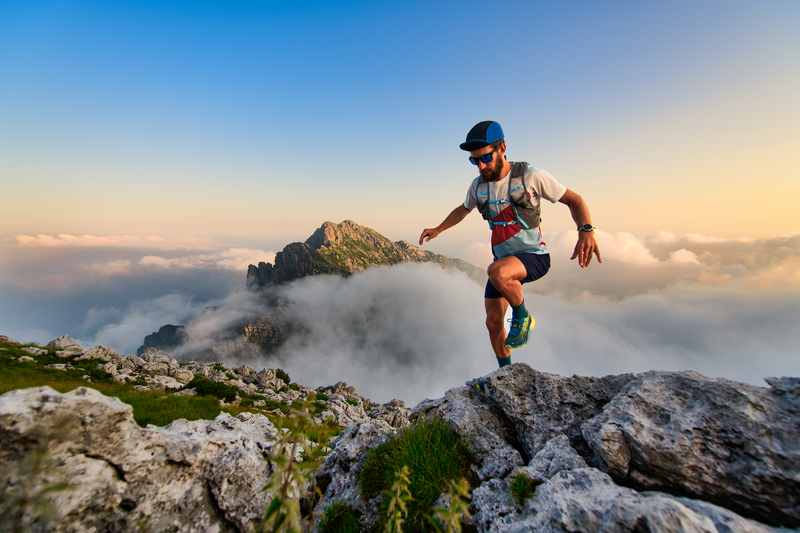
95% of researchers rate our articles as excellent or good
Learn more about the work of our research integrity team to safeguard the quality of each article we publish.
Find out more
ORIGINAL RESEARCH article
Front. Bacteriol. , 07 September 2023
Sec. Pathogenesis, Vaccines, and Immunity of Bacterial Infections
Volume 2 - 2023 | https://doi.org/10.3389/fbrio.2023.1240807
This article is part of the Research Topic Editors' Showcase: Pathogenesis, Vaccines, and Immunity of Bacterial Infections View all 8 articles
Background: Neisseria gonorrhoeae (gonococcus) is the causative agent of the sexually transmitted disease gonorrhea, for which no vaccines exist. Efforts are being made to identify potential vaccine protein antigens, and in this study, an immunoproteomics approach was used to identify protein signatures in gonococci that were recognized by sera from patients with gonorrhea.
Methods: Sera from patients with uncomplicated gonorrhea and from controls were reacted on Western blot with gonococcal whole-cell lysate separated by 2D electrophoresis. Reactive bands were excised and digested, and peptides were analyzed by mass spectrometry to identify protein hits. Proteins were analyzed with in-silico bioinformatics tools (PSORTb v3.0, CELLO, SOSUI-GramN, LipoP 1.0, SignalP 5.0, TMHMM 2.0, eggNOG-mapper 5.0) to select for surface-exposed/outer membrane proteins (OMPs) and exclude cytoplasmic proteins and most periplasmic proteins. Sera were tested for bactericidal activity against homologous and heterologous gonococcal strains.
Results: Patient sera reacted with 180 proteome bands, and 18 of these bands showed ≥2-fold increased reactivity compared with sera from individuals (n = 5) with no history of gonococcal infection. Mass spectrometry produced peptide signatures for 1,107 proteins, and after bioinformatics analyses, a final collection of 33 proteins was produced that contained 24 OMPs/extracellular proteins never previously studied to our knowledge, 6 proteins with homologs in Neisseria meningitidis that can generate functional immune responses, and 3 unknown proteins. The sera showed little or no significant bactericidal activity, which may be related to the immunoproteomic identification of contraindicated proteins Rmp and H.8 that can generate blocking antibodies.
Conclusion: Studies on the vaccine potential of these newly identified proteins deserve consideration.
Neisseria gonorrhoeae (Ng, gonococcus) is the causative agent of the sexually transmitted disease gonorrhea. There are no effective vaccines for preventing gonococcal infection, and several key indicators justify the need for research in this area. Worldwide, there are ~87 million cases of gonococcal infection reported annually by the World Health Organization (Rowley et al., 2019), with the majority in the least developed/low-to-middle income countries. However, this number is probably an underestimate due to unreported asymptomatic infection. Gonococci infect the mucosal epithelium of the genitourinary tract; in men, infection of the urethra causes urethritis and painful discharge, and in women, localized infection of the endo/ectocervix leads to a mucopurulent cervicitis. However, in approximately 10%–25% of untreated women, the bacteria can ascend into the upper reproductive tract. The host response to this ascending infection can manifest as the clinical syndrome of pelvic inflammatory disease (PID), which can leave patients with long-term and/or permanent sequelae such as chronic pelvic pain, fallopian tube damage (salpingitis), endometritis, ectopic pregnancy, and infertility. These outcomes impact significantly on the health of women worldwide. There is also a risk of co-infection with other sexually transmitted disease pathogens, e.g., human immunodeficiency virus, Treponema pallidum, and Chlamydia. Antibiotics have successfully treated patients with gonorrhea since the 1940s, but gonococci have developed resistance to every single class of antibiotic used. Today, multi-antibiotic-resistant bacteria are circulating and compromising treatment, making paramount the need for other control measures such as effective vaccines (Jerse and Deal, 2013; Wetzler et al., 2016).
The incubation period for gonococcal infection is unclear but may be between ~1 and 8 days before symptoms appear. The comprehensive review from Lovett and Duncan (2018) summarizes the natural life cycle of gonococcal infection and estimates that up to ~33% of exposed individuals do not develop infection. Gonococcal infection can be symptomatic or asymptomatic, with the former persisting for at least 14 days and can be up to 1 year and the latter persisting for as long as 165 days but often eventually progressing to symptomatic presentations. What is known is that gonococci can be spontaneously cleared in up to 25% of infected individuals, but the mechanisms are unknown. The gonococcus displays extensive antigenic and phase variation and clearly can manipulate the human immune response (Russell et al., 2019; Walker et al., 2023). The hallmarks of uncomplicated, symptomatic purulent urethritis and endo/ectocervicitis are a robust inflammatory response, characterized by the influx of neutrophils, and the production of inflammatory cytokines. In addition to this cell-mediated inflammatory response, there are many reports of serum and genital tract antibody production during uncomplicated gonorrhea and that some antibodies recognizing gonococcal surface antigens can kill bacteria via a complement-dependent mechanism or through opsonophagocytosis. However, antibody responses during uncomplicated gonorrhea, i.e., following infection of the lower urogenital tract, rectum, or pharynx, are generally considered weak and of short duration, and there is no induction of protective immunity based on memory. Thus, individuals can suffer from repeat infections, and the gonococcus employs several mechanisms to inhibit the development of B- and T-cell immunity (Walker et al., 2023), including molecular mimicry, Ig proteolytic cleavage, immune avoidance through inhibition of bactericidal antibody function, LOS sialylation, manipulation of apoptosis, and reprogramming of neutrophil and macrophage functions. However, the picture is more complex, and adaptive immune responses appear to vary according to the severity of gonococcal infection. For example, women with PID syndromes or individuals with systemic infection(s) (Humbert and Christodoulides, 2019) have increased antibody production and antibody-dependent complement-mediated serum bactericidal activity, and memory is induced (Kasper et al., 1977; Buchanan et al., 1980; Hook et al., 1984). The adaptive immune response therefore appears to be greater when gonococci penetrate deeper tissues resulting in more severe disease.
Thus, gonococci can manipulate the host and avoid the immune response during its infection life cycle, and in-depth details can be found in the comprehensive review from Walker et al. (2023). There is an increasing body of data from mouse studies showing that gonococci suppress adaptive Th1 and Th2 immune responses and induce Th17 innate immune responses that the bacterium is subsequently able to resist (Russell et al., 2019). In the complex cytokine milieu produced by gonococcal infection, Th17-associated cytokines such as IL-17 and IL-22 are produced, but not cytokines such as IFN-γ or IL-4 that are associated with Th1 or Th2 immune responses (Song et al., 2008; Feinen et al., 2010). TGF-β was found to be important for inducing the Th17 response and for suppressing adaptive immunity (Liu et al., 2011), and the addition of anti-TGF-β antibodies enabled the production of anti-gonococcal antibodies and Th1/Th2 cells secreting IFN-γ and IL-4 and the clearance of infection in the mouse and immunological memory. Similarly, inhibiting IL-10, which normally suppresses adaptive immunity, contributes to the development of protective antibody responses. Thus, there is a potential strategy to overcome the gonococcus’ ability to limit innate and adaptive immune responses by developing a vaccine containing protective surface-exposed antigens administered with anti-cytokine antibodies (Russell et al., 2019).
Gonococcal vaccines that have entered clinical trials have all largely failed (Rice et al., 2017). A killed whole-cell vaccine gave no evidence of protection in a controlled experiment in a population of Inuit with high incidence and prevalence of gonococcal infection in Northern Canada (Greenberg, 1975). A purified single-antigen pilus-based vaccine did protect human volunteers (rate ~50%) from experimental urethral infection with the homologous strain (Brinton et al., 1982) but showed no protection against a heterologous strain expressing antigenically variant pili (Tramont and Boslego, 1985) or in a large-scale vaccination field trial in high-risk US military personnel stationed in Korea (Boslego et al., 1991). In the 1970s, a trial of outer membrane protein (OMP) PorB (previous nomenclature protein I or PI) isolated from the gonococcus [but contaminated with lipooligosaccharide (LOS), Rmp (previous nomenclature protein III or PIII), and Opa (previous nomenclature protein II or PII) proteins] found that immunity against PorB, LOS, and Opa “transpired theoretically to predict protection from infection,” whereas Rmp was “subversive for PorB immunogenicity in humans and enhanced the probability of infection” (Gulati et al., 2016). Since these failed trials, fundamental research has attempted to identify putative vaccine candidates from microbiological–biochemical studies of the gonococcus and using technologies that exploit whole genome(s) information, e.g., proteomics, bioinformatics, and transcriptomics (Baarda and Sikora, 2015; Mcclure et al., 2015; Zielke et al., 2016; Almonacid-Mendoza et al., 2018; Balzano et al., 2018; El-Rami et al., 2019; Gulati et al., 2019). None of these newly identified candidates has progressed yet to the phase I human challenge model, and vaccine development is complicated by the lack of known correlates of protection for N. gonorrhoeae.
Identifying proteins that can induce antibodies with complement-dependent bactericidal and/or opsonic activity against gonococci is still one major goal for advancing progress in gonococcal vaccinology, and the ability of antigens to induce functional antibodies is potentially important for protection against infection and perhaps a surrogate for vaccine efficacy (Rice et al., 2017). In the early 1980s, a study of patients presenting at a hospital in the South of England with symptoms of acute gonorrhea or as sexual contacts of an index case was reported by Heckels and colleagues (Zak et al., 1984). This study described the properties (i.e., auxotype, serovar; PorB, Opa, and Pilin Mr) of 25 gonococcal isolates from different sites in seven groups of sexual partners. To detect antibodies to gonococcal surface proteins, serum from each patient was tested against the homologous surface-labeled gonococcal isolate, and all sera were tested against a heterologous surface-labeled strain P9, in a radioimmunoprecipitation assay followed by one-dimensional (1D) SDS-PAGE. This protocol identified antibodies in all of the serum samples to a variety of gonococcal surface proteins and structures, including pili, PorB, and Opa, unknown proteins of molecular weight (Mr) ~28–31 kDa, and a common unknown surface protein of 43 kDa (Zak et al., 1984).
For the current study, we applied an immunoproteomics and bioinformatics approach to test several of the sera from the original Heckels et al. study, against the heterologous strain P9 to try and identify additional putative cross-reactive vaccine candidates. Our focus was on identifying principally proteins that had some element of “surface exposure,” whether in the outer membrane (OM), secreted and/or proteins with extracellular domains, the rationale being that all could potentially be under immune surveillance as a consequence of presenting biological functions that are important for the gonococcal life cycle, e.g., as adhesins/invasins, pumps, porins, and autotransporters. Such proteins could potentially be targeted by Th1 and Th2 adaptive immune responses and thus be developed into vaccine candidates capable of overcoming gonococcal immunosuppression. The surface of the gonococcus, like most Gram-negative bacteria (Sun et al., 2022), is composed of an OM containing an abundance of OM proteins (OMPs) that are structurally transmembrane β-barrels with surface-exposed loops/domains, as well as lipoproteins (Hooda et al., 2017b) and LOS. In the current study, we used a range of bioinformatics tools to assess the potential localization of proteins that were recognized by immune sera, to identify lipoproteins, and to determine other properties such as the presence of signal peptides and transmembrane domains. In addition, the study also served to demonstrate serum reactivity with many other candidate proteins that have been identified by other technologies.
Neisseria gonorrhoeae strain P9-17, a 1B-26 serovar isolate (ND: P1.18-10,43: F1-26: ST-1926, Pil+ Opab+), was originally isolated from a patient with gonococcal prostatitis (Ward et al., 1970). P9-17 is our reference laboratory strain, which we have used extensively in studies of gonococcal pathogenesis and for vaccine development. The strains isolated from patients with gonorrhea are shown in Table 1. Gonococci were grown on supplemented GC agar plates (Zak et al., 1984) incubated at 37°C in an atmosphere containing 5% (v/v) CO2. Bacteria were also grown in the presence of deferoxamine mesylate salt (Desferal, Merck Gillingham, United Kingdom) to deplete iron and promote the expression of proteins involved in iron uptake. Briefly, an overnight culture of bacteria was used to inoculate 10 mL of supplemented GC broth without ferric nitrate; the bacterial mixture was adjusted to an optical density (OD) of 0.1 at λ595 nm and incubated at 37°C with shaking (200 rpm) for 2 h or until OD had doubled. Iron stress was initiated by adding Desferal to a final concentration of 50 µM followed by incubation for a further 4 h (Cornelissen, 2019). Bacteria were also grown in normal supplemented GC broth (with ferric nitrate) and without Desferal.
To prepare whole-cell lysates, bacteria grown in broth were centrifuged at 3,400×g for 5 min at 4°C, and the cell pellet was suspended in 500 µL of PBS and sonicated with an MSE Soniprep 150 (5 cycles of 20 s on and 20 s off). OMs were prepared by extraction in 0.2 M of lithium acetate, pH 5.8 buffer with stirring with glass beads for mechanical disruption, as described previously (Heckels, 1977; Williams et al., 2007). The protein content of lysates and OM preparations was estimated with a bicinchoninic acid (BCA) assay, and both preparations were stored at −20°C until required.
Archival human serum samples were available for immunoproteomics from a study of antigenic variation during infection with N. gonorrhoeae, published in 1984 by Heckels and colleagues (Zak et al., 1984). In this study, all patients presented at a hospital in the South of England, during 1980–1982, with symptoms of acute gonorrhea or they were sexual contacts of an index case. Samples taken with a cotton wool swab from the urethra of men and from the cervix and urethra of women were inoculated onto agar to culture gonococci, and a blood sample was taken at the same time and stored at −70°C. In addition to the published 25 gonococcal isolates and the sera collected from the different sites in the seven groups of sexual partners, another 26 gonococcal isolates and sera were collected from an additional 11 groups of sexual partners that had attended the same clinic. A further 10 isolates and serum samples were collected from 8 men with urethritis and from 2 women with cervicitis, who did not belong to a group. These samples were not analyzed by radioimmunoprecipitation assay. In total, 61 gonococcal isolates from the time of the study were collected and stored. From the original study, 4 serum samples were available from the published 7 groups of sexual partners (Zak et al., 1984), 6 serum samples were available from among the additional 11 groups of sexual partners, and a serum sample was available from each of the 10 individual patients. Thus, there were 20 individual serum samples available for immunoproteomics analysis along with the homologous gonococcal isolate (Table 1). Sera (n = 5) were also collected at the same time from laboratory staff with no known history of gonococcal infection, to act as non-infection controls.
Proteins were separated according to their isoelectric pH in the first dimension and then by their molecular weight in the second dimension. Whole-cell lysates prepared from N. gonorrhoeae P9-17 grown with and without Desferal were separated using an Agilent 3100 isoelectric focusing (IEF) system and Agilent 3100 OFFGEL Fractionator kit, following the manufacturer’s instructions. Preliminary experiments showed that a concentration of 1 mg/mL of lysate was optimal for electrophoresis and Western blotting reactivity with human sera (data not shown). The system has a liquid phase recovery of proteins (Hörth et al., 2006). Briefly, for IEF, proteins were focused on 24-cm-long, pH 3–10 immobilized pH gradient (IPG) strips (GE Healthcare, Hatfield, United Kingdom). Strips were placed with the gel side up into the tray, and a frame with 24 fraction wells was inserted into the tray, above the IPG strip, and pressed down gently without disrupting the gel. Each fraction has a predicted pH value increasing from fraction 1 (3.35–3.61) to fraction 24 (9.39–9.65) (Hörth et al., 2006). After securing the tray, 40 µL of rehydration solution [protein OFFGEL stock solution (1.25×) diluted with dH2O] was pipetted into each well of the frame to hydrate and swell the gel. Electrode pads were wetted with a rehydration solution, and two of them were stuck together and put on both sides of the IPG strip, assuring that the wetted electrode pads were touching the frame. The pads were replaced every 24 h during the fractionation. The rehydration solution was left for 15 min to swell the IPG gel, and 150 µL of OFFGEL protein sample solution was pipetted into each well of the frame. The tray was placed on the instrument platform, and cover fluid (GE Healthcare) was applied onto the IPG strip ends, following the manufacturer’s instructions. Electrodes were attached to the tray and the “OG24PRO1” program, suitable for 24-cm-long strips, was used.
For electrophoresis of IEF fractions, hand-cast gels were made using 12% (w/v) acrylamide, Mini-PROTEAN® Tetra Hand Cast System (Bio-Rad, Watford, United kingdom), and 15 well combs. The gels were loaded with PageRuler (Thermo Scientific™, Abingdon, United Kingdom, 5 µL/well) molecular weight ladder, followed by collected fractions 1 to 12 or 13 to 24 in duplicate from the Agilent 3100 OFFGEL fractionator system. Gel electrophoresis was done at 200 V for 50–60 min or until the 10-kDa mark of the ladder reached the bottom of the Tetra Cell buffer tank (Bio-Rad). Each experiment to test a single serum sample required four gels: two gels with fractions 1 to 24 were used for immunoblotting, and two gels with fractions 1 to 24 were stained with colloidal blue staining kit (Invitrogen™ Paisley, Scotland, United Kingdom) following the manufacturer’s instructions.
Proteins were transferred from two gels to nitrocellulose membranes as described previously (Christodoulides et al., 1998). The membranes were blocked with 5% (w/v) non-fat dried milk in Tris-buffered saline containing 0.05% (v/v) Tween-20 (TTBS) and reacted with each serum at a 1/200 dilution and overnight at 4°C, conditions determined from pilot experiments with 1D SDS-PAGE and Western blotting using different antibody concentrations, incubation times, and temperatures. After incubation with primary sera, membranes were washed three times for 5 min with TTBS and reacted with goat anti-human lgG (H+L)-AP conjugate (Bio-Rad) at a 1/3,000 dilution for 1 h at RT. Bands were visualized with 4-bromo-3-chloro-3-indoyl phosphate (BCIP) and nitro blue tetrazolium (NBT).
The levels of reactivity were scored by densitometry readings as positive (+) through to strongly positive (+++). Western blots were done in duplicate for each serum, and the scores are the average of the two measurements of densitometry.
Bands were excised from SDS-PAGE gels that were stained with MS-appropriate colloidal blue, and gel pieces were processed using an in-gel digestion protocol (Shevchenko et al., 2006). Gels were washed with Milli-Q water, and bands of interest were cut with a clean scalpel blade and transferred into 1.5 mL size microcentrifuge tubes containing 150 µL of Milli-Q water. Each gel piece was mixed with 500 µL of acetonitrile (Merck), incubated at RT for 10 min, and centrifuged briefly, and the liquid was removed. Dithiothreitol solution (50 µL) was added to completely cover the gel pieces, and tubes were incubated on a Dri-Block heater (Techne, St Neots, United Kingdom) at 56°C for 30 min. Next, acetonitrile (500 µL) was added with incubation at RT for 10 min. Samples were centrifuged at 12,000×g for 1 min, the supernatant was removed, and iodoacetamide solution (50 µL) was added, with incubation in the dark at RT for 20 min. After incubation, ammonium bicarbonate (100 mM, 300 µL) was added to each sample, with incubation and occasional mixing at RT for 30 min. Acetonitrile (500 µL) was added to each sample, with incubation and occasional mixing at RT until gel pieces became white and shrunk. All liquid was removed by gentle centrifugation.
Digestion of gel pieces was done with trypsin; briefly, dry gel pieces were mixed with 50 µL of trypsin buffer [10 mM of ammonium bicarbonate containing 10% (v/v) acetonitrile] and left at 4°C for 90 min, followed by the addition of ammonium bicarbonate (20 µL/sample) and overnight incubation at 37°C. For peptide extraction after overnight digestion, 15 µL of 25 mM ammonium bicarbonate was added to each sample followed by incubation at 37°C for 30 min. Gel pieces were centrifuged at 13,000×g for 1 min, and supernatants were collected into fresh microcentrifuge tubes. Then, dry gel pieces were mixed with 100 µL of extraction buffer (formic acid/acetonitrile, 1:2 ratio) and incubated at 37°C for 15 min with shaking. After incubation, gel pieces with extraction buffer were pooled with the corresponding supernatants from microcentrifuge tubes and centrifuged at 13,000×g for 3 min. Supernatants were collected into fresh Eppendorf tubes and lyophilized at 45°C until no liquid remained in the tubes and then stored at 4°C for mass spectrometry (MS) analysis.
Nanocapillary liquid chromatography-tandem mass spectrometry (GeLC-MS/MS) was used to catalog peptides and proteins in selected N. gonorrhoeae P9-17 bands (Schirle et al., 2003). Peptide hits were searched against the database of predicted protein sequences for the P9-17 genome (https://pubmlst.org/bigsdb?db=pubmlst_neisseria_isolates&page=query, ID 36675, accession http://www.ebi.ac.uk/ena/data/view/ERR977394). Data were analyzed with ProteinLynx Global Server, version 2.05.
Protein hits generated by MS were analyzed using publicly available resources (El-Rami and Sikora, 2019). Subcellular localization and prediction of OM, cytoplasmic membrane (CM), periplasmic (P), and cytoplasmic (C) proteins was done with PSORTb v3.0 (https://www.psort.org/psortb/) (Yu et al., 2010), CELLO (http://cello.life.nctu.edu.tw/) (Yu et al., 2004), and SOSUI-GramN (https://harrier.nagahama-i-bio.ac.jp/sosui/sosuigramn/sosuigramn_submit.html) (Imai et al., 2008) programs. The LipoP 1.0 software program (http://www.cbs.dtu.dk/services/LipoP/) (Juncker et al., 2003) was used to predict lipoprotein signal peptide cleavage by signal peptidase I/II. The SignalP 5.0 (http://www.cbs.dtu.dk/services/SignalP/) (Nielsen, 2017; Almagro Armenteros et al., 2019) was used to help distinguish between signal peptides. The TMHMM 2.0 program (http://www.cbs.dtu.dk/services/TMHMM/) (Möller et al., 2001) was used to predict transmembrane helices and regions, and the eggNOG-mapper 5.0 (http://eggnog-mapper.embl.de/) (Huerta-Cepas et al., 2019) program was used to predict orthology and provide some functional annotation of proteins. Amino acid sequences were also examined for the presence of cysteine residues and scored for 0, 1, 2, 3, 4, and 5 or more residues. Allergenic proteins and allergenic regions in a protein were predicted in silico using the AlgPred 2.0 web server, with a cutoff value of 0.5 (Sharma et al., 2021). Hypothetical/unknown protein amino acid sequences were also run through the STRING database (Szklarczyk et al., 2019) and NCBI’s Conserved Domain Database (CDD) (Marchler-Bauer et al., 2017). BLAST+ 2.13.0 [BLAST: Basic Local Alignment Search Tool (nih.gov)] (Altschul et al., 1990; Boratyn et al., 2012) and UniProt (Uniprot, 2021) were used for protein homology searching.
The human complement-dependent serum bactericidal assay (hSBA) was based on the protocol described by Mcquillen et al. (1994) with minor modifications (Christodoulides et al., 1993; Humbert and Christodoulides, 2018). Gonococci were grown overnight on GC agar plates for ≤16 h, subcultured onto fresh GC plates, and used for experiments after 6–7 h of growth, and assays were done in sterile 96-well microtiter plates with lids (Greiner Bio-One, Stonehouse, United Kingdom), with wells containing 25 µL of bacteria (~1,000 CFU) in Dulbecco’s modification of PBS (PBSB), 17 µL of normal human serum (NHS) as the exogenous complement source, and 10–25 µL of serial dilutions of pooled test serum and made to a final volume of 100 µL with PBSB containing 1% (v/v) decomplemented fetal calf serum (dFCS). NHS from a single source (laboratory staff with no history of gonococcal or meningococcal infection and with written consent provided) was used for all the hSBA experiments and prescreened for its ability to support the complement-mediated killing of gonococcal strain P9-17, using murine sera raised to OM as a reference antiserum of known bactericidal activity (Almonacid-Mendoza et al., 2018). Control wells contained no serum or serum from sham-immunized animals, and decomplemented NHS was prepared by heat inactivation in a water bath at 56°C for 30 min, to prove that bactericidal activity was due to a complement-mediated mechanism and not to other factors present in the sera. To confirm that the bactericidal assay was working, each assay included wells containing anti-P9-17 OM serum of known bactericidal activity, e.g., the 50% endpoint serum dilution, as a positive control. Plates were incubated for 1 h at 37°C in a humidified atmosphere with 5% (v/v) CO2. Aliquots of 15 µL were plated in triplicate on GC agar plates, and colonies were counted 24–48 h later (ProtoCOL, Synoptics Ltd., Cambridge, UK). Human serum complement-dependent bactericidal activity was determined from the numbers of bacteria surviving in the presence of serum, and complement was compared with the numbers surviving with complement but without test serum. The 50% hSBA titers for each serum pool were determined from a minimum of n = 3 independent experiments and are presented as the mean values with the range observed between the experiments. For certain serum-sensitive strains, hSBA experiments were done similarly with gonococci grown in the presence of cytidine-5′-monophospho-N-acetylneuraminic acid (CMP-NANA) (Elkins et al., 1992; De La Paz et al., 1995). Serum resistance of gonococci is due to sialylation of a Gal beta 1-4GlcNAc group on a conserved 4.5-kDa LOS component by CMP-NANA, and this is catalyzed by a gonococcal sialyl transferase enzyme. Thus, the addition of CMP-NANA to media for growing serum-sensitive gonococci imparts serum resistance and makes the strain(s) useful for in-vitro bactericidal assays. Briefly, 1 mL of a 1-mg/mL solution of CMP-NANA sodium salt (Sigma, Gillingham, United Kingdom) was filter-sterilized, spread onto a surface of a 20-mL GC agar plate, and allowed to diffuse into the agar to give a final concentration of 50 µg/mL (Elkins et al., 1992; De La Paz et al., 1995). Single colonies grown on plates without CMP-NANA for 16 h at 37°C in an atmosphere of 5% (v/v) CO2 were grown as a lawn on the CMP-NANA plates for a further 16 h. Sialylated bacteria were then harvested and immediately used in hSBA assays.
Immunoproteomics was done with the human sera on whole-cell lysates of the heterologous N. gonorrhoeae strain P9-17, produced from bacteria grown in culture with Desferal, in order to induce the expression of iron-repressible proteins (West and Sparling, 1985). The use of whole-cell lysates was selected over OM preparations so as not to exclude any proteins that might be extracellular/secreted. Preliminary experiments were done to compare fractions of whole-cell lysates prepared from gonococci grown with and without Desferal; these were separated by IEF and SDS-PAGE, and examination of the protein profiles showed that the addition of Desferal appeared to increase the number and intensity of the proteins (Supplementary Figures 1A, B). In addition, and prior to testing with human sera, further preliminary experiments were done to validate the choice of whole-cell lysates prepared from gonococci grown with Desferal, whereby fractions were blotted and reacted with murine antisera raised to P9-17 OM (Almonacid-Mendoza et al., 2018). Proteins on immunoblots of Desferal whole-cell lysates appeared to show stronger reactivity with anti-OM sera than proteins on immunoblots from whole-cell lysates without Desferal (Supplementary Figures 1C, D).
Next, we tested the 20 patient and 5 control sera individually on Western blots of the whole-cell lysates separated by IEF and SDS-PAGE. Western blots are shown in Supplementary Figure 2 for all patient sera and in Supplementary Figure 3 for all control sera. We also prepared a pool of the sera from the 20 patients and from the 5 controls and tested each by Western blot on a whole-cell lysate prepared from P9-17 gonococci grown with Desferal. This experiment with the pooled sera was repeated twice and then each band of immunological reactivity was marked and recorded to create a “reference reactivity pattern,” numbering the bands from left to right in the fractions and from top to bottom from the largest to smallest proteins (Figure 1). This reactivity pattern was used to identify and select the gel bands for excision for GeLC-MS/MS. The total number of identified bands was 180, and they were recorded as present or absent in individual immunoblots of the sera from 20 gonorrhea patients and from the 5 controls (Table 2, Supplementary Table 1). The inclusion criterion used for selecting bands for GeLC-MS/MS was a ratio of band reactivity at least >2-fold higher than the control. Of the 180 bands, 18 fit this criterion, and these are marked on a representative immunoblot of pooled gonorrhea patient sera (Figure 1) with recorded levels of reactivity summarized in Table 2. The number of patient sera showing positive reactivity ranged from 40% (8/20 sera reacting with bands 44, 74, 81, and 175) up to 85% (for 17/20 sera reacting with band 162) (Table 2). By contrast, the reactivity of control sera was uniformly lower, ranging from 0% (no reactivity against bands 10, 74, 166, and 175) to between 20% and 40% for the other bands.
Figure 1 Western immunoblot of the reactivity of a pool of serum from patients with gonorrhea against heterologous gonococcal strain P9-17. A pool of sera from patients (n = 20) with gonorrhea was reacted against heterologous gonococcal strain P9-17. There were 180 bands of immunoreactivity, and the numbered bands refer to those that were excised from the corresponding gel(s) for mass spectrometry. These bands show reactivity >2-fold higher than the reactivity observed with control sera. The images are representative of n = 2 immunoblots. The immunoblot images for all patient sera and control sera are shown in Supplementary Figures 2, 3.
Table 2 Reactivity of sera from gonorrhea patients and controls on Western blots of heterologous P9-17 and the recorded levels of reactivity.
The 18 identified bands were excised from the corresponding gels, and extracted peptides were analyzed by GeLC-MS/MS. A total of 1,107 proteins were identified and PSORTb was used initially to predict subcellular localization (Table 3, Supplementary Table 3). Among the 1,107 proteins, we identified 818 that were cytoplasmic (C), 67 that belonged to the cytoplasmic membrane (CM), 34 that were periplasmic (P), 57 that were assigned to the outer membrane (OM), and 2 that were extracellular (E). There were also 134 proteins that PSORTb could not assign to a compartment and classified as unknown (U) localization.
The cytoplasmic and cytoplasmic membrane proteins were removed from the list, as well as some proteins that were identified multiple times in different compartments. The rationale for removing the C, CM, and IM proteins is that these do not have surface exposure and thus are not under immune surveillance. Any antibody reactivity with such proteins most likely follows bacterial lysis rather than from functional surface expression. This reduction produced a list of 93 target proteins. The proteins were analyzed also with CELLO (Supplementary Table 4) and SOSUI-GramN (Supplementary Table 5) programs to create a “Consensus” final and more rigorous prediction of localization based on these three programs. We then used additional bioinformatics tools to refine our list of proteins, focusing on those that are potentially surface-exposed, i.e., located in the OM, extracellular/secreted, SPII lipoprotein, and proteins identified with virulence functions such as adhesins/invasins and toxins. The rationale for focusing on these proteins is their potential roles in pathogenesis and immune surveillance and the fact that they may show antigenic and phase variation, which are suggestive of immune tracking and thus potential targets for vaccine development based on the premise of inducing functional immune responses targeted at these antigens.
In addition, the amino acid sequences were analyzed by LipoP (Supplementary Table 6) to determine the presence of lipoproteins, particularly those that have SPII cleavage sites, since SPII enzymes play a critical role in exporting lipid-modified proteins (Dalbey et al., 2012). In general, SPII lipoproteins were retained as this is suggestive of surface exposure. We also analyzed the proteins with TMHMM2 (Supplementary Table 7), which is a deep learning model for transmembrane topology prediction and classification and currently the best-performing method for predicting α-helical and β-barrel transmembrane protein topology (Krogh et al., 2001). The outputs shown from TMHMM2 as described in the legend of Supplementary Table 14 and those proteins with outputs from TMHMM2 probably describe the presence of signal peptides. We used SignalP (Supplementary Table 8) to distinguish between the different types of signal peptides in the proteins, with a preference for Sec/SPII signals that identify lipoprotein SPs transported by the Sec translocon and cleaved by Signal Peptidase II (Lsp). eggNOG-mapper was used to try and provide functional annotations for those proteins that we identified without a named function (Supplementary Table 9). The program confirmed in most cases the preferred names for the proteins and provided descriptions to complete the protein name in Supplementary Table 14. Finally, we counted the cysteine residues manually in the proteins and, in general, excluded all proteins with three or five or more residues and retained proteins with one, two, or four residues (Supplementary Table 9). The rationale of doing this was practical for downstream expression of the proteins in recombinant form since those with three, five, or more residues are more difficult to produce recombinantly. Finally, the list was narrowed further by excluding all cytoplasmic membrane proteins, any newly annotated cytoplasmic proteins, false periplasmic proteins that were actually cytoplasmic, and any obvious periplasmic proteins that have intracellular functions, e.g., as enzymes.
The final list of 70 proteins with data from all the bioinformatics analyses is shown in Supplementary Table 10 and re-ordered in Supplementary Table 14. The list contains i) novel proteins that have not been studied as potential gonococcal vaccine candidates to our knowledge, ii) proteins that have been studied already as potential Neisseria vaccine candidates, and iii) proteins that can be excluded from study due to their lack of surface exposure.
We examined whether any of the patient sera had any bactericidal activity toward the homologous infecting strain and against the heterologous strain P9-17. Preliminary experiments showed that using dilutions of sera >1/4 showed no significant bactericidal activity, and as serum volumes were limiting, only 13 individual patient sera and 2 control sera could be tested at 1/4. However, we could test a pool of the sera from the 20 gonorrhea patients and of the 5 controls. Homologous strain SU121 was the only strain sensitive to human complement and grown on CMP-NANA plates (Table 1). The SBA data showed that only sera from patients 4 and 7 killed ~50% of their homologous gonococcal strain at a 1/4 dilution (Figure 2). The remaining sera did not achieve a 50% threshold of killing when tested at this dilution against either homologous or heterologous strain. Pooled patient sera killed ~30% of heterologous P9-17 gonococci, whereas individual and pooled control sera showed no activity (Figure 2). The growth of gonococci with Desferal did not improve the minimal SBA observed for any of the sera (data not shown).
Figure 2 Serum bactericidal activity of sera from patients with gonorrhea. Serum from patients with gonorrhea (n = 13) were tested for bactericidal activity against their respective homologous gonococcal isolate and against the heterologous strain P9-17. Sera from controls (n = 2) were tested against the heterologous strain, as well as a pool of all n = 20 serum samples and n = 5 control samples. The 50% hSBA titers for each serum pool were determined from a minimum of n = 3 independent experiments and are presented as the mean values with the range observed within the experiments.
We identified 70 gonococcal proteins in total within excised bands that showed increased immunoreactivity with patient sera compared with sera from individuals with no known history of gonococcal infection. There were many proteins that, to our knowledge, have not been examined as vaccine antigens, with no information in the literature on their ability to induce bactericidal and/or opsonizing antibodies and/or to clear gonococcal infection in the intravaginal mouse infection model. We also found proteins that have been identified already by previous mining studies, e.g., using proteomics and in-silico bioinformatics and RNA sequencing (Mcclure et al., 2015; Almonacid-Mendoza et al., 2018; Zhu et al., 2019; Baarda et al., 2021) and many important and well-characterized protein antigens that have been reported previously to induce bactericidal antibodies against gonococci or against meningococci by their respective meningococcal homologs. Identification of the latter demonstrates that an immunoproteomics approach can complement other experimental antigen discovery methods.
We identified the OM-located lipoprotein adhesin MafA 2/3 (Supplementary Table 14, number 1), which is returned as a single locus in PubMLST/Neisseria database (NGO1393/1584). Maf proteins are a family of OM/secreted toxins in pathogenic Neisseria species (Jamet and Nassif, 2015), and recent studies have shown that the MafA protein of Neisseria meningitidis formed oligomers on the OM. A protein of ~36 kDa, which may be a MafA protein, has been postulated to function as a glycolipid-binding adhesin, interacting with gangliotriosylceramide (GgO3) and gangliotetraosylceramide (GgO4) receptors expressed on human endocervical cells (Paruchuri et al., 1990). MafA 2/3 (NGO1393/1584) exhibits 97% identity to the product of the mafA1 gene (AAF62309.1) of N. meningitidis MC58. The recent study from Baarda et al. (2021) reported that strain FA 1090, which was used to annotate the genome of our strain P9-17, possessed four copies of MafA: two pairs of identical loci, designated MafA 1/4 (NGO1067 and NGO1972) and MafA 2/3 (NGO1393/1584). Their data suggested that the two MafA subtypes were evolutionarily distinct, and the alleles associated with each subtype were closely related (Baarda et al., 2021). A vaccine containing MafA may therefore need representatives from both subfamilies to provide strain coverage. Antisera also recognized NGO0225 (Supplementary Table 14, number 9), a polymorphic toxin MafB class 2 protein. MafB is a toxin secreted by pathogenic Neisseria spp. (Jamet and Nassif, 2015) specifically via the novel MafA protein secretion pathway (Arenas et al., 2020). In meningococci, MafB is a fratricidal toxin that can inhibit the growth of strains that do not produce the corresponding immunity protein MafI (Arenas et al., 2015).
HpuA (NGO1495, Supplementary Table 14, number 2) and HpuB (NGO2109, Supplementary Table 14, number 3) are OMPs that are TonB-dependent hemoglobin/lactoferrin/transferrin family receptor proteins (Schryvers and Stojiljkovic, 1999). Pathogenic Neisseria can use hemoglobin as an iron source, and the only likely receptor in gonococci consists of the OM lipoprotein, HpuA, and a transmembrane protein, HpuB. Growth on hemoglobin is dependent on the inner membrane TonB and requires both HpuA and HpuB (Chen et al., 1996; Chen et al., 1998). Jerse et al. showed in the mouse intravaginal infection model that there was an increased frequency of phase variants in the HpuA/B receptor with “on” (hemoglobin+) occurring in ~50% of the infected mice and the presence of hemoglobin in inflammatory exudates selected for hemoglobin+ phase variants during infection (Jerse et al., 2002). Thus, under iron-restricted conditions in the genital tract, switching on of the HpuA/B complex provides a potential vaccine target.
We identified protein NGO10270 (Supplementary Table 14, number 10), a PilC/PilC2 pilus assembly/adherence protein, ostensibly located in the periplasm. PilC is a minor pilus tip-located protein adhesin (Rudel et al., 1995b) that undergoes phase and structural variation and is associated with the bacterial cell surface (Rahman et al., 1997). PilC1 plays an important role in pilus-mediated adherence of gonococci to human epithelial cells (Rudel et al., 1992; Kirchner and Meyer, 2005) and in natural competence for transformation (Rudel et al., 1995a). PilC2 has been shown also to mediate adherence of meningococci to epithelial cells (Morand et al., 2009). Although surface exposure of PilC makes the protein an attractive vaccine candidate, concerns over phase and structural variation possibly preclude its use.
NGO1092 (Supplementary Table 14, number 4) is a large molecular weight uncharacterized OMP (181 kDa), and UniProt and BLAST analysis identified this as a PLxRFG domain-containing protein, that with high confidence (90% similarity) is a phage-associated protein. Neisseria gonorrhoeae genomes encode for several phages, though they have not been isolated. Gonococcal phage-associated proteins have been reported to be able to associate closely with bacterial OMP (Piekarowicz et al., 2020), which may account for their recognition by patient sera. Recently, antisera generated in rabbits immunized with purified filamentous NGOΦfil phages were shown to bind to the structural NgoΦ6fil proteins present in phage particles and to gonococci, but not to react with gonococcal OMP. Notably, sera to these phage proteins had bactericidal activity and blocked the adhesion of gonococci to tissue culture cells (Kłyż and Piekarowicz, 2018).
There was one extracellular protein NGO0554 (Supplementary Table 14, number 5), whose gene was highly upregulated in gonococci transiently exposed to H2O2, suggesting a role in protection against host oxidative damage (Stohl et al., 2005). NGO0648 (Supplementary Table 14, number 8) is an SPII membrane lipoprotein, which was identified in a study of RNA transcripts to enable phenotypic test of antibiotic susceptibility in gonococci exposed to ciprofloxacin (Khazaei et al., 2018). NGO1847 (Supplementary Table 14, number 13) is a DUF (domain of unknown function) 560 domain-containing protein, and such domains are found within the OM transmembrane barrel of SLAM (surface lipoprotein assembly modulator) family proteins (Hooda et al., 2017a).
Other proteins identified for possible future study include the OMP AsmA (NGO1344, Supplementary Table 14, number 14), which has been shown to contribute to cell envelope integrity (Baarda et al., 2018). There was also a large molecular weight OM/extracellular autotransporter domain-containing IgA protease (NGO0275, Supplementary Table 14, number 15), and human sera from patients with gonorrhea and sera from immunized rabbits have been shown to contain broadly cross-reactive neutralizing activity (Lomholt et al., 1995). Surface-exposed BamE (NGO1780, Supplementary Table 14, number 16) is part of the OMP assembly complex and involved in the assembly and insertion of β-barrel proteins into the OM (Sikora et al., 2018). LtgE (NGO0608, Supplementary Table 14, number 17) is a lytic transglycosylase (LT), located in the periplasm, and its function and whether it can translocate to the OM, as predicted for other LTs such as LtgA and LtgD, is unknown (Chan et al., 2012). MtrC (NGO1365, Supplementary Table 14, number 18) is a periplasmic membrane fusion lipoprotein, part of the gonococcal efflux pump that contributes to the survival of gonococci exposed to human neutrophils and their antimicrobial components (Handing et al., 2018). In our study, NGO1873 (Supplementary Table 14, number 22), the peptidoglycan-binding protein LysM, was predicted to be in the periplasm, but Leuzzi et al. (2013) speculated that NGO1873 is able to reach the OM when the Rmp protein acts as a bridge between the OM and peptidoglycan layer. There was also the protein NGO2104 (Supplementary Table 14, number 24), identified as Fic (filamentation induced by cyclic AMP) toxin, whose cellular location was unknown. We kept the protein in our candidate list as Fic proteins are widespread in bacteria, and various pathogens produce Fic proteins as bacterial toxins that are delivered into host cells via type III or type IV secretion systems (Roy and Cherfils, 2015). Thus, these Fic proteins contribute to pathogenicity and may be potential vaccine targets.
NGO1440 (Supplementary Table 14, number 41) is annotated as the peripheral membrane protein MacA (Kobayashi et al., 2001), an ABC transporter protein that is part of the MacA–MacB efflux pump that recognizes and exports macrolide antibiotics (Rouquette-Loughlin et al., 2005). Another ABC transporter protein identified was NosD (NGO7400 and NGO7395, Supplementary Table 14, numbers 42 and 65), which was predicted to be periplasmic or possibly extracellular in location. NGO0626 (Supplementary Table 14, number 48) is the membrane-bound lytic murein transglycosylase B (ltgD), which is involved in the release of cytotoxic peptidoglycan fragments by gonococci. We annotated ltgD as a periplasmic protein, whereas in fact, unlike other lytic transglycosylases, ltgD is a lipoprotein that localizes to the OM and thus should be immune-accessible (Schaub et al., 2016).
There were several proteins ostensibly found in the periplasm that have not been studied as vaccine antigens but are potential candidates based on some evidence for their surface exposure. Antisera recognized the periplasmic PPIase proteins SurA (NGO1714 and NGO0766, Supplementary Table 14, numbers 45 and 64) and PrsA (NGO1655, Supplementary Table 14, number 61), but there is evidence that these PPIases can be secreted (Unal and Steinert, 2014). NGO0138 (Supplementary Table 14, number 53) is SPII lipoprotein DegQ, a probable serine protease in gonococci that is likely anchored to the inner or OM by the lipid tail (Volokhina et al., 2011). Interestingly, vaccination with an unrelated purified recombinant DegQ protein protected fish against infection by Vibrio harveyi (Zhang et al., 2008).
Several of the gonococcal proteins in our list that have not been examined as vaccine antigens, to our knowledge, have homolog proteins in meningococci whose biological functions and/or antigenicity have been reported. The OM-located TonB-dependent receptor ZnuD (NGO1205, Supplementary Table 14, number 34) is a member of the ferric uptake regulator regulon and is involved specifically in the uptake of zinc. Murine antisera to the meningococcal homolog were shown to be bactericidal for meningococci (Stork et al., 2010), and the gonococcal homolog was identified as a potential therapeutic target (Zielke et al., 2014; Baarda et al., 2021). We identified the peptidyl-prolyl cis-trans isomerase macrophage inhibitory potentiator (MIP) protein (NGO1225, Supplementary Table 14, number 33), and murine antisera generated to a truncated form of the meningococcal homolog have been shown to kill gonococci (Humbert and Christodoulides, 2018). The serine endopeptidase autotransporter NalP (NGO5610, Supplementary Table 14, number 6) is an OM lipoprotein with extracellular exposure consistent with the properties of autotransporter proteins. The homolog NalP in meningococci has been extensively studied and shown to be phase variable, to have proteolytic activity for other autotransporter proteins, and to be involved in initiating biofilm formation and for survival in the blood (Van Ulsen et al., 2003; Echenique-Rivera et al., 2011; Arenas et al., 2013). The latter appears to be dependent on the ability of NalP to cleave human complement C3 and facilitate the degradation of C3b (Del Tordello et al., 2014). However, in gonococci, the nalP gene is a pseudogene because of the presence of several termination codons, and so gonococci do not encode full-length NalP proteins (Turner et al., 2002; Del Tordello et al., 2014). This might limit its potential as a vaccine candidate, though our data suggest that antibodies in patients’ sera recognize the N-terminal truncated portion of gonococcal NalP.
NGO1152 (Supplementary Table 14, number 54) is ArtJ, a periplasmic SPII lipoprotein that functions in transporting arginine. Notably, the meningococcal homolog (NMB1612/NEIS1533) has been shown to have surface exposure and to induce functional bactericidal antibodies in mice (Hung et al., 2015). In addition, the ArtJ proteins of Chlamydia trachomatis and C. pneumoniae, which were also considered to be in the periplasm but shown to contain amino acid regions that were exposed on bacterial surfaces, have been investigated as vaccine candidates (Soriani et al., 2010).
NGO0217 (Supplementary Table 14, number 23) is the gonococcal FbpA (iron ABC transporter substrate-binding protein), and although not tested itself for its ability to induce bactericidal antibodies, the 37-kDa ferric-binding meningococcal homolog has been reported to induce bactericidal antibodies (Gomez et al., 1998). Moreover, NGO0217 is homologous to the meningococcal NMB0634 FbpA, and immunoreactivity was detected by immunoproteomics in sera from human volunteers vaccinated with experimental N. meningitidis serogroup B vaccines based on strain H44/76 detoxified L3 lipooligosaccharide (LOS)-derived outer membrane vesicles (OMV) or the licensed Cuban vaccine, VA-MENGOC-BC (Williams et al., 2014). NGO11165 (Supplementary Table 14, number 36) is annotated as the surface-exposed ComP minor pilin, which is responsible for the recognition and binding of DNA and for DUS recognition (Cehovin et al., 2013). No vaccine studies have been reported for gonococcal ComP, but studies have been done with the meningococcal homolog, which has been shown to be recognized by sera from patients convalescing from meningococcal infection (Cehovin et al., 2011). When testing the vaccine potential of meningococcal ComP, rabbit antisera were non-bactericidal, although no details were provided of how the antisera were raised. However, ComP may potentially be precluded from vaccine development as it has been reported to be present in gonococci at very low levels (Wolfgang et al., 1999).
It is worth stressing that all these mentioned meningococcal homologs, i.e., ZnuD, MIP, NalP, ArtJ, FbpA, and ComP, are all OM, surface-exposed proteins in meningococci. It is also worth noting that all the sera came from patients presenting in a hospital with symptoms of acute gonorrhea or they were sexual contacts of an index case. There is no information on whether these patients had any prior exposure to meningococci (either symptomatically or asymptomatically), but if that had been the case, it is possible that their sera have antibodies to these meningococcal homologs that cross-react with gonococci.
Our immunoproteomics study identified three proteins that had no assigned NGO number and were annotated as hypothetical. These were proteins PCKMOKAE_00078, PCKMOKAE_01763, and PCKMOKAE_02133 (Supplementary Table 14, numbers 7, 11, and 12, respectively). It would be premature to exclude these three proteins without gaining more information about their function, location, and possible antigenicity. Of these three, PCKMOKAE_01763 contains a TPR (tetratricopeptide repeat protein) motif and could be related to an OM subunit bacteriophage N4 receptor (Kiino and Rothman-Denes, 1989).
In our study, we found many gonococcal proteins that have been identified previously using other experimental approaches and that have been examined already as potential vaccine antigens. The majority were OMPs and surface-exposed and included BamA (NGO1801, Supplementary Table 14, number 26) (Zielke et al., 2016), which is part of the OM protein assembly complex that is involved in assembly and insertion of β-barrel proteins into the OM (Noinaj et al., 2013); the multidrug efflux transporter OM subunit MtrE (NGO1363, Supplementary Table 14, number 28) (Wang et al., 2018); the OM-located LPS assembly protein LptD (NGO1715, Supplementary Table 14, number 29) (Zielke et al., 2014; Zielke et al., 2016); TonB-dependent transferrin-binding protein TbpA (NGO1495, Supplementary Table 14, number 31) and transferrin-binding protein B, TbpB (NGO1496, Supplementary Table 14, number 35) (Price et al., 2007); PilQ (NGO0094, Supplementary Table 14, number 32), which is required for type IV pilus extrusion (Haghi et al., 2012); the OMP assembly factor Tam A (NGO1956, Supplementary Table 14, number 39), which is part of the OMP85 family (Zielke et al., 2016; Sikora et al., 2018); and lipoprotein MetQ (NGO2139, Supplementary Table 14, number 40), which binds L-methionine with high affinity and is involved in gonococcal adherence to cervical epithelial cells (Zielke et al., 2016; Semchenko et al., 2017) and shortens experimental murine infection (Sikora et al., 2020).
It is worth noting that there are two proteins annotated as NGO1495 in our study, which is probably due to imprecise annotation from the genome. These two proteins are PCKMOKAE_01272 (protein 2 in Supplementary Table 14) and PCKMOKAE_01566 (protein 31 in Supplementary Table 14, the TbpA). The proteins are however different, regardless of annotation, as the Mr values are different; the former contains one cysteine residue, whereas the latter contains seven cysteine residues, and Clustal alignment shows that there is only 41% similarity between the amino acid sequences (Supplementary Figure 4).
NGO1812 (Supplementary Table 14, number 27) is the OM porin PorB, which has long been recognized as the immunodominant protein, capable of inducing bactericidal antibodies in laboratory animals when administered as a recombinant protein (Wetzler et al., 1988; Wetzler et al., 1992), DNA vaccine (Zhu et al., 2004), a component of OMV (Zhu et al., 2005), and through the use of synthetic peptides (Heckels et al., 1990; Elkins et al., 1992). Although PorB is one of the few antigens that can successfully accelerate clearance of cervico-vaginal gonococci in the mouse model (Rice et al., 2017), a PorB-containing vaccine is probably unrealistic as it would need to include many variants to provide a broad coverage of circulating strains in the population. This is because of variability in the amino acid sequences of the surface-exposed loops, in response to immune surveillance (Heckels et al., 1990).
In addition to the OMP, some proteins that were assigned to the periplasm in our current study have been shown to be surface-exposed and to generate immune responses. For example, we identified NGO1981 (Supplementary Table 14, number 46), the adhesin complex protein (ACP), which functions as a C-type lysozyme inhibitor and has been shown to induce bactericidal antibodies for gonococci (Almonacid-Mendoza et al., 2018). Although probably located on the inner leaflet of the OM, it has been reported that a proportion of the protein does become surface-exposed and released extracellularly (Ragland et al., 2018; Ragland et al., 2020). We also identified SliC (NGO1063, Supplementary Table 14, number 30), the second C-type lysozyme inhibitor of the MliC family, which is in the OM (Zielke et al., 2018). SliC plays a critical role in the colonization of genital tract mucosae during infection in the female mouse model of gonorrhea, although no data are available as to whether immunization induces bactericidal antibodies or reduces colonization. Targeting lysozyme inhibitor proteins could be a useful strategy to reduce the ability of gonococci to survive in the lysozyme-rich genital tract. Another protein assigned putatively to the periplasm is an SPII lipoprotein NGO0690 (Supplementary Table 14, number 52), which is involved possibly in threonine biosynthesis and pilin antigenicity and was recently shown to induce bactericidal antibodies (Zhu et al., 2019).
Immunoproteomics identified two proteins that were recognized by human sera but are contraindicated for inclusion in any gonococcal vaccine. NGO1577 (Supplementary Table 14, number 25) is the OmpA-like OMP Rmp, antibodies to which can block the bactericidal action of anti-lipooligosaccharide (LOS) and anti-PorB antibodies present in immune serum (Rice et al., 1994). In addition, the presence of natural antibodies to OmpA of Enterobacteriaceae has been shown to cross-react with Rmp and block the bactericidal activity of normal human serum and immune human serum against gonococci (Rice, 1989). Notably, the trial of the gonococcal OMV that was enriched with PorB, but contaminated with Rmp, suggested that Rmp antibodies may have diminished the bactericidal response induced by both PorB and LOS (Gulati et al., 2016). Indeed, clearance of gonococci in the mouse intravaginal colonization model induced by anti-LOS antibodies could be abrogated by the presence of anti-Rmp antibodies (Gulati et al., 2015).
NGO0994 (Supplementary Table 14, number 37) is defined as the H.8/Lip/azurin protein, and antibodies have been detected in convalescent sera of patients with gonorrhea and disseminated infection (Black et al., 1985), although they are not protective (Brooks and Lammel, 1989). In addition, antibodies to the meningococcal Lip protein were not bactericidal (Tinsley et al., 1992). Moreover, antimeningococcal Lip antibodies have blocking effects on the bactericidal activities of human sera and of anti-Factor H binding protein antibodies (Ray et al., 2011). Given that our gonorrhea patient sera recognized both proteins, it is possible that the low levels of bactericidal activity observed against homologous and heterologous strains in our study are due to the presence of these blocking anti-Rmp and anti-Lip antibodies.
We identified several proteins that are, possibly, unlikely vaccine candidates. They are principally periplasmic proteins, which by their general nature would not be normally exposed to the immune system, and their recognition by gonococcal antisera probably reflects immune recognition of components released from lysed gonococci. They include TcyA (NGO0372, Supplementary Table 14, number 19), which is an SPII amino acid ABC transporter substrate-binding lipoprotein; NGO2056 (Supplementary Table 14, number 21), which is a thiamine ABC transporter substrate-binding protein (El-Rami et al., 2019); NGO0571 (Supplementary Table 14, number 43), which is a periplasmic peptidoglycan DD-metalloendopeptidase family protein that is expressed under manganese-induced oxidative stress (Wu et al., 2010); NGO0327 (Supplementary Table 14, number 44), which is the penicillin-binding protein 4 (Stefanova et al., 2004); NGO0574 (Supplementary Table 14, number 66), which is an α-carbonic anhydrase domain-containing protein; and DsbC (NGO1438, Supplementary Table 14, number 47) and DsbA/DsbL (NGO1717, Supplementary Table 14, number 50), which are membrane-bound periplasmic SPII lipoproteins involved in OMP assembly.
There is no information on NGO0250 (Supplementary Table 14, number 58), identified as a periplasmic ClpB protease; in Escherichia coli, ClpB protease is part of a protein-disaggregating multichaperone system (Barnett et al., 2000). NGO0214 (Supplementary Table 14, number 59) is GlmU, a periplasmic phosphate acetyltransferase, which has been shown to catalyze the conversion of N-acetylglucosamine 1-phosphate into UDP-N-acetylglucosamine, which is a key metabolite in the syntheses of gonococcal LOS, peptidoglycan, and sialic acids (Ullrich and Van Putten, 1995). NGO0168 (Supplementary Table 14, number 60) is the MntC protein, a ZnuA-like zinc ABC transporter substrate-binding protein, which is a periplasmic-binding protein that is essential for the protection of gonococci against oxidative stress. An mntC mutant had reduced intracellular survival in a human cervical epithelial cell infection model and reduced biofilm formation, suggesting that the transporter is important for gonococcal survival in the host (Lim et al., 2008). NGO0101 (cytochrome c4, cycA, Supplementary Table 14, number 62) was identified as a cell-envelope-associated protein that was differentially expressed in response to normal human serum (Zielke et al., 2016).
NGO2092 (Supplementary Table 14, number 49) is FetB, a periplasmic iron siderophore ABC transporter substrate-binding SPII lipoprotein (Carson et al., 1999). NGO1494 (Supplementary Table 14, number 51) is PotF, another SPII lipoprotein that is required for the activity of the bacterial periplasmic transport system of polyamines such as putrescine found in genital tract secretions (Goytia et al., 2015). NGO1767 (Supplementary Table 14, number 55) is a periplasmic heme-binding catalase protein KatA that provides protection against hydrogen peroxide-induced oxidative stress (Zheng et al., 1992). NGO2029 (Supplementary Table 14, number 56) is a periplasmic component of the ubiquinol (reduced product of ubiquinone)–cytochrome c reductase complex (complex III or cytochrome b-c1 complex), which is a respiratory chain that generates an electrochemical potential coupled to ATP synthesis and an unlikely vaccine candidate. NGO1549 (Supplementary Table 14, number 57) is the FtsN protein that is part of the cell division interactome of N. gonorrhoeae (Zou et al., 2017). It is unlikely to be a vaccine candidate since FtsN has been postulated to form a bridge between the cell division machinery located in the cytoplasmic membrane and the peptidoglycan layer in the periplasm, e.g., in E. coli (Yang et al., 2004). NGO0757 (Supplementary Table 14, number 63) is annotated as a refolding chaperone of the Spy/CpxP family, which has been hypothesized to be involved in P pilus formation (Isabella and Clark, 2011).
NGO1081 (Supplementary Table 14, number 67) is the CMP-N-acetylneuraminate-beta-galactosamide-alpha-2,3-sialyltransferase, which plays a crucial role in sialylation of LOS, which imparts serum resistance to gonococci and decreases antibody binding and the bactericidal effects of neutrophils and antimicrobial peptides. Although the enzyme is periplasmic/or inner membrane linked and thus not a target vaccine candidate, it may be a target for new therapies (Lewis et al., 2015). Two pilus-associated proteins were also identified—periplasmic PilN (NGO0097, Supplementary Table 14, number 68) and a PilT protein domain-containing protein, also known as a type II toxin–antitoxin system toxin FitB (NGO0907, Supplementary Table 14, number 69). The latter was retained from the immunoproteomics analysis as it carried the toxin descriptor. PilN is a putative pilus assembly protein identified in the inner membrane by quantitative proteomics (Zielke et al., 2014) and FitB (“toxin”) or its partner FitA (“antitoxin”), or the FitAB complex, has been hypothesized to slow intracellular replication and intracellular trafficking of gonococci and to bind gonococcal DNA (Mattison et al., 2006). FitB also shares features of the cytoplasmic nucleotide-binding protein PilT. A protein identified as a predicted amido-phosphoribosyltransferase of the ComF family protein (PCKMOKAE_00797 with no ascribed NGO number and unclear location, Supplementary Table 14, number 70) was also recognized by immune sera. Though uncharacterized in Neisseria, ComF of other bacteria are pilin-like polypeptides and play a role in natural DNA translocation and are probably anchored in the cytoplasmic membrane (Busch et al., 1999). Another protein (PCKMOKAE_00288, with no ascribed NGO number, Supplementary Table 14, number 20) was identified in the periplasm as a putative zinc-binding DUF302 domain-containing protein; BLAST analysis showed ~64% homology of this protein with a putative fluoride ion transporter CrcB in Brucella spp., which UniProt ascribed to the inner cell membrane. Gonococcal sera also showed reactivity with the PilO protein (NGO0096, Supplementary Table 14, number 38), which is predicted to be part of an inner membrane transenvelope protein complex that facilitates pilus function (Tammam et al., 2011), which probably excludes this as a viable vaccine target.
A potentially important factor for further refinement of the final list could be the possibility of the proteins being allergens or containing possible allergenic regions. We ran the 70 candidate proteins through the AlgPred 2.0 program, which identified 57 of the proteins as possible “non-Allergens” and 13 proteins as possible “Allergens” (Supplementary Table 11). The identified possible “Allergens” were PilQ (NGO0094), PilO (NGO0096), MafB (NGO0225), LtgE (NGO0608), membrane lipoprotein (NGO0648), H.8 (NGO0994), phage-associated protein (NGO1092), ArtJ (NGO1152), FtsN (NGO1549), KatA (NGO1767), PorB (NGO1812), a DUF560 domain-containing protein (NGO1847), and MetQ (NGO2139). Clearly, some of these are important vaccine candidates already tested experimentally, and the balance between immunogenicity and allergenicity for candidate proteins should be examined before any decision is made for final exclusion.
There were many proteins within the list of vaccine candidates that were annotated as hypothetical/unknown (Table 1). To estimate their potential role, we ran the protein sequences of all 70 candidates through the STRING database using N. gonorrhoeae FA 1090 as the search organism and through the NCBI Conserved Domain Database (CDD). STRING did not annotate the hypothetical proteins PCKMOKAE_00078, PCKMOKAE_0079, PCKMOKAE_00288, PCKMOKAE_02133, and PCKMOKAE_01763, and the CCD could only find superfamily annotations for PCKMOKAE_00288 as COG3439 (superfamily cl21600) and PCKMOKAE_01763 as PEP-TPR lipoprotein (TIGR02917, superfamily cl37187) (Supplementary Tables 12, 13). COG3439/DUF302 is an uncharacterized domain with a widespread phylogenetic distribution that appears to be homologous to the N-terminal domains of RNAse H3 and the E. coli endoribonuclease toxin RnlA. TIGR02917, superfamily cl37187, is reported to occur strictly within a subset of Gram-negative bacterial species with the proposed PEP-CTERM/exosortase system, and all bacteria with PEP-CTERM have both OM and exopolysaccharide (EPS) production genes (Haft et al., 2006).
In this immunoproteomics study, we reacted sera from patients with gonorrhea against a well-characterized heterologous gonococcal laboratory strain to identify any novel immunoreactive proteins. The study has some limitations, notably the limited number of sera remaining from the original study, whereupon it would have been interesting to examine all the sera from all the infection groups, which included samples obtained from heterosexual couples with transmissible gonococci (Zak et al., 1984). However, these samples were spent and thus unavailable for our study. In addition, it would be interesting to compare sera collected more recently, to see if the immunoproteomic patterns of reactivity are in any way different from the samples we collected ~40 years ago. Such studies could include sera collected from individuals living in high and low-to-middle income countries, as well as, for example, from sex workers who are subject to repeated gonococcal exposure and from men who have sex with men. The data from Table 2 also show that there is evidently more reactivity with patient sera than with control sera; however, increasing the numbers of control sera examined may allow us to do a statistical analysis to determine whether there are systematic differences between the patients with acute gonorrhea and the controls. Furthermore, the peptide “hits” from our MS/MS analyses could be searched against databases of predicted protein sequences from gonococcal genomes other than P9-17, and this could increase our knowledge of antigen diversity. Another potential limitation is that the second dimension for separating the proteins was done under denaturing conditions with SDS; thus, it is possible that we lose conformational epitopes. For future studies, OM lysates could be separated under “native” non-denaturing conditions to examine whether reactive sera identify additional protein “hits.” It would also be interesting to know what impact, if any, does vaccination with meningococcal serogroup B protein vaccines have on gonococcal immunoproteomic profiles.
To summarize, we identified 70 gonococcal proteins using a strict bioinformatics analysis to be present in Western blot bands that were immunoreactive with sera from patients with gonorrhea. Although we appreciate that serum reactivity could be due to the presence of other proteins in these bands, the bioinformatics analyses have refined a candidate list of new gonococcal proteins that could be examined as potential vaccine candidates. To refine this list for practical vaccine studies of potential novel antigens, we would exclude the following: 1) the 23 periplasmic proteins that lack surface exposure, 2) the 2 contraindicated proteins that can generate blocking antibodies, and 3) the 12 proteins previously studied and shown to induce anti-gonococcal bactericidal and/or other functional responses. This would leave a collection of 33 proteins that could potentially be investigated as vaccine antigens, comprised of novel proteins never previously studied (n = 24), proteins with homologs in meningococci that can generate functional immune responses (n = 6), and proteins (n = 3) that could be important antigens once their biological function(s) and location have been deduced experimentally (Supplementary Table 14). A systematic approach could involve testing all 33 proteins, which is like the approach taken with reverse vaccinology to produce the meningococcal vaccine Bexsero, in which all the putative surface-exposed or secreted proteins that could be expressed successfully as recombinant proteins (350 out of 570) were used to immunize mice for antigenicity studies (Pizza et al., 2000). A more refined approach could investigate first the most novel and lesser characterized proteins, by name and/or function; for example, the first 13 proteins in Supplementary Table 14 include OMPs MafA2/3, HpuA and HpuB, NGO0648, NGO1092, NGO1847, autotransporter NalP, extracellular/exposed proteins such as PilC/PilC2 assembly protein, and the hypothetical NGO0554, PCKMOKAE_00078, PCKMOKAE_01763, and PCKMOKAE_02133. The list for testing would inevitably lessen, for example due to complications in producing recombinant proteins with large Mr (>100 kDa) or proteins cytotoxic to bacterial expression strains, e.g., the MafB class 32 polymorphic toxin (Dijokaite-Guraliuc, unpublished observation). Alternative approaches to consider for these antigens are protein expression in non-bacterial systems and the generation of B-cell and T-cell peptide chimera antigens. A second round of testing could be for those proteins that are functionally named and characterized, for example AsmA, BamE, LtgE, MtrC, LysM, Fic, MacA, NosD, LtgD, SurA, PrsA, and DegQ, as well as the homologs of ZnuD, MIP, ArtJ, FbpA, and ComP. We propose that these 33 proteins join the list of potential candidates for further vaccine studies.
The datasets presented in this study can be found in online repositories. The names of the repository/repositories and accession number(s) can be found in the article/Supplementary Material.
Ethical approval was not required for the studies involving humans because samples were collected over 40 years ago and used previously. The studies were conducted in accordance with the local legislation and institutional requirements. The human samples used in this study were acquired from a study of patients in the early 1980s presenting at a hospital in the South of England with symptoms of acute gonorrhea or as sexual contacts of an index case as reported by Heckels and colleagues (Zak et al., 1984). Written informed consent to participate in this study was not required from the participants or the participants’ legal guardians/next of kin in accordance with the national legislation and the institutional requirements.
AD-G did all the experimental work in the laboratory. MH and DC contributed to student training and in-silico analyses. PS was responsible for the proteomics supervision and data generation. MC supervised the project. MC, AD-G, MH, PS, DC, and JH analyzed the data. MC wrote the paper. All authors reviewed, edited, and revised the manuscript.
This work was supported by GlaxoSmithKline (unnumbered).
AD-G was a student at the University of Southampton and participated in a postgraduate studentship program at GlaxoSmithKline (GSK): the studentship was sponsored by GSK 80% and the University of Southampton 20%. MC and JH have previously received funding from GlaxoSmithKline (GSK) for research into Neisseria vaccines and received royalties for a licensed patent.
The remaining authors declare that the research was conducted in the absence of any commercial or financial relationships that could be construed as a potential conflict of interest.
The author MC declared that he is an editorial board member of Frontiers, at the time of submission. This had no impact on the peer review process and the final decision.
All claims expressed in this article are solely those of the authors and do not necessarily represent those of their affiliated organizations, or those of the publisher, the editors and the reviewers. Any product that may be evaluated in this article, or claim that may be made by its manufacturer, is not guaranteed or endorsed by the publisher.
Supplementary Tables 1–13 and the GeLC-MS/MS data (Band_001–Band_018) are deposited at https://eprints.soton.ac.uk/477752/ for review. Supplementary Figures 1-4 and Supplementary Table 14 can be found online at: https://www.frontiersin.org/articles/10.3389/fbrio.2023.1240807/full#supplementary-material.
Almagro Armenteros J. J., Tsirigos K. D., Sonderby C. K., Petersen T. N., Winther O., Brunak S., et al. (2019). Signalp 5.0 improves signal peptide predictions using deep neural networks. Nat. Biotechnol. 37 (4), 420–423. doi: 10.1038/S41587-019-0036-Z
Almonacid-Mendoza H. L., Humbert M. V., Dijokaite A., Cleary D. W., Soo Y., Hung M. C., et al. (2018). Structure of the recombinant Neisseria gonorrhoeae adhesin complex protein (Rng-Acp) and generation of murine antibodies with bactericidal activity against gonococci. Msphere 3 (5). doi: 10.1128/Msphere.00331-18
Altschul S. F., Gish W., Miller W., Myers E. W., Lipman D. J. (1990). Basic local alignment search tool. J. Mol. Biol. 215 (3), 403–410. doi: 10.1016/S0022-2836(05)80360-2
Arenas J., Catón L., Van Den Hoeven T., De Maat V., Cruz Herrero J., Tommassen J. (2020). The outer-membrane protein mafa of Neisseria meningitidis constitutes A novel protein secretion pathway specific for the fratricide protein Mafb. Virulence 11 (1), 1701–1715. doi: 10.1080/21505594.2020.1851940
Arenas J., De Maat V., Caton L., Krekorian M., Herrero J. C., Ferrara F., et al. (2015). Fratricide activity of Mafb protein of N. Meningitidis strain B16b6. BMC Microbiol. 15, 156. doi: 10.1186/S12866-015-0493-6
Arenas J., Nijland R., Rodriguez F. J., Bosma T. N., Tommassen J. (2013). Involvement of three meningococcal surface-exposed proteins, the heparin-binding protein nhba, the alpha-peptide of Iga protease and the autotransporter protease nalp, in initiation of biofilm formation. Mol. Microbiol. 87 (2), 254–268. doi: 10.1111/Mmi.12097
Baarda B. I., Martinez F. G., Sikora A. E. (2018). Proteomics, bioinformatics and structure-function antigen mining for gonorrhea vaccines. Front. Immunol. 9. doi: 10.3389/Fimmu.2018.02793
Baarda B. I., Sikora A. E. (2015). Proteomics of Neisseria gonorrhoeae: the treasure hunt for countermeasures against an old disease. Front. Microbiol. 6. doi: 10.3389/Fmicb.2015.01190
Baarda B. I., Zielke R. A., Holm A. K., Sikora A. E. (2021). Comprehensive bioinformatic assessments of the variability of Neisseria gonorrhoeae vaccine candidates. Msphere 6 (1). doi: 10.1128/Msphere.00977-20
Balzano P., Lourenco AP., Zhu T., Genco C., Massari P. (2018). Identification of new gonococcal vaccine targets. Conference Proceeding in 21st International Pathogenic Neisseria Conference, Asilomar, CA, USA, September, 2018.
Barnett M. E., Zolkiewska A., Zolkiewski M. (2000). Structure and activity of clpb from Escherichia coli. Role of the amino-and -carboxyl-terminal domains. J. Of Biol. Chem. 275 (48), 37565–37571. doi: 10.1074/Jbc.M005211200
Black J. R., Black W. J., Cannon J. G. (1985). Neisserial antigen H.8 is immunogenic in patients with disseminated gonococcal and meningococcal infections. J.Infect.Dis. 151, 650–657. doi: 10.1093/infdis/151.4.650
Boratyn G. M., Schaffer A. A., Agarwala R., Altschul S. F., Lipman D. J., Madden T. L. (2012). Domain enhanced lookup time accelerated blast. Biol. Direct 7, 12. doi: 10.1186/1745-6150-7-12
Boslego J. W., Tramont E. C., Chung R. C., Mcchesney D. G., Ciak J., Sadoff J. C., et al. (1991). Efficacy trial of A parenteral gonococcal pilus vaccine in men. Vaccine 9, 154–162. doi: 10.1016/0264-410X(91)90147-X
Brinton C., Wood S., Brwon A., Labik A., Lee S., Polen S., et al. (1982). The development of A neisserial pilus vaccine for gonorrhea and meningococcal meningitis. Sem Infect. Dis Vol. Iv: Bacterial Vaccines, 140–159.
Brooks G. F., Lammel C. J. (1989). Humoral immune response to gonococcal infections. Clin. Microbiol. Rev. 2 (Supplement), S5–S10. doi: 10.1128/CMR.2.Suppl.S5
Buchanan T. M., Esenbach D. A., Knapp J. S., Holmes K. K. (1980). Gonococcal salpingitis is less likely to recur with Neisseria gonorrhoeae of the same principal outer membrane protein antigen type. Am. J. Of Obstetrics And Gynecology 138, 978–980. doi: 10.1016/0002-9378(80)91091-1
Busch S., Rosenplänter C., Averhoff B. (1999). Identification and characterization of come and comf, two novel pilin-like competence factors involved in natural transformation of Acinetobacter Sp. Strain bd413. Appl. And Environ. Microbiol. 65 (10), 4568–4574. doi: 10.1128/Aem.65.10.4568-4574.1999
Carson S. D., Klebba P. E., Newton S. M., Sparling P. F. (1999). Ferric enterobactin binding and utilization by Neisseria gonorrhoeae. J. Of Bacteriology 181 (9), 2895–2901. doi: 10.1128/Jb.181.9.2895-2901.1999
Cehovin A., Kroll J. S., Pelicic V. (2011). Testing the vaccine potential of pilv, pilx and comp, minor subunits of Neisseria meningitidis type iv pili. Vaccine 29 (40), 6858–6865. doi: 10.1016/J.Vaccine.2011.07.060
Cehovin A., Simpson P. J., Mcdowell M. A., Brown D. R., Noschese R., Pallett M., et al. (2013). Specific dna recognition mediated by A type iv pilin. Proc. Natl. Acad. Sci. U.S.A. 110 (8), 3065–3070. doi: 10.1073/Pnas.1218832110
Chan Y. A., Hackett K. T., Dillard J. P. (2012). The lytic transglycosylases of Neisseria gonorrhoeae. Microbial Drug Resistance (Larchmont N.Y.) 18 (3), 271–279. doi: 10.1089/Mdr.2012.0001
Chen C. J., Elkins C., Sparling P. F. (1998). Phase variation of hemoglobin utilization in Neisseria gonorrhoeae. Infect. Immun. 66 (3), 987–993. doi: 10.1128/Iai.66.3.987-993.1998
Chen C. J., Sparling P. F., Lewis L. A., Dyer D. W., Elkins C. (1996). Identification and purification of A hemoglobin-binding outer membrane protein from Neisseria gonorrhoeae. Infect. Immun. 64 (12), 5008–5014. doi: 10.1128/Iai.64.12.5008-5014.1996
Christodoulides M., Brooks J. L., Rattue E., Heckels J. E. (1998). Immunisation with recombinant class 1 outer membrane protein from Neisseria meningitidis: influence of liposomes and adjuvants on antibody avidity, recognition of native protein and the induction of A bactericidal immune response against meningococci. Microbiology 144, 3027–3037. doi: 10.1099/00221287-144-11-3027
Christodoulides M., Mcguinness B. T., Heckels J. E. (1993). Immunization with synthetic peptides containing epitopes of the class 1 outer-membrane protein of Neisseria meningitidis: production of bactericidal antibodies on immunization with A cyclic peptide. J. Gen. Microbiol. 139 (8), 1729–1738. doi: 10.1099/00221287-139-8-1729
Cornelissen C. N. (2019). “Generation of metal-depleted conditions for in vitro growth of Neisseria gonorrhoeae,” in Neisseria gonorrhoeae: methods and protocols. Ed. Christodoulides M. (New York, Ny: Springer New York), 217–231.
Dalbey R. E., Wang P., Van Dijl J. M. (2012). Membrane proteases in the bacterial protein secretion and quality control pathway. Microbiol. Mol. Biol. Rev. 76 (2), 311–330. doi: 10.1128/Mmbr.05019-11
De La Paz H., Cooke S. J., Heckels J. E. (1995). Effect of sialylation of Neisseria gonorrhoeae on recognition and complement mediated killing by monoclonal antibodies directed against different outer membrane antigens. Microbiology 141 (4), 913–920. doi: 10.1099/13500872-141-4-913
Del Tordello E., Vacca I., Ram S., Rappuoli R., Serruto D. (2014). Neisseria meningitidis nalp cleaves human complement C3, facilitating degradation of C3b and survival in human serum. Proc. Natl. Acad. Sci. U.S.A. 111 (1), 427–432. doi: 10.1073/Pnas.1321556111
Echenique-Rivera H., Muzzi A., Del Tordello E., Seib K. L., Francois P., Rappuoli R., et al. (2011). Transcriptome analysis of Neisseria meningitidis in human whole blood and mutagenesis studies identify virulence factors involved in blood survival. PloS Pathog. 7 (5), E1002027. doi: 10.1371/Journal.Ppat.1002027
Elkins C., Carbonetti N. H., Varela V. A., Stirewalt D., Klapper D. G., Sparling P. F. (1992). Antibodies to N-terminal peptides of gonococcal porin are bactericidal when gonococcal lipopolysaccharide is not sialylated. Mol. Microbiol. 6, 2617–2628. doi: 10.1111/j.1365-2958.1992.tb01439.x
El-Rami F. E., Sikora A. E. (2019). “Bioinfromatics workflow for gonococcal proteomics,” in Neisseria gonorrhoeae : methods and protocols. Ed. Christodoulides M. (New York: Springer/Humana Press), 185–207.
El-Rami F. E., Zielke R. A., Wi T., Sikora A. E., Unemo M. (2019). Quantitative proteomics of the 2016 who Neisseria gonorrhoeae reference strains surveys vaccine candidates and antimicrobial resistance determinants. Mol. Cell Proteomics 18 (1), 127–150. doi: 10.1074/Mcp.Ra118.001125
Feinen B., Jerse A. E., Gaffen S. L., Russell M. W. (2010). Critical role of th17 responses in A murine model of Neisseria gonorrhoeae genital infection. Mucosal Immunol. 3, 312–321. doi: 10.1038/mi.2009.139
Gomez J. A., Criado M. T., Ferreiros C. M. (1998). Bactericidal activity of antibodies elicited against the Neisseria meningitidis 37-kda ferric binding protein (Fbpa) with different adjuvants. FEMS Immunol. Med. Microbiol. 20 (1), 79–86. doi: 10.1016/S0928-8244(97)00109-0
Goytia M., Hawel L., Dhulipala V. L., Joseph S. J., Read T. D., Shafer W. M. (2015). Characterization of A spermine/spermidine transport system reveals A novel dna sequence duplication in Neisseria gonorrhoeae. FEMS Microbiol. Lett. 362 (16). doi: 10.1093/Femsle/Fnv125
Gulati S., Mu X., Zheng B., Reed G. W., Ram S., Rice P. A. (2015). Antibody to reduction modifiable protein increases the bacterial burden and the duration of gonococcal infection in A mouse model. J. Infect. Dis. 212 (2), 311–315. doi: 10.1093/Infdis/Jiv024
Gulati S., Pennington M. W., Czerwinski A., Carter D., Zheng B., Nowak N. A., et al. (2019). Preclinical efficacy of A lipooligosaccharide peptide mimic candidate gonococcal vaccine. Mbio 10 (6), E02552–E02519. doi: 10.1128/Mbio.02552-19
Gulati S., Su X., Le W., Zheng B., Madico GE., Ram S., et al (2016). Human studies in gonococcal infection: do failed vaccine trials and clinical/transmission studies shed light? Conference proceeding in 20th International Pathogenic Neisseria Conference, Manchester, UK, September, 2016.
Haft D. H., Paulsen I. T., Ward N., Selengut J. D. (2006). Exopolysaccharide-associated protein sorting in environmental organisms: the pep-cterm/epsh system. Application of A novel phylogenetic profiling heuristic. BMC Biol. 4, 29. doi: 10.1186/1741-7007-4-29
Haghi F., Peerayeh S. N., Siadat S. D., Zeighami H. (2012). Recombinant outer membrane secretin pilq(406-770) as A vaccine candidate for serogroup B Neisseria meningitidis. Vaccine 30 (9), 1710–1714. doi: 10.1016/J.Vaccine.2011.12.076
Handing J. W., Ragland S. A., Bharathan U. V., Criss A. K. (2018). The mtrcde efflux pump contributes to survival of Neisseria gonorrhoeae from human neutrophils and their antimicrobial components. Front. In Microbiol. 9. doi: 10.3389/Fmicb.2018.02688
Heckels J. E. (1977). The surface properties of Neisseria gonorrhoeae: isolation of the major components of the outer membrane. J. Gen. Microbiol. 99, 333–341. doi: 10.1099/00221287-99-2-333
Heckels J. E., Virji M., Tinsley C. R. (1990). Vaccination against gonorrheoa: the potential protective effect of immunization with A synthetic peptide containing A conserved epitope of gonococcal outer membrane protein ib. Vaccine 8, 225–230. doi: 10.1016/0264-410X(90)90050-V
Hooda Y., Lai C. C. L., Moraes T. F. (2017a). Identification of A large family of slam-dependent surface lipoproteins in gram-negative bacteria. Front. In Cell. And Infection Microbiol. 7. doi: 10.3389/fcimb.2017.00207
Hooda Y., Shin H. E., Bateman T. J., Moraes T. F. (2017b). Neisserial surface lipoproteins: structure, function and biogenesis. Pathog. Dis. 75 (2). doi: 10.1093/Femspd/Ftx010
Hook E. W. 3rd, Olsen D. A., Buchanan T. M. (1984). Analysis of the antigen specificity of the human serum immunoglobulin G immune response to complicated gonococcal infection. Infect. Immun. 43 (2), 706–709. doi: 10.1128/Iai.43.2.706-709.1984
Hörth P., Miller C. A., Preckel T., Wenz C. (2006). Efficient fractionation and improved protein identification by peptide offgel electrophoresis*. Mol. Cell. Proteomics 5 (10), 1968–1974. doi: 10.1074/Mcp.T600037-Mcp200
Huerta-Cepas J., Szklarczyk D., Heller D., Hernandez-Plaza A., Forslund S. K., Cook H., et al. (2019). ). Eggnog 5.0: A hierarchical, functionally and phylogenetically annotated orthology resource based on 5090 organisms and 2502 viruses. Nucleic Acids Res. 47 (D1), D309–D314. doi: 10.1093/Nar/Gky1085
Humbert M. V., Christodoulides M. (2018). Immunization with recombinant truncated Neisseria meningitidis-macrophage infectivity potentiator (Rt-nm-mip) protein induces murine antibodies that are cross-reactive and bactericidal for Neisseria gonorrhoeae. Vaccine 27, 3926–3936. doi: 10.1016/J.Vaccine.2018.05.069
Humbert M. V., Christodoulides M. (2019). Atypical, yet not infrequent, infections with Neisseria species. Pathogens 9 (1). doi: 10.3390/Pathogens9010010
Hung M., Humbert M., Laver J., Phillips R., Heckels J., Christodoulides M. (2015). A putative amino acid abc transporter substrate-binding protein, nmb1612, from Neisseria meningitidis, induces murine bactericidal antibodies against meningococci expressing heterologous nmb1612 proteins. Vaccine 33 (36), 4486–4494. doi: 10.1016/J.Vaccine.2015.07.032
Imai K., Asakawa N., Tsuji T., Akazawa F., Ino A., Sonoyama M., et al. (2008). Sosui-gramn: high performance prediction for sub-cellular localization of proteins in gram-negative bacteria. Bioinformation 2 (9), 417–421. doi: 10.6026/97320630002417
Isabella V. M., Clark V. L. (2011). Deep sequencing-based analysis of the anaerobic stimulon in Neisseria gonorrhoeae. BMC Genomics 12, 51. doi: 10.1186/1471-2164-12-51
Jamet A., Nassif X. (2015). Characterization of the maf family of polymorphic toxins in pathogenic Neisseria species. Microb. Cell 2 (3), 88–90. doi: 10.15698/Mic2015.03.194
Jerse A. E., Crow E. T., Bordner A. N., Rahman I., Cornelissen C. N., Moench T. R., et al. (2002). Growth of Neisseria gonorrhoeae in the female mouse genital tract does not require the gonococcal transferrin or hemoglobin receptors and may be enhanced by commensal lactobacilli. Infect. Immun. 70 (5), 2549–2558. doi: 10.1128/Iai.70.5.2549-2558.2002
Jerse A. E., Deal C. D. (2013). Vaccine research for gonococcal infections: where are we? Sex Transm Infect. 89 (Suppl 4), Iv63–Iv68. doi: 10.1136/Sextrans-2013-051225
Juncker A. S., Willenbrock H., Von Heijne G., Brunak S., Nielsen H., Krogh A. (2003). Prediction of lipoprotein signal peptides in gram-negative bacteria. Protein Sci. 12 (8), 1652–1662. doi: 10.1110/ps.0303703
Kasper D. L., Rice P. A., Mccormick W. M. (1977). Bactericidal antibody in genital infection due to Neisseria gonorrhoeae. J. Infect. Dis. 135 (2). doi: 10.1093/Infdis/135.2.243
Khazaei T., Barlow J. T., Schoepp N. G., Ismagilov R. F. (2018). Rna markers enable phenotypic test of antibiotic susceptibility in Neisseria gonorrhoeae after 10 Minutes of ciprofloxacin exposure. Sci. Rep. 8 (1), 11606. doi: 10.1038/S41598-018-29707-W
Kiino D. R., Rothman-Denes L. B. (1989). Genetic analysis of bacteriophage N4 adsorption. J. Bacteriol 171 (9), 4595–4602. doi: 10.1128/Jb.171.9.4595-4602.1989
Kirchner M., Meyer T. F. (2005). The pilc adhesin of the Neisseria type iv pilus - binding specificities and new insights into the nature of the host cell receptor. Mol. Microbiol. 56 (4), 945–957. doi: 10.1111/j.1365-2958.2005.04600.x
Kłyż A., Piekarowicz A. (2018). Phage proteins are expressed on the surface Of Neisseria gonorrhoeae and are potential vaccine candidates. PloS One 13 (8), E0202437–E0202437. doi: 10.1371/Journal.Pone.0202437
Kobayashi N., Nishino K., Yamaguchi A. (2001). Novel macrolide-specific abc-type efflux transporter in Escherichia coli. J. Bacteriol 183 (19), 5639–5644. doi: 10.1128/Jb.183.19.5639-5644.2001
Krogh A., Larsson B., Von Heijne G., Sonnhammer E. L. (2001). Predicting transmembrane protein topology with A hidden markov model: application to complete genomes. J. Mol. Biol. 305 (3), 567–580. doi: 10.1006/Jmbi.2000.4315
Leuzzi R., Nesta B., Monaci E., Cartocci E., Serino L., Soriani M., et al. (2013). Neisseria gonorrhoeae Piii has A role on ng1873 outer membrane localization and is involved in bacterial adhesion to human cervical and urethral epithelial cells. BMC Microbiol. 13, 251. doi: 10.1186/1471-2180-13-251
Lewis L. A., Gulati S., Burrowes E., Zheng B., Ram S., Rice P. A. (2015). A-2,3-sialyltransferase expression level impacts the kinetics of lipooligosaccharide sialylation, complement resistance, and the ability of Neisseria gonorrhoeae to colonize the murine genital tract. Mbio 6 (1). doi: 10.1128/Mbio.02465-14
Lim K. H., Jones C. E., Vanden Hoven R. N., Edwards J. L., Falsetta M. L., Apicella M. A., et al. (2008). Metal binding specificity of the mntabc permease of Neisseria gonorrhoeae and its influence on bacterial growth and interaction with cervical epithelial cells. Infect. Immun. 76 (8), 3569–3576. doi: 10.1128/Iai.01725-07
Liu Y., Feinen B., Russell M. W. (2011). New concepts in immunity to Neisseria gonorrhoeae: innate responses and suppression of adaptive immunity favor the pathogen, not the host. Front. Microbiol. 2. doi: 10.3389/Fmicb.2011.00052
Lomholt H., Lind I., Kilian M. (1995). Neisseria gonorrhoeae Iga1 proteases share epitopes recognized by neutralizing antibodies. Vaccine 13 (13), 1213–1219. doi: 10.1016/0264-410x(95)00057-8
Lovett A., Duncan J. A. (2018). Human immune responses and the natural history of Neisseria gonorrhoeae infection. Front. Immunol. 9. doi: 10.3389/Fimmu.2018.03187
Marchler-Bauer A., Bo Y., Han L., He J., Lanczycki C. J., Lu S., et al. (2017). Cdd/sparcle: functional classification of proteins via subfamily domain architectures. Nucleic Acids Res. 45 (D1), D200–D203. doi: 10.1093/Nar/Gkw1129
Mattison K., Wilbur J. S., So M., Brennan R. G. (2006). Structure of fitab from Neisseria gonorrhoeae bound to dna reveals A tetramer of toxin-antitoxin heterodimers containing pin domains and ribbon-helix-helix motifs. J. Biol. Chem. 281 (49), 37942–37951. doi: 10.1074/Jbc.M605198200
Mcclure R., Nudel K., Massari P., Tjaden B., Su X., Rice P. A., et al. (2015). The gonococcal transcriptome during infection of the lower genital tract in women. PloS One 10 (8), E0133982. doi: 10.1371/Journal.Pone.0133982
Mcquillen D. P., Gulati S., Rice P. A. (1994). Complement-mediated bacterial killing assays. Methods Enzymol. 236, 137–147. doi: 10.1016/0076-6879(94)36013-8
Möller S., Croning M. D., Apweiler R. (2001). Evaluation of methods for the prediction of membrane spanning regions. Bioinformatics 17 (7), 646–653. doi: 10.1093/Bioinformatics/17.7.646
Morand P. C., Drab M., Rajalingam K., Nassif X., Meyer T. F. (2009). Neisseria meningitidis differentially controls host cell motility through pilc1 and Pilc2 components of type iv pili. PloS One 4 (8), e6834. doi: 10.1371/journal.pone.0006834
Nielsen H. (2017). Predicting secretory proteins with signalp. Methods Mol. Biol. 1611, 59–73. doi: 10.1007/978-1-4939-7015-5_6
Noinaj N., Kuszak A. J., Gumbart J. C., Lukacik P., Chang H., Easley N. C., et al. (2013). Structural insight into the biogenesis of B-barrel membrane proteins. Nature 501 (7467), 385–390. doi: 10.1038/Nature12521
Paruchuri D. K., Seifert H. S., Ajioka R. S., Karlsson K. A., So M. (1990). Identification and characterization of A Neisseria gonorrhoeae gene encoding A glycolipid-binding adhesin. Proc. Natl. Acad. Sci. U.S.A. 87, 333–337. doi: 10.1073/pnas.87.1.333
Piekarowicz A., Klyz A., Adamczyk-Poplawska M., And Stein D. C. (2020). Association of host proteins with the broad host range filamentous phage ngophi6 of Neisseria gonorrhoeae. PloS One 15 (10), E0240579. doi: 10.1371/Journal.Pone.0240579
Pizza M., Scarlato V., Masignani V., Giuliani M. M., Arico B., COmanducci M., et al. (2000). Identification of vaccine candidates against serogroup B meningococcus by whole-genome sequencing. Science 287 (5459), 1816–1820. doi: 10.1126/science.287.5459.1816
Price G. A., Masri H. P., Hollander A. M., Russell M. W., Cornelissen C. N. (2007). Gonococcal transferrin binding protein chimeras induce bactericidal and growth inhibitory antibodies in mice. Vaccine 25 (41), 7247–7260. doi: 10.1016/J.Vaccine.2007.07.038
Ragland S. A., Gray M. C., Melson E. M., Kendall M. M., Criss A. K. (2020). Effect of lipidation on the localization and activity of A lysozyme inhibitor in Neisseria gonorrhoeae. J. Bacteriol 202 (8), 10.1128/Jb.00633–19. doi: 10.1128/JB.00633-19
Ragland S. A., Humbert M. V., Christodoulides M., And Criss A. K. (2018). Neisseria gonorrhoeae employs two protein inhibitors to evade killing by human lysozyme. PloS Pathog. 14 (7), E1007080. doi: 10.1371/Journal.Ppat.1007080
Rahman M., Kallstrom H., Normark S., Jonsson A. B. (1997). Pilc of pathogenic Neisseria is associated with the bacterial cell surface. Mol. Microbiol. 25, 11–25. doi: 10.1046/j.1365-2958.1997.4601823.x
Ray T. D., Lewis L. A., Gulati S., Rice P. A., Ram S. (2011). Novel blocking human igg directed against the pentapeptide repeat motifs of Neisseria meningitidis Lip/H.8 and Laz Lipoproteins. J. Immunol. 186 (8), 4881–4894. doi: 10.4049/Jimmunol.1003623
Rice P. A. (1989). Molecular basis for serum resistance in Neisseria gonorrhoeae. Clin. Microbiol. Rev. 2 (Suppl), S112–S117. doi: 10.1128/Cmr.2.Suppl.S112
Rice P. A., Mcquillen D. P., Gulati S., Jani D. B., Wetzler L. M., Blake M. S., et al. (1994). Serum resistance of Neisseria gonorrhoeae. Does it thwart the inflammatory response and facilitate the transmission of infection? Ann. Of New York Acad. Of Sci. 730, 7–14. doi: 10.1111/j.1749-6632.1994.tb44234.x
Rice P. A., Shafer W. M., Ram S., Jerse A. E. (2017). Neisseria gonorrhoeae: drug resistance, mouse models, and vaccine development. Annu. Rev. Microbiol. 71, 665–686. doi: 10.1146/Annurev-Micro-090816-093530
Rouquette-Loughlin C. E., Balthazar J. T., Shafer W. M. (2005). Characterization of the maca–macb efflux system in Neisseria gonorrhoeae. J. Of Antimicrobial Chemotherapy 56 (5), 856–860. doi: 10.1093/Jac/Dki333
Rowley J., Vander Hoorn S., Korenromp E., Low N., Unemo M., Abu-Raddad L. J., et al. (2019). Chlamydia, gonorrhoea, trichomoniasis and syphilis: global prevalence and incidence estimate. Bull. World Health Organ 97 (8), 548–562p. doi: 10.2471/Blt.18.228486
Roy C. R., Cherfils J. (2015). Structure and function of fic proteins. Nat. Rev. Microbiol. 13 (10), 631–640. doi: 10.1038/Nrmicro3520
Rudel T., Facius D., Barten R., Scheuerpflug I., Nonnenmacher E., Meyer T. F. (1995a). Role of pill and the phase-variable pilc protein in natural competence for transformation of Neisseria gonorrhoeae. Proc. Natl. Acad. Sci. U.S.A. 92, 7986–7990. doi: 10.1073/pnas.92.17.7986
Rudel T., Scheuerpflug I., Meyer T. (1995b). Neisseria pilc protein identified as type-4 pilus tip-located adhesin. Nature 373, 357–359. doi: 10.1038/373357a0
Rudel T., Van Putten J. P. M., Gibbs C. P., Haas R., Meyer T. F. (1992). Interaction of 2 variable proteins (Pile and pilc) required for pilus-mediated adherence of Neisseria gonorrhoeae to human epithelial cells. Mol.Microbiol. 6, 3439–3450. doi: 10.1111/j.1365-2958.1992.tb02211.x
Russell M. W., Jerse A. E., Gray-Owen S. D. (2019). Progress toward A gonococcal vaccine: the way forward. Front. Immunol. 10. doi: 10.3389/Fimmu.2019.02417
Schaub R. E., Chan Y. A., Lee M., Hesek D., Mobashery S., Dillard J. P. (2016). Lytic transglycosylases ltga and ltgd perform distinct roles in remodeling, recycling and releasing peptidoglycan in Neisseria gonorrhoeae. Mol. Microbiol. 102 (5), 865–881. doi: 10.1111/Mmi.13496
Schirle M., Heurtier M. A., Kuster B. (2003). Profiling core proteomes of human cell lines by one-dimensional page and liquid chromatography-tandem mass spectrometry. Mol.Cell Proteomics. 2 (12), 1297–1305. doi: 10.1074/mcp.M300087-MCP200
Schryvers A. B., Stojiljkovic I. (1999). Iron acquisition systems in the pathogenic Neisseria. Mol. Microbiol. 32 (6), 1117–1123. doi: 10.1046/j.1365-2958.1999.01411.x
Semchenko E. A., Day C. J., And Seib K. L. (2017). Metq of Neisseria gonorrhoeae is A surface-expressed antigen that elicits bactericidal and functional blocking antibodies. Infect. Immun. 85 (2), E00898–E00816. doi: 10.1128/Iai.00898-16
Sharma N., Patiyal S., Dhall A., Pande A., Arora C., Raghava G. P. S. (2021). Algpred 2.0: an improved method for predicting allergenic proteins and mapping of ige epitopes. Brief Bioinform. 22 (4). doi: 10.1093/Bib/Bbaa294
Shevchenko A., Tomas H., Havlis J., Olsen J. V., Mann M. (2006). In-gel digestion for mass spectrometric characterization of proteins and proteomes. Nat. Protoc. 1 (6), 2856–2860. doi: 10.1038/Nprot.2006.468
Sikora A. E., Gomez C., Le Van A., Baarda B. I., Darnell S., Martinez F. G., et al. (2020). A novel gonorrhea vaccine composed of metq lipoprotein formulated with cpg shortens experimental murine infection. Vaccine 38 (51), 8175–8184. doi: 10.1016/J.Vaccine.2020.10.077
Sikora A. E., Wierzbicki I. H., Zielke R. A., Ryner R. F., Korotkov K. V., Buchanan S. K., et al. (2018). Structural and functional insights into the role of bamd and bame within the B-barrel assembly machinery in Neisseria gonorrhoeae. J. Biol. Chem. 293 (4), 1106–1119. doi: 10.1074/Jbc.Ra117.000437
Song W., Condron S., Mocca B. T., Veit S. J., Hill D., Abbas A., et al. (2008). Local and humoral immune responses against primary and repeat Neisseria gonorrhoeae genital tract infections of 17beta-estradiol-treated mice. Vaccine 26 (45), 5741–5751. doi: 10.1016/J.Vaccine.2008.08.020
Soriani M., Petit P., Grifantini R., Petracca R., Gancitano G., Frigimelica E., et al. (2010). Exploiting antigenic diversity for vaccine design: the chlamydia artj paradigm. J. Biol.Chem. 285 (39). doi: 10.1074/Jbc.M110.118513
Stefanova M. E., Tomberg J., Davies C., Nicholas R. A., Gutheil W. G. (2004). Overexpression and enzymatic characterization of Neisseria gonorrhoeae penicillin-binding protein 4. Eur. J. Biochem. 271 (1), 23–32. doi: 10.1046/J.1432-1033.2003.03886.X
Stohl E. A., Criss A. K., Seifert H. S. (2005). The transcriptome response of Neisseria gonorrhoeae to hydrogen peroxide reveals genes with previously uncharacterized roles in oxidative damage protection. Mol. Microbiol. 58 (2), 520–532. doi: 10.1111/J.1365-2958.2005.04839.X
Stork M., Bos M. P., Jongerius I., De Kok N., Schilders I., Weynants V. E., et al. (2010). An outer membrane receptor of Neisseria meningitidis involved in zinc acquisition with vaccine potential. PloS Pathog. 6, E1000969. doi: 10.1371/Journal.Ppat.1000969
Sun J., Rutherford S. T., Silhavy T. J., Huang K. C. (2022). Physical properties of the bacterial outer membrane. Nat. Rev. Microbiol. 20 (4), 236–248. doi: 10.1038/S41579-021-00638-0
Szklarczyk D., Gable A. L., Lyon D., Junge A., Wyder S., Huerta-Cepas J., et al. (2019). String V11: protein-protein association networks with increased coverage, supporting functional discovery in genome-wide experimental datasets. Nucleic Acids Res. 47 (D1), D607–D613. doi: 10.1093/Nar/Gky1131
Tammam S., Sampaleanu L. M., Koo J., Sundaram P., Ayers M., Chong P. A., et al. (2011). Characterization of the piln, pilo and pilp type iva pilus subcomplex. Mol. Microbiol. 82 (6), 1496–1514. doi: 10.1111/J.1365-2958.2011.07903.X
Tinsley C. R., Virji M., And Heckels J. E. (1992). Antibodies recognising A variety of different structural motifs on meningococcal lip antigen fail to demonstrate bactericidal activity. J.Gen.Microbiol. 138, 2321–2328. doi: 10.1099/00221287-138-11-2321
Tramont E. C., Boslego J. W. (1985). Pilus vaccines. Vaccine 3 (1), 3–10. doi: 10.1016/0264-410X(85)90003-9
Turner D. P., Wooldridge K. G., Ala’aldeen D. A. (2002). Autotransported serine protease A of Neisseria meningitidis: an immunogenic, surface-exposed outer membrane, and secreted protein. Infect.Immun. 70 (8), 4447–4461. doi: 10.1128/IAI.70.8.4447-4461.2002
Ullrich J., Van Putten J. P. (1995). Identification of the gonococcal glmu gene encoding the enzyme N-acetylglucosamine 1-phosphate uridyltransferase involved in the synthesis of udp-glcnac. J. Bacteriol 177 (23), 6902–6909. doi: 10.1128/Jb.177.23.6902-6909.1995
Unal C. M., Steinert M. (2014). Microbial peptidyl-prolyl cis/trans isomerases (Ppiases): virulence factors and potential alternative drug targets. Microbiol. Mol. Biol. Rev. 78 (3), 544–571. doi: 10.1128/MMBR.00015-14
Uniprot C. (2021). Uniprot: the universal protein knowledgebase in 2021. Nucleic Acids Res. 49 (D1), D480–D489. doi: 10.1093/Nar/Gkaa1100
Van Ulsen P., Van Alphen L., Ten Hove J., Fransen F., van der Ley P., Tommassen J. (2003). A neisserial autotransporter nalp modulating the processing of other autotransporters. Mol. Microbiol. 50 (3), 1017–1030. doi: 10.1046/J.1365-2958.2003.03773.X
Volokhina E. B., Grijpstra J., Stork M., Schilders I., Tommassen J., Bos M. P. (2011). Role of the periplasmic chaperones skp, sura, and degq in outer membrane protein biogenesis in Neisseria meningitidis. J. Bacteriol 193 (7), 1612–1621. doi: 10.1128/Jb.00532-10
Walker E., Van Niekerk S., Hanning K., Kelton W., Hicks J. (2023). Mechanisms of host manipulation by Neisseria gonorrhoeae. Front. In Microbiol. 14. doi: 10.3389/Fmicb.2023.1119834
Wang S., Xue J., Lu P., Ni C., Cheng H., Han R., et al. (2018). Gonococcal mtre and its surface-Expressed loop 2 are immunogenic and elicit bactericidal antibodies. J. Infect. 77 (3), 191–204. doi: 10.1016/J.Jinf.2018.06.001
Ward M. E., Watt P. J., Glyn A. A. (1970). Gonococci in urethral exudates possess A virulence factor lost on subculture. Nature 227, 382–384. doi: 10.1038/227382a0
West S. E. H., Sparling P. F. (1985). Response of Neisseria gonorrhoeae to iron limitation - alterations in expression of membrane-proteins without apparent siderophore production. Infect.Immun. 47, 388–394. doi: 10.1128/iai.47.2.388-394.1985
Wetzler L. M., Blake M. S., Barry K., Gotschlich E. C. (1992). Gonococcal porin vaccine evaluation - comparison of por proteosomes, liposomes, and blebs isolated from rmp deletion mutants. J. Infect. Dis. 166, 551–555. doi: 10.1093/infdis/166.3.551
Wetzler L. M., Blake M. S., Gotschlich E. C. (1988). Characterization and specificity of antibodies to protein I of Neisseria gonorrhoeae produced by injection with various protein I - adjuvant preparations. J. Exp. Med. 168, 1883–1897. doi: 10.1084/jem.168.5.1883
Wetzler L. M., Feavers I. M., Gray-Owen S. D., Jerse A. E., Rice P. A., Deal C. D. (2016). Summary and recommendations from the national institute of allergy and infectious diseases (Niaid) workshop “Gonorrhea vaccines: the way forward”. Clin. Vacc Immunol. 23 (8), 656–663. doi: 10.1128/Cvi.00230-16
Williams J. N., Skipp P. J., Humphries H. E., Christodoulides M., O’connor C. D., Heckels J. E. (2007). Proteomic analysis of outer membranes and vesicles from wild-type serogroup B Neisseria meningitidis and A lipopolysaccharide-deficient mutant. Infect. Immun. 75 (3), 1364–1372. doi: 10.1128/IAI.01424-06
Williams J. N., Weynants V., Poolman J. T., Heckels J. E., Christodoulides M. (2014). Immuno-proteomic analysis of human immune responses to experimental Neisseria meningitidis outer membrane vesicle vaccines identifies potential cross-reactive antigens. Vaccine 32, 1280–1286. doi: 10.1016/J.Vaccine.2013.12.070
Wolfgang M., Van Putten J. P. M., Hayes S. F., Koomey M. (1999). The comp locus of Neisseria gonorrhoeae encodes A type iv prepilin that is dispensable for pilus biogenesis but essential for natural transformation. Mol. Microbiol. 31 (5), 1345–1357. doi: 10.1046/j.1365-2958.1999.01269.x
Wu H. J., Seib K. L., Srikhanta Y. N., Edwards J., Kidd S. P., Maguire T. L., et al. (2010). Manganese regulation of virulence factors and oxidative stress resistance in Neisseria gonorrhoeae. J. Of Proteomics 73 (5), 899–916. doi: 10.1016/j.jprot.2009.12.001
Yang J. C., Van Den Ent F., Neuhaus D., Brevier J., Lowe J. (2004). Solution structure and domain architecture of the divisome protein Ftsn. Mol. Microbiol. 52 (3), 651–660. doi: 10.1111/J.1365-2958.2004.03991.X
Yu C. S., Lin C. J., Hwang J. K. (2004). Predicting subcellular localization of proteins for gram-negative bacteria by support vector machines based on N-peptide compositions. Protein Sci. 13 (5), 1402–1406. doi: 10.1110/ps.03479604
Yu N. Y., Wagner J. R., Laird M. R., Melli G., Rey S., Lo R., et al. (2010). Psortb 3.0: improved protein subcellular localization prediction with refined localization subcategories and predictive capabilities for all prokaryotes. Bioinformatics 26 (13), 1608–1615. doi: 10.1093/Bioinformatics/Btq249
Zak K., Diaz J. L., Jackson D., Heckels J. E. (1984). Antigenic variation during infection with Neisseria gonorrhoeae: detection of antibodies to surface proteins in sera of patients with gonorrhea. J. Infect. Dis. 149, 166–173. doi: 10.1093/infdis/149.2.166
Zhang W. W., Sun K., Cheng S., Sun L. (2008). Characterization of degqvh, A serine protease and A protective immunogen from A pathogenic Vibrio harveyi strain. Appl. Environ. Microbiol. 74 (20), 6254–6262. doi: 10.1128/Aem.00109-08
Zheng H. Y., Hassett D. J., Bean K., Cohen M. S. (1992). Regulation of catalase in Neisseria gonorrhoeae. Effects of oxidant stressand exposure to human neutrophils. J.Clin.Invest. 90, 1000–1006. doi: 10.1172/JCI115912
Zhu T., Mcclure R., Harrison O. B., Genco C., Massari P. (2019). Integrated bioinformatic analyses and immune characterization of new Neisseria gonorrhoeae vaccine antigens expressed during natural mucosal infection. Vaccines (Basel) 7 (4). doi: 10.3390/Vaccines7040153
Zhu W., Thomas C. E., And Sparling P. F. (2004). Dna immunization of mice with A plasmid encoding Neisseria gonorrhea porb protein by intramuscular injection and epidermal particle bombardment. Vaccine 22 (5-6), 660–669. doi: 10.1016/j.vaccine.2003.08.036
Zhu W., Thomas C. E., Chen C. J., Van Dam C. N., Johnston R. E., Davis N. L., et al. (2005). Comparison of immune responses to gonococcal porb delivered as outer membrane vesicles, recombinant protein, or Venezuelan equine encephalitis virus replicon particles. Infect. Immun. 73 (11), 7558–7568. doi: 10.1128/Iai.73.11.7558-7568.2005
Zielke R. A., Le Van A., Baarda B. I., Herrera M. F., Acosta C. J., Jerse A. E., et al. (2018). Slic is A surface-displayed lipoprotein that is required for the anti-lysozyme strategy during Neisseria gonorrhoeae infection. PloS Pathog. 14 (7), E1007081. doi: 10.1371/Journal.Ppat.1007081
Zielke R. A., Wierzbicki I. H., Baarda B. I., Gafken P. R., Soge O. O., Holmes K. K., et al. (2016). Proteomics-driven antigen discovery for development of vaccines against gonorrhea. Mol. Cell Proteomics 15 (7), 2338–2355. doi: 10.1074/Mcp.M116.058800
Zielke R. A., Wierzbicki I. H., Weber J. V., Gafken P. R., Sikora A. E. (2014). Quantitative proteomics of the Neisseria gonorrhoeae cell envelope and membrane vesicles for the discovery of potential therapeutic targets. Mol. Cell Proteomics 13 (5), 1299–1317. doi: 10.1074/Mcp.M113.029538
Keywords: Neisseria gonorrhoeae, gonorrhoea, immuno-proteomics, vaccine, antigen
Citation: Dijokaite-Guraliuc A, Humbert MV, Skipp P, Cleary DW, Heckels JE and Christodoulides M (2023) An immunoproteomics study of antisera from patients with gonorrhea identifies novel Neisseria gonorrhoeae proteins. Front. Bacteriol. 2:1240807. doi: 10.3389/fbrio.2023.1240807
Received: 15 June 2023; Accepted: 09 August 2023;
Published: 07 September 2023.
Edited by:
Paola Massari, Tufts University, United StatesReviewed by:
Trevor F Moraes, University of Toronto, CanadaCopyright © 2023 Dijokaite-Guraliuc, Humbert, Skipp, Cleary, Heckels and Christodoulides. This is an open-access article distributed under the terms of the Creative Commons Attribution License (CC BY). The use, distribution or reproduction in other forums is permitted, provided the original author(s) and the copyright owner(s) are credited and that the original publication in this journal is cited, in accordance with accepted academic practice. No use, distribution or reproduction is permitted which does not comply with these terms.
*Correspondence: Myron Christodoulides, bWM0QHNvdG9uLmFjLnVr
†Present address: David W. Cleary, Institute of Microbiology and Infection, College of Medical and Dental Sciences, University of Birmingham, Birmingham, United Kingdom
Disclaimer: All claims expressed in this article are solely those of the authors and do not necessarily represent those of their affiliated organizations, or those of the publisher, the editors and the reviewers. Any product that may be evaluated in this article or claim that may be made by its manufacturer is not guaranteed or endorsed by the publisher.
Research integrity at Frontiers
Learn more about the work of our research integrity team to safeguard the quality of each article we publish.