- 1Department of the Built Environment, Aalborg University, Copenhagen, Denmark
- 2ETH Zürich, Institute of Construction and Infrastructure Management (IBI), Chair of Sustainable Construction, Zürich, Switzerland
Buildings play a vital role in reaching the targets stated by the Intergovernmental Panel on Climate Change to limit global warming to 1.5 degrees. Increasing the use of wood in construction is a proposed upcoming strategy to reduce the embodied greenhouse gas emissions of buildings. This study examines existing life cycle assessments of wooden buildings. The aim is to investigate embodied greenhouse gas emission results reported, as well as methodological approaches applied in existing literature. The study applies the protocol for Systematic Literature Reviews and finds 79 relevant papers. From the final sample, the study analyses 226 different scenarios in-depth in terms of embodied emissions, life cycle assessment method, life cycle inventory modelling and biogenic carbon approach. The analysis shows that the average reported values of embodied greenhouse gas emissions of wooden buildings are one-third to half of the embodied emissions reported from buildings in general. Additionally, from the analysis of the final sample we find that the majority of wooden building life cycle assessments apply similar methods and often leave out biogenic carbon from the assessment or simply do not declare it. This implies that the focus on variability in the different methods applied in wooden building life cycle assessments needs to be increased to establish the relationship between methodological choices and embodied emissions of wooden buildings. Further, transparency and conformity in biogenic carbon accounting in life cycle assessments is essential to enhance comparability between life cycle assessment studies and to avoid distortions in embodied GHG emission results.
Introduction
Sustainability and the Built Environment
The anthropogenic impact on the basic functioning of the Earth System are increasing and the ecological stability may now be threatened (Steffen et al., 2004; Rockström et al., 2009; Steffen et al., 2015; Hildebrandt, 2016). Because of this, the sustainability agenda continues to gain attention by consumers, decision-makers and industries all over the world (Hildebrandt, 2016). In 2018, the Intergovernmental Panel on Climate Change stated that all anthropogenic activities must reach a level of net zero Greenhouse Gas (GHG) emissions by 2050 to limit global warming to 1.5 degrees and to avoid climate crisis (IPCC, 2018). Further, in 2015 measures on climate change mitigation were also integrated into the Sustainability Development Goals to support the sustainable transformation of our society (United Nations, 2015).
Buildings play an important role in reaching GHG emission reduction targets and in the transformation towards a sustainable society. Globally, buildings and construction account for 39% of global energy related GHG emissions (International Energy Agency for Global Alliance for Buildings and Construction, 2019). Of this sector contribution, 28% relates to the operational GHG emissions and the remaining 11% relates to the energy used to produce building and construction materials (also referred to as the embodied emissions) (International Energy Agency for Global Alliance for Buildings and Construction, 2019; World Green Building Council, 2019). Even though the building sector in many years have focused on reducing the operational energy emissions, recent studies suggest that buildings still hold a potential to reduce the GHG emissions from buildings by at least a factor 2.5 (Zimmermann et al., 2020a). To reach this potential, both the emissions from operational energy as well as the embodied emissions must be reduced.
An established method to quantify the emissions of a building is Life Cycle Assessment (LCA). LCA is a standardized method to assess the environmental impacts throughout the life cycle of a product or process under study (European Commission and Joint Research Centre, 2012; Hauschild et al., 2018). In an European context, the standards that frame LCAs on a building and building product level are EN 15978 and EN 15804 (CEN, 2019; CEN, 2012). The standards define the overall structure of building LCAs as well as relevant life cycle stages (see Figure 1). Traditionally, the standards as well as building LCAs in general take basis in the attributional approach to LCA, which focus on answering the question “what environmental impact is the product responsible for?” (Hauschild et al., 2018). However, another approach that is relevant in the context of building LCAs, but not as commonly applied, is consequential LCAs. Consequential LCA focus on answering the question “what are the environmental consequences of consuming the product?” (Hauschild et al., 2018). In other words, consequential LCAs consider future impacts that may happen due to a change in demand whereas attributional LCAs consider impacts that has happened and can be attributed to the product or activity under study (Weidema, 2003; Curran et al., 2005; Bamber et al., 2020).
Wooden Buildings and Reducing the Embodied GHG Emissions
As reducing the embodied GHG emissions of buildings become increasingly important different sustainability strategies gains significance. Examples of sustainability strategies, which are increasingly important in relation to the built environment, are circular economy (Fellner et al., 2017; Pomponi and Moncaster, 2017; Ghisellini et al., 2018; Nußholz et al., 2019; Andersen et al., 2020), design for disassembly (Densley Tingley and Davison, 2012; Eberhardt et al., 2019), renovating rather than building new and using more wood in construction (Salazar and Meil, 2009; Sodagar et al., 2011; Carcassi et al., 2020; Churkina et al., 2020).
In recent years in particular, the focus on wood in construction has increased, and buildings containing a larger share of wood-based materials are getting more and more common (Jensen and Craig, 2019). Through photosynthesis, wood absorbs and sequesters CO2 from the air, resulting in a decrease of the atmospheric CO2 level (Prentice et al., 2001). The carbon sequestered by wood and other bio-based materials are commonly referred to as biogenic carbon. At the end of life, when the wood either decomposes or is incinerated at incineration plants, the sequestered carbon is released back into the air, resulting in an equivalent increase of atmospheric CO2 (Prentice et al., 2001; Brandão et al., 2013; Hoxha et al., 2020). Due to woods’ inherent ability to absorb and release CO2 from and to the atmosphere, an increased use of wood in buildings is often promoted as a strategy to reduce the embodied GHG emissions of buildings (Prentice et al., 2001). However, when seeking to quantify the embodied GHG emissions of a building, these inherent mechanisms of wood cause further complexities and as a consequence, skepticism regarding the ‘true’ environmental potential of wood in construction remains.
Methodological Challenges of Wooden Building LCAs
In traditional building LCAs, two different approaches to account for biogenic carbon uptake and release in wood-based materials exist. The first approach excludes biogenic carbon completely from the assessment under the assumption that wood is carbon neutral. The approach is commonly referred to as the 0/0 approach, where the CO2 uptake during trees’ growth is equivalent to the CO2 released at the end of life (Hoxha et al., 2020). In contrast, the second approach to account biogenic carbon of wood products is referred to as the −1/+1 approach. The −1/+1 approach includes biogenic carbon in the assessment and as for the 0/0 approach, the −1/+1 approach accounts biogenic carbon as carbon neutral during the life cycle (Hoxha et al., 2020). Even though, the −1/+1 approach includes biogenic carbon following the standardized method to calculate the biogenic carbon content in wood (CEN, 2014). In the product stage the uptake of CO2 is accounted as −1, whereas in the end of life stage the release of CO2 is accounted as an equivalent +1 (see Figure 2) (Hoxha et al., 2020). If applied correctly, the two different approaches should provide the same results. However, the −1/+1 approach poses a risk of misleading results in cases where only the uptake of biogenic carbon is included in the assessment. On the other hand, the −1/+1 approach has the advantage of increasing the transparency of the biogenic carbon flows throughout the life cycle, although the representation of the biogenic carbon flow - and the timing of it - is simplified. Further, it should be noted that the fundamental assumption that wood is carbon neutral throughout the life cycle only proves correct if the wood is harvested sustainably.
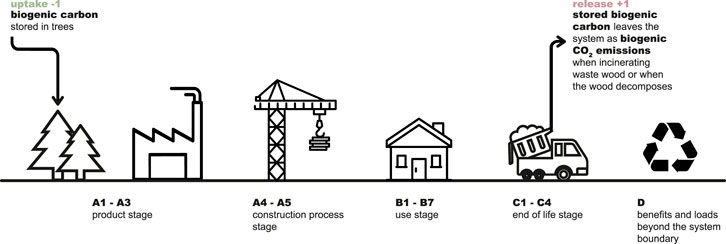
FIGURE 2. Overview of biogenic carbon counting in a building life cycle according to the −1/+1 approach.
A further complexity regarding LCA of wooden buildings is the timing of biogenic carbon uptake and release. Traditional building LCAs aggregate all emissions that occur during the life cycle of a building into one point in time, typically corresponding to the production year (Hellweg et al., 2003; Ryberg et al., 2018; Hoxha et al., 2020). This means that the time in which the emissions occur are insignificant and that all emissions are given the same weight no matter when they occur (Hellweg et al., 2003). To accommodate this issue (Levasseur et al., 2010), developed the dynamic LCA (DLCA) approach, where emissions are provided with a characterization factor for the point in time they occur (Levasseur et al., 2010).
Finally, another discussed issue regarding increasing the use of wood in buildings is the sole focus on one environmental aspect, namely GHG emissions. The sole focus on GHG emissions poses a risk of burdens shifting to other environmental aspects. For instance, human managed land has proven to have an enormous impact on the environments’ ability to sequester carbon (Jørgensen, 2014; De Rosa et al., 2018; Stiebert et al., 2019; Hoxha et al., 2020). Therefore, land use and land use change are important aspects to consider when evaluating if increasing the use of wood in buildings can, in fact, reduce the environmental impact of buildings.
Research Questions
To eliminate some of these uncertainties regarding the methods, it is relevant to assess which methods existing literature apply, and how different methodological approaches affect the reported embodied GHG emissions. Thus, this study investigates LCA case studies of wooden buildings to answer the following research questions:
I. Which embodied GHG emission results are reported in existing literature for wooden building LCAs?
II. How are methodological issues related to wooden building LCAs addressed in existing literature?
Methods
Systematic Compilation of Literature
The literature was collected and analyzed following the protocol for Systematic Literature Review (SLR) as well as the ‘snowball’ approach (de Almeida Biolchini et al., 2007; Higgins and Green, 2008; Wohlin, 2014). Based on the research aim, we carried out a systematic literature search using four primary keywords and a list of secondary keywords (see Table 1).
From the search, we matched the keywords to the title, abstract or keywords on the databases “Scopus”, “Web of Science” and “Google Scholar”. The search was limited to English-written journal papers, however due to the authors’ native language, also reports and grey literature in Danish, Swedish and Norwegian was also accepted. Furthermore, no time boundaries were set, and therefore some literature dates back to the early 1990s. The initial search based on keywords resulted in 893 papers, reports and grey literature (see Figure 3).
Data Filtering Process and Exclusion Criteria
After the initial literature search, we first filtered the literature based on an analysis of the abstracts, and second filtered the literature based on an analysis of the introduction and conclusion. Moreover, we filtered the literature to meet the criteria listed. We performed the filtering process conservatively, meaning that literature where it was uncertain whether it met the following criteria, was retained.
• The study must be specific to the building sector
• The study must focus on embodied environmental impacts in the built environment
• The study must focus on whole buildings, building elements, building components or building materials in wood
• The study must provide a sufficient level of information regarding the type of wood in construction, methodological choices and environmental performance—more specifically embodied GHG emissions
• The study must solely focus on new constructions excluding building renovations and building extensions
After the filtering process, we found 70 papers from the databases “Scopus”, “Web of Science” and “Google Scholar” relevant. In addition to the literature search in the three databases, we added 24 relevant papers that the authors knew beforehand. Afterwards, the collected literature was used for a snowball search, where the authors checked the reference lists of the literature to assure that no relevant sources were left out. The snowball search resulted in another 46 papers and reports.
The literature was then transferred to a reference management software from which we carried out the third filtering process—a full paper/report in-depth analysis. Studies that only focused on the product level, as well as studies that did not provide sufficient information to complete the analysis, were excluded. In this study, “sufficient information” was defined as information on the built area, the reference study period and the total embodied GHG emissions. From the in-depth analysis, 79 papers and reports were found relevant (Salazar and Meil, 2009; Sodagar et al., 2011; Hoxha et al., 2020), (Buchanan and Honey, 1994; Buchanan and Levine, 1999; Börjesson and Gustavsson, 2000; Aye et al., 2012; Dahlstrøm et al., 2012; Brown et al., 2013; Buchanan et al., 2013; Darby et al., 2013; Ajayi et al., 2015; Fouquet et al., 2015; Ding and Forsythe, 2016; Skullestad et al., 2016; Balasbaneh and Bin Marsono, 2017; Amiri Fard et al., 2019; Emami et al., 2019; Svortevik et al., 2020), (Gustavsson et al., 2006; Gustavsson and Sathre, 2006; Gerilla et al., 2007; Frenette et al., 2008; Gustavsson and Joelsson, 2010; Kahhat et al., 2011; Griffin et al., 2013; Fu et al., 2014; Grant et al., 2014; Islam et al., 2015; Liu et al., 2016; Häfliger et al., 2017; Lessard et al., 2018; Kamali et al., 2019; Rønning et al., 2019), (Peuportier, 2001; Monahan and Powell, 2011; Nässén et al., 2012; Van Ooteghem and Xu, 2012; Damvad Analytics, 2016; Larsson et al., 2016; Motuzienė et al., 2016; Peñaloza et al., 2016; Sinha et al., 2016; Milaj et al., 2017; Schiavoni et al., 2017; Moncaster et al., 2018; Sandanayake et al., 2018; Petrovic et al., 2019a; Moschetti et al., 2019; Pierobon et al., 2019; Schneider-Marin et al., 2020), (Wallhagen et al., 2011; Passer et al., 2012; Iddon and Firth, 2013; Ximenes and Grant, 2013; Takano et al., 2014; Hofmeister et al., 2015; Takano et al., 2015; Švajlenka et al., 2017; Švajlenka and Kozlovská, 2017; Švajlenka and Kozlovská, 2018; Tavares et al., 2019; Zeitz et al., 2019), (Winistorfer et al., 2005; Hacker et al., 2008; Carre, 2011; Monteiro and Freire, 2012; Mosteiro-Romero et al., 2014; Stephan and Crawford, 2014; Tonooka et al., 2014; Kurkinen et al., 2015; Laura Tschümperlin et al., 2016; Lawania and Biswas, 2016; Bukoski et al., 2017; Ohta, 2017; Lavagna et al., 2018; Lobaccaro et al., 2018; Petrovic et al., 2019b; Pittau et al., 2019).
Most of the collected papers and reports examine a number of different scenarios. For instance, papers and reports may assess different scenarios that vary in building types, biogenic carbon approaches (i.e. 0/0 approach or −1/+1 approach), LCA approaches (i.e. attributional or consequential) and LCI modelling approaches (i.e. process, hybrid, input-output). The three LCI modelling approaches relates to how the LCI database is composed. Process-based LCI include all relevant inputs and outputs for a process from literature and research and can be costly and time-consuming affair. In contrast, input-output LCI is based on economic models from statistical data and is typically a fast and low-budget way of calculating emissions. Finally, hybrid LCI combines process-based and input-output LCI to get the advantages of the two methods (Suh and Huppes, 2005; Müller and Schebek, 2013). All relevant scenarios in the final sample of 79 papers were included, resulting in a total amount of 226 scenarios (see Figure 3).
Data Extraction and Classification
In the in-depth analysis, meta-data from the studies were extracted systematically in a data extraction table. The data extraction table was established to collect all relevant meta-data regarding building case type, building characteristic, LCA method and embodied GHG emissions (see data extraction table in Supplementary Information). The building cases in the studies were categorized in the following building typologies: “residential—single family”, “residential—multi family”, “office” and “other”. Further, in line with the study aim, the data extraction table was established to focus especially on the LCA approach, the LCI modelling approach and the biogenic carbon approach (see Table 2). In terms of biogenic carbon approach, the studies were categorized both according to the biogenic carbon approach (i.e. including or excluding biogenic carbon) and according to the system boundaries (i.e. “Cradle to Gate with Options”, “Cradle to Grave excl. module D” or “Cradle to Grave incl. module D”) (see Table 2). Finally, as the study mainly aims to investigate methodological approaches in regards to embodied GHG emissions in wooden building LCAs, details on the operational energy was excluded from the data extraction.
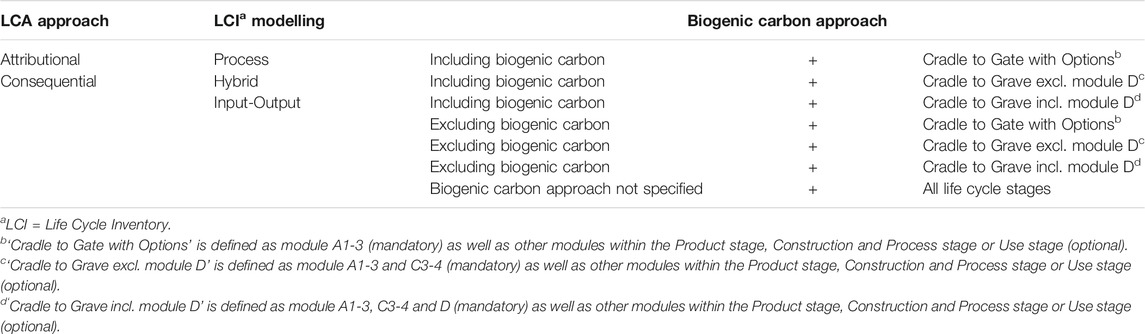
TABLE 2. Categories as defined in data extraction table regarding LCA approaches, LCI modelling approach and biogenic carbon approaches.
Data Normalization
After we extracted all data, we normalized the total embodied GHG emissions of each scenario to kg CO2e per m2 annually based on the built area and the reference study period. However, as the studies assume different reference study periods and are therefore not directly comparable, the total embodied GHG emissions were also normalized to a reference study period of 50 years (Eq. 1). This reference study period is an arbitrary point in time selected to compare across studies rather than representing the actual building service life and atmosphere.
Results and Discussion
Final Sample Meta-analysis
The meta-analysis of the studies in the final sample reveals that the majority of the scenarios are from European countries (61%), whereas only 16% of the scenarios are from Oceania followed by 14% from North America and 8% from Asia. None of the studies are from countries in South America, Africa or other regions (see Table 3).
Further, the meta-analysis showed that only 9% of the scenarios in the final sample consider all life cycle stages including module D. In contrast, most of the scenarios (91%) exclude module D from the assessment. These scenarios either include modules within all other life cycle stages, namely the Product, Construction process, Use and End of Life stage (51%), or only include modules within the Product, Construction process, Use stage (40%).
In terms of biogenic carbon, we find that only 2% of the scenarios report the impacts from biogenic carbon separately, whereas 98% do not specify the biogenic carbon content in details. In the same way, only 12% of the scenarios account for the timing of biogenic carbon throughout the life cycle by assessing the impacts according to the dynamic LCA approach.
As one concern of increasing wood consumption is the impact caused on environmental aspects such as land use and land use change, we analyzed the environmental indicators considered in the scenarios. The meta-analysis reveals that most of the studies include environmental indicators related to GHG emissions and other indicators related to energy demand, acidification, toxicity or ozone depletion, as example. In contrast, only 4% of the scenarios include environmental indicators related to direct or indirect land use and land use change.
Embodied GHG Emissions of Wooden Buildings
The analysis of the studies in the final sample shows that the majority of the scenarios are residential buildings (73%), followed by other types of buildings (19%) and office buildings (8%) (see Figure 4). However, the analysis shows no clear difference in embodied GHG emissions across building types. This result is also supported by the findings in (Zimmermann et al., 2020b; Wiik et al., 2020). The average embodied GHG emissions ranges from 3.9 kgCO2e/m2a50 for the category “Residential—multi family” to 4.0 kgCO2e/m2a50 for the category “Office”, 4.3 kgCO2e/m2a50 for the category “Other” and 4.7 kgCO2e/m2a50 for the category “Residential—single family”.
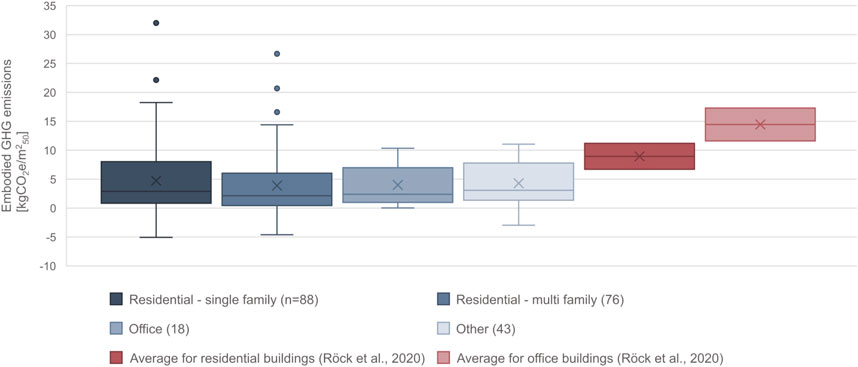
FIGURE 4. Embodied GHG emissions distributed on building types and compared to the results from (Röck et al., 2020). The red boxes represent the average range in embodied GHG emissions for residential and office buildings found by (Röck et al., 2020). The dots in the plot indicate the outliers of the dataset. The boxes and the line within the boxes indicate the quartiles of the dataset (25 percentile, 50 percentile (median) and 75 percentile). The x indicates the average. The error bars outside the boxes indicate the deviation in the data.
Figure 4 compares the embodied GHG emissions of the considered studies to the findings in the review study by Röck et al. (Röck et al., 2020). In brief, the review study by (Röck et al., 2020) seeks to establish the relationship between embodied and operational GHG emissions by investigating the life cycle GHG emissions in a comprehensive sample of residential and office buildings reported in literature. The sample studied by (Röck et al., 2020) distinguishes from the sample considered in this study by not only considering wooden buildings, but considering buildings with all types of materials. The red boxes in Figure 4 present the range in average embodied GHG emissions for residential and office buildings found by (Röck et al., 2020). For residential buildings (Röck et al., 2020), finds that the embodied GHG emissions range from 6.7 kgCO2e/m2a50 to 11.2 kgCO2e/m2a50, whereas for office buildings the embodied GHG emissions range from 11.6 kgCO2e/m2a50 to 17.3 kgCO2e/m2a50 (see Figure 4) (Röck et al., 2020).
The results clearly show that the scenarios investigated in this study provide lower embodied GHG emissions when compared to studies examined by (Röck et al., 2020). Based on the weighted average, we find that the embodied GHG emissions of the residential buildings in this study is almost a factor 0.6 lower than the residential buildings in (Röck et al., 2020). Likewise, Figure 4 shows that the embodied GHG emissions of the office buildings in this study is approximately a factor 0.3 lower than the office buildings in (Röck et al., 2020) based on the weighted average.
Embodied GHG Emissions and LCA Method
The analysis reveals that the included studies in the final sample are rather homogeneous in regard to which LCA methods they apply. As presented in Figure 5, 96% of the scenarios considered in the analysis apply an attributional LCA method. In contrast only 4% of the scenarios apply a consequential approach and these scenarios originate from just two individual studies. On average, the attributional LCAs result in 4.4 kgCO2e/m2a50. This is higher than the average embodied GHG emissions for the consequential scenarios, which is 2.6 kgCO2e/m2a50. However, as the final sample only includes seven consequential scenarios (as presented in Figure 5), no clear tendency can be identified.
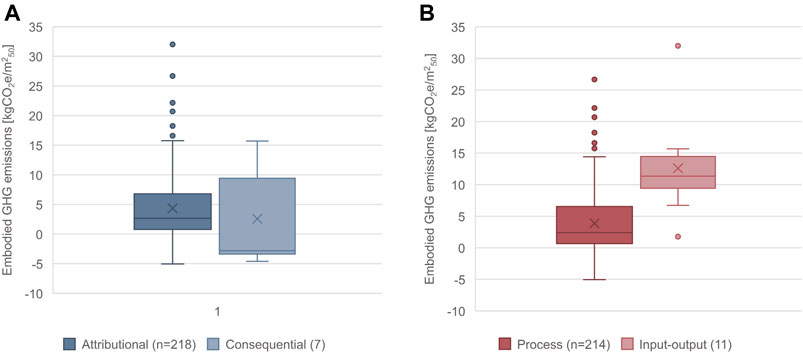
FIGURE 5. Embodied GHG emissions distributed on LCA method (A) and LCI modelling (B). The dots in the plot indicate the outliers of the dataset. The boxes and the line within the boxes indicate the quartiles of the dataset (25 percentile, 50 percentile (median) and 75 percentile). The x indicates the average. The error bars outside the boxes indicate the deviation in the data.
Moreover, Figure 5 shows that most of the scenarios use a process-based life cycle inventory (LCI) modelling approach (95%). Conversely, only 5% of the 226 scenarios use an input-output-based LCI and these originate from just seven individual studies. Also, from Figure 5 it is noticeable that no studies apply a hybrid-based LCI. This homogeneous approach to LCI modelling in wooden building LCAs makes it difficult to identify how the LCI modelling affect the embodied GHG emissions of wooden buildings. However, Figure 5 indicates that scenarios using an input-output-based LCI generally results in higher embodied GHG emissions compared to scenarios using a process-based LCI. The scenarios using a process-based LCI result in 3.9 kgCO2e/m2a50 on average, whereas the scenarios using an input-output-based LCI result in 12.6 kgCO2e/m2a50 on average. Altogether, the results indicate that studies applying an input-output-based LCI provide a factor 3.2 higher embodied GHG emissions compared to studies that apply a process-based LCI. This divergence between process-based and input-output-based results mirrors the fundamental difference in the completeness of scope by the two LCI approaches (Crawford, 2008).
Biogenic Carbon Approaches in LCA
Figure 6 reveals that 74% of the 226 scenarios in the final sample does not specify how biogenic carbon is assessed in the study. Only 26% of the scenarios clearly state if - and how - biogenic carbon is assessed in the building LCA.
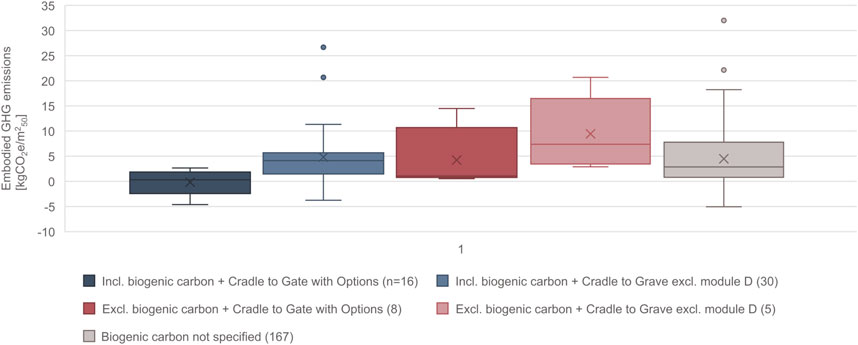
FIGURE 6. Embodied GHG emissions distributed on approach to biogenic carbon counting and system boundaries. The dots in the plot indicate the outliers of the dataset. The boxes and the line within the boxes indicate the quartiles of the dataset (25 percentile, 50 percentile (median) and 75 percentile). The x indicates the average. The error bars outside the boxes indicate the deviation in the data.
The analysis of the studies shows, as presented in Figure 6, that scenarios within the category ‘Incl. biogenic carbon + Cradle to Gate with Options’ result in the lowest embodied GHG emissions when compared to the other approaches analyzed in this study. The average embodied GHG emissions of the scenarios within the category ‘Incl. biogenic carbon + Cradle to Gate with Options’ is −0.2 kgCO2e/m2a50. The negative embodied GHG emissions within this category is due to the studies applying the −1/+1 approach to account for biogenic carbon while at the same time disregarding the End of Life stage from the assessment. Thereby, only the uptake of carbon in the Product stage is included in the assessment, and is not counterbalanced with a release of biogenic carbon at the End of Life as recommended by European standards. Hence, the biogenic carbon is not considered carbon neutral throughout the life cycle, but rather carbon negative.
Since both the −1/+1 and the 0/0 approach assumes that biogenic carbon is counted as carbon neutral throughout the building life cycle, applying the −1/+1 approach or the 0/0 approach in a ‘Cradle to Grave excl. module D’-study should provide the same results. However, as presented on Figure 6, the category ‘Incl. biogenic carbon + Cradle to Grave excl. module D’ has markedly lower embodied GHG emissions on average compared to the category ‘Excl. biogenic carbon + Cradle to Grave excl. module D’. The category ‘Incl. biogenic carbon + Cradle to Grave excl. module D’ results in embodied GHG emissions of an average 4.8 kgCO2e/m2a50, whereas the category ‘Excl. biogenic carbon + Cradle to Grave excl. module D’ results in embodied GHG emissions of 9.5 kgCO2e/m2a50 on average. This difference between the two categories is distinct. Nonetheless, it is noticed that the category ‘Incl. biogenic carbon + Cradle to Grave excl. module D’ do not just includes scenarios applying the −1/+1 approach, but rather covers a variety of different approaches to biogenic carbon counting, including several types of dynamic modelling. Therefore, the average embodied GHG emissions for this category becomes lower than the embodied GHG emissions for the category ‘Excl. biogenic carbon + Cradle to Grave excl. module D’. The category ‘Incl. biogenic carbon + Cradle to Grave excl. module D’ and the different approaches to include biogenic carbon are further analyzed in Including biogenic carbon in LCA.
Additionally, Figure 6 shows that the scenarios within the category “Biogenic carbon not specified” result in embodied GHG emissions of 4.5 kgCO2e/m2a50 on average and that the scenarios included in this category highly vary in terms of embodied GHG emissions. These variations in embodied GHG emissions are most likely a result of different methodological approaches to biogenic carbon accounting in the different studies.
Including Biogenic Carbon in LCA
Looking further into the category ‘Incl. biogenic carbon + Cradle to Grave excl. module D’, the results show a high variation in embodied GHG emissions due to different ways to account for biogenic carbon. In Figure 7, the scenarios within this category is further divided into two categories: scenarios that apply a −1/+1 approach, and scenarios that apply a dynamic approach. The results presented in Figure 7 suggest that dynamic LCA studies typically provide lower embodied GHG emissions when compared to LCA studies that apply the −1/+1 approach to account for biogenic carbon. The average embodied GHG emissions for the dynamic studies are 4.0 kgCO2e/m2a50, whereas the studies applying a −1/+1 approach results in average embodied GHG emissions of 5.9 kgCO2e/m2a50. Also, from Figure 6 it is noticeable that the dynamic LCA studies result in a wide range of both positive and negative embodied GHG emissions. However, analyzing the dynamic LCAs within the category ‘Incl. biogenic carbon + Cradle to Grave excl. module D’ shows that the scenarios originate from just three individual studies. Thus, it is difficult to conclude how the two approaches affect the embodied GHG emissions.
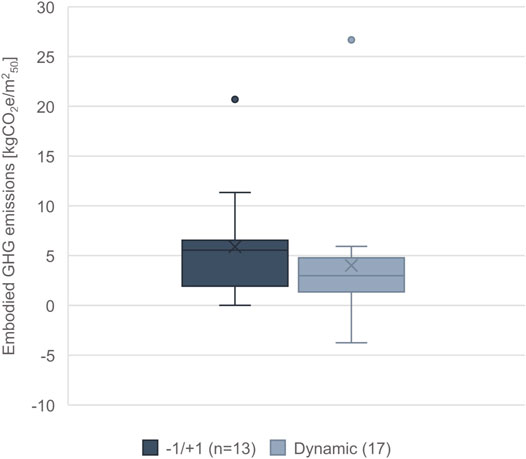
FIGURE 7. Embodied GHG emissions distributed on approach to biogenic carbon counting and system boundaries. The dots in the plot indicate the outliers of the dataset. The boxes and the line within the boxes indicate the quartiles of the dataset (25 percentile, 50 percentile (median) and 75 percentile). The x indicates the average. The error bars outside the boxes indicate the deviation in the data.
Discussion
Wooden Buildings in LCA Studies
One aim of the study was to investigate how existing literature on wooden building LCAs reports embodied GHG emission results. In Embodied GHG Emissions of Wooden Buildings we found that irrespective of building type, the building cases in this study generally present lower values of embodied GHG emissions than the building cases included in (Röck et al., 2020). Both studies, this study as well as the one by (Röck et al., 2020), consider a broad sample of building LCAs. In addition, the studies examine building cases with different building characteristics such as year of construction, region, building type and energy performance. Finally, the studies also include case studies that apply various methodological approaches. Altogether, this study and the study by (Röck et al., 2020) both include a broad sample of data with similar variations in building characteristics as well as methodological approaches. Therefore, it is argued that the lower average embodied GHG emissions in this study when compared to (Röck et al., 2020) can be ascribed to the extensive use of wood in the building cases included in this study. The findings are supported by (Hart et al., 2021) which found that from a sample of 127 configurations, timber frames has a 43% reduced GHG impact potential when compared to concrete and steel frames (Hart et al., 2021). Likewise (Hafner and Schäfer, 2017), suggest that timber construction has a reduced GHG impact potential of 9–56% when compared to mineral alternatives (Hafner and Schäfer, 2017). A study for the UK’s Committee on Climate Change found that substituting masonry with timber frames pose an approximately 20% reduction in embodied GHG emissions (Spear et al., 2019). These studies highlight that the choice of materials in buildings matter and has the potential to reduce the embodied GHG emissions of a building considerably. Again, this is supported by a study by (Zimmermann et al., 2020b) that shows that the embodied GHG emissions per m2 vary by a factor 1.8–2.6 when assessing 60 different building cases with the same scope and LCA method (Zimmermann et al., 2020b).
Variability in Building LCA Methods
The analysis of the existing literature reveals a lack of variability in LCA studies of wooden building types. From the meta-analysis in Final Sample Meta-Analysis, we found that the sample primarily include case studies of residential buildings, followed by building cases within the category ‘Other’ and ‘Office’. Even though the meta-analysis reveals a research gap, where mostly residential buildings are examined in existing literature, the sample reflects the actual distribution quite precisely between residential buildings and non-residential buildings in Europe as well in the U.S. The European distribution between residential buildings and non-residential buildings is 74 and 26% respectively (EU Buildings Datamapper, 2020) while the U.S. distribution in 2015 was 72 and 28% for residential and commercial buildings respectively (U.S. Energy Information Administration, 2015; U.S. Energy Information Administration, 2018). In comparison, the sample in this study distributes as 73% residential buildings and 27% non-residential buildings (including both categories: “Office” and “Other”). Moreover, the FOREST EUROPE report from 2015 states that the majority of the wooden buildings constructed in Europe are residential buildings (FOREST EUROPE, 2015). Especially in Northern Europe, between 85 and 95% of the single family houses are wooden buildings (more specifically in Norway, Sweden and Finland) (FOREST EUROPE, 2015). This is also supported by the 2018 IEA Global Status Report that reports a markedly larger use of wood as structural material in residential buildings compared to non-residential buildings (International Energy Agency and Global Alliance for Buildings and Construction, 2018).
Further, the study presents a clear absence of variability in the methodological approaches applied in wooden building LCAs. As presented in Embodied GHG Emissions of Wooden Buildings, the majority of the studies (83%) apply a static and attributional LCA approach using a process-based LCI, whereas few studies (17%) apply methods such as consequential or dynamic LCA. Altogether, the literature assessed in this study takes a quite narrow approach to LCA methods, thus outlining a significant gap in knowledge. Therefore, it is difficult to establish the relationship between methodological approaches and the embodied GHG emissions. The narrow approach to wooden building LCA case studies should be the focus of future research. Ultimately, building LCA case studies should investigate consequential approaches to gain an understanding of the market dynamics and supply scenarios when increasing the use of wood in buildings, as well as investigating the dynamic approaches to understand the details of the timing in biogenic carbon accounting. Additionally, future studies should focus on examining different types of inventory data in-depth to identify pros and cons of using the types of inventory data in relation to wooden building LCAs.
Increasing Awareness on Biogenic Carbon in Building LCAs
The approach to biogenic carbon accounting in LCA can affect the total embodied GHG emissions of a building. In Biogenic Carbon Approaches in LCA, we found that the scenarios within the category ‘Incl. biogenic carbon + Cradle to Gate with Options’ has lower embodied GHG emissions compared to the other categories. From the further analysis, we found that 16 out of 226 scenarios apply the −1/+1 approach to account for biogenic carbon while assessing Cradle to Gate with Options. By doing so, these studies do not assume carbon neutrality throughout the life cycle despite it being a fundamental premise of the −1/+1 approach. Instead, these studies include uptake of carbon in the product stage while do not include the release of carbon at the end of life. This way of accounting biogenic carbon disregards mass balances within the system, skews the embodied life cycle emissions and typically results in unrealistic low embodied GHG emissions.
Further, by looking into the different approaches to include biogenic carbon we found that the dynamic LCAs vary greatly in terms of the embodied GHG emissions. A thorough analysis of these results reveals that the variation in embodied GHG emissions is because the studies apply different dynamic approaches to account for biogenic carbon. This is supported by a study by (Resch et al., 2021) that finds that methodological uncertainties in dynamic LCAs affect the embodied emissions heavily (Resch et al., 2021). In this study, some of the scenarios consider either biogenic carbon uptake before harvesting or after harvesting (Peñaloza et al., 2016). Biogenic carbon uptake before harvesting is the business-as-usual in building LCAs, where the trees are assumed to sequester carbon before harvesting and production processes. Conversely, biogenic carbon uptake after harvesting assumes that the equivalent amount of trees start to regrow after harvesting and production processes (Hoxha et al., 2020). Another dynamic LCA included in this study applies GWPbio factors developed by Guest, Cherubini and Strømman to account for the timing of biogenic carbon (Guest et al., 2013; Pomponi and Moncaster, 2018). The GWPbio factors are characterization factors that account for the rotation period in the forests as well as the storage period (Guest et al., 2013). The many different approaches to dynamic LCA underline the need for investigating the dynamics of biogenic carbon accounting in-depth and include biogenic carbon in the most suitable way. In addition, the many different methodological approaches point to the need of developing standardized methods to assess biogenic carbon in dynamic as well as static building LCAs.
Also, as presented in Figure 6, we found that the majority of the studies do not specify how they include biogenic carbon in the assessment. As the results within the “not specified”-category vary from negative to quite high embodied GHG emissions, it indicates that this category is a mix of studies that include biogenic carbon and studies that exclude biogenic carbon from the assessment. Some studies most likely include biogenic carbon as a part of the inventory data and impact assessment. Likewise, other studies might exclude biogenic carbon completely from the assessment without clearly stating it. Even though it would be expected that the literature included in this study would be of higher quality as it is primarily academic and peer-reviewed literature, the issues related to biogenic carbon accounting clearly illustrates a lack of transparency and conformity in LCA studies. A study by (Crawford, 2008) also underlines these issues in building LCAs in general and stress the need for immediate action to ensure that embodied emissions will not become a ““second wave” of performance gap in environmental assessments of buildings” (Crawford, 2008).
However, in relation to environmental assessments of wooden buildings, the issues of transparency and awareness on biogenic carbon accounting might also lie in the poor quality of environmental data available in Environmental Product Declarations (EPDs) and in public databases. In previous European Standards concerning environmental assessments of building products only life cycle modules A1-3 should be declared in EPDs and no requirements to separately declare the biogenic carbon content was stated (CEN, 2012). As a consequence, biogenic carbon was usually accounted as carbon neutral and did not gain much attention or there was a risk of skewed embodied emissions if biogenic carbon was not accounted for correctly (as seen in cases within the category ‘Incl. biogenic carbon + Cradle to Gate with Options’). Now, the issues regarding biogenic carbon accounting is gaining attention within the European Standards as well as within the LCA community. The new version of the European Standard is developed to address some of the issues regarding biogenic carbon accounting and to increase the transparency on reporting biogenic carbon impacts. According to the new European standard EN15804:2012 + A2:2019 new EPDs should declare the life cycle modules A1-3, C1-4 and D as well as report the fossil and biogenic GHG emission results separately in the categories GWPbiogenic and GWPfossil (CEN, 2019). This new approach to assessing and reporting GHG emissions for building materials increases the transparency regarding biogenic carbon accounting and helps standardizing the environmental data on a product level.
Limitations of Sample
A limitation of this study is that the synthesis did not account for differences in the inventory scope of the case studies. In the data extraction table, studies were categorized according to which building parts they included following the categories: “Structure only”, “Structure, shell and cladding”, “Whole building”, “Other” and “Not specified”. However, the results in Results and Discussion were not analyzed by category and consequently, the embodied GHG emission results are a mix of all categories. Even though this is a limitation of the study, this is not considered a limitation that alters the conclusions of the analysis as there is no clear relationship between the building parts that are included in the studies and the methods that are applied.
Similarly, we categorized the studies in regards to system boundaries (see Data Filtering Process and Exclusion Criteria). While excluding all emissions from operational energy and water use from the analysis (life cycle stage B6 and B7), we included all other life cycle stages in the synthesis of the results. As a consequence, the studies do not include the same life cycle stages and this affects the total embodied GHG emissions. Even though this is dealt with by categorizing the studies in the three categories “Cradle to Gate with Options”, “Cradle to Grave excl. module D” and “Cradle to Grave incl. module D”, there is still a risk that studies, which include many life cycle stages, result in high embodied GHG emissions. On the contrary, the analysis shows no clear coherence between system boundary and methods applied and therefore it is not considered as a limitation that affect the conclusions.
A final limitation of the study is that we did not account for differences in the defined built area in the investigated studies. For studies that do report a built area there are several ways of defining the area depending on the country’s definition. For instance, the built area can be defined as the gross floor area, net floor area, heated floor area and internal floor area. Currently, there is no standardized way of calculating the different types floor areas across countries (Dario Trabucco and William Douglas Miranda, 2019; Miranda and Trabucco, 2019) and therefore we did not account for the differences in floor area in the assessment. However, this can potentially have a significant impact on the results. For instance, Richard Jackson found a difference of 24% in the measured floor area in Dubai due to the different measurements models applied because no standardized measurements model existed (Jackson, 2016). As comparing studies across countries gains importance not only in regards sustainability targets, but also in regards to energy consumption targets, construction cost, occupancy ratio etc., it is crucial that the denominators such as the floor area are comparable and reported transparently.
Conclusion
This study investigated 79 papers of wooden building LCAs in a Systematic Literature Review. The 79 papers feature a total of 226 different scenarios varying in types of building cases and applied LCA methods. All scenarios were analyzed in-depth and data from each paper were extracted in a data extraction table. From the analysis we found that the wooden building LCA studies generally result in a factor 0.3–0.6 of the embodied GHG emissions found in a review study of a mixed sample of building cases. Additionally, the analysis reveals that existing LCA studies apply a rather narrow approach to LCA and lacks variability in the methods applied. In fact, 95% of the scenarios considered apply an attributional LCA method and use a process-based LCI modelling approach. Thus, it is difficult to establish a clear relationship between the applied methodological approach in LCA and the embodied GHG emissions of wooden buildings. Moreover, the analysis shows that only 26% of the studies clearly specify if—and how - they assess biogenic carbon. Again, this underlines the lack of variability as well as transparency on biogenic carbon accounting in wooden building LCAs. For future research, it is recommended that the relationship between different methodological approaches and the embodied emissions of wooden buildings are investigated to eliminate uncertainties within the industry regarding the environmental potential of wooden buildings. Further, the focus on biogenic carbon accounting in building LCAs should be increased to enhance the comparability between wooden building LCAs and to limit concerns regarding the “true” environmental potential of wooden buildings.
Author Contributions
CA: conceptualization, methodology, data curation, visualization, writing—original draft FR: conceptualization, data curation, supervision, writing—reviewing and editing HG: supervision, writing—reviewing and editing BH: conceptualization, supervision, writing—reviewing and editing.
Funding
This work was supported financially by the Villum Foundation (Grant numbers 00029297).
Conflict of Interest
The authors declare that the research was conducted in the absence of any commercial or financial relationships that could be construed as a potential conflict of interest.
Publisher’s Note
All claims expressed in this article are solely those of the authors and do not necessarily represent those of their affiliated organizations, or those of the publisher, the editors and the reviewers. Any product that may be evaluated in this article, or claim that may be made by its manufacturer, is not guaranteed or endorsed by the publisher.
Acknowledgments
The authors would like to thank the Villum Foundation for funding this research. Also, the authors wish to thank our colleagues Liv Kristensen Stranddorf and Buket Tozan for great help and assistance in the data extraction process.
Abbreviations
CO2, carbon dioxide; DLCA, Dynamic Life Cycle Assessment; EPD, Environmental Product Declaration; GHG, Greenhouse Gas emissions; GWP, Global Warming Potential; LCA, Life Cycle Assessment;
References
Ajayi, S. O., Oyedele, L. O., Ceranic, B., Gallanagh, M., and Kadiri, K. O. (2015). Life Cycle Environmental Performance of Material Specification: a BIM-Enhanced Comparative Assessment. Int. J. Sust. Building Tech. Urban Dev. 6, 14–24. doi:10.1080/2093761x.2015.1006708
Amiri Fard, F., Jafarpour, A., and Nasiri, F. (2019). Comparative Assessment of Insulated concrete wall Technologies and wood-frame walls in Residential Buildings: a Multi-Criteria Analysis of Hygrothermal Performance, Cost, and Environmental Footprints. Adv. Building Energ. Res. 15, 466–498. doi:10.1080/17512549.2019.1600583
Andersen, C. E., Kanafani, K., Zimmermann, R. K., Rasmussen, F. N., and Birgisdóttir, H. (2020). Comparison of GHG Emissions from Circular and Conventional Building Components. Build Cities 1, 379. doi:10.5334/bc.55
Aye, L., Ngo, T., Crawford, R. H., Gammampila, R., and Mendis, P. (2012). Life Cycle Greenhouse Gas Emissions and Energy Analysis of Prefabricated Reusable Building Modules. Energy and Buildings 47, 159–168. doi:10.1016/j.enbuild.2011.11.049
Balasbaneh, A. T., and Bin Marsono, A. K. (2017). Strategies for Reducing Greenhouse Gas Emissions from Residential Sector by Proposing New Building Structures in Hot and Humid Climatic Conditions. Building Environ. 124, 357–368. doi:10.1016/j.buildenv.2017.08.025
Bamber, N., Turner, I., Arulnathan, V., Li, Y., Zargar Ershadi, S., Smart, A., et al. (2020). Comparing Sources and Analysis of Uncertainty in Consequential and Attributional Life Cycle Assessment: Review of Current Practice and Recommendations. Int. J. Life Cycle Assess. 25, 168–180. doi:10.1007/s11367-019-01663-1
Börjesson, P., and Gustavsson, L. (2000). Greenhouse Gas Balances in Building Construction: Wood versus concrete from Life-Cycle and forest Land-Use Perspectives. Energy Policy 28, 575–588. doi:10.1016/S0301-4215(00)00049-5
Brandão, M., Levasseur, A., Kirschbaum, M. U. F., Weidema, B. P., Cowie, A. L., Jørgensen, S. V., et al. (2013). Key Issues and Options in Accounting for Carbon Sequestration and Temporary Storage in Life Cycle Assessment and Carbon Footprinting. Int. J. Life Cycle Assess. 18, 230–240. doi:10.1007/s11367-012-0451-6
Brown, N. (2013). “Basic Energy and Global Warming Potential Calculations at an Early Stage in the Development of Residential Properties,” in Sustain. Energy Build. Smart Innov. Syst. Technol., Vol. 22. Editors A. Hakansson, M. Höjer, and R. J. L. Howlett (Berlin, Heidelberg: Springer), 613–622. doi:10.1007/978-3-642-36645-1_57
Buchanan, A., John, S., and Love, S. (2013). Life Cycle Assessment and Carbon Footprint of Multistorey Timber Buildings Compared with Steel and concrete Buildings. New Zeal J. For. 57, 9–18.
Buchanan, A. H., and Honey, B. G. (1994). Energy and Carbon Dioxide Implications of Building Construction. Energy and Buildings 20, 205–217. doi:10.1016/0378-7788(94)90024-8
Buchanan, A. H., and Levine, S. B. (1999). Wood-based Building Materials and Atmospheric Carbon Emissions. Environ. Sci. Pol. 2, 427–437. doi:10.1016/S1462-9011(99)00038-6
Bukoski, J. J., Chaiwiwatworakul, P., and Gheewala, S. H. (2017). The Life Cycle Assessment of an Energy-Positive Peri-Urban Residence in a Tropical Regime. J. Ind. Ecol. 21, 1115–1127. doi:10.1111/jiec.12494
Carcassi, O. B., De Angelis, E., Iannaccone, G., Malighetti, L. E., Masera, G., and Pittau, F. (2020). “Bio-based Materials for the Italian Construction Industry: Buildings as Carbon Sponges,” in Regen. Built Environ. From a Circ. Econ. Perspect. Res. Dev. Editors S. Della Torre, S. Cattaneo, and C. Z. A. Lenzi (Cham: Springer), 237–247. doi:10.1007/978-3-030-33256-3_23
Carre, A. (2011). A Comparative Life Cycle Assessment of Alternative Constructions of a Typical Australian House Design.
Cen, (2019). EN 15804:2012+A2:2019 - Sustainability of Construction Works - Environmental Product Declarations - Core Rules for the Product Category of Construction Products.
Cen, (2012). EN 15978 - Sustainability of Construction Works - Assessment of Environmental Performance of Buildings - Calculation Method. Brussels: CEN – European Committee for Standardization.
Cen, (2014). EN 16449 Wood and wood-based Products - Calculation of the Biogenic Carbon Content of wood and Conversion to Carbon Dioxide.
Churkina, G., Organschi, A., Reyer, C. P. O., Ruff, A., Vinke, K., Liu, Z., et al. (2020). Buildings as a Global Carbon Sink. Nat. Sustain. 3, 269–276. doi:10.1038/s41893-019-0462-4
Crawford, R. H. (2008). Validation of a Hybrid Life-Cycle Inventory Analysis Method. J. Environ. Manage. 88, 496–506. doi:10.1016/j.jenvman.2007.03.024
Curran, M. A., Mann, M., and Norris, G. (2005). The International Workshop on Electricity Data for Life Cycle Inventories. J. Clean. Prod. 13, 853–862. doi:10.1016/J.JCLEPRO.2002.03.001
Dahlstrøm, O., Sørnes, K., Eriksen, S. T., and Hertwich, E. G. (2012). Life Cycle Assessment of a Single-Family Residence Built to Either Conventional- or Passive House Standard. Energy and Buildings 54, 470–479. doi:10.1016/j.enbuild.2012.07.029
Damvad Analytics, (2016). Potentialer Og Barrierer for Brugen Af Træ Og Bæredygtigt Træ I Byggeriet.
Darby, H. J., Elmualim, A. A., and Kelly, F. (2013). A Case Study to Investigate the Life Cycle Carbon Emissions and Carbon Storage Capacity of a Cross Laminated Timber, Multi-Storey Residential Building. Munich: Sustain. Build. Conf.
Dario Trabucco, D., and William Douglas Miranda, W. (2019). Measuring the Floor Area of Buildings: Problems of Consistency and a Solution. Jcea 13, 107–114. doi:10.17265/1934-7359/2019.02.005
de Almeida Biolchini, J. C., Mian, P. G., Natali, A. C. C., Conte, T. U., and Travassos, G. H. (2007). Scientific Research Ontology to Support Systematic Review in Software Engineering. Adv. Eng. Inform. 21, 133–151. doi:10.1016/j.aei.2006.11.006
De Rosa, M., Pizzol, M., and Schmidt, J. (2018). How Methodological Choices Affect LCA Climate Impact Results: the Case of Structural Timber. Int. J. Life Cycle Assess. 23, 147–158. doi:10.1007/s11367-017-1312-0
Densley Tingley, D., and Davison, B. (2012). Developing an LCA Methodology to Account for the Environmental Benefits of Design for Deconstruction. Building Environ. 57, 387–395. doi:10.1016/j.buildenv.2012.06.005
Ding, G., and Forsythe, P. (2016). A Comparative Study of Floor Construction on Sloping Sites: an Analysis of Cumulative Energy Demand and Greenhouse Gas Emissions. Ceb 16, 33–49. doi:10.5130/ajceb.v16i1.4813
Eberhardt, L. C. M., Birgisdóttir, H., and Birkved, M. (2019). Life Cycle Assessment of a Danish Office Building Designed for Disassembly. Building Res. Inf. 47, 666–680. doi:10.1080/09613218.2018.1517458
Emami, N., Heinonen, J., Marteinsson, B., Säynäjoki, A., Junnonen, J.-M., Laine, J., et al. (2019). A Life Cycle Assessment of Two Residential Buildings Using Two Different LCA Database-Software Combinations: Recognizing Uniformities and Inconsistencies. Buildings 9, 20. doi:10.3390/buildings9010020
Eu Buildings Datamapper (2020). Share of Non-residential in Total Building Floor Area. available at: https://ec.europa.eu/energy/eu-buildings-datamapper_en (Accessed November 24, 2020).
European Commission, Joint Research Centre (2012). The International Reference Life Cycle Data System (ILCD) Handbook. doi:10.2788/85727
Fellner, J., Lederer, J., Scharff, C., and Laner, D. (2017). Present Potentials and Limitations of a Circular Economy with Respect to Primary Raw Material Demand. J. Ind. Ecol. 21, 494–496. doi:10.1111/jiec.12582
Fouquet, M., Levasseur, A., Margni, M., Lebert, A., Lasvaux, S., Souyri, B., et al. (2015). Methodological Challenges and Developments in LCA of Low Energy Buildings: Application to Biogenic Carbon and Global Warming Assessment. Building Environ. 90, 51–59. doi:10.1016/j.buildenv.2015.03.022
Frenette, C. D., Derome, D., Beauregard, R., and Salenikovich, A. (2008). Identification of Multiple Criteria for the Evaluation of Light-Frame wood wall Assemblies. J. Building Perform. Simulation 1, 221–236. doi:10.1080/19401490802527661
Fu, F., Luo, H., Zhong, H., and Hill, A. (2014). Development of a Carbon Emission Calculations System for Optimizing Building Plan Based on the LCA Framework. Math. Probl. Eng. 2014, 1–13. doi:10.1155/2014/653849
Gerilla, G. P., Teknomo, K., and Hokao, K. (2007). An Environmental Assessment of wood and Steel Reinforced concrete Housing Construction. Building Environ. 42, 2778–2784. doi:10.1016/j.buildenv.2006.07.021
Ghisellini, P., Ripa, M., and Ulgiati, S. (2018). Exploring Environmental and Economic Costs and Benefits of a Circular Economy Approach to the Construction and Demolition Sector. A Literature Review. J. Clean. Prod. 178, 618–643. doi:10.1016/j.jclepro.2017.11.207
Grant, A., Ries, R., and Kibert, C. (2014). Life Cycle Assessment and Service Life Prediction. J. Ind. Ecol. 18, 187–200. doi:10.1111/jiec.12089
Griffin, C. T., Douville, E., Thompson, B., and Hoffman, M. (2013). A Multi-Performance Comparison of Long-Span Structural Systems. Struct. Archit., 1668–1676. doi:10.1201/b15267-23010.1201/b15267-231
Guest, G., Cherubini, F., and Strømman, A. H. (2013). Global Warming Potential of Carbon Dioxide Emissions from Biomass Stored in the Anthroposphere and Used for Bioenergy at End of Life. J. Ind. Ecol. 17, 20–30. doi:10.1111/j.1530-9290.2012.00507.x
Gustavsson, L., and Joelsson, A. (2010). Life Cycle Primary Energy Analysis of Residential Buildings. Energy and Buildings 42, 210–220. doi:10.1016/j.enbuild.2009.08.017
Gustavsson, L., Pingoud, K., and Sathre, R. (2006). Carbon Dioxide Balance of wood Substitution: Comparing concrete- and wood-framed Buildings. Mitig Adapt Strat Glob. Change 11, 667–691. doi:10.1007/s11027-006-7207-1
Gustavsson, L., and Sathre, R. (2006). Variability in Energy and Carbon Dioxide Balances of wood and concrete Building Materials. Building Environ. 41, 940–951. doi:10.1016/j.buildenv.2005.04.008
Hacker, J. N., De Saulles, T. P., Minson, A. J., and Holmes, M. J. (2008). Embodied and Operational Carbon Dioxide Emissions from Housing: A Case Study on the Effects of thermal Mass and Climate Change. Energy and Buildings 40, 375–384. doi:10.1016/j.enbuild.2007.03.005
Häfliger, I.-F., John, V., Passer, A., Lasvaux, S., Hoxha, E., Saade, M. R. M., et al. (2017). Buildings Environmental Impacts' Sensitivity Related to LCA Modelling Choices of Construction Materials. J. Clean. Prod. 156, 805–816. doi:10.1016/j.jclepro.2017.04.052
Hafner, A., and Schäfer, S. (2017). Comparative LCA Study of Different Timber and mineral Buildings and Calculation Method for Substitution Factors on Building Level. J. Clean. Prod. 167, 630–642. doi:10.1016/J.JCLEPRO.2017.08.203
Hart, J., D'Amico, B., and Pomponi, F. (2021). Whole‐life Embodied Carbon in Multistory Buildings: Steel, concrete and Timber Structures. J. Ind. Ecol. 25, 403–418. doi:10.1111/JIEC.13139
Hauschild, M. Z., Rosenbaum, R. K., and Olsen, S. I. (2018). Life Cycle Assessment: Theory and Practice. Springer International Publishing. doi:10.1007/978-3-319-56475-3
Hellweg, S., Hofstetter, T. B., and Hungerbühler, K. (2003). Discounting and the Environment Should Current Impacts Be Weighted Differently Than Impacts Harming Future Generations?. Int. J. LCA 8, 8–18. doi:10.1007/BF02978744
Higgins, J. P., and Green, S. (2008). Cochrane Handbook for Systematic Reviews of Interventions. Chichester, UK: John Wiley & Sons. doi:10.1002/9780470712184
Hildebrandt, S. (2016). Bæredygtig global udvikling - FN’s 17 verdensmål i et dansk perspektiv. vol. 4. 1st ed. Copenhagen: Jurist- og Økonomforbundets Forlag.
Hofmeister, T. B., Kristjansdottir, T., Time, B., and Wiberg, A. H. (2015). Life Cycle GHG Emissions from a Wooden Load-Bearing Alternative for a ZEB Office Concept. Oslo: SINTEF Academic Press.
Hoxha, E., Passer, A., Saade, M. R. M., Trigaux, D., Shuttleworth, A., Pittau, F., et al. (2020). Biogenic Carbon in Buildings: a Critical Overview of LCA Methods. Build Cities 1, 504–524. doi:10.5334/bc.46
Iddon, C. R., and Firth, S. K. (2013). Embodied and Operational Energy for New-Build Housing: A Case Study of Construction Methods in the UK. Energy and Buildings 67, 479–488. doi:10.1016/j.enbuild.2013.08.041
International Energy Agency, Global Alliance for Buildings and Construction (2018). 2018 Global Status Report towards a Zero-Emission, Efficient and Resilient Buildings and Construction Sector.
International Energy Agency for Global Alliance for Buildings and Construction (2019). 2019 Global Status Report for Buildings and Construction towards a Zero-Emissions, Efficient and Resilient Buildings and Construction Sector.
IPCC (2018). Global Warming of 1.5°C. An IPCC Special Report on the Impacts of Global Warming of 1.5°C above Pre-industrial Levels and Related Global Greenhouse Gas Emission Pathways, in the Context of Strengthening the Global Response to the Threat of Climate Change. Geneva, Switzerland.
Islam, H., Jollands, M., Setunge, S., Haque, N., and Bhuiyan, M. A. (2015). Life Cycle Assessment and Life Cycle Cost Implications for Roofing and Floor Designs in Residential Buildings. Energy and Buildings 104, 250–263. doi:10.1016/j.enbuild.2015.07.017
Jensen, A. V., and Craig, N. (2019). Wood in Construction - 25 Cases of Nordic Good Practice. doi:10.6027/Nord2019-010
Jørgensen, S. V. (2014). Environmental Assessment of Biomass Based Materials: With Special Focus on the Climate Effect of Temporary Carbon Storage. Denmark: Technical University of Denmark.
Kahhat, R., Crittenden, J., Sharif, F., Fonseca, E., Li, K., Sawhney, A., et al. (2011). Environmental Impacts over the Life Cycle of Residential Buildings Using Different Exterior Wall Systems. J. Infrastruct Syst. 15, 52–53. doi:10.1061/(ASCE)1076-0342(2009)15
Kamali, M., Hewage, K., and Sadiq, R. (2019). Conventional versus Modular Construction Methods: A Comparative Cradle-To-Gate LCA for Residential Buildings. Energy and Buildings 204, 109479. doi:10.1016/j.enbuild.2019.109479
Kurkinen, E-L., Norén, J., Peñaloza, D., Al-Ayish, N., and During, O. (2015). Energi Och Klimateffektiva Byggsystem. Göteborg, Sweden: Miljövärdering av olika stomalternativ.
Larsson, M., Erlandsson, M., Malmqvist, T., and Kellner, J. (2016). Byggandets Klimatpåverkan Livscykelberäkning Av Klimatpåverkan För Ett Nyproducerat Flerbostadshus Med Massiv Stomme Av Trä.
Laura Tschümperlin, A., Frischknecht, R., Pfäffli, K., Schultheiss, M., Knecht, K., and Edelmann, A. (2016). Zielwert Gesamtumweltbelastung Gebäude Ergänzungsarbeiten mit Fokus auf den Einfluss der Technisierung auf die Umwelt-belastung von Büro-und Wohnbauten.
Lavagna, M., Baldassarri, C., Campioli, A., Giorgi, S., Dalla Valle, A., Castellani, V., et al. (2018). Benchmarks for Environmental Impact of Housing in Europe: Definition of Archetypes and LCA of the Residential Building Stock. Building Environ. 145, 260–275. doi:10.1016/j.buildenv.2018.09.008
Lawania, K. K., and Biswas, W. K. (2016). Achieving Environmentally Friendly Building Envelope for Western Australia's Housing Sector: A Life Cycle Assessment Approach. Int. J. Sust. Built Environ. 5, 210–224. doi:10.1016/j.ijsbe.2016.04.005
Lessard, Y., Anand, C., Blanchet, P., Frenette, C., and Amor, B. (2018). LEED V4: Where Are We Now? Critical Assessment through the LCA of an Office Building Using a Low Impact Energy Consumption Mix. J. Ind. Ecol. 22, 1105–1116. doi:10.1111/jiec.12647
Levasseur, A., Lesage, P., Margni, M., Deschênes, L., and Samson, R. (2010). Considering Time in LCA: Dynamic LCA and its Application to Global Warming Impact Assessments. Environ. Sci. Technol. 44, 3169–3174. doi:10.1021/es9030003
Liu, Y., Guo, H., Sun, C., and Chang, W.-S. (2016). Assessing Cross Laminated Timber (CLT) as an Alternative Material for Mid-rise Residential Buildings in Cold Regions in China-A Life-Cycle Assessment Approach. Sustainability 8, 1047. doi:10.3390/su8101047
Lobaccaro, G., Wiberg, A. H., Ceci, G., Manni, M., Lolli, N., and Berardi, U. (2018). Parametric Design to Minimize the Embodied GHG Emissions in a ZEB. Energy and Buildings 167, 106–123. doi:10.1016/j.enbuild.2018.02.025
Milaj, K., Sinha, A., Miller, T. H., and Tokarczyk, J. A. (2017). Environmental Utiliy of wood Substitution in Commercial Buildings Using Life-Cycle Analysis. Wood Fiber Sci. 49, 1–21.
Miranda, W., and Trabucco, D. (2019). Buildings Surface Measurements: Creation of an International Standard. CTBUH J. 34, 8.
Monahan, J., and Powell, J. C. (2011). An Embodied Carbon and Energy Analysis of Modern Methods of Construction in Housing: A Case Study Using a Lifecycle Assessment Framework. Energy and Buildings 43, 179–188. doi:10.1016/j.enbuild.2010.09.005
Moncaster, A. M., Pomponi, F., Symons, K. E., and Guthrie, P. M. (2018). Why Method Matters: Temporal, Spatial and Physical Variations in LCA and Their Impact on Choice of Structural System. Energy and Buildings 173, 389–398. doi:10.1016/j.enbuild.2018.05.039
Monteiro, H., and Freire, F. (2012). Life-cycle Assessment of a House with Alternative Exterior walls: Comparison of Three Impact Assessment Methods. Energy and Buildings 47, 572–583. doi:10.1016/j.enbuild.2011.12.032
Moschetti, R., Brattebø, H., and Sparrevik, M. (2019). Exploring the Pathway from Zero-Energy to Zero-Emission Building Solutions: A Case Study of a Norwegian Office Building. Energy and Buildings 188-189, 84–97. doi:10.1016/j.enbuild.2019.01.047
Mosteiro-Romero, M., Krogmann, U., Wallbaum, H., Ostermeyer, Y., Senick, J. S., and Andrews, C. J. (2014). Relative Importance of Electricity Sources and Construction Practices in Residential Buildings: A Swiss-US Comparison of Energy Related Life-Cycle Impacts. Energy and Buildings 68, 620–631. doi:10.1016/j.enbuild.2013.09.046
Motuzienė, V., Rogoža, A., Lapinskienė, V., and Vilutienė, T. (2016). Construction Solutions for Energy Efficient Single-Family House Based on its Life Cycle Multi-Criteria Analysis: A Case Study. J. Clean. Prod. 112, 532–541. doi:10.1016/j.jclepro.2015.08.103
Müller, B., and Schebek, L. (2013). Input-Output-based Life Cycle Inventory. J. Ind. Ecol. 17, 504–516. doi:10.1111/JIEC.12018
Nässén, J., Hedenus, F., Karlsson, S., and Holmberg, J. (2012). Concrete vs. wood in Buildings - an Energy System Approach. Building Environ. 51, 361–369. doi:10.1016/j.buildenv.2011.11.011
Nußholz, J. L. K., Nygaard Rasmussen, F., and Milios, L. (2019). Circular Building Materials: Carbon Saving Potential and the Role of Business Model Innovation and Public Policy. Resour. Conservation Recycling 141, 308–316. doi:10.1016/j.resconrec.2018.10.036
Ohta, I. (2017). Embodied CO2 Evaluation of a Zero Life-Cycle CO2 Home:A Case Study of an Actual Industrialized Home. J. Asian Architecture Building Eng. 16, 231–237. doi:10.3130/jaabe.16.231
Passer, A., Kreiner, H., and Maydl, P. (2012). Assessment of the Environmental Performance of Buildings: A Critical Evaluation of the Influence of Technical Building Equipment on Residential Buildings. Int. J. Life Cycle Assess. 17, 1116–1130. doi:10.1007/s11367-012-0435-6
Peñaloza, D., Erlandsson, M., and Falk, A. (2016). Exploring the Climate Impact Effects of Increased Use of Bio-Based Materials in Buildings. Construction Building Mater. 125, 219–226. doi:10.1016/j.conbuildmat.2016.08.041
Petrovic, B., Myhren, J. A., Zhang, X., Wallhagen, M., and Eriksson, O. (2019). Life Cycle Assessment of a Wooden Single-Family House in Sweden. Appl. Energ. 251, 113253. doi:10.1016/j.apenergy.2019.05.056
Petrovic, B., Myhren, J. A., Zhang, X., Wallhagen, M., and Eriksson, O. (2019). Life Cycle Assessment of Building Materials for a Single-Family House in Sweden. Energ. Proced. 158, 3547–3552. doi:10.1016/j.egypro.2019.01.913
Peuportier, B. L. P. (2001). Life Cycle Assessment Applied to the Comparative Evaluation of Single Family Houses in the French Context. Energy and Buildings 33, 443–450. doi:10.1016/S0378-7788(00)00101-8
Pierobon, F., Huang, M., Simonen, K., and Ganguly, I. (2019). Environmental Benefits of Using Hybrid CLT Structure in Midrise Non-residential Construction: An LCA Based Comparative Case Study in the U.S. Pacific Northwest. J. Building Eng. 26, 100862. doi:10.1016/j.jobe.2019.100862
Pittau, F., Dotelli, G., Arrigoni, A., Habert, G., and Iannaccone, G. (2019). Massive Timber Building vs. Conventional Masonry Building. A Comparative Life Cycle Assessment of an Italian Case Study. IOP Conf. Ser. Earth Environ. Sci. 323, 012016. doi:10.1088/1755-1315/323/1/012016
Pomponi, F., and Moncaster, A. (2017). Circular Economy for the Built Environment: A Research Framework. J. Clean. Prod. 143, 710–718. doi:10.1016/j.jclepro.2016.12.055
Pomponi, F., and Moncaster, A. (2018). Scrutinising Embodied Carbon in Buildings: The Next Performance gap Made Manifest. Renew. Sust. Energ. Rev. 81, 2431–2442. doi:10.1016/j.rser.2017.06.049
Prentice, I. C., Farquhar, G. D., Fasham, M. J. R., Goulden, M. L., Heimann, M., Jaramillo, V. J., et al. (2001). The Carbon Cycle and Atmospheric Carbon Dioxide.
Resch, E., Andresen, I., Cherubini, F., and Brattebø, H. (2021). Estimating Dynamic Climate Change Effects of Material Use in Buildings-Timing, Uncertainty, and Emission Sources. Building Environ. 187, 107399. doi:10.1016/j.buildenv.2020.107399
Röck, M., Saade, M. R. M., Balouktsi, M., Rasmussen, F. N., Birgisdottir, H., Frischknecht, R., et al. (2020). Embodied GHG Emissions of Buildings - the Hidden challenge for Effective Climate Change Mitigation. Appl. Energ. 258, 114107. doi:10.1016/j.apenergy.2019.114107
Rockström, J., Steffen, W. L., Noone, K., Persson, Å., Chapin, F., Lambin, E., et al. (2009). Planetary Boundaries: Exploring the Safe Operating Space for Humanity. Ecol. Soc. 14, 1. doi:10.5751/es-03180-140232
Rønning, A., Prestrud, K., Saxegård, S., Haave, S. S., and Lysberg, M. (2019). Klimagassregnskap Av Tre-Og Betongkonstruksjoner: Kontorbygning - 4, 8 Og 16 Etasjer.
Ryberg, M. W., Owsianiak, M., Richardson, K., and Hauschild, M. Z. (2018). Development of a Life-Cycle Impact Assessment Methodology Linked to the Planetary Boundaries Framework. Ecol. Indicators 88, 250–262. doi:10.1016/j.ecolind.2017.12.065
Salazar, J., and Meil, J. (2009). Prospects for Carbon-Neutral Housing: the Influence of Greater wood Use on the Carbon Footprint of a Single-Family Residence. J. Clean. Prod. 17, 1563–1571. doi:10.1016/j.jclepro.2009.06.006
Sandanayake, M., Lokuge, W., Zhang, G., Setunge, S., and Thushar, Q. (2018). Greenhouse Gas Emissions during Timber and concrete Building Construction -A Scenario Based Comparative Case Study. Sust. Cities Soc. 38, 91–97. doi:10.1016/j.scs.2017.12.017
Schiavoni, S., Sambuco, S., Rotili, A., D’Alessandro, F., and Fantauzzi, F. (2017). A nZEB Housing Structure Derived from End of Life Containers: Energy, Lighting and Life Cycle Assessment. Build. Simul. 10, 165–181. doi:10.1007/s12273-016-0329-9
Schneider-Marin, P., Harter, H., Tkachuk, K., and Lang, W. (2020). Uncertainty Analysis of Embedded Energy and Greenhouse Gas Emissions Using BIM in Early Design Stages. Sustainability 12, 2633. doi:10.3390/su12072633
Sinha, R., Lennartsson, M., and Frostell, B. (2016). Environmental Footprint Assessment of Building Structures: A Comparative Study. Building Environ. 104, 162–171. doi:10.1016/j.buildenv.2016.05.012
Skullestad, J. L., Bohne, R. A., and Lohne, J. (2016). “High-rise Timber Buildings as a Climate Change Mitigation Measure - A Comparative LCA of Structural System Alternatives,” in SBE16 Tallin Helsinki Conf. Build Green Renov. Deep, Vol. 96 (Tallin and Helsinki: Elsevier), 96, 112–123. doi:10.1016/j.egypro.2016.09.112
Sodagar, B., Rai, D., Jones, B., Wihan, J., and Fieldson, R. (2011). The Carbon-Reduction Potential of Straw-Bale Housing. Building Res. Inf. 39, 51–65. doi:10.1080/09613218.2010.528187
Spear, M., Hill, C., Norton, A., and Price, C. (2019). Wood in Construction in the UK: An Analysis of Carbon Abatement Potential.
Steffen, W., Sanderson, A., Tyson, P., Jäger, J., Matson, P., Moore, B., et al. (2004). Global Change and the Earth System - A Planet under Pressure.
Steffen, W., Richardson, K., Rockström, J., Cornell, S. E., Fetzer, I., Bennett, E. M., et al. (2015). Planetary Boundaries: Guiding Human Development on a Changing Planet. Science 347, 1259855. doi:10.1126/science.1259855
Stephan, A., and Crawford, R. H. (2014). A Multi-Scale Life-Cycle Energy and Greenhouse-Gas Emissions Analysis Model for Residential Buildings. Architectural Sci. Rev. 57, 39–48. doi:10.1080/00038628.2013.837814
Stiebert, S., Echeverria, D., Grass, P., and Kitson, L. (2019). Emission Omissions: Carbon Accounting Gaps in the Built Environment.
Suh, S., and Huppes, G. (2005). Methods for Life Cycle Inventory of a Product. J. Clean. Prod. 13, 687–697. doi:10.1016/J.JCLEPRO.2003.04.001
Švajlenka, J., and Kozlovská, M. (2018). Houses Based on wood as an Ecological and Sustainable Housing Alternative-Case Study. Sustainability 10, 1502. doi:10.3390/su10051502
Švajlenka, J., and Kozlovská, M. (2017). Modern Method of Construction Based on wood in the Context of Sustainability. Civil Eng. Environ. Syst. 34, 127–143. doi:10.1080/10286608.2017.1340458
Švajlenka, J., Kozlovská, M., and Spišáková, M. (2017). The Benefits of Modern Method of Construction Based on wood in the Context of Sustainability. Int. J. Environ. Sci. Technol. 14, 1591–1602. doi:10.1007/s13762-017-1282-6
Svortevik, V. J., Engevik, M. B., and Kraniotis, D. (2020). Use of Cross Laminated Timber (CLT) in Industrial Buildings in Nordic Climate - A Case Study. IOP Conf. Ser. Earth Environ. Sci. 410, 012082. doi:10.1088/1755-1315/410/1/012082
Takano, A., Hafner, A., Linkosalmi, L., Ott, S., Hughes, M., and Winter, S. (2015). Life Cycle Assessment of wood Construction According to the Normative Standards. Eur. J. Wood Prod. 73, 299–312. doi:10.1007/s00107-015-0890-4
Takano, A., Winter, S., Hughes, M., and Linkosalmi, L. (2014). Comparison of Life Cycle Assessment Databases: A Case Study on Building Assessment. Building Environ. 79, 20–30. doi:10.1016/j.buildenv.2014.04.025
Tavares, V., Lacerda, N., and Freire, F. (2019). Embodied Energy and Greenhouse Gas Emissions Analysis of a Prefabricated Modular House: The “Moby” Case Study. J. Clean. Prod. 212, 1044–1053. doi:10.1016/j.jclepro.2018.12.028
Tonooka, Y., Takaguchi, H., Yasui, K., and Maeda, T. (2014). Life Cycle Assessment of a Domestic Natural Materials wood House. Energ. Proced. 61, 1634–1637. doi:10.1016/j.egypro.2014.12.313
United Nations (2015). UN Sustainable Development Goals. available at: https://sdgs.un.org/goals (accessed November 30, 2020).
U.S. Energy Information Administration (2018). Commercial Buildings Energy Consumption Survey (CBECS). available at: https://www.eia.gov/consumption/commercial/(Accessed August 3, 2021).
U.S. Energy Information Administration (2015). Residential Energy Consumption Survey (RECS). available at: https://www.eia.gov/consumption/residential/(Accessed August 3, 2021).
Van Ooteghem, K., and Xu, L. (2012). The Life-Cycle Assessment of a Single-Storey Retail Building in Canada. Building Environ. 49, 212–226. doi:10.1016/j.buildenv.2011.09.028
Wallhagen, M., Glaumann, M., and Malmqvist, T. (2011). Basic Building Life Cycle Calculations to Decrease Contribution to Climate Change - Case Study on an Office Building in Sweden. Building Environ. 46, 1863–1871. doi:10.1016/j.buildenv.2011.02.003
Wiik, M., Selvig, E., Fuglseth, M., Lausselet, C., Resch, E., Andresen, I., et al. (2020). GHG Emission Requirements and Benchmark Values for Norwegian Buildings. IOP Conf. Ser. Earth Environ. Sci. 588, 022005. doi:10.1088/1755-1315/588/2/022005
Winistorfer, P., Chen, Z., Lippke, B., and Stevens, N. (2005). Energy Consumption and Greenhouse Gas Emissions Related to the Use, Maintenance, and Disposal of a Residential Structure. Wood Fiber Sci. 37, 128–139.
Wohlin, C. (2014). Guidelines for Snowballing in Systematic Literature Studies and a Replication in Software Engineering. doi:10.1145/2601248.2601268
Ximenes, F. A., and Grant, T. (2013). Quantifying the Greenhouse Benefits of the Use of wood Products in Two Popular House Designs in Sydney, Australia. Int. J. Life Cycle Assess. 18, 891–908. doi:10.1007/s11367-012-0533-5
Zeitz, A., Griffin, C. T., and Dusicka, P. (2019). Comparing the Embodied Carbon and Energy of a Mass Timber Structure System to Typical Steel and concrete Alternatives for Parking Garages. Energy and Buildings 199, 126–133. doi:10.1016/j.enbuild.2019.06.047
Keywords: GHG emissions, wood, biogenic carbon, buildings, LCA, sustainability, case studies
Citation: Andersen CE, Rasmussen FN, Habert G and Birgisdóttir H (2021) Embodied GHG Emissions of Wooden Buildings—Challenges of Biogenic Carbon Accounting in Current LCA Methods. Front. Built Environ. 7:729096. doi: 10.3389/fbuil.2021.729096
Received: 22 June 2021; Accepted: 17 August 2021;
Published: 31 August 2021.
Edited by:
Marcella Saade, Graz University of Technology, AustriaReviewed by:
Graziano Salvalai, Politecnico di Milano, ItalyDimitrios Kraniotis, Oslo Metropolitan University, Norway
Copyright © 2021 Andersen, Rasmussen, Habert and Birgisdóttir. This is an open-access article distributed under the terms of the Creative Commons Attribution License (CC BY). The use, distribution or reproduction in other forums is permitted, provided the original author(s) and the copyright owner(s) are credited and that the original publication in this journal is cited, in accordance with accepted academic practice. No use, distribution or reproduction is permitted which does not comply with these terms.
*Correspondence: C. E. Andersen, caa@build.aau.dk