localized load action.
Self-reinforced cementitious composites can be used as an alternative to the reinforcement requirement in 3D-printed structures. The study by (Soltan and Li, 2018) introduced an Engineered Cementitious Composite (ECC) with the inclusion of short polymer fibers to increase the tensile strain hardening behavior. The study also examines the effects of the fresh-state workability of several compositional ingredients. The fibers used are polyvinyl alcohol (PVA) fiber cut to 12 mm. It also proved the robust tensile strain-hardening behavior of the ECC composition. The compressive strength of the composition gained quickly to 30 MPa in 6 days. Also, the tensile strength of the proposed mix reached up to 6 MPa. ECC is expected to attain structural integrity and durability without steel reinforcement. 3DP-ECC with suitable printability and buildability characteristics shows the same tensile ductility as cast ECC (Li V. C. et al., 2020).
A novel mix design for ECC with ultra-high molecular weight polyethylene (PE) was developed and examined (Zhu et al., 2021). Four-point bending test results showed that the printed ECC beams presented a ductile failure mode along with strain-hardening behavior. Different beams are printed and tested for the experimental study with varying tool paths, loading directions, and superposition types. It is also found that the mechanical performance of the 3D printed ECC beams depend on the geometric configuration indicating the importance of the printing path for 3D printed elements. The printing path of the structural 3DP elements needs to be configured to get maximum mechanical performance. Due to the non-availability of the reinforcements, the printed ECC beams showed less rigidity and deflection than the RC beam. Hence, this study recommended increasing the elastic modulus through material optimization or providing reinforcement required to reduce the deflection.
Interlayer reinforcement enhances the element’s interlayer strength and overall strength. In a study by (Geneidy et al., 2020), “C”-shaped staples 3D printed using polylactic acid (PLA) filaments were used as an interlayer reinforcement. The printing head is modified to accommodate a staple ejector, which deploys staples in varying interlocking orientations into the printing layers trailing the printing head. This approach of providing interlayer reinforcement showed a small improvement in the flexural strength of the elements.
Another form of “in-process” reinforcing method during 3D printing is given by (Marchment and Sanjayan, 2020a). It utilizes a reinforcing technique where a deformed reinforcement bar is penetrated into the freshly printed 3D printed layers. The bond varies along the length of the penetration depth due to differences in the level of deformation or disturbances experienced by the concrete layers due to the penetrating bars. In the case of multiple-layer concrete prints, multiple bars are penetrated one above the other with sufficient lap length to have a better bond. Pull-out tests are performed on different penetration lengths and compared with penetrated sand bed lengths samples. From the bond test, it is found that the penetrations above 90 mm caused significant damage.
Another bar-based reinforcement technique is proposed by (Aramburu et al., 2022). The passive rebars are inserted into hollow channels within a 3D-printed mortar geometry. Then the gap between the rebars and the hollow channels is filled with grouts to achieve sufficient bond strength. Mechanical tests were performed and found that the bonding strength with shear stresses lay within the range of 16.75 MPa and 18 MPa. Also, it was found that during the testing, no specimen failed due to debonding between the filling mortar and the 3D printed cylinder nor because of debonding of the cylinder and the concrete poured outside it. Thus, it indicates the bond strength between the rebars and the filling grout material.
A novel reinforcement technique was introduced by (Hass and Bos, 2020) using screw-based reinforcement. Contrary to the bar being pushed into the 3D printed elements, which is a translation movement, this technique involved the usage of a screw that combines both rotational and translational motion. It avoids the formation of voids during mechanical inter-lock, achieving a very good bond. It takes into account that the print motor is still highly malleable for some time, even after the deposition of layers, to insert the screws. Finally, 3-point bending tests on printed specimens are done to prove the enhancement in mechanical properties. Also, pull-out tests are conducted to show that the failure in print mortar led to the pull-out failure, validating this methodology’s feasibility.
In a few studies, screws and nails have been seen as having the potential to increase interlayer bonding and hence limit the issues faced by low interlayer bonding. Perrot et al. (2020) proposed a method of using steel nails through deposited layers to improve multi-layer bonding. It was demonstrated that the interlayer bonding could be improved by using nails if the proper orientation is chosen and the surface of the nails has enough roughness to bond with the mortar. Further (Baz et al., 2021), conducted experiments to test the reinforcing of the successive layers using screws and nails, which was not done in any study till then and a 4-point bending test results suggested to use nails instead of screws.
Wang et al. (2021) studied adding a new U-shaped nail into the concrete during the printing process. The mechanical process improvements are analyzed by visualizing the bridging effect and the dowel action of the applied U-nails. It was found in the study that the ultimate tensile strength and shear strength of the concrete increased by 145% and 220%, respectively, with U-nails. The study also recommends U-nails with a filament thickness of 2–2.5 mm for optimal improvement in interlayer strength.
The studies discussed in Section 4.1 on reinforcement are summarized in Table 1. It is predominantly classified based on the phase of printing where the reinforcement is introduced. It also classifies based on the mode of reinforcement addition and the direction of addition. Vertical refers to the reinforcement being added perpendicular to the printing direction and mainly across the printing layers. The horizontal direction of reinforcement addition refers to the addition of reinforcement along the direction of printing. Overall, it is found that adding reinforcing elements directly in the mix using fibers adds little to the strength of the concrete 3DP element. The addition of reinforcement elements after the completion of printing mainly refers to the addition of reinforcement either by penetrating/inserting the printed layers in their fresh state or embedding the rebar in the hollow sections/gaps of the printed layers in their hardened state. Inserting rebars at the hardened state mostly involves printing small individual segments and then assembling them. The bonding between the reinforcing elements and the concrete is brought out by bonding agents, predominantly mortar grouts. In the case of fresh state after the printing process, bonding is brought out due to the insertion of reinforcing elements. The primary concern here is the damage caused to the printed layers. Inserting reinforcing elements during the printing process improves the tensile strength of 3DP elements. Still, it adds complexity to the printing process by adding the complexity of the placement of reinforcing elements and the time associated with it.
A design tool has also been developed in the study by (Craveiro et al., 2017), which helps in the resource-efficient fabrication of 3D-graded structural building components. A material database where all the material characterization data are stored, and this data is used to help in material optimization. This study concludes by recommending the development of a multi-material print head to enable the other resource-efficient graded building of concrete elements.
3D printing technology provides designers with more design freedom for execution. Topological Optimization (TO) effectively exploits design freedom to get optimally shaped engineering structures. Vantyghem et al. (2019) presents a study to determine the optimal printing path and the ideal location for reinforcement, optimal design of fiber-reinforced concrete elements, and minimizing the thermal transmittance through concrete components while the overall material usage is restricted.
A work on powder-based 3DP by (Xiong et al., 2020) proposed a structural connectivity control method based on bi-directional evolutionary structural optimization to solve the problem of removing unbonded powder from the voids by generating tunnels connecting the voids with external boundaries. Though there are numerous research studies on the 3DCP elements, the mechanical behavior of reinforced 3DCP beams is not well understood in comparison with the mechanical behavior of conventional RC beams. The authors (Chandra et al., 2020) propose an analytical model using finite element software to estimate the mechanical behavior of the 3DP RC beams. This study’s analytical model accurately predicted the maximum flexural strength of the 3DP RC beams. But the initial model was found to have limitations in predicting the initial stiffness. These limitations are addressed by (Chandra et al., 2021). The improved analytical model is developed in this study, where the predictions of the local failure were accurately identified. Further, the enhanced analytical model could be expanded to analyze the Precast beams (PC).
The Finite Element (FE) study is also used to model the other common failure mechanisms like a failure due to elastic buckling or failure due to plastic collapse in 3DCP (Nguyen-Van et al., 2022). The developed FE model was found to accurately predict the deformation and failure modes of the 3DP. The FE model was also subjected to other sensitivity and parametric analyses to find the influence of other parameters like printing speed, buildability, and extrusion width.
To apply 3DP to large structures, the evolution of the workability of the mix with time needs to be modeled precisely. Ma and Wang (2018) emphasized the importance of measuring fresh and hardened properties to control the printing mix’s flowability, extrudability, buildability, setting time, mechanical property, and shrinkage properties. Recently, many techniques have been developed for transferring the 3DCP from the laboratory to large-scale construction. Contrary to the laboratory 3DCP, the large-scale monolithic 3DCP requires more complex procedures, and various challenges and potential risks must be considered (Hack et al., 2020; Khan et al., 2020; Souza et al., 2020).
CONPrint3D technology has been developed by (Mechtcherine et al., 2019) as an alternative to manually constructed masonry walls. It is a truck-mounted mobile system with an extended robotic arm. It is a solution for printing using a coarse aggregate mix, like conventional concrete. Still, studies are to be done on improving the positional accuracy while printing, possibly because long manipulating arms get more significant deformations during the printing process. Also, studies can be done in the future regarding the integration of reinforcement into the process, safety issues, harsh weather conditions, etc.
In this method, printing is done using a multi-axis robotic arm which helps in navigating the printing nozzle. Keating et al. (2017) developed a compound arm 3D printing system, which has material storing and transporting ability, and the system can be moved on wheels before and after printing. But the system’s reach where the accurate points can be printed is limited. Also, only fine-grained materials can be printed using this particular system. A system of printing with a mobile team was developed by (Zhang et al., 2018), which helps make the large-scale printing process scalable by printing with limited movement of the robots. Though the study provided a unique way, optimization studies still need to eliminate the clashes within the robots and between the robot and printed structure.
Naboni et al. (2022) presented an environment-aware 3DCP system by employing a depth camera on a robotic system to collect geometric information in the form of a point. The collected information and environment-aware generative design algorithms are used to plan the tool path for the fabrication of the elements. The uneven printing bed, obstacles in the printing environment, etc. are detected from the point cloud, and the generative design path for a space-filling curve is adjusted according to the detected parameters. The results showed good printing-environment awareness of such a system and its influence on the printing process, which can make a huge difference in effectively controlling the printing process in large-scale printing systems.
The WASP Company developed a delta-style 3D printer, and the maximum length of the components generated was about 3 m. There was a clear disadvantage of this approach: assembly and disassembly for every on-site building construction. Tongji University and the Chinese Green print company tried limiting the disadvantage of the WASP company 3D printer by making the gantry system move linearly on rails and print with a limit of 10 m to the structure’s height. The printer used a maximum of 15 mm aggregate size or printing in an on-site construction project (Ji et al., 2019).
In this method of 3DCP, the assemblies are prefabricated at the site and then assembled or installed at the site. In 2016, a footbridge was constructed, “Parque de Castilla Footbridge,” in Madrid. 8 U-shaped segments were printed using extrusion-based 3DCP (de la Fuente et al., 2022). Another bicycle bridge was built by the Eindhoven University of Technology (TU/e) using the “Design by Testing” approach (Salet et al., 2018) in the Netherlands. The ideology was to test every step in an off-site facility before executing it at the original site. This helped in mitigating the challenges and hazards. The bridge was hoisted to the position, and in-situ tests were done by loading the bridge with a total load of 57 kN before opening it for public use.
Assaad et al. (2020) focussed on developing a modular approach for producing 3DP beam and column members using conventional reinforcement bars. In this study, cubic-shaped structures are printed with four holes in the corner to insert longitudinal rebars and grouting. The structural beam is formed by assembling different modules and joining them using high-strength epoxy resin. The flexural moment capacity of 3D printed elements is 22% lower than the cast-in-place conventional concrete beams. The reduction in moment capacity can be attributed to the modular design as the reinforcements, transfer of stress and moments vary significantly from the conventional construction method. This signifies that the geometry and orientation of rebars significantly affect the structural properties of concrete 3DP elements.
Asprone et al. (2018a) studied a novel approach for fabricating a reinforced concrete structure using individual elements of 3DP elements. They developed a mechanism where individual segments of concrete 3D elements are printed and attached using an external reinforcement system. Combinedly, the developed beam acts as a single unit in taking up the flexural loads. On initial testing, the flexural stiffness of the developed beam results is comparable with that of the conventional RC beam. But the failure of the printed elements at local junctions reduced the overall ability of the beam to carry non-linear flexural loads.
3DP of formwork is the most commonly used strategy for fabricating structural columns. In this approach, formwork is printed using different materials per the column’s required shape. Then reinforcement is either kept manually, or techniques like dispersed reinforcing are used, which also involves manual casting.
Burger, Lloret-Fritschi, Taha, et al. (2020) presented a novel approach to combining FDM-3DP formwork and simultaneous casting of a fast-hardening, set-on-demand concrete, the “Eggshell process.” It enabled the printing of a thin formwork using polylactic acid (PLA), polypropylene (PP), and polyethylene terephthalate glycol (PET-G). Though it shows good potential by printing the columns with complex geometry, there is a need for studies in areas such as integration of reinforcement while printing and finding a trade-off between the geometry and the reinforcing placing method to be used.
Anton et al. (2021) introduced a new 3DCP prefabrication platform for customized columns. The study merged computational design and 3DCP and proposed an evaluation method for geometric complexity. A permanent formwork is prefabricated in a complex geometrical shape and the column is cast manually. However, there are still a few points that need to be addressed, the testing of structural properties of the final columns, there was crack formation due to the freeze-thaw cycle at specific parts of the column, and hence a study is required to eliminate the effect.
Permanent 3DCP formwork combines 3DCP with traditional construction methods (Wang et al., 2022). Khoshnevis et al. (2006) did a study on the fabrication of vertical concrete formwork using Contour Crafting (CC) manually inserting ties in between layers and casting the elements manually. It can significantly reduce waste and labor. Studies are required in the direction of the structural performance of 3D printed formwork structures, which depends on coordination between the formwork and post-cast concrete. Wang et al. (2022) did a study to get a relationship between the mechanical properties at the interface of formwork and the casted concrete of the final product, the roughness of 3D printed formwork, and the time gap between the printing of formwork and casting of concrete. The optimum roughness and the time gap between the printing of formwork and casting were presented. An attempt was made by (Katzer and Skoratko, 2022) to 3D print plastic formworks for steel fiber-reinforced concrete columns. Different groups were made based on the shape of the formwork. Experiment and analysis proved plastic-concrete columns to be a good alternative to conventionally cast concrete. Also, this gave great flexibility in casting columns in various shapes (fractal, pentagon, etc.).
3D printing can help develop new and complex geometries of 3D printed formworks which were earlier not possible in traditional formwork. Such structures are very much optimized and improve the functional aspects of the stair structure. A study by (Jipa et al., 2019) involves printing two prototype stair structures to evaluate the potential of 3D printing for the fabrication of stairs. Some of the challenges include the problem of reinforcement, which is difficult to entrain in a complex geometric shape, the concreting freedom, which reduces as the geometry becomes complex and thin-walled, fabrication time, and the overall geometric limitation.
Extrusion is the most commonly used concrete 3D printing technique. It has various advantages and automates the construction process. Yet, it faces challenges like inter-layer bonding, tensile reinforcement integration, table structures with overhang, and final surface (Hack and Kloft, 2020). To overcome these limitations, studies are being conducted to perform concrete printing using the Shotcrete technique. Shotcrete is a method in which concrete is sprayed over the reinforcements with pressure. This technique has been used in construction for a long time (Heidarnezhad and Zhang, 2022). Hence, keeping in mind the challenges faced in the extrusion method and the potential of shotcrete to overcome these challenges (Hack and Kloft, 2020), conducted an experiment by 3D printing a slender reinforced wall element using the shotcrete technique. Though research is still needed to overcome a few limitations, this study demonstrated an alternative and feasible way of 3D Printing, especially the structural elements, to the more common extrusion-based 3D Printing. Later another study was conducted by (Kloft et al., 2020) who worked towards developing a system to print reinforced columns. The study successfully demonstrated a way to integrate conventional reinforcement bars into the 3D concrete printing process. Due to the concept being new in the area of 3D printing, various areas need research, like, structural performance analysis of the shotcrete 3DCP elements, overcoming the positional accuracy barrier, and high surface roughness.
To resolve the issues related to the computational modeling of reinforcement integration into 3D printing (Spangenberg et al., 2022), developed a computational fluid dynamics model that can help stimulate the flow of concrete around reinforcement, this model was validated by experiments, and both numerical and experimental results agreed relatively well.
Mengesha et al. (2023) developed a numerical model using the layer-wise FEM and pseudo-density approach. This approach tried to incorporate the layering feature of 3DCP, and the variation in material properties due to the time dependency of the curing process was captured. The model could reliably estimate the failure mechanisms in a concrete wall. A numerical model was developed by (Asprone et al., 2018a) to predict the flexural response of 3DP RC beams. The RC beam is developed by printing individual 3D-printed elements and joining them together using external reinforcements. The numerical model study helped decipher the impact on the concrete joints and the steel external reinforcement sections, concluding that further in-depth study is required for practical implementation at sites.
This review grouped the publications based on issues addressed and the technologies developed for implementing the 3D printing process of structural elements. From sections 3 and 4, the major problems found concerning structural load-carrying members are discussed in Table 2.
Table 2 gives the areas and the issues/problems that need to be addressed for the successful large-scale implementation of concrete 3DP. Further, the following significant conclusions can be arrived.
• Multiple reinforcement methodologies are available, each with problems and associated issues. Hence, the selection of reinforcement methodology is subjective and shall be decided depending on the application involved.
• A proper combination of mix design (with/without mix reinforcing elements like the addition of fibers), additional reinforcement technique (steel bars/cables, etc.), and the inter-layer bonding technique (materials/design to increase the bonding surface area) is required to achieve maximum structural strength capacities. More studies on the potential combination techniques shall be studied to attain maximum strength-carrying capacities.
• Also, the design and geometry of the elements influence the interlayer bonding strength. This adds to the complexity of the problem in achieving required flexural and tensile strength requirements. It makes this an objective function with multiple parameters that must be solved for large-scale industrial implementation.
• There are many unconventional reinforcement strategies, but reinforcement selection should also consider the automation level. Reinforcements like barbed wires and cables must be synchronized with the printing process and involve much manual work. Automating the entire process is essential to realize the advantages of 3D printing.
• Though there are studies with the entrainment of reinforcement fibers or cables along with the concrete printing process to enhance the structural properties of the element, the evolution of the rheological properties of the concrete with time is not studied extensively. It needs to be given more importance as understanding rheological properties requires application in varied environmental conditions.
Apart from the above points, the review of other structural member’s implementation studies brought out the following points.
• There are limited analytical or numerical models to predict the failure pattern or the fractural energy of the 3D-printed structural members.
• The shotcrete method of printing is gaining interest and momentum. It provides an alternative and faster approach to extrusion-based 3D printing while allowing the integration of reinforcement in a comparatively easier way than the other methods being studied. However, further research is needed on the strength aspects of shotcrete 3D-printed columns and walls. Also, procedures must be well-defined to develop highly automated digital fabrication systems.
• Researchers and organizations are implementing large-scale 3D printing systems. But, there is huge scope for future studies in improving productivity, further automating the process, improving positional accuracy of systems, integrating robots in the process, and incorporating safety issues and weather conditions while developing the process.
• 3D Printed formworks are great in terms of saving the conventional formwork cost and giving flexibility in design. But, due to their complex shapes, reinforcement integration becomes a problem. Also, these formworks need to be tested against harsh climatic conditions they may get exposed to; studies to eliminate these challenges are desired.
• Topology optimization helps in saving the material, making the structure lightweight while maintaining the structural performance. However, studies like incorporating advanced properties like layer cohesion, relaxation shrinkage, the release of hydration energy, incorporating anisotropic behavior of 3D printed material, and a trade-off between geometric complexity and ease of integrating reinforcement are needed for its successful and complete transition into 3D Concrete Printing
The paper summarizes state-of-the-art methodologies related to the fabrication, testing, and optimization of 3D-printed structural elements. It is concluded that research in this area is increasing exponentially. However, it is still in a nascent stage, and more studies are needed to realize 3D printing technology as a large-scale construction technology.
BR developed the review methodology. SS compiled review of concrete 3D printing process. AP and SB compiled literature on reinforced concrete printing technologies.
The authors declare that the research was conducted in the absence of any commercial or financial relationships that could be construed as a potential conflict of interest.
All claims expressed in this article are solely those of the authors and do not necessarily represent those of their affiliated organizations, or those of the publisher, the editors and the reviewers. Any product that may be evaluated in this article, or claim that may be made by its manufacturer, is not guaranteed or endorsed by the publisher.
Al-Qutaifi, S., Nazari, A., and Bagheri, A. (2018). Mechanical properties of layered geopolymer structures applicable in concrete 3D-printing. Constr. Build. Mater 176, 690–699. doi:10.1016/j.conbuildmat.2018.04.195
Amba, J. C., Balayssac, J. P., and Détriché, C. H. (2010). Characterisation of differential shrinkage of bonded mortar overlays subjected to drying. Mater. Structures/Materiaux Constr. 43, 297–308. doi:10.1617/s11527-009-9489-8
Amran, M., Abdelgader, H. S., Onaizi, A. M., Fediuk, R., Ozbakkaloglu, T., Rashid, R. S. M., et al. (2022). 3D-printable alkali-activated concretes for building applications: A critical review. Constr. Build. Mater 319, 126126. doi:10.1016/j.conbuildmat.2021.126126
Anton, A., Jipa, A., Reiter, L., and Dillenburger, B. (2020). Fast complexity - additive manufacturing for bespoke concrete slabs. Springer International Publishing. doi:10.1007/978-3-030-49916-7
Anton, A., Reiter, L., Wangler, T., Frangez, V., Flatt, R. J., and Dillenburger, B. (2021). A 3D concrete printing prefabrication platform for bespoke columns. Autom. Constr. 122, 103467. doi:10.1016/j.autcon.2020.103467
Aramburu, A., Calderon-Uriszar-Aldaca, I., and Puente, I. (2022). Bonding strength of steel rebars perpendicular to the hardened 3D-printed concrete layers. Constr. Build. Mater 340, 127827. doi:10.1016/j.conbuildmat.2022.127827
Asprone, D., Auricchio, F., Menna, C., and Mercuri, V. (2018a). 3D printing of reinforced concrete elements: Technology and design approach. Constr. Build. Mater 165, 218–231. doi:10.1016/j.conbuildmat.2018.01.018
Asprone, D., Menna, C., Bos, F. P., Salet, T. A. M., Mata-Falcón, J., and Kaufmann, W. (2018b). Rethinking reinforcement for digital fabrication with concrete. Cem. Concr. Res. 112, 111–121. doi:10.1016/j.cemconres.2018.05.020
Assaad, J. J., Yassin, A. A., Alsakka, F., and Hamzeh, F. (2020). A modular approach for steel reinforcing of 3D printed concrete-preliminary study. Sustain. Switz. 12, 4062–18. doi:10.3390/SU12104062
Baz, B., Aouad, G., Khalil, N., and Remond, S. (2021). Inter-layer reinforcement of 3D printed concrete elements. Asian J. Civ. Eng. 22, 341–349. doi:10.1007/s42107-020-00317-0
Baz, B., Aouad, G., Leblond, P., Al-Mansouri, O., D’hondt, M., and Remond, S. (2020). Mechanical assessment of concrete – steel bonding in 3D printed elements. Constr. Build. Mater 256, 119457. doi:10.1016/j.conbuildmat.2020.119457
Bi, M., Tran, P., Xia, L., Ma, G., and Xie, Y. M. (2022). Topology optimization for 3D concrete printing with various manufacturing constraints. Addit. Manuf. 57, 102982. doi:10.1016/j.addma.2022.102982
Bi, M., Tran, P., and Xie, Y. M. (2020). Topology optimization of 3D continuum structures under geometric self-supporting constraint. Addit. Manuf. 36, 101422. doi:10.1016/J.ADDMA.2020.101422
Bos, F. P., Ahmed, Z. Y., Jutinov, E. R., and Salet, T. A. M. (2017a). Experimental exploration of metal cable as reinforcement in 3D printed concrete. Materials 10, 1314. doi:10.3390/ma10111314
Bos, F. P., Ahmed, Z. Y., Wolfs, R. J. M., and Salet, T. A. M. (2017b). “3D printing concrete with reinforcement,” in High tech concrete: Where technology and engineering meet - proceedings of the 2017 fib symposium (Springer International Publishing), 2484–2493. doi:10.1007/978-3-319-59471-2_283
Brackett, D., Panesar, A., Ashcroft, I., Wildman, R., and Hague, R. (2013). An optimization based design framework for multi-functional 3D printing. doi:10.26153/TSW/15584
Brameshuber, W. (2006). Report 36: Textile reinforced concrete-state-of-the-art report of RILEM TC 201-TRC. Available at: https://books.google.com/books?hl=en&lr=&id=nV4JmLPlqV0C&oi=fnd&pg=PA1&ots=hWKyXGirjM&sig=4vMbvhlSyEUWCfbmupLSv-HEHSo [Accessed July 28, 2022].
Burger, J., Lloret-Fritschi, E., Taha, N., Scotto, F., Demoulin, T., Mata-Falcón, J., et al. (2020). “Design and fabrication of a non-standard, structural concrete column using eggshell: Ultra-thin, 3D printed formwork,” in RILEM bookseries (Springer), 1104–1115. doi:10.1007/978-3-030-49916-7_105
Buswell, R. A., Leal de Silva, W. R., Jones, S. Z., and Dirrenberger, J. (2018). 3D printing using concrete extrusion: A roadmap for research. Cem. Concr. Res. 112, 37–49. doi:10.1016/j.cemconres.2018.05.006
Cao, X., Yu, S., Cui, H., and Li, Z. (2022). 3D printing devices and reinforcing techniques for extruded cement-based materials: A review. Buildings 12, 453–519. doi:10.3390/buildings12040453
Carstensen, J. v. (2020). Topology optimization with nozzle size restrictions for material extrusion-type additive manufacturing. Struct. Multidiscip. Optim. 62, 2481–2497. doi:10.1007/s00158-020-02620-5
Chandra, J., Pudjisuryadi, P., Antoni, A., and Wibowo, H. (2020). Analytical modeling of 3D-printed reinforced concrete beams. IOP Conf. Ser. Mater Sci. Eng. 930, 012041. doi:10.1088/1757-899X/930/1/012041
Chandra, J., Wibowo, H., Wijaya, D., Purnomo, F. O., Pudjisuryadi, P., and Antoni, A. (2021). Modeling and analysis of 3D-printed reinforced and prestressed concrete beams. IOP Conf. Ser. Earth Environ. Sci. 907, 012009. doi:10.1088/1755-1315/907/1/012009
Cohen, Z. (2019). Hold Up: Machine Delay in Architectural Design, Robotic Fabr. Archit. Art Des. 126–138. doi:10.1007/978-3-319-92294-2_10
Cohen, Z., and Carlson, N. (2020). Piling and pressing: Towards a method of 3D printing reinforced concrete columns. Constr. Robot. 4, 61–73. doi:10.1007/s41693-020-00029-6
Craveiro, F., Bartolo, H. M., Gale, A., Duarte, J. P., and Bartolo, P. J. (2017). A design tool for resource-efficient fabrication of 3d-graded structural building components using additive manufacturing. Autom. Constr. 82, 75–83. doi:10.1016/j.autcon.2017.05.006
Curbach, M. (2013). Concrete light - possibilities and visions. Struct. Concr. 14, 87–88. doi:10.1002/SUCO.201390010
Curbach, M., Graf, W., Jesse, D., Sickert, J. U., and Weiland, S. (2007). Segmentbrücke aus Textilbewehrtem Beton Konstruktion, Fertigung, Numerische Berechnung. Beton- Stahlbetonbau 102, 342–352. doi:10.1002/BEST.200700550
de la Fuente, A., Blanco, A., Galeote, E., and Cavalaro, S. (2022). Structural fibre-reinforced cement-based composite designed for particle bed 3D printing systems. Case study Parque de Castilla Footbridge in Madrid. Cem. Concr. Res. 157, 106801. doi:10.1016/j.cemconres.2022.106801
Ding, L., Wei, R., and Che, H. (2014). Development of a BIM-based automated construction system. Procedia Eng. 85, 123–131. doi:10.1016/J.PROENG.2014.10.536
Falliano, D., de Domenico, D., Ricciardi, G., and Gugliandolo, E. (2020). 3D-printable lightweight foamed concrete and comparison with classical foamed concrete in terms of fresh state properties and mechanical strength. Constr. Build. Mater 254, 119271. doi:10.1016/j.conbuildmat.2020.119271
Farina, I., Fabbrocino, F., Carpentieri, G., Modano, M., Amendola, A., Goodall, R., et al. (2016). On the reinforcement of cement mortars through 3D printed polymeric and metallic fibers. Compos B Eng. 90, 76–85. doi:10.1016/j.compositesb.2015.12.006
Fiuk, G., and Mrzygłód, M. W. (2020). Topology optimization of structures with stress and additive manufacturing constraints. J. Theor. Appl. Mech. 58, 459–468. doi:10.15632/JTAM-PL/118899
Gaynor, A. T., and Guest, J. K. (2016). Topology optimization considering overhang constraints: Eliminating sacrificial support material in additive manufacturing through design. Struct. Multidiscip. Optim. 54, 1157–1172. doi:10.1007/s00158-016-1551-x
Gebhard, L., Mata-Falcón, J., Anton, A., Burger, J., Lloret-Fritschi, E., Reiter, L., et al. (2020). Aligned interlayer fibre reinforcement and post-tensioning as a reinforcement strategy for digital fabrication. RILEM Bookseries 28, 622–631. doi:10.1007/978-3-030-49916-7_63
Gebhard, L., Mata-Falcón, J., Anton, A., Dillenburger, B., and Kaufmann, W. (2021). Structural behaviour of 3D printed concrete beams with various reinforcement strategies. Eng. Struct. 240, 112380. doi:10.1016/j.engstruct.2021.112380
Geneidy, O., Kumarji, S., Dubor, A., and Sollazzo, A. (2020). Simultaneous reinforcement of concrete while 3D printing. RILEM Bookseries, 895–905. doi:10.1007/978-3-030-49916-7_87
Gries, T., Raina, M., Quadflieg, T., and Stolyarov, O. (2016). Textile Fibre Composites in Civil Engineering, 3–24. doi:10.1016/B978-1-78242-446-8.00002-1Manufacturing of textiles for civil engineering applications
Hack, N., Dörfler, K., Walzer, A. N., Wangler, T., Mata-Falcón, J., Kumar, N., et al. (2020). Structural stay-in-place formwork for robotic in situ fabrication of non-standard concrete structures: A real scale architectural demonstrator. Autom. Constr. 115, 103197. doi:10.1016/J.AUTCON.2020.103197
Hack, N., and Kloft, H. (2020). Shotcrete 3D printing technology for the fabrication of slender fully reinforced freeform concrete elements with high surface quality: A real-scale demonstrator. RILEM Bookseries 28, 1128–1137. doi:10.1007/978-3-030-49916-7_107
Hack, N., and Lauer, W. V. (2014). Mesh-mould: Robotically fabricated spatial meshes as reinforced concrete formwork. Archit. Des. 84, 44–53. doi:10.1002/ad.1753
Hambach, M., and Volkmer, D. (2017). Properties of 3D-printed fiber-reinforced Portland cement paste. Cem. Concr. Compos 79, 62–70. doi:10.1016/j.cemconcomp.2017.02.001
Hass, L., and Bos, F. (2020). Bending and pull-out tests on a novel screw type reinforcement for extrusion-based 3D printed concrete. RILEM Bookseries 28, 632–645. doi:10.1007/978-3-030-49916-7_64
Heidarnezhad, F., and Zhang, Q. (2022). Shotcrete based 3D concrete printing: State of art, challenges, and opportunities. Constr. Build. Mater 323, 126545. doi:10.1016/j.conbuildmat.2022.126545
Heineman, J. J., Lind, R. F., Chesser, P. C., Post, B. K., Boulger, A. M., Roschli, A., et al. (2019). “Exploration of a cable-driven 3D printer for concrete tower structures. Solid Freeform Fabrication,” in Proceedings of the 30th Annual International Solid Freeform Fabrication Symposium - An Additive Manufacturing Conference (Knoxville, Tennessee: Oak Ridge National Laboratory), 1853–1860Available at: https://repositories.lib.utexas.edu/bitstream/handle/2152/90523/2019-154-Heineman.pdf?sequence=2&isAllowed=y.
Hojati, M., Memari, A. M., Zahabi, M., Wu, Z., Li, Z., Park, K., et al. (2022). Barbed-wire reinforcement for 3D concrete printing. Autom. Constr. 141, 104438. doi:10.1016/j.autcon.2022.104438
Izadgoshasb, H., Kandiri, A., Shakor, P., Laghi, V., and Gasparini, G. (2021). Predicting compressive strength of 3D printed mortar in structural members using machine learning. Appl. Sci. Switz. 11, 10826. doi:10.3390/app112210826
Ji, G., Ding, T., Xiao, J., Du, S., Li, J., and Duan, Z. (2019). A 3D printed ready-mixed concrete power distribution substation: Materials and construction technology. Materials 12, 1540. doi:10.3390/ma12091540
Jipa, A., and Dillenburger, B. (2022). 3D printed formwork for concrete: State-of-the-Art, opportunities, challenges, and applications. 3D Print Addit. Manuf. 9, 84–107. doi:10.1089/3dp.2021.0024
Jipa, A., Giacomarra, F., Giesecke, R., Chousou, G., Pacher, M., Dillenburger, B., et al. (2019). “3D-printed formwork for bespoke concrete stairs from computational design to digital fabrication,” in Proceedings: SCF 2019 - ACM Symposium on Computational Fabrication. doi:10.1145/3328939.3329003
Katzer, J., and Skoratko, A. (2022). Using 3D printed formworks for the creation of steel fibre reinforced concrete-plastic columns. Constr. Build. Mater 337, 127586. doi:10.1016/j.conbuildmat.2022.127586
Keating, S. J., Leland, J. C., Cai, L., and Oxman, N. (2017). Toward site-specific and self-sufficient robotic fabrication on architectural scales. Sci. Robot. 2, eaam8986. doi:10.1126/SCIROBOTICS.AAM8986
Khan, M. S., Sanchez, F., and Zhou, H. (2020). 3-D printing of concrete: Beyond horizons. Cem. Concr. Res. 133, 106070. doi:10.1016/J.CEMCONRES.2020.106070
Khoshnevis, B., Carlson, A., Leach, N., and Thangavelu, M. (2012). “Contour crafting simulation plan for lunar settlement infrastructure buildup,” in Proceeding of the Thirteenth ASCE Aerospace Division Conference on Engineering, Science. doi:10.1061/9780784412190.155
Khoshnevis, B., Hwang, D., Yao, K.-T., and Yeh, Z. (2006). Mega-scale fabrication by contour crafting. Int. J. Industrial Syst. Eng. 1, 301–320. doi:10.1504/IJISE.2006.009791
Kim, K., Park, S., Kim, W. S., Jeong, Y., and Lee, J. (2017). Evaluation of shear strength of RC beams with multiple interfaces formed before initial setting using 3D printing technology. Materials 10, 1349. doi:10.3390/ma10121349
Kizilkanat, A. B., Kabay, N., Akyüncü, V., Chowdhury, S., and Akça, A. H. (2015). Mechanical properties and fracture behavior of basalt and glass fiber reinforced concrete: An experimental study. Constr. Build. Mater 100, 218–224. doi:10.1016/j.conbuildmat.2015.10.006
Kloft, H., Empelmann, M., Hack, N., Herrmann, E., and Lowke, | D. (2020). Reinforcement strategies for 3D-concrete-printing. Civ. Eng. Des. 2, 131–139. doi:10.1002/CEND.202000022
Kreiger, E. L., Kreiger, M. A., and Case, M. P. (2019). Development of the construction processes for reinforced additively constructed concrete. Addit. Manuf. 28, 39–49. doi:10.1016/j.addma.2019.02.015
Kuo, Y.-H., Cheng, C.-C., Lin, Y.-S., and San, C.-H. (2018). Support structure design in additive manufacturing based on topology optimization. Struct. Multidiscip. Optim. 57, 183–195. doi:10.1007/s00158-017-1743-z
Langelaar, M. (2017). An additive manufacturing filter for topology optimization of print-ready designs. Struct. Multidiscip. Optim. 55, 871–883. doi:10.1007/S00158-016-1522-2/FIGURES/11
Langelaar, M. (2016). Topology optimization of 3D self-supporting structures for additive manufacturing. Addit. Manuf. 12, 60–70. doi:10.1016/j.addma.2016.06.010
Lao, W., Li, M., and Tjahjowidodo, T. (2021). Variable-geometry nozzle for surface quality enhancement in 3D concrete printing. Addit. Manuf. 37, 101638. doi:10.1016/J.ADDMA.2020.101638
Larsen, I. L., and Thorstensen, R. T. (2020). The influence of steel fibres on compressive and tensile strength of ultra high performance concrete: A review. Constr. Build. Mater 256, 119459. doi:10.1016/j.conbuildmat.2020.119459
Latifi Rostami, S. A., Ghoddosian, A., Kolahdooz, A., and Zhang, J. (2022). Topology optimization of continuum structures under geometric uncertainty using a new extended finite element method. Eng. Optim. 54, 1692–1708. doi:10.1080/0305215x.2021.1957860
Le, T. T., Austin, S. A., Lim, S., Buswell, R. A., Law, R., Gibb, A. G. F., et al. (2012). Hardened properties of high-performance printing concrete. Cem. Concr. Res. 42, 558–566. doi:10.1016/J.CEMCONRES.2011.12.003
Li, V. C., Bos, F. P., Yu, K., McGee, W., Ng, T. Y., Figueiredo, S. C., et al. (2020a). On the emergence of 3D printable engineered, strain hardening cementitious composites (ECC/SHCC). Cem. Concr. Res. 132, 106038. doi:10.1016/j.cemconres.2020.106038
Li, Z., Hojati, M., Wu, Z., Piasente, J., Ashrafi, N., Duarte, J. P., et al. (2020b). Fresh and hardened properties of extrusion-based 3D-printed cementitious materials: A review. Sustain. Switz. 12, 5628–5633. doi:10.3390/su12145628
Liu, H., Liu, C., Wu, Y., Bai, G., He, C., Yao, Y., et al. (2022). 3D printing concrete with recycled coarse aggregates: The influence of pore structure on interlayer adhesion. Cem. Concr. Compos 134, 104742. doi:10.1016/J.CEMCONCOMP.2022.104742
Liu, M., Zhang, Q., Tan, Z., Wang, L., Li, Z., and Ma, G. (2021). Investigation of steel wire mesh reinforcement method for 3D concrete printing. Archives Civ. Mech. Eng. 21, 24–18. doi:10.1007/s43452-021-00183-w
Lloret-Fritschi, E., Scotto, F., Gramazio, F., Kohler, M., Graser, K., Wangler, T., et al. (2019). Challenges of real-scale production with smart dynamic casting. RILEM Bookseries 19, 299–310. doi:10.1007/978-3-319-99519-9_28
Lu, B., Weng, Y., Li, M., Qian, Y., Leong, K. F., Tan, M. J., et al. (2019). A systematical review of 3D printable cementitious materials. Constr. Build. Mater 207, 477–490. doi:10.1016/j.conbuildmat.2019.02.144
Ma, G., Bai, G., Wang, L., and Wang, F. (2022). Explosion resistance of 3D printing ultra-high performance concrete based on contact explosion tests. Int. J. Impact Eng. 169, 104316. doi:10.1016/J.IJIMPENG.2022.104316
Ma, G., and Wang, L. (2018). A critical review of preparation design and workability measurement of concrete material for largescale 3D printing. Front. Struct. Civ. Eng. 12, 382–400. doi:10.1007/s11709-017-0430-x
Marchment, T., and Sanjayan, J. (2020a). Bond properties of reinforcing bar penetrations in 3D concrete printing. Autom. Constr. 120, 103394. doi:10.1016/j.autcon.2020.103394
Marchment, T., and Sanjayan, J. (2020b). Mesh reinforcing method for 3D concrete printing. Autom. Constr. 109, 102992. doi:10.1016/j.autcon.2019.102992
Markin, V., Sahmenko, G., Nerella, V. N., Nather, M., and Mechtcherine, V. (2019). Investigations on the foam concrete production techniques suitable for 3D-printing with foam concrete. IOP Conf. Ser. Mater Sci. Eng. 660, 012039. doi:10.1088/1757-899X/660/1/012039
Martens, P., Mathot, M., Bos, F., and Coenders, J. (2018). Optimising 3D printed concrete structures using topology optimisation. fib Symp., 301–309. doi:10.1007/978-3-319-59471-2_37/FIGURES/1
McGee, W., Ng, T. Y., Yu, K., and Li, V. C. (2020). Extrusion nozzle shaping for improved 3DP of engineered cementitious composites (ECC/SHCC). RILEM Bookseries 28, 916–925. doi:10.1007/978-3-030-49916-7_89
Mechtcherine, V., Michel, A., Liebscher, M., and Schmeier, T. (2020a). Extrusion-based additive manufacturing with carbon reinforced concrete: Concept and feasibility study. Materials 13, 2568. doi:10.3390/ma13112568
Mechtcherine, V., Michel, A., Liebscher, M., Schneider, K., and Großmann, C. (2020b). Mineral-impregnated carbon fiber composites as novel reinforcement for concrete construction: Material and automation perspectives. Autom. Constr. 110, 103002. doi:10.1016/j.autcon.2019.103002
Mechtcherine, V., Nerella, V. N., Will, F., Näther, M., Otto, J., and Krause, M. (2019). Large-scale digital concrete construction – CONPrint3D concept for on-site, monolithic 3D-printing. Autom. Constr. 107, 102933. doi:10.1016/J.AUTCON.2019.102933
Meisel, N. A., Watson, N., Bilén, S. G., Duarte, J. P., and Nazarian, S. (2022). Design and system considerations for construction-scale concrete additive manufacturing in remote environments via robotic arm deposition. 3D Print Addit. Manuf. 9, 35–45. doi:10.1089/3dp.2020.0335
Mengesha, M., Schmidt, A., Göbel, L., and Lahmer, T. (2023). Numerical modeling of 3D concrete printing wall structure to reliably estimate the failure mechanisms. RILEM Bookseries 38, 240–247. doi:10.1007/978-3-031-07746-3_24
Naboni, R., Breseghello, L., and Sanin, S. (2022), Environment-aware 3D concrete printing through robot-vision.
Nerella, V. N., Hempel, S., and Mechtcherine, V. (2019). Effects of layer-interface properties on mechanical performance of concrete elements produced by extrusion-based 3D-printing. Constr. Build. Mater 205, 586–601. doi:10.1016/J.CONBUILDMAT.2019.01.235
Nguyen, N. T., Bui, T. T., and Bui, Q. B. (2022). Fiber reinforced concrete for slabs without steel rebar reinforcement: Assessing the feasibility for 3D-printed individual houses. Case Stud. Constr. Mater. 16, e00950. doi:10.1016/J.CSCM.2022.E00950
Nguyen-Van, V., Nguyen-Xuan, H., Panda, B., and Tran, P. (2022). 3D concrete printing modelling of thin-walled structures. Structures 39, 496–511. doi:10.1016/j.istruc.2022.03.049
Panda, B., Chandra Paul, S., and Jen Tan, M. (2017a). Anisotropic mechanical performance of 3D printed fiber reinforced sustainable construction material. Mater Lett. 209, 146–149. doi:10.1016/j.matlet.2017.07.123
Panda, B., Mohamed, N. A. N., Paul, S. C., Singh, G. V. P. B., Tan, M. J., and Šavija, B. (2019a). The effect of material fresh properties and process parameters on buildability and interlayer adhesion of 3D printed concrete. Materials 12, 2149. doi:10.3390/ma12132149
Paolini, A., Kollmannsberger, S., and Rank, E. (2019). Additive manufacturing in construction : A review on processes , applications , and digital planning methods. Addit. Manuf. 30, 100894. doi:10.1016/j.addma.2019.100894
Paul, S. C., van Zijl, G. P. A. G., and Gibson, I. (2018). A review of 3D concrete printing systems and materials properties: Current status and future research prospects. Rapid Prototyp. J. 24, 784–798. doi:10.1108/RPJ-09-2016-0154
Perkins, I., and Skitmore, M. (2015). Three-dimensional printing in the construction industry: A review. Int. J. Constr. Manag. 15, 1–9. doi:10.1080/15623599.2015.1012136
Perrot, A., Jacquet, Y., Rangeard, D., Courteille, E., and Sonebi, M. (2020). Nailing of layers: A promisingway to reinforce concrete 3D printing structures. Materials 13, 1518. doi:10.3390/ma13071518
Perrot, A., Rangeard, D., and Pierre, A. (2016). Structural built-up of cement-based materials used for 3D-printing extrusion techniques. Mater. Structures/Materiaux Constr. 49, 1213–1220. doi:10.1617/s11527-015-0571-0
Rahul, A. v., and Santhanam, M. (2020). Evaluating the printability of concretes containing lightweight coarse aggregates. Cem. Concr. Compos 109, 103570. doi:10.1016/J.CEMCONCOMP.2020.103570
Rushing, T. S., Al-Chaar, G., Eick, B. A., Burroughs, J., Shannon, J., Barna, L., et al. (2017). Investigation of concrete mixtures for additive construction. Rapid Prototyp. J. 23, 74–80. doi:10.1108/rpj-09-2015-0124
Safonov, A. A. (2019). 3D topology optimization of continuous fiber-reinforced structures via natural evolution method. Compos Struct. 215, 289–297. doi:10.1016/J.COMPSTRUCT.2019.02.063
Şahin, H. G., and Mardani-Aghabaglou, A. (2022). Assessment of materials, design parameters and some properties of 3D printing concrete mixtures; a state-of-the-art review. Constr. Build. Mater 316, 125865. doi:10.1016/j.conbuildmat.2021.125865
Salet, T. A. M., Ahmed, Z. Y., Bos, F. P., and Laagland, H. L. M. (2018). Design of a 3D printed concrete bridge by testing. doi:10.1080/17452759.2018.147606413,222–23610.1080/17452759.2018.1476064
Scheurer, M., Dittel, G., and Gries, T. (2020). Potential for the integration of continuous fiber-based reinforcements in digital concrete production. doi:10.1007/978-3-030-49916-7_70
Schmidt, M. P., Couret, L., Gout, C., and Pedersen, C. B. W. (2020). Structural topology optimization with smoothly varying fiber orientations. Struct. Multidiscip. Optim. 62, 3105–3126. doi:10.1007/s00158-020-02657-6
Schneider, K., Michel, A., Liebscher, M., and Mechtcherine, V. (2018). Verbundverhalten mineralisch gebundener und polymergebundener Bewehrungsstrukturen aus Carbonfasern bei Temperaturen bis 500 °C. Beton- Stahlbetonbau 113, 886–894. doi:10.1002/BEST.201800072
Scholzen, A., Chudoba, R., and Hegger, J. (2012). Dünnwandiges Schalentragwerk aus textilbewehrtem Beton: Entwurf, Bemessung und baupraktische Umsetzung. Beton- Stahlbetonbau 107, 767–776. doi:10.1002/BEST.201200044
Shakor, P., Nejadi, S., and Paul, G. (2019a2019). A study into the effect of different nozzles shapes and fibre-reinforcement in 3D printed mortar. Materials 12, 1708. doi:10.3390/MA12101708
Shakor, P., Nejadi, S., Paul, G., and Malek, S. (2019b). Review of emerging additive manufacturing technologies in 3d printing of cementitious materials in the construction industry. Front. Built Environ. 4. doi:10.3389/fbuil.2018.00085
Shakor, P., Nejadi, S., Sutjipto, S., Paul, G., and Gowripalan, N. (2020). Effects of deposition velocity in the presence/absence of E6-glass fibre on extrusion-based 3D printed mortar. Addit. Manuf. 32, 101069. doi:10.1016/j.addma.2020.101069
Shakor, P., Renneberg, J., Nejadi, S., and Paul, G. (2017). Optimisation of different concrete mix designs for 3D printing by utilizing 6DOF industrial robot. ISARC 2017 - Proc. 34th Int. Symposium Automation Robotics Constr., 268–275. doi:10.22260/ISARC2017/0036
Siddika, A., Mamun, M. A., Ferdous, W., Saha, A. K., and Alyousef, R. (2020). 3D-printed concrete: Applications, performance, and challenges. J. Sustain Cem. Based Mater 9, 127–164. doi:10.1080/21650373.2019.1705199
Soltan, D. G., and Li, V. C. (2018). A self-reinforced cementitious composite for building-scale 3D printing. Cem. Concr. Compos 90, 1–13. doi:10.1016/j.cemconcomp.2018.03.017
Souza, M. T., Ferreira, I. M., Guzi de Moraes, E., Senff, L., and Novaes de Oliveira, A. P. (2020). 3D printed concrete for large-scale buildings: An overview of rheology, printing parameters, chemical admixtures, reinforcements, and economic and environmental prospects. J. Build. Eng. 32, 101833. doi:10.1016/J.JOBE.2020.101833
Spangenberg, J., Leal da Silva, W. R., Mollah, M. T., Comminal, R., Juul Andersen, T., and Stang, H. (2022). Integrating reinforcement with 3D concrete printing: Experiments and numerical modelling. RILEM Bookseries, 379–384. doi:10.1007/978-3-031-06116-5_56
Strano, G., Hao, L., Everson, R. M., and Evans, K. E. (2013). A new approach to the design and optimisation of support structures in additive manufacturing. Int. J. Adv. Manuf. Technol. 66, 1247–1254. doi:10.1007/s00170-012-4403-x
Sun, J., Aslani, F., Lu, J., Wang, L., Huang, Y., and Ma, G. (2021). Fibre-reinforced lightweight engineered cementitious composites for 3D concrete printing. Ceram. Int. 47, 27107–27121. doi:10.1016/j.ceramint.2021.06.124
Tay, Y. W. D., Li, M. Y., and Tan, M. J. (2019a). Effect of printing parameters in 3D concrete printing: Printing region and support structures. J. Mater Process Technol. 271, 261–270. doi:10.1016/J.JMATPROTEC.2019.04.007
Tay, Y. W. D., Ting, G. H. A., Qian, Y., Panda, B., He, L., and Tan, M. J. (2019b). Time gap effect on bond strength of 3D-printed concrete. Virtual Phys. Prototyp. 14, 104–113. doi:10.1080/17452759.2018.1500420
Tiryaki, M. E., Zhang, X., and Pham, Q. C. (2019). Printing-while-moving: A new paradigm for large-scale robotic 3D printing. In Proceeding of the IEEE International Conference on Intelligent Robots and Systems, 2286–2291. doi:10.1109/IROS40897.2019.8967524
Vanek, J., Galicia, J. A. G., and Benes, B. (2014). Clever support: Efficient support structure generation for digital fabrication. Comput. Graph. Forum 33, 117–125. doi:10.1111/CGF.12437
Vantyghem, G., Boel, V., and Corte, W. D. (2019). Compliance, stress-based and multi-physics topology optimization for 3D-printed concrete structures. Springer International Publishing. doi:10.1007/978-3-319-99519-9
Vantyghem, G., de Corte, W., Shakour, E., and Amir, O. (2020). 3D printing of a post-tensioned concrete girder designed by topology optimization. Autom. Constr. 112, 103084. doi:10.1016/J.AUTCON.2020.103084
Wang, L., Jiang, H., Li, Z., and Ma, G. (2020). Mechanical behaviors of 3D printed lightweight concrete structure with hollow section. Archives Civ. Mech. Eng. 20, 16. doi:10.1007/s43452-020-00017-1
Wang, L., Ma, G., Liu, T., Buswell, R., and Li, Z. (2021). Interlayer reinforcement of 3D printed concrete by the in-process deposition of U-nails. Cem. Concr. Res. 148, 106535. doi:10.1016/j.cemconres.2021.106535
Wang, L., Yang, Y., Yao, L., and Ma, G. (2022). Interfacial bonding properties of 3D printed permanent formwork with the post-casted concrete. Cem. Concr. Compos 128, 104457. doi:10.1016/j.cemconcomp.2022.104457
Weng, Y., Li, M., Zhang, D., Tan, M. J., and Qian, S. (2021). Investigation of interlayer adhesion of 3D printable cementitious material from the aspect of printing process. Cem. Concr. Res. 143, 106386. doi:10.1016/j.cemconres.2021.106386
Wolfs, R. J. M., Bos, F. P., and Salet, T. A. M. (2018). Early age mechanical behaviour of 3D printed concrete: Numerical modelling and experimental testing. Cem. Concr. Res. 106, 103–116. doi:10.1016/J.CEMCONRES.2018.02.001
Wu, P., Wang, J., and Wang, X. (2016). A critical review of the use of 3-D printing in the construction industry. Autom. Constr. 68, 21–31. doi:10.1016/j.autcon.2016.04.005
Xiong, Y., Yao, S., Zhao, Z.-L., and Xie, Y. M. (2020). A new approach to eliminating enclosed voids in topology optimization for additive manufacturing. Addit. Manuf. 32, 101006. doi:10.1016/j.addma.2019.101006
Yang, K. K., Zhu, J. H., Wang, C., Jia, D. S., Song, L. L., and Zhang, W. H. (2018). Experimental validation of 3D printed material behaviors and their influence on the structural topology design. Comput. Mech. 61, 581–598. doi:10.1007/s00466-018-1537-1
Yang, R., Zhu, Y., Lan, Y., Zeng, Q., Peng, Y., and Wang, Z. (2022a). Differences in micro grain/fiber distributions between matrix and interlayer of cementitious filaments affected by extrusion molding. Addit. Manuf. 60, 103236. doi:10.1016/j.addma.2022.103236
Yang, Y., Wu, C., Liu, Z., Wang, H., and Ren, Q. (2022b). Mechanical anisotropy of ultra-high performance fibre-reinforced concrete for 3D printing. Cem. Concr. Compos 125, 104310. doi:10.1016/J.CEMCONCOMP.2021.104310
Zhang, J., Wang, J., Dong, S., Yu, X., and Han, B. (2019). A review of the current progress and application of 3D printed concrete. Compos Part A Appl. Sci. Manuf. 125, 105533. doi:10.1016/j.compositesa.2019.105533
Zhang, X., Li, M., Lim, J. H., Weng, Y., Tay, Y. W. D., Pham, H., et al. (2018). Large-scale 3D printing by a team of mobile robots. Autom. Constr. 95, 98–106. doi:10.1016/J.AUTCON.2018.08.004
Zhu, B., Pan, J., Zhou, Z., and Cai, J. (2021). Mechanical properties of engineered cementitious composites beams fabricated by extrusion-based 3D printing. Eng. Struct. 238, 112201. doi:10.1016/j.engstruct.2021.112201
Keywords: concrete 3D printing, structural elements, reinforcement methods, mechanical properties, large-scale implementation, topology optimization, numerical modelling
Citation: Raphael B, Senthilnathan S, Patel A and Bhat S (2023) A review of concrete 3D printed structural members. Front. Built Environ. 8:1034020. doi: 10.3389/fbuil.2022.1034020
Received: 01 September 2022; Accepted: 08 December 2022;
Published: 04 January 2023.
Edited by:
Vagelis Plevris, Qatar University, QatarReviewed by:
Mahua Mukherjee, Indian Institute of Technology Roorkee, IndiaCopyright © 2023 Raphael, Senthilnathan, Patel and Bhat. This is an open-access article distributed under the terms of the Creative Commons Attribution License (CC BY). The use, distribution or reproduction in other forums is permitted, provided the original author(s) and the copyright owner(s) are credited and that the original publication in this journal is cited, in accordance with accepted academic practice. No use, distribution or reproduction is permitted which does not comply with these terms.
*Correspondence: Benny Raphael, YmVubnlAaWl0bS5hYy5pbg==
Disclaimer: All claims expressed in this article are solely those of the authors and do not necessarily represent those of their affiliated organizations, or those of the publisher, the editors and the reviewers. Any product that may be evaluated in this article or claim that may be made by its manufacturer is not guaranteed or endorsed by the publisher.
Research integrity at Frontiers
Learn more about the work of our research integrity team to safeguard the quality of each article we publish.