- 1Department of Civil Engineering, Ozyegin University, Istanbul, Turkey
- 2Faculty of Bioscience Engineering, Ghent University, Ghent, Belgium
Recent research in the field of concrete materials showed that it might be possible to develop a smart cement-based material that is capable of remediating cracks by Microbial-induced calcium carbonate precipitation (MICP). The early remediation of microcracks enables the design of cement-based systems with an elongated service life with a sustainable approach. However, the main challenge of the application is to extend the viability of the cells against the restrictive environment of cement-paste. These cells have to tolerate the highly alkaline conditions of cement paste, survive the mixing process, and remain viable even when access to nutrients is limited. This paper summarizes a novel study undertaken to investigate the self-healing efficiency of Sporosarcina pasteurii (S. pasteurii) cells immobilized on zeolite and sepiolite minerals having the same particle size. This manuscript reports an extensive experimental study to understand the factors influencing the efficiency of immobilization barriers, such as composition and reactivity. To obtain the bio-additive, the bacterial cells were immobilized without nutrients and additional nutrients were only provided during the curing stage after crack initiation. Screening of the healing process was done with ultrasonic pulse velocity (UPV) testing and stereomicroscopy. Further evaluation on performance was done by evaluating the decrease in water absorption capacity. The healing precipitate was characterized through Environmental Scanning Electron Microscope (ESEM) and Fourier-Transform infrared spectroscopy (FTIR). With this approach, the cracks on mortar surface were sealed and the water absorption capacity of the so-called self-healed mortar decreased compared to its counterpart cracked mortar samples. Sepiolite was found to be a more suitable bedding for the microorganisms compared to zeolite, therefore samples containing sepiolite exhibited a higher performance in terms of crack healing. The results showed that while vegetative cell immobilization on locally available materials is a simple and economically feasible approach the healing capacity of bacterial cells can be hindered due to the reactivity of the mineral.
Introduction
Globally, concrete (the cement-based material) is the second most used material after water. While concrete intrinsically uses low embodied energy materials, the large volume of cement production means they account for approximately 8% of global emissions and significantly impact global warming. For each ton of cement produced, ca. 540 kg of CO2 is emitted (IEA et al., 2009). The cement and concrete industry have been transitioning to more sustainable and equitable strategies to reduce CO2 emissions, and also to reach the goals of the Paris Agreement which commits governments to keeping global warming well below 2°C Celsius (2DC). Although the European cement and concrete industry works on the development of advanced technologies for carbon capture storage or utilization (CCS/CCU), there are still several major technical and economic challenges that should be surmounted. These technologies require a massive investment to equip the cement plants to capture the total CO2 emitted (UN Environment et al., 2018). In addition, even though the unit operation cost can be reduced, the cumulative cost will still be high due to the high-volume production. A structural approach could extend the service life of the structures to decrease the demand for cement (UN Environment et al., 2018). Utilization of MICP in cement-based systems can enable immediate crack remediation, and reduce permeability to damaging substances, thereby extending the life span of structures (De Muynck et al., 2010; Zhang et al., 2015; Wang et al., 2016). Even though eliminating cement from structural concrete is not feasible, low-carbon cement-based systems with prolongated service life will decrease the demand for cement production, eventually reducing the economic and technological burden on the cement industry.
MICP is a biochemical process in which microorganisms stimulate the formation of calcium carbonate (CaCO3) (Mann, 2001). With this approach, tensile or flexural cracks as wide as 0.7 mm were remediated and the water permeability of mortar was reduced (Jonkers and Schlangen, 2007; Wiktor and Jonkers, 2011; Wang J.Y. et al., 2012; Wang et al., 2014b). Crack healing through MICP requires a suitable bacterial culture and the nutrients to support metabolic activity and create the proper environment for crack closure. The main challenge of the application is to find a microorganism that can tolerate the highly alkaline conditions of cement paste, can survive the mixing process, and can remain viable with limited access to nutrients (Tiago et al., 2004). A simple approach by means of limiting the labor work in processing is adding the vegetative bacterial cells directly to the mix. Previously, Bundur et al. (2017b) showed that vegetative S. pasteurii cells could survive in mortar up to 11 months when they were added to the mix without any encapsulations. The cells that remained viable in the mortar were found to be effective in remediation of the microstructure when internal microcracks (Liu et al., 2016) and flexural surface cracks in 7-day-old samples (Amiri et al., 2018). However, limited viability and lack of O2 decreased the performance of CaCO3 yield throughout the crack depth. The precipitation was found to be limited only to the crack mouth in microscale cracks (Amiri et al., 2018). However, considering larger surface cracks, the amount of retained viable cells may not be able to precipitate sufficient biogenic CaCO3 to seal the cracks. Thus, it is crucial to develop a simpler and natural protection system to improve the robustness of bacterial cells against the restrictive environment in the cement-based matrix.
Concerns regarding the viability of the cells and limited crack healing led researchers to propose various encapsulation methodologies, particularly for dormant state endospores. The encapsulation methods consist of embedding the endospores in a protective covering, e.g., inorganic lightweight porous aggregates (LWAs) (Wiktor and Jonkers, 2011), a polymeric membrane (Bang et al., 2010; Wang J. et al., 2012), microcapsules (Wang et al., 2014b), hydrogels (Wang et al., 2014a), and natural minerals (Wang J.Y. et al., 2012; Alazhari et al., 2018). Amongst all these approaches, LWAs and hydrogels have shown the most promising developments regarding viability. The methods were promising in terms of healing cracks at various ages of concrete, but to-date most of the studies revealed that cracks could be healed in samples as old as 28 days.
Instead of using synthetic encapsulation covering, a more sustainable approach could be achieved by using natural additives or natural lightweight aggregates. Throughout the literature, studies showed that several alternatives, such as diatomaceous earth (DE), metakaolin, zeolites, and expanded clay, could be suitable for protection of the bacteria based on their effects on compressive strength and setting; in particular DE was found to be effective in the self-healing of cracks (Wang J.Y. et al., 2012; Erşan et al., 2015). Nevertheless, considering the natural resources, the list of these natural protective barriers could be extended. A correct choice of the protection barrier and application methodology are crucial for further development of self-healing concrete. Up-to-date studies focus on immobilization of endospores to minerals having nano-sized pores resulting with a longer crack-healing period (>40 days). Herein, the main challenge is to decrease the duration of crack-healing by immobilizing vegetative cells on minerals which might result in a decrease in healing period by eliminating the time to activate the endospores. This paper contributes to the literature by providing an extensive experimental evaluation for use of sepiolite as an immobilization barrier to vegetative bacterial cells. The study aims to valorize two local minerals, sepiolite and zeolite, in development of self-healing concrete, leading to a breakthrough on how different carrier materials can actually influence the self-healing ability of vegetative Sporosarcina pasteurii cells. An experimental program was established to validate the hypotheses on how (a) the minerals can protect the vegetative cells from the alkaline environment and (b) the incorporation of vegetative cells rather than the endospores will increase the degree rate of crack-remediation.
Materials and Methods
Microorganism Selection and Growth
Leibniz institute- german collection of microorganisms and cell cultures S. pasteurii (DSMZ 33) cells were grown in a Urea-corn steep liqueur (CSL)-sodium acetate nutrient medium (UCSL) medium, which includes tris base (0.13 M), CSL (15 g), sodium acetate (10 g), and urea (20 g) per liter of distilled (DI) water. The pH of the medium was adjusted to 9. S. paste cells were aerobically cultured in a sterilized nutrient medium with shaking conditions (175 rpm) at 30°C. The cells were incubated until they reached their stationary phase (109 CFU/mL). Then, the cells were collected from the culture by centrifuging at 6,300 g for 15 min. The cells were washed twice by PBS (Phosphate Buffer Solution) and stored at 4°C until immobilizing.
Material Selection
The vegetative bacterial cells were immobilized on sepiolite (SEP) and clinoptilolite zeolite (ZEO). The immobilization procedure was simply achieved by submerging the minerals to an aqueous solution, either PBS or UCSL medium, for 24 h. Therefore, a so-called absorption capacity of the minerals was specified as 24-h absorption capacity. This was calculated by measuring the total amount of water absorbed by the mineral after they were submerged in water for 24 h. The 24-h absorption capacity of the sepiolite and zeolite were 80 and 25%, respectively. The particle size of the minerals and cement was determined by a Mastersizer 2000 particle size analyzer with a Hydro MU 2000 (Malvern, Worcestershire, United Kingdom) wet dispersion unit. To prevent hydration of the cement particles, the cement particles were dispersed in ethanol. The average particle sizes of minerals and cement were also summarized in Figure 1.
Mortar samples were prepared using OPC CEM I 42.5R and standard sand according to the norm EN 196-1. A polycarbooxylate ether (PCE) superplasticizer was used to maintain the same workability criterion for all samples. The water to cement ratio (w/c) was kept at 0.45.
Immobilization Procedure
Ass mentioned in section “Material Selection,” immobilization of bacterial cells was achieved by simply submerging the minerals in a bacterial suspension. To immobilize the cells on minerals, vegetative S. pasteurii cells were collected as was stated in section “Microorganism Selection and Growth.” A set of bio-based additive was prepared by immobilizing the bacterial cells without any nutrients (labeled as “B” series). For this set, 2 g of viable S. pasteurii cells were resuspended in a sterilized 50 mL PBS solution and then 22.5 g of minerals were added to the suspension. Immobilization on minerals was achieved with shaking conditions (175 rpm) at 30°C for 24 h. A second set of bio-based additive (labeled as “BN” series) was also prepared, which has nutrients along the immobilized bacterial cells in which 1 g of cells on half of the required minerals was as stated and the other half was saturated with nutrient medium. Impregnated minerals were removed from the incubator and any remaining solution was filtered through MN615 A Grade I filter paper, and the obtained saturated minerals were then partially dried in an oven (at 40°C) until saturated surface dry (SSD) condition was achieved. Excess aqueous suspension content on the minerals was calculated by subtracting the known weight of minerals, bacterial cells, and theoretical absorbed PBS from the final weight of oven dry slurry. This value was subtracted from the mixing water content. At last, the third set was prepared as control (labeled as “C” series) by saturating the minerals to PBS without cells. Minerals were used as an addition to the mix by 5% of cement weight. However, considering the absorption capacity, the bio-based additive including the minerals, cell, and aqueous suspension were 9% for sepiolite- and 6.5% for zeolite-containing samples.
Preparation of Mortar Sample
Mortar samples were prepared by ASTM C305-14 (ASTM International, 2014). The water- to-cement ratio (w/c) and sand-to-cement ratios were kept at 0.45 and 3, respectively. To provide flexural resistance during crack initiation, 12-mm micro synthetic fibers were added to mortar (2 g/m3 of mortar). A polycarboxylate ether (PCE)-based superplasticizer (BASF) was used to maintain the required workability of mortars in terms of flow table diameter based on ASTM C1437-15. PCE was added into mortar mixes until the desired flow was reached (±10% of the control neat mortar). The mortar samples were then cast into 40 x 40 x 160 mm molds and kept in a humid environment at 21°C for 24 h. Then, the samples were removed from molds and further cured in a moist environment until testing (22°C). Table 1 summarizes the composition and flow behavior of different mixes used in this study. Considering the three different curing regimes, nine beams were prepared for each set of mixes. Figure 2 summarizes the operational flow chart for the experimental design.
Crack Formation and Curing Conditions
The cracks were formed after the 28th day of mixing. The samples were removed from the curing environment and cracked under a 3-point flexural loading using a servo hydraulic displacement-controlled device (0.05 mm/sec). Upon unloading, the remaining crack width ranged from 0.30 ± 0.05 mm. A set (three samples) of control samples were not cracked for further analysis (NCR). Once the cracks were formed, a set of cracked samples were cured in water and another set was cured in nutrient medium containing UCSL and Calcium Acetate (UCSL-CA medium). The curing process was done by submerging the samples into curing solutions for 2 days and then leaving them in ambient conditions for 2 days. This 2-day period curing process was applied until at least 80% crack sealing was observed. The curing solution was replaced every week upon completion of two full submersion periods.
Crack remediation was evaluated by periodic ultrasonic pulse velocity (UPV) and stereomicroscopy analyses. To investigate the self-healing in cement-based mortar, cracked beams were periodically observed under NIKON Inc. SMZ745T Stereomicroscope and analyzed with CLEMAX visual analysis system. Air-dried samples were analyzed weekly for crack sealing. Upon examining the images obtained with a stereomicroscope, further analyses were performed to determine the % crack closure in the cracks by a java-based image processing program “ImageJ.” First, the images obtained at 50× magnification were readjusted to pixels / μm. The “Threshold” filter was used to define the crack surfaces more pronounced in the images for crack width measurements (Figure 3). The crack width measurements were taken at 10 different points on each cracked sample after 7, 14, 21, and 28 days of curing (Figure 3B). Special care was given to take the measurements exactly at the points. The % crack closure was calculated by equation (1) as follows:
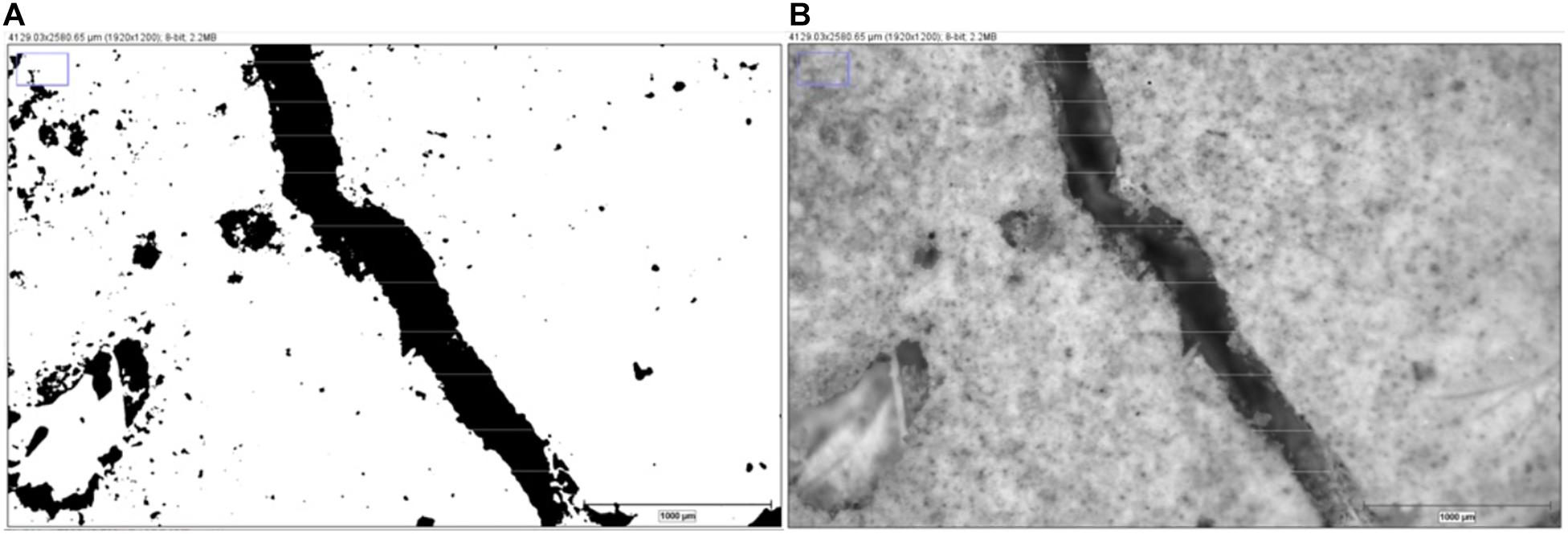
Figure 3. Crack width evaluation for zeolite even without cells (ZEOC) specimens. (A) Threshold filter, (B) measurement points through crack surface.
where wi is the initial crack width measurement right after cracking and w7.,14.,21.,28. is the crack width measurement obtained after 7, 14, 21, and 28 days of curing. The results are presented as % crack closure within the 95% confidence interval.
To evaluate the crack closure performance, a UPV test was also conducted according to ASTM C597-16 Standard Test Method for Pulse Velocity Through Concrete. The test was simply conducted by measuring the transverse time of longitudinal stress waves generated by electro-acoustical transducer. Transverse time is affected by the cracks and voids located inside the concrete. Measurements were taken weekly to evaluate the healing performance until complete closure was seen. Prior to the test, transducers and the sides of the beams were carefully wiped in order to obtain a smooth contact zone. Also, a calibration check was conducted before each test with a calibration rod. The test was conducted while the samples were at an air-dry condition. A viscous Grease was applied to the transducer faces as a coupling agent in order to eliminate air at the contact surfaces. Measurements were taken by simply pressing the transmitting and receiving transducers to the two sides of the beam where they were located opposite to each other. Transducers were connected to the pulse generator and the resonant frequency was adjusted to 20 kHz. Afterwards, pulse velocity was calculated by simply dividing the sample length (0.16 m) to the time recorded (s) from the measurement.
Water Absorption Test
The influence of crack healing was quantified in terms of the change in water absorption. Water absorption capacity of the healed mortar samples was calculated by RILEM 25 PEM II-6 test (Commission 25-PEM Protection et Érosion Des Monuments, 1980). Upon full crack closure, the samples were removed from the curing medium and put in an oven at 40°C until an equilibrium was maintained in the mass change within the range of ±0.1%. When a stationary mass change was achieved, samples were partially covered with silicon to restrain the water penetration. All adjacent sides of the cracked region were coated with silicone. The cracked uncoated surface was then held in a water bath of 10 ± 1 mm height. Dry weight (Wd) of the samples were measured before the submersion. Specimens were periodically removed from the water bath and the wet mass of samples (Ww) were measured at 15 and 30 min and1, 2, 3, 8, 24, 48, and 120 h. During weighing, the samples were carefully wiped with a towel without disturbing the precipitates sealing the crack. After the measurement, specimens were put back into the water immediately. Water absorption coefficient (k) was calculated with the following formula (2):
where k [kg/ (m2.s0.5)] is the water absorption coefficient, t is time, Q (kg) is the absorbed mass of water mass (Ww - Wd), and A (m2) is the submerged surface area for the specimen. Four different sets of samples were evaluated for each set: (1) Urea-CSL-Calcium Acetate nutrient medium curing, (2) Water Curing, (3) Air curing at ambient conditions, and (4) Control samples without any cracks and cured in ambient conditions.
Characterization of the Precipitates
In order to qualitatively characterize the precipitates in the cracked region, samples were collected from the crack surface after cracking the beams into two parts following the water absorption test. The characterization was done by ESEM and FTIR. The final collected samples were further split into pieces to a dimension smaller than 1 cm3 for ESEM and gently ground into powder form for FTIR. Morphology of the specimens were analyzed with a FEI-Philips XL30 ESEM with Field Emission Gun (FEG). The accelerating voltage was kept at 10 kV while the working distance was held at 10 ± 1 mm at various magnifications. The samples were gold-coated prior to the analysis.
The samples were slightly ground to coarse powder form for FTIR analysis. Approximately 5 mg of the collected samples were chemically characterized by using FTIR (Bruker Equinox 55IR Spectrometer, United States) with a resolution of 4 cm–1 within a range of 3,000–600 cm–1. FTIR spectroscopy was used to provide qualitative evidence for CaCO3 presence at the crack interface and evaluate the crystal structure of precipitate.
Assessing the Number of Viable Cell Retention in Mortar
Viability of cells was assessed after the water absorption testing (approximately 60 days after mixing). A part of the samples obtained from the cross section was used to determine the bacterial viability. First, the collected samples were ground with a sterilized pestle and mortar. Then, approximately 20 ± 1 g of the powdered samples were put in the 50 ml sterile centrifuge tubes. Then, the pre-prepared and autoclaved UCSL-Sodium Acetate medium was injected into the tubes. Approximately 25 ± 5 ml nutrient medium was used for every tube. Tubes were then left to the incubator in shaking conditions at 30°C for 30 min hour. Afterwards, tubes were taken out of the incubator and submerged into water in an ultrasonic bath. Sonication was applied for 20 min at 30°C in order to separate the bacteria from the matrix.
After the sonication, supernatant liquid was taken out with a sterile syringe and suspended into a 200 mL of new UCSLS growth medium and incubated in shaking conditions at 30°C for 24 h. Special care was taken not to mix any matrix sediment with the supernatant. Viable plate count method was used to determine the number of viable cells in the supernatant (Herigstad et al., 2001). The counts were done on duplicates of samples from each beam.
Results and Discussion
Visual Crack Closure in “Self-Healing” Cement-Based Systems
This study investigates the so-called self-healing capacity of S. pasteurii cells immobilized on zeolite and sepiolite minerals. The initial evaluation was done during the healing process via stereomicroscopy. For all samples, visual crack evaluation was done on triplicates of samples in each set. The specimens belonging to the same set showed similar crack- sealing trend. Since the visual crack healing was almost the same for each set, herein one representative image of series with samples was shown. Figure 4 shows a representative image for 28-day old control samples containing only saturated minerals without any bacterial cells. While there was not any precipitation observed in SEPC sample, there was a significant amount of precipitation observed in the zeolite even without cells (ZEOC) sample, particularly that cured in water. The difference in % crack closure for both SEPC and ZEOC samples (and all samples) was summarized in Table 2.
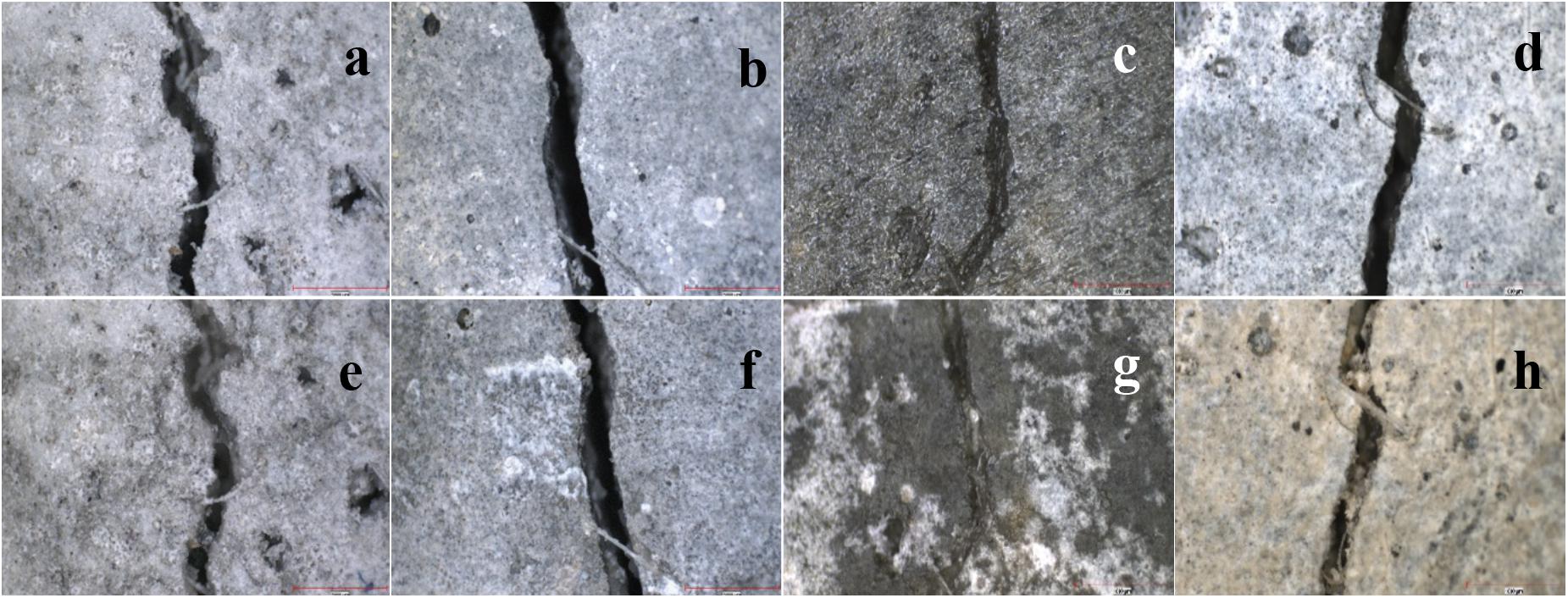
Figure 4. Stereomicroscopy images of the cracks (0.3–0.4 μm) in control specimens. (a,b) SEPC sample right after cracking, (c,d) zeolite even without cells (ZEOC) sample right after cracking, (e) SEPC sample after 28 days of water curing, (f) SEPC sample after 28 days of UCSL- CA medium; (g) ZEOC sample after 28 days of water curing, (h) ZEOC sample after 28 days of UCSL- CA medium.
Incorporation of ZEOC resulted in over 70% crack closure in samples cured with water, while there was not any crack closure yielded in samples containing only sepiolite (SEPC) without bacterial cells. Even though the average particle size of both sepiolite and zeolite were the same, incorporation of zeolite triggered a latent reaction with water curing. The impact of zeolite with water curing was also pronounced as precipitates filling the surface pores. Previously, incorporation of sepiolite was found to effective in terms of improving both fresh and hardened state (Kavas et al., 2004; Chunran and Shicong, 2019). Sepiolite is a well-known rheology modifier and can also improve mechanical properties due to its micro fibrous nature providing interlocking at a microscale. However, zeolites can be classified as one of the most promising types of high-quality natural pozzolans (Seraj et al., 2016). The clinoptilolite zeolite used in this study contained 65–75% SiO2 and 10–12% Al2O3 (data not shown) with a d50 of 60 μm. Mertens et al. (2009) showed that surface area, cation content, and ease of cation exchange in the zeolites were important for short term reactivity (1–3 days). The clinoptilolite zeolites containing high K+ content and ease of K+-exchange could react very fast after 3 days compared to those possessing Ca+-exchange and Na+-exchange. Relatedly, the clinoptilolite zeolite used in this study contains 2.5–3.8% K2O and CaO. Although the exact reaction kinetics is not known, the higher crack closure in ZEOC samples could be attributed to pozzolanic activity. Relatedly, sepiolite is a 2:1 non-reactive clay that cannot trigger pozzolonic activity unless they are calcined or mechanically agitated (Khalifa et al., 2020). Even if they are calcinated their reactivity is still uncertain. Therefore, it could be concluded that zeolite could trigger partial self-healing even without cells. Since the minerals were saturated, the self-healing capacity of zeolite could be attributed to a possible internal curing mechanism. At last, the crack sealing in control ZEOC samples were found to be less efficient when the sample was cured in UCSL-CA medium at pH 9. The higher pH of the nutrient medium might limit the cation exchange in zeolite, reducing the reactivity.
Incorporation of bacterial cells with sepiolite (SEP-B) yielded a significantly higher % crack closure compared to the mixes where bacterial cells were incorporated by zeolites (ZEO-B). Figure 5 shows a representative image of the series for 28-day old samples where the bacterial cells were immobilized on minerals without any nutrients (ZEO-B and SEP-B). A full crack closure (98%) was obtained in SEP-B samples cured in UCSL-CA medium, while there was a slight increase in the amount of crack closure in their counterpart ZEO-B sample cured in nutrient medium. Sepiolite, being a less reactive mineral with a higher porosity, provided a better efficiency in terms of cell immobilization such that there was even 50% crack closure in the SEP-B sample cured in water (see Table 2). This enables a better immobilization for the bacterial cells used in this study (S. pasteurii cells, which are about 1–2 μm size). In fact, throughout the literature it was also known that sepiolite is a suitable bedding environment for various types of microorganisms (Ranalli et al., 1997). Previous studies revealed that it might be possible that sepiolite can actually absorb the bacterial cells in its pores, enabling higher resistance to alkaline conditions (Tezer and Bundur, unpublished). However, most of the pores in zeolites are in a nanoscale range (Bhaskar et al., 2017). In such a case, the bacterial cells were absorbed on the surface of zeolite particles rather than actually being absorbed in these nano-sized pores. Since the bacterial cells were not actually embedded in the mineral, they might be more prone to the negative impacts of an alkaline environment. It might be possible that most of the cells could be dead, thus could only trigger limited precipitation (which will be further discussed in section “Efficiency of Barriers on Extending Cell-Viability”). Bhaskar et al. (2017) immobilized Sporosarcina ureae and S. pasteurii spores on zeolites having an average particle size of 0.8 mm (maximum of 1.4 mm) with their nutrients. While S. pasteurii spores exhibited a better self-healing capacity in terms of reducing the sorptivity and chloride permeability, full crack healing was obtained in 120 days. It should also be noted that the samples were cracked at 7 days and cured in water after cracking (Bhaskar et al., 2017). Early age cracking might also increase the efficiency of spores in terms of crack efficiency compared to cracking samples at 28 days. Herein, immobilizing spores on zeolites can be more advantageous compared to vegetative cells due to their smaller size, but then the duration of self-healing was significantly extended since the incorporation of spores requires a certain time for activation.
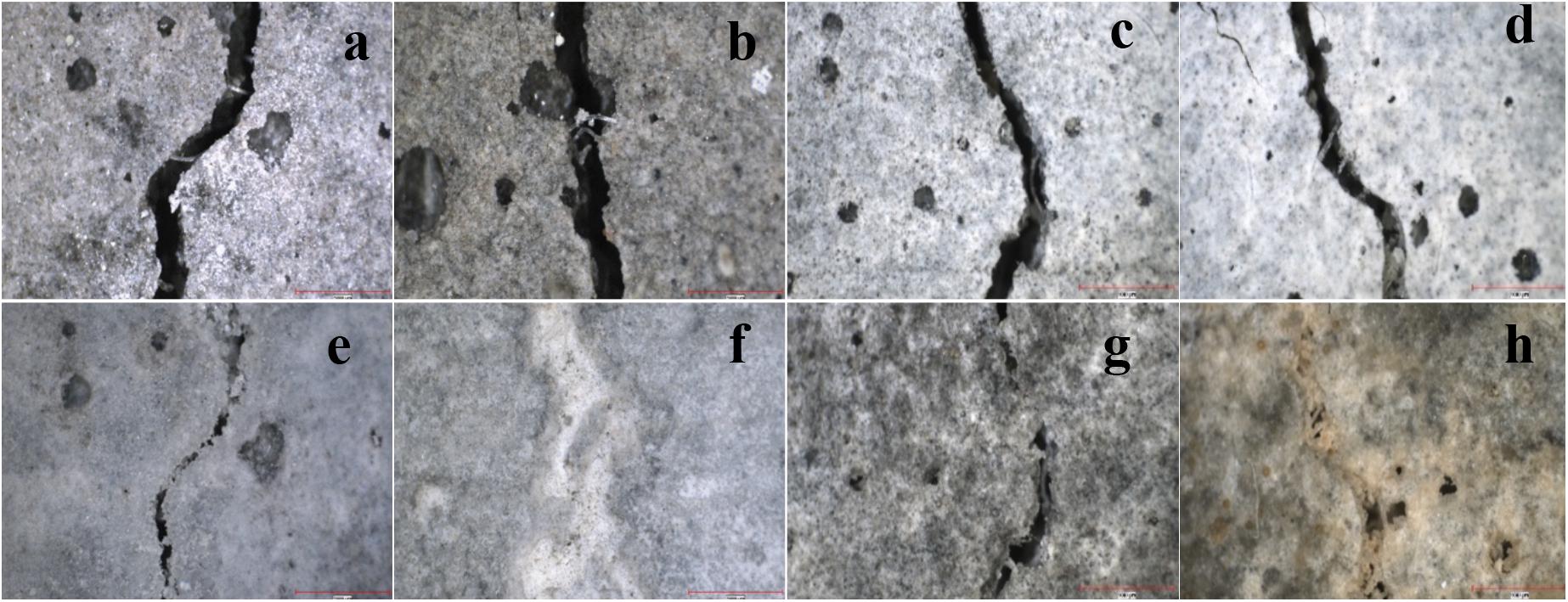
Figure 5. Stereomicroscopy images of the cracks (0.3–0.4 μm) in B series samples. (a,b) SEP-B samples right after cracking, (c,d) containing zeolite, ZEO-B, right after cracking (e) SEP-B samples after 28 days of water curing, (f) SEP-B samples after 28 days of UCSL- CA medium, (g) ZEO-B sample after 28 days of water curing, (h) ZEO-B sample after 28 days of UCSL- CA medium.
At last, a final set of samples were prepared by immobilizing S. pasteurii cells along with their nutrients (SEP-BN and ZEO-BN series). Figure 6 represent images from triplicates of samples for 28-day old SEP-BN and ZEO-BN samples. Similar to B series, bacterial cells immobilized on sepiolite with UCSL-CA medium showed a superior performance in terms of visual crack sealing compared to those immobilized on zeolites. While SEP-BN sample cured in UCSL-CA medium resulted in a 100% crack recovery, there was a 40% decrease in crack closure in the SEP-BN sample cured in water compared to its counterpart SEP-B sample cured in water. This might be due to the different number of bacterial cells used in these two mixes, such that SEP-B contained 2 g per 450 g cement while SEP-BN contained 1 g per 450 g cement. In both of these samples, the cells were stressed not only due to the hardened cement paste but also due to the lack of nutrients. Higher dosage of bacterial cells in SEP-B might increase the viable cell retention at 28 days compared to SEP-BN, resulting in a higher degree of self-healing (see Table 2). Even if there was a difference between SEP-B and SPE-BN, it could be accurate to conclude that sepiolite is a suitable barrier for bacterial cells and can trigger almost complete crack sealing when a continuous flow of nutrients is provided. It should also be mentioned that almost 80% crack sealing was achieved after 21 days of curing, which is a relatively faster crack remediation compared with what was observed in the literature, where mostly endospores were immobilized on minerals (Wang J.Y. et al., 2012; Bhaskar et al., 2017). This was attributed to the use of vegetative cells rather than using endospores, which eliminates the time to reactivate the cells. However, the extent of cell viability and crack healing ability at later ages (beyond 6 months) should be evaluated to establish a fair comparison.
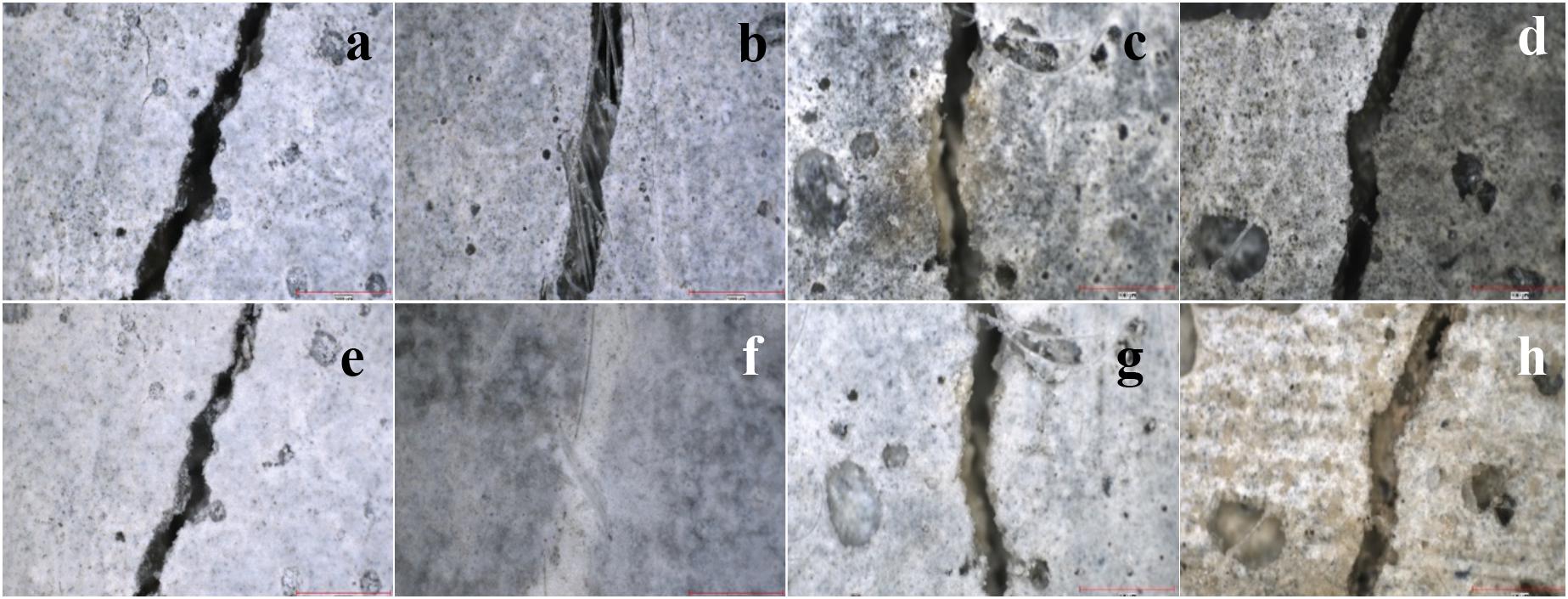
Figure 6. Stereomicroscopy images of the cracks (0.3–0.4 μm) in BN series samples. (a,b) SEP-BN samples right after cracking, (c,d) containing zeolite, ZEO-BN, right after cracking, (e) SEP-BN samples after 28 days of water curing, (f) SEP-BN samples after 28 days of UCSL- CA medium, (g) ZEO-BN sample after 28 days of water curing, (h) ZEO-BN sample after 28 days of UCSL- CA medium.
An opposite trend was observed in zeolite such that the % crack closure was as low as 28% when the nutrients were provided in the curing solution. Thus, the crack closure observed in samples containing zeolite is mostly due to the reactivity of the mineral rather than possible MICP. Considering the fact that UCSL-CA curing reduced the % crack closure in the ZEO-C sample, the relative high pH of the nutrient medium reduces the reactivity of the mineral. The slightly higher crack healing efficiency observed in ZEO-B sample cured in the UCSL-CA sample could again be attributed to the higher dosage of bacterial cells resulting in a higher degree of viable cell retention after 28 days, leading to a limited precipitation once the nutrient medium was provided. Even though zeolites were not a suitable bedding material for the bacterial cells, there is still a high potential of application to actually trigger self-healing with zeolites through an internal curing mechanism.
A UPV test was conducted as a semi-quantitative method to evaluate the crack closure. This test enabled the evaluation of the possible crack closure not only on the crack mouth but rather through the depth of the crack. Figure 7 represents the change in UPV before cracking, right after cracking, and following a 28-day curing period. The change in UPV can be an indicator of crack healing and improved concrete quality. The waves would propagate faster in a denser environment (i.e., mortar ∼3,000 m/s) than they would a moist environment (i.e., water ∼1,500 m/s) (Ramachandran and Beaudoin, 2001; Basaran, 2013). Sound waves propagate through the medium within a defined time period and whenever a change develops, such as cracks and pores, the velocity of the waves is correlated with the variance in the environment. When the relative velocity of a wave changes from time zero in a definite time frame, then this might suggest that the path of the waves have changed. Throughout the literature, it was also agreed that very high velocities (>4,570 m/s) indicated very good concrete quality, while very low velocity ranges (<3,050 m/s) were indicative of poor concrete quality (Ramachandran and Beaudoin, 2001). Thus, the increase in velocity can be an indicator of an improvement in quality of the material (Ramachandran and Beaudoin, 2001). The sudden decrease in UPV upon cracking indicates a reduction in quality and therefore the relative increase in UPV during curing is a sign of improved quality. There was an 8 ± 2% decrease in UPV upon cracking in all samples and the highest UPV change after cracking recorded was in SEPBN-UCSLCA, which was only 4%. Herein, the changes in UPV were not precise enough to drive a conclusion on quality of concrete.
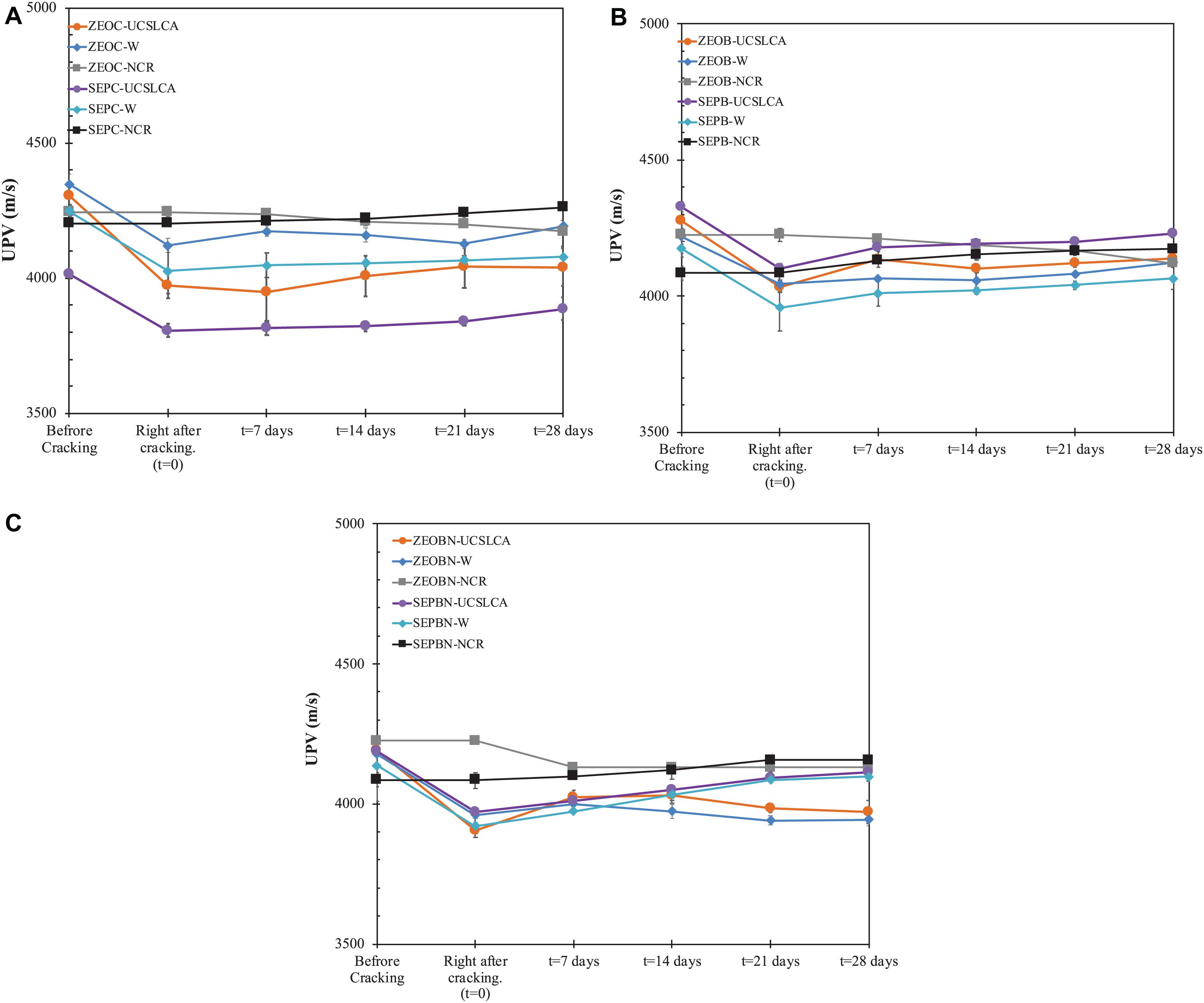
Figure 7. UPV profile obtained from samples after cracking. (A) Control (C) series, (B) B series samples, (C) BN series samples. C Series, Only minerals; B series, Bacterial cells + Minerals; BN series, Bacterial cells + Minerals + Nutrient medium; UCSL-CA, Urea-CSL-Calcium Acetate nutrient medium curing; W, Water Curing; NCR, No cracked samples cured in ambient conditions.
However, there was a correlation between the change in UPV from right after cracking to the end of curing (Figure 7) and % visual crack closure (Table 2). The higher the UPV change, the higher % of crack closure observed. However, this correlation was not that precise such that while the % crack closure in SEPC-UCSLCA sample was only 3.4%, the sample performed the highest UPV change (80 m/s) among the control samples. This might suggest that the change in UPV might be due to of autogenous healing occurring within the crack depth. Even though the samples were dried under ambient conditions for 2 days, there might be a chance that some moisture was present in the crack or pores, which might hinder precise UPV measurements during the curing process.
Influence of Self-Healing on Water Absorption
To determine the water absorption coefficient, the water absorption capacity of healed samples was plotted as a function of time and the slope of this graph shows the water absorption coefficient (k) value. A representative plot is given in Figure 8 for ZEOC control samples. The k value was calculated from slope of Q vs. time plot and summarized in Figure 9.
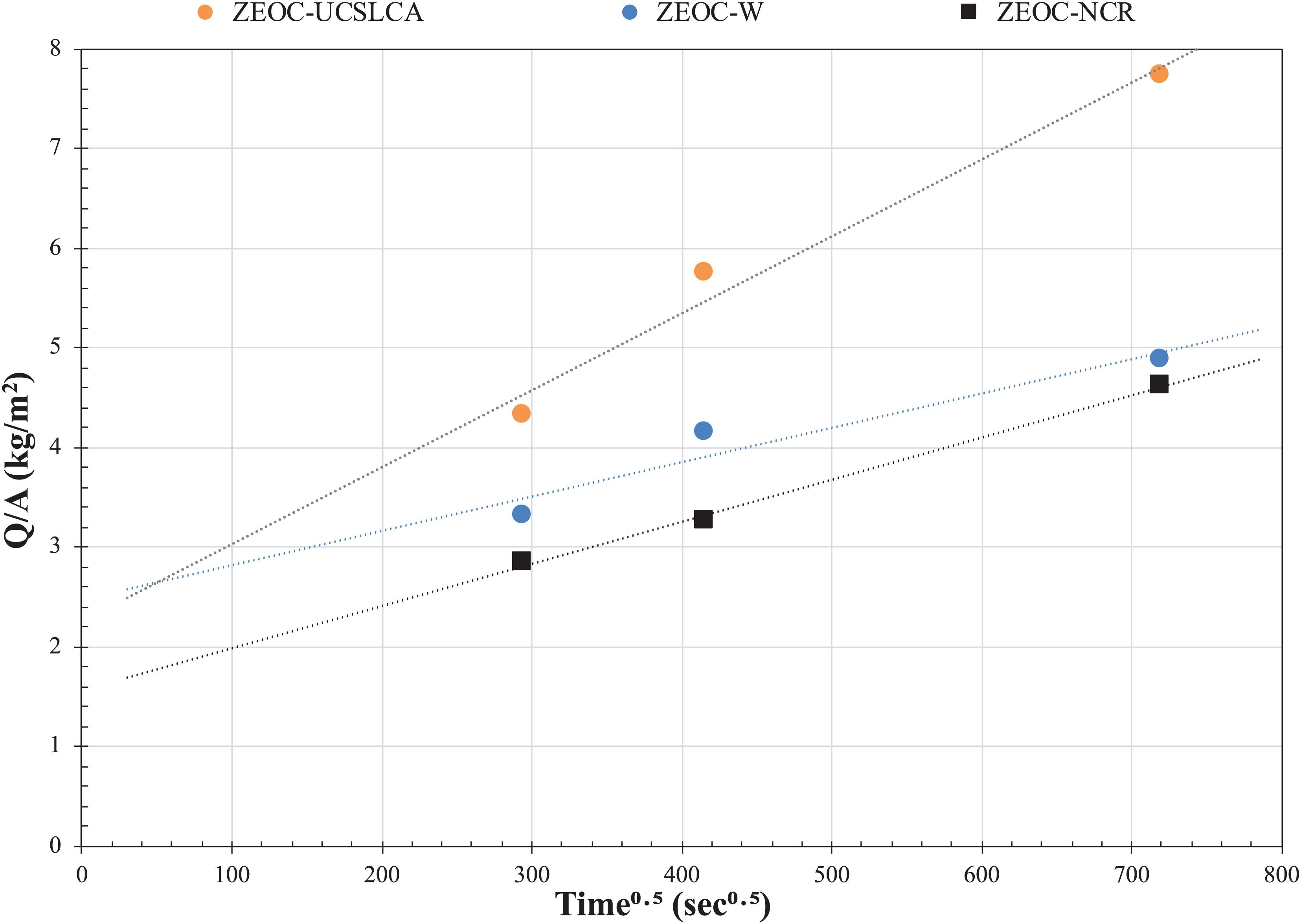
Figure 8. Representative water absorption profile for zeolite even without cells (ZEOC) sample as a function of time. C Series, Only minerals; UCSL-CA, Urea-CSL-Calcium Acetate nutrient medium curing; W, Water Curing; NCR, No cracked samples cured in ambient conditions.
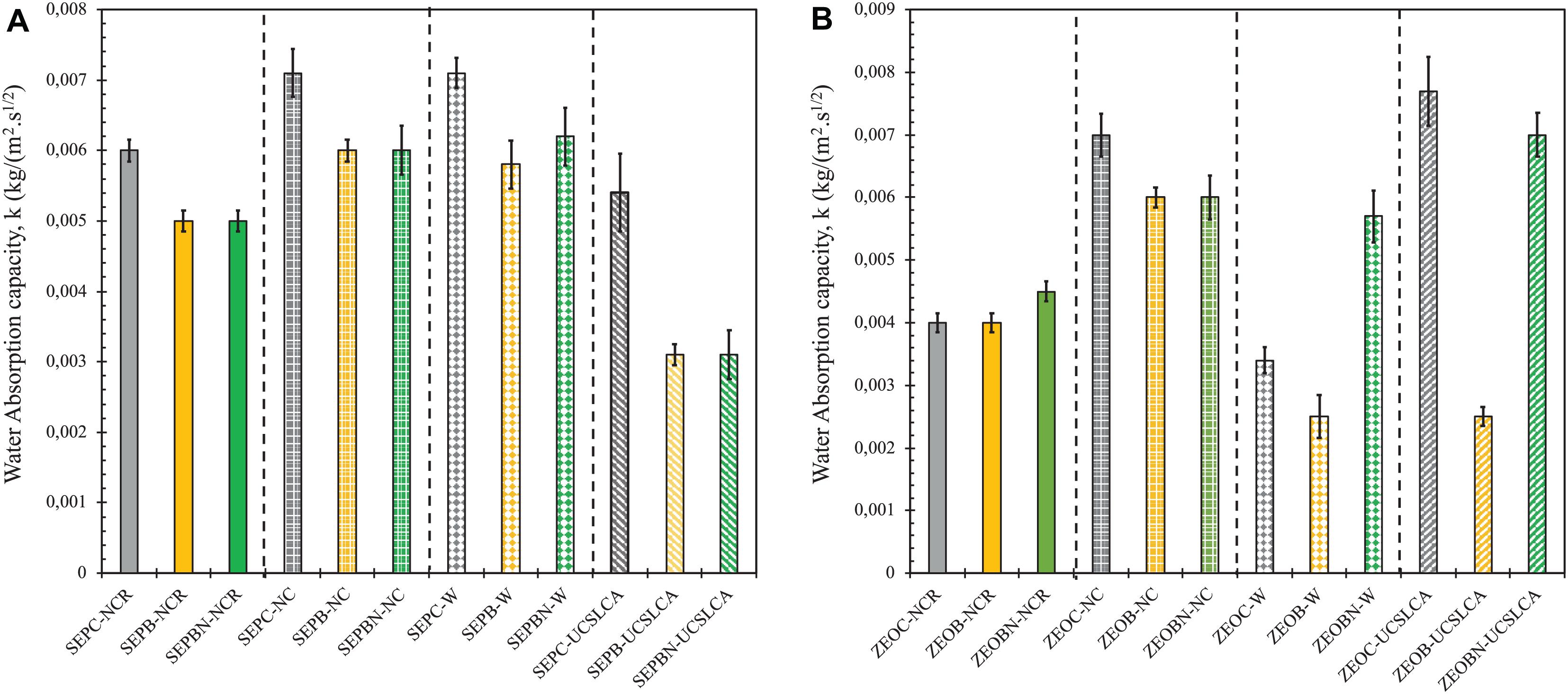
Figure 9. Water absorption capacity of samples after crack remediation. (A) Bacterial cells immobilized on sepiolite, (B) bacterial cells immobilized on zeolite. C Series, Only minerals; B series, Bacterial cells + Minerals; BN series, Bacterial cells + Minerals + Nutrient medium; UCSL-CA, Urea-CSL-Calcium Acetate nutrient medium curing; W, Water Curing; NCR, No cracked samples cured in ambient conditions; NC, Cracked and kept at ambient conditions without curing.
Samples prepared with sepiolite immobilization showed a more consistent behavior in terms of relating the change in k value to visual crack sealing. Such that the cracks were completely sealed in visual analysis in the SEPB and SEPBN sample cured in UCSL-CA medium. There was ∼ 50% reduction in k value both in SEPB and SEPBN samples compared to their negative control SEPB and SEPBN kept at ambient conditions. Relatedly, there was a 45% reduction in k value in SEPB and SEPBN samples compared to the SEPC sample also cured in nutrient medium (Figure 8). This was also in line with the 55 ± 2% increase in UPV in the SEPB and SEPBN samples compared to their negative control SEPC. The partial % crack sealing in SEPB and SEP BN samples cured in water (53.3 and 17.09% in Table 2) led to only a 3% decrease in water absorption compared to their counterpart NC samples. This might indicate that the sealing was only observed in the crack mouth in the SEPB sample cured in water rather through the crack depth. Another possible explanation could be related to the morphology and stability of the biogenic CaCO3 precipitate. MICP could lead precipitation of different CaCO3 polymorphs, such as calcite, vaterite, and dolomite, which have different stabilities at different environmental conditions (Mann, 2001). Previously, our studies showed that incorporation of chemical admixtures could interfere with the morphology of the precipitate. Such that use of air-entraining agents resulted in less stable vaterite formation rather than formation of stable calcite (Amiri et al., 2018). Limited nutrient access in SEPBN samples cured in water might actually trigger vaterite or another crystal rather than calcite, which might also affect its impact on reducing the water absorption. The FTIR analysis revealed that CH and AFt were main phases present in the crack interface in the SEPBN sample cured in water (which will be further discussed in section “Characterization of the Healing Product”), which might lead to visual crack sealing but not be efficient enough to decrease the water absorption.
Relatedly, immobilizing bacterial cells on zeolite was inefficient in terms of reducing the water absorption. A higher degree of efficiency was observed in water-cured ZEOC and ZEOB samples (51 and 58%, respectively), as well as the ZEOB sample cured in nutrient medium. The healing efficiency of ZEOB cured in water is vague since the whole mechanism could be solely due to the presence of zeolites and not the cells. While there was a 58% decrease in the ZEOB-UCSLCA sample compared to ZEOB-NC samples, the relative decrease in ZEOB-UCSLCA sample compared to ZEOC-NC sample was 10%. This might actually be an indication of possible bacterial activity. Further research has to be done to understand the physio-chemical changes in zeolite and through the cracks to understand the healing mechanism. However, it could simply be concluded that a significant decrease in water absorption (>50%) can only be possible if at least 60% of the crack width was sealed.
At last, the healing was more effective when the bacterial cells were immobilized on sepiolite and the specimens were immersed in the medium with urea and calcium acetate (i.e., additional [Ca+2] source). Incorporation of nutrients during casting limits the precipitation because not only can a limited amount of nutrients be provided during mixing, but the cells also cannot access the nutrients in the hardened cement paste. Additional nutrients are required to induce more precipitation that could completely fill the cracks in the specimens containing bacterial cells. This reinforces the argument that the presence of nutrients, particularly urea and an additional calcium source, in a curing medium is an essential factor for promoting bio-based self- healing of cement-based materials.
Characterization of the Healing Product
Upon completion of a water absorption test, the samples were cracked into two pieces and further FTIR analysis was done on precipitates collected from samples. Figure 10 shows the representative FTIR spectra obtained from samples showing substantial crack healing in zeolite-containing samples and Table 3 summarizes the FTIR characterization for all the samples tested in the evaluation. The information collected from the samples obtained from crack surfaces also confirmed that the sealing precipitate is mostly CaCO3 and CSH. Besides calcite, different polymorphs, such as aragonite and vaterite were also found in samples, particularly for samples prepared with zeolite. This indicates that, while the crack sealing observed in samples containing sepiolite solely relies on carbonation, the samples containing zeolite exhibited a mutual reaction mechanism of latent hydration and carbonation. Herein, it is valid to assume that the carbonation is due to the urealytic activity of the inoculated bacterial cells. Based on the data obtained by crack-healing and micro- structural changes in the crack, sepiolite could also be a carrier for the bacterial cells to trigger biomineralization for self-healing. On the other hand, while there was not any crystal obtained in the SEPBN-W sample, there was a partial precipitate formation in the SEPB-W sample which was not a polymorph of CaCO3 (Tezer and Bundur, unpublished). Thus, there is a correlation between the morphology of precpitate and the decrease in water absorption.
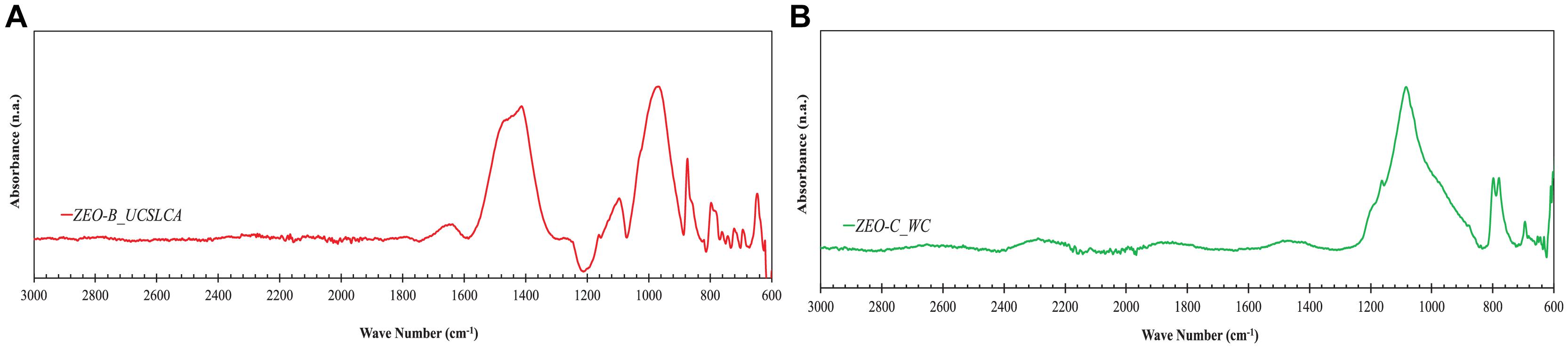
Figure 10. Representative Fourier-Transform infrared spectroscopy (FTIR) spectroscopy analysis of the precipitate collected from crack surfaces (samples showed substantial crack healing). (A) ZEO-B sample cured in water, (B) ZEO-C sample cured in water.
To characterize the biomineral formed inside the crack surface, further evaluation was done by ESEM analysis. After a water absorption test, the samples were broken into two pieces and precipitates were collected from crack surfaces. The analyses were done on samples in which a feasible amount of precipitate was observed. Figure 11 summarizes the ESEM images of the dominant precipitate from the inner crack surface of samples containing bacterial cells after 28 days’ nutrient medium curing. In fact, the findings of the ESEM evaluation were consistent with FTIR analysis such that CaCO3 crystals were found in the form of calcite for precipitates collected from SEP-B, SEP-BN, and ZEO-B samples cured in UCSL-CA medium (see Figures 11c,d,g) and ZEO-B sample cured in water (Figure 11e). In addition, ettringite (AFt) crystals were mostly present in the ZEO-BN sample regardless of curing regime (Figures 11f,h). Even though there were CaCO3 precipitates in the form of calcite, there were few distinct indications of bacterial cells found in samples prepared with bacterial cells immobilized in sepiolite. Previously, our studies revealed that sepiolite might actually create a suitable bedding environment for the bacterial cells due to its relatively larger pores compared to other minerals like diatomaceous earth, which might actually hinder visual indication of cells on the precipitates (Tezer, 2020). The cells might actually be locating in-between an interface between sepiolite minerals and CaCO3 precipitates.
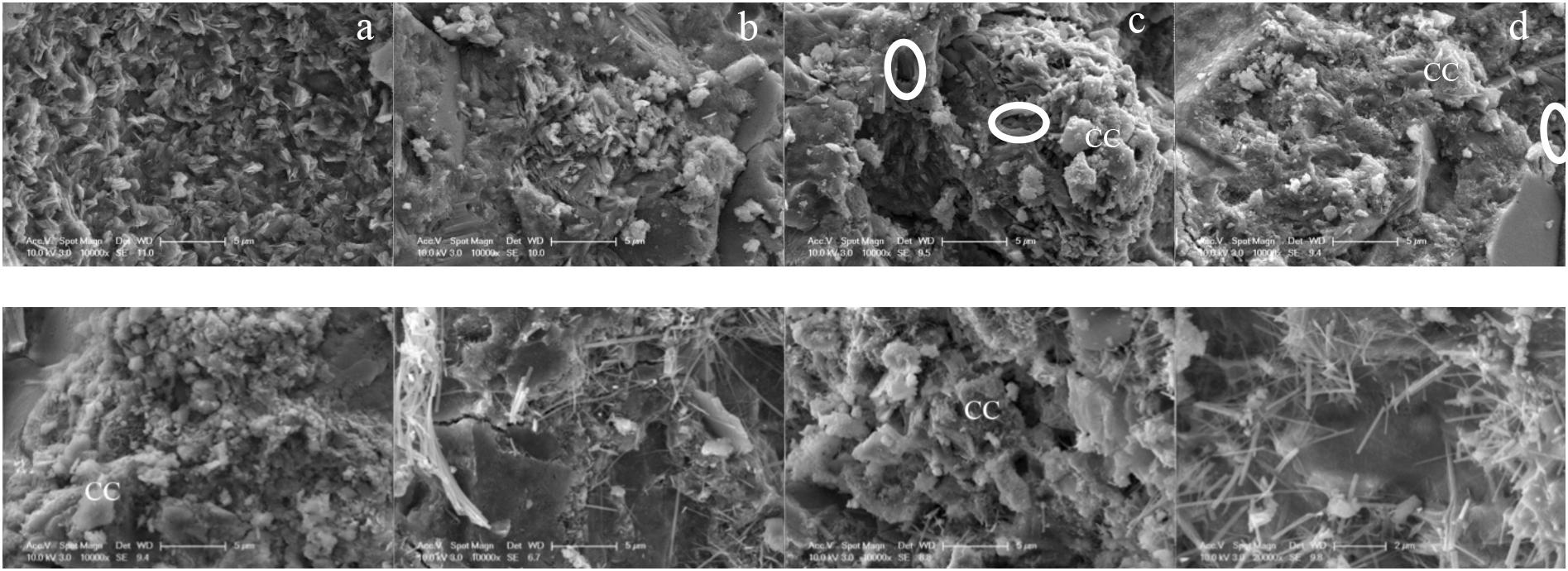
Figure 11. ESEM images of the crack surface obtained from specimens (a) SEP-B sample cured in water (b) SEP-BN sample cured in water (c) SEP-B sample cured in UCSL-CS medium (d) SEP-BN sample cured in UCSL-CS medium (e) ZEO-B sample cured in water (f) ZEO-BN sample cured in water (g) ZEO-B sample cured in UCSL-CS medium (h) ZEO-BN sample cured in UCSL-CS medium. Scale: 5 μm. Circles indicate the indentation of bacterial cells. CC, Calcium Carbonate.
Efficiency of Barriers on Extending Cell-Viability
An important aspect of this study was the evaluation of bacterial cell viability within the cement-based mortar to understand the efficiency of both minerals in protecting the cells from the alkaline environment of concrete. Bacteria count of the specimens were conducted by drop plate method as specified in section “Water Absorption Test.” The viability evaluation was done right after a water absorption test. Results were presented as CFU (cell forming units) per gram of mortar (Table 4). In fact, sepiolite was found to be a more efficient carrier for S. pasteurii cells compared to zeolite in terms of visual crack sealing and reducing the water absorption. The viability evaluation was in line with previous findings such that bacterial cells immobilized on sepiolite showed a significant viable cell retention which could trigger MICP in cracks. It should be mentioned that sepiolite was efficient to maintain a high cell concentration that could induce CaCO3 even after 60 days of mixing. The inefficiency of bacterial cells immobilized on zeolites could be attributed to low cell viability; in fact, the bacterial cells were all killed. Previous studies showed that the lowest S. pasteurii cell concentration should be in the degree of 106 CFU/g of mortar to trigger MICP (Bundur et al., 2017a). Previously, zeolites have been used to induce anti-fungal properties in cement-based systems (Park et al., 2009). Yet, the interaction of bacteria cells with zeolites might be either due to the self-cleaning property of zeolites or the high reactivity of the mineral hindering the cell viability. Further investigations are being done to compare the results with highly reactive metakaolin to understand if the mineral reactivity affects the cell viability.
A similar trend was also observed in two-phase samples, however, the performance of protection barriers was almost the same. Compared to Bac samples the remaining viable cell number was significantly lower, at least by 103 cells per g mortar. This was expected since the quantity of bacteria was slightly lower in two-phase samples compared to Bac samples (see Table 1). This also supports the idea that the presence of the UCSLC medium was crucial for the cells.
It should also be noted that there is not a direct correlation between the initial cell content used in the mix and final cell concentration obtained after crack healing. The initial 2 g of bacterial cells correspond to almost 109 CFU/mL grown in 300 mL nutrient medium for 24 h for B series of mixes (Tezer, 2020). Similarly, for 1 g it is 109 CFU/mL grown in 150 mL nutrient medium for 24 h for BN series samples. This corresponds to a cell concentration of approximately ∼108 CFU/g of fresh mortar for both samples. Even though the initial and final cell concentration cannot be directly correlated, the number of cells was substantially decreased by water curing, whereas the cell concentration was increased in samples cured in nutrient medium. Since the samples were held in ambient conditions before cracking and there is not a suitable environment in hardened cement-based matrix prior to cracking, it is a valid assumption to claim that cell growth was initiated once nutrients were provided upon cracking. This is in line with most of the hypothesis that claimed that endospores enable the cells to be dormant for extended periods and, when exposed to a suitable environment again, this reactivates the bacteria into a metabolic state and initiates growth (Zhang et al., 2015). These results prove that the sepiolite could be used to protect the bacteria from extreme environments. Once the cracks were initiated, nutrient medium curing might initiate growth within the crack surface and trigger MICP. However, water curing alone is not sufficient to trigger growth. This result was aligned with the discussions present in the literature for the minimal concentrations needed to obtain MICP (Bundur et al., 2017a). Thus, it would be correct to conclude that an external source of nutrients in curing solution is not only required to induce MICP but also keep the cells viable.
Finally, this experimental study was done to validate if incorporation of vegetative cells immobilized on minerals could increase the degree and rate of crack-remediation. By the end of the evaluation, it was found that the degree of crack healing was related to the viability of cells such that zeolite, being an anti-bacterial mineral, resulted in a lower viability of cells, and therefore in a lower degree of crack sealing. In contrast, sepiolite, being a more suitable bedding material for the cells, kept the cells viable for an extended time period, resulting in a crack closure of >80% in less than 28 days. In terms of rate of crack healing, immobilization of cells on sepiolite triggered almost full crack closure in 28 days. Previous studies in similar systems showed that crack healing can take around 40–45 days (Wang J.Y. et al., 2012; Amiri et al., 2018). Thus, incorporation of vegetative cells rather than endospores also reduced the duration taken to achieve full crack closure.
Conclusion
This study was undertaken to investigate the possible use of a clinoptilolite zeolite and sepiolite as immobilization barriers for S. pasteurii cells to trigger crack remediation in self-healing cement-based mortar. Self-healing was evaluated in terms of visual crack sealing, change in UPV and water absorption capacity, as well as viable cell retention. Nonetheless, sepiolite was found to be more efficient to immobilize S. pasteurii cells to design so called self-healing cement-based mortar. These results indicated that the addition of bacterial cells immobilized on sepiolite promoted self-healing with a higher efficiency compared to the addition of solely minerals. Cracks with an average width of 0.3 mm were almost completely filled by the precipitate when the samples were subjected to nutrient-medium-curing under wetting and drying cycles. Results showed that cells require additional nutrient sources, such as urea and calcium acetate either in the mix or as curing. In addition, a faster crack remediation compared with what was observed in the literature was shown, where mostly endospores were immobilized on minerals, which was attributed to the use of vegetative cells rather than using endospores, eliminating the time to reactivate the cells. Even though zeolites were not a suitable bedding for the bacterial cells, the mineral itself was found to be efficient to trigger self-healing, which needs to be further evaluated. While there was not a direct correlation with the visual crack healing and the change in UPV, it was concluded that a significant decrease in water absorption can only be achieved if a visual crack sealing of at least 60% was obtained. Sepiolite was also found to be efficient in terms of keeping the bacterial cells viable, such that almost full viable cell retention was observed when the samples were subjected to nutrient medium curing. The healing mechanisms in the presence of nutrients was found to be dependent on the continuous flow of nutrients. Such that refreshing the nutrients continuously upon cracking might initiate growth within the crack surface and trigger MICP sealing the cracks. However, there needs to be an adequate amount of nutrients present in the crack environment to promote self-healing through bacterial cells. Therefore, it is crucial to develop a sound methodology to provide more nutrients internally by eliminating the need for an external curing process. This will enable the development of economically feasible and optimized bacteria-based crack repair toward a more realistic self-healing mechanism.
Data Availability Statement
The original contributions presented in the study are included in the article/supplementary material, further inquiries can be directed to the corresponding author/s.
Author Contributions
IS and MT: conceptualization of the work, experimental analysis, data curation and analysis, methodology, drafting the plots and tables, and writing the original draft. ZB: conceptualization of the work, funding acquisition, project management and administration, methodology, supervision, resources, and writing—review and editing. All authors contributed to the article and approved the submitted version.
Funding
This research was conducted with financial assistance from the Scientific and Technical Research Council (TUBITAK) of Turkey Project: MAG-118M327.
Conflict of Interest
The authors declare that the research was conducted in the absence of any commercial or financial relationships that could be construed as a potential conflict of interest.
References
Alazhari, M., Trupti, S., Andrew, H., Richard, C., and Kevin, P. (2018). Application of expanded perlite encapsulated bacteria and growth media for self-healing concrete. Constr. Build. Mater. 160, 610–619. doi: 10.1016/j.conbuildmat.2017.11.086
Amiri, A., Mahzad, A., and Zeynep, B. B. (2018). Crack remediation in mortar via biomineralization: effects of chemical admixtures on biogenic calcium carbonate. Constr. Build. Mater. 190, 317–325. doi: 10.1016/j.conbuildmat.2018.09.083
ASTM International (2014). ASTM C305-14 Standard Practice for Mechanical Mixing of Hydraulic Cement Pastes And Mortars of Plastic Consistency. West Conshohocken, PA: ASTM International. doi: 10.1520/C0305-14.2
Bang, S. S., Lippert, J. J., Yerra, U., Mulukutla, S., and Ramakrishnan, V. (2010). Microbial calcite, a bio-based smart nanomaterial in concrete remediation. Int. J. Smart Nano Mater. 1, 28–39. doi: 10.1080/19475411003593451
Basaran, Z. (2013). Biomineralization in Cement Based Materials: Inoculation of Vegetative Cells. Ph.D, thesis, University of Texas at Austin, Austin, TX.
Bhaskar, S., Anwar Hossain, K. M., Lachemi, M., Wolfaardt, G., and Otini Kroukamp, M. (2017). Effect of self-healing on strength and durability of zeolite-immobilized bacterial cementitious mortar composites. Cem. Concr. Compos. 82, 23–33. doi: 10.1016/j.cemconcomp.2017.05.013
Bundur, Z. B., Amiri, A., Ersan, Y. C., Boon, N., and De Belie, N. (2017a). Impact of air entraining admixtures on biogenic calcium carbonate precipitation and bacterial viability. Cem. Concr. Res. 98, 44–49. doi: 10.1016/j.cemconres.2017.04.005
Bundur, Z. B., Bae, S., Jo Kirisits, M., and Ferron, R. D. (2017b). Biomineralization in self-healing cement-based materials: investigating the temporal evolution of microbial metabolic state and material porosity. J. Mater. Civil Eng. 29, 1–8. doi: 10.1061/(ASCE)MT.1943-5533.0001838
Chunran, W., and Shicong, K. (2019). Effects of high-calcium sepiolite on the rheological behaviour and mechanical strength of cement pastes and mortars. Constr. Build. Mater. 196, 105–114.
Commission 25-PEM Protection et Érosion Des Monuments (1980). Essais recommandés pour mesurer l’altération des pierres et évaluer l’efficacité des méthodes de traitement [Recommended tests to measure the deterioration of stone and to assess the effectiveness of treatment methods]. Matér. Constr. 13, 175–253. doi: 10.1007/BF02473564
De Muynck, W., De Belie, N., and Verstraete, W. (2010). Microbial carbonate precipitation in construction materials: a review. Ecol. Eng. 36, 118–136. doi: 10.1016/j.ecoleng.2009.02.006
Environment, U. N., Scrivener, K. L., John, V. M., and Gartner, E. M. (2018). Eco-efficient cements: potential economically viable solutions for a low-CO 2 cement-based materials industry ✩. Cem. Concr. Res. 114, 2–26. doi: 10.1016/j.cemconres.2018.03.015
Erşan, Y. Ç, Bravo Da Silva, F., Boon, N., Verstraete, W., and De Belie, N. (2015). Screening of bacteria and concrete compatible protection materials. Constr. Build. Mater. 88, 196–203. doi: 10.1016/j.conbuildmat.2015.04.027
Herigstad, B., Hamilton, M., and Heersink, J. (2001). How to optimize the drop plate method for enumerating bacteria. J. Microbiol. Methods. 44, 121–129. doi: 10.1016/S0167-7012(00)00241-4
IEA, WBCSD, and Cement Technology Road-map (2009). Carbon Emissions Reductions up to 2050. Paris: OECD/IEA; WBCSD. Available online at: http://wbcsdcement.org/pdf/technology/WBCSD-IEA_Cement%20Roadmap.pdf
Jonkers, H. M., and Schlangen, E. (2007). “Crack repair by concrete-immobilized bacteria,” in Proceedings of the First International Conference on Self Healing Materials, 1–7.
Kavas, T., Sabah, E., and Çelik, M. S. (2004). Structural properties of sepiolite-reinforced cement composite. Cem. Concr. Res. 34, 2135–2139.
Khalifa, A. Z., Cizer, Ö, Pontikes, Y., Heath, A., Patureau, P., Bernal, S. A., et al. (2020). Advances in alkali-activation of clay minerals. Cem. Concr. Res. 132:106050. doi: 10.1016/j.cemconres.2020.106050
Liu, S., Bundur, Z. B., Zhu, J., and Ferron, R. D. (2016). Evaluation of self-healing of internal cracks in biomimetic mortar using coda wave interferometry. Cem. Concr. Res. 83, 70–78. doi: 10.1016/j.cemconres.2016.01.006
Mann, S. (2001). Biomineralization: Principles and Concepts in Bioinorganic Materials Chemistry. New York, NY: Oxford.
Mertens, G., Snellings, R., Van Balen, K., Bicer-Simsir, B., Verlooy, P., and Elsen, J. (2009). Pozzolanic reactions of common natural zeolites with lime and parameters affecting their reactivity. Cem. Concr. Res. 39, 233–240. doi: 10.1016/j.cemconres.2008.11.008
Park, S. K., Kim, J. H. J., Nam, J. W., Phan, H. D., and Kim, J. K. (2009). Development of anti-fungal mortar and concrete using zeolite and zeocarbon microcapsules. Cem. Concr. Compos. 31, 447–453. doi: 10.1016/j.cemconcomp.2009.04.012
Ramachandran, V. C., and Beaudoin, J. J. (2001). Handbook of Analytical Techniques in Concrete Science and Technology: Principles, Techniques and Applications, 1st Edn, ed. V. C. Ramachandran (Norwich, NY: Noyes Publications).
Ranalli, G., Chiavarini, M., Guidetti, V., Marsala, F., Matteini, M., Zanardini, E., et al. (1997). The use of microorganisms for the removal of sulphates on artistic stoneworks. Int. Biodeterior. Biodegradation 40, 255–261. doi: 10.1016/S0964-8305(97)00054-1
Seraj, S., Ferron, R. D., and Juenger, M. C. G. (2016). Calcining natural zeolites to improve their effect on cementitious mixture workability. Cem. Concr. Res. 85, 102–110. doi: 10.1016/j.cemconres.2016.04.002
Tezer, M. M. (2020). Development of Two-Phase Biological Self-Healing Agents for Cement-Based Mortar. Ph.D, thesis, Ozyegin University, Istanbul.
Tiago, I., Chung, A. P., and Verissimo, A. (2004). Bacterial diversity in a nonsaline alkaline environment: heterotrophic aerobic populations. Appl. Environ. Microbiol. 70, 7378–7387. doi: 10.1128/AEM.70.12.7378-7387.2004
Wang, J., Ersan, Y. C., Boon, N., and De Belie, N. (2016). Application of microorganisms in concrete: a promising sustainable strategy to improve concrete durability. Appl. Microbiol. Biotechnol. 100, 2993–3007. doi: 10.1007/s00253-016-7370-6
Wang, J., Van Tittelboom, K., De Belie, N., and Verstraete, W. (2012). Use of silica gel or polyurethane immobilized bacteria for self-healing concrete. Constr. Build. Mater. 26, 532–540. doi: 10.1016/j.conbuildmat.2011.06.054
Wang, J. Y., De Belie, N., and Verstraete, W. (2012). Diatomaceous earth as a protective vehicle for bacteria applied for self-healing concrete. J. Ind. Microbiol. Biotechnol. 39, 567–577. doi: 10.1007/s10295-011-1037-1
Wang, J. Y., Snoeck, D., Van Vlierberghe, S., Verstraete, W., and De Belie, N. (2014a). Application of hydrogel encapsulated carbonate precipitating bacteria for approaching a realistic self-healing in concrete. Constr. Build. Mater. 68, 110–119. doi: 10.1016/j.conbuildmat.2014.06.018
Wang, J. Y., Soens, H., Verstraete, W., and De Belie, N. (2014b). Self-healing concrete by use of microencapsulated bacterial spores. Cem. Concr. Res. 56, 139–152. doi: 10.1016/j.cemconres.2013.11.009
Wiktor, V., and Jonkers, H. M. (2011). Quantification of crack-healing in novel bacteria-based self-healing concrete. Cem. Concr. Compos. 33, 763–770. doi: 10.1016/j.cemconcomp.2011.03.012
Keywords: mortar, self-healing, biomineralization, zeolite, sepiolite
Citation: Sandalci I, Tezer MM and Basaran Bundur Z (2021) Immobilization of Bacterial Cells on Natural Minerals for Self-Healing Cement-Based Materials. Front. Built Environ. 7:655935. doi: 10.3389/fbuil.2021.655935
Received: 19 January 2021; Accepted: 22 March 2021;
Published: 13 April 2021.
Edited by:
Prannoy Suraneni, University of Miami, United StatesReviewed by:
Tanvir Qureshi, University of the West of England, United KingdomAssed Haddad, Federal University of Rio de Janeiro, Brazil
Copyright © 2021 Sandalci, Tezer and Basaran Bundur. This is an open-access article distributed under the terms of the Creative Commons Attribution License (CC BY). The use, distribution or reproduction in other forums is permitted, provided the original author(s) and the copyright owner(s) are credited and that the original publication in this journal is cited, in accordance with accepted academic practice. No use, distribution or reproduction is permitted which does not comply with these terms.
*Correspondence: Zeynep Basaran Bundur, WmV5bmVwLmJhc2FyYW5Ab3p5ZWdpbi5lZHUudHI=